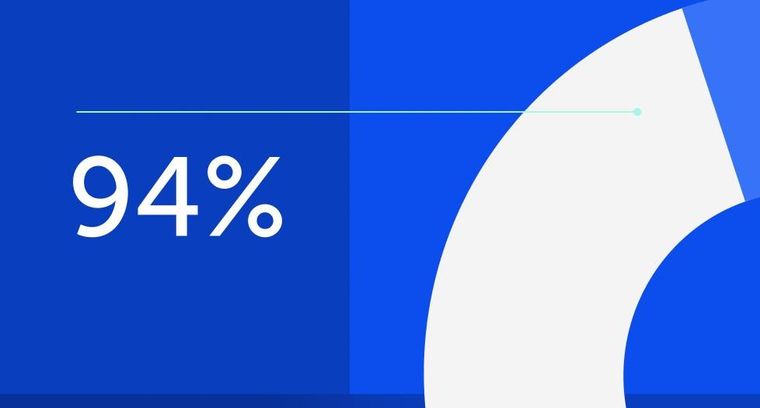
94% of researchers rate our articles as excellent or good
Learn more about the work of our research integrity team to safeguard the quality of each article we publish.
Find out more
BRIEF RESEARCH REPORT article
Front. Pharmacol., 13 September 2022
Sec. Experimental Pharmacology and Drug Discovery
Volume 13 - 2022 | https://doi.org/10.3389/fphar.2022.978337
This article is part of the Research TopicFibrosis: Etiology, Pathophysiology, Measurements, and TherapyView all 19 articles
Lactacystin is a specific proteasome inhibitor that blocks the hydrolysis of intracellular proteins by ubiquitin/proteasome system inhibition. The administration of lactacystin to rats induced hypertension and remodeling of the left ventricle and aorta. This study tested whether lactacystin induces structural and fibrotic rebuilding of the kidneys and whether melatonin and captopril can prevent these potential changes. Six weeks of lactacystin administration to rats increased their average systolic blood pressure (SBP). In the kidneys, lactacystin reduced glomerular density, increased the glomerular tuft area, and enhanced hydroxyproline concentrations. It also elevated the intraglomerular proportion including the amounts of collagen (Col) I and Col III. Lactacystin also raised the tubulointerstitial amounts of Col I and the sum of Col I and Col III with no effect on vascular/perivascular collagen. Six weeks of captopril treatment reduced SBP, while melatonin had no effect. Both melatonin and captopril increased glomerular density, reduced the glomerular tuft area, and lowered the hydroxyproline concentration in the kidneys. Both drugs reduced the proportion and total amounts of intraglomerular and tubulointerstitial Col I and Col III. We conclude that chronic lactacystin treatment stimulated structural and fibrotic remodeling of the kidneys, and melatonin and captopril partly prevented these alterations. Considering the effect of lactacystin on both the heart and kidneys, chronic treatment with this drug may be a prospective model of cardiorenal damage suitable for testing pharmacological drugs as protective agents.
Hypertension and diabetes are the leading causes of chronic kidney disease (CKD) (Hamrahian and Falkner, 2017). The pathogenesis of CKD in hypertension is multifactorial, involving genetic alterations, oxidative stress, endothelial dysfunction, and renin-angiotensin-aldosterone system (RAAS) disturbances (Mennuni et al., 2014). These factors promote inflammation, fibrosis, and both a reduced nephron count and a lower glomerular filtration rate (GFR) (Schlondorff, 2008). CKD is associated with a worsening cardiovascular prognosis and constitutes a serious health and social condition. Thus, there is a continuous search for new ways of protection in experimental models of hypertension.
Cardiovascular homeostasis is tightly bound to proper protein turnover, which is controlled by the ubiquitin-proteasome system (UPS). Proteasomes are multisubunit protease complexes that degrade damaged proteins in all parts of the cells (Pagan et al., 2013). The proteasomes specific to the heart involve a number of proteins with various biological impacts, such as atrogin (known as muscle atrophy F-box) participating in myocardial remodeling (Li et al., 2004); murine double minute 2 (MDM2), a ubiquitin ligase that mediates p53 participating on myocardial hypertrophy modulation (Chatterjee et al., 2011); the calcineurin-nuclear factor of the activated T cells (NFAT) pathway that mediates cardiac remodeling (Tang et al., 2010); sarcomere-associated protein MuRF1 associated with heart failure (Willis et al., 2009); or Nedd4 containing E3 ligase controlling the biological impact of multifunctional vascular endothelial growth factor (VEGF) (Murdaca et al., 2004). In the kidney, Nedd4L/Nedd4-2 seems to participate in distal nephron salt sensitivity (Ishigami et al., 2020), and ubiquitin-conjugating enzyme E2 contributes to HUWE1-mediated degradation of tubulointerstitial fibrosis (Wang et al., 2022). Pharmacological interference with proteasomes is emerging as a potential approach to influence various pathological processes.
Lactacystin is a proteasome inhibitor that blocks the hydrolysis and degradation of intracellular proteins by the UPS (Craiu et al., 1997). Chronic lactacystin administration induces a mild but significant rise in systolic blood pressure (BP) along with fibrotic remodeling of the left ventricle (LV) in Wistar rats (Simko et al., 2017), and hypertrophy of the aorta in L-NAME (L-NG-nitro arginine methyl ester)-induced hypertension (Vrankova et al., 2010). Several hypothetical mechanisms for hemodynamic alterations and heart and vessel damage by lactacystin have been proposed, such as enhanced oxidative stress (Vrankova et al., 2010; Huseby et al., 2016; Parajuli, 2019), decreased NO bioavailability (Simko et al., 2017; Sharma et al., 2020), sympathetic nervous system activation (Congo Carbajosa et al., 2015) and modification of various cytosolic, nuclear, and myofibrillar protein turnovers (Mearini et al., 2008). However, data on lactacystin’s renal effects are not available.
