- 1State Key Laboratory of Southwestern Chinese Medicine Resources, Innovative Institute of Chinese Medicine and Pharmacy, Chengdu University of Traditional Chinese Medicine, Chengdu, China
- 2Acupuncture and Tuina School, Chengdu University of Traditional Chinese Medicine, Chengdu, China
Coronavirus disease (COVID-19) has spread worldwide and its effects have been more devastating than any other infectious disease. Importantly, patients with severe COVID-19 show conspicuous increases in cytokines, including interleukin (IL)-6, monocyte chemoattractant protein (MCP)-1, IL-8, tumor necrosis factor (TNF)-α, IL-1, IL-18, and IL-17, with characteristics of the cytokine storm (CS). Although recently studied cytokine inhibitors are considered as potent and targeted approaches, once an immunological complication like CS happens, anti-viral or anti-inflammation based monotherapy alone is not enough. Interestingly, certain isoquinoline alkaloids in Coptis chinensis Franch. (CCFIAs) exerted a multitude of biological activities such as anti-inflammatory, antioxidant, antibacterial, and immunomodulatory etc, revealing a great potential for calming CS. Therefore, in this timeline review, we report and compare the effects of CCFIAs to attenuate the exacerbation of inflammatory responses by modulating signaling pathways like NF-ĸB, mitogen-activated protein kinase, JAK/STAT, and NLRP3. In addition, we also discuss the role of berberine (BBR) in two different triggers of CS, namely sepsis and viral infections, as well as its clinical applications. These evidence provide a rationale for considering CCFIAs as therapeutic agents against inflammatory CS and this suggestion requires further validation with clinical studies.
1 Introduction
In 2019, the first outbreak of coronavirus disease (COVID-19) led to a serious public health event which threatened global health. According to a Lancet report, the main cause of COVID-19 death is acute respiratory distress syndrome (ARDS) (Hu et al., 2021). Moreover, a growing body of clinical data suggests that cytokine storm (CS) and inflammatory signaling pathway transduction are two crucial factors contributing to ARDS in patients with COVID-19 (Choudhary et al., 2021). CS not only worsens the severity of infection, but also affects the heart, liver, kidney, gastrointestinal system, and the central nervous system, eventually leading to multiorgan failure (MOF) (Ye et al., 2020). Therefore, early recognition and timely treatment of CS is of great significance for treating critical patients and for reducing the mortality rate.
CS is an inflammatory syndrome in which cytokines are abnormally released in response to infection and other stimuli. During the process, a mass of pro-inflammatory cytokines and growth factors, typically including IL-18, IL-6, IL-17, IL-1β/-1α, IFN-γ, and TNF-α, as well as chemokines are released to fuel the CS (Huang et al., 2020), (Zhu et al., 2020). Meanwhile, a population of immune cells is infected during this process, causing a sustained inflammatory response (Channappanavar and Perlman, 2017). Thus, controlling the inflammatory response by immunomodulators and reducing or antagonizing cytokine levels are effective measures to calm CS (Weckmann and Alcocer-Varela, 1996), (Ye et al., 2020). At present, various of cytokine inhibitors have been applied in the CS treatment, including IL-6-antagonists (i.e., siltuximab), IL-1-antagonists (i.e., anakinra), IL-17-antagonists (i.e., secukinumab), TNF-α-blockers (i.e., infliximab), and INF-α-inhibitors (i.e., sifalimumab), as well as immunomodulators glucocorticoids (Li et al., 2020b), (Ye et al., 2020). However, it is worth noting that these drugs are a double-edged sword in the context of antiviral infections. Although the therapeutic effect of inhibitors is remarkable, their application has some unavoidable adverse reactions. For example, tocilizumab posed mild liver test disturbances (Jamilloux et al., 2020). Additionally, prolonged indiscriminate suppression of inflammation raises concerns about the ability to clear the pathogen, as well as the increased risk of secondary infection. For example, the recipients of emapalumab occurred (bacterial, viral, and opportunistic) infections and multiple organ dysfunction syndrome (Jamilloux et al., 2020). Consequently, for treating viral infection and excessive inflammatory complications, drugs that reduce inflammation and modulate innate immune response without compromising the adaptive immune response may more effectively manage CS patients (Manjili et al., 2020).
Coptis chinensis Franch. (CCF) (Huang Lian), is a widely used traditional Chinese herbal medicine, which has been reported to exhibit antibacterial, anti-oxidant, anti-hyperglycemic, and anti-inflammatory activities (Meng et al., 2018). We traced the database of literature published within the last decade and found that isoquinoline alkaloids from CCF demonstrate potential to calm CS. For instance, Berberine (BBR), coptisine (COP), and palmatine (PAL) all belong to the CCF isoquinoline alkaloids (CCFIAs) (Meng et al., 2018), as well as the anti-inflammatory compounds of CCF (Li et al., 2015). CCFIAs inhibit the production of the inflammatory cytokines and mediators such as IL-1α/β, IL-6, IL-17, IFN-γ, TNF-α, nitric oxide (NO), prostaglandins (PGs), leukotrienes, and reactive oxygen species (ROS) (Li et al., 2015). The molecular mechanisms underlying the immunomodulatory and anti-inflammatory effects of CCFIAs include downregulation of toll-like receptors (TLRs), and inflammation-associated pathways, representative ones include nuclear factor-κB (NF-κB), mitogen-activated protein kinase (MAPK), Januskinase/Signal transducer and activator of transcription (JAK/STAT) and inflammasome NLRP3 (Wang et al., 2019), (Li et al., 2019), (Sun et al., 2019), (Yao et al., 2019).
In this review, we elaborate the underlying molecular mechanisms of CS progress, then we retrieve articles concerning the application of CCFIAs in the treatment of cytokine storm related inflammatory diseases from PubMed, Web of Science and Geenmedical through electronic and manual retrieval, a total of 520 publications were identified, 75 of which were included in this systematic review. Additionally, we present recent experimental data on the inhibition of pro-inflammatory mediators’ production by CCFIAs, as well as compare the similarities and distinctions in mechanisms/effects among CCFIAs. Furthermore, we summarize the therapeutic role in sepsis and viral infections and clinical application of BBR. Given that CCFIAs have antiviral and antibacterial effects in addition to downregulating cytokine production, they may be more promising therapeutic candidates for preventing infection-associated CS than drugs with only antimicrobial or anti-inflammatory activity.
2 Cytokine storm
CS is a systemic inflammatory response by a dysregulated immune system which refers to those situations of overly exuberant inflammation leading to critical conditions, such as ARDS, disseminated intravascular coagulation (DIC) or MOF (Jamilloux et al., 2020). In early stages of COVID-19, SARS-CoV-2 enters the host cell and attaches to angiotensin-converting enzyme 2 (ACE2), which is the key participant in the pathogenesis of COVID-19 (Iwasaki et al., 2021) (Shown in Figure 1). Rapidly, activation of the innate immune response and hypercytokinemia occur in COVID-19 patients, activated pathogenic Th1 cells secrete proinflammatory cytokines, such as GM-CSF and IL-6. GM-CSF further activates CD14+CD16+inflammatory monocytes to produce large quantities of IL-6, TNF-α, and other cytokines (MCP-1, IL-1β, IL-17), followed by recruitment and activation of abundant inflammatory cells, for example, neutrophils form neutrophil extracellular traps (NETs) to facilitate cytokine release with the positive feedback, and monocytes migrate to the lung and further derive into macrophage or monocyte derived dendritic cells (Zhou et al., 2020) (Huang et al., 2020). On the other hand, severe patients have a temporary immunodeficient state in vivo, characterized by a delayed type-I IFN response and lymphopenia, which may explain CS and more severe diseases (Huang et al., 2020), (Channappanavar et al., 2016). The delayed secretion of type Ⅰ and Ⅲ IFNs, including IFN α/β leads to an excessive late immune response, and generalized hyper-inflammation in lung that induces acute lung injury (Channappanavar et al., 2016), (Kim et al., 2021). Subsequently, there are lung infiltration by monocytes, macrophages and neutrophils, as well as recruiting mediators. These acute inflammatory mechanisms damage the pulmonary microvascular and alveolar barrier and cause vascular leakage and alveolar edema, converging to ARDS, and initiate CS in the lung (Channappanavar et al., 2016), (Quirch et al., 2020). In all these conditions, IL-1β, IL-18, IFN-γ, and IL-6 are key mediators of hyperinflammation. Finally, chemokines release can attract extra inflammatory cells to migrate into the inflammation site that intensify CS and may have indirect impacts on MOF, specially kidneys, liver, and heart (Ahmadian et al., 2021), (Bavishi et al., 2020), (Alqahtani and Schattenberg, 2020). In addition, ACE2 was expressed in vital organs (lung, heart, intestine, brain, kidney, liver, etc.,). Thus SARS-CoV-2 also directly damages the target organ by binding to ACE2, and then exacerbation by inflammatory responses (Iwasaki et al., 2021).
As previously mentioned, cytokines play a central role as inflammatory mediators in CS. Generally, cytokine secretion is mediated by three pathways, namely 1) the angiotensin II/angiotensin receptor type 1 (AT1R) pathway (Ni et al., 2020); 2) the ACE2 signaling pathway (Chen et al., 2010); and 3) innate immune signaling pathways, including pattern recognition receptors (PRRs) such as TLRs, RIG-1 (Park and Iwasaki, 2020), and inflammasomes containing NLRP3 (Shah, 2020), AIM2 (Junqueira et al., 2021). Among these, the activation of the innate immune response system is the most difficult to control and meanwhile the easiest way to potentiate excessive release of cytokines. In principle, controlling an ongoing inflammatory response by specifically or nonspecifically targeting inflammatory cytokines or related signaling pathways can be considered a promising choice for therapeutic strategy for CS. Crucial roles for the inflammatory signaling pathways and downstream cytokines are shown in (Figure 2).
