- Department of Neuroscience, Physiology, and Pharmacology, University College London, London, United Kingdom
The expanding roles of macrophages in physiological and pathophysiological mechanisms now include normal tissue homeostasis, tissue repair and regeneration, including neuronal tissue; initiation, progression, and resolution of the inflammatory response and a diverse array of anti-microbial activities. Two hallmarks of macrophage activity which appear to be fundamental to their diverse cellular functionalities are cellular plasticity and phenotypic heterogeneity. Macrophage plasticity allows these cells to take on a broad spectrum of differing cellular phenotypes in response to local and possibly previous encountered environmental signals. Cellular plasticity also contributes to tissue- and stimulus-dependent macrophage heterogeneity, which manifests itself as different macrophage phenotypes being found at different tissue locations and/or after different cell stimuli. Together, plasticity and heterogeneity align macrophage phenotypes to their required local cellular functions and prevent inappropriate activation of the cell, which could lead to pathology. To execute the appropriate function, which must be regulated at the qualitative, quantitative, spatial and temporal levels, macrophages constantly monitor intracellular and extracellular parameters to initiate and control the appropriate cell signaling cascades. The sensors and signaling mechanisms which control macrophages are the focus of a considerable amount of research. Ion channels regulate the flow of ions between cellular membranes and are critical to cell signaling mechanisms in a variety of cellular functions. It is therefore surprising that the role of ion channels in the macrophage biology has been relatively overlooked. In this review we provide a summary of ion channel research in macrophages. We begin by giving a narrative-based explanation of the membrane potential and its importance in cell biology. We then report on research implicating different ion channel families in macrophage functions. Finally, we highlight some areas of ion channel research in macrophages which need to be addressed, future possible developments in this field and therapeutic potential.
Introduction
Belonging to the innate branch of the immune system, macrophages are important in the killing of invading microbes, the removal of noxious material; the initiation, propagation and resolution of inflammatory responses, the progression of tissue repair and the maintenance of homeostasis (Murray and Wynn, 2011; Wynn et al., 2013). However, an inappropriate, exaggerated or dysfunctional macrophage response can lead to host tissue damage and contribute to a variety of pathologies (Belchamber and Donnelly, 2017; Crayne et al., 2019). To carry out their required biological functions macrophages need to produce qualitatively, quantitatively and temporally the appropriate biomolecules. They achieve this by utilizing a complex series of interconnected, feedback rich, signalling cascades (Raza et al., 2008; Drexler et al., 2008). These signalling pathways receive input from numerous cellular sensors, which allow the macrophage to respond in a timely and appropriate manner to extracellular and intracellular signals, which are often in a state of constant flux, to produce the appropriate response. Indeed, Mosser et al. has recently referred to macrophages as “transducers of the body” (Mosser et al., 2020). This diversity of functions and flexibility of response to environmental cues results in macrophages showing phenotypic heterogeneity. How macrophage populations are classified is highly debatable and depends on various criteria including tissue location/lineage, current cellular functionality or activation state and the granularity of the classification profiles used. However, a convenient, if simplified, way to classify them is pro-inflammatory M1 macrophages and anti-inflammatory M2 macrophages (Smith et al., 2016; Gordon and Plüddemann, 2017; Gessain et al., 2020).
Ion channels control the movement of ions, such as Na+, K+, and Ca2+, across cell membranes, both plasma and intracellular membranes such as mitochondrial, lysosomal, endoplasmic reticulum membranes and nuclear membranes, and are known to be important cellular sensors and participate in cell signalling cascades in numerous cell types (Selezneva et al., 2022).
Here we will provide an up-to-date overview of ion channels known to be present and functional in macrophages. First, we give a brief introduction to membrane potential and how it could impact macrophage biology. Then, beginning with potassium (K+) channels, which are the main regulators of cell membrane potential, we review the evidence which supports a role for ion channels, Table 1, in shaping macrophage behaviour. Finally, we discuss prospects in this area of research and the potential of macrophage ion channels as therapeutic targets.
Membrane potential and macrophages
While the concentrations of ions on each side of a membrane are predominantly determined by transporters, passive movement of ions across the membrane is controlled by ion channels. The principal difference between channels and transporters is that “open” ion channels allow passive diffusion of ions down their electrochemical gradient, whereas transporters depend on the use of energy to move ions, usually, against their gradient (Purves et al., 2001). The membrane potential is a measurement of the difference in electrical potential across a membrane. The gold standard for measuring membrane potential involves the use of electrophysiological methods, however, due to the highly dynamic nature of macrophage plasma membranes, particularly activated macrophages, electrophysiological measurements using patch-clamp electrodes can be a challenge. Nevertheless, several studies, using a variety of electrophysiological methodologies and configurations, have reported the resting plasma membrane potential for non-activated monocytes and macrophages. Gallin and Gallin reported the resting plasma membrane potential of human macrophages as −14.5 ± 5 mV (Gallin and Gallin, 1977), while another study reported the membrane potential of human macrophages as −42 ± 14 mV (Mukherjee et al., 1983). In both these studies cells were isolated from human peripheral blood, although it appears that in the latter study macrophages spent less time in culture. Recordings of the resting plasma membrane potential of macrophages isolated from different species, tissues and cell lines, and using various isolating protocols have also been made (Castranova et al., 1979; dos Reis et al., 1979; Gallin and Livengood, 1980; Gallin, 1981; Ince et al., 1983). The picture that emerges is a high degree of variation between different macrophage populations, and it has been speculated that this may reflect the functional characteristics of the macrophage populations investigated (Mukherjee et al., 1983; Zhang et al., 2010). In line with this idea, recent work using a differentiated macrophage cell line, THP-1 cells, has reported that M2 macrophages have a lower resting plasma membrane potential than M1 macrophages (Li et al., 2016). The significance of the membrane potential is that it impacts certain biological processes, e.g. the driving force for Ca2+ entry into a cell when channels selective for this ion are open and membrane lipid dynamics (Zhou X. et al., 2015). Therefore, membrane potential can influence the activation thresholds, efficiency and magnitude of cellular responses (Kadir et al., 2018), and could have significant effects on macrophage activity/function regulating cell signalling molecules and cascades located within or in close proximity to the membrane.
In addition to plasma membrane, intracellular membrane potentials can also regulate cellular and organelle functions, an example being the role of the proton channel Hv1 in the regulation of NADPH oxidase-2 (NOX2) activity in granulocytes (DeCoursey, 2016). In macrophages, mitochondrial membrane potential is known to regulate cellular functions (Wang et al., 2021), and it is expected that the membrane potential of other organelles (Figure 1) will have regulatory roles in macrophages too (Selezneva et al., 2021). However, the majority of research on ion channels in macrophages is focused on the plasma membrane.
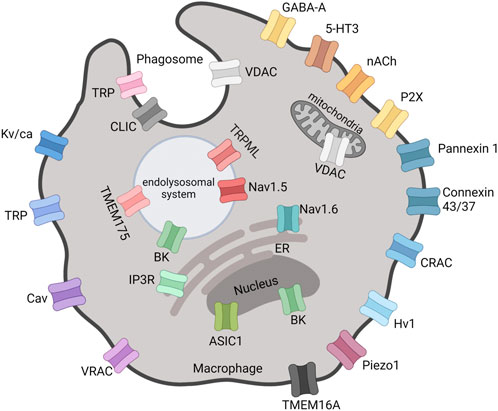
FIGURE 1. Locations of functional ion channels reported in macrophages. Mitochondria and nucleus are double-membrane-bounded organelles with ion channels located in the inner and/or outer membranes. The outer nuclear membrane is continuous with the ER. VDAC, Voltage-Dependent Anion Channel; GABA-A, Gamma-Aminobutyric Acid type A receptor; 5-HT3, 5-Hydroxytryptamine3 receptor; nACh, Nicotinic Acetylcholine receptor; CRAC, Ca2+ Release-Activated Channel; Hv, Proton voltage-gated channel; VRAC, Volume-Regulated Anion Channel; Cav, Ca2+ voltage-gated channel; TRP, Transient Receptor Potential; Kv/Ca, K+ voltage-gated and/or Ca2+-activated channel; BK, Big K+ channel; Nav, Na+ voltage-gated channel; TMEM, Transmembrane Protein; IP3R, Inositol 1,4,5-trisphosphate receptor; ER, Endoplasmic Reticulum; ASIC, Acid-Sensing Ion Channel; CLIC, Cl− Intracellular Channel. Created with BioRender.com.
It is evident that ion channels regulate ion flux and membrane potential and therefore signalling events at or local to membranes. These signalling outcomes will be dependent on the type of ion channel(s) being activated. Therefore, starting with potassium channels, in the next section we will review the research linking members of different ion channel families with signalling and activation states of macrophages.
Potassium (K+) ion channels
K+ channels are the most widely distributed of all the ion channels and are classified into families and subfamilies based on their structure and properties (Alexander et al., 2011). The main function of membrane K+ channels is to control the membrane potential. In general, it appears that monocyte-macrophage differentiation and macrophage activation increase the diversity of K+ channels expressed by the cell (Vicente et al., 2003; Yoshida, 2017).
