- 1Neuroscience and Mental Health Innovation Institute, Cardiff University, Cardiff, United Kingdom
- 2Division of Neuroscience, School of Biosciences, Cardiff University, Cardiff, United Kingdom
- 3Institute of Psychopharmacology, Central Institute of Mental Health, Medical Faculty Mannheim, University of Heidelberg, Mannheim, Germany
Ras/Raf/MEK/ERK (Ras-ERK) signaling has been demonstrated to play a role in the effects of drugs of abuse such as cocaine and alcohol, but has not been extensively examined in nicotine-related reward behaviors. We examined the role of Ras Guanine Nucleotide Releasing Factor 2 (RasGRF2), an upstream mediator of the Ras-ERK signaling pathway, on nicotine self-administration (SA) in RasGRF2 KO and WT mice. We first demonstrated that acute nicotine exposure (0.4 mg/kg) resulted in an increase in phosphorylated ERK1/2 (pERK1/2) in the striatum, consistent with previous reports. We also demonstrated that increases in pERK1/2 resulting from acute (0.4 mg/kg) and repeated (0.4 mg/kg, 10 daily injections) exposure to nicotine in WT mice were not present in RasGRF2 KO mice, confirming that RasGRF2 at least partly regulates the activity of the Ras-ERK signaling pathway following nicotine exposure. We then performed intravenous nicotine SA (0.03 mg/kg/infusion for 10 days) in RasGRF2 KO and WT mice. Consistent with a previous report using cocaine SA, RasGRF2 KO mice demonstrated an increase in nicotine SA relative to WT controls. These findings suggest a role for RasGRF2 in the reinforcing effects of nicotine, and implicate the Ras-ERK signaling pathway as a common mediator of the response to drugs of abuse.
Introduction
Worldwide tobacco smoking rates have been on the decline for several years, due in large part to the MPOWER measures instituted by the World Health Organization (World Health Organization, 2021). Nevertheless, tobacco remains one of the leading causes of preventable deaths worldwide, with an estimated 8 million smoking-related deaths annually (World Health Organization, 2021). Nicotine is the primary reinforcing component of tobacco, and continued tobacco use can result in nicotine dependence in humans. Particularly worrisome is the dramatic increase in the use of electronic nicotine delivery systems (ENDS) or e-cigarettes, especially among adolescents, which may lead to the use of traditional tobacco products (Leventhal et al., 2015; Ren and Lotfipour, 2019; Park et al., 2020). In addition to the potential development of nicotine dependence, the use of ENDS likely have health consequences similar to those described for traditional tobacco products, such as cardiovascular and respiratory diseases (Glynos et al., 2018; Davidson and Fox, 2020; Raja et al., 2021). Thus, continuing to identify and understand the molecular mechanisms that underlie nicotine reinforcement is critical to the development of strategies to further counteract tobacco use and pharmacotherapies for nicotine dependence.
The extracellular signal-regulated kinases (ERK) cascade (Ras/Raf/MEK/ERK; Ras-ERK), a member of the mitogen-activated protein kinase (MAPK) family, is an important signaling pathway linking signals from cell surface receptors with changes in gene expression (Grewal et al., 1999; Orton et al., 2005). This pathway has been implicated in the acute and long-term effects of drugs of abuse, including cocaine and other psychostimulants (Valjent et al., 2006; Papale et al., 2016; Bernardi et al., 2019), alcohol (Sanna et al., 2002; Faccidomo et al., 2009; Agoglia et al., 2015; Faccidomo et al., 2015), and nicotine (Brunzell et al., 2003; Valjent et al., 2004; Valjent et al., 2005; Wilar et al., 2019), likely regulating striatal drug-dependent synaptic plasticity (Girault et al., 2007; Cerovic et al., 2013). In terms of nicotine, administration (0.4 mg/kg) in mice has been demonstrated to increase the phosphorylation of ERK1/2 (pERK1/2) in the ventral and dorsal striatum (Valjent et al., 2004; Valjent et al., 2005; but see Brunzell et al., 2003), mesolimbic dopamine structures that have both been shown to mediate neuroadaptations associated with drug dependence (Koob and Volkow, 2010; Lobo and Nestler, 2011). In addition, studies of nicotine conditioned place preference in mice have demonstrated the potential involvement of ERK in nicotine reward, as nicotine conditioning increased pERK1/2 relative to saline conditioning in the nucleus accumbens (NAc) (Wilar et al., 2019; Jia et al., 2021). And self-administration studies have also demonstrated the involvement of ERK and downstream targets of ERK in nicotine reinforcement (Konu et al., 2001; Li et al., 2012; Gomez et al., 2015). Thus, ERK is clearly involved in the molecular and behavior effects of nicotine; however, less is known about the role of upstream regulators of ERK that may mediate these effects.
Ras guanine nucleotide exchange factors (GEFs) are critical mediators of the activity of the Ras-ERK signaling pathway. For example, Ras Guanine Nucleotide Releasing Factor 2 (RasGRF2) is one of several GEFs that mediates the cycling of the Ras family of small GTPases from an inactive GDP-bound state to an active GTP-bound state in a Ca2/calmodulin-dependent way via various neurotransmitter receptors (Zippel et al., 1997; Quilliam et al., 2002; Pamonsinlapatham et al., 2009; Feig, 2011), allowing the transduction of extracellular signals to downstream effectors such as MEK and ERK (Takai et al., 2001; Feig, 2011). Thus, given the role of the Ras-ERK cascade in drug-mediated plasticity, RasGRF2 likely functions as a critical mediator of reinforcement processes that precipitate drug dependence.
We recently demonstrated that cocaine self-administration (SA) was increased in RasGRF2 knock-out (KO) mice relative to their wild-type littermates (WT), which we attributed to a decrease in cocaine reinforcement and compensatory increase in intake to achieve similar putative reward (Bernardi et al., 2019). Importantly, these alterations in cocaine intake were accompanied by an attenuation of the cocaine-induced increase in pERK in the ventral and dorsal striatum in RasGRF2 KO mice. Furthermore, these molecular and behavioral findings in RasGRF2 KO mice were replicated in C57Bl/6 mice using the MEK inhibitor PD325901. These findings suggest that RasGRF2 mediates the cellular response to cocaine via Ras-ERK signaling (Bernardi et al., 2019). RasGRF2 has also been demonstrated to mediate alcohol reinforcement, as RasGRF2 KO mice showed a reduction in alcohol drinking and an attenuation of the increase in dopamine induced by alcohol in the nucleus accumbens (NAc) (Stacey et al., 2012). Thus, RasGRF2 likely plays a general role in reward processes by mediating the activity of the Ras-ERK pathway in striatal regions (Mazzucchelli and Brambilla, 2000; Stornetta and Zhu, 2011).
The goal of the current set of experiments was to examine the role of RasGRF2 in nicotine reinforcement using nicotine self-administration in a separate cohort of RasGRF2 KO and WT mice. We first sought to demonstrate a role for ERK resulting from acute nicotine administration in male C57Bl/6N mice by performing immunohistochemistry for pERK1/2 in the striatum. We also performed pERK1/2 immunohistochemistry in RasGRF2 KO and WT male mice in these regions following both acute and repeated nicotine administration. We then performed nicotine SA in RasGRF2 KO mice and WT littermate controls; here we used both male and female mice to identify potential sex differences in the effects of the RasGRF2 KO on nicotine reinforcement.
