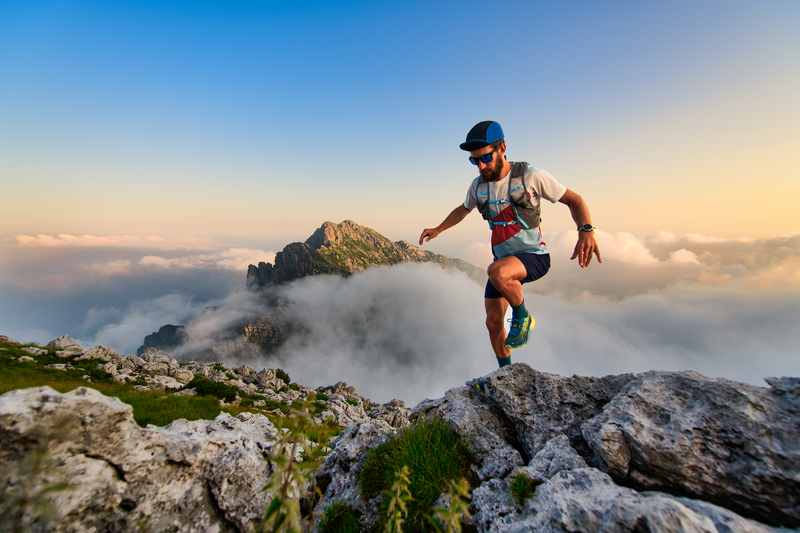
95% of researchers rate our articles as excellent or good
Learn more about the work of our research integrity team to safeguard the quality of each article we publish.
Find out more
BRIEF RESEARCH REPORT article
Front. Pharmacol. , 19 September 2022
Sec. Experimental Pharmacology and Drug Discovery
Volume 13 - 2022 | https://doi.org/10.3389/fphar.2022.988335
This article is part of the Research Topic Drug Application and Delivery Targeting Glycolysis-Associated Diseases: Novel Insights and Mechanism View all 4 articles
Lactoferrin (LF) is an iron binding glycoprotein of the transferrin family with a wide spectrum of biological effects, including anti-cancer activity. However, the detailed molecular mechanisms of anti-cancer activity of LF have not been fully determined. In this study, we tried to clarify cytotoxic functions of LF on various cell lines under hypoxic conditions and elucidate those molecular mechanisms. Cytotoxic activity of LF on cell lines was found to have a range of sensitivities. Hypoxia decreased sensitivity to LF in KD (lip fibroblast) but increased that in HSC2 (oral squamous cell carcinoma). Expression analyses further revealed that LF treatments increased hypoxic HIF-1α, -2α and p53 proteins in KD but attenuated them in HSC2 cells, and decreased HIF-1 target gene, DEC2, in KD but increased it in HSC2, suggesting a possible relationship between LF-modified DEC2 expression and HIF-α protein. MTT assay strikingly demonstrated that cells expressing mutant-type p53 (MT5) were more sensitive to LF than control HepG2 (hepatoma), suggesting an important role of the p53 signal. Knock-down of TP53 (p53 gene) interestingly reduced sensitivity to LF in HepG2, suggesting that p53 may be a target of LF cytotoxic activity. Further analyses with a ferroptosis promoter or inhibitor demonstrated that LF increased ACSL4 in hypoxic MT5, suggesting LF-induced ferroptosis in cells expressing mutant-type p53. In conclusion, hypoxia was found to regulate cytotoxic activities of LF differently among various cell lines, possibly through the p53 signaling pathway. LF further appeared to regulate ferroptosis through a modification of ACSL4 expression.
Lactoferrin (LF) is an 80-kD iron binding glycoprotein of the transferrin family with a wide spectrum of biological effects, including anti-bacterial, anti-inflammatory, immunomodulatory, and anti-cancer activities (Rodrigues et al., 2009; Kruzel et al., 2017; Kell et al., 2020). LF is found in various fluids secreted from glandular systems, including tears, perspiration, and milk, so it is generally considered to be non-toxic (Iglesias-Figueroa et al., 2019). Bovine lactoferrin (bLF) is now approved as a nutritional product by the Therapeutic Goods Administration (TGA, Australia), the Food and Drug Administration (FDA, United States), and the European Food Safety Authority (EFSA). Purified bLF has been further proven in multiple mouse models to enhance production of interferon-γ (IFN-γ), caspase-1, and interleukin-18 (IL-18), as well as lead to an increase of immune cell numbers (Iigo et al., 2004; Takakura et al., 2006; Siqueiros-Cendón et al., 2014). bLF has been also reported to inhibit colon, esophagus, lung, and bladder carcinogenesis in rats when administered orally in the post-initiation stage, suggesting it as a potential therapeutic agent (Iigo et al., 1999; Ushida et al., 1999; Masuda et al., 2000; Tsuda et al., 2000; Tsuda et al., 2002; Ramirez-Rico et al., 2022). However, the detailed molecular mechanisms of anti-cancer activity of LF have not been fully determined.
Tissue hypoxia is well-known to contribute to biologically aggressive tumor phenotypes and emergence of therapeutic resistance through reprograming of glucose metabolism (Höckel and Vaupel, 2001; Muz et al., 2015; Petrova et al., 2018; Sørensen and Horsman, 2020). In solid tumors, cancer cells are often exposed to low oxygen tension (hypoxia), low pH, and low nutrition due to inadequate vasculature, which cause a variety of biological changes through alterations of the expression of various genes (Keith and Simon, 2007; Jing et al., 2019; Abou Khouzam et al., 2021). Hypoxia-inducible factor-1α and -2α (HIF-1α and HIF-2α) are key transcription factors regulating a variety of hypoxia-inducible genes. Activated HIFs interact with cognate hypoxia-response elements (HRE) of target gene promoters under hypoxic conditions. To date, many HIF-target genes have been identified and their functional significance has been analyzed in a variety of cancers (Semenza and Wang, 1992; Wincewicz et al., 2007; Kaluz et al., 2009; Liu et al., 2012; Albanese et al., 2021; Kunej, 2021). We have identified several novel hypoxia-inducible genes and revealed their functions related to response to radiation- and chemo-therapy (Miyazaki et al., 2002; Nakamura et al., 2008; Shirakura et al., 2010; Bhawal et al., 2011; Khalesi et al., 2013; Nakamura et al., 2018). Hypoxia is also known to induce stress in the tissue microenvironment, which activates p53 signals (Zhang C et al., 2021). Tumor suppressor p53 protein works as a guardian of genome stability, regulator of the cell cycle, and conveyor of cell death signals including apoptosis and ferroptosis (Liu and Gu, 2022). Ferroptosis is a recently identified mode of cell death that is initiated by oxidative perturbations of the intracellular microenvironment and can be inhibited by iron chelators and lipophilic antioxidant (Galluzzi et al., 2018). Because LF itself is a natural iron chelator, albeit a more hydrophilic antioxidant (Mulder et al., 2018; Bielecka et al., 2022), it is expected to modify ferroptosis, especially in cancer cells (Zhang Z et al., 2021).
