- 1Department of Hand Surgery, The Second Hospital of Jilin University, Changchun, China
- 2Department of Spinal Surgery, The Second Hospital of Jilin University, Changchun, China
- 3Department of Gastrointestinal Colorectal Surgery, China-Japan Union Hospital of Jilin University, Changchun, China
- 4Department of Orthopedics, The Second Hospital of Jilin University, Changchun, China
Bone tissue engineering (BTE) has become a hopeful potential treatment strategy for large bone defects, including bone tumors, trauma, and extensive fractures, where the self-healing property of bone cannot repair the defect. Bone tissue engineering is composed of three main elements: progenitor/stem cells, scaffold, and growth factors/biochemical cues. Among the various biomaterial scaffolds, hydrogels are broadly used in bone tissue engineering owing to their biocompatibility, controllable mechanical characteristics, osteoconductive, and osteoinductive properties. During bone tissue engineering, angiogenesis plays a central role in the failure or success of bone reconstruction via discarding wastes and providing oxygen, minerals, nutrients, and growth factors to the injured microenvironment. This review presents an overview of bone tissue engineering and its requirements, hydrogel structure and characterization, the applications of hydrogels in bone regeneration, and the promising roles of hydrogels in bone angiogenesis during bone tissue engineering.
1 Introduction
Bone is the largest tissue in the human body with several pivotal physiological functions, such as detoxification and maintaining body shape, as well as its considerable role as an endocrine organ in keeping and releasing mineral substances (DiGirolamo et al., 2012; Xue et al., 2021). Bone defects, including infection, trauma, and tumor, could impair its functions. Although the self-regenerative property of bone tissue repairs the defects, bone regeneration cannot heal when defects exceed critical size (ASTM, 2014). As a gold standard, autografts are used to repair critical-sized bone defects in orthopedics due to their excellent osseointegration, osteoconductivity, and osteoinductivity properties (Zhu et al., 2021). Despite promising outcomes, autografts also have some critical limitations, including disease transfer, inflammation, and lack of donor bone transplantation (Younger and Chapman, 1989; St John et al., 2003). Additionally, autografts have a heavy economic burden on healthcare systems. It has been estimated that the bone graft substitutes market will reach 5 × 109 $ in 2025 (Lobb et al., 2019). In Germany, it has been reported that autologous and allogenic bone graft percentages changed up to −14.3% and +74.1%, respectively, between 2008–2018, whereas the using biomaterials increased up to +134.4% (Rupp et al., 2022). Bone tissue engineering (BTE) has been developed to address bone failures with new prospects.
Several biomaterials, as scaffolds, have been designed and tested in BTE owing to their unique characteristics. Polylactic acid (PLA), polyglycolic acid (PGA), poly (lactic-co-glycolic acid) (PLGA), poly ɛ-caprolactone (PCL), polyethylene glycol (PEG), polybutylene terephthalate (PBT), polyethylene terephthalate (PET), polyvinyl alcohol (PVA), polypropylene fumarate (PPF), and polyacrylic acid (PAA) have been approved by FDA for BTE as polymeric scaffolds (Ghassemi et al., 2018). Other commercial products also have been manufactured for bone regeneragtion, including OSTEOSET®, OsSatura®, Cortoss®, Vitoss BA®,TruGraft™, ChronOS®, Healos®, and Calciresorb C35® (Ge et al., 2008; Xue et al., 2022). In addition to their potency in delivering various factors that are required for cell survival and differentiation, scaffolds should have non-immunogenicity, non-cytotoxicity, and good biodegradability and biocompatibility properties (Dixon and Gomillion, 2021). Moreover, it has been shown that the vascularization system of scaffold plays a central role in the failure or success of bone repair by providing oxygen, minerals, growth factors, and nutrients, as well as discarding waste products from the regeneration microenvironment (Diomede et al., 2020). Thus, the development of scaffolds with available blood supply and coupling the angiogenesis with osteogenesis is a challenge in BTE. Hydrogels have attracted attention in BTE because of their good biocompatibility and porous structure, similar to the extracellular matrix (ECM). The soft texture of hydrogels also can minimize their inflammatory response in contact with adjacent cells and tissues (Buwalda et al., 2017). In this review, we summarized the requirements of BTE, the properties of hydrogels and their application in BTE, and the importance of angiogenesis during BTE. Finally, we focused on the effects of hydrogel-based scaffolds in promoting bone angiogenesis.
2 Bone tissue engineering and its requirements
Several processes and compartments play essential roles in bone regeneration and healing, including homing progenitor cells, osteoblast and osteocyte formation, ECM, and osteoid mineralization (Wang et al., 2013). The ECM is defined as a 3D and non-cellular structure containing specific polysaccharides and proteins secreted by cells into the extracellular space. Bone ECM comprises organic (collagen type I, glycoproteins, proteoglycans, small integrin-binding ligands N-linked glycoproteins, and γ-carboxyglutamate-containing proteins) and inorganic (hydroxyapatite) components (Lin et al., 2020). During bone defect healing, two main processes are involved: intramembranous ossification and endochondral ossification. Intramembranous ossification is associated with a direct transition of progenitor cells to bone-forming osteoblasts and forms the flat bones of the jaw and skull, whereas endochondral ossification is associated with a cartilage intermediate before the formation of bone and forms long bones (Bahney et al., 2019; Dewey and Harley, 2021). The BTE aims to prepare biomaterials to mimic ECM roles in mineralized matrix deposition and support cellular attachment after introducing them to the defect site. To this end, the compartments of BTE are reviewed in detail in the following sections.
2.1 Scaffolding materials
Scaffolds are temporary mechanical structures consisting of metals, ceramics, and polymers, in most cases, to provide an environment for ECM mimicking and bone remodeling with negligible complications (Kantaros et al., 2016). To design an ideal scaffold in BTE, four main types of requirements could be considered: biomaterial composition (ceramics, polymers, composites, etc.), structural features (bioinspired, customized shapes, biomimetic, surface topography, mechanical properties, pore interconnection, and high porosity), biological requirements (smart, bioactive, non-toxic, bioresorbable, biodegradable, non-immunogenic, and biocompatible), and fabrication process (conventional techniques: solvent casting, gas foaming, and freeze drying; and advanced techniques: rapid prototyping and electrospinning) (Roseti et al., 2017). The advantages and disadvantages of the most used scaffolds are listed in Table 1.
Among the structural features, the scaffold’s pore size and porosity are determining factors in the success of BTE due to their roles in the delivery of nutrients and vascularization. Although the high porosity of scaffolds guarantees the ingrowth of bone cells, their excessive porosity may negatively affect mechanical properties (Kuttor et al., 2014). There is evidence that pores with a size of almost 100 μm favor nutrient transport and cell migration (Zhang B. et al., 2018), whereas pores with ≥200 μm support vascularization and bone formation (Walpole et al., 2009). Studies also concluded that small pores (50–100 μm) were optimal for prompting endochondral ossification and large pores with 100–300 μm improved intramembranous ossification and enhanced vascularization (Wu et al., 2010; Daugela et al., 2018). In addition to pore size, grain size (structural dimension or the dimension of particles or grains within the scaffold and the size between the pores) of scaffolds also affects cellular adhesion, differentiation, and proliferation. For instance, Zhang et al. (2014) found that surface microstructural features of tricalcium phosphate (TCP) ceramics, TCP-S and TCP-B, have an indispensable role in their osteoinduction capacity. They reported that TCP-S (grain size < 1 μm) could stimulate osteogenic differentiation of human bone marrow stromal cells (BMSCs), characterized by overexpression of osteocalcin and osteopontin and increasing the activity of alkaline phosphatase (ALP) in vitro, compared with TCP-B with 3–4 μm grain size. After 12 weeks, the implantation of TCP-S into dog dorsal muscles led to the induction of bone formation, whereas TCP-B implants could not form any bone tissue. Furthermore, the optimal diameter for pore interconnectivity reported ranges from 700 to 1,200 μm, which supports the infiltration depth and bone deposition (Ghayor and Weber, 2018; Collins et al., 2021). Topography also could affect the osteogenic potential of progenitor cells within scaffolds. For instance, Zhou et al. (2016) constructed five nanorod-shaped 3D topographies with different interrod spacing and similar nanorod diameters and investigated their osteogenic effects on MSCs. They reported that interrod spacings <96 nm displayed dramatically enhanced osteogenic differentiation of MSCs, but constructs with interrod spacings >137 nm inhibited osteogenesis. Xu et al. (2017) found that fabricating islandlike structures on the nanofiber scaffold with chitosan provides appropriate interface for preosteoblast cell adhesion and proliferation and enhances their bone-forming capability. The stiffness is another property of scaffolds in determining the outcome of BTE. There is evidence that the stiffness of scaffolds affects cellular behavior in bone tissue, such as their proliferation, differentiation, migration, and contractility (Ping et al., 2021). In a study, Zhang J. et al. (2020) constructed 3D bone-like tissue constructs and evaluated the effects of scaffold stiffness on their osteogenesis potential. They indicated that soft scaffolds (0.66 kPa) not only induced osteogenic differentiation of stem cells and increased ALP activity, but also remarkably improved ECM mineralization and cellular organization compared with stiff scaffolds (5.4 kPa). On the other hand, Chatterjee et al. (2010) reported that the higher hydrogel stiffness could enhance osteogenic differentiation and later mineralization. Due to the conflicting reports, the effect of scaffold stiffness on osteogenic differentiation requires more investigation.
2.2 Cells
Although cell-free scaffolds have been applied in BTE, providing exogenous cells with osteogenesis potential is crucial for the damaged tissue lacking osteoprogenitor cells (Maisani et al., 2017). To this end, various cells have been used in BTE, including embryonic stem cells (ESCs), MSCs, dental stem cells, adipose-derived stem cells (ADSCs), and induced pluripotent stem cells (iPSCs).
ESCs, derived from the blastocyst’s inner cell mass (ICM), are pluripotent stem cells with the capability to differentiate into all cell types that teratoma formation in vivo and ethical restrictions are their concerns, whereas MSCs are considered “safe cells” for tissue regeneration (Lalu et al., 2012; Goradel et al., 2018). The osteogenic properties of MSCs could be related to their potential to generate a pro-osteogenic microenvironment through the secretion of paracrine factors. For instance, Ogata et al. (2017) revealed that cytokines in the secretomes of MSCs play a central role in osteoclastogenesis, as well as the recruitment and proliferation of angiogenic and osteogenic cells. Another study indicated that MSCs not only could improve bone regeneration and angiogenesis by producing various growth factors in a critical size calvarial defect, but also could suppress immune response initiation with paracrine effects (Gao X. et al., 2014). Moreover, scaffolds and their products also are involved in the osteogenic differentiation of MSCs. Naruphontjirakul et al. (2019) found that spherical monodispersed strontium containing bioactive nanoparticles (Sr-BGNPs) induced differentiation of MSCs into bone-forming cells and improved ECM mineralization. Mechanistically, Sr ions were the main actor in the osteogenic differentiation of MSCs without any cytotoxicity effects on their viability. ADSCs attracted increasing attention in bone regeneration due to their easy isolation and harvest and their proliferative and differentiative capacity into osteogenic and angiogenic linages (Paduano et al., 2017). For example, Calabrese et al. (2016) showed that combining human ADSCs (hADSCs) with collagen/hydroxyapatite scaffold promoted the differentiation of hADSCs into osteoblasts, characterized by ECM mineralization and maturation and upregulation of osteocalcin, osteopontin, and osterix. In another study, Jin et al. (2019) compared the osteogenic and angiogenic potential of ADSCs and dental pulp stem cells (DPSCs). They concluded that ADSCs showed higher osteogenic potential and upregulated osteoblast-related genes with superior mineral deposition, whereas DPSCs promoted angiogenesis with higher proliferative and migratory potential. Moreover, their implantation into a mandibular defect indicated that ADSCs could promote greater and faster bone regeneration in rats after 6 weeks.