Materials and methods
Animals
Male C57Bl/6N (Charles River, Germany) and male and female RasGRF2 (Fernandez-Medarde et al., 2002) KO mice and their WT littermate controls were single-housed in a temperature-controlled (21°C) environment maintained on a 12-h light-dark cycle (lights on at 6 a.m.). Food and water was available ad libitum. All experiments were performed in accordance with EU guidelines on the care and use of laboratory animals and were approved by the local animal care committee (Regierungspräsidium Karlsruhe, Germany). All experiments were conducted during the light phase between 8:00 and 13:00 h.
Drugs
(-)-Nicotine ditartrate (Sigma-Aldrich, Germany) was dissolved in physiological saline (0.9% NaCl) for 0.4 mg/kg (10 ml/kg IP) administration for peripheral acute and repeated studies and 0.03 mg/kg/35 µl infusion for intravenous SA (pH to approximately 7.0 using NaOH). All doses are based on the free base weight of nicotine.
Apparatus and procedures
pERK immunohistochemistry in male C57Bl/6N and RasGRF2 mice
For acute nicotine exposure, male C57Bl/6N or RasGRF2 KO and WT mice were habituated to saline injections for 4 days. The following day, mice were injected with nicotine or saline (0.4 mg/kg IP; C57Bl/6N mice, n = 5 per group; RasGRF2 KO and WT mice, n = 3–4 per group). For repeated nicotine exposure, male RasGRF2 KO and WT mice were given 10 daily injections of nicotine or saline (0.4 mg/kg IP; 4–5 per group).
Twenty minutes following the acute injection or final injection following repeated exposure, mice were anesthetized with isoflurane and transcardially perfused with phosphate-buffered saline (PBS) and 4% paraformaldehyde (PFA) in PBS. Brains were removed and placed in 4% PFA in PBS for 24 h for post-fixation, and then transferred into 20% sucrose in PBS for at least 24 h and finally into 30% sucrose in PBS until processing. Immunohistochemistry was performed following the protocol described previously (Bernardi et al., 2019; Olevska et al., 2021). Free-floating sections were rinsed in TBS and then incubated for 15 min in a quenching solution containing 3% H2O2 and 10% methanol. One hour after blocking in 5% normal goat serum and 0.1% Triton X-100, sections were incubated overnight at 4°C with anti-phospho-p44/42 MAP kinase (Thr202/Tyr204) (1:1,000, Cell Signaling Technology Cat# 4370L, RRID:AB_231511). The next day, slices were rinsed in TBS and incubated with biotinylated goat anti-rabbit IgG (1:200, Vector Laboratories Cat# BA-1000, RRID:AB_2313606) for 2 h at room temperature. Detection of the bound antibodies was carried out using a standard peroxidase-based method (ABC-kit, Vectastain, Vector Labs), followed by incubation with a DAB and H2O2 solution. Images were acquired from the dorsal and ventral striatum with a bright field microscope (Leica DM2000LED Macro/micro imaging system) at ×20 magnification. Neuronal quantification was carried out using ImageJ software. The total number of pERK1/2-positive cells was counted in the dorsal and ventral striatum in 2–4 rostral sections per mouse. The pERK count from one animal in the acute experiment was identified as a significant outlier in the dorsal striatum using Grubbs’ test and thus the animal was removed from all further analyses.
Nicotine self-administration in male and female RasGRF2 mice
Nicotine SA was assessed in 8 operant chambers (Med Associates, United States) housed in light- and sound-attenuating cubicles. Each chamber (24.1 cm × 20.3 cm × 18.4 cm) is equipped with two levers (left and right), a food dispenser and a drug delivery system connected via infusion pump (PHM-100, Med-Associates, United States) located outside the cubicle. Operant chambers were controlled using Med-PC IV (Med Associates, United States) software. Male and female RasGRF2 mice first underwent lever training with 14 mg sweetened food pellets (TestDiet, United States), as previously described (Bernardi and Spanagel, 2013). Following lever training, mice were implanted with an indwelling intravenous catheter (made in-house) into the jugular vein. Catheter patency was maintained with 0.15 ml heparinized saline (100 i.u./ml) containing Baytril (0.7 mg/ml) administered daily throughout the experiment. After 3 days recovery, mice underwent daily nicotine SA for 10 consecutive days. Nicotine (0.03 mg/kg/35 µl infusion) delivery was contingent upon pressing on the active lever under an FR2 schedule of reinforcement and paired with the 20s presentation of a blinking light stimulus (Conditioned Stimulus, CS), which also served as a timeout period, during which lever presses were not reinforced. The number of nicotine infusions achieved was limited to 10 and 15 infusions during sessions 1 and 2, respectively, the achievement of which resulted in the end of the session. The session continued for 1 h if these limits were not met. This limit was to ensure that excessive intake during initial access to nicotine did not become aversive. This procedure results in sustained nicotine intake across trials (personal observation from R.E. Bernardi). Days 1 and 2 are depicted in all figures, but not included in data analyses. Sessions 3–10 were 1 h in duration. Presses on the inactive lever were recorded but had no scheduled consequences. Nicotine SA was assessed in male and female RasGRF2 WT (male, n = 10; female, n = 13) and KO (male, n = 12; female, n = 11) mice.
Statistical analysis
Statistical analyses were conducted using SPSS software (StatSoft, United States). Immunohistochemical data were conducted using two-way ANOVAs followed by Bonferroni-corrected independent samples t-tests, where indicated. All SA data were analyzed across days 3–10 using ANOVAs, as indicated, followed by Bonferroni-corrected independent samples t-tests, where indicated. Significance was set at p < 0.05.
Results
pERK is significantly increased in the striatum of male C57Bl/6N mice
Following acute nicotine injections, male C57Bl/6N mice demonstrated a significant increase in the number of pERK1/2-positive cells in response to nicotine relative to saline controls in the striatum. Figure 1A shows the mean number of pERK1/2-positive cells (±SEM) in the ventral and dorsal striatum following acute saline (n = 5) or nicotine (n = 5) administration in C57Bl/6N mice. A two-way ANOVA (region x treatment) of the number of pERK1/2-positive cells revealed a significant main effect of treatment [F (1, 16) = 8.1, p = 0.011], but no other significant effects [Fs < 1]. Figure 1B shows representative slices of pERK1/2-positive cells from the ventral and dorsal striatum of C57Bl6N mice administered saline or nicotine.
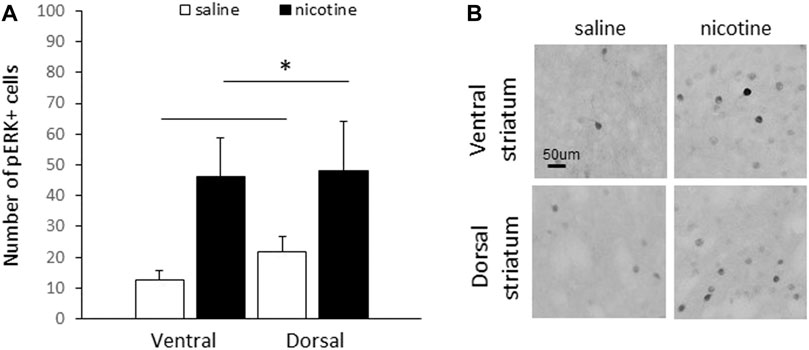
FIGURE 1. Acute nicotine increased striatal pERK in male C57Bl6/N mice. (A) An acute injection of nicotine (0.4 mg/kg IP) resulted in a significant increase in the number of pERK1/2-positive cells relative to saline controls in the striatum (n = 5/group). Data represent the mean number of pERK1/2-positive cells (±SEM) in each condition in the ventral and dorsal striatum. (B) Representative slices showing pERK-positive cells from the ventral and dorsal striatum of male C57Bl6N mice that received acute nicotine or saline injections. *p < 0.05, main effect of treatment (nicotine relative to saline).
