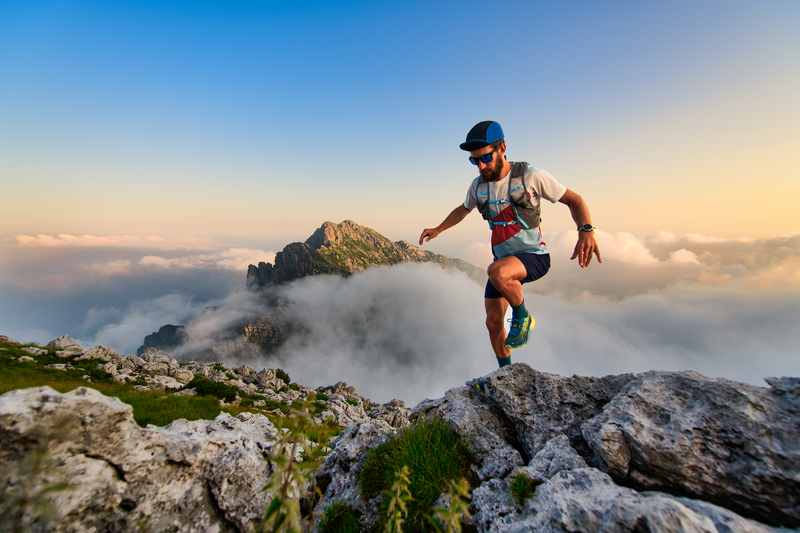
95% of researchers rate our articles as excellent or good
Learn more about the work of our research integrity team to safeguard the quality of each article we publish.
Find out more
REVIEW article
Front. Pharmacol. , 19 August 2022
Sec. Pharmacology of Anti-Cancer Drugs
Volume 13 - 2022 | https://doi.org/10.3389/fphar.2022.990505
Nanoparticles based on single-component synthetic polymers, such as poly (lactic acid-co-glycolic acid) (PLGA), have been extensively studied for antitumor drug delivery and adjuvant therapy due to their ability to encapsulate and release drugs, as well as passively target tumors. Amphiphilic block co-polymers, such as polyethylene glycol (PEG)-PLGA, have also been used to prepare multifunctional nanodrug delivery systems with prolonged circulation time and greater bioavailability that can encapsulate a wider variety of drugs, including small molecules, gene-targeting drugs, traditional Chinese medicine (TCM) and multi-target enzyme inhibitors, enhancing their antitumor effect and safety. In addition, the surface of PEG-PLGA nanoparticles has been modified with various ligands to achieve active targeting and selective accumulation of antitumor drugs in tumor cells. Modification with two ligands has also been applied with good antitumor effects, while the use of imaging agents and pH-responsive or magnetic materials has paved the way for the application of such nanoparticles in clinical diagnosis. In this work, we provide an overview of the synthesis and application of PEG-PLGA nanoparticles in cancer treatment and we discuss the recent advances in ligand modification for active tumor targeting.
Tumor malignancies are the second leading cause of death worldwide and their treatment remains expensive and complex (Wiśniewski et al., 2020). Tumor drug therapies mainly include treatment with cytotoxic small molecules, drugs targeting genes or other molecules, as well as active substances of traditional Chinese medicine (TCM). Although more than half of the 170 drugs used for cancer treatment target specific molecules (Levêque and Becker, 2019), chemotherapy and adjuvant TCM treatment are also widely used in clinical practice due to their relatively low price and good therapeutic effect. However, current therapeutic agents suffer from low bioavailability, rapid elimination in vivo, high toxicity to normal host cells, and low retention at the tumor site. Peptides used in anti-tumor therapy have the advantages of good membrane permeability and high specificity, but their disadvantages such as high cost, short circulating half-life and rapid clearance in vivo limit their wide clinical application (Firer and Gellerman, 2012; Ciobanasu, 2021). The new generation of nanocarrier materials MOFs (metal organic frameworks) has certain potential in disease diagnosis and drug targeted delivery (Karami et al., 2021). However, problems such as the high cost of MOFs synthesis, premature drug release caused by rapid metabolism in vivo or clearance by the immune system, carrier stability, and lack of biocompatibility studies still indicate that clinical application of MOFs needs more exploration (Cai et al., 2020).
Nanoparticles (NPs) have been identified as ideal carriers for various types of drugs due to their biodegradability, biocompatibility, storage stability, and easy surface modification (Mitchell et al., 2021). In addition, their particle size and unique surface properties make them suitable for passive targeting of solid tumors: due to the enhanced permeability and retention (EPR) effect, NPs can be retained within the rich blood vessels and on the extensive vascular surfaces in the tumor tissue, where there is no lymphatic reflux to clear them away. For instance, NPs based on the US Food and Drug Administration (FDA)-approved poly (lactic acid-co-glycolic acid) (PLGA) have been widely used to encapsulate almost all types of antitumor drugs, offering good biodegradability, minimal systemic toxicity, and high bioavailability (Khan et al., 2016a). However, their application is limited because intravenously administered PLGA NPs are easily opsonized and rapidly cleared by the reticular endothelial system (Noori Koopaei et al., 2014).
In order to improve the properties of PLGA NPs and achieve long-term therapeutic effects, polyethylene glycol (PEG) has been conjugated with PLGA to construct a new type of amphiphilic block co-polymer nanoplatform, PEG-PLGA NPs. Compared with unPEGylated PLGA nanoparticles, PEGylated PLGA nanoparticles showed a characteristic improvement in the symptoms of multiple sclerosis in mice (Li P. Y. et al., 2021), indicating their potential to improve immune tolerance. Pegylated lipid-PLGA hybrid NPs can significantly reduce the fusion phenomenon of nanoparticles during storage, and further improve the internalization of cell uptake experiments while improving stability (Hu et al., 2015). In studies of PLGA-based magnetic nanoparticles, PEGylation reduces neurotoxicity and improves the stability of the loaded therapeutic DNA in primary hippocampal neurons (Cui et al., 2019). PEGylation also improves the biocompatibility of PLGA-based contrast agent composite nanomaterials to some extent (Xu et al., 2015). The novel preparation showed improved drug encapsulation efficiency and controlled release, especially of chemotherapeutic drugs, active TCM substances, and gene-targeting drugs. In addition, PEG-PLGA NPs exhibited high stability, good bioavailability, and enhanced passive targeting ability by the EPR effect, which promoted the targeted accumulation of the drug at the tumor site and improved its safety.