2.3 Biochemical factors
To initiate the repair process, biochemical factors, in addition to scaffold and cells, are required to provide signals for attracting inflammatory and progenitor cells to the injured site. Three types of cues are required to induce osteogenesis: physical (mechanical action and morphology), chemical (composition differences and crosslinking), and biological. Among these cues, the physical factors are mentioned throughout the review; thus, we focus on chemical and biological factors here.
It has been shown that the mineral contents could affect the bone healing process. For instance, Zheng et al. (2014) found that β-TCP addition to the collagen solution showed notably better biological and mechanical properties in scaffolds. Mechanistically, the released Ca2+ from β-TCP activated calcium-sensitive receptors, leading to better newly formed bone in terms of quality and quantity without adverse effects. In another study, Wu et al. (2012) constructed cobalt (Co)-containing mesoporous bioactive glass (Co-MBG) scaffolds for mimicking hypoxia in BTE, in which Co acted as a chemical inducer of hypoxia-inducible factor 1α (HIF-1α). They reported that Co ions remarkably upregulated HIF-1α, vascular endothelial growth factor (VEGF), and bone-related genes in MSCs, and supported the proliferation and attachment of MSCs to the scaffold. In addition to the mineral contents, crosslinking is another chemical factor affecting BTE. There is evidence that collagen crosslinking plays a critical role in stimulating angiogenesis and stabilizing its conformation (Lindert et al., 2016). Kikuchi et al. (2004) indicated that adding glutaraldehyde, as a crosslinker, to hydroxyapatite/collagen (HA/Col) could improve the mechanical characteristics of the scaffold. Although glutaraldehyde can lead to adverse immune responses in the host system, its use in 8% is considered non-toxic and safe (Krishnakumar et al., 2019).
Various growth factors have been incorporated into the scaffolds to accelerate bone regeneration, including VEGF, bone morphogenetic proteins (BMPs), transforming growth factor β (TGFβ), insulin-like growth factor (IGF), and fibroblast growth factors (FGFs) (Azevedo and Pashkuleva, 2015). These growth factors are classified into inflammatory, osteogenic, and angiogenic. Since inflammation is the first step in healing bone fractures and inflammatory cells are recruited to the injured site, inflammatory factors are vital in BTE, such as macrophage colony-stimulating factor (M-CSF), interleukin-1 (IL-1), IL-6, and FGF2 (De Witte et al., 2018). Multiple pro-osteogenic growth factors have been used to recruit progenitor cells and promote their differentiation into bone-forming cells, including BMPs, IGF, FGF, TGFβ, and platelet-derived growth factor (PDGF) (Lienemann et al., 2012). The FDA has approved BMP-2 and BMP-7 incorporation in bone regeneration systems (Farokhi et al., 2016). Furthermore, IGF-1 acts as a mitogenic factor and is released when osteoclasts resorb fractured bone matrix, promoting the growth and differentiation of embryonic cells to osteoblasts (Kim et al., 2012; Nyberg et al., 2016). Angiogenic factors are discussed in detail in the following sections.
3 Hydrogel scaffolds in bone tissue engineering
Hydrogels are 3D network structures with high water content (more than 90%) that are formed with crosslinking among hydrophilic polymers. Hydrogels promote cell survival, proliferation, and differentiation relying on providing a microenvironment similar to ECM in terms of mechanics and architecture. They are widely classified into two classes: natural hydrogels, which are derived from natural polymers, including collagen, hyaluronic acid (HA), alginate, chitosan, gelatin, and fibrin; and synthetic hydrogels, which are derived from chemically modified natural biopolymers or synthetic polymers (Zhu and Marchant, 2011). Although naturally derived hydrogels are attractive for BTE owing to their good biocompatibility, they suffer from the potential immunogenic response, uncontrollable biodegradation rate, low mechanical strength, low stiffness, and variability in production. The poor mechanical properties of hydrogels limit their application as load-bearing structures (Li J. et al., 2022; Charlet et al., 2022). On the other hand, synthetic hydrogels, such as polycaprolactone (PLC), polylactic acid (PLA), polyoxyethylene (PEO), poly (vinyl alcohol) (PVA), and poly (ethylene glycol) (PEG), possess more extended durability, tough mechanical strength, and flexible structure, while lacking biological activity (Xue et al., 2021). In addition to classification based on their origin, hydrogels are also classified based on their durability (durable and degradable), response to environmental stimuli (conventional and smart), charge (neutral, cationic, anionic, and ampholytic), structure (semi-crystalline and amorphous), and composition (homopolymer, copolymer, and semi-interpenetrating network) (El-Sherbiny and Yacoub, 2013). Various parameters have been determined to characterize hydrogels, including morphology, chemical composition, mechanical properties, biocompatibility and biodegradability, and swelling, which are evaluated by different techniques (Figure 1).
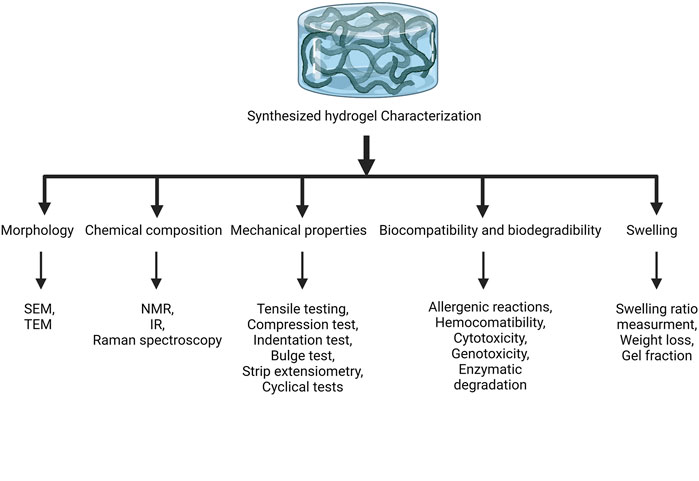
FIGURE 1. Characterization of hydrogels. The synthesized hydrogels are characterized by evaluating their morphology, chemical composition, mechanical properties, biocompatibility and biodegradability, and swelling. SEM, scanning electron microscope; TEM, transmission electron microscope; NMR, nuclear magnetic resonance; IR, infrared.
Hydrogels in BTE require some primary properties: compatibility with tissue and cells, osteoconductive activity, and osteoinductive activity. The ability of hydrogels to form new bone on their surface refers to osteoconduction, while the osteoinduction characteristic is related to the ability of hydrogels to absorb endogenous growth factors, induce the migration of stem cells into the scaffold, and promote bone formation (Yue et al., 2020). The development of hydrogels in BTE tries to cover the primary properties. For instance, Kazemi-Aghdam et al. (2021) introduced Icariin (IC)-loaded modified halloysite nanotubes (mHNTs) into chitosan hydrogel to develop a nanocomposite hydrogel for BTE. In the IC@mHNTs construct, IC acted as a bone inducer agent that promotes osteogenic differentiation. The in vitro studies demonstrated that the scaffold is a biocompatible structure that enhances the proliferation and differentiation of encapsulated MSCs. In another study, Saravanan et al. (2018) investigated the properties and bone regeneration capacity of thermosensitive chitosan (CS) and glycerophosphate (GP) based hydrogel containing graphene oxide (GO) (CS/GP/GO). They indicated that the CS/GP/GO hydrogel with pores >150 μm and ∼50% porosity was biocompatible to MSCs, promoting osteogenic differentiation of MSCs, characterized by overexpression of osteocalcin, ALP, Runt-related transcription factor 2 (Runx2), and Type -1 collagen. Also, the potential of carbon dots/hydroxyapatite/poly (vinyl alcohol) (CDs/HA/PVA) double-network (DN) hydrogel for BTE was reported by Liu et al. (2020) through the ability of the hydrogel to support MSCs proliferation and differentiation as well as repair bone defects in vivo. Evidence shows that the mechanical properties, degradation behavior, swelling behavior, and water content of CDs/HA/PVA DN hydrogel are determined by the number of freezing/thawing cycles (Wang Y. et al., 2019). Recently, Dibazar et al. fabricated a hydrogel nanocomposite containing bacterial polyglucuronic acid (PGU) and sodium alginate (Alg) composite with carbon nanofibers (CNFs) to study its bone regeneration properties. The hydrogel with interconnected pores architecture and biocompatible characteristics was hemocompatible with insignificant hemolysis induction. Furthermore, the PGU/Alg/CNFs hydrogel supported the growth of bone cells, suggesting it as a new scaffold for BTE. Table 2 summarizes the application of other hydrogels in BTE and their therapeutic effects on bone regeneration.
4 Importance of angiogenesis in bone development and repair
It has been reported that the vasculature system not only transports nutrients and oxygen and recruits cells within the bone, but also plays crucial roles in regulating osteogenesis and bone repair (Grosso et al., 2017; Di Maggio and Banfi, 2022). Therefore, fabricating scaffolds supplying functional blood is considered a challenge for BTE to couple osteogenesis and angiogenesis. Several factors and mediators regulate osteogenesis and type H vessel formation, including VEGF, Notch, HIF-1α, SLIT3, and PDGF-BB, which are produced by endothelial cells (ECs), osteoblasts, osteoclasts, and chondrocytes (Figure 2). The roles of factors are discussed below in detail.
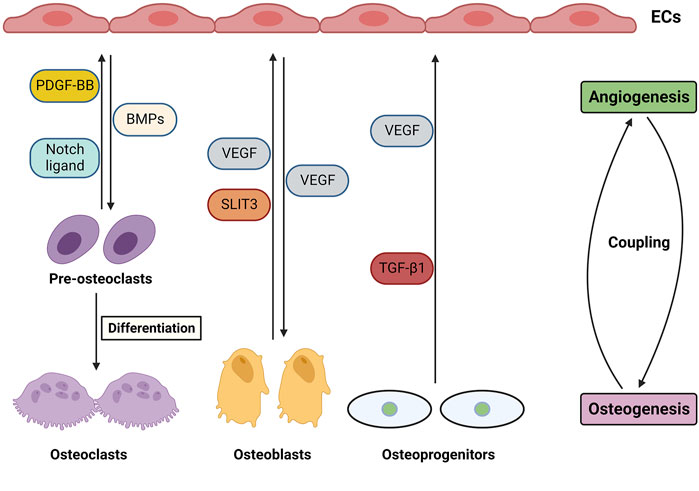
FIGURE 2. Angiogenesis and osteogenesis coupling in bones through cellular and molecular mediators and their crosstalk.