pERK is significantly increased in the striatum following acute nicotine in male WT, but not RasGRF2 KO mice
Following acute nicotine injections, male WT mice demonstrated an increase in the number of pERK1/2-positive cells in response to nicotine relative to saline controls in both the ventral and dorsal striatum. This increase in the number of pERK1/2-positive cells was not present in either the ventral or dorsal striatum of RasGRF2 KO mice (n = 3–4/group). Figure 2A shows the mean number of pERK1/2-positive cells (±SEM) in the ventral striatum in RasGRF2 KO and WT mice sacrificed following an acute injection of saline or nicotine. A two-way ANOVA (genotype × treatment) of the number of pERK1/2-positive cells revealed significant main effects of treatment [F (1, 9) = 6.1, p = 0.035] and a significant genotype × treatment interaction [F (1, 9) = 9.9, p = 0.012], but no effect of genotype [F (1, 9) = 2.7, p = 0.14]. Independent samples t-tests revealed a significant increase in the number of pERK1/2-positive cells in WT mice administered nicotine relative to saline-treated mice [t (5) = 3.8, p = 0.012; Bonferroni-corrected α/2 = 0.025] that was not present in KO mice [t (4) = 0.43, p = 0.69; Bonferroni-corrected α/2 = 0.025]. Figure 2B shows the mean number of pERK1/2-positive cells (±SEM) in the dorsal striatum in RasGRF2 KO and WT mice sacrificed following an acute injection of saline or nicotine. A two-way ANOVA (genotype x treatment) of the number of pERK1/2-positive cells revealed significant main effects of genotype [F (1, 9) = 23.2, p = 0.001] and treatment [F (1, 9) = 28.6, p < 0.0005], and a genotype × treatment interaction [F (1, 9) = 39.0, p < 0.0005]. Independent samples t-tests revealed a significant increase in the number of pERK1/2-positive cells in WT mice administered nicotine relative to saline-treated mice [t (5) = 9.1, p < 0.0005; Bonferroni-corrected α/2 = 0.025] that was not present in KO mice [t (4) = 0.57, p = 0.60; Bonferroni-corrected α/2 = 0.025]. Figure 2C shows representative slices of pERK-positive cells from the ventral and dorsal striatum of RasGRF2 KO and WT mice administered saline or nicotine.
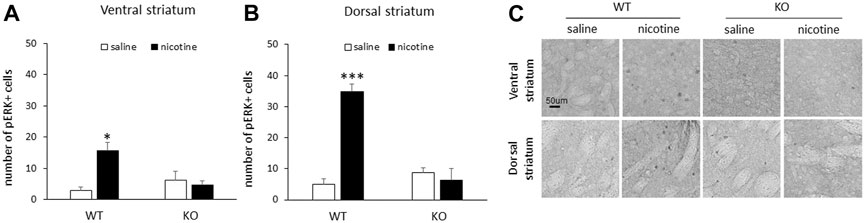
FIGURE 2. Acute nicotine failed to increase striatal pERK in male RasGRF2 KO mice. (A) An acute injection of nicotine (0.4 mg/kg IP) resulted in a significant increase in the number of pERK1/2-positive cells relative to saline controls in the ventral striatum of male RasGRF2 WT, but not KO mice (n = 3–4/group). Data represent the mean number of pERK1/2-positive cells (±SEM) in each condition. (B) An acute injection of nicotine (0.4 mg/kg IP) resulted in a significant increase in the number of pERK1/2-positive cells relative to saline controls in the dorsal striatum of male RasGRF2 WT, but not KO mice (n = 3–4/group). Data represent the mean number of pERK1/2-positive cells (±SEM) in each condition. (C) Representative slices showing pERK-positive cells from the ventral and dorsal striatum of male RasGRF2 WT and KO mice that received acute nicotine or saline injections. *p < 0.025; ***p < 0.0005.
pERK is significantly increased in the striatum following repeated nicotine in male WT, but not RasGRF2 KO mice
Following repeated nicotine exposure, male WT mice demonstrated an increase in the number of pERK1/2-positive cells in response to nicotine relative to saline controls in both the ventral and dorsal striatum. This increase in the number of pERK1/2-positive cells was not present in either the ventral or dorsal striatum of RasGRF2 KO mice (n = 4–5/group). Figure 3A shows the mean number of pERK1/2-positive cells (±SEM) in the ventral striatum in RasGRF2 KO and WT mice sacrificed on day 10 following repeated injections. A two-way ANOVA (genotype × treatment) of the number of pERK1/2-positive cells revealed significant main effects of genotype [F (1, 14) = 25.0, p < 0.0005] and treatment [F (1, 14) = 8.4, p = 0.012], and a significant genotype × treatment interaction [F (1, 14) = 7.5, p = 0.016]. Independent samples t-tests revealed a significant increase in the number of pERK1/2-positive cells in WT mice administered nicotine relative to saline-treated mice [t (7) = 3.3, p = 0.013; Bonferroni-corrected α/2 = 0.025] that was not present in KO mice [t (7) = 0.15, p = 0.88; Bonferroni-corrected α/2 = 0.025]. Figure 3B shows the mean number of pERK1/2-positive cells (±SEM) in the dorsal striatum in RasGRF2 KO and WT mice sacrificed on day 10 following repeated injections. A two-way ANOVA (genotype × treatment) of the number of pERK1/2-positive cells revealed significant main effects of genotype [F (1, 14) = 7.8, p = 0.014] and treatment [F (1, 14) = 12.3, p = 0.003], but no significant genotype × treatment interaction [F (1, 14) = 0.51, p = 0.49]. Independent samples t-tests revealed a significant increase in the number of pERK1/2-positive cells in WT mice administered nicotine relative to saline-treated mice [t (7) = 3.6, p = 0.008; Bonferroni-corrected α/2 = 0.025] that was not present in KO mice [t (7) = 1.7, p = 0.12; Bonferroni-corrected α/2 = 0.025]. Figure 3C shows representative slices of pERK-positive cells from the ventral and dorsal striatum of RasGRF2 KO and WT mice administered repeated saline or nicotine.
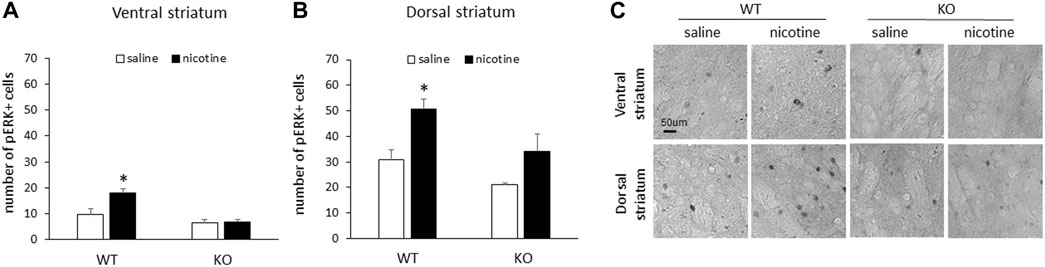
FIGURE 3. Repeated nicotine failed to increase striatal pERK in male RasGRF2 KO mice. (A) 10 days of nicotine (0.4 mg/kg IP) injections resulted in a significant increase in the number of pERK1/2-positive cells relative to saline controls in the ventral striatum of male RasGRF2 WT, but not KO mice (n = 4–5/group). Data represent the mean number of pERK1/2-positive cells (±SEM) in each condition. (B) 10 days of nicotine (0.4 mg/kg IP) injections resulted in a significant increase in the number of pERK1/2-positive cells relative to saline controls in the dorsal striatum of male RasGRF2 WT, but not KO mice (n = 4–5/group). Data represent the mean number of pERK1/2-positive cells (±SEM) in each condition. (C) Representative slices showing pERK-positive cells from the ventral and dorsal striatum of RasGRF2 WT and KO mice that received acute nicotine or saline injections. *p < 0.025.