The surface of PEG-PLGA NPs has also been modified with various ligands, such as glycyrrhetinic acid, chondroitin sulfate, alendronate, polyethylenimine, iRGD (the arginine-glycine-aspartate peptide), and estradiol, in order to allow the NPs to target tumors not only passively but also actively. Extensive studies on the mechanism of highly invasive and metastatic tumors have revealed a large number of abnormally expressed proteins, such as cell adhesion molecules, that can serve as new targets for PEG-PLGA NPs. Cell adhesion molecules are a general class of cell surface transmembrane proteins that mediate cell-cell and cell-extracellular matrix adhesion, especially in tumors. The epithelial cell adhesion molecule (EpCAM) is highly expressed in tumors and it helps regulate the epithelial-mesenchymal transition, giving it a key role in the invasion and metastasis of tumor cells; this molecule can bind specifically to EpCAM aptamer on NPs (Fagotto and Aslemarz, 2020). CD44 is also upregulated in several tumor cell types, and it serves as a marker of cancer stem cells; it can bind specifically to hyaluronic acid on NPs (Chen et al., 2018). The arginine-glycine-aspartate (iRGD) peptide on NPs binds with high affinity to the integrin receptor, which is abundantly expressed on certain tumor types; the peptide can then be internalized, taking the NP and its drug cargo inside the target cells (Davoodi and Shafiee, 2022). The folate receptor is overexpressed in certain tumor types such as ovarian cancer and non-small cell lung cancer, and this has been exploited in several appraoches to develop high-affinity folates for targeted cancer treatment (Ledermann et al., 2015), as well as folate conjugates for chemotherapy, photothermal therapy, and diagnostic imaging (Li et al., 2015; Liu et al., 2018). Another study showed that biotin, a safe water-soluble vitamin, can bind strongly to biotin receptors and the surface of pharmaceutical preparations, showing great potential as an active targeting strategy for cancer treatment (Wang et al., 2020). Furthermore, the great demand of tumor cells for iron leads them to overexpress transferrin receptors on their surface, which might provide another strategy for targeted therapy (Luck and Mason, 2013).
In this review, we discuss the formulation principles and properties of PEG-PLGA NPs and summarize the recent advances in their modification and application as drug delivery systems for targeted cancer treatment (Figure 1). The synthesis provided here may guide the development of new antitumor formulations with improved in vivo pharmacokinetics, enhanced passive and active targeting, as well as high drug efficiency for effective precision medicine.
FIGURE 1. Schematic of applications of PEG-PLGA nanoparticles. EPR, enhanced permeability and retention enhanced permeability and retention; PEG, polyethylene glycol; PLGA, poly (lactic acid-co-glycolic acid); siRNA, short interfering RNA.
Due to its great biocompatibility and biodegradability, PLGA has been widely used in the preparation of NPs (Lakkireddy and Bazile, 2016). Although PLGA NPs are good carriers for hydrophilic and hydrophobic drugs, their application is limited due to protein opsonization and rapid clearance by the reticuloendothelial system. Adagen was the first PEGylated protein drug approved by the FDA as a treatment for severe combined immunodeficiency (Suk et al., 2016). When PEG covalently binds to the drug surface, it will block antigen determinants to affect antigen-antibody binding to inhibit the immunoreaction. The immunogenicity of ricin against anti-ricin serum can be reduced through PEG modification, which covers epitopes and receptors involved in immune recognition (Hu et al., 2002). PEGylating a genetically engineered form of alginate lyase significantly reduced its ability to be recognized by antibodies from New Zealand rabbits and humans (Lamppa et al., 2011). Similarly, PEGylating porcine follicle-stimulating hormone protected the hormone from immune recognition (Uchiyama et al., 2010). The PEG surface barrier can also protect the drug from enzymatic degradation and rapid elimination by the kidney, prolonging the half-life of the drug in vivo. PEGylating recombinant human interleukin-11 (IL-11) not only enhanced its pharmacological activity, but also prolonged its retention time in plasma by reducing the liver and kidney clearance of IL-11 (Takagi et al., 2007) (Menkhorst et al., 2009). Modifying PLGA NPs with PEG improves their surface hydrophilicity and prolongs circulation time (Noori Koopaei et al., 2014), giving them substantial promise as drug carriers (Haggag et al., 2018; Dunn et al., 2019). These NPs consist of a PEG shell and a PLGA core that can effectively encapsulate hydrophilic and hydrophobic drugs (Figure 2).
FIGURE 2. Structure of a PEG-PLGA nanoparticle. PEG, polyethylene glycol; PLGA, poly (lactic acid-co-glycolic acid).
Nanoprecipitation and double emulsion-solvent evaporation are the two main methods for the synthesis of PEG-PLGA NPs; they take advantage of the self-assembly of PEG and PLGA at a specific ratio and temperature. Below we describe only the basic synthetic routes and characteristics of the two methods, since we cannot cover the large variability in excipients, component proportions and reaction conditions that have been explored.
Nanoprecipitation is a simple preparation method for NPs with narrow particle size distribution that requires low amounts of surfactant, generates few toxic products, and can be performed on a large scale. For the preparation of PEG-PLGA NPs, PEG and PLGA are dissolved in a suitable solvent, mainly acetone, and then added to an aqueous phase to complete their self-assembly. The solvent is finally removed by dialysis or volatilization, and PEG-PLGA NPs are collected. Using this method, PEG-PLGA NPs loaded with a manganese (II) complex were prepared and they showed excellent encapsulation and drug-loading efficiency, leading to good therapeutic effect against breast cancer stem cells (Eskandari and Suntharalingam, 2019). In another study, honokiol-loaded NPs prepared by nanoprecipitation were modified to obtain nanocarriers with high-loading capacity, which enhanced anti-breast cancer activity in vitro and in vivo (Haggag et al., 2020). Nevertheless, further research is still needed to clarify how the choice of organic or aqueous phase, encapsulated drug, temperature, pH, and sequence of reagent addition influence drug loading and encapsulation into NPs (Almoustafa et al., 2017).
In the double emulsion-solvent evaporation method, PEG, PLGA and drug are added into an organic solvent (oil phase) to prepare a water-in-oil (W/O) emulsion. The resulting emulsion is then added into a water phase, and the mixture is homogenized by sonication to obtain a W/O/W emulsion (Chen et al., 2019; Shen and TanTai, 2020). Evaporation of the organic solvent followed by filtration yields drug-loaded PEG-PLGA NPs.
Similar to the O/W single emulsion-solvent evaporation method, this approach is used to encapsulate proteins and hydrophilic drugs and limit their diffusion out of the NPs, thereby improving entrapment efficiency and sustained release (Zhang et al., 2014). For instance, this method was used to prepare salidroside-loaded PEG-PLGA NPs with high entrapment efficiency by adjusting the glycolic acid/lactic acid molar ratio and the molecular weight of PLGA. The resulting preparation showed low polydispersity index, high zeta potential, and good release and cytotoxicity properties in vitro, indicating that the behavior of PEG-PLGA NPs strongly depends on composition and choice of raw materials (Fang et al., 2014). Endostar-loaded PEG-PLGA NPs were also prepared by double emulsion-solvent evaporation, and they showed sustained and controlled drug release properties as well as specific tumor targeting ability in vivo (Hu and Zhang, 2010).