The aim of this study was to test whether lactacystin induces structural and fibrotic rebuilding of the kidneys. Furthermore, we sought to determine whether melatonin prevents these potential alterations. Melatonin (N-acetyl-5-methoxytryptamine), the main product of the pineal gland, not only regulates biological circadian rhythms (Reiter et al., 2020), but exerts various pleiotropic protective effects on the heart, vasculature (Reiter et al., 2010; Simko et al., 2019, 2020; Domínguez-Rodríguez et al., 2021; Tobeiha et al., 2022) and kidneys (Russcher et al., 2012; Hrenák et al., 2013; Hrenak et al., 2015; Shi et al., 2019). The effects of melatonin were compared with captopril, a classical angiotensin-converting enzyme (ACE) inhibitor, which exerts a well-established antiremodeling action in several organs and various pathologies (Pfeffer et al., 1992; Pechánová et al., 1997; Simko et al., 2010, 2014; Repová-Bednárová et al., 2013) including lactacystin-induced LV remodeling (Simko et al., 2017).
The experiments were conducted in conformance with the Guide for the Care and Use of Laboratory Animals published by the US National Institutes of Health (NIH Publication No. 85-23, revised 1996). The study protocol was approved by the ethics committee of the Institute of Pathological Physiology, Faculty of Medicine, at Comenius University in Bratislava, Slovakia.
Thirty-two adult (12-week-old) weight-matched male Wistar rats (obtained from the Department of Toxicology and Laboratory Animals Breeding, Slovak Academy of Sciences, Dobra Voda, Slovakia) were randomly divided into four groups (n = 8 per group) and treated for 6 weeks as follows: control (C; untreated), lactacystin (Lac; 5 μg/kg/day), lactacystin plus captopril (Lac + Cap; 5 μg/kg/day lactacystin + 100 mg/kg/day captopril), and lactacystin plus melatonin (Lac + Mel; 5 μg/kg/day lactacystin + 10 mg/kg/day melatonin). Lactacystin, captopril, and melatonin were dissolved in drinking water and their concentrations were adjusted to daily water consumption to ensure the correct dosage. The melatonin solutions were offered in non-transparent bottles to protect them from light.
Lactacystin and melatonin were purchased from Sigma-Aldrich Chemie, Munich, Germany, and captopril from Egis Pharmaceuticals, Budapest, Hungary.
The rats were housed in individual cages, maintained under standard laboratory conditions (12:12-h light-dark cycle at 22–24°C temperature and 45%–65% humidity), and fed a regular pelleted diet ad libitum. Systolic blood pressure (SBP) was measured once a week in each animal by non-invasive tail-cuff plethysmography (Hugo-Sachs Elektronik, Freiburg, Germany). After 6 weeks of treatment, the rats were euthanized by isoflurane inhalation. Two halves of the left kidney were harvested for subsequent analyses: one half was fixed in 4% formaldehyde for histopathological analysis. The other was snap-frozen at −80°C for determination of hydroxyproline concentration.
The kidney samples fixed in 4% formaldehyde were embedded in paraffin and cut into 5 μm-thick sections. Then, two sets of sections per sample were deparaffinized, rehydrated, and stained, one with hematoxylin-eosin (H-E) for glomerular morphometry, and the other with picrosirius red (PSR) for quantitative analysis of kidney fibrosis. Photomicrographs were taken using a NIKON Eclipse Ti C2+ microscope (NIKON, Tokyo, Japan) with transmitted or polarized light and subsequently analyzed with NIKON NIS-Elements Analysis software (NIKON, Tokyo, Japan) and ImageJ version 1.52p for Windows (National Institutes of Health, Bethesda, MD, United States).
The H-E-stained sections were analyzed using transmitted light microscopy at ×10 magnification and NIKON NIS-Elements Analysis software to assess glomerular morphometry, as previously described (Pechanova et al., 2006; Hrenák et al., 2013; Stanko et al., 2020). Glomerular density was determined by counting preserved glomeruli in a 1 mm2 digital frame put over the kidney cortex at 10 microscopic fields per section; i.e., 80 digital frames were investigated per group. The glomerular tuft area was determined by measuring perpendicular maximum and minimum diameters (dmax and dmin, respectively) of 10 random glomerular tufts per section used for subsequent tuft area calculation as follows: glomerular tuft area = π(dmax/2) (dmin/2); i.e., 80 glomerular tuft areas were calculated per group.
The PSR-stained sections were analyzed with polarized light microscopy, set at ×100 magnification, and ImageJ to allow for a quantitative assessment of kidney fibrosis, as previously described (Seccia et al., 2008; Stanko et al., 2020). Due to the birefringence shift by PSR, the thick type I collagen (Col I) was shown in red-orange shades, and the thin type III collagen (Col III) was visualized in green-yellow shades. Thus, the Col I and Col III volumes were determined as the percentage of red-orange and green-yellow shaded areas in a particular region of interest (ROI) by setting the appropriate “hue” thresholds of the color spectrum in ImageJ. To assess glomerular fibrosis, 40 ROIs per section of 50 × 50 μm were placed in intraglomerular space; i.e., 320 intraglomerular ROIs were investigated per group. To assess tubulointerstitial fibrosis, 40 ROIs per section of 192 × 72 μm were placed at the interstitial cortex without glomeruli or vessels; i.e., 320 tubulointerstitial ROIs were investigated per group. To evaluate the amount of vascular/perivascular fibrosis, five ROIs per section were examined, tight-cropping a cross-section captured artery with a diameter between 50 and 100 μm; i.e., 40 vascular/perivascular ROIs were investigated per group.
The histopathological analysis was performed by an experienced investigator blinded to the group identity.
The kidney samples, snap-frozen and stored at −80°C, were dried at 100°C for 24 h and then hydrolyzed using a solution of 6 mol/L HCl. The hydroxyproline concentration was determined spectrophotometrically at 550 nm (Reddy and Enwemeka, 1996; Hrenák et al., 2013).
The results are presented as the mean ± SEM. The one-way, two-tailed analysis of variance (ANOVA), followed by a Holm-Sidak multiple comparisons test, was used for statistical analysis. p values below 0.05 were considered statistically significant. The statistical analysis was conducted using GraphPad Prism 8 for Windows (GraphPad Software, La Jolla, CA, United States).
The SBP averaged over 6 weeks of treatment was 120.07 ± 0.58 mmHg in controls, and lactacystin raised the value by 6% (p < 0.05). In the lactacystin group, captopril lowered the average SBP by 18% (p < 0.05), while melatonin had no effect on average SBP (Figure 1A).