4.1 VEGF
There is evidence that the VEGF family is the master regulator of angiogenesis. The VEGF family is composed of five members, including VEGF-A, -B, -C, -D, and placental growth factor (PlGF), and three cognate receptors, including VEGF-R1, -R2, and -R3 (Goradel et al., 2017; Hashemi Goradel et al., 2018). Although angiogenesis is a complex process, VEGF-A and VEGF-R2 are the key ligand and receptor involved in angiogenesis (Goradel et al., 2019). Various cell types in the bone microenvironment, such as recruited inflammatory cells and osteoprogenitors, express VEGFs that interact with their receptors on osteoclasts, pericytes, and again inflammatory cells and osteoprogenitors (Hu and Olsen, 2017).
Deleting VEGF and its receptors in osteoblasts and osteoblast precursors have been reported to impair their differentiation, leading to low bone density and bone development (Liu et al., 2012; Duan et al., 2015; Duan et al., 2016). Berendsen and Olsen (2014) indicated that VEGF directly controls the fate of mesenchymal stromal progenitor, in which VEGF blocks adipogenesis and stimulates osteoblastic differentiation. Interestingly, they found that the intracellular VEGF determines the fate of stem cells, not the exogenous one. Furthermore, the interaction between the VEGF family members with the ECM could regulate angiogenesis. Different affinity degrees of three major isoforms of VEGF-A transcript resulting from alternative splicing to ECM and the balance between their binding and diffusivity in physiological conditions plays an important role in controlling the osteogenic microenvironment (Gianni-Barrera et al., 2020; Di Maggio and Banfi, 2022). It is worth noting that type H vessels, which are characterized by higher expression of Endomucin (Emcn) and CD31, could induce osteogenesis by stimulating the proliferation and differentiation of osteoprogenitor cells (Peng et al., 2020).
4.2 Notch
The Notch receptors (Notch1–5) initiate signaling pathways after binding to their ligands, including Delta-like (Dll) 1, 3, or 4 and the Jagged family of Serrate homologs (Jag1, and Jag2), leading to cleavage and translocation of Notch intracellular domain (NICD) into the nucleus which coordinates cell proliferation, apoptosis, and differentiation (Pakvasa et al., 2021). It has been shown that the Notch signaling pathway plays dual roles in bone tissue development depending on cell type, the timing of signaling activation, and the stage of cell differentiation. The activation of Notch signaling promotes the differentiation of MSCs into osteoblasts and bone mineralization and suppresses osteogenesis via inhibiting the Wnt/β-catenin pathway (Luo et al., 2019). Recently, Kraus et al. (2022) showed that Notch signaling activation could accelerate cartilage callus conversion into bone and increase bone repair, whereas the inhibition of the signaling pathway delays the transformation of the cartilage callus into bone. In another study, Goel et al. (2019) reported that Notch signaling suppression in osteoclasts improves bone formation rate and healing in mice, characterized by larger bony calluses and increased bone mineral density. Additionally, blood flow in type H vessels regulates Notch signaling; a low rate suppresses the pathway, leading to defective osteogenesis and angiogenesis (Ramasamy et al., 2016). On the other hand, there is evidence that activation of Notch signaling could induce the formation of type H vessels (Tang et al., 2021). Liao et al. (2017) investigated the angiogenesis-osteogenesis coupling in MSCs in response to dual stimulation with BMP-9 and Notch signaling. They indicated that NICD1 promoted osteogenic differentiation of MSCs in a BMP-9-dependent manner. Furthermore, the implantation of transduced MSCs with BMP-9 and NICD1 in combination with a biocompatible scaffold induced bone formation with upregulation of angiogenic regulators and extensive vascularization.
4.3 HIF-1α
Under normoxic conditions, the hypoxia-inducible factor-1 α (HIF-1α) transcription factor undergoes the ubiquitin-proteasome degradation pathway following hydroxylation of the lysine and proline residues on the oxygen-dependent degradation domain and interacts with the Von Hippel–Lindau (VHL) E3 ubiquitin ligase (Pereira et al., 2006). To adapt cells with hypoxia conditions, the stable HIF-1α forms a heterodimer with HIF-1β, leading to the translocation of HIF-1α to the nucleus and activating the transcription of various target genes (Jin et al., 2020). There is evidence that HIF-1α is involved in both osteogenesis and angiogenesis and their coupling. Due to the hypoxic condition of the bone microenvironment in defects, Zhang J. et al. (2018) used preconditioned MSCs with hypoxia to repair bone. The hypoxic MSCs overexpressed HIF-1α, which enhanced the osteogenesis and angiogenesis potential of MSCs and improved their survival rate under severe conditions in vitro. The transplantation of hypoxic MSCs into critical-sized mandible defects in aged rats also improved bone defects. Also, the highly expressed HIF-1α MSCs, in combination with calcium-magnesium phosphate cement (CMPC) scaffold, repaired critical-sized calvarial defects in rats by promoting the upregulation of osteogenic markers (Zou et al., 2011). Due to the stabilizing effect of cobalt ions on HIF-1α, Quinlan et al. (2015) incorporated resorbable bioactive glass particles with cobalt ions into the glass network to fabricate bioactive glass/collagen–glycosaminoglycan scaffolds. They reported that the constructed scaffolds supported osteoblast proliferation and osteogenesis and promoted tubule formation. Recently, Amir et al. (2022) indicated that HIF-1α plays an indispensable role in BMP-9-mediated preosteoblasts differentiation into osteoblasts and vascularization, whereas HIF-1α knockdown or inhibition significantly suppressed the expression of osteogenic markers and matrix mineralization in a BMP-9-dependent manner. In another study, Guo et al. (2020) revealed that the protective activity of salidroside on bone loss is related to its stimulatory effects on angiogenesis-osteogenesis coupling via regulating the HIF-1α/VEGF axis.
4.4 SLIT3
Although slit guidance ligand 3 (SLIT3) is considered an axon-guidance molecule, there is evidence that it also plays physiological functions outside the nervous system, including carcinogenesis, regulation of stem cells, immunoregulation, and skeletal development (Li N. et al., 2020). Kim B.-J. et al. (2018) revealed that SLIT3 could stimulate the proliferation and migration of osteoblasts through the activation of β-catenin. It also inhibited the differentiation of osteoclast in an autocrine manner, resulting in suppression of bone resorption, whereas Slit3 or its receptor, Robo1, deficient mice showed osteopenic phenotypes because of promoting bone resorption and inhibition of bone formation. Furthermore, higher levels of circulating SLIT3 were related to increased bone mass in postmenopausal women. In another study, Xu et al. (2018) tried to clarify how to type H vessels positively coordinate bone formation. They demonstrated that osteoblast-derived SLIT3 acts as a proangiogenic factor, promoting bone formation and increasing type H vessel numbers. On the other hand, Slit3 deletion in osteoblast cells not only reduced skeletal type H vessels, but also decreased osteoblasts’ activity and reduced bone formation. Moreover, SLIT3-mutant mice exhibited defective fracture repair, whereas exogenous SLIT3 administration improved bone healing. These results suggest the essential roles of SLIT3 in coupling osteogenesis and angiogenesis.
4.5 PDGF-BB
Platelet-derived growth factor (PDGF) family consists of five members, including PDGF-AA, PDGF-AB, PDGF-BB, PDGF-CC, and PDGF-DD, in which PDGF-BB is a potent angiogenic, chemoattractant, and mitogenic molecule that is considered as a regulatory factor in tissue regeneration (Fredriksson et al., 2004; Wang C. et al., 2019). There is evidence that PDGF-BB can promote MSCs-based bone regeneration (Caplan and Correa, 2011; Zhang N. et al., 2021). In this regard, Zhang M. et al. (2018) investigated how PDGF-BB promotes MSCs-based bone regeneration by genetically modifying PDGF-BB-overexpressing MSCs. They exhibited that PDGF-BB not only suppressed adipogenic differentiation and enhanced osteogenic differentiation of MSCs, but also increased the migration of ECs and angiogenesis through regulation of the PI3K/AKT and ERK1/2 pathways. Also, PDGF-BB-overexpressing MSCs improved osteogenesis and angiogenesis during bone regeneration in a critical-sized rat calvarial defect model. Xie et al. (2014) found that the secreted PDGF-BB from preosteoclasts increased type H vessel numbers and bone formation during bone modeling remodeling. In contrast to the mentioned studies, Luvizuto et al. (2016) reported that the supplementation of biomaterials with PDGF-BB had no remarkable effects on bone formation. Despite the beneficial effects, PDGF-BB has a short half-life within the blood; thus, local and sustained delivery of PDGF-BB is essential to achieve ideal outcomes.
5 Hydrogel scaffolds promote bone angiogenesis
Before considering the importance of hydrogel scaffolds in providing structures for enhancing bone angiogenesis, it is worth noting that hydrogel effectively creates contiguous hyaline articular cartilage. Owing to the avascular structure of cartilage, its self-healing ability is limited during cartilage damage. On the other hand, biomaterials for the replacement of hyaline cartilage that lubricates joint movement exhibited undesirable side effects (Beddoes et al., 2016). To overcome these side effects, hydrogels have been introduced as promising material due to their excellent lubrication ability, producing contiguous hyaline articular cartilage matrix and seamless integration with native cartilage under the regular mechanical forces, constructing matchable structures with the different hyaline cartilage present in the body (Beddoes et al., 2016; Meppelink et al., 2016). Regarding the importance of angiogenesis during bone development and regeneration, fabricating hydrogel scaffolds with angiogenic properties or supporting systems for angiogenesis besides their osteogenesis capacity is an advantage for BTE (Figure 3). We divided harnessing hydrogel capcities in the activation of angiogenesis and bone regeneration into four strategies as below.
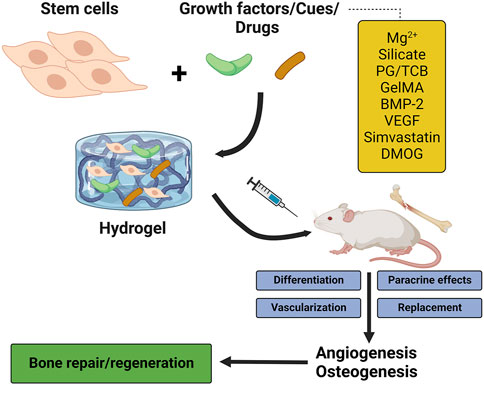
FIGURE 3. The stimulatory effects of hydrogels on osteogenesis and angiogenesis. PG/TCB, poly ethylene glycol maleate citrate (PEGMC) with β-TCP; GelMA, gelatin methacrylate; BMP-2, bone morphogenetic protein 2; VEGF, vascular endothelial growth factor; DMOG, dimethyloxallylglycine.