Although chemotherapy remains the main treatment approach for cancer, chemotherapeutic drugs suffer from low targeting ability, low cytotoxicity, fast elimination, serious side effects, and high drug resistance. PEG-PLGA NPs have emerged as a novel formulation with great biocompatibility and non-immunogenicity that can improve the solubility, stability, and safety of chemotherapeutic drugs for the treatment of various cancer types (Table 1). For example, paclitaxel (PTX)-loaded PEG-PLGA NPs rapidly prepared by microwave synthesis showed similar cytotoxicity to Taxol (Dunn et al., 2019). Satisfactory pharmacokinetic and pharmacodynamic results have also been reported for docetaxel (DTX)- and PTX-loaded PEG-PLGA NPs (Wei et al., 2013; Noori Koopaei et al., 2014). In another study, 5-fluorouracil (5-FU)-loaded PEG-PLGA NPs improved the encapsulation, controlled release, and efficacy of the drug against solid Ehrlich carcinoma, while reducing the drug’s adverse effects (Haggag et al., 2018). PEG-PLGA NPs encapsulating doxorubicin (DOX) (Nagahama et al., 2015), bendamustine (Khan et al., 2016b), Endostar (Hu and Zhang, 2010), metformin (Faramarzi et al., 2019), and manganese (II) complex (Eskandari and Suntharalingam, 2019) have shown good sustained release and anticancer properties in vitro and in vivo. In addition, PEG-PLGA NPs have been used for the co-delivery of chemotherapeutic drugs, such as 5-FU and chrysin or sorafenib and pigment epithelium-derived factor for colorectal cancer therapy, as well as gefitinib and quercetin for lung cancer treatment (Chen et al., 2019; Khaledi et al., 2020; Shen and TanTai, 2020). The co-loaded NPs demonstrated better sustained release performance, targetability, and tumor growth inhibition than single drug-loaded NPs. These results suggest that PEG-PLGA NPs can be used for the synergistic treatment of tumors, while reducing the frequency of drug administration.
Active TCM substances have attracted increasing attention as antitumor drugs or adjuvant therapy for chemotherapy due to their multi-target ability. However, their short half-life, rapid metabolism, low bioavailability, and poor targeting ability significantly limit their application. Therefore, the encapsulation of such active substances into shells (nanoparticles, liposomes, gels, vesicles, etc.), such as PEG-PLGA NPs, can improve their properties and anticancer efficacy (Table 1). For example, chrysin-loaded PEG-PLGA NPs upregulated miR-34a and showed higher solubility and inhibitory activity than free chrysin against AGS cell growth (Mohammadian et al., 2015). PEG-PLGA NPs co-loaded with chrysin and 5-FU or curcumin also exhibited significant synergistic anticancer effects in colorectal cancer treatment (Bagheri et al., 2018; Khaledi et al., 2020). Similarly, PEG-PLGA NPs carrying both di-indolylmethane and ellagic acid effectively reduced the viability of pancreatic cancer cells and suppressed tumor growth and angiogenesis (Mousa et al., 2020). In addition, PEG-PLGA NPs loaded with ginsenosides showed better oncogene regulation, anticancer synergism, drug uptake, half-life, and safety than the corresponding free drugs (Voruganti et al., 2015). PEG-PLGA NPs co-loaded with drugs and active TCM monomers such as icariin (Alhakamy, 2021), salidroside (Fang et al., 2014), and honokiol (Haggag et al., 2020) have also shown anticancer synergistic effects and sustained release. For instance, lupeol-loaded PEG-PLGA NPs enhanced the sensitivity of hepatocellular cancer to radiotherapy (Xie et al., 2021), which may provide a new research direction for antitumor drug resistance. These results suggest that the encapsulation of existing and newly discovered active TCM substances into PEG-PLGA NPs can promote their application in cancer treatment.
NPs have special structure, chemical properties, and passive targeting, which allow them to encapsulate nonspecific or targeted drugs and TCM. Although NPs can enhance the targeting ability of antitumor drugs due to the ERP effect and passive enhanced permeability, they still lack the ability to target malignant cells (Tuwahatu et al., 2018; Barkat et al., 2021). To promote active targeting, the surface of PEG-PLGA NPs has been modified with various ligands, such as folate, aptamer, transferrin, and hyaluronic acid (Table 2).
TABLE 2. Recent applications of ligand-modified PEG-PLGA nanoparticles as drug carriers for actively targeted cancer therapy.
For example, the 5′-NH2-modified EpCAM aptamer was covalently bound on the surface of DOX-loaded PEG-PLGA NPs, and the modified NPs showed stronger inhibitory activity on tumor growth in nude mice bearing non-small cell lung cancer xenografts and higher cytotoxicity against A549 cells than unmodified NPs (Alibolandi et al., 2015a). In addition, EpCAM aptamer-conjugated PEG-PLGA NPs enhanced the cellular uptake and cytotoxicity of DOX against human breast adenocarcinoma cells (Alibolandi et al., 2015a; Alibolandi et al., 2015b). Conjugation of A10 aptamer to PEG-PLGA NPs loaded with triplex-forming oligonucleotides led to specific targeting of prostate cancer cells and inhibition of tumor growth, and the modified NPs silenced the androgen receptor gene more effectively than unmodified NPs (Jiao et al., 2016).
Transferrin has also been added to drug-loaded NPs due to its non-toxicity, biodegradability, and low expression in most normal tissues. For example, thymoquinone-loaded PEG-PLGA NPs modified with transferrin significantly induced cancer cell apoptosis by regulating the p53/miR-34a/miR-16 axis (Upadhyay et al., 2019). Multidrug-loaded PEG-PLGA NPs decorated with transferrin increased the intracerebral accumulation of the drugs and showed good anti-glioma efficacy in vivo (Zhang et al., 2019).
Since biotin receptors are overexpressed in cancer cells, NPs modified with biotin have been developed. Among them, 15,16-dihydrotanshinone I-loaded PEG-PLGA NPs modified with biotin prevented HeLa cell proliferation by downregulating reactive oxygen species and triggering G2/M phase cycle arrest (Luo et al., 2018). DOX-loaded PEG-PLGA NPs modified with biotin have shown good potential in vitro and in vivo for active, targeted therapy of breast cancer (Singh et al., 2017).
Hyaluronic acid can specifically bind to CD44 molecular receptors, which are involved in regulating specific cell-cell and cell-matrix interactions. Cisplatin-loaded PEG-PLGA NPs modified with hyaluronic acid were internalized to a greater extent than unmodified NPs by human ovarian cancer (SKOV-3) cells overexpressing CD44, and the modified NPs were more toxic against those cells (Alam et al., 2017).
Folate, which is highly expressed in a wide range of tumor cells, was also conjugated to PEG-PLGA NPs, affording nanomaterials with superior cytotoxicity and improved cellular uptake due to the specific binding of folate to the corresponding receptors (Singh et al., 2015). Similarly, folate-decorated PEG-PLGA NPs loaded with sorafenib showed high cellular uptake, antiproliferation activity and antitumor effects against BEL7402 cancer cells (Li et al., 2015).