Ca2+ is a key second messenger in cell signalling with concentration, spatial and temporal parameters all contributing to information conveyed by this divalent cation. K+ channels are important in responding to and regulating Ca2+ fluxes in cells. For example, extracellular ATP activation of P2Y receptors results in intracellular Ca2+ oscillations and promotes IL-6 transcription in macrophages (Hanley et al., 2004). Ca2+ -activated K+ channels contribute to the intermediate signalling pathways associated with this event by mediating membrane potential oscillations (Hanley et al., 2004). A tight coupling between the Ca2+-activated K+ channel, KCa3.1, and store-operated Ca2+ entry (SOCE) is reported to exist in macrophages. Activated by Ca2+ influx through Ca2+ release-activated Ca2+ (CRAC) channels, KCa3.1 amplifies CRAC current, prolongs Ca2+ signalling and Ca2+ store refilling (Gao et al., 2010). Macrophages infiltrating into inflamed tissues (Strøbæk et al., 2013), atherosclerotic lesions (Toyama et al., 2008) and transplanted organs have all been shown to express elevated levels of KCa3.1. Toyama and others demonstrated KCa3.1 inhibitors, TRAM-34 and clotrimazole, to significantly reduce macrophage infiltration into atherosclerotic plaques and consequently reduce atherosclerosis development in blood vessels (Toyama et al., 2008). Similarly, Xu and others showed that blocking KCa3.1 shifts macrophage polarization towards M2 macrophage phenotype and stabilises atherosclerotic plaques reducing rupture (Xu et al., 2017). Besides atherosclerosis, Chung and others showed that voltage- and Ca2+- activated K+ ion channels are capable of influencing macrophage migration by altering the cell volume and shape with implications for neurodegenerative disorders such as HIV1-associated dementia (Chung et al., 2002). Voltage- and Ca2+- activated K+ channel blockers, charybodotoxin, margatoxin, agatoxin and apamin, were reported to inhibit macrophage migration in the brain, therefore, possibly affecting the levels of neuronal damage (Chung et al., 2002). Taken together, KCa3.1 channels seem to have potential to modulate the intracellular signalling responses of macrophages and contribute to M1/M2 macrophage polarization.
Following several years of controversies about the expression of voltage-gated potassium channels in macrophages (Mackenzie et al., 2003; Park et al., 2006; Felipe et al., 2010), the work of Felipe and others established the presence of both Kv1.3 and Kv1.5 in macrophages and microglia, a type of resident macrophage found in the central nervous system. Kv1.3 and Kv1.5 form heterotetrameric functional channels (Vicente et al., 2006; Villalonga et al., 2007; Villalonga et al., 2010), and depending on the composition of Kv1.3/Kv1.5 in this tetramer, the ion channels take on biophysical and pharmacological characteristics more closely resembling either Kv1.3 or Kv1.5 (Vicente et al., 2006; Vicente et al., 2008; Villalonga et al., 2007; Villalonga et al., 2010; Felipe et al., 2010). This highlights the importance of ion channel subunit composition to channel functionality. Kv1.3 and Kv1.5 have distinctive channel kinetics, i.e. conductance, activation and inactivation rates, pharmacology and functions in immune cells (Felipe et al., 2010) (Table 2). In macrophages Kv1.3 is involved in setting the resting membrane potential (Mackenzie et al., 2003; Felipe et al., 2010). Vicente and others reported the reduced activity of voltage-gated K+ channels in macrophages inhibits inducible nitric oxide synthase (iNOS) expression (Vicente et al., 2003). In microglia Kv1.3 blockers reduce production of proinflammatory factors IL-6, TNFα and the enzyme cyclooxygenase-2, protecting from microglia-mediated brain injury (Peng et al., 2014; Fordyce et al., 2005). One possible hypothesis is that Kv1.3 is essential for the immune cell physiology, while Kv1.5 contributes to the fine tuning of immune cell responses.
Inwardly-rectifying potassium channels allow K+ to more easily enter the cell than exit and play a role in setting the membrane potential and are denoted by Kir. The inwardly-rectifying Kir2.1 was reported to be the target of memantine’s anti-inflammatory effects (Tsai et al., 2013), influence macrophage functional activity and was down-regulated by LPS (Vicente et al., 2003). ATP-sensitive potassium channels (KATP) are also expressed in macrophages are composed of four pore-forming inwardly rectifying Kir6.1/Kir6.2 subunits and four regulatory sulfonylurea receptor subunits. KATP channels in macrophages have been seen to modulate cell membrane potential properties, with blockers of the channels promoting a M2 phenotype (Ling et al., 2013; Li et al., 2016), as well as regulate microglial activity (Ortega et al., 2012). A two-pore domain K+ channel (TWIK2), which can be activated by a number of chemical and physical parameters including pH, oxygen tensions and stretch, was identified as an essential upstream mechanism for the ATP-induced NLRP3 inflammasome activation (Wu et al., 2021; Di et al., 2018).
Research on Ca2+-activated K+ channel in macrophages has also shed light on the role of ion channels in non-plasma membrane locations. The large conductance Ca2+- and voltage-activated K+ channel, KCa1.1, also known as BK channel, has been reported to be present in both plasma and nuclear membranes in macrophages (Yoshida, 2017; Selezneva et al., 2021). Plasma membrane BK channels were found to regulate a disintegrin and metalloprotease domain 17 (ADAM17) enzyme and, hence, the release of TNFα and IL-6 receptor-α from activated macrophages (Yoshida, 2017), while previous studies had reported BK to be central in the NF-κB dependent inflammatory response of macrophages to bacterial LPS (Papavlassopoulos et al., 2006). Interestingly, BK channels appear to be predominantly intracellular in resting macrophages and appear upregulated on the plasma membrane upon LPS stimulation (Yoshida, 2017). BK channels located on the nuclear envelope (NE) were shown to regulate cAMP response element binding protein (CREB) phosphorylation in macrophages (Selezneva et al., 2021). Moreover, this study reported that nuclear Ca2+ and calmodulin dependent kinases II and IV were involved in regulation of CREB by nuclear BK channels in macrophages (Selezneva et al., 2021). Similar findings were previously reported in hippocampal neurones (Li et al., 2014). BK channels have been also found on the NE of microglia and were proposed to be involved in microglial activation, facilitating NO and cytokine production, potentially by regulating Ca2+ and K+ fluxes in the nucleus (Yang et al., 2019). A recent study reported lysosomal BK channels in macrophages to be functionally coupled with transient receptor potential (TRP)ML, discussed below, and regulate large particle phagocytosis through modulating lysosomal exocytosis (Sun et al., 2020). This study highlights an important phenomenon of ion channel coordination to facilitate cell signalling events. Finally, deficiency of transmembrane protein 175 (TMEM175), a K+ channel associated with the endolysosomal system has also been shown to result in accelerated autophagosome-lysosome fusion in macrophages (Cang et al., 2015).
Sodium (Na+) ion channels
The recognised role of Na+ channels in cell physiology is the propagation of an action potential and depolarization of the plasma membrane. It would, therefore, appear that these channels would not play a role in macrophage biology, see below Electrical Excitability section. However, seven of the nine voltage-gated Na+ channel (Nav) family members (Nav1.1, Nav1.3–1.7, and Nav1.9) (Carrithers et al., 2007; Sun et al., 2019) have been detected in macrophages, with many of them being associated with intracellular membranes. Nav1.5 is expressed in late endosomes and phagolysosomes of macrophages and is reported to regulate phagocytosis and endosomal acidification through triggering Na+ efflux and intra-endosomal pH reduction (Carrithers et al., 2007). A later study showed Nav1.5 to regulate mycobacteria phagocytosis and phagosome maturation through organelle polarization and localized Ca2+ oscillations (Carrithers et al., 2011). Another Na+ channel, Nav1.6, has also been reported to regulate phagocytosis in macrophages and microglia (Craner et al., 2005), and it localizes to cytoskeletal filaments and endoplasmic reticulum (Carrithers et al., 2007). Several studies have also suggested a role for Nav-mediated regulation of macrophage phagocytosis in inflammatory disease progression including myelin degradation in multiple sclerosis (Black et al., 2013), experimental autoimmune encephalomyelitis (Craner et al., 2005) and atherosclerotic lesion development (Sun et al., 2019). Neosaxitoxin, a local anaesthetic and at nanomolar concentrations a selective inhibitor of six Nav isoforms (Nav1.1–1.4, 1.6, and 1.7) (Walker et al., 2012), has also been shown to inhibit LPS-induced release of NO produced by iNOS, TNFα and IL-1β from macrophages, thus indicating a role for Nav in inflammatory mediator release from macrophages (Montero et al., 2020).
Decrease in pH has long been associated with acute and inflammatory diseases (Kellum et al., 2004). The Na+-permeable channels, acid-sensing ion channels (ASICs), ASIC1, ASIC2a, and ASIC3, have also been identified in macrophages, with ASIC1 immunolocalising to the nucleus, and ASIC2a and ASIC3 being mostly found throughout cell cytoplasm (Ni et al., 2018). Of significance, extracellular acidosis and ASIC activation were found to promote migration, endocytosis, cytokine production and apoptosis in macrophages (Ni et al., 2018; Kong et al., 2013; Foster et al., 2021). It is rather fitting that some classical physiological changes associated with inflammation, such as heat and low pH, may be important sensory cues for macrophages, see the following sections.
Calcium (Ca2+) ion channels
As would be predicted, intracellular Ca2+ regulates a variety of functions in macrophages, ranging from cytokine production to phagocytosis (Vaeth et al., 2015). CRAC channels are highly selective for Ca2+ compared to other cations and are activated by depletion of ER Ca2+ stores (Shim et al., 2015). Briefly, depletion of ER Ca2+ stores leads to conformational changes in stromal interaction molecules (STIM) 1 and 2 located on the ER. These conformational changes allow STIM 1 and 2 to oligomerize and form clusters “puncta”, into which CRAC is recruited, resulting in SOCE. ORAI1 is the gene encoding the CRAC channel protein, but the two terms are often used interchangeably. Chauhan et al. reported ORAI1/CRAC to mediate the basal Ca2+ influx in macrophages (Chauhan et al., 2018). A tight coupling between CRAC channels, SOCE, and KCa3.1 channels is thought to exist in macrophages, where Ca2+-activated KCa3.1 channels amplify the CRAC current, prolonging Ca2+ signalling and store repletion (Gao et al., 2010). Loss-of-function mutations in ORAI1 and STIM1 genes abolish CRAC channel function and SOCE in immune cells, resulting in CRAC channelopathies (Shaw and Feske, 2012). However, controversies regarding CRAC/SOCE and macrophage functions exist. STIM1 was reported to be essential for the FcγR-mediated Ca2+ entry, phagocytosis, inflammatory cytokine production and autoimmune inflammation (Braun et al., 2009). Another study identified CRAC channels as a principal link between chemokine receptor stimulation and the activation of focal adhesion-related tyrosine kinase Pyk2 and MAPK pathways leading to inflammatory mediator secretion in macrophages (del Corno et al., 2001). Loss of Ca2+ entry via CRAC/TRPC1 was found to induce ER stress and apoptosis in macrophages (Conceicao et al., 2019). However, other studies have questioned the importance of Ca2+ (Hishikawa et al., 1991; Greenberg et al., 1991; McNeil et al., 1986) and SOCE in macrophage function (Vaeth et al., 2015). Recently it has been shown that M1 and M2 macrophages exhibit different Ca2+ entry mechanisms, with M2 macrophages being associated with ORAI1, and TRPC1 channels being associated with M1 macrophages (Nascimento Da Conceicao et al., 2021). Loss of either TRPC1 or ORAI1 limited the functionality of M1 and M2 macrophages respectively. Three other TRP family channels, TRPA1, TRPM7, and TRPP2, have also been reported to mediate the non-ORAI1 component of SOCE in macrophages (Liu et al., 2021). This again highlights the important interplay between ion channels and macrophage functions.