5.1 Ion-incorporated hydrogels
Due to the stimulatory effects of Mg2+ on cell differentiation and neovascularization in the bone microenvironment (Yoshizawa et al., 2014; Yu et al., 2017), Zhang X. et al. (2021) constructed an Mg2+-incorporating dual-crosslinked hydrogel using photopolymerization strategy and Mg-S coordination. The constructed hydrogel supported the adhesion, proliferation, spreading, and osteogenic differentiation of MSCs. The Mg2+-enriched hydrogel also enhanced the formation of tube-like structures and the number of branches in ECs, indicating its stimulatory effects on angiogenesis. The implantation of the Mg2+-enriched hydrogel into rats with a calvarial defect model improved bone formation and angiogenesis, characterized by upregulation of CD31 and higher density of blood vessels. Another ion that is incorporated into hydrogel scaffolds is the silicate ion due to its neovascularization capacity. Dashnyam et al. (2019) fabricated a silicate-shelled hydrogel fiber scaffold and examined its effect on bone regeneration. The released silicate and calcium ions from the scaffold upregulated angiogenic markers, including HIF1-α, bFGF, endothelial nitric oxide synthase (eNOS), KDR, and VEGF, and enhanced tubular networking in ECs. The implanted scaffold also improved bone formation and angiogenesis in rats. The released ions from the hydrogels could recruit progenitor cells to the bone microenvironment and stimulate the endogenous expression of VEGF, collectively resulting in bone repair (Yang et al., 2022). In another study, Sun et al. (2021) indicated that cobalt-incorporated hydrogels promote osteogenesis and angiogenesis. The tunable nanocomposite hydrogel with controlled release of cobalt ions induced osteogenesis and neovascularization and repaired the calvarial defect in a rat model because the released cobalt ion can mimic hypoxia to stimulate angiogenesis.
5.2 Vesicle-encapsulated hydrogels
In addition to the ions, MSC-derived vesicles also contribute to coupling osteogenesis and angiogenesis. For instance, exosomes affect cell-cell communication by carrying various materials and secret chemical signals (Zhang B. et al., 2021). It has been reported that pro-angiogenesis and pro-osteogenesis properties of MSCs-derived exosomes are associated with their effects on the activation of the HIF-1α/VEGF and the BMP-2/Smad1/Runx2 signaling pathways (Zhang L. et al., 2020). Recently, Wu D. et al. (2022) found that hydrogel-encapsulated small extracellular vesicles (sEVs) derived from MSCs with excellent thermosensitive properties could augment bone regeneration and repair calvarial defects in vivo. Mechanistically, MSCs-derived exosomes carry miR-21 that targets Sprouty2 (Spry2), leading to angiogenesis enhancement. Since Spry2 is an antagonist of fibroblast growth factor (FGF), there is evidence that Spry2 could control the integrity and quiescence of ECs and downregulate the angiogenesis process (Wietecha et al., 2011; Peier et al., 2013). Also, the pro-angiogenesis activity of exosomal miR-21 is associated with its modulatory effects on the NOTCH1/DLL4 pathway (Zhang Y. et al., 2021). In another study, Cheng et al. (2022) revealed that Nidogen1-enriched EVs (EV-NID1) enhanced the migration and angiogenesis potential of rat arterial endothelial cells (RAECs) by targeting myosin-10 which led to a reduction in the adhesion strength of RAECs. Moreover, loading EV-NID1 into a composite hydrogel accelerated angiogenesis and bone regeneration in an in vivo model of the femoral defect. NID1 is an ECM protein and an essential component of the vascular basement membrane that supports vascular and endothelium integrity (Marchand et al., 2019). Therefore, combining the delivery capacity of hydrogel with EVs with a high capacity to carry biological factors is a promising strategy to repair bone defects.
5.3 Hydrogels with antibacterial and immunomodulatory capabilities
Besides the osteogenesis and angiogenesis capacity, scaffolds need to be antibacterial with immunomodulatory capabilities. To this end, Cheng et al. (2020) constructed an injectable hydrogel with osteogenesis, angiogenesis, and antibacterial and called it ‘three-in-one’ platform, in which 4-arm-polyethylene glycol-thiol (4-arm-PEG-SH) hydrogel filled with liposomes-calcium phosphate nanoparticles (Lip#CaP). Firstly, they showed that the PEG-40Lip@DFO#CaP system is biocompatible owing to desirable effects on the viability of ECs. Furthermore, the treatment of ECs and preosteoblast cells with PEG-40Lip@DFO#CaP revealed the angiogenesis and osteogenesis potential of the hydrogel. The PEG-40Lip@DFO#CaP system also indicated antibacterial activities against Escherichia coli, Staphylococcus epidermis, and Staphylococcus aureus owing to carrying Ag+ and PEG in its structure. Lastly, they demonstrated that the implantation of the PEG-40Lip@DFO#CaP system into a rat calvarial critical-size defect model remarkably promoted osteogenesis and angiogenesis after 8 weeks. In another study, Ji et al. (2020) developed MSC-loaded thermosensitive hydroxypropyl chitin hydrogel (HPCH) incorporated with a 3D poly (ε-caprolactone) (PCL)/nano-hydroxyapatite (nHA) scaffold for BTE. The PCL/nHA + HPCH scaffold promoted the osteogenic differentiation of MSCs, characterized by the upregulation of osteopontin, osteocalcin, and Runx. Additionally, the PCL/nHA + HPCH scaffold enhanced angiogenesis by activating macrophages to secret VEGF and PDGF-BB. Furthermore, the MSCs-HPCH platform exerted immunomodulatory effects on macrophages by suppressing the M1 phenotype and their transition toward M2. Indeed, M1 macrophages secret VEGF and are involved in the initiation of angiogenesis; whereas M2 are responsible for the later stages of angiogenesis, stabilizing the vasculature, and typically secreting PDGF-BB. Consistent with in vitro results, the MSC-encapsulated PCL/nHA + HPCH hybrid scaffold enhanced bone formation and angiogenesis in vivo. Recently, Wu Z. et al. (2022) indicated that lithium (Li) -modified bioglass-hydrogel stimulates osteogenesis and neovascularization in a high glucose microenvironment by polarizing the differentiation of macrophage in vitro. Macrophage polarization from M1 toward M2 provided an anti-inflammatory microenvironment and relieved inflammation, resulting in bone regeneration in a diabetic rat bone defect. Similarly, Li D. et al. (2022) showed that incorporating TGF-β1 into Li-based hydrogel promotes both osteogenesis and angiogenesis, in which TGF-β1 guides macrophage polarization toward the M2 phenotype and Li ions enhance osteogenesis. Figure 4 represents how a hydrogel-based scaffold enhances angiogenesis by modulating macrophage phenotype.
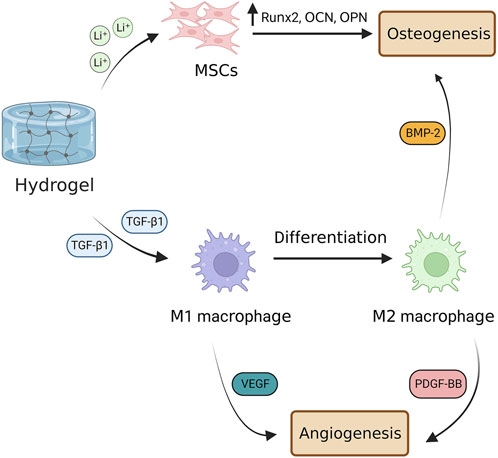
FIGURE 4. Hydrogel-based scaffolds enhance angiogenesis by modulating macrophage phenotype. Hydrogel-based scaffolds carry various ions and biomolecules, such as Li+ ions that promote differentiation of MSCs into bone-forming cells and TGF-β1 that stimulates M1 macrophage polarization toward M2 phenotype. M1 macrophages are involved in the early stage of bone angiogenesis by secreting VEGF, whereas M2 ones promote angiogenesis in the late stage by secreting PDGF-BB. M2 macrophage also is involved in osteogenesis through the secretion of BMP-2. TGF-β1, transforming growth factor beta 1; VEGF, vascular endothelial growth factor; PDGF-BB, platelet-derived growth factor-BB; BMP-2, bone morphogenetic protein 2.
5.4 Other strategies
Liu et al. (2019) developed a bioinspired 3D gelatin-methacrylate (Gel-MA) hydrogel incorporated with ECs and MSCs to study the capacity of the scaffold in bone vascularization. They reported that the co-culture of ECs and MSCs in the hydrogel system could promote angiogenesis both in vitro and in vivo. In this system, the differentiation of MSCs toward pericytes was the underlying mechanism of vascularization. In another study, Anada et al. (2019) prepared a bone-mimetic 3D hydrogel structure using GelMA, octacalcium phosphate (OCP), and ECs, and studied its osteogenesis-angiogenesis potential. The amount of OCP in the GelMA was a determinant factor in the proliferation and differentiation of MSCs; a higher amount of OCP decreased cell proliferation, while a higher amount of OCP increased othe steogenic differentiation of MSCs. Furthermore, GelMA concentration affects athe ngiogenesis and sprouting capacity of ECs; a higher GelMA concentration inhibits sprout formation. Chen et al. (2020) designed a gelatin-polyhedral oligomeric silsesquioxane (POSS) hybrid hydrogel (Gel-POSS) by an esterification reaction for BTE. They indicated that the introduction of POSS into gelatin provides a proper microenvironment for incubating ECs and MSCs on the hydrogel and supports angiogenic tube formation and extension. The addition of BMP-2 and VEGF to the hydrogel scaffold and its implantation into critical-sized rat calvarial defects revealed that the hydrogel supported the sustained release of the growth factors in vivo, leading to a higher blood vessel formation in defect regions. It has been shown that incorporating POSS moieties into biomaterials could enhance their matrix stiffness and make them porous structures (Wu et al., 2018; Tamburaci et al., 2019). Although simvastatin has a stimulatory effect on bone formation and simvastatin/poloxamer 407 hydrogel exhibited weak immunogenicity and low toxicity (Pillai and Panchagnula, 2003; Zhao et al., 2014; Tan et al., 2016), poor mechanical properties of thermosensitive simvastatin/hydrogel limit their application in BTE. On the other hand, although titanium alloys have low stiffness like cortical bone and are hopeful scaffolds for orthopedic uses, they are poorly compatible with bone growth owing to their bio-inert nature. Liu et al. (2016) designed 3D-printed porous titanium scaffolds (Ti6Al4V) containing simvastatin/hydrogel to use the advantages of both structures. They found that the constructed scaffolds not only significantly increased bone formation and bone mineral density, but also enhanced angiogenesis around and in the scaffolds. Dimethyloxallylglycine (DMOG) is another drug incorporated into the hydrogel scaffold in BTE. DMOG is an angiogenic drug that could improve both osteogenesis and vessel formation during bone repair. Mechanistically, the stimulatory effects of DMOG on osteogenesis and angiogenesis are due to HIF-1α activation (Yegappan et al., 2019). Therefore, incorporating pro-osteogenesis and pro-angiogenesis drugs into hydrogel scaffolds is a hopeful strategy to accelerate bone regeneration.