In this study, we undertook to clarify the cytotoxic functions of LF on various cell lines under hypoxic conditions and further understanding of those molecular mechanisms. LF was found to have cytotoxic activities on hypoxic cells with a range of sensitivities, possibly involving the p53-signaling pathway.
Most chemicals were analytical grade and were purchased from FUJIFILM Wako Pure Chemicals (Osaka, Japan), Sigma-Aldrich (St. Louis, MO, United States). Bovine lactoferrin (FUJIFILM Wako Pure Chemicals), Erastin (Selleckchem, Huston, TX, United States), or UAMC-3203 (Selleckchem). Bleomycin was kindly provided by Nippon Kayaku Company.
Human cell lines TIG-3 (lung fibroblast), KD (lip fibroblast), HSC2 (oral squamous cell carcinoma), MCF-7 (breast cancer), HeLa (cervical cancer), and HepG2 (hepatoma) were purchased from American Type Culture Collection (ATCC) or the Japanese Cancer Research Resource Bank (JCRRB) and have been maintained as original stocks. The cells were normally maintained in MEM, DMEM, or RPMI1640 (NACALAI TESQUE, Inc., Kyoto, Japan) containing 10% fetal bovine serum (FBS; BioWhittaker, Verviers, Belgium). HepG2 cells expressing mutant-type p53 were generated as previously described (Fukazawa et al., 2019). Briefly, pCMX empty plasmid vector or pCMX-p53-R248W were stably transfected into HepG2 cells, and stable clones were selected with medium containing G418 (C4: pCMX transfected cells; MT5: p53-R248W transfected cells). For expression analyses, cells (1 × 106 cells/10 cm diameter dish) were treated without or with the indicated concentrations of LF and subsequently cultured under normoxic (21% O2) or hypoxic (1% O2 in hypoxic chamber) conditions for 24 h. They were then harvested via centrifugation and cell pellets were store at −80°C until use.
Drug-induced cytotoxicity was evaluated with a conventional MTT dye reduction assay. Briefly, 4 × 103 cells were seeded in each well of 96-Micro Well Plates (NUNCLON, NUNC, Roskilde, Denmark) with regular medium. After incubation without or with LF or UAMC-3203 for 24 h, cells were exposed to various concentrations of LF, Bleomycin, or Erastin, for 72 h under normoxic or hypoxic conditions. Then 10 μl of 0.4% MTT reagent and 0.1 M sodium succinate were added to each well, and after 2 h incubation, 150 μl of DMSO was added to dissolve the purple formazan precipitate. The formazan dye was measured spectrophotometrically (570–650 nm) with a MAXline® Microplate Reader (Molecular Devices Corp., Sunnyvale, CA, United States). The cytotoxic effect of each treatment was assessed by IC50 value (inhibitory drug concentration producing 50% cell growth: drug concentration of 50% optical density of control). For knock-down experiments, nonspecific (siNS, No. 1027310), DEC2 (siDEC2, SI00428008), or TP53 (siTP53, SI0051779) siRNA (QIAGEN, Inc., Valencia, CA) was transfected with LipofectamineTM RNAiMAX (Thermo Fisher Scientific Inc.) into HepG2 cells (2 × 103/well of 96-Micro Well Plates) for 4 h, and then the cells were treated without or with LF under normoxic or hypoxic conditions for 72 h. Cell viabilities were evaluated with the MTT assay as above, and measured spectrophotometrical values were compared.
To analyze protein expression, whole cell extracts were prepared from cultured cells with or without hypoxic treatment as previously described (Tanimoto et al., 2000). 50 μg of extracts was blotted onto nitrocellulose filters following SDS-polyacrylamide gel electrophoresis. Anti-HIF-1α (610959, BD Pharmingen, San Diego, CA, United States), anti-HIF-2α (#7096, Cell Signaling TECHNOLOGY, Danvers, MA, United States), anti-E-cadherin (GTX100443, GeneTex, Irvine, CA, United States), anti-N-cadherin (GTX127345, GeneTex), anti-Vimentin (O91D3, BioLegend Inc., San Diego, CA, United States), anti-p53 (Ab-6, #OP43, ONCOGENE RESEARCH PRODUCTS, Boston, MA, United States), or anti-β-actin (A5441, Sigma) were used as primary antibodies, diluted 1:5000, 1:1000, 1:1000, or 1:5000, respectively. A 1:2000 dilution of anti-mouse IgG or anti-rabbit IgG horseradish peroxidase conjugate (#7076, #7074, Cell Signaling TECHNOLOGY) was used as a secondary antibody. Immunocomplexes were visualized by using the enhanced chemiluminescence reagent SuperSignal West Pico PLUS (Thermo Fisher Scientific K.K, Tokyo).
Total RNA was prepared from frozen cell pellets by using NucleoSpin® RNA (MACHEREY-NAGEL GmbH&Co., KG, Düren, Germany) according to the manufacturer’s instructions. To avoid detecting genomic or plasmid DNA with RT-PCR, all of the RNA samples were treated with DNase, which was included in the kit. 1 µg of total RNA was reverse-transcribed using a High-Capacity cDNA ArchiveTM Kit (Applied Biosystems, Foster City, CA, United States). A two-hundredth aliquot of the cDNA was subjected to quantitative RT-PCR using primers (final concentration 200 nM each) and MGB probe (final concentration 100 nM; the Universal Probe Library [UPL], Roche Diagnostics, Tokyo, Japan) sets (Supplementary Table S1) for CA9, DEC1, DEC2, BCL2, BAX, CDKN1A, CDH1, CDH2, VIM, SLC7A11, GPX4, ACSL4, HIF1A, EPAS1, and TP53. Pre-Developed TaqManTM Assay Reagents (Applied Biosystems) were used for ACTB as an internal control. PCR reactions were carried out with a 7500 Real-Time PCR System (Applied Biosystems) under standard conditions. Relative gene expression levels were calculated by using ACTB expression as the denominator for each cell line.