PEG-PLGA NPs decorated with glycyrrhetinic acid were able to concentrate the drug artesunate in liver cancer cells (Pan et al., 2020). 5-FU-loaded PEG-PLGA NPs modified with chondroitin bound to the chondroitin sulfate receptors overexpressed on various tumor cell types, leading to higher cytotoxicity and less hemolytic effects than unmodified NPs (Yadav et al., 2010).
Targeting the tumor microenvironment has also emerged as an effective strategy for cancer treatment. For example, alendronate-loaded PEG-PLGA NPs were modified with hydroxyapatite, an abundant mineral in bone tissues with high affinity for bisphosphonates, and the formulation accumulated in bone tumors in vivo (Swami et al., 2014). PEG-PLGA NPs that were co-loaded with sorafenib and metapristone and were conjugated with LFC131, a peptide inhibitor of CXCR4 (Zheng et al., 2019), showed promise in bypassing CXCR4-mediated resistance of hepatocellular carcinoma tumor cells to the widely used drug sorafenib.
Recent studies have investigated the role of modified PEG-PLGA NPs in photoacoustic imaging and photothermal tumor therapy. For example, folate-conjugated PEG-PLGA NPs were co-loaded with indocyanine green, perfluorohexane, and PTX to prepare NPs that could be simultaneously used for photoacoustic and enhanced ultrasound echo imaging as well as for active targeting of tumors overexpressing folate receptors (Liu et al., 2018). PEG-PLGA NPs that were loaded with pH-sensitive croconaine815 and decorated with iRGD showed strong photoacoustic signal enhancement and effectively inhibited tumor growth, serving as a novel strategy for in vivo multiplexed photoacoustic imaging and pH-responsive photothermal therapy (Li S. et al., 2021). In addition, PTX-loaded PEG-PLGA NPs that were decorated with the peptides Pep-1 (CGEMGWVRC) and CGKRK (Cys-Gly-Lys-Arg-Lys) enhanced the antiglioma efficacy of PTX by inhibiting angiogenesis and killing cancer cells (Lv et al., 2016).
Cancer gene therapy uses nucleic acids, oligopeptides and proteins to treat tumors by regulating the expression of related genes. However, these drugs cannot be extensively used in clinical practice due to their fast degradation and easy elimination in the blood (Kim et al., 2016). To address these problems, polymer NPs such as PEG-PLGA NPs have been extensively studied as drug carriers due to their unique size, simple modification, good biocompatibility, stability, and low toxicity (Xin et al., 2017). For example, verapamil-modified PEG-PLGA NPs loaded with SN38 enhanced the expression of apoptosis-related genes (BAX/BCL2) and delayed drug resistance in colorectal cancer cells (Nagheh et al., 2017). PEG-PLGA NPs containing a novel peptide inhibiting the protein “Ras protein-regulator of chromosome condensation 1” may be able to inhibit breast cancer metastasis (Haggag et al., 2019). PEG-PLGA NPs co-loaded with DTX and short interfering RNA targeting the oncogene TUBB3 or co-loaded with sorafenib and metapristone targeting the SDF-1/CXCR4 axis have also shown promise against oncogenes in hepatocellular carcinoma (Zheng et al., 2019; Conte et al., 2021). PEG-PLGA NPs that were loaded with a “thymidine kinase–p53–nitroreductase” triple therapeutic gene and decorated with polyethylenimine were able to inhibit growth of hepatocellular carcinoma tumors (Sukumar et al., 2020).
All formulations developed for biomedical or clinical use must be non-toxic and comply with the relevant biosafety regulations, while showing good biocompatibility, especially for intravenous or intraperitoneal injection. Although PLGA and PEG have been approved by the FDA as safe and biodegradable, few studies have explored the safety of PEG-PLGA NPs in vivo for further clinical application. For example, blank PEG-PLGA NPs showed negligible inhibitory effects on the growth of human colon adenocarcinoma SW-480 cells, confirming their good biocompatibility (Dimchevska et al., 2017). PEG-PLGA NPs loaded with bendamustine led to nearly 4-fold lower hemolysis than NPs without the PEG-PLGA matrix, also indicating good biocompatibility (Khan et al., 2016b). In addition, no significant cytotoxicity was observed for blank PEG-PLGA NPs toward HeLa cells (Luo et al., 2018), while the intraperitoneal injection of blank or honokiol-loaded PEG-PLGA NPs did not cause substantial damage to the liver or kidney of mice with breast cancer tumors (Haggag et al., 2020), further indicating the safety of these nanocarriers in vivo. Similarly, PEG-PLGA NPs loaded with the anticancer ginsenoside 25-OCH3-PPD did not cause histopathology in the liver, kidneys, lungs, spleen, heart, or brain of mice bearing PC3 xenograft tumors (Voruganti et al., 2015), while estradiol-decorated DTX-loaded PEG-PLGA NPs showed negligible cytotoxicity in MCF-7 cells and minimal hepatotoxicity in mice (Jain et al., 2015). A recent study analyzed the plasma activation levels of complement C3 induced by the intravenous injection of PEG-PLGA NPs conjugated with anti-cathepsin K antibodies. The results revealed no significant upregulation of complement C3 after treatment with the modified NPs, suggesting that they not induce an immune response (Camardo et al., 2020). DOX-loaded PEG-PLGA NPs decorated with biotin showed low hemolytic activity and cytotoxicity and proved to be safe relative to free DOX (Singh et al., 2017). Odorranalectin-modified PEG-PLGA NPs were found to be non-toxic to human lung adenocarcinoma Calu-3 cells and showed negligible toxicity and immunogenicity in the nasal cavity in toads and rats (Wen et al., 2011). PEG-PLGA NPs modified with both Pep-1 and CGKRK peptides were also shown to be non-toxic to major organs in mice (Lv et al., 2016).
Nevertheless, the influence of different formulation ratios, molecular weights, and synthesis methods on the safety of PEG-PLGA NPs has not been adequately explored. It is important to analyze the relationship between the toxicity of NP carriers and their physical characteristics, including polydispersity index, zeta potential, entrapment efficiency, and morphology. In fact, the entire process from raw materials to synthesis and modification of drug-loaded PEG-PLGA NPs should be rigorously optimized to maximize safety before clinical trials. Ultimately, uniform guidelines for synthesizing and formulating PEG-PLGA NPs are needed in order to ensure their efficacy as drug carriers for targeted cancer treatment.
The rapid growth of nanotechnology has led to the emergence of many novel therapeutic methods such as nanodrug delivery systems. The present review shows that amphiphilic block copolymer PEG-PLGA NPs can be safely used as drug nanocarriers that show sustained release properties as well as improved drug bioavailability and stability in vivo. PEG-PLGA NPs modified with ligands can target specific receptors on the tumor surface, enhancing tumor targeting. However, the biocompatibility, toxicity, and safety of these nanocarriers require further research to guarantee their clinical application. New cancer-specific target molecules are constantly being discovered, and studies should continue to explore how to modify NPs in order to recognize tumors. This approach may create new possibilities for precision anti-cancer treatment and diagnostic imaging.