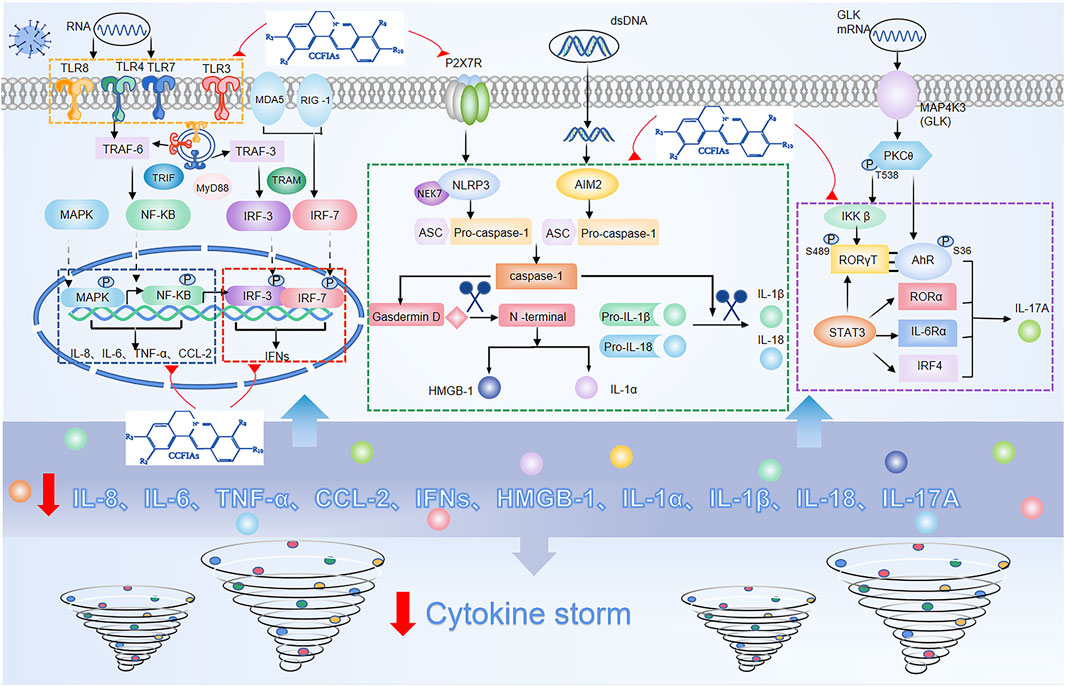
FIGURE 2. Overactivation of innate immune signaling pathways and modulation of these pathways by isoquinoline alkaloids in Coptis Chinensis Franch.
In response to viral infection, PRRs recognize and bind to pathogen-associated molecular patterns (PAMPs), triggering activation of the innate immune response system of the invading virus (Medzhitov, 2007). Among PRRs, the most typical ones are TLRs, whose activation eventually causes IRF3, NF-ĸB, MAPK, and JAK/STAT to be activated (Lim and Staudt, 2013). One of the major pathways for NF-ĸB activation after virus infection is via the MyD88, the other is the angiotensin II(Ang II) pathway. Ang II via AT1R activates NF-κB, and metalloprotease 17 (ADAM17), which generates the mature form of TNF-α (Fara et al., 2020). Subsequently, ADAM17 induces STAT3 activation, which coactivates the IL-6 amplifier (AMP) with NF-κB, and further activates various proinflammatory factors, such as IL-6, IL-8, MCP-1, and VEGF (Cortese et al., 2020). In addition, similar with NF-κB, MAPK also mainly regulates the release of IL-6 and TNF-α (Asiedu et al., 2021). With regard to the route of IFN-γ release, IRF3 is widely expressed. Besides, IRF3 also directly induces the expression of cytokines other than type I IFNs, including CXCL10, IL-12, IL-23, and IL-15 (Brownell et al., 2014), (Koshiba et al., 2013). In addition to lL-6 activation combined with NF-κB, STAT3 also directly activates IL-17A, and acts in conjunction with MAP4K3 to selectively promotes IL-17A transcription by inducing the AHR–ROR–γ T complex (Chuang et al., 2019). Additionally, nlrp3-mediated caspase-1 typical inflammatory pathway leads to the formation of active IL-1β, IL-18, IL-1α, and HMGB1, while the direct substrate of caspase-11’s atypical inflammatory pathway is IL-1α (Bulek et al., 2020), (Toldo and Abbate, 2018), (Wu et al., 2021).
Collectively, the activation of the multiple cytokine pathways described above can result in sudden and acute increase in the circulating levels of various proinflammatory cytokines, and lead to an overactivation of the inflammatory response. Importantly, the breakdown of mechanisms that tightly regulate inflammatory signaling pathways can be the underlying cause of uncontrolled inflammatory responses. Meanwhile, the binding of different receptors and ligands results in signal cascade amplification, which increases the probability of CS. This suggests that in addition to antiviral therapeutics during the initial phase of the infection, appropriate therapies targeting inflammatory signaling pathways and their downstream components, may be required to dampen the risk of CS due to dysregulation of inflammatory responses.
3 CCFIAs protects against inflammation by inhibiting proinflammatory cytokines via regulating signaling pathways
CCF was first recorded in Sheng Nong’s Herbal Classic, and listed as a representative medicine for eliminating dampness by bitter and cool. Additionally, CCF is also a powerful and commonly utilized herb documented in several ancient medical books, such as “Jin-Gui Yao-Lue”, and especially used as the monarch drug in many prescriptions, with functions to clear away heat, resolve dampness, purge fire, and perform detoxification. Clinically, it has also been applied for treating diverse inflammatory-related diseases, such as sepsis, diabetes mellitus and ulcerative colitis (Liu et al., 2021), (Xie et al., 2022), (Ran et al., 2019). Moreover, the studies have shown that CCF controls the development of the disease by regulating signaling pathways and cytokine secretion (Wu et al., 2016). Nevertheless, modern research has demonstrated that the major pharmacodynamic components of CCF that exerts biological activity are isoquinoline alkaloids. Which have anti-inflammatory, antibacterial, antioxidant and hypolipidemic effects (Meng et al., 2018).
Among all isoquinoline alkaloids, BBR, COP and PAL, have the most abundant quantitation, which all belong to protoberberine alkaloids with similar structures, and are the main bioactive components of CCF to exert anti-inflammatory, antibacterial and immunomodulatory effects (Li et al., 2015) (Figure 3). Hence, we propose in (Tables 1, 2) a summarized outcome of available in vitro and in vivo studies associated with mitigating inflammatory cytokine by CCFIAs, thereby supporting its likely therapeutic benefits against CS. Moreover, these studies demonstrated CCFIAs play a role in inhibiting the production and activation of inflammatory factors by regulating multiple inflammatory signaling pathways (Figure 2).
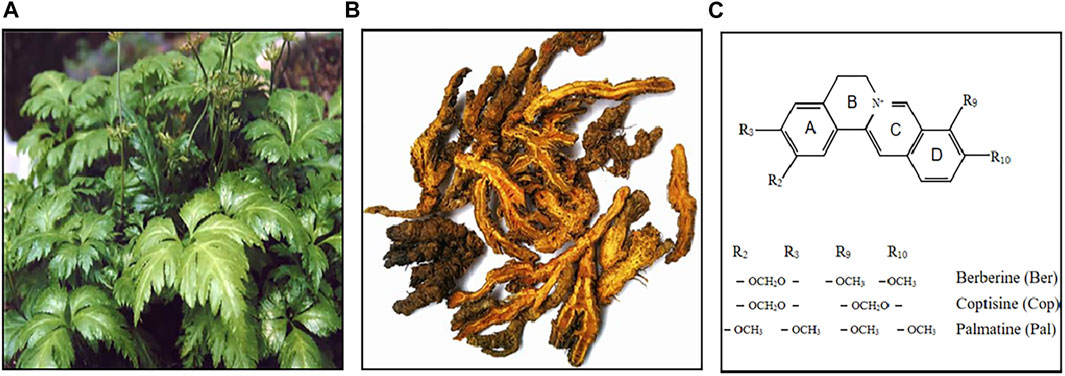
FIGURE 3. Coptis Chinensis Franch. Whole plant (A), dry root (B), chemical structure of berberine, coptisine, and palmatine (C).
3.1 Modulation of BBR on signaling transductions of inflammatory pathways
The mechanism of BBR regulation on pro-inflammatory cytokines has been extensively investigated and engages TLR signaling and three main inflammatory signaling pathways include NF-κB, JAK/STAT, and MAPK (Li et al., 2019), (Wang et al., 2019), (Sun et al., 2019). Additionally, the inhibition for NLRP3 inflammasomes also plays a significant role in its anti-inflammatory effect (Yao et al., 2019). In brief, the mechanism of BBR is mainly through direct inhibition of multiple inflammatory pathways, or via regulation of one signaling pathway to restrain another, or indirectly by modulation of pathway-related genes.
Numerous studies published in recent years have shown that upregulation of the NF-κB signaling pathway is associated with the generation and development of several inflammatory diseases, and it plays a dominant part in the hyperinflammatory response and CS activation (Acar et al., 2018). However, many studies have shown that BBR could directly inhibit the activation of NF-κB signaling pathway by downregulating NF-κB expression (p65/p50 subunits), retarding the phosphorylation and degradation of IκB, and suppressing the NF-κB translocation from cytoplasm to the nucleus (Li et al., 2019), (Yu et al., 2019), (Song et al., 2020), (Li et al., 2016). On the other hand, BBR inhibited the NF-κB pathway by regulating other signaling molecules. For example, BBR suppressed the expression of TLR4 and downregulated MyD88, exerting the broad-spectrum anti-inflammatory actions via downregulation of TLR4-MyD88-NF-κB pathway (Xu et al., 2018), (Zhang et al., 2011), (Li et al., 2020). Moreover, BBR significantly restrained neuroinflammation and brain injury via the HMGB1/TLR4/NF-κB signaling pathway (Zhu et al., 2018). Nevertheless, how BBR modulated HMGB1/NF-κB pathway remains unknown. Importantly, BBR was reported a potent Sirt1 activator, and it could significantly upregulate Sirt1 expression while restrain NF-κB activation (Zhang et al., 2020), (Zhang et al., 2017). In addition, BBR also induced activation of AKT1/SOCS1 and AMPK, thereby inhibiting phosphorylation of NF-κB (Liu et al., 2018), (Pei et al., 2019).
MAPK pathway consists of three well-known serine–threonine protein kinases, including extra-cellular receptor-activated kinase (ERK), p38, and c-Jun N-terminalkinase (JNK) (Seger and Krebs, 1995). The JNK and p38 pathways play a pivatal role in inflammation and tissue homeostasis, more importantly, a study of the effect of p38/MAPK inhibitors on SARS-CoV infected mice reported an 80% increase in survival after treatment (Grimes and Grimes, 2020). It has been reported that BBR inhibited pro-inflammatory responses via directly suppressing the phosphorylation of MAPK, including ERK, p38 and JNK (Wang et al., 2014), (Li et al., 2019). Interestingly, BBR does not always regulate the three kinases simultaneously. In two studies, BBR interfered the phosphorylation of ERK and P38, but not JNK, thereby restraining neutrophilic infiltration and inflammatory cytokine production (Wang et al., 2019), (Xu et al., 2015). Notably, BBR reduced gene expression of microRNA-21, a key regulator of inflammatory cell infiltration and mast cell recruitment, through downregulation of the p38 signaling pathway, ultimately resulting in counteracting allergic inflammation (Li et al., 2018).