In addition to already discussed CRAC channels, L-type voltage-gated Ca2+ channels have been identified in macrophages and suggested to modulate macrophage responses to infection, with roles in reactive oxygen species generation, survival and autophagy (Antony et al., 2015; Sharma et al., 2016). Inositol 1,4,5-trisphosphate receptors, IP3Rs, are ubiquitous Ca2+ channels mediating Ca2+ release primarily from endoplasmic reticulum stores, have also been implicated in macrophage Ca2+ signalling, polarization (Liu et al., 2019) and cytokine production (Staats et al., 2016). However, how many of these functions are dependent on SOCE activation will require further research (Sampieri et al., 2018). Finally, Ca2+-permeable two-pore channels, TPCs, found on membranes of the endolysosomal system, together with TRPML channels (see below), have been found to regulate endolysosomal osmo-/mechano-sensation and volume regulation (Chen et al., 2021) and drive macrophage phagocytosis (Davis et al., 2020). TRP channels also account for a significant part of research concerning Ca2+-permeable ion channels in macrophages, although they can show varying degrees of selectivity for different cations (Table 3).
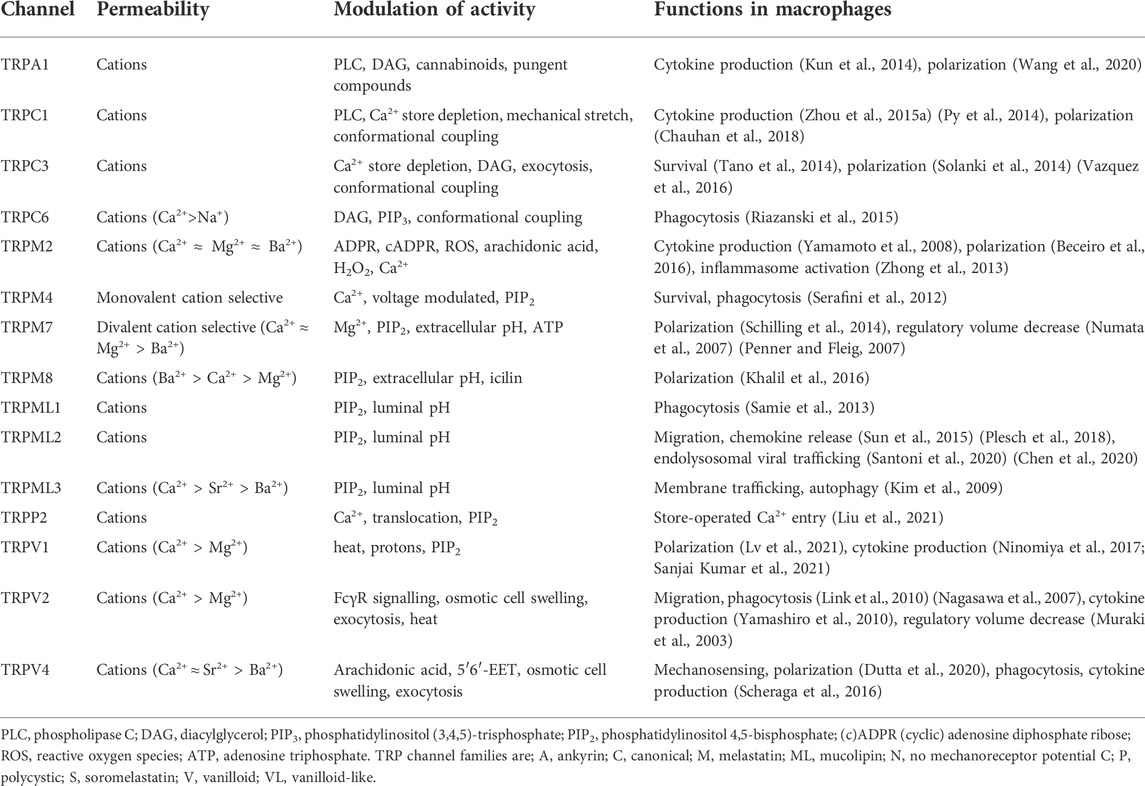
TABLE 3. A summary of Transient Receptor Potential (TRP) ion channels identified in macrophages to date.
Transient receptor potential (TRP) ion channels
TRP channels form a large superfamily of cation channels which are important cell sensors. Split into nine families; A, C, M, ML, N, P, S, V, and VL; these can be further divided into subfamilies and display diversity in their permeability to different cations and responses to different chemical and physical stimuli, see Table 3, (Venkatachalam and Montell, 2007; Bouron et al., 2015; Feng et al., 2014). When considering the variety of cellular functions macrophages have, and the dynamic tissue environments in which they perform these functions, it is not surprising that a number of TRP subfamilies have been demonstrated to play regulatory roles in macrophages (Table 3) (Link et al., 2010; Zhong et al., 2013; Schilling et al., 2014; Solanki et al., 2014; Zhou X. et al., 2015; Sun et al., 2015; Khalil et al., 2016; Chauhan et al., 2018; Wang et al., 2020).
Taking the TRP family in alphabetical order, TRPA1 channel was reported to regulate the expression of pro-inflammatory neuropeptides, cytokines and chemokines, exert a mucosal protection in colitis (Kun et al., 2014) and reduce atherosclerosis progression by suppressing macrophage M1 polarization (Wang et al., 2020). Similarly, a recent study showed that ablation of TRPA1 exacerbates M1 macrophage infiltration and renal inflammation after renal ischemia-reperfusion injury in mice (Ma and Wang, 2021).
TRPC1 was found to be crucial for the proinflammatory responses to bacterial infections through stimulating protein kinase C α pathway, NF-κB/Jun kinase nuclear translocation and cytokine release (Zhou Y. et al., 2015) (Py et al., 2014). TRPC3 also contributes to macrophage M1 polarization (Solanki et al., 2014; Vazquez et al., 2016; Chauhan et al., 2018) and appears to be an obligatory component of survival mechanisms in human and murine macrophages (Tano et al., 2014). TRPC6 was suggested to have roles in phagocytosis and restore microbicidal function in compromised alveolar macrophages from cystic fibrosis patients (Riazanski et al., 2015).
In the melastatin family of TRP channels plasma membrane TRPM2 was shown to control chemokine production in monocytes, such as macrophage inflammatory protein-2 production (CXCL2) (Yamamoto et al., 2008; Zong et al., 2022). Zhong et al. identified TRPM2 as a key factor linking oxidative stress and NLRP3 inflammasome activation (Zhong et al., 2013). In this study TRPM2 deficient macrophages displayed impaired NLRP3 inflammasome activation and subsequently IL-1β secretion (Zhong et al., 2013). A recent study identified a TRPM2–CD36 axis in macrophages as a critical molecular mechanism underlying atherogenesis (Zong et al., 2022). However, there is some controversy whether TRPM2 promotes inflammation or protects against it. While reactive oxygen species activate TRPM2, one study (Di et al., 2012) reported an ability of TRPM2 to inhibit reactive oxygen species production in macrophages and prevent endotoxin-induced lung inflammation. Similarly, another study reported that the absence of TRPM2 results in greater reactive oxygen species and inflammatory mediator production in macrophages, promotes macrophage M1 polarization and increases gastric inflammation (Beceiro et al., 2016). Moving to other members of this TRP family, TRPM4 was reported to regulate the AKT signalling pathway, phagocytic function and macrophage survival in sepsis (Serafini et al., 2012). Both TRPM7 and TRPM8 have been suggested to have roles in macrophage polarization. TRPM7 blockers were reported to prevent macrophage M2 polarization and inhibitory effects of IL-4 and M-CSF on TNFα production (Schilling et al., 2014). TRPM8 in macrophages determines M1 and M2 actions by regulating TNFα and IL-10 production (Khalil et al., 2016).
TRPML channels belong to the mucolipin TRP subfamily and primarily localize to membrane-bounded vesicles along the endocytosis and exocytosis pathways. In the endolysosomal system TRPML2 was established to be primarily expressed in recycling endosomes, whereas TRPML3 and TRPML1 in early endosomes and late endosomes/lysosomes, respectively (Sun et al., 2015; Plesch et al., 2018). A study by Samie et al. reported that TRPML1 knockout and blockade in macrophages causes inhibition of large particle phagocytosis, whereas TRPML1 overexpression and agonists facilitate the process (Samie et al., 2013). The authors suggest a mechanism, where the binding of phagocytosis target by macrophage leads to PIP2-dependent TRPML1 activation and resulting Ca2+ release induces lysosomal exocytosis (Samie et al., 2013). Furthermore, TRPML1 together with BK channels was suggested to be involved in the fusion of phagosomes with lysosomes (Dayam et al., 2015; Cao et al., 2015; Sun et al., 2020) and enhance the degradative lysosome function through transcription factor TFEB activation (Gray et al., 2016). Interestingly, Sun and others reported that TRPML2 expression in macrophages significantly increases upon toll-like receptor activation, while the levels of other TRPML subfamily members do not seem to change with macrophage activation (Sun et al., 2015). Sun and others also showed that TRPML2 knockout leads to reduced production of chemokines such as CCL2 and impaired recruitment of peripheral macrophages (Sun et al., 2015). In support of this, a later study reported that TRPML2 agonist stimulates macrophage migration and the release of CCL2 from macrophages (Plesch et al., 2018). Recently, a role for TRPML2 in endolysosomal viral trafficking and viral infection has been suggested (Santoni et al., 2020; Chen et al., 2020). TRPML3 is thought to have a role in the regulation of endocytosis, membrane trafficking and autophagy (Kim et al., 2009). A recent study reported TRPML3 in alveolar macrophages to regulate elastase MMP-12 uptake, influencing chronic obstructive pulmonary disease development (Spix et al., 2022).