ML, LL, DZ, and XW contributed to conception and design of the study. DZ, LL, JW, HZ, ZZ, and GX organized the database. ML, LL, DZ, and XW wrote the first draft of the manuscript. All authors contributed to manuscript revision, read, and approved the submitted version.
This work was supported by Key Project from the Department of Science and Technology, Sichuan Province (2019YFS0116). The present study was also supported by Joint Project from Luzhou City and Southwest Medical University (20ykdz0008) and scientific and technological achievements transformation project from Southwest Medical University (05/00180006).
The authors declare that the research was conducted in the absence of any commercial or financial relationships that could be construed as a potential conflict of interest.
All claims expressed in this article are solely those of the authors and do not necessarily represent those of their affiliated organizations, or those of the publisher, the editors and the reviewers. Any product that may be evaluated in this article, or claim that may be made by its manufacturer, is not guaranteed or endorsed by the publisher.
The Supplementary Material for this article can be found online at: https://www.frontiersin.org/articles/10.3389/fphar.2022.990505/full#supplementary-material
Alam, N., Koul, M., Mintoo, M. J., Khare, V., Gupta, R., Rawat, N., et al. (2017). Development and characterization of hyaluronic acid modified PLGA based nanoparticles for improved efficacy of cisplatin in solid tumor. Biomed. Pharmacother. = Biomedecine Pharmacother. 95, 856–864. doi:10.1016/j.biopha.2017.08.108
Alhakamy, N. A. (2021). Development and evaluation of icariin-loaded PLGA-PEG nanoparticles for potentiation the proapoptotic activity in pancreatic cancer cells. AAPS PharmSciTech 22 (8), 252. doi:10.1208/s12249-021-02111-w
Alibolandi, M., Ramezani, M., Abnous, K., Sadeghi, F., Atyabi, F., Asouri, M., et al. (2015a). In vitro and in vivo evaluation of therapy targeting epithelial-cell adhesion-molecule aptamers for non-small cell lung cancer. J. Control. Release 209, 88–100. doi:10.1016/j.jconrel.2015.04.026
Alibolandi, M., Ramezani, M., Sadeghi, F., Abnous, K., and Hadizadeh, F. (2015b). Epithelial cell adhesion molecule aptamer conjugated PEG-PLGA nanopolymersomes for targeted delivery of doxorubicin to human breast adenocarcinoma cell line in vitro. Int. J. Pharm. 479 (1), 241–251. doi:10.1016/j.ijpharm.2014.12.035
Almoustafa, H. A., Alshawsh, M. A., and Chik, Z. (2017). Technical aspects of preparing PEG-PLGA nanoparticles as carrier for chemotherapeutic agents by nanoprecipitation method. Int. J. Pharm. 533 (1), 275–284. doi:10.1016/j.ijpharm.2017.09.054
Bagheri, R., Sanaat, Z., and Zarghami, N. (2018). Synergistic effect of free and nano-encapsulated chrysin-curcumin on inhibition of hTERT gene expression in SW480 colorectal cancer cell line. Drug Res. 68 (6), 335–343. doi:10.1055/s-0043-121338
Barkat, A., Beg, S., Panda, S. K., S Alharbi, K., Rahman, M., and Ahmed, F. J. (2021). Functionalized mesoporous silica nanoparticles in anticancer therapeutics. Semin. Cancer Biol. 69, 365–375. doi:10.1016/j.semcancer.2019.08.022
Cai, M., Chen, G., Qin, L., Qu, C., Dong, X., Ni, J., et al. (2020). Metal organic frameworks as drug targeting delivery vehicles in the treatment of cancer. Pharmaceutics 12 (3). doi:10.3390/pharmaceutics12030232
Camardo, A., Carney, S., and Ramamurthi, A. (2020). Assessing the targeting and fate of cathepsin k antibody-modified nanoparticles in a rat abdominal aortic aneurysm model. Acta Biomater. 112, 225–233. doi:10.1016/j.actbio.2020.05.037
Chen, C., Zhao, S., Karnad, A., and Freeman, J. W. (2018). The biology and role of CD44 in cancer progression: Therapeutic implications. J. Hematol. Oncol. 11 (1), 64. doi:10.1186/s13045-018-0605-5
Chen, Y., Li, N., Xu, B., Wu, M., Yan, X., Zhong, L., et al. (2019). Polymer-based nanoparticles for chemo/gene-therapy: Evaluation its therapeutic efficacy and toxicity against colorectal carcinoma. Biomed. Pharmacother. = Biomedecine Pharmacother. 118, 109257. doi:10.1016/j.biopha.2019.109257
Conte, C., Monteiro, P. F., Gurnani, P., Stolnik, S., Ungaro, F., Quaglia, F., et al. (2021). Multi-component bioresponsive nanoparticles for synchronous delivery of docetaxel and TUBB3 siRNA to lung cancer cells. Nanoscale 13 (26), 11414–11426. doi:10.1039/d1nr02179f
Ciobanasu, C. (2021). Peptides-based therapy and diagnosis. Strategies for non-invasive therapies in cancer. J. Drug Targeting 29 (10), 1063–1079. doi:10.1080/1061186X.2021.1906885
Cui, Y., Li, X., Zeljic, K., Shan, S., Qiu, Z., and Wang, Z. (2019). Effect of PEGylated magnetic PLGA-PEI nanoparticles on primary hippocampal neurons: Reduced nanoneurotoxicity and enhanced transfection efficiency with magnetofection. ACS Appl. Mat. Interfaces 11 (41), 38190–38204. doi:10.1021/acsami.9b15014
Davoodi, Z., and Shafiee, F. (2022). Internalizing RGD, a great motif for targeted peptide and protein delivery: A review article. Drug Deliv. Transl. Res. doi:10.1007/s13346-022-01116-7
Dimchevska, S., Geskovski, N., Koliqi, R., Matevska-Geskovska, N., Gomez Vallejo, V., Szczupak, B., et al. (2017). Efficacy assessment of self-assembled PLGA-PEG-PLGA nanoparticles: Correlation of nano-bio interface interactions, biodistribution, internalization and gene expression studies. Int. J. Pharm. 533 (2), 389–401. doi:10.1016/j.ijpharm.2017.05.054
Dunn, S. S., Luft, J. C., and Parrott, M. C. (2019). Zapped assembly of polymeric (ZAP) nanoparticles for anti-cancer drug delivery. Nanoscale 11 (4), 1847–1855. doi:10.1039/c8nr09944h