JAK/STAT pathway has multiple members, namely four Janus kinases (JAK1-3 and TYK2) and seven STATs (STAT1-4, 5a, 5b, and 6) (Leonard and O'Shea, 1998), (O'Shea et al., 2015). Several studies have reported that BBR inhibited the release of downstream inflammatory cytokines including IFN-γ, IL-6, IL-17A, IL-18, TNF-α, and IL-1β by directly preventing phosphorylation of JAK and STAT (Liu et al., 2016), (Ma et al., 2020), (Song et al., 2022). Importantly, there is growing evidence that JAK-STAT signaling pathway plays a critical role in Th1 and Th17 differentiation and cytokine secretion, and that BBR can suppress TH1/TH17-mediated inflammatory responses by modulating the JAK/STAT signaling pathway. In experimental autoimmune myocarditis (EAM) and experimental autoimmune myasthenia gravis (EAMG) rat models, BBR was found to inhibit autoimmune-induced Th17 and Th1 responses by downregulating STAT and JAK phosphorylation respectively, and eventually led to decreased expression of pro-inflammatory cytokines and chemokines (Liu et al., 2016), (Song et al., 2022). Furthermore, a study provided the new underlying molecular mechanism of BBR’s modulatory action on the JAK/STAT signaling pathway. Li et al. (2020c) announced that BBR prevented the phosphorylation and activation of JAK/STAT members by suppressing Oncostatin M, whose functions as inducing the expression of inflammatory genes, and participates in mucosal inflammation and tissue damage.
Even after its well-established anti-inflammatory potential, the effect of BBR on NLRP3 inflammasome stimulation and downstream pathways has still not elucidated completely. Some studies have shown that BBR significantly ameliorated NLRP3 inflammasome activation and the subsequent pyroptosis process by directly reducing NLRP3, GSDMD-N expression, and caspase-1 activity (Mai et al., 2020), (Dinesh and Rasool, 2017), (Yao et al., 2019). In addition, BBR was also observed to reversed NLRP3 inflammatory assembly by inhibiting the TLR4/Myd88/NF-κB signaling pathway (Huang et al., 2018). Prior to this, few studies have demonstrated that TLR4/Myd88/NF-κB is involved in the regulation of NLRP3 inflammasome. It is worth noting that a study for the first time elucidated the direct binding target of BBR is NIMA-related kinase 7 (NEK7), rather than inhibiting the NF-κB and TLR4 pathways (Zeng et al., 2021). BBR could directly prevent the NEK7-NLRP3 interaction via methylenedioxy binding to the R121, which is residue of NEK7 and located exactly in the key interaction domain of NEK7-NLRP3, therefore the inhibition of BBR for NEK7-NLRP3 is specific, in other words, BBR did not inhibit the activation of IL-1β induced by other inflammasomes including AIM2 or NLRC4 (Zeng et al., 2021), (Sharif et al., 2019).
In addition to regulating these major inflammatory signaling pathways, BBR can also target other signaling molecules. For example, BBR effectively restrained the over-activation of TGF-β1/Smads signaling to attenuate the airway inflammation of chronic obstructive pulmonary disease (Wang et al., 2019). Furthermore, PI3K/Akt is also an important target for inhibiting inflammatory responses (Dinesh and Rasool, 2018), (Fang et al., 2018). Dinesh et al. found that BBR has a therapeutic effect on IL-21/IL-21R mediated signaling pathway in RA pathogenesis by inhibiting the PI3K/Akt signaling pathway and downstream elements (Dinesh and Rasool, 2018). It is worth noting that the regulatory effect of BBR on all the above signaling pathways was not single-targeted, but mostly counteracted excessive inflammatory responses by acting on multiple pathways simultaneously (Gu et al., 2021), (Zhu et al., 2019), (Li et al., 2019).
3.2 Modulation of COP on signaling transductions of inflammatory pathways
COP and BBR have the same parent nuclear structure, except that two methoxy groups on ring D of BBR are replaced by a methylenedioxy group, resulting in being inferior to BBR in terms of polarity, and less anti-inflammatory and antibacterial activity than BBR (Li et al., 2015). Similarly, COP also exerts modulatory effects on various inflammatory signals, including NF-κB, MAPK, PI3K/Akt pathways and NLRP3 inflammasome (Wang et al., 2021), (Feng et al., 2017), (Fu et al., 2018), (Wu et al., 2019). However, unlike BBR which downregulated TLR4/MyD88/NF-κB pathway to exert broad-spectrum anti-inflammatory effect, COP had no effect on expressions of TLR-4 and Myd88 as well as LPS binding to TLR-4 in LPS-induced RAW264.7 cells, suggesting that COP may not block downstream pro-inflammatory pathways such as NF-κB, NLRP3 and MAPK through TLR-4 signaling (Xu et al., 2018), (Wu et al., 2019). But it is noteworthy that COP could suppress the activation of the NF-κB pathway by directly inhibiting caspase-1 (Wu et al., 2019). As for NLRP3 inflammasome, BBR could block P2X7R activation and interfere with ATP/P2X7 interactions, whereas COP did not inhibit LPS plus ATP-mediated P2X7R overexpression, thus indicating that COP did not prevent NLRP3 inflammasome activation by inhibiting P2X7R (Wu et al., 2019). In addition, COP also inhibited the expression of downstream inflammatory cytokines TNF-a, IL-1β, and IL-6 by restraining MAPK signaling, and similar with BBR, via blocking phosphorylation of p38 and JNK without suppressing activation of ERK in most inflammatory models (Feng et al., 2017), (Chen et al., 2017), (Choi et al., 2017). Unfortunately, there is no in-depth study on the anti-inflammatory role of COP in regulating JAK/STAT signaling pathway by far.
3.3 Modulation of PAL on signaling transductions of inflammatory pathways
PAL also has the parent nuclear structure of proto-berberine, which differs from BBR in that a methylenedioxy on the A ring is replaced by two methylene groups, so they both have similar biological activities of anti-inflammation, antibacteria and immunomodulation (Li et al., 2015). Similar with BBR and COP, PAL also exerted anti-inflammatory effects by inhibiting NF-κB (Yan et al., 2017), (Ma et al., 2021). Of note, Yan et al. found PAL treatment downregulated the gene levels of TLR4, CD14 and TRIF in TLR4 signaling pathways, but it did not affect MyD88 expression. In other words, PAL downregulated NF-κB expression and inhibited NF-κB by restraining TRIF-dependent TLR4 pathways, which was different from the effect of BBR on inhibiting the TLR4-MyD88-NF-κB pathway (Li et al., 2020), (Yan et al., 2017). Interestingly, inhibition of NLRP3 inflammasome activation by PAL is associated with enhanced mitochondrial autophagy, and Mai et al. (2019) found that PAL activated PINK1/Parkin-mediated mitophagy to inhibit the activation of NLRP3 inflammasomes, thus preventing excessive inflammation caused by NLRP3 inflammasome activation. Similarly, BBR also could inactivate the NLRP3 inflammasome via induction of mitophagy in another BNIP3-dependent manner (Liu et al., 2020). Besides, in a Helicobacter pylori-induced induced model of chronic atrophic gastritis (CAG), PAL was shown to inhibits the expression of MMP-10 and IL-8 through the ADAM17/EGFR axis and exerts anti-inflammatory (Chen et al., 2020).
As mentioned in the previous paragraphs, CCFIAs are pleiotropic compounds that all demonstrate regulatory effects on NF-κB, MAPK, Akt signaling pathways and NLRP3 inflammasome. In addition, their regulatory objects and mechanisms are also specific, for example, BBR supresses NLRP3 inflammasome by directly acting on NEK7 (Zeng et al., 2021), COP inhibits inflammatory response by inhibiting Rho/ROCK pathway (Guo et al., 2013), and Pal can directly block ADAM17/EGFR signaling (Chen et al., 2020). Therefore, taken together, we anticipate that CCFIAs modulation of inflammatory pathways could exert potential therapeutic benefits against CS and its associated risks.
4 BBR ameliorates CS induced by sepsis and viral infections
David et al. proposed a unifying definition of cytokine storm in their paper “Cytokine Storms”, published in the New England Journal of Medicine, the definition is based on the following judging requirements: elevated circulating cytokine levels, acute systemic inflammatory symptoms, and secondary organ dysfunction (Fajgenbaum and June, 2020). This is a major breakthrough in this area, as there has been no consensus on a definition before. Recent clinical data has shown that infection is probably the most common trigger of CS, in other words, bacterial or viral infections induce the production of multiple cytokines, which result in fever, cell death, coagulopathy, and MOF. However, BBR demonstrated promising therapeutic effects in pathological models of CS caused by infection.
Several studies have confirmed that BBR dramatically attenuated tissue damage and death rate in mice challenged with LPS, Escherichia coli (E. coli), or caecal ligation and puncture (CLP) induced sepsis (Lee et al., 2017), (Pierpaoli et al., 2021), (Li et al., 2015) (Table 3). In LPS-induced acute lung injury (ALI) mice, Chen et al. (2022) found that BBR improved lung permeability while reduced lung injury. Mechanistically, BBR attenuated the expression of NLRP3 via regulating the Phosphorylate-NF-κB, as well as directly inhibited NLRP3 protein and modulated NLRP3 inflammasome pathways. In another study, HMGB1 was found to be a biomarker of BBR for sepsis. 13-ethylberberine (13-EBR) promoted the activation of AMPK and p38/MAPK to inhibit HMGB1, whose excessive accumulation leds to fatal endotoxemia and sepsis. Furthermore, 13-EBR inhibited the activation of NF-κB by activating AMPK, decreased the levels of HMGB1 and iNOS, and alleviated lung and liver injury (Lee et al., 2017). In addition, BBR reduced immune cell infiltration in lung tissue and improved survival rate in septic mice via inhibiting activation of NF-κB and upregulation of several pro-inflammatory transcription factors, like P-STAT3, KIF4 and Myc (Wang et al., 2020).