Extensive research has been carried out on TRPV1 and its role in pain, and while a number of drugs have entered clinical trials, these drugs are associated with significant side effects which has limited their utility, see Table 4 (Moran, 2018). In macrophages TRPV1 inhibits M1 polarization via Ca2+/CaMKII/Nrf2 signalling pathway (Lv et al., 2021) and influences cytokine production in models of osteoarthritis, sepsis (Ninomiya et al., 2017), inflammatory tissue fibrosis (Okada et al., 2011) and viral infection (Sanjai Kumar et al., 2021). Interestingly, a recent study suggested that inflammation-mediated macrophage polarisation induces TRPV1/TRPA1 heteromeric channel formation with key roles in endometriosis pain (Zhu et al., 2022). A second member of TRPV subfamily, TRPV2 channel, plays regulatory roles in a number of macrophage functions including chemotaxis and early phagocytosis (Link et al., 2010; Nagasawa et al., 2007), podosome formation (Nagasawa and Kojima, 2012) and cytokine production (Yamashiro et al., 2010). Moreover, TRPV2 and TRPM7 in macrophages are thought to control regulatory volume decrease (RVD), which leads to NLRP3 inflammasome activation (Santoni et al., 2015; Compan et al., 2012; Liedtke, 2006). During RVD TRPV2 is translocated to the plasma membrane where it induces cellular permeabilization, while TRPM7 acts as a controller of the regulatory volume decrease process (Muraki et al., 2003). TRPM7 blockade was reported to delay the RVD, reduce cell permeabilization and IL-1β release in macrophages (Numata et al., 2007; Penner and Fleig, 2007). An interesting member of TRPV family is TRPV4. It responds to mechanical stimuli and has been shown to regulate macrophage phagocytosis and cytokine secretion (Scheraga et al., 2016). A more recent study by Scheraga R. et al (2020) established that TRPV4 enhances macrophage bacterial clearance and reduces proinflammatory secretion of cytokines through MAPK molecular pathway switching (Scheraga R. et al., 2020). TRPV4 roles in macrophages appear to be important in inflammation and disease progression where mechanical stimuli may be of significance e.g. ventilator/chemically-induced injury (Hamanaka et al., 2010; Balakrishna et al., 2014; Scheraga R. G. et al., 2020) and tuberculosis (Naik et al., 2020). A role for TRPV4 in matrix stiffness-induced macrophage polarization has also been suggested (Dutta et al., 2020). Finally, there are reports that TRPV4 can contribute to the formation of specialised macrophage phenotypes (Goswami et al., 2017, 2021).
Together these studies highlight the importance and complexity of these “sensory” channels in macrophages, and we refer the reader to some in-depth reviews of this channel superfamily (Santoni et al., 2018; Nguyen et al., 2022). However, it is important to discriminate in ion channel knock-down studies if the reported effects are directly as a result of ion channel modulation in the macrophage or via an up-stream mechanism, e.g. ion channel modulation in T lymphocytes can subsequently impact macrophage activity (Okada et al., 2011; Ma and Wang, 2021). With time, more roles for TRP channels in macrophage regulation are being established. Functional modulation of TRP channels in macrophages may represent a rich area for the development of therapies to treat a range of inflammatory diseases.
Ligand-gated ion channels (LGICs)
By comparison to voltage-gated ion channels, which open to changes in membrane potential, a LGIC opens in response to the binding of certain chemical messengers. Therefore, the opening of a LGIC will not only be determined by its expression but also the concentration of the relevant ligand which has access to the ligand-binding site on LGIC. This is an important caveat when investigating LGICs in macrophages since many of the endogenous ligands for LGICs are not found in tissues at sufficient concentrations to activate the channel and are not traditionally synthesised by cells found in inflammatory lesions. Nevertheless, this does not negate the possibility that synthetic openers, blockers and allosteric modulators of macrophage LGICs may have therapeutic value.
P2X receptors are a family of ATP-gated cation channels comprising seven subtypes, P2X1-7. Although several P2X receptor mRNA transcripts, P2X1, P2X3-5 and P2X7, were detected in macrophages, to date only P2X4 and P2X7 have been reported to have defined functional roles in macrophages, with their activation being associated with the onset of inflammatory reactions (Kessler et al., 2011; Bowler et al., 2003). The stimulation of P2X4, which has approximately 100-fold higher affinity for ATP than P2X7 (Jarvis and Khakh, 2009; Asatryan et al., 2018), in macrophages was found to trigger Ca2+ influx and p38 MAPK phosphorylation, causing cytosolic phospholipase A2 activation and cyclooxygenase-dependent release of the lipid mediator prostaglandin E2 (Ulmann et al., 2010). Another study reported a role for P2X4 in chemokine secretion by macrophages (Layhadi et al., 2018). In microglia P2X4 was reported to trigger the release of brain-derived neurotrophic factor and initiate a core pain signalling pathway (Beggs et al., 2012; Masuda et al., 2014). Many studies agree that P2X7 plays a prominent stimuli-dependent role in controlling inflammasome activation and macrophage function. P2X7 activation was reported to promote the efflux of K+, activate the NLRP3 inflammasome and cause release of IL-1β from macrophages (Ferrari et al., 1997; Ferrari et al., 2006; Piccini et al., 2008; Barberà-Cremades et al., 2012; Mariathasan et al., 2006; Muñoz-Planillo et al., 2013; Pétrilli et al., 2007). Moreover, Franceschini et al. showed that P2X7 and NLRP3 inflammasome closely interact and colocalize at discrete subplasmalemmal sites in microglia and macrophages (Franceschini et al., 2015). Absence of the P2X7 was demonstrated to result in an inability of macrophages to release IL-1β from macrophages in response to ATP and, therefore, impaired cytokine signalling cascades (Solle et al., 2001). Hemichannel Pannexin 1 was found to be required for the IL-1β release by the ATP-gated P2X7 (discussed below) (Pelegrin and Surprenant, 2006). There have also been reports of P2X7 receptor involvement in ATP-induced ATP release (Suadicani et al., 2006), microvesicle shedding (MacKenzie et al., 2001) and phagocytosis (Gu et al., 2010).
Nicotinic acetylcholine (nACh) α7 receptors are another ligand-gated cation-selective channel which contributes to shaping macrophage behaviour. Interestingly, these channels expressed in macrophages participate in a so-called anti-inflammatory vagal reflex or cholinergic anti-inflammatory pathway which integrates the nervous and immune system. In this reflex acetylcholine (ACh) has an anti-inflammatory effect on macrophages (Wang et al., 2002). Studies show that nAChα7 receptor activation in macrophages is capable of modulating inflammation by inhibiting NF-κB nuclear translocation (Yoshikawa et al., 2006), activating the JAK2/STAT3 pathway (de Jonge et al., 2005; Joe et al., 2011) and selectively inhibiting pro-inflammatory cytokine production while leaving anti-inflammatory cytokines undisturbed (Báez-Pagán et al., 2015; Borovikova et al., 2000; Wang et al., 2002). Tsoyi et al. have suggested that nAChα7 receptor activation in macrophages can mediate anti-inflammatory effects by activating the PI3K/Akt/Nrf-2 pathway and upregulating heme oxygenase-1 (Tsoyi et al., 2011). Due to the high levels of cholinesterase activity in tissues a valid question to ask is the source of ACh which activates macrophage nACh receptors. Importantly, along with the splenic nerves research now indicates that immune cells act as a source of ACh in the spleen and this contributes to the anti-inflammatory vagal reflex (Fujii et al., 2017), although it must be acknowledged that human nAChα7 receptors require high concentration of ACh (EC50 = 128 ± 12 μM) to be activated (Gill et al., 2011). Other nACh receptor subunits have been reported in macrophages including α4β2 (Kiguchi et al., 2012; Kiguchi et al., 2018), α2, α5 and α10 (Kawashima et al., 2007), however, their functions in macrophages require further investigation.
In addition to the above mentioned nACh and P2X receptors, 5-Hydroxytryptamine 3 (5-HT3) receptor is another LGIC found in macrophages where it is thought to modulate cytokine secretion (Seidel et al., 2008; Maehara et al., 2015). The role of 5-HT (serotonin) in inflammation is historically associated with the vasculature (Majno and Palade, 1961), with the majority of peripheral 5-HT being stored in platelets and released upon platelet activation. However, other possible sources of peripheral serotonin could include lymphocytes, macrophages and mast cells (Herr et al., 2017). Maehara and others suggest a therapeutic potential for 5-HT3 receptor antagonists targeting peritoneal macrophages expressing the receptors in post-operative ileus (Maehara et al., 2015).
The presence of functional Cl−-permeable gamma-aminobutyric acid (GABA) type A receptors in macrophages has also recently been reported (Kim et al., 2018). GABA is one of the principal neurotransmitters in the brain and is long associated with GABAergic cells, however, GABA can be synthesised by a number of cells including B lymphocytes (Zhang et al., 2021). Interestingly, GABA has been attributed with both inhibitory effects on phagocyte activation and pro-inflammatory functions (Bhandage and Barragan, 2021). While GABA appears to promote monocyte differentiation into anti-inflammatory macrophages that secrete IL-10 (Zhang et al., 2021), the treatment of macrophages with GABA or GABAergic drugs was shown to promote autophagy activation, enhance phagosomal maturation and antimicrobial responses against mycobacterial infection (Kim et al., 2018). Propofol, a commonly used anaesthetic agent, was reported to bind to GABAA receptors in macrophages and inhibit macrophage phagocytosis, with potential implications for post-surgical wound healing (Shiratsuchi et al., 2009). Furthermore, a recent study highlights a role for GABAA receptors in macrophages in pressure overload-induced heart failure (Bu et al., 2021).