6 Conclusion
To date, various techniques have been developed to prepare hydrogels with desirable properties for BTE, including non-toxicity, good biocompatibility, controllability, and enhanced performance. Although more and more studies reported the advantages and benefits of hydrogels in BTE, the lack of clinical studies signifying their value limited their translation into the market. Some questions must be considered when designing polymers to construct hydrogels for BTE. First, will the hydrogel be used in vitro or in vivo? 2) Does the hydrogel constructed as 3D architecture or a space-filling one? 3) What is the fate of hydrogel in the long term? 4) Should cells be inert or be adhered to the hydrogel polymer? Currently, angiogenesis is a key bottleneck in BTE that is necessary to be overcome and considered. A thorough understanding of the bone repair phases to develop a reliable hydrogel-based scaffold is a required avenue for future studies.
Author contributions
Study conception and design: FG. Drafting of the manuscript: JL, LY, and KL. Critical revision: FG and KL. Approve final version: All authors.
Funding
This study was supported by Science and Technology Department of Jilin Province, focused on Development and Application of AI and Big Data-enabled System for Accurate Diagnosis and Prediction of Diabetic Foot (No. 20210204137YY).
Conflict of interest
The authors declare that the research was conducted in the absence of any commercial or financial relationships that could be construed as a potential conflict of interest.
Publisher’s note
All claims expressed in this article are solely those of the authors and do not necessarily represent those of their affiliated organizations, or those of the publisher, the editors and the reviewers. Any product that may be evaluated in this article, or claim that may be made by its manufacturer, is not guaranteed or endorsed by the publisher.
References
Amir, M. S., Chiba, N., Seong, C. H., Kusuyama, J., Eiraku, N., Ohnishi, T., et al. (2022). HIF-1α plays an essential role in BMP9-mediated osteoblast differentiation through the induction of a glycolytic enzyme, PDK1. J. Cell. Physiol. 237, 2183–2197. doi:10.1002/jcp.30752
Amiryaghoubi, N., Pesyan, N. N., Fathi, M., and Omidi, Y. (2020). Injectable thermosensitive hybrid hydrogel containing graphene oxide and chitosan as dental pulp stem cells scaffold for bone tissue engineering. Int. J. Biol. Macromol. 162, 1338–1357. doi:10.1016/j.ijbiomac.2020.06.138
Anada, T., Pan, C.-C., Stahl, A. M., Mori, S., Fukuda, J., Suzuki, O., et al. (2019). Vascularized bone-mimetic hydrogel constructs by 3D bioprinting to promote osteogenesis and angiogenesis. Int. J. Mol. Sci. 20, 1096. doi:10.3390/ijms20051096
ASTM (2014). Standard guide for pre-clinical in vivo evaluation in critical size segmental bone defects. Pennsylvania, United States: ASTM International. ASTM F2721-09.
Azevedo, H. S., and Pashkuleva, I. (2015). Biomimetic supramolecular designs for the controlled release of growth factors in bone regeneration. Adv. Drug Deliv. Rev. 94, 63–76. doi:10.1016/j.addr.2015.08.003
Bahney, C. S., Zondervan, R. L., Allison, P., Theologis, A., Ashley, J. W., Ahn, J., et al. (2019). Cellular biology of fracture healing. J. Orthop. Res. 37, 35–50. doi:10.1002/jor.24170
Beddoes, C. M., Whitehouse, M. R., Briscoe, W. H., and Su, B. (2016). Hydrogels as a replacement material for damaged articular hyaline cartilage. Mater. (Basel) 9, 443. doi:10.3390/ma9060443
Berendsen, A. D., and Olsen, B. R. (2014). How vascular endothelial growth factor-A (VEGF) regulates differentiation of mesenchymal stem cells. J. Histochem. Cytochem. 62, 103–108. doi:10.1369/0022155413516347
Bose, S., and Tarafder, S. (2012). Calcium phosphate ceramic systems in growth factor and drug delivery for bone tissue engineering: A review. Acta Biomater. 8, 1401–1421. doi:10.1016/j.actbio.2011.11.017
Buwalda, S. J., Vermonden, T., and Hennink, W. E. (2017). Hydrogels for therapeutic delivery: Current developments and future directions. Biomacromolecules 18, 316–330. doi:10.1021/acs.biomac.6b01604
Calabrese, G., Giuffrida, R., Fabbi, C., Figallo, E., Lo Furno, D., Gulino, R., et al. (2016). Collagen-hydroxyapatite scaffolds induce human adipose derived stem cells osteogenic differentiation in vitro. PLoS One 11, e0151181. doi:10.1371/journal.pone.0151181
Caplan, A. I., and Correa, D. (2011). PDGF in bone formation and regeneration: New insights into a novel mechanism involving MSCs. J. Orthop. Res. 29, 1795–1803. doi:10.1002/jor.21462
Charlet, A., Bono, F., and Amstad, E. (2022). Mechanical reinforcement of granular hydrogels. Chem. Sci. 13, 3082–3093. doi:10.1039/d1sc06231j
Chatterjee, K., Lin-Gibson, S., Wallace, W. E., Parekh, S. H., Lee, Y. J., Cicerone, M. T., et al. (2010). The effect of 3D hydrogel scaffold modulus on osteoblast differentiation and mineralization revealed by combinatorial screening. Biomaterials 31, 5051–5062. doi:10.1016/j.biomaterials.2010.03.024
Chen, M., Zhang, Y., Zhang, W., and Li, J. (2020). Polyhedral oligomeric silsesquioxane-incorporated gelatin hydrogel promotes angiogenesis during vascularized bone regeneration. ACS Appl. Mat. Interfaces 12, 22410–22425. doi:10.1021/acsami.0c00714
Cheng, P., Cao, T., Zhao, X., Lu, W., Miao, S., Ning, F., et al. (2022). Nidogen1-enriched extracellular vesicles accelerate angiogenesis and bone regeneration by targeting Myosin-10 to regulate endothelial cell adhesion. Bioact. Mat. 12, 185–197. doi:10.1016/j.bioactmat.2021.10.021
Cheng, R., Xin, T., Liu, L., Wang, F., Ye, T., Deng, L., et al. (2020). A “three-in-one” injectable hydrogel platform with osteogenesis, angiogenesis and antibacterial for guiding bone regeneration. Appl. Mat. Today 20, 100763. doi:10.1016/j.apmt.2020.100763
Collins, M. N., Ren, G., Young, K., Pina, S., Reis, R. L., and Oliveira, J. M. (2021). Scaffold fabrication technologies and structure/function properties in bone tissue engineering. Adv. Funct. Mat. 31, 2010609. doi:10.1002/adfm.202010609
Dashnyam, K., Buitrago, J. O., Bold, T., Mandakhbayar, N., Perez, R. A., Knowles, J. C., et al. (2019). Angiogenesis-promoted bone repair with silicate-shelled hydrogel fiber scaffolds. Biomater. Sci. 7, 5221–5231. doi:10.1039/c9bm01103j
Daugela, P., Pranskunas, M., Juodzbalys, G., Liesiene, J., Baniukaitiene, O., Afonso, A., et al. (2018). Novel cellulose/hydroxyapatite scaffolds for bone tissue regeneration: In vitro and in vivo study. J. Tissue Eng. Regen. Med. 12, 1195–1208. doi:10.1002/term.2651
De Witte, T.-M., Fratila-Apachitei, L. E., Zadpoor, A. A., and Peppas, N. A. (2018). Bone tissue engineering via growth factor delivery: From scaffolds to complex matrices. Regen. Biomater. 5, 197–211. doi:10.1093/rb/rby013
Dewey, M. J., and Harley, B. A. C. (2021). Biomaterial design strategies to address obstacles in craniomaxillofacial bone repair. RSC Adv. 11, 17809–17827. doi:10.1039/d1ra02557k
Di Maggio, N., and Banfi, A. (2022). The osteo-angiogenic signaling crosstalk for bone regeneration: Harmony out of complexity. Curr. Opin. Biotechnol. 76, 102750. doi:10.1016/j.copbio.2022.102750
DiGirolamo, D. J., Clemens, T. L., and Kousteni, S. (2012). The skeleton as an endocrine organ. Nat. Rev. Rheumatol. 8, 674–683. doi:10.1038/nrrheum.2012.157
Diomede, F., Marconi, G. D., Fonticoli, L., Pizzicanella, J., Merciaro, I., Bramanti, P., et al. (2020). Functional relationship between osteogenesis and angiogenesis in tissue regeneration. Int. J. Mol. Sci. 21, 3242. doi:10.3390/ijms21093242
Dixon, D. T., and Gomillion, C. T. (2021). Conductive scaffolds for bone tissue engineering: Current state and future outlook. J. Funct. Biomater. 13, 1. doi:10.3390/jfb13010001
Duan, X., Bradbury, S. R., Olsen, B. R., and Berendsen, A. D. (2016). VEGF stimulates intramembranous bone formation during craniofacial skeletal development. Matrix Biol. 52, 127–140. doi:10.1016/j.matbio.2016.02.005
Duan, X., Murata, Y., Liu, Y., Nicolae, C., Olsen, B. R., and Berendsen, A. D. (2015). Vegfa regulates perichondrial vascularity and osteoblast differentiation in bone development. Development 142, 1984–1991. doi:10.1242/dev.117952
El-Sherbiny, I. M., and Yacoub, M. H. (2013). Hydrogel scaffolds for tissue engineering: Progress and challenges. Glob. Cardiol. Sci. Pract. 2013, 316–342. doi:10.5339/gcsp.2013.38
Farokhi, M., Mottaghitalab, F., Shokrgozar, M. A., Ou, K.-L., Mao, C., and Hosseinkhani, H. (2016). Importance of dual delivery systems for bone tissue engineering. J. Control. Release 225, 152–169. doi:10.1016/j.jconrel.2016.01.033
Fredriksson, L., Li, H., and Eriksson, U. (2004). The PDGF family: Four gene products form five dimeric isoforms. Cytokine Growth Factor Rev. 15, 197–204. doi:10.1016/j.cytogfr.2004.03.007
Gao, C., Deng, Y., Feng, P., Mao, Z., Li, P., Yang, B., et al. (2014). Current progress in bioactive ceramic scaffolds for bone repair and regeneration. Int. J. Mol. Sci. 15, 4714–4732. doi:10.3390/ijms15034714
Gao, X., Usas, A., Proto, J. D., Lu, A., Cummins, J. H., Proctor, A., et al. (2014). Role of donor and host cells in muscle-derived stem cell-mediated bone repair: Differentiation vs. paracrine effects. FASEB J. 28, 3792–3809. doi:10.1096/fj.13-247965
Ge, Z., Jin, Z., and Cao, T. (2008). Manufacture of degradable polymeric scaffolds for bone regeneration. Biomed. Mat. 3, 022001. doi:10.1088/1748-6041/3/2/022001
Ghassemi, T., Shahroodi, A., Ebrahimzadeh, M. H., Mousavian, A., Movaffagh, J., and Moradi, A. (2018). Current concepts in scaffolding for bone tissue engineering. Arch. bone Jt. Surg. 6, 90–99.