In addition to alleviating the systemic inflammatory response, BBR also significantly improved DIC and MOF caused by sepsis (Yuan et al., 2021), (Shi et al., 2021), (Chen et al., 2021). A study has indicated that BBR and the derivatives could attenuate coagulation activation, organ dysfunction and further decreased lethality in bacterial sepsis. The mechanism is that BBR blocked the caspase-11 inflammatory pathway by inhibiting the cytoplasmic translocation of LPS via blocking Msr1, a scavenger receptor that mediates endocytosis of LPS (Yuan et al., 2021). In an experimental model of sepsis-associated encephalopathy (SAE), BBR targeted HMGB1/RAGE signaling to suppress the quantity of inflammatory events of cell factors and astrocyte activation in the cerebrum of SAE mice, thereby alleviating cognitive impairment caused by sepsis (Shi et al., 2021) Moreover, septic cardiomyopathy (SCM) is the most common type of sepsis-related organ dysfunction. Chen et al. (2021) found that BBR reduced myocardial injury in sepsis rats, by inhibiting sepsis-induced TLR4/NF-κB signal pathway activation and decreasing the expression levels of TNF-a, IL-1β and other inflammatory factors.
Apart from serving as monotherapy, BBR can be used in combination with other drugs or as an adjuvant for sepsis-treatment. For example, BBR in combination with yohimbine reduced the tissue concentrations of MCP-1, MIP-1α and MIP-2 in the lung, liver and kidney, thus decreasing neutrophil tissue infiltration and multi-organ damage in CLP induced sepsis (Wang et al., 2016). In E. coli induced sepsis mice, BBR alone did not reduce bacterial load in mice, but when combined with imipenem, BBR enhanced its antibacterial effect and improved mouse survival rates. Moreover, BBR counteracted the massive pro-inflammatory effect during sepsis, thus making it suitable as an adjunctive treatment for E. coli-induced sepsis (Pierpaoli et al., 2021).
Disseminated viral infections can also trigger severe CS, including SARS coronavirus, such as SARS-CoV-2, and other influenza viruses, like H1N1. BBR has been reported to display antiviral and anti-inflammatory effects on a variety of viral infection models. Polyinosinic polycytidylic acid (poly I:C), a synthetic analog of double-stranded RNA (dsRNA), was used to provoke a hyper-inflammatory reaction in macrophages. Kim et al. (2021) found that BBR significantly restrained the phosphorylation of p38/MAPK, ERK1/2, IkB-α, and STAT3, as well as the production of NO, PGE2 and other inflammatory mediators in poly I:C-induced RAW 264.7 cells. In addition, BBR relieved pulmonary inflammation and reduced necrosis and inflammatory cell infiltration induced by H1N1 viral infection in mice, and the mechanism is related to suppressing of TLR7 signaling pathway (Yan et al., 2018). Likewise, BBR reduced lung injury and mortality in 10LD50 influenza virus-infected BALb/C mice (Liu et al., 2020). A recent study revealed the role of BBR to be either an effective treatment for COVID-19 inflammation or a possible component of a treatment, given that it enhanced the viability of SARS-CoV-2 Spike Protein stimulating cells and decreased the cytokines, such as TNF-α and IL-6 (Gu et al., 2021).
Severe cases of sepsis and viral infection are both important triggers for CS. Collectively, in addition to significantly inhibiting the production of cytokines that induced by infection (IFN-λ, TNF-α, IL-1β, IL-6, IL-8, G-CSF, GM-CSF, VEGF, MCP-1, and MIP-1), BBR also exerts antiviral and antibacterial activities. And mounting evidence witnessed by in vitro and in vivo studies suggested that BBR could interfere systemic inflammatory responses, organ dysfunction/injury and life-threatening conditions caused by hyperinflammatory response.
5 Clinical application of BBR in inflammatory diseases
Clinical studies have investigated the benefits of BBR in human health and diseases involving immune disorders and inflammation of various organs (Table 4). In a clinical trial aimed at investigating the anti-inflammatory effects of BBR in children with diarrhea, Chen et al. found that oral administration of BBR hydrochloride (0.2 g/day, for 1 week) significantly reduced the serum levels of pro-inflammatory factors, including TNF-α and IL-6 (Chen et al., 2015). Importantly, another study has shown that BBR (900 mg/day, for 14 days) also could significantly reverse the changes in IL-6, TNF-α and C-reactive protein (CRP) levels in patients with severe COVID-19 with diarrhoea (Zhang et al., 2021). In another double-blind randomized controlled trial, Li et al. (2010) reported that the patients received 300-mg tablets of BBR orally three times daily for 2 weeks reduced the incidence and severity of radiation-induced acute intestinal symptoms (RIAIS), which was presumed to be an inflammatory process involving in cytokines and reactive oxygen metabolites. Furthermore, a randomized, double-blind phase I trial on patients with ulcerative colitis (UC) has been shown that treatment with BBR (900 mg/day, for 3 months) significantly decreased colonic tissue inflammation, and indicated a trend of decreasing plasma levels of pro-inflammatory cytokines such as TNF-α, IL-2, IL-6, IL-8, and IL-4 (Xu et al., 2020). Collectively, the above four studies demonstrated the improvement of BBR in diarrhea and gastrointestinal inflammation.
In another clinical study, treatment with BBR (300 mg, t. i.d., for 30 days) in addition to standard significantly decreased MMP-9, ICAM-1, CRP, IL-6, MCP-1 and VCAM-1 in acute coronary syndrome (ACS) patients, and BBR may become an adjunct therapy for ACS patients following undergoing percutaneous coronary intervention since via its anti-inflammatory effect (Meng et al., 2012). Reportedly, BBR (20 mg/kg, for 6 weeks) significantly reduced the incidence of radiation-induced lung injury (RILI) and decreased the levels of ICAM-1 and TGF-β, which are leading to lung injury (Liu et al., 2008). Additionally, in a randomized and single-blind study, BBR (300 mg, t. i.d, for 15 days) significantly reduced serum levels of CRP, TNF-α and IL-6 in patients with acute myocardial infarction compared with control, indicating that BBR provides cardiac protection against cardiac injury (Qing et al., 2018). Interestingly, patients with hypertriglyceridemia are more likely to trigger CS after COVID-19 infection, while BBR reduced blood glucose, triglycerides and cholesterol by directly regulating hepatic lipid metabolism, which might relate to the activation of hepatic AMPK pathway (Yan et al., 2015), (Fajgenbaum and June, 2020).
However, toxicity and safety should be considered in priority when evaluating drug efficacy. It has been reported that the LD50 value of BBR was, respectively, 329 (oral), 9.0386 (i.v) and 57.6103 (i.p) mg/kg, which indicates BBR has relatively wide range of safety, and the common adverse events were mild rash, occasional nausea, vomiting, and fever (Kheir et al., 2010). On the other hand, the dose-effect relationship determines the effectiveness of the drug. Modern pharmacological studies have demonstrated that BBR (50 mg/kg) can be distributed in the heart, spleen, liver, kidney, brain, intestinal tract, muscle and fat and other tissues after oral administration. Except for the intestinal concentration of 4,000 ng/g, other tissues or organ concentration is 200 ng/g. Of note, there was an obvious non-linear relationship between the concentration of BBR in plasma and the oral dose (Chen et al., 2020), and even the highest dosage applied in animal study actually cannot reach the minimum concentration level used in cell experiments dueing to the poor absorption and low availability. Hence, more investigation is needed to improve the bioavailability of BBR and meanwhile reach the balance between toxicological safety and therapeutic efficacy.
In brief, these clinical trials have demonstrated effects of BBR include antioxidation, immune modulation, lipid-metabolism-modulating. And a wide therapeutic window of anti-inflammatory by targeting multiple organs and targets in inflammation response. However, here are still important issues regarding the therapeutic efficacy of the modulatory effects of BBR on pro-inflammatory signaling pathways, therefore, further high-quality clinical trials are needed to determine and validate the modulatory effects of BBR.
6 Future perspectives
As mentioned above, CCFIAs exerts potent anti-inflammatory activities through modulatory effect on several signaling pathways, but most reports focused on reducing inflammatory responses and reversing pathogenesis development through classic inflammatory pathways. However, we should also dig into other novel signaling pathways or other aspects of known signaling pathways, such as Wnt, Notch, and MITA, etc., (Sun et al., 2018), (Keewan and Naser, 2020), (Zhou et al., 2019). The targets of these signaling pathways also provide a reference for studying the mechanism of CCFIAs for CS treatment, which has vital clinical implications for the future development of CCFIAs as CS inhibitors. In addition, compared with agents showing only antiviral or anti-inflammatory activity, CCFIAs may exhibit more promising therapeutic potential with respect to the treatment of CS fueled by infection, but the molecular mechanism of CCFIAs in human body has not been fully revealed, thus further clinical studies are in urgent need to support the clinical application of CCFIAs.
To date, there have been many studies on the efficacy and mechanisms of BBR, COP, and PAL in various models of inflammation, whereas comparatively little attention has been devoted to other isoquinoline alkaloids in CCF, such as jatrorrhizine (JH) and epiberberine (EPI). However, JH has been shown to prevent the progression of rheumatoid arthritis (RA) by controling the intensive inflammation. Qiu et al. found that JH inhibited the production of inflammatory mediators such as TNF-α, IL-1β, IL-6, MMP-2, and MMP-3 by blocking the activation of ERK, P38, and NF-κB signaling pathways, suggesting that JH may play a therapeutic role in RA through regulating multiple targets (Qiu et al., 2018). In addition, EPI in the fingerprints possessed positive and relative higher contribution in the anti-inflammatory of CCF, and might be one of the main anti-inflammatory components (Li et al., 2015).
As described previously, CCFIAs are insoluble in water and extensively metabolized in vivo, and accordingly their oral absorption and bioavailability are poor, which may restrain biological activity. In recent years, researchers have tried different strategies to improve oral bioavailability of CCFIAs. For example, when BBR was made into nanosuspension, compared with active pharmaceutical ingredients for BBR, the solubility and pharmacological activity of nanopreparation was enhanced (Wang et al., 2015). Besides, specific nanoformulations will also improve permeability and oral bioavailability, such as solid dispersions, nanoemulsions and liposomes (Li et al., 2017), (Xu et al., 2019), (Li et al., 2018). However, strategies to improve oral bioavailability of CCFIAs have only been validated at animal and cellular levels, lacking robust clinical findings, thus more long-term clinical trials are needed.
7 Concluding remarks
Although few findings are showing the effectiveness of CCFIAs treatment in COVID-19 disease, they have been proved to prevent excessive inflammation by modulating inflammatory signaling pathways and their downstream components in various in vitro and in vivo studies. In addition, the aforementioned evidence demonstrated that BBR ameliorates CS induced by sepsis and viral infection, thereby suggesting the beneficial modulatory effects of CCFIAs in reducing CS.