Nicotine self-administration is increased in male and female RasGRF2 KO mice
RasGRF2 KO and WT mice differed in nicotine SA, with RasGRF2 KO mice demonstrating an increase in responding on the nicotine-associated lever and nicotine intake. A three-way ANOVA (sex × genotype × day) of days 3–10 revealed no significant sex effects on nicotine intake [sex: F < 1; sex × genotype: F < 1; sex × day: F (3.6, 152.7) = 1.5, p = 0.22; sex × genotype × day: F (3.6, 152.7) = 2.0, p = 0.10]. Thus, the data for male and female mice were combined for subsequent analyses (n = 23 mice/group). Figure 4A shows the mean (±SEM) responding on the active and inactive levers during 10 daily sessions of nicotine SA in RasGRF2 KO mice and WT controls. A three-way ANOVA of lever responses during 1 h sessions on days 3–10 (lever × day × genotype) revealed a significant main effect of lever [F (1, 44) = 145.7, p < 0.0005], indicating a distinction between the active and inactive levers, and a significant effect of day [F (4.1, 181.1) = 6.8, p < 0.0005] and day × lever interaction [F (4.0, 173.9) = 13.1, p < 0.0005]. Most importantly, there was a significant main effect of genotype [F (1, 44) = 11.3, p = 0.002], as well as a significant lever × genotype interaction [F (1, 44) = 6.5, p = 0.015], indicating a difference in responding between KO and WT controls over the final 8 days of nicotine SA. No other effects reached significance [day × genotype: F (4.1, 181.1) = 1.8, p = 0.14; lever × day × genotype: F (4.0, 173.9) = 1.6, p = 0.18]. Independent samples t-tests confirmed that RasGRF2 KO mice responded significantly more on the active lever than WT mice [t (44) = 3.3, p = 0.002; Bonferroni-corrected α = 0.025] across days 3–10, but the two groups did not differ on inactive lever pressing [t (44) = 1.9, p = 0.066; Bonferroni-corrected α = 0.025], indicating a selective increase in responding on the nicotine-associated lever by RasGRF2 KO mice relative to WT controls. Figure 4B shows the mean (±SEM) number of nicotine infusions received during 10 days of nicotine SA in RasGRF2 KO mice and WT controls. A two-way ANOVA of nicotine reinforcers during 1 h sessions on days 3–10 (day × genotype) revealed significant main effects of genotype [F (1, 44) = 9.5, p = 0.004], and day [F (3.7, 163.4) = 13.2, p < 0.0005], but no day × genotype interaction [F (3.7, 163.4) = 1.8, p = 0.13]. These findings indicate a significant increase in nicotine intake in RasGRF2 KO mice relative to WT controls over the final 8 days of nicotine SA.
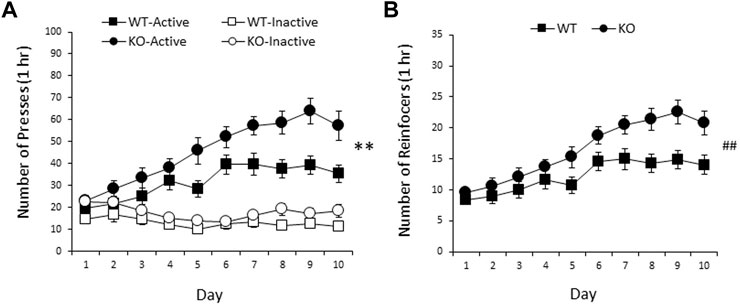
FIGURE 4. Nicotine SA in male and female RasGRF2 KO mice and WT controls. (A) RasGRF2 KO mice demonstrated an increase in responding on the nicotine-associated lever relative to WT controls (n = 23/group). Data represent mean number of presses (±SEM) on the active and inactive levers during 10 daily 1 h sessions of nicotine SA (0.03 mg/kg/35 µl infusion). (B) RasGRF2 KO mice demonstrated an increase in the number of nicotine reinforcers achieved relative to WT controls. Data represent mean number of nicotine reinforcers (±SEM) achieved during 10 daily 1 h sessions of nicotine SA (0.03 mg/kg/35 µl infusion). **p < 0.005; ##p < 0.005, main effect of genotype.
Discussion
Here we sought to extend our previous findings of the contribution of RasGRF2 to cocaine SA using nicotine SA in RasGRF2 KO. In the current study we used the peripheral administration of nicotine to demonstrate that nicotine reliably increases pERK1/2 in the striatum following both acute and repeated injections, an effect not present in RasGRF2 KO mice. Furthermore, RasGRF2 KO mice demonstrated an increase in nicotine SA relative to WT mice. These data suggest that RasGRF2 is involved in the reinforcing properties of nicotine likely via its regulation of activity of the Ras-ERK pathway. Furthermore, these data and those of previous studies of alcohol and cocaine SA further implicate the Ras-ERK signaling pathway as a common mediator of drug reinforcement.
Our demonstration of an increase in the number of pERK1/2-positive cells in the striatum of C57Bl/6N mice following acute nicotine as compared to saline administration is consistent with previous reports that showed acute nicotine-induced increases in ERK1/2 in the striatum using an identical dose of nicotine (Valjent et al., 2004; Valjent et al., 2005). Furthermore, although our focus here was on striatal regions, due to the fact that both the ventral and dorsal striatum have been demonstrated to play a role in the rewarding/reinforcing properties of drugs of abuse, including nicotine (Pascual et al., 2009; Veeneman et al., 2012; Veeneman et al., 2015), acute nicotine has also previously been demonstrated to increase pERK1/2 in several other brain areas that are important for drug-mediated neuroadaptations and consequent drug-seeking responses, such as the PFC, central nucleus of the amygdala, and bed nucleus of the stria terminalis (Valjent et al., 2004; Koob and Volkow, 2010).
RasGRF2 WT mice demonstrated an acute nicotine-induced increase in pERK1/2 in the ventral striatum, consistent with our findings above and previous reports (Valjent et al., 2004; Valjent et al., 2005). RasGRF2 KO mice failed to show this increase. In addition, nicotine also induced an increase in pERK1/2-positive cells relative to saline-treated controls in the dorsal striatum in RasGRF2 WT mice, also absent in RasGRF2 KO mice. These findings suggest that the attenuation of an acute nicotine-induced increase in pERK1/2 is at least partly mediated through the Ras-ERK pathway via RasGRF2. These findings are also consistent with a general role of striatal Ras-ERK signaling in the acute effects of a variety of drugs of abuse. For example, we previously demonstrated that an injection of acute cocaine in mice resulted in an increase in pERK1/2 that was impaired when preceded by administration of the mitogen-activated protein kinase (MEK; an upstream regulator of ERK) inhibitor PD325901 (Papale et al., 2016; see also Valjent et al., 2000). Similar results demonstrating MEK inhibitor attenuation of drug-mediated increases in pERK in striatal regions in rodents have been found with other drugs of abuse, including amphetamine (Shi and McGinty, 2006), morphine (Mouledous et al., 2007), and alcohol (R.E. Bernardi, unpublished results), suggesting a critical role of the Ras-ERK signaling pathway in the cellular response to drugs of abuse.