FIGURE 1. Effect of captopril (Lac + Cap) and melatonin (Lac + Mel) on average systolic blood pressure (SBP) (A), renal hydroxyproline concentration (B), and kidney morphology: glomerular numerical density per 1 mm2 (C) and glomerular tuft area (D) of lactacystin-treated (Lac) rats; glomerular numerical density (E) and detailed images of the glomerular content (F) in lactacystin-treated rats: H-E-stained sections at ×10 (E) and ×100 (F) magnification using transmitted light microscopy; mesangial cell proliferation with extracellular matrix expansion (arrow). C, controls; *p < 0.05 vs. C; #p < 0.05 vs. Lac.
The glomerular numerical density was 7.06 ± 0.28 per mm2 in controls; lactacystin decreased this value by 37% (p < 0.05). In the lactacystin group, both captopril and melatonin augmented the glomerular numerical density by 53% and 44% (p < 0.05 for both measures), respectively (Figures 1C,E).
The glomerular tuft area was 5.173 ± 198 μm2 in controls, and lactacystin increased this value by 65% (p < 0.05). In the lactacystin group, both captopril and melatonin reduced the glomerular tuft area by 33% and 41% (p < 0.05 for both measures), respectively (Figures 1D,F).
The kidney hydroxyproline concentration was 0.15 ± 0.05 mg/g in controls, and lactacystin raised (p < 0.05) it by 139%. In the lactacystin group, both captopril and melatonin lowered (p < 0.05) the kidney hydroxyproline concentration by 23% (Figure 1B).
For intraglomerular ROIs, the volume of Col I and Col III in controls was 0.90 ± 0.23 and 1.16 ± 0.30%, respectively. Lactacystin increased the proportion of both Col I and Col III by 101% and 103% (p < 0.05 for both measures), respectively. In the lactacystin group, captopril lowered the proportion of both Col I and Col III by 93% (p < 0.05 for both measures). Similarly, melatonin reduced the proportion of both Col I and Col III by 76% and 79% (p < 0.05 for both measures), respectively. The sum of Col I and Col III volume in intraglomerular ROIs was 2.06 ± 0.53% in controls, and lactacystin increased this value by 102% (p < 0.05). In the lactacystin group, both captopril and melatonin decreased the sum of Col I and Col III volume by 93% and 78% (p < 0.05 for both measures), respectively. The ratio of Col I to Col III (Col I/Col III) was 0.78 ± 0.09 in controls, and neither lactacystin, captopril, nor melatonin had any effect on the ratio (Figure 2).
FIGURE 2. Effect of captopril (Lac + Cap) and melatonin (Lac + Mel) on glomerular fibrosis of lactacystin-treated (Lac) rats. PSR-stained section at ×100 magnification using polarized light microscopy (A), the volume of collagen I (Col I) (B), collagen III (Col III) (C), the sum of collagen I + III (D), the Col I/Col III ratio (E) and PSR-stained sections at ×200 magnification using polarized microscopy showing collagen I in red and collagen III in yellow (F). C, controls; ROI, region of interest depicted as the shaded rectangle. Intraglomerular ROI dimensions: 50 μm × 50 μm. Scale bar: 50 μm. *p < 0.05 vs. C; #p < 0.05 vs. Lac.
For tubulointerstitial ROIs, the volume of Col I and Col III in controls were 2.57 ± 0.81 and 3.88 ± 1.34%, respectively. Lactacystin increased the proportion of Col I by 139% (p < 0.05) and enhanced the proportion of Col III by 131% (ns). In the lactacystin group, captopril reduced the proportion of both Col I and Col III by 94% and 96% (p < 0.05 for both measures), respectively. Similarly, melatonin decreased the proportion of both Col I and Col III by 83% and 84% (p < 0.05 for both measures), respectively. The sum of Col I and Col III volume in tubulointerstitial ROIs was 6.45 ± 2.13% in controls, and lactacystin increased this value by 134% (p < 0.05). In the lactacystin group, both captopril and melatonin reduced the sum of Col I and Col III volume by 95% and 84% (p < 0.05 for both measures), respectively. Col I/Col III was 0.78 ± 0.11 in controls, and lactacystin, captopril, or melatonin had no effect on the ratio (Figure 3).
FIGURE 3. Effect of captopril (Lac + Cap) and melatonin (Lac + Mel) on tubulointerstitial fibrosis of lactacystin-treated (Lac) rats. PSR-stained section at ×100 magnification using polarized light microscopy (A), the volume of collagen I (Col I) (B), collagen III (Col III) (C), the sum of collagen I + III (D), the Col I/Col III ratio (E) and PSR-stained sections at ×100 magnification using polarized microscopy showing collagen I in red and collagen III in yellow (F). C, controls; ROI, region of interest depicted as the shaded rectangle. Tubulointerstitial ROI dimensions: 72 μm × 192 μm. Scale bar: 50 μm. *p < 0.05 vs. C; #p < 0.05 vs. Lac.
In vascular/perivascular ROIs, Col I and Col III’s volume in controls was 0.80 ± 0.12 and 0.64 ± 0.13%, respectively. Lactacystin had no significant effect on the volume of Col I and Col III. In the lactacystin group, captopril reduced the proportion of both Col I and Col III by 76% and 90% (p < 0.05 for both measures), respectively, while melatonin had no effect. The sum of Col I and Col III volume in vascular/perivascular ROIs was 1.44 ± 0.24% in controls, and lactacystin did not significantly change this parameter. In the lactacystin group, captopril decreased the sum of Col I and Col III volume by 82% (p < 0.05), and melatonin had no effect. Col I/Col III was 1.45 ± 0.18 in controls, and lactacystin had no significant effect on the ratio. In the lactacystin group, captopril increased Col I/Col III ratio by 226% (p < 0.05), and melatonin had no effect (Figure 4).
FIGURE 4. Effect of captopril (Lac + Cap) and melatonin (Lac + Mel) on vascular/perivascular fibrosis of lactacystin-treated (Lac) rats. PSR-stained section at ×100 magnification using polarized light microscopy (A), the volume of collagen I (Col I) (B), collagen III (Col III) (C), the sum of collagen I + III (D), the Col I/Col III ratio (E) and PSR-stained sections at ×100 magnification using polarized microscopy showing collagen I in red and collagen III in yellow (F). C, controls; ROI, region of interest depicted as the shaded rectangle. Vascular/perivascular ROI dimensions: from 50 × 100 μm to 200 × 300 μm. Scale bar: 50 μm. *p < 0.05 vs. C; #p < 0.05 vs. Lac.