Eskandari, A., and Suntharalingam, K. (2019). A reactive oxygen species-generating, cancer stem cell-potent manganese(ii) complex and its encapsulation into polymeric nanoparticles. Chem. Sci. 10 (33), 7792–7800. doi:10.1039/c9sc01275c
Fagotto, F., and Aslemarz, A. (2020). EpCAM cellular functions in adhesion and migration, and potential impact on invasion: A critical review. Biochim. Biophys. Acta. Rev. Cancer 1874 (2), 188436. doi:10.1016/j.bbcan.2020.188436
Fang, D.-L., Chen, Y., Xu, B., Ren, K., He, Z.-Y., He, L.-L., et al. (2014). Development of lipid-shell and polymer core nanoparticles with water-soluble salidroside for anti-cancer therapy. Int. J. Mol. Sci. 15 (3), 3373–3388. doi:10.3390/ijms15033373
Faramarzi, L., Dadashpour, M., Sadeghzadeh, H., Mahdavi, M., and Zarghami, N. (2019). Enhanced anti-proliferative and pro-apoptotic effects of metformin encapsulated PLGA-PEG nanoparticles on SKOV3 human ovarian carcinoma cells. Artif. Cells Nanomed. Biotechnol. 47 (1), 737–746. doi:10.1080/21691401.2019.1573737
Firer, M. A., and Gellerman, G. (2012). Targeted drug delivery for cancer therapy: The other side of antibodies. J. Hematology and Oncology 5, 70. doi:10.1186/1756-8722-5-70
Haggag, Y. A., Ibrahim, R. R., and Hafiz, A. A. (2020). Design, formulation and in vivo evaluation of novel honokiol-loaded PEGylated PLGA nanocapsules for treatment of breast cancer. Int. J. Nanomedicine 15, 1625–1642. doi:10.2147/IJN.S241428
Haggag, Y. A., Matchett, K. B., Falconer, R. A., Isreb, M., Jones, J., Faheem, A., et al. (2019). Novel ran-RCC1 inhibitory peptide-loaded nanoparticles have anti-cancer efficacy in vitro and in vivo. Cancers 11 (2), E222. doi:10.3390/cancers11020222
Haggag, Y. A., Osman, M. A., El-Gizawy, S. A., Goda, A. E., Shamloula, M. M., Faheem, A. M., et al. (2018). Polymeric nano-encapsulation of 5-fluorouracil enhances anti-cancer activity and ameliorates side effects in solid Ehrlich Carcinoma-bearing mice. Biomed. Pharmacother. = Biomedecine Pharmacother. 105, 215–224. doi:10.1016/j.biopha.2018.05.124
Hu, R.-G., Zhai, Q.-W., He, W.-J., Mei, L., and Liu, W.-Y. (2002). Bioactivities of ricin retained and its immunoreactivity to anti-ricin polyclonal antibodies alleviated through pegylation. Int. J. Biochem. Cell Biol. 34 (4), 396–402. doi:10.1016/s1357-2725(01)00128-5
Hu, S., and Zhang, Y. (2010). Endostar-loaded PEG-PLGA nanoparticles: In vitro and in vivo evaluation. Int. J. Nanomedicine 5, 1039–1048. doi:10.2147/IJN.S14753
Hu, Y., Hoerle, R., Ehrich, M., and Zhang, C. (2015). Engineering the lipid layer of lipid-PLGA hybrid nanoparticles for enhanced in vitro cellular uptake and improved stability. Acta Biomater. 28, 149–159. doi:10.1016/j.actbio.2015.09.032
Jain, S., Spandana, G., Agrawal, A. K., Kushwah, V., and Thanki, K. (2015). Enhanced antitumor efficacy and reduced toxicity of docetaxel loaded estradiol functionalized stealth polymeric nanoparticles. Mol. Pharm. 12 (11), 3871–3884. doi:10.1021/acs.molpharmaceut.5b00281
Jiao, J., Zou, Q., Zou, M. H., Guo, R. M., Zhu, S., and Zhang, Y. (2016). Aptamer-modified PLGA nanoparticle delivery of triplex forming oligonucleotide for targeted prostate cancer therapy. Neoplasma 63 (4), 569–575. doi:10.4149/neo_2016_410
Karami, A., Mohamed, O., Ahmed, A., Husseini, G. A., and Sabouni, R. (2021). Recent advances in metal-organic frameworks as anticancer drug delivery systems: A review. Anti-cancer Agents In Medicinal Chemistry 21 (18), 2487–2504. doi:10.2174/1871520621666210119093844
Khaledi, S., Jafari, S., Hamidi, S., Molavi, O., and Davaran, S. (2020). Preparation and characterization of PLGA-PEG-PLGA polymeric nanoparticles for co-delivery of 5-Fluorouracil and Chrysin. J. Biomater. Sci. Polym. Ed. 31 (9), 1107–1126. doi:10.1080/09205063.2020.1743946
Khan, I., Gothwal, A., Sharma, A. K., Kesharwani, P., Gupta, L., Iyer, A. K., et al. (2016a). PLGA nanoparticles and their versatile role in anticancer drug delivery. Crit. Rev. Ther. Drug Carr. Syst. 33 (2), 159–193. doi:10.1615/CritRevTherDrugCarrierSyst.2016015273
Khan, I., Gothwal, A., Sharma, A. K., Qayum, A., Singh, S. K., and Gupta, U. (2016b). Biodegradable nano-architectural PEGylated approach for the improved stability and anticancer efficacy of bendamustine. Int. J. Biol. Macromol. 92, 1242–1251. doi:10.1016/j.ijbiomac.2016.08.004
Kim, J., Kim, J., Jeong, C., and Kim, W. J. (2016). Synergistic nanomedicine by combined gene and photothermal therapy. Adv. Drug Deliv. Rev. 98, 99–112. doi:10.1016/j.addr.2015.12.018
Lakkireddy, H. R., and Bazile, D. (2016). Building the design, translation and development principles of polymeric nanomedicines using the case of clinically advanced poly(lactide(glycolide))-poly(ethylene glycol) nanotechnology as a model: An industrial viewpoint. Adv. Drug Deliv. Rev. 107, 289–332. doi:10.1016/j.addr.2016.08.012
Lamppa, J. W., Ackerman, M. E., Lai, J. I., Scanlon, T. C., and Griswold, K. E. (2011). Genetically engineered alginate lyase-PEG conjugates exhibit enhanced catalytic function and reduced immunoreactivity. PloS One 6 (2), e17042. doi:10.1371/journal.pone.0017042
Ledermann, J. A., Canevari, S., and Thigpen, T. (2015). Targeting the folate receptor: Diagnostic and therapeutic approaches to personalize cancer treatments. Ann. Oncol. 26 (10), 2034–2043. doi:10.1093/annonc/mdv250
Levêque, D., and Becker, G. (2019). The role of therapeutic drug monitoring in the management of safety of anticancer agents: A focus on 3 cytotoxics. Expert Opin. Drug Saf. 18 (11), 1009–1015. doi:10.1080/14740338.2019.1662395