Following 10 days of peripheral nicotine administration, RasGRF2 WT mice demonstrated a significant increase in pERK1/2 in both the ventral and dorsal striatum, similar to our findings above following acute injections. RasGRF2 KO mice failed to show this increase in the ventral or dorsal striatum, although in the dorsal striatum there was a modest increase in pERK1/2 that failed to reach significance, due to a single animal showing a high pERK1/2 expression following nicotine administration. These findings again suggest that nicotine produces reliable increases in pERK1/2 in brain areas associated with the effects of drugs of abuse, and implicate RasGRF2 and the Ras-ERK pathway as a likely mediator of these effects. A previous study found no differences in pERK1 or pERK2 in the NAc following chronic administration, though differences in hypothesis and subsequent study design (nicotine in drinking water) likely explain this contrasting result (Brunzell et al., 2003). In fact, Yang et al. (2021) recently demonstrated that 7 days of peripheral nicotine injections in rats resulted in an increase in pERK1 and pERK2 in the NAc relative to saline controls. Furthermore, a loss of nicotine-induced increases in pERK has been correlated with impairments in nicotine CPP (Wilar et al., 2019; Jia et al., 2021).
Nicotine SA in RasGRF2 KO mice resulted in an increase in SA relative to WT mice. These data replicate our previous findings of cocaine SA in RasGRF2 KO and WT mice (Bernardi et al., 2019). Given our pERK1/2 findings, this result likely represents a compensatory increase in intake resulting from decreased nicotine reward resulting from the RasGRF2 KO—a decrease in the magnitude of the nicotine reinforcer that requires an increase in intake to achieve a similar putative subjective effect—similar to attenuation of reward via decreases in dose or pharmacological means that results in compensatory increases in intake (De Wit and Wise, 1977; Ettenberg et al., 1982; Caine and Koob, 1994; Thomsen and Caine, 2006; Faccidomo et al., 2009). For example, Faccidomo et al. (2009) demonstrated that the MEK inhibitor SL327 administered prior to daily sessions increased alcohol SA (Faccidomo et al., 2009), a result also interpreted as an increase in intake to compensate for decreased alcohol reward. These findings are also consistent with a previous result showing a loss in alcohol-induced dopamine increase in the NAc and dorsal striatum in RasGRF2 KO mice relative to WT controls, resulting in decreased alcohol reinforcement (Stacey et al., 2012). Thus, RasGRF2 likely plays a general modulatory role in drug-mediated behaviors.
In the SA study outlined here, both male and female RasGRF2 KO and WT mice were used to identify potential sex-dependent differences in nicotine SA and its mediation by RasGRF2. Previous studies of nicotine SA in rodents have demonstrated either increased intake in males, no differences in intake between males and females, or higher intake in females relative to males (Donny et al., 2000; Feltenstein et al., 2012; Bernardi et al., 2016; Flores et al., 2019; Chen et al., 2020; Chellian et al., 2021; Leyrer-Jackson et al., 2021). Here we found no sex-dependent differences in nicotine intake in WT mice; however, we did not measure other specific aspects of nicotine SA that may differ among males and females, such as motivation, extended-access procedures, reinstatement, and treatment effectiveness, which are likely relevant to clinical outcomes in humans. We also found no sex-dependent difference in the alteration in nicotine intake as a function of the RasGRF2 KO. We do not know of any previous rodent study that has examined the effect of manipulation of components of the Ras-ERK pathway on measures of drug reinforcement in both males and females, although one previous cocaine conditioned place preference study demonstrated that following testing, pERK levels in the NAc and preference scores did not differ between male and female rats (Nygard et al., 2013). Thus, RasGRF2 mediation of nicotine SA is likely sex-independent.
Previous studies have implicated ERK as a coincident detector of D1R-mediated signaling and NMDA receptor activation in the striatum critical to the regulation of drug-dependent synaptic plasticity in the striatum (Zhang et al., 2004; Valjent et al., 2005; Jiao et al., 2007; Bertran-Gonzalez et al., 2008; Fasano et al., 2009; Ren et al., 2010; Morella et al., 2020). For example, both NMDA and D1 antagonists have been shown to block ERK phosphorylation in the striatum in response to a variety of drugs of abuse (Valjent et al., 2000; Valjent et al., 2004; Valjent et al., 2005). The striatal increase in pERK induced by drugs of abuse was also attenuated in D1 KO mice relative to controls (Valjent et al., 2005). Furthermore, previous studies have implicated RasGRF2 in the synaptic plasticity associated with both NMDA- (Tian et al., 2004; Jin and Feig, 2010; Jin et al., 2014) and D1-mediated stimulation (Stacey et al., 2012). Thus, in terms of nicotine and other drugs of abuse, RasGRF2 likely mediates drug reward via the activity of ERK in response to D1- and NMDA-mediated signaling.
It should be noted that although our results here are consistent with those of our previous cocaine paper using RasGRF2 KO mice (Bernardi et al., 2019), in that nicotine SA was increased in RasGRF2 KO mice relative to WT controls and KO mice failed to show the increase in pERK1/2 in response to nicotine demonstrated in WT mice, we used peripheral injections of nicotine for our acute and chronic immunohistochemical findings. Although the dose of nicotine for IP injection was virtually identical to the amount achieved via daily SA (based on mean intake), our peripheral nicotine injections do not represent a measure of reward with which to directly link changes in pERK1/2 expression. Thus it is possible that changes in nicotine-induced pERK1/2 expression in the striatum do not reflect alterations in reinforcement associated with nicotine SA. However, previous work has implicated alterations in pERK1/2 expression with changes in nicotine reward (Wilar et al., 2019; Jia et al., 2021). For example, Wilar et al. (2019) demonstrated that pERK1/2 was increased following nicotine CPP in WT mice relative to vehicle-treated animals, but this increase in pERK1/2 expression and subsequent nicotine CPP was absent in Dopamine D2 receptor KO mice. Furthermore, in our previous cocaine paper, in which we demonstrated a direct link between cocaine reinforcement and the expression of pERK1/2 (Bernardi et al., 2019; see also Papale et al., 2016), we also demonstrated that the behavioral effect of pERK1/2 inhibition on SA was dependent on whether the manipulation was peripheral/global or site-specific. In other words, both the RasGRF2 KO and peripheral administration of the MEK inhibitor PD325901 resulted in an increase in cocaine intake, while viral-mediated RasGRF2 knockdown and MEK inhibition in the NAc both resulted in a decrease in cocaine SA. Further studies will need to determine the precise mechanisms by which these global and site-specific findings produce differing effects on behavior but an identical inhibition of the expression of pERK1/2, and whether similar findings occur with nicotine SA. Nonetheless, these findings suggest that alterations in pERK may mediate drug intake.
In summary, we demonstrated that RasGRF2 is involved in nicotine reinforcement associated with operant SA, likely by regulating the activity of ERK. In combination with previous findings, these studies further implicate a common mechanism for the Ras-ERK pathway in the effects of drugs of abuse.
Data availability statement
The raw data supporting the conclusion of this article will be made available by the authors, without undue reservation.
Ethics statement
The animal study was reviewed and approved by the Regierungspräsidium Karlsruhe.