Six weeks of lactacystin administration raised the average SBP. In kidneys, lactacystin reduced glomerular density, increased the glomerular tuft area, and enhanced the hydroxyproline concentration. Lactacystin likewise elevated the intraglomerular proportion and the sum of Col I and Col III, the tubulointerstitial proportion of Col I and the sum of Col I and Col III without an effect on vascular/perivascular collagen. Six weeks of captopril treatment reduced SBP, while melatonin had no effect. Both melatonin and captopril raised glomerular density, reduced the glomerular tuft area, and decreased hydroxyproline concentration in the kidneys. Both drugs reduced the proportion and sum of intraglomerular and tubulointerstitial Col I and Col III.
Since the UPS controls the protein turnover of both regulatory and structural proteins, it becomes an attractive target for pharmacological interventions. Lactacystin represents the classical, first discovered proteasome inhibitor, reported in 1991 (Ōmura and Crump, 2019). Among other effects, lactacystin is considered to be an inhibitor of nuclear factor kappa B (NF-κB) transcription factor. Since NF-κB is assumed to be a checkpoint for hypertrophic growth mediated by humoral factors, inhibitors of NF-κB, such as lactacystin, are considered a possible way of protection. The net effect of lactacystin is challenging to estimate for two reasons: first, NF-κB is not only an essential factor for proliferation (Bellas et al., 1995); it may also interfere with nitric oxide synthesis, having the opposite effect (Vrankova et al., 2010; Simko et al., 2017). Moreover, lactacystin not only specifically blocks the degradation of the NF-κB inhibitor IκBα but can also modify other proteins involved in signaling processes (Vrankova et al., 2010).
In line with this, surprisingly, lactacystin did not reduce but increased blood pressure and fibrotic rebuilding in the left ventricle (Simko et al., 2017). Thus, lactacystin administration-induced BP elevation was recently characterized as a novel model of experimental hypertension (Vrankova et al., 2010; Simko et al., 2017; Sharma et al., 2020). Proteasome inhibition may cause hypertension either because of an increased endogenous protein inhibitor of neuronal nitric oxide synthase, leading to decreased NO bioavailability in the paraventricular nucleus (Sharma et al., 2020), or from tyrosine hydroxylase upregulation and activation in the hypothalamus and brainstem (Congo Carbajosa et al., 2015), both resulting in increased sympathetic outflow. Bearing in mind the remodeling of the heart (Simko et al., 2017) and aorta (Vrankova et al., 2010) in this model, it seems to be of importance to disclose whether lactacystin could act in a similar pro-proliferative way in hypertensive kidneys.
In this experiment, chronic lactacystin treatment was associated with the loss of glomeruli, indicated by decreased glomerular density and simultaneous glomerular hypertrophy, reflected in increased glomerular tuft area. Some authors consider the reduction of nephron density to be a risk factor for hypertension and CKD progression (Kanzaki et al., 2020). Furthermore, lactacystin treatment was associated with enhanced hydroxyproline concentration and site-specific fibrotic rebuilding of renal tissue. Published data suggest that chronic hypertension leads to the accumulation and dysregulation of extracellular matrix in the kidneys, resulting in renal fibrosis (Schlondorff, 2008). The fibrotic changes in the kidneys are common 1) in the glomerulus—glomerulosclerosis; 2) in the tubulointerstitium—interstitial fibrosis; and 3) in the vessels—arteriosclerosis and perivascular fibrosis (Bülow and Boor, 2019). The most abundant collagens expressed in the kidneys are types I and III. Col I forms long, thick, and stiff fibrils, decreasing tissue compliance (Sopakayang et al., 2012). In the early stages of renal fibrosis, Col I deposits in the glomerulus, tubulointerstitial space, and arterial wall (Alexakis et al., 2006). Col III is mainly found in softer tissues and is more distensible than Col I (Silver et al., 2001). In renal fibrosis, the expression of Col III increases in both interstitium and glomeruli (Alexakis et al., 2006). In the present study, an excessive deposition of Col I and Col III was observed in the glomerulus and tubulointerstitial space in lactacystin-treated hypertensive rats.
ACE inhibitor captopril reduces fibrosis associated with target organ damage. Indeed, captopril decreased the concentration of soluble collagen in the LV of rats with combined continuous light and L-NAME-induced hypertension (Simko et al., 2010), attenuated LV collagen deposition in SHR (Zhao et al., 2015), and reduced LV fibrosis in mice with transverse aortic constriction (Zhang et al., 2019) and Sprague-Dawley rats with L-NAME-induced hypertension (Sonoda et al., 2017). Similarly, in kidneys captopril reduced interstitial renal fibrosis in neonatal dogs with partial urethral obstruction (PUO) (Shirazi et al., 2007), ameliorated fibrosis in rats with PUO (Shirazi et al., 2014), and downregulated interstitial fibrosis in rats with unilateral ureteral obstruction (Hosseinian et al., 2019). In line with these data, our results show reduced intraglomerular, tubulointerstitial, and perivascular collagen accumulation and renal hydroxyproline concentration after 6 weeks of captopril administration in lactacystin-treated rats. In this experiment, captopril reduced the proportion and sum of intraglomerular and tubulointerstitial Col I and Col III, lowered the sum of vascular/perivascular Col I and Col III and increased the vascular/perivascular ratio of Col I to Col III, while reducing the kidney hydroxyproline concentration.
Melatonin exerts a vast number of pleiotropic protective effects on various tissues, including fibrosis amelioration. The antifibrotic effects of melatonin have been observed in the LV of lactacystin-treated rats (Simko et al., 2017), of SHRs (Simko et al., 2009), and in the LV and aorta of 24-h continuous light-exposed rats (Repová-Bednárová et al., 2013; Simko et al., 2014). In the kidneys, melatonin reduced renal fibrosis in mice with unilateral ureteral obstruction (Li et al., 2020), adenine-induced CKD mice (Yoon et al., 2020), diabetic mice (Li et al., 2019; Fan et al., 2020), prenatally diclofenac sodium injected rats (Khoshvakhti et al., 2015), and in human renal proximal tubule epithelial cells on a high glucose diet (Han et al., 2020). Accordingly, in this study, 6 weeks of melatonin treatment prevented intraglomerular and tubulointerstitial fibrosis development, alongside reduced renal hydroxyproline concentration in rats administered lactacystin. Although melatonin failed to reduce SBP in this experiment, its antifibrotic effects were likely a result of melatonin’s direct, local actions, such as obvious antioxidant and radical scavenging actions (Simko et al., 2009; Reiter et al., 2018), modulation of the sympathetic and renin-angiotensin system (Simko and Paulis, 2013), and increasing NO bioavailability and antiproliferative action (Paulis and Simko, 2007; Simko and Paulis, 2007, 2013; Simko and Pechanova, 2009; Domínguez-Rodríguez et al., 2021).