Further macrophage ion channels
In addition to the members of the large ion channel families a number of other ion channels have been reported to influence macrophage behaviour. Proton voltage-gated channel, Hv1, is reported to regulate the respiratory burst during phagocytosis, limit membrane depolarization and intracellular acidosis in macrophages (DeCoursey, 2010) (Ramsey et al., 2009). In microglia, Hv1 was reported to enhance reactive oxygen species generation contributing to brain damage at early time points after ischemic stroke (Wu et al., 2012).
Voltage-Dependent Anion Channels (VDAC), initially described as mitochondria-associated porins (Schein et al., 1976), have been identified on plasma (Bàthori et al., 1999) and phagosomal membranes as well. In addition to the pivotal roles of mitochondria in cellular metabolism (Ramond et al., 2019), in macrophages VDACs appear to play a more negative role during infection, being crucial for bacterial growth and survival within phagosomes. The channels were reported to colocalize and interact with bacterial proteins and aid bacterial lipid translocation (Danelishvili et al., 2017).
Several studies report the presence of functional volume-regulated anion channels, VRACs, in macrophages. Burrow and others suggested VRAC to be a functional link between sphingolipid and purinergic signalling systems in macrophages, with roles in phagocytosis, migration and inflammation (Burow et al., 2014). A recent study by Green et al. reports LRRC8A, a pore-forming subunit of VRAC, to be essential for hypotonicity-induced NLRP3 inflammasome activation in macrophages (Green et al., 2020).
Cl− intracellular channels (CLIC), in particular CLIC1, is a family of ion channels permeable to Cl− ions in macrophages, which was reported to regulate macrophage function through modulation of phagosomal acidification and reactive oxygen species production (Jiang et al., 2012). Additionally, iNOS-induced nuclear translocation of another family member, CLIC4, was reported to be essential for macrophage transition from a pro- to anti-inflammatory state (Malik et al., 2012). Furthermore, both CLIC1 and CLIC4 have been suggested to be involved in the NLRP3 inflammasome regulation (Domingo-Fernández et al., 2017). One more Cl− channel found in macrophages is transmembrane protein 16A, TMEM16A (Shi et al., 2020), which has been reported to mediate microRNA9-induced TGF-β/Smad2 signalling implicated in LPS-induced sepsis (Zhen et al., 2019; Sui et al., 2020; Bai et al., 2021).
A number of studies have pointed to the presence of hemichannels and gap junctions in macrophages (Levy et al., 1976; Porvaznik and MacVittie, 1979; Eugenín et al., 2003; Jara et al., 1995). Plasma membrane hemichannels are one half of gap junction channels which allow direct ion transfer between cytoplasms of contacting cells and thus allow metabolic and electrical synchronization between the contacting cells. Hemichannels Connexin 43 (Eugenín et al., 2003; Jara et al., 1995; Beyer and Steinberg, 1991; Dosch et al., 2019), Connexin 37 (Kwak et al., 2002; Lu et al., 2018) and Pannexin 1 (Chen et al., 2019; Adamson and Leitinger, 2014; Pelegrin and Surprenant, 2006) have been identified in macrophages to date and proposed to affect macrophage activation and a range of functions (Rodjakovic et al., 2021), including macrophage migration (Shen et al., 2018), ATP signalling (Pelegrin and Surprenant, 2006) and cytokine production (Dosch et al., 2019). Importantly, growing evidence suggests that the presence of hemichannels and gap junctions in macrophages is crucial for many physiological and pathophysiological processes, such as heartbeat propagation (Hulsmans et al., 2017), immunomodulatory communication in the lungs (Westphalen et al., 2014) and intercellular communication in intestine (Al-Ghadban et al., 2016).
Finally, a novel mechanically-activated cation channel Piezo1 has been reported in macrophages. Piezo1 roles are proposed to include mechanical stimuli sensing, potentially via Piezo1 and integrins crosstalk and modulation of macrophage polarization (Atcha et al., 2021a; Atcha et al., 2021b), toll-like receptor 4-mediated enhancement of macrophage bactericidal activity (Geng et al., 2021) and macrophage migration (unpublished data). Recently, this channel has also been demonstrated to be a key regulator of erythrocyte phagocytosis by macrophages and iron metabolism (Ma et al., 2021). Since iron metabolism, inflammation and immunity are intimately linked (Ganz and Nemeth, 2015), this area of research deserves further consideration. Piezo1 is considered to be a promising candidate for therapeutic intervention against atherosclerosis and other cardiovascular diseases (Umar Shinge et al., 2022), and it will be interesting if this channel along with other mechano-sensing channels represent a mechanism by which physical stimuli together with chemical stimuli, such as PAMPs and DAMPs, can be integrated.
Research questions, future prospects and therapeutics
Methodology
The gold standard technique for identifying functional ion channels in a membrane is patch clamping electrophysiology. Being a common technique used to obtain electrophysiological data from many cell types, it can be technically challenging in cells which have dynamic membranes, as is the case for activated macrophages (Pi et al., 2014). Macrophages are heterogeneous populations of cells, and, since patch clamping experiments are carried out on single cells, investigators need to be aware of sampling issues, i.e. macrophages which are amenable to electrophysiological recordings may not represent bulk phenotypic behaviour of the macrophage population. Interestingly, research in neurons has developed Patch-seq protocols, where electrophysiological and transcriptomic data can be collected from the patched cell (Lipovsek et al., 2021). To the best of our knowledge this technique has not been investigated in macrophages to date, however, this could represent a powerful technique by which macrophage functional phenotypes could be linked to gene expression profiles. Cells have also been patch clamped in-situ e.g. neurons in brain slices. It could therefore be possible to patch clamp macrophages in tissue slices, e.g. spleen, granuloma, or even tumours to investigate tumour associated macrophage (TAM) activity. This methodology may also overcome some of the macrophage plasticity issues encountered when investigating these cells in-vitro.
One limitation to patch clamping is that it is low throughput, although automation of this technique is possible for some cell types. To investigate ion-channel effects in multiple cells simultaneously in vitro, fluorescent dyes can be used, however, these dyes require to be of sufficient spatial and temporal resolution to characterise ion channel dynamics (Haworth and Brackenbury, 2019). Furthermore, ion channels may have functions which are not dependent on their “channel” function. An example of this is the recent demonstration that the role of voltage-gated Ca2+ channels in regulating T-lymphocyte function is independent of cation flux (Cahalan, 2022). To investigate this possibility in macrophages a combination of electrophysiological, genomic, proteome and interactome methodologies will be required.
Care must also be taken when using pharmacological and genetic approaches to investigate ion channel functions. Channel blockers/openers must be chosen carefully and their relative selectivity for a particular target should be considered. This is especially important in ion channel research where the selectivity of pharmacological tools is often dependent on the subunit composition and dynamics of the ion channel. Also, it must be recognised that some ion channels can be found at multiple locations within the cell, where they may play different roles in cellular pathways. This confounding factor must be taken into account when using pharmacological and genetic approaches to manipulate ion channel activities and investigate their downstream effects.
Ion channel coordination
The coordination of different ion channels in a single cell to orchestrate a particular cellular function is well recognised in cell types such as neurons and smooth muscle. Evidence suggests such coordinated behaviour of ion channels is also present in macrophages. One example would be K+ channel opening creating a driving force for Ca2+ entry into the cells (see above). A number of ion channels, TWIK2, TRP, P2X, VRAC, and CLIC channels, are reported to regulate NLRP3 inflammasome (Li et al., 2021). Ion channels can also regulate cell volume changes which activate NLRP3. TRPV2 and TRPM7 in macrophages are thought to control the RVD (Liedtke, 2006; Compan et al., 2012; Santoni et al., 2015). Moreover, TRPM2-mediated Ca2+ influx has been suggested to be important for inflammasome activation (Zhong et al., 2013). Cytosolic K+ efflux is a common trigger involved in inflammasome activation. P2X7 activation was reported to promote the efflux of K+ and activate NLRP3 in macrophages (Ferrari et al., 1997, 2006; Mariathasan et al., 2006; Piccini et al., 2008; Barberà-Cremades et al., 2012; Muñoz-Planillo et al., 2013) (Pétrilli et al., 2007). Recently, Di et al. identified a K+ channel TWIK2 as another mediator of K+ efflux and inflammasome activation in macrophages (Wu et al., 2021) (Di et al., 2018). Finally, intracellular Cl− efflux, an event downstream of K+ efflux, is also reported to be critical upstream event for inflammasome activation. Two Cl− selective ion channels, VRAC and CLIC, have been reported to participate in inflammasome regulation in macrophages (Green et al., 2020) (Domingo-Fernández et al., 2017). The important point to make is that combinations of ion channels are involved in inflammasome activation; and these combinations will be dependent on the stimuli. Phagocytosis and polarization are other examples of macrophage functions discussed in this review, which are modulated by integrated ion channel networks (Table 1). It would not be surprising if the effects of individual ion channels on macrophage behaviour are in fact dependent on the actions of other channels. If so, the integration of these ion channel “circuits” with traditional cell signalling pathways in macrophages will need to be investigated.