Li, P. Y., Bearoff, F., Zhu, P., Fan, Z., Zhu, Y., Fan, M., et al. (2021a). PEGylation enables subcutaneously administered nanoparticles to induce antigen-specific immune tolerance. J. Control. Release 331, 164–175. doi:10.1016/j.jconrel.2021.01.013
Li, S., Lui, K.-H., Li, X., Fang, X., Lo, W.-S., Gu, Y.-J., et al. (2021b). pH-triggered poly(ethylene glycol)-Poly(lactic acid/glycolic acid)/croconaine nanoparticles-assisted multiplexed photoacoustic imaging and enhanced photothermal cancer therapy. ACS Appl. Bio Mat. 4 (5), 4152–4164. doi:10.1021/acsabm.0c01578
Li, Y.-J., Dong, M., Kong, F.-M., and Zhou, J.-P. (2015). Folate-decorated anticancer drug and magnetic nanoparticles encapsulated polymeric carrier for liver cancer therapeutics. Int. J. Pharm. 489 (1-2), 83–90. doi:10.1016/j.ijpharm.2015.04.028
Liu, F., Chen, Y., Li, Y., Guo, Y., Cao, Y., Li, P., et al. (2018). Folate-receptor-targeted laser-activable poly(lactide-co-glycolic acid) nanoparticles loaded with paclitaxel/indocyanine green for photoacoustic/ultrasound imaging and chemo/photothermal therap. Int. J. Nanomedicine 13, 5139–5158. doi:10.2147/IJN.S167043
Luck, A. N., and Mason, A. B. (2013). Structure and dynamics of drug carriers and their interaction with cellular receptors: Focus on serum transferrin. Adv. Drug Deliv. Rev. 65 (8), 1012–1019. doi:10.1016/j.addr.2012.11.001
Luo, J., Meng, X., Su, J., Ma, H., Wang, W., Fang, L., et al. (2018). Biotin-modified polylactic- co-glycolic acid nanoparticles with improved antiproliferative activity of 15, 16-dihydrotanshinone I in human cervical cancer cells. J. Agric. Food Chem. 66 (35), 9219–9230. doi:10.1021/acs.jafc.8b02698
Lv, L., Jiang, Y., Liu, X., Wang, B., Lv, W., Zhao, Y., et al. (2016). Enhanced antiglioblastoma efficacy of neovasculature and glioma cells dual targeted nanoparticles. Mol. Pharm. 13 (10), 3506–3517. doi:10.1021/acs.molpharmaceut.6b00523
Menkhorst, E., Salamonsen, L., Robb, L., and Dimitriadis, E. (2009). IL11 antagonist inhibits uterine stromal differentiation, causing pregnancy failure in mice. Biol. Reprod. 80 (5), 920–927. doi:10.1095/biolreprod.108.073601
Mitchell, M. J., Billingsley, M. M., Haley, R. M., Wechsler, M. E., Peppas, N. A., and Langer, R. (2021). Engineering precision nanoparticles for drug delivery. Nat. Rev. Drug Discov. 20 (2), 101–124. doi:10.1038/s41573-020-0090-8
Mohammadian, F., Abhari, A., Dariushnejad, H., Zarghami, F., Nikanfar, A., Pilehvar-Soltanahmadi, Y., et al. (2015). Upregulation of mir-34a in AGS gastric cancer cells by a PLGA-PEG-PLGA chrysin nano formulation. Asian pac. J. Cancer Prev. 16 (18), 8259–8263. doi:10.7314/apjcp.2015.16.18.8259
Mousa, D. S., El-Far, A. H., Saddiq, A. A., Sudha, T., and Mousa, S. A. (2020). Nanoformulated bioactive compounds derived from different natural products combat pancreatic cancer cell proliferation. Int. J. Nanomedicine 15, 2259–2268. doi:10.2147/IJN.S238256
Nagahama, K., Kawano, D., Oyama, N., Takemoto, A., Kumano, T., and Kawakami, J. (2015). Self-assembling polymer micelle/clay nanodisk/doxorubicin hybrid injectable gels for safe and efficient focal treatment of cancer. Biomacromolecules 16 (3), 880–889. doi:10.1021/bm5017805
Nagheh, Z., Irani, S., Mirfakhraie, R., and Dinarvand, R. (2017). SN38-PEG-PLGA-verapamil nanoparticles inhibit proliferation and downregulate drug transporter ABCG2 gene expression in colorectal cancer cells. Prog. Biomater. 6 (4), 137–145. doi:10.1007/s40204-017-0073-y
Noori Koopaei, M., Khoshayand, M. R., Mostafavi, S. H., Amini, M., Khorramizadeh, M. R., Jeddi Tehrani, M., et al. (2014). Docetaxel loaded PEG-PLGA nanoparticles: Optimized drug loading, in-vitro cytotoxicity and in-vivo antitumor effect. Iran. J. Pharm. Res. 13 (3), 819–833. doi:10.22037/IJPR.2014.1533
Pan, X., Liu, S., Ju, L., Xi, J., He, R., Zhao, Y., et al. (2020). Preparation, evaluation, and in vitro cytotoxicity studies of artesunate-loaded glycyrrhetinic acid decorated PEG-PLGA nanoparticles. Drug Dev. Ind. Pharm. 46 (11), 1889–1897. doi:10.1080/03639045.2020.1825475
Shen, Y., and TanTai, J. (2020). Co-delivery anticancer drug nanoparticles for synergistic therapy against lung cancer cells. Drug Des. devel. Ther. 14, 4503–4510. doi:10.2147/DDDT.S275123
Singh, R., Kesharwani, P., Mehra, N. K., Singh, S., Banerjee, S., and Jain, N. K. (2015). Development and characterization of folate anchored Saquinavir entrapped PLGA nanoparticles for anti-tumor activity. Drug Dev. Ind. Pharm. 41 (11), 1888–1901. doi:10.3109/03639045.2015.1019355
Singh, Y., Durga Rao Viswanadham, K. K., Kumar Jajoriya, A., Meher, J. G., Raval, K., Jaiswal, S., et al. (2017). Click biotinylation of PLGA template for biotin receptor oriented delivery of doxorubicin hydrochloride in 4T1 cell-induced breast cancer. Mol. Pharm. 14 (8), 2749–2765. doi:10.1021/acs.molpharmaceut.7b00310
Suk, J. S., Xu, Q., Kim, N., Hanes, J., and Ensign, L. M. (2016). PEGylation as a strategy for improving nanoparticle-based drug and gene delivery. Adv. Drug Deliv. Rev. 99, 28–51. doi:10.1016/j.addr.2015.09.012
Sukumar, U. K., Rajendran, J. C. B., Gambhir, S. S., Massoud, T. F., and Paulmurugan, R. (2020). SP94-Targeted triblock copolymer nanoparticle delivers thymidine kinase-p53-nitroreductase triple therapeutic gene and restores anticancer function against hepatocellular carcinoma in vivo. ACS Appl. Mat. Interfaces 12 (10), 11307–11319. doi:10.1021/acsami.9b20071
Swami, A., Reagan, M. R., Basto, P., Mishima, Y., Kamaly, N., Glavey, S., et al. (2014). Engineered nanomedicine for myeloma and bone microenvironment targeting. Proc. Natl. Acad. Sci. U. S. A. 111 (28), 10287–10292. doi:10.1073/pnas.1401337111