Ghayor, C., and Weber, F. E. (2018). Osteoconductive microarchitecture of bone substitutes for bone regeneration revisited. Front. Physiol. 9, 960. doi:10.3389/fphys.2018.00960
Ghosh, M., Halperin-Sternfeld, M., Grinberg, I., and Adler-Abramovich, L. (2019). Injectable alginate-peptide composite hydrogel as a scaffold for bone tissue regeneration. Nanomaterials 9, 497. doi:10.3390/nano9040497
Gianni-Barrera, R., Di Maggio, N., Melly, L., Burger, M. G., Mujagic, E., Gürke, L., et al. (2020). Therapeutic vascularization in regenerative medicine. Stem Cells Transl. Med. 9, 433–444. doi:10.1002/sctm.19-0319
Goel, P. N., Moharrer, Y., Hebb, J. H., Egol, A. J., Kaur, G., Hankenson, K. D., et al. (2019). Suppression of Notch signaling in osteoclasts improves bone regeneration and healing. J. Orthop. Res. 37, 2089–2103. doi:10.1002/jor.24384
Goradel, N. H., Asghari, M. H., Moloudizargari, M., Negahdari, B., Haghi-Aminjan, H., and Abdollahi, M. (2017). Melatonin as an angiogenesis inhibitor to combat cancer: Mechanistic evidence. Toxicol. Appl. Pharmacol. 335, 56–63. doi:10.1016/j.taap.2017.09.022
Goradel, N. H., Hour, F. G., Negahdari, B., Malekshahi, Z. V., Hashemzehi, M., Masoudifar, A., et al. (2018). Stem cell therapy: A new therapeutic option for cardiovascular diseases. J. Cell. Biochem. 119, 95–104. doi:10.1002/jcb.26169
Goradel, N. H., Mohammadi, N., Haghi-Aminjan, H., Farhood, B., Negahdari, B., and Sahebkar, A. (2019). Regulation of tumor angiogenesis by microRNAs: State of the art. J. Cell. Physiol. 234, 1099–1110. doi:10.1002/jcp.27051
Grosso, A., Burger, M. G., Lunger, A., Schaefer, D. J., Banfi, A., and Di Maggio, N. (2017). It takes two to tango: Coupling of angiogenesis and osteogenesis for bone regeneration. Front. Bioeng. Biotechnol. 5, 68. doi:10.3389/fbioe.2017.00068
Guo, Q., Yang, J., Chen, Y., Jin, X., Li, Z., Wen, X., et al. (2020). Salidroside improves angiogenesis-osteogenesis coupling by regulating the HIF-1α/VEGF signalling pathway in the bone environment. Eur. J. Pharmacol. 884, 173394. doi:10.1016/j.ejphar.2020.173394
Hashemi Goradel, N., Ghiyami-Hour, F., Jahangiri, S., Negahdari, B., Sahebkar, A., Masoudifar, A., et al. (2018). Nanoparticles as new tools for inhibition of cancer angiogenesis. J. Cell. Physiol. 233, 2902–2910. doi:10.1002/jcp.26029
Hu, K., and Olsen, B. R. (2017). Vascular endothelial growth factor control mechanisms in skeletal growth and repair. Dev. Dyn. 246, 227–234. doi:10.1002/dvdy.24463
Ji, X., Yuan, X., Ma, L., Bi, B., Zhu, H., Lei, Z., et al. (2020). Mesenchymal stem cell-loaded thermosensitive hydroxypropyl chitin hydrogel combined with a three-dimensional-printed poly (ε-caprolactone)/nano-hydroxyapatite scaffold to repair bone defects via osteogenesis, angiogenesis and immunomodulation. Theranostics 10, 725–740. doi:10.7150/thno.39167
Jin, Q., Yuan, K., Lin, W., Niu, C., Ma, R., and Huang, Z. (2019). Comparative characterization of mesenchymal stem cells from human dental pulp and adipose tissue for bone regeneration potential. Artif. cells, nanomedicine, Biotechnol. 47, 1577–1584. doi:10.1080/21691401.2019.1594861
Jin, X., Dai, L., Ma, Y., Wang, J., and Liu, Z. (2020). Implications of HIF-1α in the tumorigenesis and progression of pancreatic cancer. Cancer Cell Int. 20, 273–311. doi:10.1186/s12935-020-01370-0
Kantaros, A., Chatzidai, N., and Karalekas, D. (2016). 3D printing-assisted design of scaffold structures. Int. J. Adv. Manuf. Technol. 82, 559–571. doi:10.1007/s00170-015-7386-6
Kazemi-Aghdam, F., Jahed, V., Dehghan-Niri, M., Ganji, F., and Vasheghani-Farahani, E. (2021). Injectable chitosan hydrogel embedding modified halloysite nanotubes for bone tissue engineering. Carbohydr. Polym. 269, 118311. doi:10.1016/j.carbpol.2021.118311
Kikuchi, M., Matsumoto, H. N., Yamada, T., Koyama, Y., Takakuda, K., and Tanaka, J. (2004). Glutaraldehyde cross-linked hydroxyapatite/collagen self-organized nanocomposites. Biomaterials 25, 63–69. doi:10.1016/s0142-9612(03)00472-1
Kim, B.-J., Lee, Y.-S., Lee, S.-Y., Baek, W.-Y., Choi, Y. J., Moon, S. A., et al. (2018). Osteoclast-secreted SLIT3 coordinates bone resorption and formation. J. Clin. Invest. 128, 1429–1441. doi:10.1172/JCI91086
Kim, G., Park, Y. S., Lee, Y., Jin, Y. M., Choi, D. H., Ryu, K.-H., et al. (2018). Tonsil-derived mesenchymal stem cell-embedded in situ crosslinkable gelatin hydrogel therapy recovers postmenopausal osteoporosis through bone regeneration. PLoS One 13, e0200111. doi:10.1371/journal.pone.0200111
Kim, S., Kang, Y., Krueger, C. A., Sen, M., Holcomb, J. B., Chen, D., et al. (2012). Sequential delivery of BMP-2 and IGF-1 using a chitosan gel with gelatin microspheres enhances early osteoblastic differentiation. Acta Biomater. 8, 1768–1777. doi:10.1016/j.actbio.2012.01.009
Kolambkar, Y. M., Dupont, K. M., Boerckel, J. D., Huebsch, N., Mooney, D. J., Hutmacher, D. W., et al. (2011). An alginate-based hybrid system for growth factor delivery in the functional repair of large bone defects. Biomaterials 32, 65–74. doi:10.1016/j.biomaterials.2010.08.074
Kraus, J. M., Giovannone, D., Rydzik, R., Balsbaugh, J. L., Moss, I. L., Schwedler, J. L., et al. (2022). Notch signaling enhances bone regeneration in the zebrafish mandible. Development 149, dev199995. doi:10.1242/dev.199995
Krishnakumar, G. S., Sampath, S., Muthusamy, S., and John, M. A. (2019). Importance of crosslinking strategies in designing smart biomaterials for bone tissue engineering: A systematic review. Mat. Sci. Eng. C 96, 941–954. doi:10.1016/j.msec.2018.11.081
Kuttor, A., Szalóki, M., Rente, T., Kerényi, F., Bakó, J., Fábián, I., et al. (2014). Preparation and application of highly porous aerogel-based bioactive materials in dentistry. Front. Mat. Sci. 8, 46–52. doi:10.1007/s11706-014-0231-2
Lalu, M. M., McIntyre, L., Pugliese, C., Fergusson, D., Winston, B. W., Marshall, J. C., et al. (2012). Safety of cell therapy with mesenchymal stromal cells (SafeCell): A systematic review and meta-analysis of clinical trials. PLoS One 7, e47559. doi:10.1371/journal.pone.0047559
Li, D., Yang, Z., Zhao, X., Luo, Y., Zhou, W., Xu, J., et al. (2022). Osteoimmunomodulatory injectable Lithium-Heparin hydrogel with Microspheres/TGF-β1 delivery promotes M2 macrophage polarization and osteogenesis for guided bone regeneration. Chem. Eng. J. 435, 134991. doi:10.1016/j.cej.2022.134991
Li, J., Gao, L., Xu, R., Ma, S., Ma, Z., Liu, Y., et al. (2022). Fibers reinforced composite hydrogels with improved lubrication and load-bearing capacity. Friction 10, 54–67. doi:10.1007/s40544-020-0389-9
Li, N., Inoue, K., Sun, J., Niu, Y., Lalani, S., Yallowitz, A., et al. (2020). Osteoclasts are not a source of SLIT3. Bone Res. 8, 11–19. doi:10.1038/s41413-020-0086-3
Li, Y., Jahr, H., Zhou, J., and Zadpoor, A. A. (2020). Additively manufactured biodegradable porous metals. Acta Biomater. 115, 29–50. doi:10.1016/j.actbio.2020.08.018
Liao, J., Wei, Q., Zou, Y., Fan, J., Song, D., Cui, J., et al. (2017). Notch signaling augments BMP9-induced bone formation by promoting the osteogenesis-angiogenesis coupling process in mesenchymal stem cells (MSCs). Cell. Physiol. biochem. 41, 1905–1923. doi:10.1159/000471945
Lienemann, P. S., Lutolf, M. P., and Ehrbar, M. (2012). Biomimetic hydrogels for controlled biomolecule delivery to augment bone regeneration. Adv. Drug Deliv. Rev. 64, 1078–1089. doi:10.1016/j.addr.2012.03.010
Lin, X., Patil, S., Gao, Y.-G., and Qian, A. (2020). The bone extracellular matrix in bone formation and regeneration. Front. Pharmacol. 11, 757. doi:10.3389/fphar.2020.00757
Lindert, U., Cabral, W. A., Ausavarat, S., Tongkobpetch, S., Ludin, K., Barnes, A. M., et al. (2016). MBTPS2 mutations cause defective regulated intramembrane proteolysis in X-linked osteogenesis imperfecta. Nat. Commun. 7, 11920–12012. doi:10.1038/ncomms11920
Liu, H., Li, W., Liu, C., Tan, J., Wang, H., Hai, B., et al. (2016). Incorporating simvastatin/poloxamer 407 hydrogel into 3D-printed porous Ti6Al4V scaffolds for the promotion of angiogenesis, osseointegration and bone ingrowth. Biofabrication 8, 045012. doi:10.1088/1758-5090/8/4/045012
Liu, J., Chuah, Y. J., Fu, J., Zhu, W., and Wang, D.-A. (2019). Co-culture of human umbilical vein endothelial cells and human bone marrow stromal cells into a micro-cavitary gelatin-methacrylate hydrogel system to enhance angiogenesis. Mat. Sci. Eng. C 102, 906–916. doi:10.1016/j.msec.2019.04.089
Liu, L., Yang, B., Wang, L.-Q., Huang, J.-P., Chen, W.-Y., Ban, Q., et al. (2020). Biomimetic bone tissue engineering hydrogel scaffolds constructed using ordered CNTs and HA induce the proliferation and differentiation of BMSCs. J. Mat. Chem. B 8, 558–567. doi:10.1039/c9tb01804b
Liu, Y., Berendsen, A. D., Jia, S., Lotinun, S., Baron, R., Ferrara, N., et al. (2012). Intracellular VEGF regulates the balance between osteoblast and adipocyte differentiation. J. Clin. Invest. 122, 3101–3113. doi:10.1172/JCI61209
Lobb, D. C., DeGeorge, B. R., and Chhabra, A. B. (2019). Bone graft substitutes: Current concepts and future expectations. J. Hand Surg. Am. 44, 497–505. doi:10.1016/j.jhsa.2018.10.032