Author contributions
IM was involved in the acquisition, analysis, and interpretation of data, as well as drafting the manuscript. VP was involved in the acquisition of data and drafting the manuscript. CC-L was involved in the acquisition, analysis, and interpretation of data. RB and RS were involved in the interpretation of data and drafting the manuscript. REB was involved in the conception, design, acquisition, analysis and interpretation of data, and drafting the manuscript.
Funding
Financial support for this work was provided by the Bundesministerium für Bildung und Forschung (BMBF)-funded SysMedSUDs consortium (FKZ: 01ZX1909A) and the Deutsche Forschungsgemeinschaft (DFG, German Research Foundation)—Project-ID 402170461—TRR 265 (Heinz et al., 2020).
Conflict of interest
The authors declare that the research was conducted in the absence of any commercial or financial relationships that could be construed as a potential conflict of interest.
Publisher’s note
All claims expressed in this article are solely those of the authors and do not necessarily represent those of their affiliated organizations, or those of the publisher, the editors and the reviewers. Any product that may be evaluated in this article, or claim that may be made by its manufacturer, is not guaranteed or endorsed by the publisher.
References
Agoglia, A. E., Sharko, A. C., Psilos, K. E., Holstein, S. E., Reid, G. T., and Hodge, C. W. (2015). Alcohol alters the activation of ERK1/2, a functional regulator of binge alcohol drinking in adult C57BL/6J mice. Alcohol. Clin. Exp. Res. 39 (3), 463–475. doi:10.1111/acer.12645
Bernardi, R. E., Olevska, A., Morella, I., Fasano, S., Santos, E., Brambilla, R., et al. (2019). The inhibition of RasGRF2, but not RasGRF1, alters cocaine reward in mice. J. Neurosci. 39 (32), 6325–6338. doi:10.1523/JNEUROSCI.1120-18.2019
Bernardi, R. E., and Spanagel, R. (2013). The ClockΔ19 mutation in mice fails to alter the primary and secondary reinforcing properties of nicotine. Drug Alcohol Depend. 133 (2), 733–739. doi:10.1016/j.drugalcdep.2013.08.024
Bernardi, R. E., Zohsel, K., Hirth, N., Treutlein, J., Heilig, M., Laucht, M., et al. (2016). A gene-by-sex interaction for nicotine reward: Evidence from humanized mice and epidemiology. Transl. Psychiatry 6 (7), e861. doi:10.1038/tp.2016.132
Bertran-Gonzalez, J., Bosch, C., Maroteaux, M., Matamales, M., Herve, D., Valjent, E., et al. (2008). Opposing patterns of signaling activation in dopamine D1 and D2 receptor-expressing striatal neurons in response to cocaine and haloperidol. J. Neurosci. 28 (22), 5671–5685. doi:10.1523/JNEUROSCI.1039-08.2008
Brunzell, D. H., Russell, D. S., and Picciotto, M. R. (2003). In vivo nicotine treatment regulates mesocorticolimbic CREB and ERK signaling in C57Bl/6J mice. J. Neurochem. 84 (6), 1431–1441. doi:10.1046/j.1471-4159.2003.01640.x
Caine, S. B., and Koob, G. F. (1994). Effects of dopamine D-1 and D-2 antagonists on cocaine self-administration under different schedules of reinforcement in the rat. J. Pharmacol. Exp. Ther. 270 (1), 209–218.
Cerovic, M., d'Isa, R., Tonini, R., and Brambilla, R. (2013). Molecular and cellular mechanisms of dopamine-mediated behavioral plasticity in the striatum. Neurobiol. Learn. Mem. 105, 63–80. doi:10.1016/j.nlm.2013.06.013
Chellian, R., Behnood-Rod, A., Wilson, R., and Bruijnzeel, A. W. (2021). Rewarding effects of nicotine self-administration increase over time in male and female rats. Nicotine Tob. Res. 23 (12), 2117–2126. doi:10.1093/ntr/ntab097
Chen, Y. C., Fowler, J. P., Wang, J., Watson, C. J. W., Sherafat, Y., Staben, A., et al. (2020). The novel CYP2A6 inhibitor, DLCI-1, decreases nicotine self-administration in mice. J. Pharmacol. Exp. Ther. 372 (1), 21–29. doi:10.1124/jpet.119.260653
Davidson, K. R., Fox, D. L., and Smith, M. L. (2020). More on the pathology of vaping-associated lung injury. Reply. N. Engl. J. Med. 382 (4), 388–390. doi:10.1056/NEJMc1914980
De Wit, H., and Wise, R. A. (1977). Blockade of cocaine reinforcement in rats with the dopamine receptor blocker pimozide, but not with the noradrenergic blockers phentolamine or phenoxybenzamine. Can. J. Psychol. 31 (4), 195–203. doi:10.1037/h0081662
Donny, E. C., Caggiula, A. R., Rowell, P. P., Gharib, M. A., Maldovan, V., Booth, S., et al. (2000). Nicotine self-administration in rats: Estrous cycle effects, sex differences and nicotinic receptor binding. Psychopharmacol. Berl. 151 (4), 392–405. doi:10.1007/s002130000497
Ettenberg, A., Pettit, H. O., Bloom, F. E., and Koob, G. F. (1982). Heroin and cocaine intravenous self-administration in rats: Mediation by separate neural systems. Psychopharmacol. Berl. 78 (3), 204–209. doi:10.1007/BF00428151
Faccidomo, S., Besheer, J., Stanford, P. C., and Hodge, C. W. (2009). Increased operant responding for ethanol in male C57bl/6J mice: Specific regulation by the ERK1/2, but not JNK, MAP kinase pathway. Psychopharmacol. Berl. 204 (1), 135–147. doi:10.1007/s00213-008-1444-9
Faccidomo, S., Salling, M. C., Galunas, C., and Hodge, C. W. (2015). Operant ethanol self-administration increases extracellular-signal regulated protein kinase (ERK) phosphorylation in reward-related brain regions: Selective regulation of positive reinforcement in the prefrontal cortex of C57bl/6J mice. Psychopharmacol. Berl. 232 (18), 3417–3430. doi:10.1007/s00213-015-3993-z
Fasano, S., D'Antoni, A., Orban, P. C., Valjent, E., Putignano, E., Vara, H., et al. (2009). Ras-guanine nucleotide-releasing factor 1 (Ras-GRF1) controls activation of extracellular signal-regulated kinase (ERK) signaling in the striatum and long-term behavioral responses to cocaine. Biol. Psychiatry 66 (8), 758–768. doi:10.1016/j.biopsych.2009.03.014
Feig, L. A. (2011). Regulation of neuronal function by ras-GRF exchange factors. Genes Cancer 2 (3), 306–319. doi:10.1177/1947601911408077
Feltenstein, M. W., Ghee, S. M., and See, R. E. (2012). Nicotine self-administration and reinstatement of nicotine-seeking in male and female rats. Drug Alcohol Depend. 121 (3), 240–246. doi:10.1016/j.drugalcdep.2011.09.001
Fernandez-Medarde, A., Esteban, L. M., Nunez, A., Porteros, A., Tessarollo, L., and Santos, E. (2002). Targeted disruption of Ras-Grf2 shows its dispensability for mouse growth and development. Mol. Cell. Biol. 22 (8), 2498–2504. doi:10.1128/mcb.22.8.2498-2504.2002