The lactacystin administration induced fibrotic rebuilding of the kidneys. Both captopril and melatonin reduced the proportion and sum of intraglomerular and tubulointerstitial Col I and Col III and hydroxyproline concentration in the kidneys. Although captopril more prominently reduced Col I and Col III accumulation in the vascular/perivascular area compared to melatonin, the Col I/Col III ratio rose. Considering the stiffness of Col I, the findings suggest that captopril might cause renal capillaries to become more rigid, altering renal perfusion. On the contrary, melatonin did not significantly change the renal vascular/perivascular fibrosis while maintaining a normal Col I/Col III ratio. Since it concurrently reduced glomerular fibrosis, melatonin may play a role in maintaining adequate glomerular perfusion and filtration. Thus, melatonin may be comparable or superior to ACE inhibition because of its distinct effect on renal collagen composition, potentially contributing to improved renal perfusion and filtration rate.
In a model of lactacystin-induced hypertension and organ damage, both melatonin and captopril raised glomerular density, reduced the glomerular tuft area, and lowered the kidney hydroxyproline concentration. Both drugs reduced the proportion and sum of intraglomerular and tubulointerstitial Col I and Col III. As melatonin failed to reduce SBP, its antifibrotic effects were supposedly delivered by melatonin’s direct, local pleiotropic effects.
Considering the fibrotic remodeling of the left ventricle observed in previous works and the site-specific fibrotic rebuilding of the kidneys observed in this study, it seems reasonable to suggest that chronic treatment with lactacystin may be a perspective model of cardiorenal damage. Furthermore, we showed that melatonin, similar to captopril, is a promising means of protection against hypertensive kidney damage.
It would be of interest to correlate the renal histopathologic findings with immunohistochemical analysis of growth factors, angiogenic factors, and markers of collagen turnover in the kidneys. Indeed, according to the literature, there is a correlation between the histopathological evidence of renal fibrosis and profibrotic renal tissue markers, such as transforming growth factor-β1 (TGF-β1), connective tissue growth factor (CTGF), and vascular endothelial growth factor A (VEGF-A) (Lopes et al., 2019). Moreover, the inhibition of matrix metalloproteinase (MMP)-9 (Wang et al., 2019) and decreased expression of MMP-1 (Nazneen et al., 2002) resulted in decreased histological evidence of renal fibrosis. However, these analyses were beyond the scope and possibilities of the present histopathological study.
The raw data supporting the conclusion of this article will be made available by the authors, without undue reservation.
The animal study was reviewed and approved by the Ethical Committee of the Institute of Pathophysiology, Faculty of Medicine, Comenius University, Bratislava, Slovakia.
KR, PS, TB, and FS conceived and designed the study and drafted the manuscript. KR, PS, TB, KK, SA, AB, and MA performed the experiments and acquired data. All authors participated in data analysis and interpretation, manuscript revision, and approved the submitted version.
This work was supported by the VEGA grants for scientific research Nos. 1/0035/19 and APVV-20-0421.
The authors declare that the research was conducted in the absence of any commercial or financial relationships that could be construed as a potential conflict of interest.
All claims expressed in this article are solely those of the authors and do not necessarily represent those of their affiliated organizations, or those of the publisher, the editors and the reviewers. Any product that may be evaluated in this article, or claim that may be made by its manufacturer, is not guaranteed or endorsed by the publisher.
Alexakis, C., Maxwell, P., and Bou-Gharios, G. (2006). Organ-specific collagen expression: Implications for renal disease. Nephron. Exp. Nephrol. 102, e71–75. doi:10.1159/000089684
Bellas, R. E., Lee, J. S., and Sonenshein, G. E. (1995). Expression of a constitutive NF-kappa B-like activity is essential for proliferation of cultured bovine vascular smooth muscle cells. J. Clin. Invest. 96, 2521–2527. doi:10.1172/JCI118313
Bülow, R. D., and Boor, P. (2019). Extracellular matrix in kidney fibrosis: More than just a scaffold. J. Histochem. Cytochem. 67, 643–661. doi:10.1369/0022155419849388
Chatterjee, A., Mir, S. A., Dutta, D., Mitra, A., Pathak, K., and Sarkar, S. (2011). Analysis of p53 and NF-κB signaling in modulating the cardiomyocyte fate during hypertrophy. J. Cell. Physiol. 226, 2543–2554. doi:10.1002/jcp.22599
Congo Carbajosa, N. A., Carbajosa, N. A. L., Corradi, G., Verrilli, M. A. L., Guil, M. J., Vatta, M. S., et al. (2015). Tyrosine hydroxylase is short-term regulated by the ubiquitin-proteasome system in PC12 cells and hypothalamic and brainstem neurons from spontaneously hypertensive rats: Possible implications in hypertension. PLoS One 10, e0116597. doi:10.1371/journal.pone.0116597
Craiu, A., Gaczynska, M., Akopian, T., Gramm, C. F., Fenteany, G., Goldberg, A. L., et al. (1997). Lactacystin and clasto-lactacystin beta-lactone modify multiple proteasome beta-subunits and inhibit intracellular protein degradation and major histocompatibility complex class I antigen presentation. J. Biol. Chem. 272, 13437–13445. doi:10.1074/jbc.272.20.13437