Luo, Z., Shang, X., Zhang, H., Wang, G., Massey, P. A., Barton, S. R., et al. (2019). Notch signaling in osteogenesis, osteoclastogenesis, and angiogenesis. Am. J. Pathol. 189, 1495–1500. doi:10.1016/j.ajpath.2019.05.005
Luvizuto, E. R., Tangl, S., Dobsak, T., Reich, K., Gruber, R., Sonoda, C. K., et al. (2016). Effect of recombinant PDGF-BB on bone formation in the presence of β-tricalcium phosphate and bovine bone mineral matrix: A pilot study in rat calvarial defects. BMC Oral Health 16, 52–57. doi:10.1186/s12903-016-0210-3
Maisani, M., Pezzoli, D., Chassande, O., and Mantovani, D. (2017). Cellularizing hydrogel-based scaffolds to repair bone tissue: How to create a physiologically relevant micro-environment? J. Tissue Eng. 8, 2041731417712073. doi:10.1177/2041731417712073
Maisani, M., Ziane, S., Ehret, C., Levesque, L., Siadous, R., Le Meins, J., et al. (2018). A new composite hydrogel combining the biological properties of collagen with the mechanical properties of a supramolecular scaffold for bone tissue engineering. J. Tissue Eng. Regen. Med. 12, e1489–e1500. doi:10.1002/term.2569
Marchand, M., Monnot, C., Muller, L., and Germain, S. (2019). Extracellular matrix scaffolding in angiogenesis and capillary homeostasis. Seminars Cell and Dev. Biol. 89, 147–156. Elsevier. doi:10.1016/j.semcdb.2018.08.007
Meppelink, A. M., Zhao, X., Griffin, D. J., Erali, R., Gill, T. J., Bonassar, L. J., et al. (2016). Hyaline articular matrix formed by dynamic self-regenerating cartilage and hydrogels. Tissue Eng. Part A 22, 962–970. doi:10.1089/ten.TEA.2015.0577
Naruphontjirakul, P., Tsigkou, O., Li, S., Porter, A. E., and Jones, J. R. (2019). Human mesenchymal stem cells differentiate into an osteogenic lineage in presence of strontium containing bioactive glass nanoparticles. Acta Biomater. 90, 373–392. doi:10.1016/j.actbio.2019.03.038
Nyberg, E., Holmes, C., Witham, T., and Grayson, W. L. (2016). Growth factor-eluting technologies for bone tissue engineering. Drug Deliv. Transl. Res. 6, 184–194. doi:10.1007/s13346-015-0233-3
Ogata, K., Katagiri, W., and Hibi, H. (2017). Secretomes from mesenchymal stem cells participate in the regulation of osteoclastogenesis in vitro. Clin. Oral Investig. 21, 1979–1988. doi:10.1007/s00784-016-1986-x
Paduano, F., Marrelli, M., Amantea, M., Rengo, C., Rengo, S., Goldberg, M., et al. (2017). Adipose tissue as a strategic source of mesenchymal stem cells in bone regeneration: A topical review on the most promising craniomaxillofacial applications. Int. J. Mol. Sci. 18, 2140. doi:10.3390/ijms18102140
Pakvasa, M., Haravu, P., Boachie-Mensah, M., Jones, A., Coalson, E., Liao, J., et al. (2021). Notch signaling: Its essential roles in bone and craniofacial development. Genes Dis. 8, 8–24. doi:10.1016/j.gendis.2020.04.006
Park, S. H., Park, J. Y., Ji, Y. B., Ju, H. J., Min, B. H., and Kim, M. S. (2020). An injectable click-crosslinked hyaluronic acid hydrogel modified with a BMP-2 mimetic peptide as a bone tissue engineering scaffold. Acta Biomater. 117, 108–120. doi:10.1016/j.actbio.2020.09.013
Peier, M., Walpen, T., Christofori, G., Battegay, E., and Humar, R. (2013). Sprouty2 expression controls endothelial monolayer integrity and quiescence. Angiogenesis 16, 455–468. doi:10.1007/s10456-012-9330-9
Peng, Y., Wu, S., Li, Y., and Crane, J. L. (2020). Type H blood vessels in bone modeling and remodeling. Theranostics 10, 426–436. doi:10.7150/thno.34126
Pereira, T., Zheng, X., and Poellinger, L. (2006). Degradation of the hypoxia-inducible factor 1α, where Does it happen? Cell Cycle 5, 2720–2722. doi:10.4161/cc.5.23.3536
Pillai, O., and Panchagnula, R. (2003). Transdermal delivery of insulin from poloxamer gel: Ex vivo and in vivo skin permeation studies in rat using iontophoresis and chemical enhancers. J. Control. Release 89, 127–140. doi:10.1016/s0168-3659(03)00094-4
Ping, J., Zhou, C., Dong, Y., Wu, X., Huang, X., Sun, B., et al. (2021). Modulating immune microenvironment during bone repair using biomaterials: Focusing on the role of macrophages. Mol. Immunol. 138, 110–120. doi:10.1016/j.molimm.2021.08.003
Quinlan, E., Partap, S., Azevedo, M. M., Jell, G., Stevens, M. M., and O’Brien, F. J. (2015). Hypoxia-mimicking bioactive glass/collagen glycosaminoglycan composite scaffolds to enhance angiogenesis and bone repair. Biomaterials 52, 358–366. doi:10.1016/j.biomaterials.2015.02.006
Ramasamy, S. K., Kusumbe, A. P., Schiller, M., Zeuschner, D., Bixel, M. G., Milia, C., et al. (2016). Blood flow controls bone vascular function and osteogenesis. Nat. Commun. 7, 13601–13613. doi:10.1038/ncomms13601
Roseti, L., Parisi, V., Petretta, M., Cavallo, C., Desando, G., Bartolotti, I., et al. (2017). Scaffolds for bone tissue engineering: State of the art and new perspectives. Mat. Sci. Eng. C 78, 1246–1262. doi:10.1016/j.msec.2017.05.017
Rupp, M., Klute, L., Baertl, S., Walter, N., Mannala, G., Frank, L., et al. (2022). The clinical use of bone graft substitutes in orthopedic surgery in Germany—a 10-years survey from 2008 to 2018 of 1,090,167 surgical interventions. J. Biomed. Mat. Res. Part B Appl. Biomater. 110, 350–357. doi:10.1002/jbm.b.34911
Saravanan, S., Vimalraj, S., and Anuradha, D. (2018). Chitosan based thermoresponsive hydrogel containing graphene oxide for bone tissue repair. Biomed. Pharmacother. 107, 908–917. doi:10.1016/j.biopha.2018.08.072
Sarker, B., Li, W., Zheng, K., Detsch, R., and Boccaccini, A. R. (2016). Designing porous bone tissue engineering scaffolds with enhanced mechanical properties from composite hydrogels composed of modified alginate, gelatin, and bioactive glass. ACS Biomater. Sci. Eng. 2, 2240–2254. doi:10.1021/acsbiomaterials.6b00470
Seitz, H., Rieder, W., Irsen, S., Leukers, B., and Tille, C. (2005). Three-dimensional printing of porous ceramic scaffolds for bone tissue engineering. J. Biomed. Mater Res. B Appl. Biomater. 74, 782–788. doi:10.1002/jbm.b.30291
Shi, C., Yuan, Z., Han, F., Zhu, C., and Li, B. (2016). Polymeric biomaterials for bone regeneration. Ann. Jt. 1, 27. doi:10.21037/aoj.2016.11.02
St John, T. A., Vaccaro, A. R., Sah, A. P., Schaefer, M., Berta, S. C., Albert, T., et al. (2003). Physical and monetary costs associated with autogenous bone graft harvesting. Am. J. Orthop. (Belle Mead, NJ) 32, 18–23.
Sun, Y., Liu, X., Zhu, Y., Han, Y., Shen, J., Bao, B., et al. (2021). Tunable and controlled release of cobalt ions from metal–organic framework hydrogel nanocomposites enhances bone regeneration. ACS Appl. Mat. Interfaces 13, 59051–59066. doi:10.1021/acsami.1c16300
Tamburaci, S., Cecen, B., Ustun, O., Ergur, B. U., Havitcioglu, H., and Tihminlioglu, F. (2019). Production and characterization of a novel bilayer nanocomposite scaffold composed of chitosan/Si-nHap and zein/POSS structures for osteochondral tissue regeneration. ACS Appl. Bio Mat. 2, 1440–1455. doi:10.1021/acsabm.8b00700
Tan, J., Fu, X., Sun, C. G., Liu, C., Zhang, X. H., Cui, Y. Y., et al. (2016). A single CT-guided percutaneous intraosseous injection of thermosensitive simvastatin/poloxamer 407 hydrogel enhances vertebral bone formation in ovariectomized minipigs. Osteoporos. Int. 27, 757–767. doi:10.1007/s00198-015-3230-y
Tang, Y., Luo, K., Chen, Y., Chen, Y., Zhou, R., Chen, C., et al. (2021). Phosphorylation inhibition of protein-tyrosine phosphatase 1B tyrosine-152 induces bone regeneration coupled with angiogenesis for bone tissue engineering. Bioact. Mat. 6, 2039–2057. doi:10.1016/j.bioactmat.2020.12.025
Thavornyutikarn, B., Chantarapanich, N., Sitthiseripratip, K., Thouas, G. A., and Chen, Q. (2014). Bone tissue engineering scaffolding: Computer-aided scaffolding techniques. Prog. Biomater. 3, 61–102. doi:10.1007/s40204-014-0026-7
Walpole, A. R., Xia, Z., Wilson, C. W., Triffitt, J. T., and Wilshaw, P. R. (2009). A novel nano-porous alumina biomaterial with potential for loading with bioactive materials. J. Biomed. Mater Res. A 90, 46–54. doi:10.1002/jbm.a.32067
Wang, C., Liu, Y., and He, D. (2019). Diverse effects of platelet-derived growth factor-BB on cell signaling pathways. Cytokine 113, 13–20. doi:10.1016/j.cyto.2018.10.019
Wang, X., Wang, Y., Gou, W., Lu, Q., Peng, J., and Lu, S. (2013). Role of mesenchymal stem cells in bone regeneration and fracture repair: A review. Int. Orthop. 37, 2491–2498. doi:10.1007/s00264-013-2059-2
Wang, Y., Cao, X., Ma, M., Lu, W., Zhang, B., and Guo, Y. (2020). A GelMA-PEGDA-nHA composite hydrogel for bone tissue engineering. Mater. (Basel) 13, 3735. doi:10.3390/ma13173735
Wang, Y., Xue, Y., Wang, J., Zhu, Y., Zhu, Y., Zhang, X., et al. (2019). A composite hydrogel with high mechanical strength, fluorescence, and degradable behavior for bone tissue engineering. Polym. (Basel) 11, 1112. doi:10.3390/polym11071112
Wietecha, M. S., Chen, L., Ranzer, M. J., Anderson, K., Ying, C., Patel, T. B., et al. (2011). Sprouty2 downregulates angiogenesis during mouse skin wound healing. Am. J. Physiol. Circ. Physiol. 300, H459–H467. doi:10.1152/ajpheart.00244.2010