Flores, R. J., Uribe, K. P., Swalve, N., and O'Dell, L. E. (2019). Sex differences in nicotine intravenous self-administration: A meta-analytic review. Physiol. Behav. 203, 42–50. doi:10.1016/j.physbeh.2017.11.017
Girault, J. A., Valjent, E., Caboche, J., and Herve, D. (2007). ERK2: A logical and gate critical for drug-induced plasticity? Curr. Opin. Pharmacol. 7 (1), 77–85. doi:10.1016/j.coph.2006.08.012
Glynos, C., Bibli, S. I., Katsaounou, P., Pavlidou, A., Magkou, C., Karavana, V., et al. (2018). Comparison of the effects of e-cigarette vapor with cigarette smoke on lung function and inflammation in mice. Am. J. Physiol. Lung Cell. Mol. Physiol. 315 (5), L672. doi:10.1152/ajplung.00389.2017
Gomez, A. M., Sun, W. L., Midde, N. M., Harrod, S. B., and Zhu, J. (2015). Effects of environmental enrichment on ERK1/2 phosphorylation in the rat prefrontal cortex following nicotine-induced sensitization or nicotine self-administration. Eur. J. Neurosci. 41 (1), 109–119. doi:10.1111/ejn.12758
Grewal, S. S., York, R. D., and Stork, P. J. S. (1999). Extracellular-signal-regulated kinase signalling in neurons. Curr. Opin. Neurobiol. 9 (5), 544–553. doi:10.1016/s0959-4388(99)00010-0
Heinz, A., Kiefer, F., Smolka, M. N., Endrass, T., Beste, C., Beck, A., et al. (2020). Addiction Research Consortium: Losing and regaining control over drug intake (ReCoDe)-From trajectories to mechanisms and interventions. Addict. Biol. 25 (2), e12866. doi:10.1111/adb.12866
Jia, W., Wilar, G., Kawahata, I., Cheng, A., and Fukunaga, K. (2021). Impaired acquisition of nicotine-induced conditioned place preference in fatty acid-binding protein 3 null mice. Mol. Neurobiol. 58 (5), 2030–2045. doi:10.1007/s12035-020-02228-2
Jiao, H., Zhang, L., Gao, F., Lou, D., Zhang, J., and Xu, M. (2007). Dopamine D(1) and D(3) receptors oppositely regulate NMDA- and cocaine-induced MAPK signaling via NMDA receptor phosphorylation. J. Neurochem. 103 (2), 840–848. doi:10.1111/j.1471-4159.2007.04840.x
Jin, S. X., Bartolome, C., Arai, J. A., Hoffman, L., Uzturk, B. G., Kumar-Singh, R., et al. (2014). Domain contributions to signaling specificity differences between Ras-guanine nucleotide releasing factor (Ras-GRF) 1 and Ras-GRF2. J. Biol. Chem. 289 (23), 16551–16564. doi:10.1074/jbc.M114.557959
Jin, S. X., and Feig, L. A. (2010). Long-term potentiation in the CA1 hippocampus induced by NR2A subunit-containing NMDA glutamate receptors is mediated by Ras-GRF2/Erk map kinase signaling. PLoS One 5 (7), e11732. doi:10.1371/journal.pone.0011732
Konu, O., Kane, J. K., Barrett, T., Vawter, M. P., Chang, R., Ma, J. Z., et al. (2001). Region-specific transcriptional response to chronic nicotine in rat brain. Brain Res. 909 (1-2), 194–203. doi:10.1016/s0006-8993(01)02685-3
Koob, G. F., and Volkow, N. D. (2010). Neurocircuitry of addiction. Neuropsychopharmacology 35 (1), 217–238. doi:10.1038/npp.2009.110
Leventhal, A. M., Strong, D. R., Kirkpatrick, M. G., Unger, J. B., Sussman, S., Riggs, N. R., et al. (2015). Association of electronic cigarette use with initiation of combustible tobacco product smoking in early adolescence. JAMA 314 (7), 700–707. doi:10.1001/jama.2015.8950
Leyrer-Jackson, J. M., Overby, P. F., Bull, A., Marusich, J. A., and Gipson, C. D. (2021). Strain and sex matters: Differences in nicotine self-administration between outbred and recombinase-driver transgenic rat lines. Exp. Clin. Psychopharmacol. 29 (4), 375–384. doi:10.1037/pha0000376
Li, S., Li, Z., Pei, L., Le, A. D., and Liu, F. (2012). The α7nACh-NMDA receptor complex is involved in cue-induced reinstatement of nicotine seeking. J. Exp. Med. 209 (12), 2141–2147. doi:10.1084/jem.20121270
Lobo, M. K., and Nestler, E. J. (2011). The striatal balancing act in drug addiction: Distinct roles of direct and indirect pathway medium spiny neurons. Front. Neuroanat. 5, 41. doi:10.3389/fnana.2011.00041
Mazzucchelli, C., and Brambilla, R. (2000). Ras-related and MAPK signalling in neuronal plasticity and memory formation. Cell. Mol. Life Sci. 57 (4), 604–611. doi:10.1007/PL00000722
Morella, I., Hallum, H., and Brambilla, R. (2020). Dopamine D1 and glutamate receptors Co-operate with brain-derived neurotrophic factor (BDNF) and TrkB to modulate ERK signaling in adult striatal slices. Front. Cell. Neurosci. 14, 564106. doi:10.3389/fncel.2020.564106
Mouledous, L., Diaz, M. F., and Gutstein, H. B. (2007). Extracellular signal-regulated kinase (ERK) inhibition does not prevent the development or expression of tolerance to and dependence on morphine in the mouse. Pharmacol. Biochem. Behav. 88 (1), 39–46. doi:10.1016/j.pbb.2007.07.002
Nygard, S. K., Klambatsen, A., Hazim, R., Eltareb, M. H., Blank, J. C., Chang, A. J., et al. (2013). Sexually dimorphic intracellular responses after cocaine-induced conditioned place preference expression. Brain Res. 1520, 121–133. doi:10.1016/j.brainres.2013.04.060
Olevska, A., Spanagel, R., and Bernardi, R. E. (2021). Impaired contextual fear conditioning in RasGRF2 mutant mice is likely Ras-ERK-dependent. Neurobiol. Learn. Mem. 181, 107435. doi:10.1016/j.nlm.2021.107435
Orton, R. J., Sturm, O. E., Vyshemirsky, V., Calder, M., Gilbert, D. R., and Kolch, W. (2005). Computational modelling of the receptor-tyrosine-kinase-activated MAPK pathway. Biochem. J. 392 (2), 249–261. doi:10.1042/BJ20050908
Pamonsinlapatham, P., Hadj-Slimane, R., Lepelletier, Y., Allain, B., Toccafondi, M., Garbay, C., et al. (2009). p120-Ras GTPase activating protein (RasGAP): a multi-interacting protein in downstream signaling. Biochimie 91 (3), 320–328. doi:10.1016/j.biochi.2008.10.010
Papale, A., Morella, I. M., Indrigo, M. T., Bernardi, R. E., Marrone, L., Marchisella, F., et al. (2016). Impairment of cocaine-mediated behaviours in mice by clinically relevant Ras-ERK inhibitors. Elife 5, e17111. doi:10.7554/eLife.17111
Park, E., Livingston, J. A., Wang, W., Kwon, M., Eiden, R. D., and Chang, Y. P. (2020). Adolescent E-cigarette use trajectories and subsequent alcohol and marijuana use. Addict. Behav. 103, 106213. doi:10.1016/j.addbeh.2019.106213
Pascual, M. M., Pastor, V., and Bernabeu, R. O. (2009). Nicotine-conditioned place preference induced CREB phosphorylation and Fos expression in the adult rat brain. Psychopharmacol. Berl. 207 (1), 57–71. doi:10.1007/s00213-009-1630-4