Author contributions
YL: Writing–original draft and conceptualization. HW, JW, and XM: Writing–review, supervision and project administration. All authors read the manuscript and approved it.
Funding
This work is supported by the National Natural Science Foundation of China [81773974, 82104491], the National Key Research and Development Program of China [2017YFC1703904], the “Xinglin Scholar” Scientific Research Promotion Plan of Chengdu University of Traditional Chinese Medicine [030/030055224], the Central Guiding Local Science and Technology Development Special Project of Sichuan Province [2021ZYD0077], the Key R&D and Transformation Program of the Science and Technology Department of Qinghai Province [2020-SF-C33], and the Postdoctoral Science Foundation of China [2021M693789].
Conflict of interest
The authors declare that the research was conducted in the absence of any commercial or financial relationships that could be construed as a potential conflict of interest.
Publisher’s note
All claims expressed in this article are solely those of the authors and do not necessarily represent those of their affiliated organizations, or those of the publisher, the editors and the reviewers. Any product that may be evaluated in this article, or claim that may be made by its manufacturer, is not guaranteed or endorsed by the publisher.
References
Acar, L., Atalan, N., Karagedik, E. H., and Ergen, A. (2018). Tumour necrosis factor-alpha and nuclear factor-kappa B gene variants in sepsis. Balk. Med. J. 35 (1), 30–35. doi:10.4274/balkanmedj.2017.0246
Ahmadian, E., Hosseiniyan Khatibi, S. M., Razi Soofiyani, S., Abediazar, S., Shoja, M. M., Ardalan, M., et al. (2021). Covid-19 and kidney injury: Pathophysiology and molecular mechanisms. Rev. Med. Virol. 31 (3), e2176. doi:10.1002/rmv.2176
Alqahtani, S. A., and Schattenberg, J. M. (2020). Liver injury in COVID-19: The current evidence. United Eur. Gastroenterol. J. 8 (5), 509–519. doi:10.1177/2050640620924157
Asiedu, S. O., Kwofie, S. K., Broni, E., and Wilson, M. D. (2021). Computational identification of potential anti-inflammatory natural compounds targeting the p38 mitogen-activated protein kinase (MAPK): Implications for COVID-19-induced cytokine storm. Biomolecules 11 (5), 653. doi:10.3390/biom11050653
Bavishi, C., Bonow, R. O., Trivedi, V., Abbott, J. D., Messerli, F. H., and Bhatt, D. L. (2020). Special article - acute myocardial injury in patients hospitalized with COVID-19 infection: A review. Prog. Cardiovasc. Dis. 63 (5), 682–689. doi:10.1016/j.pcad.2020.05.013
Brownell, J., Bruckner, J., Wagoner, J., Thomas, E., Loo, Y. M., Gale, M., et al. (2014). Direct, interferon-independent activation of the CXCL10 promoter by NF-κB and interferon regulatory factor 3 during hepatitis C virus infection. J. Virol. 88 (3), 1582–1590. doi:10.1128/jvi.02007-13
Bulek, K., Zhao, J., Liao, Y., Rana, N., Corridoni, D., Antanaviciute, A., et al. (2020). Epithelial-derived gasdermin D mediates nonlytic IL-1β release during experimental colitis. J. Clin. Invest. 130 (8), 4218–4234. doi:10.1172/jci138103
Channappanavar, R., Fehr, A. R., Vijay, R., Mack, M., Zhao, J., Meyerholz, D. K., et al. (2016). Dysregulated type I interferon and inflammatory monocyte-macrophage responses cause lethal pneumonia in SARS-CoV-infected mice. Cell Host Microbe 19 (2), 181–193. doi:10.1016/j.chom.2016.01.007
Channappanavar, R., and Perlman, S. (2017). Pathogenic human coronavirus infections: Causes and consequences of cytokine storm and immunopathology. Semin. Immunopathol. 39 (5), 529–539. doi:10.1007/s00281-017-0629-x
Chen, H. B., Luo, C. D., Liang, J. L., Zhang, Z. B., Lin, G. S., Wu, J. Z., et al. (2017). Anti-inflammatory activity of coptisine free base in mice through inhibition of NF-κB and MAPK signaling pathways. Eur. J. Pharmacol. 811, 222–231. doi:10.1016/j.ejphar.2017.06.027
Chen, H., Liu, Q., Liu, X., and Jin, J. (2021). Berberine attenuates septic cardiomyopathy by inhibiting TLR4/NF-κB signalling in rats. Pharm. Biol. 59 (1), 121–128. doi:10.1080/13880209.2021.1877736
Chen, I. Y., Chang, S. C., Wu, H. Y., Yu, T. C., Wei, W. C., Lin, S., et al. (2010). Upregulation of the chemokine (C-C motif) ligand 2 via a severe acute respiratory syndrome coronavirus spike-ACE2 signaling pathway. J. Virol. 84 (15), 7703–7712. doi:10.1128/jvi.02560-09
Chen, H., Liu, Q. S., Qing-Sheng, L. E., Wang, X., and Pediatrics, D. (2015). Effect of berberine combined with levofloxacin on myocardial enzymes,isozymes and serum inflammatory factors in children with diarrhea. Chin. J. Biochem. Pharm..
Chen, J., Huang, Y., Bian, X., and He, Y. (2022). Berberine ameliorates inflammation in acute lung injury via NF-κB/Nlrp3 signaling pathway. Front. Nutr. 9, 851255. doi:10.3389/fnut.2022.851255
Chen, X., Wang, R., Bao, C., Zhang, J., Zhang, J., Li, R., et al. (2020). Palmatine ameliorates Helicobacter pylori-induced chronic atrophic gastritis by inhibiting MMP-10 through ADAM17/EGFR. Eur. J. Pharmacol. 882, 173267. doi:10.1016/j.ejphar.2020.173267
Choi, S. B., Bae, G. S., Jo, I. J., Song, H. J., and Park, S. J. (2017). Effects of berberine on acute necrotizing pancreatitis and associated lung injury. Pancreas 46 (8), 1046–1055. doi:10.1097/mpa.0000000000000877
Choudhary, S., Sharma, K., and Silakari, O. (2021). The interplay between inflammatory pathways and COVID-19: A critical review on pathogenesis and therapeutic options. Microb. Pathog. 150, 104673. doi:10.1016/j.micpath.2020.104673
Chuang, H. C., Chen, Y. M., Chen, M. H., Hung, W. T., Yang, H. Y., Tseng, Y. H., et al. (2019). AhR-ROR-γt complex is a therapeutic target for MAP4K3/GLK(high)IL-17A(high) subpopulation of systemic lupus erythematosus. Faseb J. 33 (10), 11469–11480. doi:10.1096/fj.201900105RR
Cortese, M., Lee, J. Y., Cerikan, B., Neufeldt, C. J., Oorschot, V. M. J., Köhrer, S., et al. (2020). Integrative imaging reveals SARS-CoV-2-induced reshaping of subcellular morphologies. Cell Host Microbe 28 (6), 853–866. e855. doi:10.1016/j.chom.2020.11.003
Dinesh, P., and Rasool, M. (2018). Berberine inhibits IL-21/IL-21R mediated inflammatory proliferation of fibroblast-like synoviocytes through the attenuation of PI3K/Akt signaling pathway and ameliorates IL-21 mediated osteoclastogenesis. Cytokine 106, 54–66. doi:10.1016/j.cyto.2018.03.005
Dinesh, P., and Rasool, M. (2017). Berberine, an isoquinoline alkaloid suppresses TXNIP mediated NLRP3 inflammasome activation in MSU crystal stimulated RAW 264.7 macrophages through the upregulation of Nrf2 transcription factor and alleviates MSU crystal induced inflammation in rats. Int. Immunopharmacol. 44, 26–37. doi:10.1016/j.intimp.2016.12.031
Fajgenbaum, D. C., and June, C. H. (2020). Cytokine storm. N. Engl. J. Med. 383 (23), 2255–2273. doi:10.1056/NEJMra2026131
Fang, C., Xie, L., Liu, C., Fu, C., Ye, W., Liu, H., et al. (2018). Berberine ameliorates neonatal necrotizing enterocolitis by activating the phosphoinositide 3-kinase/protein kinase B signaling pathway. Exp. Ther. Med. 15 (4), 3530–3536. doi:10.3892/etm.2018.5858
Fara, A., Mitrev, Z., Rosalia, R. A., and Assas, B. M. (2020). Cytokine storm and COVID-19: A chronicle of pro-inflammatory cytokines. Open Biol. 10 (9), 200160. doi:10.1098/rsob.200160
Feng, M., Kong, S. Z., Wang, Z. X., He, K., Zou, Z. Y., Hu, Y. R., et al. (2017). The protective effect of coptisine on experimental atherosclerosis ApoE(-/-) mice is mediated by MAPK/NF-κB-dependent pathway. Biomed. Pharmacother. 93, 721–729. doi:10.1016/j.biopha.2017.07.002
Fu, S., Ni, S., Wang, D., and Hong, T. (2018). Coptisine suppresses mast cell degranulation and ovalbumin-induced allergic rhinitis. Molecules 23 (11), E3039. doi:10.3390/molecules23113039
Grimes, J. M., and Grimes, K. V. (2020). p38 MAPK inhibition: A promising therapeutic approach for COVID-19. J. Mol. Cell. Cardiol. 144, 63–65. doi:10.1016/j.yjmcc.2020.05.007
Gu, L., Ke, Y., Gan, J., and Li, X. (2021). Berberine suppresses bone loss and inflammation in ligature-induced periodontitis through promotion of the G protein-coupled estrogen receptor-mediated inactivation of the p38MAPK/NF-κB pathway. Arch. Oral Biol. 122, 104992. doi:10.1016/j.archoralbio.2020.104992
Guo, J., Wang, S. B., Yuan, T. Y., Wu, Y. J., Yan, Y., Li, L., et al. (2013). Coptisine protects rat heart against myocardial ischemia/reperfusion injury by suppressing myocardial apoptosis and inflammation. Atherosclerosis 231 (2), 384–391. doi:10.1016/j.atherosclerosis.2013.10.003
Hu, B., Huang, S., and Yin, L. (2021). The cytokine storm and COVID-19. J. Med. Virol. 93 (1), 250–256. doi:10.1002/jmv.26232
Huang, C., Wang, Y., Li, X., Ren, L., Zhao, J., Hu, Y., et al. (2020). Clinical features of patients infected with 2019 novel coronavirus in Wuhan, China. Lancet 395 (10223), 497–506. doi:10.1016/s0140-6736(20)30183-5