Electrical excitability
In neurons the different composition of voltage-gated ion channels results in different firing properties (Berger and Crook, 2015) (Drion et al., 2015) but as the name suggests these ion channels are regulated my membrane voltage i.e. neurons are electrically excitable. Macrophage express an array of functional ion channels, some of which are voltage-gated. This leads us to the controversial question, are macrophages electrically excitable? While macrophages are generally considered non-excitable cells, the presence of gap junction channels Connexins in macrophages allows cardiac macrophages to facilitate electrical conduction through the distal atrioventricular node, where conducting cells densely intersperse with elongated macrophages expressing Connexin 43. When coupled to spontaneously beating cardiomyocytes via connexin-43-containing gap junctions, cardiac macrophages have a negative resting membrane potential and depolarize in synchrony with cardiomyocytes (Hulsmans et al., 2017). Moreover, computer simulations to quantitatively assess plausible effects of macrophages on electrotonically coupled cardiomyocytes showed that macrophages can depolarize resting cardiomyocytes, shorten early and prolong late action potential duration, with effects depending on coupling strength and individual macrophage electrophysiological properties, in particular resting membrane potential and presence/absence of Kir2.1 channel (Simon-Chica et al., 2021). To study this further, in-situ measurements of electrophysiological properties of macrophages in contact with other cells will have to be performed.
Therapeutic potential
As indicated in the introduction, macrophages not only have central roles in immune and inflammatory responses but participate in general homeostatic responses in the body (Murray and Wynn, 2011; Wynn et al., 2013). Therefore, it is not surprising that macrophage dysfunction may contribute to numerous pathologies and make this cell a prime target for therapeutic intervention. However, because of the central role of the macrophage in host defence, direct inhibition of macrophage functions is of limited value. A better therapeutic strategy would be to shape the macrophage actions, thus removing its aberrant effects while maintaining its desired functions. We suggest that the balance between macrophage pro-, anti-inflammatory and homeostatic responses is partly defined by ion channel actions. Therefore, could ion channel targeting drugs be used to fine-tune macrophage response in certain diseases?
Outlined above, a number of ion channels have been demonstrated to regulate macrophage biology, and a number of drugs which target these channels, predominantly in non-immune tissues/cells, have entered clinical trials or been approved for various diseases, e.g. epilepsy, cardiac dysrhythmia and anxiety (Imbrici et al., 2013; Priest and McDermott, 2015; Cojocaru et al., 2021) (Table 4). Illustrated in Table 4, drugs which target ion channels can be designed to block or open ion channels, as well as act as positive allosteric modulators. This together with various channel subunit compositions (both core and auxiliary) give researchers a large chemical space to explore when developing drugs targeting ion channels in macrophages and minimizing off target/tissue effects. Table 5 introduces the reader to several examples of diseases which may be amenable to targeting macrophage ion channels for their treatment.
Conclusion
In this review we have highlighted the current state of the literature regarding the expression and roles of ion channels in macrophages (Table 1; Figure 1). We believe that targeting ion channels in macrophages represents a viable therapeutic opportunity for the treatment of a number of diseases (Table 5). Indeed, rather than inhibiting inflammatory responses, we believe targeting ion channels may be able to shape the inflammatory response and thus provide better clinical utility.
Author contributions
AS, AJG, and DW were involved in the preparation and writing of this paper.
Conflict of interest
The authors declare that the research was conducted in the absence of any commercial or financial relationships that could be construed as a potential conflict of interest.
Publisher’s note
All claims expressed in this article are solely those of the authors and do not necessarily represent those of their affiliated organizations, or those of the publisher, the editors and the reviewers. Any product that may be evaluated in this article, or claim that may be made by its manufacturer, is not guaranteed or endorsed by the publisher.
References
Adamson, S. E., and Leitinger, N. (2014). The role of Pannexin1 in the induction and resolution of inflammation. FEBS Lett. 588, 1416–1422. doi:10.1016/J.FEBSLET.2014.03.009
Al-Ghadban, S., Kaissi, S., Homaidan, F. R., Naim, H. Y., and El-Sabban, M. E. (2016). Cross-talk between intestinal epithelial cells and immune cells in inflammatory bowel disease. Sci. Rep. 6, 29783. doi:10.1038/srep29783
Al-Karagholi, M. A. M., Ghanizada, H., Nielsen, C. A. W., Skandarioon, C., Snellman, J., Lopez Lopez, C., et al. (2020). Opening of BK Ca channels alters cerebral hemodynamic and causes headache in healthy volunteers. Cephalalgia 40, 1145–1154. doi:10.1177/0333102420940681
Al-Karagholi, M. A. M., Hansen, J. M., Severinsen, J., Jansen-Olesen, I., and Ashina, M. (2017). The K ATP channel in migraine pathophysiology: A novel therapeutic target for migraine. J. Headache Pain 18, 90. doi:10.1186/S10194-017-0800-8
Alexander, S. P. H., Mathie, A., and Peters, J. A. (2011). Guide to receptors and channels (GRAC), 5th edition. Br. J. Pharmacol. 164 (1), S1–S324. doi:10.1111/J.1476-5381.2011.01649_1.X
Alsaloum, M., Higerd, G. P., Effraim, P. R., and Waxman, S. G. (2020). Status of peripheral sodium channel blockers for non-addictive pain treatment. Nat. Rev. Neurol. 16, 689–705. doi:10.1038/S41582-020-00415-2
Antony, C., Mehto, S., Tiwari, B. K., Singh, Y., and Natarajan, K. (2015). Regulation of L-type voltage gated calcium channel CACNA1S in macrophages upon Mycobacterium tuberculosis infection. PLoS ONE 10, e0124263. doi:10.1371/JOURNAL.PONE.0124263
Asatryan, L., Ostrovskaya, O., Lieu, D., and Davies, D. L. (2018). Ethanol differentially modulates P2X4 and P2X7 receptor activity and function in BV2 microglial cells. Neuropharmacology 128, 11–21. doi:10.1016/J.NEUROPHARM.2017.09.030
Ataga, K. I., Orringer, E. P., Styles, L., Vichinsky, E. P., Swerdlow, P., Davis, G. A., et al. (2006). Dose-escalation study of ICA-17043 in patients with sickle cell disease. Pharmacotherapy 26, 1557–1564. doi:10.1592/PHCO.26.11.1557
Ataga, K. I., Reid, M., Ballas, S. K., Yasin, Z., Bigelow, C., James, L. S., et al. (2011). Improvements in haemolysis and indicators of erythrocyte survival do not correlate with acute vaso-occlusive crises in patients with sickle cell disease: A phase III randomized, placebo-controlled, double-blind study of the gardos channel blocker senicapoc (ICA-17043). Br. J. Haematol. 153, 92–104. doi:10.1111/J.1365-2141.2010.08520.X
Ataga, K. I., Staffa, S. J., Brugnara, C., and Stocker, J. W. (2021). Haemoglobin response to senicapoc in patients with sickle cell disease: A re-analysis of the phase III trial. Br. J. Haematol. 192, e129–e132. doi:10.1111/BJH.17345
Atcha, H., Jairaman, A., Holt, J., Meli, V., Nagalla, R., Veerasubramanian, P., et al. (2021a). Mechanically activated ion channel Piezo1 modulates macrophage polarization and stiffness sensing. Nat. Commun. 12, 3256. doi:10.1038/S41467-021-23482-5
Atcha, H., Meli, V. S., Davis, C. T., Brumm, K. T., Anis, S., Chin, J., et al. (2021b). Crosstalk between CD11b and Piezo1 mediates macrophage responses to mechanical cues. Front. Immunol. 12, 3505. doi:10.3389/fimmu.2021.689397
Atobe, M. (2019). Activation of transient receptor potential vanilloid (TRPV) 4 as a therapeutic strategy in osteoarthritis. Curr. Top. Med. Chem. 19, 2254–2267. doi:10.2174/1568026619666191010162850
Atobe, M., Nagami, T., Muramatsu, S., Ohno, T., Kitagawa, M., Suzuki, H., et al. (2019). Discovery of novel transient receptor potential vanilloid 4 (TRPV4) agonists as regulators of chondrogenic differentiation: Identification of quinazolin-4(3 H)-ones and in vivo studies on a surgically induced rat model of osteoarthritis. J. Med. Chem. 62, 1468–1483. doi:10.1021/ACS.JMEDCHEM.8B01615/SUPPL_FILE/JM8B01615_SI_002.CSV
Báez-Pagán, C. A., Delgado-Vélez, M., and Lasalde-Dominicci, J. A. (2015). Activation of the macrophage α7 nicotinic acetylcholine receptor and control of inflammation. J. Neuroimmune Pharmacol. 10, 468–476. doi:10.1007/S11481-015-9601-5
Bai, W., Liu, M., and Xiao, Q. (2021). The diverse roles of TMEM16A Ca2+-activated Cl− channels in inflammation. J. Adv. Res. 33, 53–68. doi:10.1016/J.JARE.2021.01.013