Domínguez-Rodríguez, A., Abreu-González, P., Báez-Ferrer, N., Reiter, R. J., Avanzas, P., and Hernández-Vaquero, D. (2021). Melatonin and cardioprotection in humans: A systematic review and meta-analysis of randomized controlled trials. Front. Cardiovasc. Med. 8, 635083. doi:10.3389/fcvm.2021.635083
Fan, Z., Qi, X., Yang, W., Xia, L., and Wu, Y. (2020). Melatonin ameliorates renal fibrosis through the inhibition of NF-κB and TGF-β1/smad3 pathways in db/db diabetic mice. Arch. Med. Res. 51, 524–534. doi:10.1016/j.arcmed.2020.05.008
Hamrahian, S. M., and Falkner, B. (2017). Hypertension in chronic kidney disease. Adv. Exp. Med. Biol. 956, 307–325. doi:10.1007/5584_2016_84
Han, Y.-S., Yoon, Y. M., Go, G., Lee, J. H., and Lee, S. H. (2020). Melatonin protects human renal proximal tubule epithelial cells against high glucose-mediated fibrosis via the cellular prion protein-TGF-β-smad signaling Axis. Int. J. Med. Sci. 17, 1235–1245. doi:10.7150/ijms.42603
Hosseinian, S., Shahraki, S., Ebrahimzadeh Bideskan, A., Shafei, M. N., Sadeghnia, H. R., Soukhtanloo, M., et al. (2019). Thymoquinone alleviates renal interstitial fibrosis and kidney dysfunction in rats with unilateral ureteral obstruction. Phytother. Res. 33, 2023–2033. doi:10.1002/ptr.6376
Hrenák, J., Arendášová, K., Rajkovičová, R., Aziriová, S., Repová, K., Krajčírovičová, K., et al. (2013). Protective effect of captopril, olmesartan, melatonin and compound 21 on doxorubicin-induced nephrotoxicity in rats. Physiol. Res. 62, S181–S189. doi:10.33549/physiolres.932614
Hrenak, J., Paulis, L., Repova, K., Aziriova, S., Nagtegaal, E. J., Reiter, R. J., et al. (2015). Melatonin and renal protection: Novel perspectives from animal experiments and human studies (review). Curr. Pharm. Des. 21, 936–949. doi:10.2174/1381612820666140929092929
Huseby, N.-E., Ravuri, C., and Moens, U. (2016). The proteasome inhibitor lactacystin enhances GSH synthesis capacity by increased expression of antioxidant components in an Nrf2-independent, but p38 MAPK-dependent manner in rat colorectal carcinoma cells. Free Radic. Res. 50, 1–13. doi:10.3109/10715762.2015.1100730
Ishigami, T., Kino, T., Minegishi, S., Araki, N., Umemura, M., Ushio, H., et al. (2020). Regulators of epithelial sodium channels in aldosterone-sensitive distal nephrons (ASDN): Critical roles of Nedd4L/nedd4-2 and salt-sensitive hypertension. Int. J. Mol. Sci. 21, E3871. doi:10.3390/ijms21113871
Kanzaki, G., Tsuboi, N., Shimizu, A., and Yokoo, T. (2020). Human nephron number, hypertension, and renal pathology. Anat. Rec. 303, 2537–2543. doi:10.1002/ar.24302
Khoshvakhti, H., Yurt, K. K., Altunkaynak, B. Z., Türkmen, A. P., Elibol, E., Aydın, I., et al. (2015). Effects of melatonin on diclofenac sodium treated rat kidney: A stereological and histopathological study. Ren. Fail. 37, 1379–1383. doi:10.3109/0886022X.2015.1073556
Li, H.-H., Kedar, V., Zhang, C., McDonough, H., Arya, R., Wang, D.-Z., et al. (2004). Atrogin-1/muscle atrophy F-box inhibits calcineurin-dependent cardiac hypertrophy by participating in an SCF ubiquitin ligase complex. J. Clin. Invest. 114, 1058–1071. doi:10.1172/JCI22220
Li, J., Li, N., Yan, S., Lu, Y., Miao, X., Gu, Z., et al. (2019). Melatonin attenuates renal fibrosis in diabetic mice by activating the AMPK/PGC1α signaling pathway and rescuing mitochondrial function. Mol. Med. Rep. 19, 1318–1330. doi:10.3892/mmr.2018.9708
Li, N., Wang, Z., Gao, F., Lei, Y., and Li, Z. (2020). Melatonin ameliorates renal fibroblast-myofibroblast transdifferentiation and renal fibrosis through miR-21-5p regulation. J. Cell. Mol. Med. 24, 5615–5628. doi:10.1111/jcmm.15221
Lopes, T. G., de Souza, M. L., da Silva, V. D., Dos Santos, M., da Silva, W. I. C., Itaquy, T. P., et al. (2019). Markers of renal fibrosis: How do they correlate with podocyte damage in glomerular diseases? PLoS One 14, e0217585. doi:10.1371/journal.pone.0217585
Mearini, G., Schlossarek, S., Willis, M. S., and Carrier, L. (2008). The ubiquitin-proteasome system in cardiac dysfunction. Biochim. Biophys. Acta 1782, 749–763. doi:10.1016/j.bbadis.2008.06.009
Mennuni, S., Rubattu, S., Pierelli, G., Tocci, G., Fofi, C., and Volpe, M. (2014). Hypertension and kidneys: Unraveling complex molecular mechanisms underlying hypertensive renal damage. J. Hum. Hypertens. 28, 74–79. doi:10.1038/jhh.2013.55
Murdaca, J., Treins, C., Monthouël-Kartmann, M.-N., Pontier-Bres, R., Kumar, S., Van Obberghen, E., et al. (2004). Grb10 prevents Nedd4-mediated vascular endothelial growth factor receptor-2 degradation. J. Biol. Chem. 279, 26754–26761. doi:10.1074/jbc.M311802200
Nazneen, A., Razzaque, M. S., Liu, D., and Taguchi, T. (2002). Possible role of Ets-1 and MMP-1 in matrix remodeling in experimental cisplatin nephropathy. Med. Electron Microsc. 35, 242–247. doi:10.1007/s007950200028
Ōmura, S., and Crump, A. (2019). Lactacystin: First-in-class proteasome inhibitor still excelling and an exemplar for future antibiotic research. J. Antibiot. 72, 189–201. doi:10.1038/s41429-019-0141-8
Pagan, J., Seto, T., Pagano, M., and Cittadini, A. (2013). Role of the ubiquitin proteasome system in the heart. Circ. Res. 112, 1046–1058. doi:10.1161/CIRCRESAHA.112.300521
Parajuli, N. (2019). A cycle of altered proteasome and reactive oxygen species production in renal proximal tubular cells. Toxicol. Forensic Med. 4, 13–17. doi:10.17140/tfmoj-4-128