Statistical tests were performed using SPSS Statistics version 17.0 (IBM). For comparing paired groups, data were analyzed with Student’s t test. For comparing more than three groups, data were analyzed with a one-way ANOVA test, and Dunnett’s test or the Tukey-Kramer HSD test was used for post-hoc group comparisons. p ≤ 0.05 was considered statistically significant.
In order to clarify whether LF exerts cytotoxic activity on various cell lines under normoxic and hypoxic conditions, IC50 values were evaluated with the MTT assay. LF showed cytotoxic activity on tested cell lines with a range of sensitivities: TIG3 and KD were relatively sensitive, MCF-7 and HeLa were resistant, and HSC2 and HepG2 were intermediate under normoxic conditions (Figure 1A). Hypoxia decreased sensitivity to LF in KD but increased that in HSC2, whereas sensitivity in other cell types was not significantly changed. Immunoblot analysis revealed that LF treatment increased hypoxic HIF-1α and -2α proteins in KD but attenuated that in HSC2 cells in a dose-dependent manner (Figure 1B). LF treatment also decreased expression of HIF-1 target gene CA9 and increased expression of DEC2 in hypoxic HSC2 but not in KD (Figure 1C). The other target gene, DEC1, did not show any trend with LF treatment in either cell line. Because it is generally known that hypoxia regulates cell differentiation, especially epithelial-mesenchymal transition (EMT) in cancer cells, effects of LF on expression of EMT markers in KD and HSC2 cells were also evaluated. Immunoblot analysis revealed that epithelial marker E-cadherin was expressed in HSC2 but not in KD, mesenchymal marker vimentin was expressed in KD but not in HSC2, and N-cadherin was expressed in both cell types (Figure 1B). Furthermore, LF treatment decreased expression of E-cadherin and N-cadherin in HSC2, but increased expression of N-cadherin and vimentin in KD. Likewise, LF treatment decreased mRNA levels of CDH1 (E-cadherin) and CDH2 (N-cadherin) in HSC2 but increased those of CDH2 and VIM (vimentin) in KD (Figure 1D). Furthermore, expression of apoptosis and cell cycle related genes was evaluated in both cell lines; BCL2, BAX, and CDKN1A in KD had higher levels of expression than those in HSC2. LF treatment, especially in HSC2, decreased expression of BCL2 and seemed to enhance hypoxic induction of CDKN1A (Figure 1E). Protein levels of tumor suppressor p53, which regulates transcription of target genes including BAX and CDKN1A, were increased with LF treatments and further enhanced under hypoxic conditions in KD, but p53 was not detected in HSC2 (Figure 1B).
FIGURE 1. Cytotoxic activity of lactoferrin in various cell lines under normoxic (21% O2) or hypoxic (1% O2) conditions. (A) Cytotoxic activity evaluated as IC50 values with the MTT assay. Statistical significance is presented as + +: p < 0.01 (vs. TIG-3), **: p < 0.01 (indicated paired samples). (B) Effects of LF treatment on HIFs (HIF-1α and HIF-2α), EMT markers (E-cadherin, N-cadherin, and vimentin), and p53 proteins in KD and HSC2 under normoxic (N) and hypoxic (H) conditions for 24 h evaluated by using immunoblot analyses. Expression level of β-actin was used as a loading control. A representative result is shown (n = 3). (C) Effects of LF treatment on expression of HIF-1 target genes, CA9, DEC1, and DEC2. (D) EMT marker genes, CDH1, CDH2, and VIM. (E) Apoptosis and cell cycle related genes, BCL2, BAX, and CDKN1A, in KD and HSC2 under normoxic (N) and hypoxic (H) conditions for 24 h evaluated by using quantitative RT-PCR. Relative gene expression level was calculated by using ACTB expression as the denominator for each cell line (n = 3). For all quantitative values, the average and SD are shown. Statistical significance is represented as +: p < 0.05 and ++: p < 0.01 (N vs H); and #: p = 0.05, *: p < 0.05, and **: p < 0.01 (indicated paired samples).
Because tumor suppressor p53 is one of the most important regulators of apoptosis and the cell cycle, HepG2 expressing mutant-type p53 was employed to clarify the role of p53-signal in cytotoxic activity of LF. MTT assay revealed that forced expression of mutant-type p53 in HepG2 (MT5 clone) increased sensitivity to LF (decreased IC50 value), strikingly in comparison with empty-vector transfected HepG2 (C4 clone) (Figure 2A). Since p53 status is also well known to affect sensitivity to DNA-damage inducing anticancer drugs, IC50 values of Bleomycin in LF-treated HepG2 were evaluated. Sensitivity to Bleomycin in MT5 was significantly higher than in C4, and hypoxia drastically decreased sensitivity in both clones (Figure 2B). LF treatment significantly sensitized both clones to Bleomycin, regardless of oxygen condition, but LF worked more effectively in C4 than in MT5. Level of BCL2 expression was higher and that of CDKN1A was lower in MT5 than in C4, and LF treatment further increased expression of CDKN1A in C4 (Figure 2C). Level of BAX expression was decreased in hypoxic C4, and LF treatment increased it in normoxic C4 and decreased it in hypoxic MT5. Expression of CA9, DEC1, and DEC2 increased in both clones under hypoxic conditions, but was not changed with LF treatment (Figure 2D). Expression of DEC2 was significantly lower in MT5 than in C4, suggesting DEC2 regulation by mutant-type p53. Expression of CDH1 and CDH2 seemed to decrease with LF treatment, and expression of CDH2 was significantly lower in MT5, the same as DEC2 (Figure 2E). Because p53 signal seemed to play a role in response to LF, knock-down experiments using siRNA were performed in HepG2. Knock-down of TP53 (p53 gene) increased cell viability of LF-treated HepG2 under normoxic and hypoxic conditions (Figure 2F).