Wu, C., Ramaswamy, Y., and Zreiqat, H. (2010). Porous diopside (CaMgSi2O6) scaffold: A promising bioactive material for bone tissue engineering. Acta Biomater. 6, 2237–2245. doi:10.1016/j.actbio.2009.12.022
Wu, C., Zhou, Y., Fan, W., Han, P., Chang, J., Yuen, J., et al. (2012). Hypoxia-mimicking mesoporous bioactive glass scaffolds with controllable cobalt ion release for bone tissue engineering. Biomaterials 33, 2076–2085. doi:10.1016/j.biomaterials.2011.11.042
Wu, D., Qin, H., Wang, Z., Yu, M., Liu, Z., Peng, H., et al. (2022). Bone mesenchymal stem cell-derived sEV-encapsulated thermosensitive hydrogels accelerate osteogenesis and angiogenesis by release of exosomal miR-21. Front. Bioeng. Biotechnol. 9, 829136. doi:10.3389/fbioe.2021.829136
Wu, L., Virdee, J., Maughan, E., Darbyshire, A., Jell, G., Loizidou, M., et al. (2018). Stiffness memory nanohybrid scaffolds generated by indirect 3D printing for biologically responsive soft implants. Acta Biomater. 80, 188–202. doi:10.1016/j.actbio.2018.09.016
Wu, Z., Bai, J., Ge, G., Wang, T., Feng, S., Ma, Q., et al. (2022). Regulating macrophage polarization in high glucose microenvironment using lithium-modified bioglass-hydrogel for diabetic bone regeneration. Adv. Healthc. Mat. 11, 2200298. doi:10.1002/adhm.202200298
Xie, H., Cui, Z., Wang, L., Xia, Z., Hu, Y., Xian, L., et al. (2014). PDGF-BB secreted by preosteoclasts induces angiogenesis during coupling with osteogenesis. Nat. Med. 20, 1270–1278. doi:10.1038/nm.3668
Xu, R., Yallowitz, A., Qin, A., Wu, Z., Shin, D. Y., Kim, J.-M., et al. (2018). Targeting skeletal endothelium to ameliorate bone loss. Nat. Med. 24, 823–833. doi:10.1038/s41591-018-0020-z
Xu, T., Yang, H., Yang, D., and Yu, Z.-Z. (2017). Polylactic acid nanofiber scaffold decorated with chitosan islandlike topography for bone tissue engineering. ACS Appl. Mat. Interfaces 9, 21094–21104. doi:10.1021/acsami.7b01176
Xue, N., Ding, X., Huang, R., Jiang, R., Huang, H., Pan, X., et al. (2022). Bone tissue engineering in the treatment of bone defects. Pharmaceuticals 15, 879. doi:10.3390/ph15070879
Xue, X., Hu, Y., Deng, Y., and Su, J. (2021). Recent advances in design of functional biocompatible hydrogels for bone tissue engineering. Adv. Funct. Mat. 31, 2009432. doi:10.1002/adfm.202009432
Yang, Z., Yang, Z., Ding, L., Zhang, P., Liu, C., Chen, D., et al. (2022). Self-adhesive hydrogel biomimetic periosteum to promote critical-size bone defect repair via synergistic osteogenesis and angiogenesis. ACS Appl. Mat. Interfaces 14, 36395–36410. doi:10.1021/acsami.2c08400
Yegappan, R., Selvaprithiviraj, V., Amirthalingam, S., Mohandas, A., Hwang, N. S., and Jayakumar, R. (2019). Injectable angiogenic and osteogenic carrageenan nanocomposite hydrogel for bone tissue engineering. Int. J. Biol. Macromol. 122, 320–328. doi:10.1016/j.ijbiomac.2018.10.182
Yoshizawa, S., Brown, A., Barchowsky, A., and Sfeir, C. (2014). Magnesium ion stimulation of bone marrow stromal cells enhances osteogenic activity, simulating the effect of magnesium alloy degradation. Acta Biomater. 10, 2834–2842. doi:10.1016/j.actbio.2014.02.002
Younger, E. M., and Chapman, M. W. (1989). Morbidity at bone graft donor sites. J. Orthop. Trauma 3, 192–195. doi:10.1097/00005131-198909000-00002
Yu, Y., Jin, G., Xue, Y., Wang, D., Liu, X., and Sun, J. (2017). Multifunctions of dual Zn/Mg ion co-implanted titanium on osteogenesis, angiogenesis and bacteria inhibition for dental implants. Acta Biomater. 49, 590–603. doi:10.1016/j.actbio.2016.11.067
Yue, S., He, H., Li, B., and Hou, T. (2020). Hydrogel as a biomaterial for bone tissue engineering: A review. Nanomaterials 10, 1511. doi:10.3390/nano10081511
Zhang, B., Huang, J., Liu, J., Lin, F., Ding, Z., and Xu, J. (2021). Injectable composite hydrogel promotes osteogenesis and angiogenesis in spinal fusion by optimizing the bone marrow mesenchymal stem cell microenvironment and exosomes secretion. Mat. Sci. Eng. C 123, 111782. doi:10.1016/j.msec.2020.111782
Zhang, B., Pei, X., Song, P., Sun, H., Li, H., Fan, Y., et al. (2018). Porous bioceramics produced by inkjet 3D printing: Effect of printing ink formulation on the ceramic macro and micro porous architectures control. Compos. Part B Eng. 155, 112–121. doi:10.1016/j.compositesb.2018.08.047
Zhang, J., Feng, Z., Wei, J., Yu, Y., Luo, J., Zhou, J., et al. (2018). Repair of critical-sized mandible defects in aged rat using hypoxia preconditioned BMSCs with up-regulation of Hif-1α. Int. J. Biol. Sci. 14, 449–460. doi:10.7150/ijbs.24158
Zhang, J., Luo, X., Barbieri, D., Barradas, A. M. C., de Bruijn, J. D., Van Blitterswijk, C. A., et al. (2014). The size of surface microstructures as an osteogenic factor in calcium phosphate ceramics. Acta Biomater. 10, 3254–3263. doi:10.1016/j.actbio.2014.03.021
Zhang, J., Wehrle, E., Adamek, P., Paul, G. R., Qin, X.-H., Rubert, M., et al. (2020). Optimization of mechanical stiffness and cell density of 3D bioprinted cell-laden scaffolds improves extracellular matrix mineralization and cellular organization for bone tissue engineering. Acta Biomater. 114, 307–322. doi:10.1016/j.actbio.2020.07.016
Zhang, L., Jiao, G., Ren, S., Zhang, X., Li, C., Wu, W., et al. (2020). Exosomes from bone marrow mesenchymal stem cells enhance fracture healing through the promotion of osteogenesis and angiogenesis in a rat model of nonunion. Stem Cell Res. Ther. 11, 38–15. doi:10.1186/s13287-020-1562-9
Zhang, M., Yu, W., Niibe, K., Zhang, W., Egusa, H., Tang, T., et al. (2018). The effects of platelet-derived growth factor-BB on bone marrow stromal cell-mediated vascularized bone regeneration. Stem Cells Int. 2018, 3272098. doi:10.1155/2018/3272098
Zhang N, N., Lo, C.-W., Utsunomiya, T., Maruyama, M., Huang, E., Rhee, C., et al. (2021). PDGF-BB and IL-4 co-overexpression is a potential strategy to enhance mesenchymal stem cell-based bone regeneration. Stem Cell Res. Ther. 12, 40–11. doi:10.1186/s13287-020-02086-8
Zhang, X., Huang, P., Jiang, G., Zhang, M., Yu, F., Dong, X., et al. (2021). A novel magnesium ion-incorporating dual-crosslinked hydrogel to improve bone scaffold-mediated osteogenesis and angiogenesis. Mat. Sci. Eng. C 121, 111868. doi:10.1016/j.msec.2021.111868
Zhang, Y., Xie, Y., Hao, Z., Zhou, P., Wang, P., Fang, S., et al. (2021). Umbilical mesenchymal stem cell-derived exosome-encapsulated hydrogels accelerate bone repair by enhancing angiogenesis. ACS Appl. Mat. Interfaces 13, 18472–18487. doi:10.1021/acsami.0c22671
Zhao, C., Qazvini, N. T., Sadati, M., Zeng, Z., Huang, S., De La Lastra, A. L., et al. (2019). A pH-triggered, self-assembled, and bioprintable hybrid hydrogel scaffold for mesenchymal stem cell based bone tissue engineering. ACS Appl. Mat. Interfaces 11, 8749–8762. doi:10.1021/acsami.8b19094
Zhao, S., Wen, F., He, F., Liu, L., and Yang, G. (2014). In vitro and in vivo evaluation of the osteogenic ability of implant surfaces with a local delivery of simvastatin. Int. J. Oral Maxillofac. Implants 29 (1), 211–220. doi:10.11607/jomi.3147
Zheng, H., Bai, Y., Shih, M., Hoffmann, C., Peters, F., Waldner, C., et al. (2014). Effect of a β-TCP collagen composite bone substitute on healing of drilled bone voids in the distal femoral condyle of rabbits. J. Biomed. Mat. Res. Part B Appl. Biomater. 102, 376–383. doi:10.1002/jbm.b.33016
Zhou, J., Li, B., Han, Y., and Zhao, L. (2016). The osteogenic capacity of biomimetic hierarchical micropore/nanorod-patterned Sr-HA coatings with different interrod spacings. Nanomedicine Nanotechnol. Biol. Med. 12, 1161–1173. doi:10.1016/j.nano.2016.01.011
Zhu, G., Zhang, T., Chen, M., Yao, K., Huang, X., Zhang, B., et al. (2021). Bone physiological microenvironment and healing mechanism: Basis for future bone-tissue engineering scaffolds. Bioact. Mat. 6, 4110–4140. doi:10.1016/j.bioactmat.2021.03.043
Zhu, J., and Marchant, R. E. (2011). Design properties of hydrogel tissue-engineering scaffolds. Expert Rev. Med. Devices 8, 607–626. doi:10.1586/erd.11.27
Keywords: bone tissue engineering, scaffold, biomaterial, hydrogel, angiogenesis
Citation: Liu J, Yang L, Liu K and Gao F (2023) Hydrogel scaffolds in bone regeneration: Their promising roles in angiogenesis. Front. Pharmacol. 14:1050954. doi: 10.3389/fphar.2023.1050954
Received: 22 September 2022; Accepted: 03 February 2023;
Published: 13 February 2023.
Edited by:
Joaquim Miguel Oliveira, University of Minho, PortugalReviewed by:
Maryam Tamaddon, University College London, United KingdomAnuj Kumar, Yeungnam University, Republic of Korea
Copyright © 2023 Liu, Yang, Liu and Gao. This is an open-access article distributed under the terms of the Creative Commons Attribution License (CC BY). The use, distribution or reproduction in other forums is permitted, provided the original author(s) and the copyright owner(s) are credited and that the original publication in this journal is cited, in accordance with accepted academic practice. No use, distribution or reproduction is permitted which does not comply with these terms.
*Correspondence: Feng Gao, ZmVuZ2dhbzgyMTAyNkAxNjMuY29t