Takagi, A., Yamashita, N., Yoshioka, T., Takaishi, Y., Sano, K., Yamaguchi, H., et al. (2007). Enhanced pharmacological activity of recombinant human interleukin-11 (rhIL11) by chemical modification with polyethylene glycol. J. Control. Release 119 (3), 271–278. doi:10.1016/j.jconrel.2007.03.009
Tuwahatu, C. A., Yeung, C. C., Lam, Y. W., and Roy, V. A. L. (2018). The molecularly imprinted polymer essentials: Curation of anticancer, ophthalmic, and projected gene therapy drug delivery systems. J. Control. Release 287, 24–34. doi:10.1016/j.jconrel.2018.08.023
Uchiyama, Y., Hosoe, M., Sato, T., and Takahashi, T. (2010). Biological and immunological characteristics of porcine follicle-stimulating hormone chemically modified with a polyethylene glycol derivative. Vet. J. 184 (2), 208–211. doi:10.1016/j.tvjl.2009.02.006
Upadhyay, P., Sarker, S., Ghosh, A., Gupta, P., Das, S., Ahir, M., et al. (2019). Transferrin-decorated thymoquinone-loaded PEG-PLGA nanoparticles exhibit anticarcinogenic effect in non-small cell lung carcinoma via the modulation of miR-34a and miR-16. Biomater. Sci. 7 (10), 4325–4344. doi:10.1039/c9bm00912d
Voruganti, S., Qin, J.-J., Sarkar, S., Nag, S., Walbi, I. A., Wang, S., et al. (2015). Oral nano-delivery of anticancer ginsenoside 25-OCH3-PPD, a natural inhibitor of the MDM2 oncogene: Nanoparticle preparation, characterization, in vitro and in vivo anti-prostate cancer activity, and mechanisms of action. Oncotarget 6 (25), 21379–21394. doi:10.18632/oncotarget.4091
Wang, Y., van Steenbergen, M. J., Beztsinna, N., Shi, Y., Lammers, T., van Nostrum, C. F., et al. (2020). Biotin-decorated all-HPMA polymeric micelles for paclitaxel delivery. J. Control. Release 328, 970–984. doi:10.1016/j.jconrel.2020.09.013
Wei, J., Wang, H., Zhu, M., Ding, D., Li, D., Yin, Z., et al. (2013). Janus nanogels of PEGylated Taxol and PLGA-PEG-PLGA copolymer for cancer therapy. Nanoscale 5 (20), 9902–9907. doi:10.1039/c3nr02937a
Wen, Z., Yan, Z., He, R., Pang, Z., Guo, L., Qian, Y., et al. (2011). Brain targeting and toxicity study of odorranalectin-conjugated nanoparticles following intranasal administration. Drug Deliv. 18 (8), 555–561. doi:10.3109/10717544.2011.596583
Wiśniewski, K., Jozwik, M., and Wojtkiewicz, J. (2020). Cancer prevention by natural products introduced into the diet-selected cyclitols. Int. J. Mol. Sci. 21 (23), E8988. doi:10.3390/ijms21238988
Xie, Y., Liu, C., Zhou, S., Wang, Q., and Tang, X. (2021). Lupeol-loaded nanoparticles enhance the radiosensitivity of hepatocellular carcinoma by inhibiting the hyperactivation in raf/mitogen-activated protein kinase and phospatidylinositol-3 kinase/mTOR pathways. J. Biomed. Nanotechnol. 17 (11), 2247–2258. doi:10.1166/jbn.2021.3194
Xin, Y., Huang, M., Guo, W. W., Huang, Q., Zhang, L. Z., and Jiang, G. (2017). Nano-based delivery of RNAi in cancer therapy. Mol. Cancer 16 (1), 134. doi:10.1186/s12943-017-0683-y
Xu, S., Yang, F., Zhou, X., Zhuang, Y., Liu, B., Mu, Y., et al. (2015). Uniform PEGylated PLGA microcapsules with embedded Fe3O4 nanoparticles for US/MR dual-modality imaging. ACS Appl. Mat. Interfaces 7 (36), 20460–20468. doi:10.1021/acsami.5b06594
Yadav, A. K., Agarwal, A., Jain, S., Mishra, A. K., Bid, H., Rai, G., et al. (2010). Chondroitin sulphate decorated nanoparticulate carriers of 5-fluorouracil: Development and in vitro characterization. J. Biomed. Nanotechnol. 6 (4), 340–350. doi:10.1166/jbn.2010.1118
Zhang, K., Tang, X., Zhang, J., Lu, W., Lin, X., Zhang, Y., et al. (2014). PEG-PLGA copolymers: Their structure and structure-influenced drug delivery applications. J. Control. Release 183, 77–86. doi:10.1016/j.jconrel.2014.03.026
Zhang, X., Zhao, L., Zhai, G., Ji, J., and Liu, A. (2019). Multifunctional polyethylene glycol (PEG)-Poly (lactic-Co-glycolic acid) (PLGA)-Based nanoparticles loading doxorubicin and tetrahydrocurcumin for combined chemoradiotherapy of glioma. Med. Sci. Monit. 25, 9737–9751. doi:10.12659/MSM.918899
Keywords: PEG, PLGA, nanoparticles, antitumor therapy, targeted modification, drug delivery
Citation: Zhang D, Liu L, Wang J, Zhang H, Zhang Z, Xing G, Wang X and Liu M (2022) Drug-loaded PEG-PLGA nanoparticles for cancer treatment. Front. Pharmacol. 13:990505. doi: 10.3389/fphar.2022.990505
Received: 10 July 2022; Accepted: 27 July 2022;
Published: 19 August 2022.
Edited by:
Li Liu, University of Texas Southwestern Medical Center, United StatesReviewed by:
Jinfang Zhu, Xinjiang Agricultural University, ChinaCopyright © 2022 Zhang, Liu, Wang, Zhang, Zhang, Xing, Wang and Liu. This is an open-access article distributed under the terms of the Creative Commons Attribution License (CC BY). The use, distribution or reproduction in other forums is permitted, provided the original author(s) and the copyright owner(s) are credited and that the original publication in this journal is cited, in accordance with accepted academic practice. No use, distribution or reproduction is permitted which does not comply with these terms.
*Correspondence: Xuan Wang, MTAxODU2Mjk5M0BxcS5jb20=; Minghua Liu, OTkwNTM4MDhAcXEuY29t
†These authors have contributed equally to this work and share first authorship
Disclaimer: All claims expressed in this article are solely those of the authors and do not necessarily represent those of their affiliated organizations, or those of the publisher, the editors and the reviewers. Any product that may be evaluated in this article or claim that may be made by its manufacturer is not guaranteed or endorsed by the publisher.
Research integrity at Frontiers
Learn more about the work of our research integrity team to safeguard the quality of each article we publish.