FIGURE 2. p53 status modifies cytotoxic activity of lactoferrin. Cytotoxic activity of (A) lactoferrin (LF) or (B) bleomycin on HepG2 transfected with pCMX empty plasmid vector (C4) or pCMX-p53-R248W (MT5) under normoxic (21% O2) and hypoxic (1% O2) conditions evaluated as IC50 values by using the MTT assay. Statistical significance is presented as ++: p < 0.01 (vs. LF 0 μg/ml of each clone), *: p < 0.05, and **: p < 0.01 (indicated paired samples). (C) Effects of LF treatment on expression of apoptosis and cell cycle related genes. (D) HIF-1 target genes. (E) EMT marker genes in C4 and MT5 under normoxic (N) and hypoxic (H) conditions for 24 h evaluated by using quantitative RT-PCR. Relative gene expression level was calculated by using ACTB expression as the denominator for each cell line (n = 3). For all quantitative values, the average and SD are shown. Statistical significance is represented as +: p < 0.05, and ++: p < 0.01 (N vs H), *: p < 0.05, and **: p < 0.01 (indicated paired samples). (F) Cytotoxic activity of lactoferrin (LF) on HepG2 transfected with control siRNA (siNS) or TP53 specific siRNA (siTP53) under normoxic (left) and hypoxic (right) conditions evaluated by using the MTT assay. Relative viability was calculated as percentage (%) of control (without LF treatment) viability. Statistical significance is presented as *: p < 0.05, and **: p < 0.01 (indicated paired samples).
In order to clarify the mechanism of cytotoxic activity of lactoferrin through p53 signal, possible involvement of ferroptosis was determined. At first, sensitivity of Erastin, which is known as a ferroptosis-inducing reagent, was evaluated in C4 and MT5, and MTT assay revealed that Erastin-induced ferroptosis seemed to occur in both C4 and MT5 (Figure 3A). IC50 values were higher in MT5 under normoxic and hypoxic conditions than in C4, and hypoxia significantly decreased sensitivity to Erastin in both clones. A ferroptosis inhibitor, UAMC-3203, drastically decreased the Erastin sensitivity in both clones under hypoxic conditions, suggesting hypoxia induced ferroptosis in those cells. Expression of the ferroptosis-regulating genes SLC7A11, GPX4, and ACSL4 was further evaluated in LF-treated C4 and MT5 under normoxic and hypoxic conditions (Figure 3B). Expression of SLC7A11 was higher in MT5 than in C4 and decreased in hypoxic C4 but not in MT5. Expression of GPX4 was slightly increased in hypoxic C4 and MT5 clones. Interestingly, expression of ACSL4 was increased in hypoxia and LF-treated MT5 but not in C4, suggesting an increase of ferroptosis just in LF-treated cells with mutant-type p53.
FIGURE 3. Possible involvement of ferroptosis in cytotoxic activity of lactoferrin under hypoxic conditions. (A) Cytotoxic activity of Erastin on HepG2 (C4) or (MT5) under normoxic (21% O2) and hypoxic (1% O2) conditions without or with ferroptosis inhibitor, UAMC-3203, were evaluated as IC50 values by using the MTT assay. Statistical significance presented as *: p < 0.05, and **: p < 0.01 (indicated paired samples). (B) Effects of LF treatment on feroptosis related genes, SLC7A11, GPX4, and ACSL4, in C4 and MT5 under normoxic (N) and hypoxic (H) conditions for 24 h evaluated by using quantitative RT-PCR. Relative gene expression level was calculated by using ACTB expression as the denominator for each cell line (n = 3). For all quantitative values, the average and SD are shown. Statistical significance is represented as +: p < 0.05, and ++: p < 0.01 (N vs H), *: p < 0.05, **: p < 0.01, and #: p = 0.05 (indicated paired samples).
In this study, inhibitory effects of LF on cell proliferation were observed in cell lines with a wide variety of sensitivities to LF. Firstly, regardless of whether cells were normal or malignant, LF inhibited cell proliferation of normal fibroblast (TIG-3 and KD), squamous cell carcinoma (HSC2), and hepatoma (HepG2) cells. LF also inhibited proliferation of MCF-7 and HeLa cells with significantly higher IC50 value than other cells, suggesting that cells derived from female-specific organs may have distinct mechanisms to resist cytotoxicity of LF. Interestingly, LF treatments oppositely affected KD and HSC2 under hypoxic conditions: Hypoxia decreased sensitivity (increased IC50 value) to LF in KD but increased sensitivity (decreased IC50 value) in HSC2. In order to clarify the molecular mechanisms of differential effects of LF on those cells, cellular characteristics were compared between those 2 cell types. At first, the effect of LF on levels of HIF-1α and -2α proteins, master regulators of the hypoxic transcriptome, were determined. Hypoxia-stabilized HIF-1α and -2α proteins in KD were strikingly found to be increased with LF treatments, but those in HSC2 were decreased. On the other hand, expression levels of HIF1A (HIF-1α gene) and EPAS1 (HIF-2α gene) did not increase in LF-treated or hypoxic KD and were not altered in HSC2, suggesting that LF affected the translational or post-translational process to regulate these proteins (Figure 1B, Supplemental Figure). It was also interestingly shown that LF affected levels of HIF-1α and HIF-2α proteins similarly, suggesting a common mechanism such as mTOR signal or PHD-pVHL axis (Albanese et al., 2021). Expressions of CA9, which is one of the most HIF-dependent target genes, in HSC2 decreased with LF treatment under hypoxic conditions concomitant with HIF-α proteins. However, its expression also slightly decreased in KD, in which HIF-1α protein level was increased with LF treatment. Although DEC1 did not show any trend of gene expression, DEC2 interestingly showed an opposite trend between KD and HSC2: LF treatment decreased DEC2 in KD but increased that in HSC2, suggesting a possible relationship between LF-modified DEC2 expression and HIF-1α protein level. Because a limited number of genes was analyzed in this study, further transcriptome analyses would be interesting if it could lead to identification of the functional genes involved in the response to LF.