Paulis, L., and Simko, F. (2007). Blood pressure modulation and cardiovascular protection by melatonin: Potential mechanisms behind. Physiol. Res. 56, 671–684. doi:10.33549/physiolres.931236
Pechánová, O., Bernátová, I., Pelouch, V., and Simko, F. (1997). Protein remodelling of the heart in NO-deficient hypertension: The effect of captopril. J. Mol. Cell. Cardiol. 29, 3365–3374. doi:10.1006/jmcc.1997.0566
Pechanova, O., Matuskova, J., Capikova, D., Jendekova, L., Paulis, L., and Simko, F. (2006). Effect of spironolactone and captopril on nitric oxide and S-nitrosothiol formation in kidney of L-NAME-treated rats. Kidney Int. 70, 170–176. doi:10.1038/sj.ki.5001513
Pfeffer, M. A., Braunwald, E., Moyé, L. A., Basta, L., Brown, E. J., Cuddy, T. E., et al. (1992). Effect of captopril on mortality and morbidity in patients with left ventricular dysfunction after myocardial infarction. Results of the survival and ventricular enlargement trial. The SAVE Investigators. N. Engl. J. Med. 327, 669–677. doi:10.1056/NEJM199209033271001
Reddy, G. K., and Enwemeka, C. S. (1996). A simplified method for the analysis of hydroxyproline in biological tissues. Clin. Biochem. 29, 225–229. doi:10.1016/0009-9120(96)00003-6
Reiter, R. J., Manchester, L. C., Fuentes-Broto, L., and Tan, D.-X. (2010). Cardiac hypertrophy and remodelling: Pathophysiological consequences and protective effects of melatonin. J. Hypertens. 28 (1), S7–S12. doi:10.1097/01.hjh.0000388488.51083.2b
Reiter, R. J., Rosales-Corral, S., and Sharma, R. (2020). Circadian disruption, melatonin rhythm perturbations and their contributions to chaotic physiology. Adv. Med. Sci. 65, 394–402. doi:10.1016/j.advms.2020.07.001
Reiter, R. J., Tan, D. X., Rosales-Corral, S., Galano, A., Zhou, X. J., and Xu, B. (2018). Mitochondria: Central organelles for melatonin’s antioxidant and anti-aging actions. Molecules 23, E509. doi:10.3390/molecules23020509
Repová-Bednárová, K., Aziriová, S., Hrenák, J., Krajčírovičová, K., Adamcová, M., Paulis, L., et al. (2013). Effect of captopril and melatonin on fibrotic rebuilding of the aorta in 24 hour light-induced hypertension. Physiol. Res. 62, S135–S141. doi:10.33549/physiolres.932592
Russcher, M., Koch, B., Nagtegaal, E., van der Putten, K., ter Wee, P., and Gaillard, C. (2012). The role of melatonin treatment in chronic kidney disease. Front. Biosci. 17, 2644–2656. doi:10.2741/4075
Schlondorff, D. O. (2008). Overview of factors contributing to the pathophysiology of progressive renal disease. Kidney Int. 74, 860–866. doi:10.1038/ki.2008.351
Seccia, T. M., Maniero, C., Belloni, A. S., Guidolin, D., Pothen, P., Pessina, A. C., et al. (2008). Role of angiotensin II, endothelin-1 and L-type calcium channel in the development of glomerular, tubulointerstitial and perivascular fibrosis. J. Hypertens. 26, 2022–2029. doi:10.1097/HJH.0b013e328309f00a
Sharma, N. M., Haibara, A. S., Katsurada, K., Liu, X., and Patel, K. P. (2020). Central angiotensin II-Protein inhibitor of neuronal nitric oxide synthase (PIN) axis contribute to neurogenic hypertension. Nitric Oxide 94, 54–62. doi:10.1016/j.niox.2019.10.007
Shi, S., Lei, S., Tang, C., Wang, K., and Xia, Z. (2019). Melatonin attenuates acute kidney ischemia/reperfusion injury in diabetic rats by activation of the SIRT1/Nrf2/HO-1 signaling pathway. Biosci. Rep. 39, BSR20181614. doi:10.1042/BSR20181614
Shirazi, M., Noorafshan, A., Bahri, M. A., and Tanideh, N. (2007). Captopril reduces interstitial renal fibrosis and preserves more normal renal tubules in neonatal dogs with partial urethral obstruction: A preliminary study. Urol. Int. 78, 173–177. doi:10.1159/000098078
Shirazi, M., Soltani, M.-R., Jahanabadi, Z., Abdollahifar, M.-A., Tanideh, N., and Noorafshan, A. (2014). Stereological comparison of the effects of pentoxifylline, captopril, simvastatin, and tamoxifen on kidney and bladder structure after partial urethral obstruction in rats. Korean J. Urol. 55, 756–763. doi:10.4111/kju.2014.55.11.756
Silver, F. H., Horvath, I., and Foran, D. J. (2001). Viscoelasticity of the vessel wall: The role of collagen and elastic fibers. Crit. Rev. Biomed. Eng. 29, 279–301. doi:10.1615/critrevbiomedeng.v29.i3.10
Simko, F., Hrenak, J., Dominguez-Rodriguez, A., and Reiter, R. J. (2020). Melatonin as a putative protection against myocardial injury in COVID-19 infection. Expert Rev. Clin. Pharmacol. 13, 921–924. doi:10.1080/17512433.2020.1814141
Simko, F., and Paulis, L. (2013). Antifibrotic effect of melatonin--perspective protection in hypertensive heart disease. Int. J. Cardiol. 168, 2876–2877. doi:10.1016/j.ijcard.2013.03.139
Simko, F., and Paulis, L. (2007). Melatonin as a potential antihypertensive treatment. J. Pineal Res. 42, 319–322. doi:10.1111/j.1600-079X.2007.00436.x
Simko, F., Pechanova, O., Pelouch, V., Krajcirovicova, K., Celec, P., Palffy, R., et al. (2010). Continuous light and L-NAME-induced left ventricular remodelling: Different protection with melatonin and captopril. J. Hypertens. 28 (1), S13–S18. doi:10.1097/01.hjh.0000388489.28213.08
Simko, F., Pechanova, O., Pelouch, V., Krajcirovicova, K., Mullerova, M., Bednarova, K., et al. (2009). Effect of melatonin, captopril, spironolactone and simvastatin on blood pressure and left ventricular remodelling in spontaneously hypertensive rats. J. Hypertens. Suppl. 27, S5–S10. doi:10.1097/01.hjh.0000358830.95439.e8