Baker, D., Pryce, G., Visintin, C., Sisay, S., Bondarenko, A. I., Vanessa Ho, W. S., et al. (2017). Big conductance calcium-activated potassium channel openers control spasticity without sedation. Br. J. Pharmacol. 174, 2662–2681. doi:10.1111/BPH.13889
Balakrishna, S., Song, W., Achanta, S., Doran, S., Liu, B., Kaelberer, M., et al. (2014). TRPV4 inhibition counteracts edema and inflammation and improves pulmonary function and oxygen saturation in chemically induced acute lung injury. Am. J. Physiol. Lung Cell. Mol. Physiol. 307, L158–L172. doi:10.1152/AJPLUNG.00065.2014
Barberà-Cremades, M., Baroja-Mazo, A., Gomez, A., Machado, F., di Virgilio, F., and Pelegrín, P. (2012). P2X7 receptor-stimulation causes fever via PGE2 and IL-1β release. FASEB J. 26, 2951–2962. doi:10.1096/FJ.12-205765
Barde, P. J., Viswanadha, S., Veeraraghavan, S., Vakkalanka, S. v., and Nair, A. (2021). A first-in-human study to evaluate the safety, tolerability and pharmacokinetics of RP3128, an oral calcium release-activated calcium (CRAC) channel modulator in healthy volunteers. J. Clin. Pharm. Ther. 46, 677–687. doi:10.1111/JCPT.13322
Bareille, P., Murdoch, R. D., Denyer, J., Bentley, J., Smart, K., Yarnall, K., et al. (2013). The effects of a TRPV1 antagonist, SB-705498, in the treatment of seasonal allergic rhinitis. Int. J. Clin. Pharmacol. Ther. 51, 576–584. doi:10.5414/CP201890
Bàthori, G., Parolini, I., Tombola, F., Szabò, I., Messina, A., Oliva, M., et al. (1999). Porin is present in the plasma membrane where it is concentrated in caveolae and caveolae-related domains. J. Biol. Chem. 274, 29607–29612. doi:10.1074/JBC.274.42.29607
Beceiro, S., Radin, J. N., Chatuvedi, R., Piazuelo, M. B., Horvarth, D. J., Cortado, H., et al. (2016). TRPM2 ion channels regulate macrophage polarization and gastric inflammation during Helicobacter pylori infection. Mucosal Immunol. 10, 493–507. doi:10.1038/mi.2016.60
Beggs, S., Trang, T., and Salter, M. (2012). P2X4R+ microglia drive neuropathic pain. Nat. Neurosci. 15, 1068–1073. doi:10.1038/NN.3155
Belchamber, K. B. R., and Donnelly, L. E. (2017). “Macrophage dysfunction in respiratory disease,” in Results and problems in cell differentiation (Springer-Verlag), 299–313. doi:10.1007/978-3-319-54090-0_12
Beltrand, J., Baptiste, A., Busiah, K., Bouazza, N., Godot, C., Boucheron, A., et al. (2019). Glibenclamide oral suspension: Suitable and effective in patients with neonatal diabetes. Pediatr. Diabetes 20, 246–254. doi:10.1111/PEDI.12823
Berger, S. D., and Crook, S. M. (2015). Modeling the influence of ion channels on neuron dynamics in Drosophila. Front. Comput. Neurosci. 9, 139. doi:10.3389/fncom.2015.00139
Beyer, E. C., and Steinberg, T. H. (1991). Evidence that the gap junction protein connexin-43 is the ATP-induced pore of mouse macrophages. J. Biol. Chem. 266, 7971–7974. doi:10.1016/S0021-9258(18)92924-8
Bhandage, A. K., and Barragan, A. (2021). GABAergic signaling by cells of the immune system: More the rule than the exception. Cell. Mol. Life Sci. 78, 5667–5679. doi:10.1007/S00018-021-03881-Z
Black, J., Newcombe, J., and Waxman, S. (2013). Nav1.5 sodium channels in macrophages in multiple sclerosis lesions. Mult. Scler. 19, 532–542. doi:10.1177/1352458512460417
Borovikova, L. v., Ivanova, S., Zhang, M., Yang, H., Botchkina, G. I., Watkins, L. R., et al. (2000). Vagus nerve stimulation attenuates the systemic inflammatory response to endotoxin. Nature 405, 458–462. doi:10.1038/35013070
Bouron, A., Kiselyov, K., and Oberwinkler, J. (2015). Permeation, regulation and control of expression of TRP channels by trace metal ions. Pflugers Arch. 467, 1143–1164. doi:10.1007/S00424-014-1590-3
Bowler, J., Bailey, R., North, R., and Surprenant, A. (2003). P2X4, P2Y1 and P2Y2 receptors on rat alveolar macrophages. Br. J. Pharmacol. 140, 567–575. doi:10.1038/SJ.BJP.0705459
Braun, A., Gessner, J., Varga-Szabo, D., Syed, S., Konrad, S., Stegner, D., et al. (2009). STIM1 is essential for Fcgamma receptor activation and autoimmune inflammation. Blood 113, 1097–1104. doi:10.1182/BLOOD-2008-05-158477
Brown, S. B., and Dransfield, I. (2008). Electric fields and inflammation: May the force be with you. ScientificWorldJournal. 8, 1280–1294. doi:10.1100/tsw.2008.158
Bruen, C., Al-Saadi, M., Michelson, E. A., Tanios, M., Mendoza-Ayala, R., Miller, J., et al. (2022). Auxora vs. placebo for the treatment of patients with severe COVID-19 pneumonia: A randomized-controlled clinical trial. Crit. Care 26, 101. doi:10.1186/S13054-022-03964-8
Bruen, C., Miller, J., Wilburn, J., Mackey, C., Bollen, T. L., Stauderman, K., et al. (2021). Auxora for the treatment of patients with acute pancreatitis and accompanying systemic inflammatory response syndrome: Clinical development of a calcium release-activated calcium channel inhibitor. Pancreas 50, 537–543. doi:10.1097/MPA.0000000000001793
Bu, J., Huang, S., Wang, J., Xia, T., Liu, H., You, Y., et al. (2021). The GABAA receptor influences pressure overload-induced heart failure by modulating macrophages in mice. Front. Immunol. 0, 670153. doi:10.3389/FIMMU.2021.670153
Burow, P., Klapperstück, M., and Markwardt, F. (2014). Activation of ATP secretion via volume-regulated anion channels by sphingosine-1-phosphate in RAW macrophages. Pflugers Arch. 467, 1215–1226. doi:10.1007/S00424-014-1561-8
Cahalan, M. D. (2022). Voltage-gated Ca2+ channel proteins do not function as ion channels in T cells. Sci. Signal. 15, 5594. doi:10.1126/SCISIGNAL.ABQ5594
Camm, A. J., Dorian, P., Hohnloser, S. H., Kowey, P. R., Tyl, B., Ni, Y., et al. (2019). A randomized, double-blind, placebo-controlled trial assessing the efficacy of S66913 in patients with paroxysmal atrial fibrillation. Eur. Heart J. Cardiovasc. Pharmacother. 5, 21–28. doi:10.1093/EHJCVP/PVY022
Cang, C., Aranda, K., Seo, Y., Gasnier, B., and Ren, D. (2015). TMEM175 is an organelle K+ channel regulating lysosomal function. Cell 162, 1101–1112. doi:10.1016/J.CELL.2015.08.002
Cao, Q., Zhong, X., Zou, Y., Zhang, Z., Toro, L., and Dong, X. (2015). BK channels alleviate lysosomal storage diseases by providing positive feedback regulation of lysosomal Ca2+ release. Dev. Cell 33, 427–441. doi:10.1016/J.DEVCEL.2015.04.010
Cao, Y., and Zheng, O. J. (2014). Tonabersat for migraine prophylaxis: A systematic review. Pain Physician 17, 1–8. doi:10.36076/PPJ.2014/17/1
Carrithers, L., Hulseberg, P., Sandor, M., and Carrithers, M. (2011). The human macrophage sodium channel NaV1.5 regulates mycobacteria processing through organelle polarization and localized calcium oscillations. FEMS Immunol. Med. Microbiol. 63, 319–327. doi:10.1111/J.1574-695X.2011.00853.X
Carrithers, M., Dib-Hajj, S., Carrithers, L., Tokmoulina, G., Pypaert, M., Jonas, E., et al. (2007). Expression of the voltage-gated sodium channel NaV1.5 in the macrophage late endosome regulates endosomal acidification. J. Immunol. 178, 7822–7832. doi:10.4049/JIMMUNOL.178.12.7822
Castranova, V., Bowman, L., and Miles, P. R. (1979). Transmembrane potential and ionic content of rat alveolar macrophages. J. Cell. Physiol. 101, 471–479. doi:10.1002/JCP.1041010313
Chauhan, A., Sun, Y., Sukumaran, P., Zangbede, F. O. Q., Jondle, C. N., Sharma, A., et al. (2018). M1 macrophage polarization is dependent on TRPC1-mediated calcium entry. iScience 8, 85–102. doi:10.1016/J.ISCI.2018.09.014
Chen, C.-C., Krogsaeter, E., Butz, E. S., Li, Y., Puertollano, R., Wahl-Schott, C., et al. (2020). TRPML2 is an osmo/mechanosensitive cation channel in endolysosomal organelles. Sci. Adv. 6, eabb5064. doi:10.1126/SCIADV.ABB5064
Chen, C. C., Krogsaeter, E., and Grimm, C. (2021). Two-pore and TRP cation channels in endolysosomal osmo-/mechanosensation and volume regulation. Biochim. Biophys. Acta. Mol. Cell Res. 1868, 118921. doi:10.1016/J.BBAMCR.2020.118921
Chen, W., Zhu, S., Wang, Y., Li, J., Qiang, X., Zhao, X., et al. (2019). Enhanced macrophage Pannexin 1 expression and hemichannel activation exacerbates lethal experimental sepsis. Sci. Rep. 9, 160. doi:10.1038/s41598-018-37232-z
Chen, Y., Raman, G., Bodendiek, S., O’Donnell, M., and Wulff, H. (2011). The KCa3.1 blocker TRAM-34 reduces infarction and neurological deficit in a rat model of ischemia/reperfusion stroke. J. Cereb. Blood Flow. Metab. 31, 2363–2374. doi:10.1038/JCBFM.2011.101
Chung, I., Zelivyanskaya, M., and Gendelman, H. E. (2002). Mononuclear phagocyte biophysiology influences brain transendothelial and tissue migration: Implication for HIV-1-associated dementia. J. Neuroimmunol. 122, 40–54. doi:10.1016/S0165-5728(01)00462-3
ClinicalTrials.gov, NCT01476098 (2016). NCT01476098. Available at: https://clinicaltrials.gov/ct2/show/NCT01476098 (Accessed August 9, 2022).
ClinicalTrials.gov, NCT02446340 (2015). NCT02446340. Available at: https://clinicaltrials.gov/ct2/show/study/NCT02446340 (Accessed August 9, 2022).
ClinicalTrials.gov, NCT02542787 (2018). NCT02542787. Available at: https://clinicaltrials.gov/ct2/show/NCT02542787 (Accessed August 9, 2022).