Secondly, histological differences between KD and HSC2 were considered, since KD is derived from mesenchymal tissue and HSC2 from epithelial tissue. Epithelial marker E-cadherin was detected in HSC2 but not in KD, and mesenchymal marker vimentin was detected in KD but not in HSC2. N-cadherin was detected in both cell types. Hypoxia seemed to increase E-cadherin protein in HSC2 and LF treatment slightly decreased it. Hypoxia seemed not to modify vimentin protein level in KD, but LF treatment increased it. N-cadherin decreased in hypoxic HSC2 but not in KD, and increased in LF-treated KD but decreased in HSC2. RT-PCR analyses clearly demonstrated that expression of those proteins was regulated at the mRNA level: expression of CDH1 and CDH2 in HSC2 decreased with LF treatment whereas CDH2 and VIM in KD increased with LF treatment. These results indicate that LF treatment enhances mesenchymal phenotype in KD but causes a loss of histological features in HSC2, suggesting that the signal relating to mesenchymal features, especially N-cadherin, might be associated with response to LF. A previous study demonstrated that bLF treatment increases expression of E-cadherin but decreases that of vimentin in an oral squamous cell carcinoma line, HOC313, in which the EMT signal was induced, resulting in an inhibition of EMT (Chea et al., 2018a). Another study also reported that bLF treatment decreased expression of snail and vimentin but increased that of cadherin in a glioblastoma cell line (Cutone et al., 2020). Although those reports did not support our findings, the molecular mechanisms of cell-type-specific differential functions of LF need to be further investigated as our study suggested.
Finally, one of the well-known differences between benign (KD) and malignant (HSC2) cell lines was clear: head and neck cancers, including oral squamous cell carcinoma, are generally recognized as having the highest rate of p53 mutation (Olivier et al., 2010), and KD has wild-type p53 but HSC2 has mutant-type p53 (NM_001126113.3:c.672 + 1G > A) (JCRB Cell Bank, 1997). In this study, p53 protein was actually detected in KD but not in HSC2. p53 is one of the most important tumor suppressor proteins regulating apoptosis and the cell cycle (Aubrey et al., 2018; Pitolli et al., 2019). LF treatment decreased BCL2 expression and slightly increased CDKN1A in HSC2, suggesting inhibition of anti-apoptotic signal and induction of cell cycle arrest in HSC2 under hypoxic conditions. A previous report similarly demonstrated that bLF activates p53 and induces p21 in cancer cells, resulting in induction of G1/S arrest (Chea et al., 2018b). Because LF seemed to regulate apoptosis and the cell cycle in cells with mutant-type p53, we employed HepG2 expressing mutant-type p53, as previously reported (Fukazawa et al., 2019). HepG2 originally expresses wild-type p53. Therefore, transfected mutant-type p53 works as a dominant-negative form in HepG2 (MT5), resulting in a modified p53 signal (Fukazawa et al., 2019). MTT assay strikingly demonstrated that cells expressing mutant-type p53 (MT5) were significantly more sensitive to LF than control HepG2 (C4), suggesting an important role of the p53 signal in LF sensitivity. Level of BCL2 expression was higher, and levels of BAX and CDKN1A were lower in MT5 than in C4, resulting in phenotypes with anti-apoptotic and progressive cell cycle (Labi et al., 2008; Borrero and El-Deiry, 2021; Chota et al., 2021; Engeland, 2022). In fact, MT5 is more resistant than C4 to the anti-cancer drug Bleomycin. Hypoxia drastically decreased sensitivity to Bleomycin, but LF treatment sensitized both clones, suggesting potential application as a sensitizer or enhancer to anti-cancer therapies. The hypoxia-inducible gene DEC2 and the histological marker gene CDH2 were expressed at lower levels in MT5 under both normoxic and hypoxic conditions, suggesting that DEC2 and N-cadherin might contribute to differences between C4 and MT5. LF treatment further decreased the level of CDH2 expression just in MT5. Taken together, mutant-type p53-modified expression of target genes, especially DEC2 and CDH2, might contribute to differential response to LF treatment. Knock-down of TP53 interestingly reduced sensitivity to LF in HepG2 under normoxic and hypoxic conditions, although inhibition of p53 signal was expected to sensitize to LF. This might suggest that p53 signal itself is a target of LF cytotoxic activity, and loss of p53 signal would imply loss of the target of LF, resulting in decreased sensitivity or an alteration of determinants. In this study, LF sensitivity decreased in hypoxic KD, in which levels of p53 protein increased, however it increased in hypoxic HSC2, in which p53 protein was not detected. Namely, mutant-type p53 in MT5 might modify p53 signal to cause a gain of some functions that are targets of LF, or other sensitivity determinants, possibly HIFs, might dominate in HSC2. Previous reports demonstrated that LF specifically upregulates TP53 gene expression in HeLa cells through the activation of NF-κB, resulting in activation of p53 downstream signal (Oh et al., 2004), but bLF also induces the phosphorylation of p53 and induces p21 in cancer cells (Chea et al., 2018a). Those reports clearly indicate p53 as one of the targets of LF, although effects of LF on mutant-type p53 or p53 status had not yet been determined.
To further clarify detailed mechanisms of the cytotoxic activity of LF, we focused on ferroptosis, which is a newly identified mechanism of cell death in the p53 downstream signal. The p53 signal pathway has recently been found to sensitize cells to ferroptosis by inhibiting SLC7A11 (Jiang et al., 2015; Tarangelo et al., 2018; Liu et al., 2020). The ACSL4/LPCAT3/LOX signal axis is also known as an intracellular pathway that promotes lipid peroxidation in ferroptosis (Yuan et al., 2016; Tang et al., 2021). Erastin was used to check the ferroptosis activity in cell lines with the different p53 status. Erastin is a ferroptosis inducer that inhibits the cysteine transporter system, resulting in inhibition of antioxidation by the glutathione peroxidase (GPX) family (Dixon et al., 2014). Although Erastin inhibited cell viability of both HepG2 C4 and MT5, MT5 was significantly more resistant to Erastin than C4. Hypoxia further reduced sensitivity to Erastin in both clones. A ferroptosis inhibitor, UAMC-3203, drastically decreased sensitivity to Erastin in both clones under hypoxic conditions, suggesting that hypoxia induces ferroptosis in those cells. In fact, ferroptosis inhibiting SLC7A11 decreased in hypoxic C4 and promoting ACSL4 increased in hypoxic MT5, suggesting that mechanisms of hypoxia-induced ferroptosis are different in those clones. Importantly, LF treatment increased ACSL4 only in hypoxic MT5, suggesting the existence of LF-induced ferroptosis in cells expressing mutant-type p53.
In conclusion, a wide variety of sensitivities to cytotoxic activity of LF was observed in various cell lines, and hypoxia was found to regulate LF sensitivity differently among different cell lines, possibly through the p53 signaling pathway including DEC2 and N-cadherin. LF was further suggested to regulate ferroptosis through expression of ACSL4, depending on p53 status. Although further investigation including comparative analyses using multiple cell lines, comprehensive analyses and animal experiments is necessary to reach a definitive conclusion, this study provides novel insight into the clinical application of lactoferrin.