Huang, Z., Ye, B., Han, J., Kong, F., Shan, P., Lu, Z., et al. (2018). NACHT, LRR and PYD domains-containing protein 3 inflammasome is activated and inhibited by berberine via toll-like receptor 4/myeloid differentiation primary response gene 88/nuclear factor-κB pathway, in phorbol 12-myristate 13-acetate-induced macrophages. Mol. Med. Rep. 17 (2), 2673–2680. doi:10.3892/mmr.2017.8189
Iwasaki, M., Saito, J., Zhao, H., Sakamoto, A., Hirota, K., and Ma, D. (2021). Inflammation triggered by SARS-CoV-2 and ACE2 augment drives multiple organ failure of severe COVID-19: Molecular mechanisms and implications. Inflammation 44 (1), 13–34. doi:10.1007/s10753-020-01337-3
Jamilloux, Y., Henry, T., Belot, A., Viel, S., Fauter, M., El Jammal, T., et al. (2020). Should we stimulate or suppress immune responses in COVID-19? Cytokine and anti-cytokine interventions. Autoimmun. Rev. 19 (7), 102567. doi:10.1016/j.autrev.2020.102567
Jiang, X. W., Zhang, Y., Zhu, Y. L., Zhang, H., Lu, K., Li, F. F., et al. (2013). Effects of berberine gelatin on recurrent aphthous stomatitis: a randomized, placebo-controlled, double-blind trial in a Chinese cohort. Oral. Surg. Oral. Med. Oral. Pathol. Oral. Radiol. 115(2), 212–217. doi:10.1016/j.oooo.2012.09.009
Junqueira, C., Crespo, Ã., Ranjbar, S., Lewandrowski, M., Ingber, J., de Lacerda, L. B., et al. (2021). SARS-CoV-2 infects blood monocytes to activate NLRP3 and AIM2 inflammasomes, pyroptosis and cytokine release. Res. Sq. 1, 1. doi:10.21203/rs.3.rs-153628/v1
Keewan, E., and Naser, S. A. (2020). The role of Notch signaling in macrophages during inflammation and infection: Implication in rheumatoid arthritis? Cells 9 (1), E111. doi:10.3390/cells9010111
Kheir, M. M., Wang, Y., Hua, L., Hu, J., Li, L., Lei, F., et al. (2010). Acute toxicity of berberine and its correlation with the blood concentration in mice. Food Chem. Toxicol. 48 (4), 1105–1110. doi:10.1016/j.fct.2010.01.033
Kim, H. J., Kim, Y. J., and Park, W. (2021a). Berberine modulates hyper-inflammation in mouse macrophages stimulated with polyinosinic-polycytidylic acid via calcium-CHOP/STAT pathway. Sci. Rep. 11 (1), 11298. doi:10.1038/s41598-021-90752-z
Kim, J. S., Lee, J. Y., Yang, J. W., Lee, K. H., Effenberger, M., Szpirt, W., et al. (2021b). Immunopathogenesis and treatment of cytokine storm in COVID-19. Theranostics 11 (1), 316–329. doi:10.7150/thno.49713
Kim, Y. M., Ha, Y. M., Jin, Y. C., Shi, L. Y., Lee, Y. S., Kim, H. J., et al. (2009). Palmatine from Coptidis rhizoma reduces ischemia-reperfusion-mediated acute myocardial injury in the rat. Food Chem. Toxicol. 47 (8), 2097–2102. doi:10.1016/j.fct.2009.05.031
Koshiba, R., Yanai, H., Matsuda, A., Goto, A., Nakajima, A., Negishi, H., et al. (2013). Regulation of cooperative function of the Il12b enhancer and promoter by the interferon regulatory factors 3 and 5. Biochem. Biophys. Res. Commun. 430 (1), 95–100. doi:10.1016/j.bbrc.2012.11.006
Lee, D. U., Ko, Y. S., Kim, H. J., and Chang, K. C. (2017). 13-Ethylberberine reduces HMGB1 release through AMPK activation in LPS-activated RAW264.7 cells and protects endotoxemic mice from organ damage. Biomed. Pharmacother. 86, 48–56. doi:10.1016/j.biopha.2016.11.099
Leonard, W. J., and O'Shea, J. J. (1998). Jaks and STATs: Biological implications. Annu. Rev. Immunol. 16, 293–322. doi:10.1146/annurev.immunol.16.1.293
Li, B., Niu, S., Geng, H., Yang, C., and Zhao, C. (2021). Berberine Attenuates Neonatal Sepsis in Mice By Inhibiting FOXA1 and NF-κB Signal Transduction Via the Induction of MiR-132-3p. Inflammation. 44 (6), 2395–2406. doi:10.1007/s10753-021-01510-2
Li, C., Ai, G., Wang, Y., Lu, Q., Luo, C., Tan, L., et al. (2020a). Oxyberberine, a novel gut microbiota-mediated metabolite of berberine, possesses superior anti-colitis effect: Impact on intestinal epithelial barrier, gut microbiota profile and TLR4-MyD88-NF-κB pathway. Pharmacol. Res. 152, 104603. doi:10.1016/j.phrs.2019.104603
Li, C. L., Tan, L. H., Wang, Y. F., Luo, C. D., Chen, H. B., Lu, Q., et al. (2019a). Comparison of anti-inflammatory effects of berberine, and its natural oxidative and reduced derivatives from Rhizoma Coptidis in vitro and in vivo. Phytomedicine 52, 272–283. doi:10.1016/j.phymed.2018.09.228
Li, G. H., Wang, D. L., Hu, Y. D., Pu, P., Li, D. Z., Wang, W. D., et al. (2010). Berberine inhibits acute radiation intestinal syndrome in human with abdomen radiotherapy. Med. Oncol. 27 (3), 919–925. doi:10.1007/s12032-009-9307-8
Li, G. X., Wang, X. M., Jiang, T., Gong, J. F., Niu, L. Y., and Li, N. (2015a). Berberine prevents intestinal mucosal barrier damage during early phase of sepsis in rat through the toll-like receptors signaling pathway. Korean J. Physiol. Pharmacol. 19 (1), 1–7. doi:10.4196/kjpp.2015.19.1.1
Li, H., Chen, C., Hu, F., Wang, J., Zhao, Q., Gale, R. P., et al. (2020b). Impact of corticosteroid therapy on outcomes of persons with SARS-CoV-2, SARS-CoV, or MERS-CoV infection: A systematic review and meta-analysis. Leukemia 34 (6), 1503–1511. doi:10.1038/s41375-020-0848-3
Li, H., Feng, C., Fan, C., Yang, Y., Yang, X., Lu, H., et al. (2020c). Intervention of oncostatin M-driven mucosal inflammation by berberine exerts therapeutic property in chronic ulcerative colitis. Cell Death Dis. 11 (4), 271. doi:10.1038/s41419-020-2470-8
Li, J., Yang, L., Shen, R., Gong, L., Tian, Z., Qiu, H., et al. (2018a). Self-nanoemulsifying system improves oral absorption and enhances anti-acute myeloid leukemia activity of berberine. J. Nanobiotechnology 16 (1), 76. doi:10.1186/s12951-018-0402-x
Li, J. Y., Wang, X. B., Luo, J. G., and Kong, L. Y. (2015b). Seasonal variation of alkaloid contents and anti-inflammatory activity of rhizoma coptidis based on fingerprints combined with chemometrics methods. J. Chromatogr. Sci. 53 (7), 1131–1139. doi:10.1093/chromsci/bmu175
Li, W., Liu, F., Wang, J., Long, M., and Wang, Z. (2018b). MicroRNA-21-Mediated inhibition of mast cell degranulation involved in the protective effect of berberine on 2, 4-dinitrofluorobenzene-induced allergic contact dermatitis in rats via p38 pathway. Inflammation 41 (2), 689–699. doi:10.1007/s10753-017-0723-1
Li, W., Yin, N., Tao, W., Wang, Q., Fan, H., and Wang, Z. (2019b). Berberine suppresses IL-33-induced inflammatory responses in mast cells by inactivating NF-κB and p38 signaling. Int. Immunopharmacol. 66, 82–90. doi:10.1016/j.intimp.2018.11.009
Li, X., He, P., Hou, Y., Chen, S., Xiao, Z., Zhan, J., et al. (2019c). Berberine inhibits the interleukin-1 beta-induced inflammatory response via MAPK downregulation in rat articular chondrocytes. Drug Dev. Res. 80 (5), 637–645. doi:10.1002/ddr.21541
Li, Y. J., Hu, X. B., Lu, X. L., Liao, D. H., Tang, T. T., Wu, J. Y., et al. (2017). Nanoemulsion-based delivery system for enhanced oral bioavailability and caco-2 cell monolayers permeability of berberine hydrochloride. Drug Deliv. 24 (1), 1868–1873. doi:10.1080/10717544.2017.1410257
Li, Z., Zheng, J., Zhang, N., and Li, C. (2016). Berberine improves airway inflammation and inhibits NF-κB signaling pathway in an ovalbumin-induced rat model of asthma. J. Asthma 53 (10), 999–1005. doi:10.1080/02770903.2016.1180530
Lim, K. H., and Staudt, L. M. (2013). Toll-like receptor signaling. Cold Spring Harb. Perspect. Biol. 5 (1), a011247. doi:10.1101/cshperspect.a011247
Liu, H., You, L., Wu, J., Zhao, M., Guo, R., Zhang, H., et al. (2020). Berberine suppresses influenza virus-triggered NLRP3 inflammasome activation in macrophages by inducing mitophagy and decreasing mitochondrial ROS. J. Leukoc. Biol. 108 (1), 253–266. doi:10.1002/jlb.3ma0320-358rr
Liu, X., Zhang, X., Ye, L., and Yuan, H. (2016). Protective mechanisms of berberine against experimental autoimmune myocarditis in a rat model. Biomed. Pharmacother. 79, 222–230. doi:10.1016/j.biopha.2016.02.015
Liu, Y., Liu, X., Hua, W., Wei, Q., Fang, X., Zhao, Z., et al. (2018). Berberine inhibits macrophage M1 polarization via AKT1/SOCS1/NF-κB signaling pathway to protect against DSS-induced colitis. Int. Immunopharmacol. 57, 121–131. doi:10.1016/j.intimp.2018.01.049
Liu, Y., Yu, H., Zhang, C., Cheng, Y., Hu, L., Meng, X., et al. (2008). Protective effects of berberine on radiation-induced lung injury via intercellular adhesion molecular-1 and transforming growth factor-beta-1 in patients with lung cancer. Eur. J. Cancer. 44 (16), 2425–2432. doi:10.1016/j.ejca.2008.07.040
Liu, Z., Wang, W., Luo, J., Zhang, Y., Zhang, Y., Gan, Z., et al. (2021). Anti-apoptotic role of sanhuang xiexin decoction and anisodamine in endotoxemia. Front. Pharmacol. 12, 531325. doi:10.3389/fphar.2021.531325
Ma, H., Zhang, Y., Wang, J., Guo, W., Hu, G., Xie, S., et al. (2021). Palmatine attenuates LPS-induced inflammatory response in mouse mammary epithelial cells through inhibiting ERK1/2, P38 and Akt/NF-кB signalling pathways. J. Anim. Physiol. Anim. Nutr. 105 (1), 183–190. doi:10.1111/jpn.13440