Quilliam, L. A., Rebhun, J. F., and Castro, A. F. (2002). A growing family of guanine nucleotide exchange factors is responsible for activation of Ras-family GTPases. Prog. Nucleic Acid. Res. Mol. Biol. 71, 391–444. doi:10.1016/s0079-6603(02)71047-7
Raja, J., Khouzam, A., Khouzam, N., and Khouzam, R. N. (2021). Smoke and heart should stay apart: A look at E cigarettes and other alternatives to conventional cigarettes, and their impact on cardiovascular health. Curr. Probl. Cardiol. 46 (3), 100640. doi:10.1016/j.cpcardiol.2020.100640
Ren, M., and Lotfipour, S. (2019). Nicotine gateway effects on adolescent substance use. West. J. Emerg. Med. 20 (5), 696–709. doi:10.5811/westjem.2019.7.41661
Ren, Z., Sun, W. L., Jiao, H., Zhang, D., Kong, H., Wang, X., et al. (2010). Dopamine D1 and N-methyl-D-aspartate receptors and extracellular signal-regulated kinase mediate neuronal morphological changes induced by repeated cocaine administration. Neuroscience 168 (1), 48–60. doi:10.1016/j.neuroscience.2010.03.034
Sanna, P. P., Simpson, C., Lutjens, R., and Koob, G. (2002). ERK regulation in chronic ethanol exposure and withdrawal. Brain Res. 948 (1-2), 186–191. doi:10.1016/s0006-8993(02)03191-8
Shi, X., and McGinty, J. F. (2006). Extracellular signal-regulated mitogen-activated protein kinase inhibitors decrease amphetamine-induced behavior and neuropeptide gene expression in the striatum. Neuroscience 138 (4), 1289–1298. doi:10.1016/j.neuroscience.2005.12.024
Stacey, D., Bilbao, A., Maroteaux, M., Jia, T., Easton, A. C., Longueville, S., et al. (2012). RASGRF2 regulates alcohol-induced reinforcement by influencing mesolimbic dopamine neuron activity and dopamine release. Proc. Natl. Acad. Sci. U. S. A. 109 (51), 21128–21133. doi:10.1073/pnas.1211844110
Stornetta, R. L., and Zhu, J. J. (2011). Ras and Rap signaling in synaptic plasticity and mental disorders. Neuroscientist 17 (1), 54–78. doi:10.1177/1073858410365562
Takai, Y., Sasaki, T., and Matozaki, T. (2001). Small GTP-binding proteins. Physiol. Rev. 81 (1), 153–208. doi:10.1152/physrev.2001.81.1.153
Thomsen, M., and Caine, S. B. (2006). Cocaine self-administration under fixed and progressive ratio schedules of reinforcement: Comparison of C57bl/6J, 129x1/SvJ, and 129S6/SvEvTac inbred mice. Psychopharmacol. Berl. 184 (2), 145–154. doi:10.1007/s00213-005-0207-0
Tian, X., Gotoh, T., Tsuji, K., Lo, E. H., Huang, S., and Feig, L. A. (2004). Developmentally regulated role for Ras-GRFs in coupling NMDA glutamate receptors to Ras, Erk and CREB. EMBO J. 23 (7), 1567–1575. doi:10.1038/sj.emboj.7600151
Valjent, E., Corvol, J. C., Pages, C., Besson, M. J., Maldonado, R., and Caboche, J. (2000). Involvement of the extracellular signal-regulated kinase cascade for cocaine-rewarding properties. J. Neurosci. 20 (23), 8701–8709. doi:10.1523/jneurosci.20-23-08701.2000
Valjent, E., Corvol, J. C., Trzaskos, J. M., Girault, J. A., and Herve, D. (2006). Role of the ERK pathway in psychostimulant-induced locomotor sensitization. BMC Neurosci. 7, 20. doi:10.1186/1471-2202-7-20
Valjent, E., Pages, C., Herve, D., Girault, J. A., and Caboche, J. (2004). Addictive and non-addictive drugs induce distinct and specific patterns of ERK activation in mouse brain. Eur. J. Neurosci. 19 (7), 1826–1836. doi:10.1111/j.1460-9568.2004.03278.x
Valjent, E., Pascoli, V., Svenningsson, P., Paul, S., Enslen, H., Corvol, J. C., et al. (2005). Regulation of a protein phosphatase cascade allows convergent dopamine and glutamate signals to activate ERK in the striatum. Proc. Natl. Acad. Sci. U. S. A. 102 (2), 491–496. doi:10.1073/pnas.0408305102
Veeneman, M. M., Broekhoven, M. H., Damsteegt, R., and Vanderschuren, L. J. (2012). Distinct contributions of dopamine in the dorsolateral striatum and nucleus accumbens shell to the reinforcing properties of cocaine. Neuropsychopharmacology 37 (2), 487–498. doi:10.1038/npp.2011.209
Veeneman, M. M., Damsteegt, R., and Vanderschuren, L. J. (2015). The nucleus accumbens shell and the dorsolateral striatum mediate the reinforcing effects of cocaine through a serial connection. Behav. Pharmacol. 26 (1-2), 193–199. doi:10.1097/FBP.0000000000000099
World Health Organization (2021). WHO report on the global tobacco epidemic 2021: Addressing new and emerging products. Geneva: World Health Organization.
Wilar, G., Shinoda, Y., Sasaoka, T., and Fukunaga, K. (2019). Crucial role of dopamine D2 receptor signaling in nicotine-induced conditioned place preference. Mol. Neurobiol. 56 (12), 7911–7928. doi:10.1007/s12035-019-1635-x
Yang, J. H., Sohn, S., Kim, S., Kim, J., Oh, J. H., Ryu, I. S., et al. (2021). Repeated nicotine exposure increases the intracellular interaction between ERK-mGluR5 in the nucleus accumbens more in adult than adolescent rats. Addict. Biol. 26 (2), e12913. doi:10.1111/adb.12913
Zhang, L., Lou, D., Jiao, H., Zhang, D., Wang, X., Xia, Y., et al. (2004). Cocaine-induced intracellular signaling and gene expression are oppositely regulated by the dopamine D1 and D3 receptors. J. Neurosci. 24 (13), 3344–3354. doi:10.1523/JNEUROSCI.0060-04.2004
Keywords: RasGRF2, nicotine, self-administration (SA), pERK, extracellar signal-regulated kinase, pERK1/2
Citation: Morella I, Pohořalá V, Calpe-López C, Brambilla R, Spanagel R and Bernardi RE (2022) Nicotine self-administration and ERK signaling are altered in RasGRF2 knockout mice. Front. Pharmacol. 13:986566. doi: 10.3389/fphar.2022.986566
Received: 05 July 2022; Accepted: 10 August 2022;
Published: 02 September 2022.
Edited by:
Cristina Núñez, University of Murcia, SpainReviewed by:
Sheketha R. Hauser, Indiana University Bloomington, United StatesKabirullah Lutfy, Western University of Health Sciences, United States
Copyright © 2022 Morella, Pohořalá, Calpe-López, Brambilla, Spanagel and Bernardi. This is an open-access article distributed under the terms of the Creative Commons Attribution License (CC BY). The use, distribution or reproduction in other forums is permitted, provided the original author(s) and the copyright owner(s) are credited and that the original publication in this journal is cited, in accordance with accepted academic practice. No use, distribution or reproduction is permitted which does not comply with these terms.
*Correspondence: Rick E. Bernardi, rick.bernardi@zi-mannheim.de
†These authors share first authorship