The original contributions presented in the study are included in the article/Supplementary Material, further inquiries can be directed to the corresponding author.
MYK analyzed the data and drafted the manuscript. TF performed the statistical analyses and edited the manuscript. MM provided materials and supervised the study. NH supervised the study. KT designed the study, analyzed the data, interpreted the results, edited the manuscript, and provided funding.
This work was partly supported by a Grant-in-Aid for Scientific Research from JSPS (JP21K100730).
We greatly appreciate the technical support provided by Ms. Chiyo Oda.
The authors declare that the research was conducted in the absence of any commercial or financial relationships that could be construed as a potential conflict of interest.
All claims expressed in this article are solely those of the authors and do not necessarily represent those of their affiliated organizations, or those of the publisher, the editors and the reviewers. Any product that may be evaluated in this article, or claim that may be made by its manufacturer, is not guaranteed or endorsed by the publisher.
The Supplementary Material for this article can be found online at: https://www.frontiersin.org/articles/10.3389/fphar.2022.988335/full#supplementary-material
Abou Khouzam, R., Brodaczewska, K., Filipiak, A., Zeinelabdin, N. A., Buart, S., Szczylik, C., et al. (2021). Tumor hypoxia regulates immune escape/invasion: Influence on angiogenesis and potential impact of hypoxic biomarkers on cancer therapies. Front. Immunol. 11, 613114. doi:10.3389/fimmu.2020.613114
Albanese, A., Daly, L. A., Mennerich, D., Kietzmann, T., and See, V. (2021). The role of hypoxia-inducible factor post-translational modifications in regulating its localisation, stability, and activity. Int. J. Mol. Sci. 22, 268. doi:10.3390/ijms22010268
Aubrey, B. J., Kelly, G. L., Janic, A., Herold, M. J., and Strasser, A. (2018). How does p53 induce apoptosis and how does this relate to p53-mediated tumour suppression? Cell Death Differ. 25, 104–113. doi:10.1038/cdd.2017.169
Bhawal, U. K., Sato, F., Arakawa, Y., Fujimoto, K., Kawamoto, T., Tanimoto, K., et al. (2011). Basic helix-loop-helix transcription factor DEC1 negatively regulates Cyclin D1. J. Pathol. 224, 420–429. doi:10.1002/path.2878
Bielecka, M., Cichosz, G., and Czeczot, H. (2022). Antioxidant, antimicrobial and anticarcinogenic activities of bovine milk proteins and their hydrolysates - a review. Int. Dairy J. 127, 105208. doi:10.1016/j.idairyj.2021.105208
Borrero, L. J. H., and El-Deiry, W. S. (2021). Tumor suppressor p53: Biology, signaling pathways, and therapeutic targeting. Biochim. Biophys. Acta. Rev. Cancer 1876, 188556. doi:10.1016/j.bbcan.2021.188556
Chea, C., Miyauchi, M., Inubushi, T., Febriyanti Ayuningtyas, N., Subarnbhesaj, A., Nguyen, P. T., et al. (2018a). Molecular mechanism of inhibitory effects of bovine lactoferrin on the growth of oral squamous cell carcinoma. PLOS ONE 13, e0191683. doi:10.1371/journal.pone.0191683
Chea, C., Miyauchi, M., Inubushi, T., Okamoto, K., Haing, S., Nguyen, P. T., et al. (2018b). Bovine lactoferrin reverses programming of epithelial-to-mesenchymal transition to mesenchymal-to-epithelial transition in oral squamous cell carcinoma. Biochem. Biophys. Res. Commun. 507, 142–147. doi:10.1016/j.bbrc.2018.10.193
Chota, A., George, B. P., and Abrahamse, H. (2021). Interactions of multidomain pro-apoptotic and anti-apoptotic proteins in cancer cell death. Oncotarget 12, 1615–1626. doi:10.18632/oncotarget.28031
Cutone, A., Colella, B., Pagliaro, A., Rosa, L., Lepanto, M. S., Bonaccorsi di Patti, M. C., et al. (2020). Native and iron-saturated bovine lactoferrin differently hinder migration in a model of human glioblastoma by reverting epithelial-to-mesenchymal transition-like process and inhibiting interleukin-6/STAT3 axis. Cell. Signal. 65, 109461. doi:10.1016/j.cellsig.2019.109461
Dixon, S. J., Patel, D. N., Welsch, M., Skouta, R., Lee, E. D., Hayano, M., et al. (2014). Pharmacological inhibition of cystine – glutamate exchange induces endoplasmic reticulum stress and ferroptosis. elife 3, e02523. doi:10.7554/eLife.02523
Engeland, K. (2022). Cell cycle regulation: p53-p21-RB signaling. Cell Death Differ. 29, 946–960. doi:10.1038/s41418-022-00988-z
Fukazawa, T., Tanimoto, K., Shrestha, L., Imura, T., Takahashi, S., Sueda, T., et al. (2019). Simulated microgravity enhances CDDP-induced apoptosis signal via p53-independent mechanisms in cancer cells. PLOS ONE 14, e0219363. doi:10.1371/journal.pone.0219363
Galluzzi, L., Vitale, I., Aaronson, S. A., Abrams, J. M., Adam, D., Agostinis, P., et al. (2018). Molecular mechanisms of cell death: Recommendations of the nomenclature committee on cell death 2018. Cell Death Differ. 25, 486–541. doi:10.1038/s41418-017-0012-4
Höckel, M., and Vaupel, P. (2001). Biological consequences of tumor hypoxia. Semin. Oncol. 28, 36–41. doi:10.1016/S0093-7754(01)90211-8
Iglesias-Figueroa, B. F., Espinoza-Sanchez, E. A., Siqueiros-Cendon, T. S., and Rascon-Cruz, Q. (2019). Lactoferrin as a nutraceutical protein from milk, an overview. Int. Dairy J. 89, 37–41. doi:10.1016/j.idairyj.2018.09.004