Ma, J., Chan, C. C., Huang, W. C., and Kuo, M. L. (2020). Berberine inhibits pro-inflammatory cytokine-induced IL-6 and CCL11 production via modulation of STAT6 pathway in human bronchial epithelial cells. Int. J. Med. Sci. 17 (10), 1464–1473. doi:10.7150/ijms.45400
Mai, C. T., Wu, M. M., Wang, C. L., Su, Z. R., Cheng, Y. Y., and Zhang, X. J. (2019). Palmatine attenuated dextran sulfate sodium (DSS)-induced colitis via promoting mitophagy-mediated NLRP3 inflammasome inactivation. Mol. Immunol. 105, 76–85. doi:10.1016/j.molimm.2018.10.015
Mai, W., Xu, Y., Xu, J., Zhao, D., Ye, L., Yu, G., et al. (2020). Berberine inhibits nod-like receptor family pyrin domain containing 3 inflammasome activation and pyroptosis in nonalcoholic steatohepatitis via the ROS/TXNIP Axis. Front. Pharmacol. 11, 185. doi:10.3389/fphar.2020.00185
Manjili, R. H., Zarei, M., Habibi, M., and Manjili, M. H. (2020). COVID-19 as an acute inflammatory disease. J. Immunol. 205 (1), 12–19. doi:10.4049/jimmunol.2000413
Medzhitov, R. (2007). Recognition of microorganisms and activation of the immune response. Nature 449 (7164), 819–826. doi:10.1038/nature06246
Meng, F. C., Wu, Z. F., Yin, Z. Q., Lin, L. G., Wang, R., and Zhang, Q. W. (2018). Coptidis rhizoma and its main bioactive components: Recent advances in chemical investigation, quality evaluation and pharmacological activity. Chin. Med. 13, 13. doi:10.1186/s13020-018-0171-3
Meng, S., Wang, L. S., Huang, Z. Q., Zhou, Q., Sun, Y. G., Cao, J. T., et al. (2012). Berberine ameliorates inflammation in patients with acute coronary syndrome following percutaneous coronary intervention. Clin. Exp. Pharmacol. Physiol. 39 (5), 406–411. doi:10.1111/j.1440-1681.2012.05670.x
Ni, W., Yang, X., Yang, D., Bao, J., Li, R., Xiao, Y., et al. (2020). Role of angiotensin-converting enzyme 2 (ACE2) in COVID-19. Crit. Care 24 (1), 422. doi:10.1186/s13054-020-03120-0
O'Shea, J. J., Schwartz, D. M., Villarino, A. V., Gadina, M., McInnes, I. B., and Laurence, A. (2015). The JAK-STAT pathway: Impact on human disease and therapeutic intervention. Annu. Rev. Med. 66, 311–328. doi:10.1146/annurev-med-051113-024537
Park, A., and Iwasaki, A. (2020). Type I and type III interferons - induction, signaling, evasion, and application to combat COVID-19. Cell Host Microbe 27 (6), 870–878. doi:10.1016/j.chom.2020.05.008
Pei, C., Zhang, Y., Wang, P., Zhang, B., Fang, L., Liu, B., et al. (2019). Berberine alleviates oxidized low-density lipoprotein-induced macrophage activation by downregulating galectin-3 via the NF-κB and AMPK signaling pathways. Phytother. Res. 33 (2), 294–308. doi:10.1002/ptr.6217
Pierpaoli, E., Cirioni, O., Simonetti, O., Orlando, F., Giacometti, A., Lombardi, P., et al. (2021). Potential application of berberine in the treatment of Escherichia coli sepsis. Nat. Prod. Res. 35 (22), 4779–4784. doi:10.1080/14786419.2020.1721729
Qing, Y., Dong, X., Hongli, L., and Yanhui, L. (2018). Berberine promoted myocardial protection of postoperative patients through regulating myocardial autophagy. Biomed. Pharmacother. 105, 1050–1053. doi:10.1016/j.biopha.2018.06.088
Qiu, H., Sun, S., Ma, X., Cui, C., Chen, G., Liu, Z., et al. (2018). Jatrorrhizine hydrochloride suppresses proliferation, migration, and secretion of synoviocytes in vitro and ameliorates rat models of rheumatoid arthritis in vivo. Int. J. Mol. Sci. 19 (5), E1514. doi:10.3390/ijms19051514
Quirch, M., Lee, J., and Rehman, S. (2020). Hazards of the cytokine storm and cytokine-targeted therapy in patients with COVID-19: Review. J. Med. Internet Res. 22 (8), e20193. doi:10.2196/20193
Ran, Q., Wang, J., Wang, L., Zeng, H. R., Yang, X. B., and Huang, Q. W. (2019). Rhizoma coptidis as a potential treatment agent for type 2 diabetes mellitus and the underlying mechanisms: A review. Front. Pharmacol. 10, 805. doi:10.3389/fphar.2019.00805
Seger, R., and Krebs, E. G. (1995). The MAPK signaling cascade. FASEB J. 9 (9), 726–735. doi:10.1096/fasebj.9.9.7601337
Shah, A. (2020). Novel coronavirus-induced NLRP3 inflammasome activation: A potential drug target in the treatment of COVID-19. Front. Immunol. 11, 1021. doi:10.3389/fimmu.2020.01021
Sharif, H., Wang, L., Wang, W. L., Magupalli, V. G., Andreeva, L., Qiao, Q., et al. (2019). Structural mechanism for NEK7-licensed activation of NLRP3 inflammasome. Nature 570 (7761), 338–343. doi:10.1038/s41586-019-1295-z
Shi, J., Xu, H., Cavagnaro, M. J., Li, X., and Fang, J. (2021). Blocking HMGB1/RAGE signaling by berberine alleviates A1 astrocyte and attenuates sepsis-associated encephalopathy. Front. Pharmacol. 12, 760186. doi:10.3389/fphar.2021.760186
Song, J., Wu, Q., Jiang, J., Sun, D., Wang, F., Xin, B., et al. (2020). Berberine reduces inflammation of human dental pulp fibroblast via miR-21/KBTBD7 axis. Arch. Oral Biol. 110, 104630. doi:10.1016/j.archoralbio.2019.104630
Song, J., Yang, J., Jing, S., Yan, C., Huan, X., Chen, S., et al. (2022). Berberine attenuates experimental autoimmune myasthenia gravis via rebalancing the T cell subsets. J. Neuroimmunol. 362, 577787. doi:10.1016/j.jneuroim.2021.577787
Sun, M. S., Zhang, J., Jiang, L. Q., Pan, Y. X., Tan, J. Y., Yu, F., et al. (2018). TMED2 potentiates cellular IFN responses to DNA viruses by reinforcing MITA dimerization and facilitating its trafficking. Cell Rep. 25 (11), 3086–3098. doi:10.1016/j.celrep.2018.11.048
Sun, S., Zhang, X., Xu, M., Zhang, F., Tian, F., Cui, J., et al. (2019). Berberine downregulates CDC6 and inhibits proliferation via targeting JAK-STAT3 signaling in keratinocytes. Cell Death Dis. 10 (4), 274. doi:10.1038/s41419-019-1510-8
Toldo, S., and Abbate, A. (2018). The NLRP3 inflammasome in acute myocardial infarction. Nat. Rev. Cardiol. 15 (4), 203–214. doi:10.1038/nrcardio.2017.161
Wang, H., Tu, S., Yang, S., Shen, P., Huang, Y., Ba, X., et al. (2019a). Berberine modulates LPA function to inhibit the proliferation and inflammation of FLS-RA via p38/ERK MAPK pathway mediated by LPA(1). Evid. Based. Complement. Altern. Med. 2019, 2580207. doi:10.1155/2019/2580207
Wang, W., Zha, G., Zou, J. J., Wang, X., Li, C. N., and Wu, X. J. (2019b). Berberine attenuates cigarette smoke extract-induced airway inflammation in mice: Involvement of TGF-β1/smads signaling pathway. Curr. Med. Sci. 39 (5), 748–753. doi:10.1007/s11596-019-2101-8
Wang, Y., Du, P., and Jiang, D. (2020). Berberine functions as a negative regulator in lipopolysaccharide -induced sepsis by suppressing NF-κB and IL-6 mediated STAT3 activation. Pathog. Dis. 78 (7), ftaa047. doi:10.1093/femspd/ftaa047
Wang, Y., Liu, J., Huang, Z., Li, Y., Liang, Y., Luo, C., et al. (2021). Coptisine ameliorates DSS-induced ulcerative colitis via improving intestinal barrier dysfunction and suppressing inflammatory response. Eur. J. Pharmacol. 896, 173912. doi:10.1016/j.ejphar.2021.173912
Wang, Y., Wang, F., Yang, D., Tang, X., Li, H., Lv, X., et al. (2016). Berberine in combination with yohimbine attenuates sepsis-induced neutrophil tissue infiltration and multiorgan dysfunction partly via IL-10-mediated inhibition of CCR2 expression in neutrophils. Int. Immunopharmacol. 35, 217–225. doi:10.1016/j.intimp.2016.03.041
Wang, Z., Chen, Z., Yang, S., Wang, Y., Huang, Z., Gao, J., et al. (2014). Berberine ameliorates collagen-induced arthritis in rats associated with anti-inflammatory and anti-angiogenic effects. Inflammation 37 (5), 1789–1798. doi:10.1007/s10753-014-9909-y
Wang, Z., Wu, J., Zhou, Q., Wang, Y., and Chen, T. (2015). Berberine nanosuspension enhances hypoglycemic efficacy on streptozotocin induced diabetic C57BL/6 mice. Evid. Based. Complement. Altern. Med. 2015, 239749. doi:10.1155/2015/239749
Weckmann, A. L., and Alcocer-Varela, J. (1996). Cytokine inhibitors in autoimmune disease. Semin. Arthritis Rheum. 26 (2), 539–557. doi:10.1016/s0049-0172(96)80042-4
Wu, J., Hu, Y., Xiang, L., Li, S., Yuan, Y., Chen, X., et al. (2016). San-huang-xie-xin-tang constituents exert drug-drug interaction of mutual reinforcement at both pharmacodynamics and pharmacokinetic level: A review. Front. Pharmacol. 7, 448. doi:10.3389/fphar.2016.00448
Wu, J., Luo, Y., Jiang, Q., Li, S., Huang, W., Xiang, L., et al. (2019). Coptisine from Coptis chinensis blocks NLRP3 inflammasome activation by inhibiting caspase-1. Pharmacol. Res. 147, 104348. doi:10.1016/j.phrs.2019.104348
Wu, J., Sun, J., and Meng, X. (2021). Pyroptosis by caspase-11 inflammasome-Gasdermin D pathway in autoimmune diseases. Pharmacol. Res. 165, 105408. doi:10.1016/j.phrs.2020.105408
Xie, Q., Li, J., Li, H., Udeshi, N. D., Svinkina, T., Orlin, D., et al. (2022). Transcription factor Acj6 controls dendrite targeting via a combinatorial cell-surface code. Neuron 110, 2299–2314. e8. doi:10.1016/j.neuron.2022.04.026
Xu, D., Wan, C., Wang, T., Tian, P., Li, D., Wu, Y., et al. (2015). Berberine attenuates cigarette smoke-induced airway inflammation and mucus hypersecretion in mice. Int. J. Clin. Exp. Med. 8 (6), 8641–8647.