Simko, F., and Pechanova, O. (2009). Potential roles of melatonin and chronotherapy among the new trends in hypertension treatment. J. Pineal Res. 47, 127–133. doi:10.1111/j.1600-079X.2009.00697.x
Simko, F., Pechanova, O., Repova Bednarova, K., Krajcirovicova, K., Celec, P., Kamodyova, N., et al. (2014). Hypertension and cardiovascular remodelling in rats exposed to continuous light: Protection by ACE-inhibition and melatonin. Mediat. Inflamm. 2014, 703175. doi:10.1155/2014/703175
Simko, F., Pechanova, O., Repova, K., Aziriova, S., Krajcirovicova, K., Celec, P., et al. (2017). Lactacystin-induced model of hypertension in rats: Effects of melatonin and captopril. Int. J. Mol. Sci. 18, E1612. doi:10.3390/ijms18081612
Simko, F., Reiter, R. J., and Paulis, L. (2019). Melatonin as a rational alternative in the conservative treatment of resistant hypertension. Hypertens. Res. 42, 1828–1831. doi:10.1038/s41440-019-0318-3
Sonoda, K., Ohtake, K., Uchida, H., Ito, J., Uchida, M., Natsume, H., et al. (2017). Dietary nitrite supplementation attenuates cardiac remodeling in l-NAME-induced hypertensive rats. Nitric Oxide 67, 1–9. doi:10.1016/j.niox.2017.04.009
Sopakayang, R., De Vita, R., Kwansa, A., and Freeman, J. W. (2012). Elastic and viscoelastic properties of a type I collagen fiber. J. Theor. Biol. 293, 197–205. doi:10.1016/j.jtbi.2011.10.018
Stanko, P., Baka, T., Repova, K., Aziriova, S., Krajcirovicova, K., Barta, A., et al. (2020). Ivabradine ameliorates kidney fibrosis in L-NAME-induced hypertension. Front. Med. 7, 325. doi:10.3389/fmed.2020.00325
Tang, M., Li, J., Huang, W., Su, H., Liang, Q., Tian, Z., et al. (2010). Proteasome functional insufficiency activates the calcineurin-NFAT pathway in cardiomyocytes and promotes maladaptive remodelling of stressed mouse hearts. Cardiovasc. Res. 88, 424–433. doi:10.1093/cvr/cvq217
Tobeiha, M., Jafari, A., Fadaei, S., Mirazimi, S. M. A., Dashti, F., Amiri, A., et al. (2022). Evidence for the benefits of melatonin in cardiovascular disease. Front. Cardiovasc. Med. 9. doi:10.3389/fcvm.2022.888319
Vrankova, S., Parohova, J., Barta, A., Janega, P., Simko, F., and Pechanova, O. (2010). Effect of nuclear factor kappa B inhibition on L-NAME-induced hypertension and cardiovascular remodelling. J. Hypertens. 28 (1), S45–S49. doi:10.1097/01.hjh.0000388494.58707.0f
Wang, H., Gao, M., Li, J., Sun, J., Wu, R., Han, D., et al. (2019). MMP-9-positive neutrophils are essential for establishing profibrotic microenvironment in the obstructed kidney of UUO mice. Acta Physiol. 227, e13317. doi:10.1111/apha.13317
Wang, Z., Dong, H., Li, M., and Liang, X.-B. (2022). Ubiquitin-conjugating enzyme UBE2Q2 participates in HUWE1-mediated protection on renal tubulointerstitial fibrosis. Sheng Li Xue Bao 74, 117–124.
Willis, M. S., Schisler, J. C., Li, L., Rodríguez, J. E., Hilliard, E. G., Charles, P. C., et al. (2009). Cardiac muscle ring finger-1 increases susceptibility to heart failure in vivo. Circ. Res. 105, 80–88. doi:10.1161/CIRCRESAHA.109.194928
Yoon, Y. M., Go, G., Yun, C. W., Lim, J. H., Lee, J. H., and Lee, S. H. (2020). Melatonin suppresses renal cortical fibrosis by inhibiting cytoskeleton reorganization and mitochondrial dysfunction through regulation of miR-4516. Int. J. Mol. Sci. 21, E5323. doi:10.3390/ijms21155323
Zhang, Y., Zhang, L., Fan, X., Yang, W., Yu, B., Kou, J., et al. (2019). Captopril attenuates TAC-induced heart failure via inhibiting Wnt3a/β-catenin and Jak2/Stat3 pathways. Biomed. Pharmacother. 113, 108780. doi:10.1016/j.biopha.2019.108780
Keywords: lactacystin, melatonin, captopril, kidney injury, fibrotic remodeling, cardiorenal damage
Citation: Repova K, Stanko P, Baka T, Krajcirovicova K, Aziriova S, Hrenak J, Barta A, Zorad S, Reiter RJ, Adamcova M and Simko F (2022) Lactacystin-induced kidney fibrosis: Protection by melatonin and captopril. Front. Pharmacol. 13:978337. doi: 10.3389/fphar.2022.978337
Received: 25 June 2022; Accepted: 25 August 2022;
Published: 13 September 2022.
Edited by:
Fujian Lu, Boston Children’s Hospital and Harvard Medical School, United StatesReviewed by:
Walied Abdo, Kafrelsheikh University, EgyptCopyright © 2022 Repova, Stanko, Baka, Krajcirovicova, Aziriova, Hrenak, Barta, Zorad, Reiter, Adamcova and Simko. This is an open-access article distributed under the terms of the Creative Commons Attribution License (CC BY). The use, distribution or reproduction in other forums is permitted, provided the original author(s) and the copyright owner(s) are credited and that the original publication in this journal is cited, in accordance with accepted academic practice. No use, distribution or reproduction is permitted which does not comply with these terms.
*Correspondence: Fedor Simko, ZmVkb3Iuc2lta29AZm1lZC51bmliYS5zaw==
†These authors have contributed equally to this work and share first authorship
Disclaimer: All claims expressed in this article are solely those of the authors and do not necessarily represent those of their affiliated organizations, or those of the publisher, the editors and the reviewers. Any product that may be evaluated in this article or claim that may be made by its manufacturer is not guaranteed or endorsed by the publisher.
Research integrity at Frontiers
Learn more about the work of our research integrity team to safeguard the quality of each article we publish.