Iigo, M., Kuhara, T., Ushida, Y., Sekine, K., Moore, M. A., and Tsuda, H. (1999). Inhibitory effects of bovine lactoferrin on colon carcinoma 26 lung metastasis in mice. Clin. Exp. Metastasis 17, 35–40. doi:10.1023/a:1026452110786
Iigo, M., Shimamura, M., Matsuda, E., Fujita, K. i., Nomoto, H., Satoh, J., et al. (2004). Orally administered bovine lactoferrin induces caspase-1 and interleukin-18 in the mouse intestinal mucosa: a possible explanation for inhibition of carcinogenesis and metastasis. Cytokine 25, 36–44. doi:10.1016/j.cyto.2003.09.009
JCRB Cell Bank (1997). p53 status. Available at: https://cellbank.nibiohn.go.jp/legacy/cellinfo/p5301.htm (Accessed on 22nd June, 2022)
Jiang, L., Kon, N., Li, T., Wang, S. J., Su, T., Hibshoosh, H., et al. (2015). Ferroptosis as a p53-mediated activity during tumour suppression. Nature 520, 57–62. doi:10.1038/nature14344
Jing, X., Yang, F., Shao, C., Wei, K., Xie, M., Shen, H., et al. (2019). Role of hypoxia in cancer therapy by regulating the tumor microenvironment. Mol. Cancer 18, 157–215. doi:10.1186/s12943-019-1089-9
Kaluz, S., Kaluzova, M., Liao, S. Y., Lerman, M., and Stanbridge, E. J. (2009). Transcriptional control of the tumor- and hypoxia-marker carbonic anhydrase 9: A one transcription factor (HIF-1) show? Biochim. Biophys. Acta 1795, 162–172. doi:10.1016/j.bbcan.2009.01.001
Keith, B., and Simon, M. C. (2007). Hypoxia-inducible factors, stem cells, and cancer. Cell 129, 465–472. doi:10.1016/j.cell.2007.04.019
Kell, D. B., Heyden, E. L., and Pretorius, E. (2020). The biology of lactoferrin, an iron-binding protein that can help defend against viruses and bacteria. Front. Immunol. 11, 1221–1315. doi:10.3389/fimmu.2020.01221
Khalesi, E., Nakamura, H., Lee, K. L., Putra, A. C., Fukazawa, T., Kawahara, Y., et al. (2013). The Krüppel-like zinc finger transcription factor, GLI-similar 1, is regulated by hypoxia-inducible factors via non-canonical mechanisms. Biochem. Biophys. Res. Commun. 441, 499–506. doi:10.1016/j.bbrc.2013.10.083
Kruzel, M. L., Zimecki, M., and Actor, J. K. (2017). Lactoferrin in a context of inflammation-induced pathology. Front. Immunol. 8, 1438. doi:10.3389/fimmu.2017.01438
Kunej, T. (2021). Integrative map of HIF1A regulatory elements and variations. Genes 12, 1526. doi:10.3390/genes12101526
Labi, V., GrespiF., , BaumgartnerF., , and Villunger, A. (2008). Targeting the bcl-2-regulated apoptosis pathway by BH mimetics: a breakthrough in anticancer therapy? Cell Death Differ. 15, 977–987. doi:10.1038/cdd.2008.37
Liu, Y., and Gu, W. (2022). P53 in ferroptosis regulation: the new weapon for the old guardian Cell Death Differ. 29, 895–910. doi:10.1038/s41418-022-00943-y
Liu, J., Zhang, C., Wang, J., Hu, W., and Feng, Z. (2020). The regulation of ferroptosis by tumor suppressor p53 and its pathway. Int. J. Mol. Sci. 21, 8387. doi:10.3390/ijms21218387
Liu, Q., Davidoff, O., Niss, K., and Haase, V. H. (2012). Hypoxia-inducible factor regulates hepcidin via erythropoietin-induced erythropoiesis. J. Clin. Invest. 122, 4635–4644. doi:10.1172/JCI63924
Masuda, C., Wanibuchi, H., Sekine, K., Yano, Y., Otani, S., Kishimoto, T., et al. (2000). Chemopreventive effects of bovine lactoferrin on N-Butyl-N-(4-hydroxybutyl)nitrosamine-induced Rat Bladder Carcinogenesis. Jpn. J. Cancer Res. 91, 582–588. doi:10.1111/j.1349-7006.2000.tb00985.x
Miyazaki, K., Kawamoto, T., Tanimoto, K., Nishiyama, M., Honda, H., and Kato, Y. (2002). Identification of functional hypoxia response elements in the promoter region of the DEC1 and DEC2 genes. J. Biol. Chem. 277, 47014–47021. doi:10.1074/jbc.M204938200
Mulder, A. M., Connellan, P. A., Oliver, C. J., Morris, C. A., and Stevenson, L. M. (2018). Bovine lactoferrin supplementation supports immune and antioxidant status in healthy human males. Nutr. Res. 28, 583–589. doi:10.1016/j.nutres.2008.05.007
Muz, B., de la Puente, P., Azab, F., and Azab, A. K. (2015). The role of hypoxia in cancer progression, angiogenesis, metastasis, and resistance to therapy. Hypoxia 3, 83–92. doi:10.2147/hp.s93413
Nakamura, H., Bono, H., Hiyama, K., Kawamoto, T., Kato, Y., Nakanishi, T., et al. (2018). Differentiated embryo chondrocyte plays a crucial role in DNA damage response via transcriptional regulation under hypoxic conditions. PLOS ONE 13, e0192136. doi:10.1371/journal.pone.0192136
Nakamura, H., Tanimoto, K., Hiyama, K., Yunokawa, M., Kawamoto, T., Kato, Y., et al. (2008). Human mismatch repair gene, MLH1, is transcriptionally repressed by the hypoxia-inducible transcription factors, DEC1 and DEC2. Oncogene 27, 4200–4209. doi:10.1038/onc.2008.58
Oh, S-M., Pyo, C. W., Kim, Y., and Choi, S. Y. (2004). Neutrophil lactoferrin upregulates the human p53 gene through induction of NF-kappaB activation cascade. Oncogene 23, 8282–8291. doi:10.1038/sj.onc.1208021
Olivier, M., Hollstein, M., and Hainaut, P. (2010). TP53 mutations in human cancers: origins, consequences, and clinical use. Cold Spring Harb. Perspect. Biol. 2, a001008. doi:10.1101/cshperspect.a001008
Petrova, V., Annicchiarico-Petruzzelli, M., Melino, G., and Amelio, I. (2018). The hypoxic tumour microenvironment. Oncogenesis 7, 10. doi:10.1038/s41389-017-0011-9
Pitolli, C., Wang, Y., Candi, E., Shi, Y., Melino, G., and Amelio, I. (2019). p53-Mediated tumor suppression: DNA-damage response and alternative mechanisms. Cancers 11, 1983. doi:10.3390/cancers11121983