Xu, H. Y., Liu, C. S., Huang, C. L., Chen, L., Zheng, Y. R., Huang, S. H., et al. (2019). Nanoemulsion improves hypoglycemic efficacy of berberine by overcoming its gastrointestinal challenge. Colloids Surf. B Biointerfaces 181, 927–934. doi:10.1016/j.colsurfb.2019.06.006
Xu, L., Zhang, Y., Xue, X., Liu, J., Li, Z. S., Yang, G. Y., et al. (2020). A phase I trial of berberine in Chinese with ulcerative colitis. Cancer Prev. Res. 13 (1), 117–126. doi:10.1158/1940-6207.Capr-19-0258
Xu, L., Zheng, X., Wang, Y., Fan, Q., Zhang, M., Li, R., et al. (2018). Berberine protects acute liver failure in mice through inhibiting inflammation and mitochondria-dependent apoptosis. Eur. J. Pharmacol. 819, 161–168. doi:10.1016/j.ejphar.2017.11.013
Yan, B., Wang, D., Dong, S., Cheng, Z., Na, L., Sang, M., et al. (2017). Palmatine inhibits TRIF-dependent NF-κB pathway against inflammation induced by LPS in goat endometrial epithelial cells. Int. Immunopharmacol. 45, 194–200. doi:10.1016/j.intimp.2017.02.004
Yan, H. M., Xia, M. F., Wang, Y., Chang, X. X., Yao, X. Z., Rao, S. X., et al. (2015). Efficacy of berberine in patients with non-alcoholic fatty liver disease. PLoS One 10 (8), e0134172. doi:10.1371/journal.pone.0134172
Yan, Y. Q., Fu, Y. J., Wu, S., Qin, H. Q., Zhen, X., Song, B. M., et al. (2018). Anti-influenza activity of berberine improves prognosis by reducing viral replication in mice. Phytother. Res. 32 (12), 2560–2567. doi:10.1002/ptr.6196
Yao, M., Fan, X., Yuan, B., Takagi, N., Liu, S., Han, X., et al. (2019). Berberine inhibits NLRP3 Inflammasome pathway in human triple-negative breast cancer MDA-MB-231 cell. BMC Complement. Altern. Med. 19 (1), 216. doi:10.1186/s12906-019-2615-4
Ye, Q., Wang, B., and Mao, J. (2020). The pathogenesis and treatment of the `Cytokine Storm' in COVID-19. J. Infect. 80 (6), 607–613. doi:10.1016/j.jinf.2020.03.037
Yu, Z. C., Cen, Y. X., Wu, B. H., Wei, C., Xiong, F., Li, D. F., et al. (2019). Berberine prevents stress-induced gut inflammation and visceral hypersensitivity and reduces intestinal motility in rats. World J. Gastroenterol. 25 (29), 3956–3971. doi:10.3748/wjg.v25.i29.3956
Yu, S. M., Cho, H., Kim, G. H., Chung, K. W., Seo, S. Y., and Kim, S. J. (2016). Berberine induces dedifferentiation by actin cytoskeleton reorganization via phosphoinositide 3-kinase/Akt and p38 kinase pathways in rabbit articular chondrocytes. Exp. Biol. Med. (Maywood). 241 (8), 800–807. doi:10.1177/1535370216631028
Yuan, C., Wu, M., Xiao, Q., Zhao, W., Li, H., Zhong, Y., et al. (2021). Blocking Msr1 by berberine alkaloids inhibits caspase-11-dependent coagulation in bacterial sepsis. Signal Transduct. Target. Ther. 6 (1), 92. doi:10.1038/s41392-021-00483-w
Zeng, Q., Deng, H., Li, Y., Fan, T., Liu, Y., Tang, S., et al. (2021). Berberine directly targets the NEK7 protein to block the NEK7-NLRP3 interaction and exert anti-inflammatory activity. J. Med. Chem. 64 (1), 768–781. doi:10.1021/acs.jmedchem.0c01743
Zhang, B. Y., Chen, M., Chen, X. C., Cao, K., You, Y., Qian, Y. J., et al. (2021). Berberine reduces circulating inflammatory mediators in patients with severe COVID-19. Br. J. Surg. 108 (1), e9–e11. doi:10.1093/bjs/znaa021
Zhang, H., Shan, Y., Wu, Y., Xu, C., Yu, X., Zhao, J., et al. (2017). Berberine suppresses LPS-induced inflammation through modulating Sirt1/NF-κB signaling pathway in RAW264.7 cells. Int. Immunopharmacol. 52, 93–100. doi:10.1016/j.intimp.2017.08.032
Zhang, Q., Piao, X. L., Piao, X. S., Lu, T., Wang, D., and Kim, S. W. (2011). Preventive effect of Coptis chinensis and berberine on intestinal injury in rats challenged with lipopolysaccharides. Food Chem. Toxicol. 49 (1), 61–69. doi:10.1016/j.fct.2010.09.032
Zhang, Y., Li, X., Zhang, Q., Li, J., Ju, J., Du, N., et al. (2014). Berberine hydrochloride prevents postsurgery intestinal adhesion and inflammation in rats. J. Pharmacol. Exp. Ther. 349 (3), 417–426. doi:10.1124/jpet.114.212795
Zhang, H. Q., Wang, H. D., Lu, D. X., Qi, R. B., Wang, Y. P., Yan, Y. X., et al. (2008). Berberine inhibits cytosolic phospholipase A2 and protects against LPS-induced lung injury and lethality independent of the alpha2-adrenergic receptor in mice. Shock. 29 (5), 617–622. doi:10.1097/SHK.0b013e318157ea14
Zhang, X. H., Peng, L., Zhang, J., Dong, Y. P., Wang, C. J., Liu, C., et al. (2020). Berberine ameliorates subarachnoid hemorrhage injury via induction of sirtuin 1 and inhibiting HMGB1/nf-κb pathway. Front. Pharmacol. 11, 1073. doi:10.3389/fphar.2020.01073
Zhou, Y., Fu, B., Zheng, X., Wang, D., Wei, H., qi, Y., et al. (2020). Aberrant pathogenic GM-CSF + T cells and inflammatory CD14 + CD16 + monocytes in severe pulmonary syndrome patients of a new coronavirus. Cold Spring Harb. Lab. 1, 1. doi:10.1101/2020.02.12.945576
Zhou, Y., Jin, J., Feng, M., and Zhu, D. (2019). Wnt signaling in inflammation in tissue repair and regeneration. Curr. Protein Pept. Sci. 20 (8), 829–843. doi:10.2174/1389203720666190507094441
Zhou, Y., Tao, H., Li, Y., Deng, M., He, B., Xia, S., et al. (2016). Berberine promotes proliferation of sodium nitroprusside-stimulated rat chondrocytes and osteoarthritic rat cartilage via Wnt/β-catenin pathway. Eur. J. Pharmacol. 789, 109–118. doi:10.1016/j.ejphar.2016.07.027
Zhu, J. R., Lu, H. D., Guo, C., Fang, W. R., Zhao, H. D., Zhou, J. S., et al. (2018). Berberine attenuates ischemia-reperfusion injury through inhibiting HMGB1 release and NF-κB nuclear translocation. Acta Pharmacol. Sin. 39 (11), 1706–1715. doi:10.1038/s41401-018-0160-1
Zhu, L., Gu, P., and Shen, H. (2019). Protective effects of berberine hydrochloride on DSS-induced ulcerative colitis in rats. Int. Immunopharmacol. 68, 242–251. doi:10.1016/j.intimp.2018.12.036
Zhu, Z., Cai, T., Fan, L., Lou, K., Hua, X., Huang, Z., et al. (2020). Clinical value of immune-inflammatory parameters to assess the severity of coronavirus disease 2019. Int. J. Infect. Dis. 95, 332–339. doi:10.1016/j.ijid.2020.04.041
Keywords: cytokine storm, inflammatory signaling pathway, Coptis chinensis franch, isoquinoline alkaloid, berberine
Citation: Lan Y, Wang H, Wu J and Meng X (2022) Cytokine storm-calming property of the isoquinoline alkaloids in Coptis chinensis Franch. Front. Pharmacol. 13:973587. doi: 10.3389/fphar.2022.973587
Received: 20 June 2022; Accepted: 16 August 2022;
Published: 06 September 2022.
Edited by:
Chiara Bolego, University of Padua, ItalyReviewed by:
Min Hong, Nanjing University of Chinese Medicine, ChinaMiguel Quirch, Texas Tech University Health Sciences Center, United States
Copyright © 2022 Lan, Wang, Wu and Meng. This is an open-access article distributed under the terms of the Creative Commons Attribution License (CC BY). The use, distribution or reproduction in other forums is permitted, provided the original author(s) and the copyright owner(s) are credited and that the original publication in this journal is cited, in accordance with accepted academic practice. No use, distribution or reproduction is permitted which does not comply with these terms.
*Correspondence: Jiasi Wu, d3VqaWFzaUBjZHV0Y20uZWR1LmNu; Xianli Meng, eGxtOTk5QGNkdXRjbS5lZHUuY24=