Ramirez-Rico, G., Drago-Serrano, M. E., Leon-Sicairos, N., and de la Garza, M. (2022). Lactoferrin: A nutraceutical with activity against colorectal cancer. Front. Pharmacol. 13, 855852. doi:10.3389/fphar.2022.855852
Rodrigues, L., Teixeira, J., Schmitt, F., Paulsson, M., and Mansson, H. L. (2009). Lactoferrin and cancer disease prevention. Crit. Rev. Food Sci. Nutr. 49, 203–217. doi:10.1080/10408390701856157
Semenza, G. L., and Wang, G. L. (1992). A nuclear factor induced by hypoxia via de novo protein synthesis binds to the human erythropoietin gene enhancer at a site required for transcriptional activation. Mol. Cell. Biol. 12, 5447–5454. doi:10.1128/mcb.12.12.5447
Shirakura, M., Tanimoto, K., Eguchi, H., Miyauchi, M., Nakamura, H., Hiyama, K., et al. (2010). Activation of the hypoxia-inducible factor-1 in overloaded temporomandibular joint, and induction of osteoclastogenesis. Biochem. Biophys. Res. Commun. 393, 800–805. doi:10.1016/j.bbrc.2010.02.086
Siqueiros-Cendón, T., Arevalo-Gallegos, S., Iglesias-Figueroa, B. F., Garcia-Montoya, I. A., Salazar-Martinez, J., and Rascon-Cruz, Q. (2014). Immunomodulatory effects of lactoferrin. Acta Pharmacol. Sin. 35, 557–566. doi:10.1038/aps.2013.200
Sørensen, B. S., and Horsman, M. R. (2020). Tumor hypoxia: Impact on radiation therapy and molecular pathways. Front. Oncol. 10, 562. doi:10.3389/fonc.2020.00562
Takakura, N., Wakabayashi, H., Yamauchi, K., and Takase, M. (2006). Influences of orally administered lactoferrin on IFN-γ and IL-10 production by intestinal intraepithelial lymphocytes and mesenteric lymph-node cells. Biochem. Cell Biol. 84, 363–368. doi:10.1139/o06-056
Tang, Z., Huang, Z., Huang, Y., Chen, Y., Huang, M., Liu, H., et al. (2021). Ferroptosis : The silver lining of cancer therapy. Front. Cell Dev. Biol. 9, 765859. doi:10.3389/fcell.2021.765859
Tanimoto, K., Makino, Y., Pereira, T., and Poellinger, L. (2000). Mechanism of regulation of the hypoxia-inducible factor-1α by the von Hippel-Lindau tumor suppressor protein. EMBO J. 19, 4298–4309. doi:10.1093/emboj/19.16.4298
Tarangelo, A., Magtanong, L., Bieging-Rolett, K. T., Li, Y., Ye, J., Attardi, L. D., et al. (2018). p53 suppresses metabolic stress-induced ferroptosis in cancer cells. Cell Rep. 22, 569–575. doi:10.1016/j.celrep.2017.12.077
Tsuda, H., Sekine, K., Takasuka, N., Toriyama-Baba, H., and Iigo, M. (2000). Prevention of colon carcinogenesis and carcinoma metastasis by orally administered bovine lactoferrin in animals. BioFactors 12, 83–88. doi:10.1002/biof.5520120113
Tsuda, H., Sekine, K., Fujita, K., and Ligo, M. (2002). Cancer prevention by bovine lactoferrin and underlying mechanisms – a review of experimental and clinical studies. Biochem. Cell Biol. 80, 131–136. doi:10.1139/o01-239
Ushida, Y., Sekine, K., Kuhara, T., Takasuka, N., Iigo, M., Maeda, M., et al. (1999). Possible chemopreventive effects of bovine lactoferrin on esophagus and lung carcinogenesis in the rat. Jpn. J. Cancer Res. 90, 262–267. doi:10.1111/j.1349-7006.1999.tb00742.x
Wincewicz, A., Sulkowska, M., Koda, M., and Sulkowski, S. (2007). Cumulative expression of HIF-1-alpha, Bax, Bcl-xL and P53 in human colorectal cancer. Pathology 39, 334–338. doi:10.1080/00313020701329765
Yuan, H., Li, X., Zhang, X., Kang, R., and Tang, D. (2016). Identification of ACSL4 as a biomarker and contributor of ferroptosis. Biochem. Biophys. Res. Commun. 478, 1338–1343. doi:10.1016/j.bbrc.2016.08.124
Zhang, C., Liu, J., Wang, J., Zhang, T., Xu, D., Hu, W., et al. (2021). The interplay between tumor suppressor p53 and hypoxia signaling pathways in cancer. Front. Cell Dev. Biol. 9, 648808. doi:10.3389/fcell.2021.648808
Keywords: lactoferrin, cytotoxic activity, hypoxia, p53, ferroptosis
Citation: Kosim MY, Fukazawa T, Miyauchi M, Hirohashi N and Tanimoto K (2022) p53 status modifies cytotoxic activity of lactoferrin under hypoxic conditions. Front. Pharmacol. 13:988335. doi: 10.3389/fphar.2022.988335
Received: 07 July 2022; Accepted: 01 September 2022;
Published: 19 September 2022.
Edited by:
Chunli Wang, Chongqing University, ChinaReviewed by:
Jie Zhou, Tongji Hospital Affiliated to Tongji University, ChinaCopyright © 2022 Kosim, Fukazawa, Miyauchi, Hirohashi and Tanimoto. This is an open-access article distributed under the terms of the Creative Commons Attribution License (CC BY). The use, distribution or reproduction in other forums is permitted, provided the original author(s) and the copyright owner(s) are credited and that the original publication in this journal is cited, in accordance with accepted academic practice. No use, distribution or reproduction is permitted which does not comply with these terms.
*Correspondence: Keiji Tanimoto, a3Rhbmltb0BoaXJvc2hpbWEtdS5hYy5qcA==
Disclaimer: All claims expressed in this article are solely those of the authors and do not necessarily represent those of their affiliated organizations, or those of the publisher, the editors and the reviewers. Any product that may be evaluated in this article or claim that may be made by its manufacturer is not guaranteed or endorsed by the publisher.
Research integrity at Frontiers
Learn more about the work of our research integrity team to safeguard the quality of each article we publish.