Cojocaru, A., Burada, E., Bălșeanu, A. T., Deftu, A. F., Cătălin, B., Popa-Wagner, A., et al. (2021). Roles of microglial ion Channel in neurodegenerative diseases. J. Clin. Med. 10, 1239. doi:10.3390/JCM10061239
Compan, V., Baroja-Mazo, A., López-Castejón, G., Gomez, A., Martínez, C., Angosto, D., et al. (2012). Cell volume regulation modulates NLRP3 inflammasome activation. Immunity 37, 487–500. doi:10.1016/J.IMMUNI.2012.06.013
Conceicao, V. N., Sun, Y., Zboril, E. K., de la Chapa, J. J., and Singh, B. B. (2019). Loss of Ca 2+ entry via Orai-TRPC1 induces ER stress, initiating immune activation in macrophages. J. Cell Sci. 133, jcs237610. doi:10.1242/JCS.237610
Craner, M., Damarjian, T., Liu, S., Hains, B., Lo, A., Black, J., et al. (2005). Sodium channels contribute to microglia/macrophage activation and function in EAE and MS. Glia 49, 220–229. doi:10.1002/GLIA.20112
Crayne, C. B., Albeituni, S., Nichols, K. E., and Cron, R. Q. (2019). The immunology of macrophage activation syndrome. Front. Immunol. 10, 119. doi:10.3389/fimmu.2019.00119
Danelishvili, L., Chinison, J. J. J., Pham, T., Gupta, R., and Bermudez, L. E. (2017). The Voltage-Dependent Anion Channels (VDAC) of Mycobacterium avium phagosome are associated with bacterial survival and lipid export in macrophages. Sci. Rep. 7, 7007–7014. doi:10.1038/s41598-017-06700-3
Davis, L. C., Morgan, A. J., and Galione, A. (2020). NAADP‐regulated two‐pore channels drive phagocytosis through endo‐lysosomal Ca2+ nanodomains, calcineurin and dynamin. EMBO J. 39, e104058. doi:10.15252/EMBJ.2019104058
Dayam, R., Saric, A., Shilliday, R., and Botelho, R. (2015). The phosphoinositide-gated lysosomal Ca(2+) channel, TRPML1, is required for phagosome maturation. Traffic 16, 1010–1026. doi:10.1111/TRA.12303
de Jonge, W., van der Zanden, E., The, F., Bijlsma, M., van Westerloo, D., Bennink, R., et al. (2005). Stimulation of the vagus nerve attenuates macrophage activation by activating the Jak2-STAT3 signaling pathway. Nat. Immunol. 6, 844–851. doi:10.1038/NI1229
DeCoursey, T. E. (2016). The intimate and controversial relationship between voltage-gated proton channels and the phagocyte NADPH oxidase. Immunol. Rev. 273, 194–218. doi:10.1111/IMR.12437
DeCoursey, T. (2010). Voltage-gated proton channels find their dream job managing the respiratory burst in phagocytes. Physiol. (Bethesda) 25, 27–40. doi:10.1152/PHYSIOL.00039.2009
del Corno, M., Liu, Q. H., Schols, D., de Clercq, E., Gessani, S., Freedman, B. D., et al. (2001). HIV-1 gp120 and chemokine activation of Pyk2 and mitogen-activated protein kinases in primary macrophages mediated by calcium-dependent, pertussis toxin–insensitive chemokine receptor signaling. Blood 98, 2909–2916. doi:10.1182/BLOOD.V98.10.2909
Di, A., Gao, X.-P., Qian, F., Kawamura, T., Han, J., Hecquet, C., et al. (2012). The redox-sensitive cation channel TRPM2 modulates phagocyte ROS production and inflammation. Nat. Immunol. 13, 29–34. doi:10.1038/NI.2171
Di, A., Xiong, S., Ye, Z., Malireddi, R. K. S., Kometani, S., Zhong, M., et al. (2018). The TWIK2 potassium efflux channel in macrophages mediates NLRP3 inflammasome-induced inflammation. Immunity 49, 56–65. doi:10.1016/J.IMMUNI.2018.04.032
di Virgilio, F., Sarti, A. C., and Grassi, F. (2018). Modulation of innate and adaptive immunity by P2X ion channels. Curr. Opin. Immunol. 52, 51–59. doi:10.1016/J.COI.2018.03.026
Domingo-Fernández, R., Coll, R. C., Kearney, J., Breit, S., and O’Neill, L. A. J. (2017). The intracellular chloride channel proteins CLIC1 and CLIC4 induce IL-1β transcription and activate the NLRP3 inflammasome. J. Biol. Chem. 292, 12077–12087. doi:10.1074/JBC.M117.797126
dos Reis, G. A., Persechini, P. M., Ribeiro, J. C., and Oliveira-Castro, G. M. (1979). Electrophysiology of phagocytic membranes. II. Membrane potential and induction of slow hyperpolarizations in activated macrophages. Biochim. Biophys. Acta 552, 331–340. doi:10.1016/0005-2736(79)90287-6
Dosch, M., Zindel, J., Jebbawi, F., Melin, N., Sanchez-Taltavull, D., Stroka, D., et al. (2019). Connexin-43-dependent ATP release mediates macrophage activation during sepsis. Elife 8, e42670. doi:10.7554/ELIFE.42670
Drexler, S. K., Kong, P. L., Wales, J., and Foxwell, B. M. (2008). Cell signalling in macrophages, the principal innate immune effector cells of rheumatoid arthritis. Arthritis Res. Ther. 10, 216. doi:10.1186/ar2481
Drion, G., O’Leary, T., and Marder, E. (2015). Ion channel degeneracy enables robust and tunable neuronal Firing rates. Proc. Natl. Acad. Sci. U. S. A. 112, E5361–E5370. doi:10.1073/pnas.1516400112
Dutta, B., Goswami, R., and Rahaman, S. (2020). TRPV4 plays a role in matrix stiffness-induced macrophage polarization. Front. Immunol. 11, 570195. doi:10.3389/FIMMU.2020.570195
Engstrøm, T., Nepper-Christensen, L., Helqvist, S., Kløvgaard, L., Holmvang, L., Jørgensen, E., et al. (2018). Danegaptide for primary percutaneous coronary intervention in acute myocardial infarction patients: A phase 2 randomised clinical trial. Heart 104, 1593–1599. doi:10.1136/HEARTJNL-2017-312774
Eser, A., Colombel, J. F., Rutgeerts, P., Vermeire, S., Vogelsang, H., Braddock, M., et al. (2015). Safety and efficacy of an oral inhibitor of the purinergic receptor P2X7 in adult patients with moderately to severely active crohn’s disease: A randomized placebo-controlled, double-blind, phase IIa study. Inflamm. Bowel Dis. 21, 2247–2253. doi:10.1097/MIB.0000000000000514
Eugenín, E. A., Brañes, M. C., Berman, J. W., and Sáez, J. C. (2003). TNF-Α plus IFN-γ induce Connexin43 expression and formation of gap junctions between human monocytes/macrophages that enhance physiological responses. J. Immunol. 170, 1320–1328. doi:10.4049/JIMMUNOL.170.3.1320
Fattori, V., Hohmann, M. S. N., Rossaneis, A. C., Pinho-Ribeiro, F. A., and Verri, W. A. (2016). Capsaicin: Current understanding of its mechanisms and therapy of pain and other pre-clinical and clinical uses. Molecules 21, E844. doi:10.3390/MOLECULES21070844
Felipe, A., Soler, C., and Comes, N. (2010). Kv1.5 in the immune system: The good, the bad, or the ugly? Front. Physiol. 1, 152. doi:10.3389/fphys.2010.00152
Feng, X., Huang, Y., Lu, Y., Xiong, J., Wong, C.-O., Yang, P., et al. (2014). Drosophila TRPML forms PI(3, 5)P2-activated cation channels in both endolysosomes and plasma membrane. J. Biol. Chem. 289, 4262–4272. doi:10.1074/JBC.M113.506501
Fernández-Carvajal, A., González-Muñiz, R., Fernández-Ballester, G., and Ferrer-Montiel, A. (2020). Investigational drugs in early phase clinical trials targeting thermotransient receptor potential (thermoTRP) channels. Expert Opin. Investig. Drugs 29, 1209–1222. doi:10.1080/13543784.2020.1825680
Ferrari, D., Chiozzi, P., Falzoni, S., Dal Susino, M., Melchiorri, L., Baricordi, O. R., et al. (1997). Extracellular ATP triggers IL-1 beta release by activating the purinergic P2Z receptor of human macrophages. J. Immunol. 159, 1451–1458.
Ferrari, D., Pizzirani, C., Adinolfi, E., Lemoli, R., Curti, A., Idzko, M., et al. (2006). The P2X7 receptor: A key player in IL-1 processing and release. J. Immunol. 176, 3877–3883. doi:10.4049/JIMMUNOL.176.7.3877
Florian, H., Meier, A., Gauthier, S., Lipschitz, S., Lin, Y., Tang, Q., et al. (2016). Efficacy and safety of ABT-126 in subjects with mild-to-moderate alzheimer’s disease on stable doses of acetylcholinesterase inhibitors: A randomized, double-blind, placebo-controlled study. J. Alzheimers Dis. 51, 1237–1247. doi:10.3233/JAD-150978
Ford, J., Milnes, J., el Haou, S., Wettwer, E., Loose, S., Matschke, K., et al. (2016). The positive frequency-dependent electrophysiological effects of the IKur inhibitor XEN-D0103 are desirable for the treatment of atrial fibrillation. Heart rhythm. 13, 555–564. doi:10.1016/J.HRTHM.2015.10.003
Fordyce, C. B., Jagasia, R., Zhu, X., and Schlichter, L. C. (2005). Microglia Kv1.3 channels contribute to their ability to kill neurons. J. Neurosci. 25, 7139–7149. doi:10.1523/JNEUROSCI.1251-05.2005
Foster, V. S., Rash, L. D., King, G. F., and Rank, M. M. (2021). Acid-sensing ion channels: Expression and function in resident and infiltrating immune cells in the central nervous system. Front. Cell. Neurosci. 15, 376. doi:10.3389/fncel.2021.738043
Franceschini, A., Capece, M., Chiozzi, P., Falzoni, S., Sanz, J. M., Sarti, A. C., et al. (2015). The P2X7 receptor directly interacts with the NLRP3 inflammasome scaffold protein. FASEB J. 29, 2450–2461. doi:10.1096/FJ.14-268714
Freedman, R., Olincy, A., Buchanan, R. W., Harris, J. G., Gold, J. M., Johnson, L., et al. (2008). Initial phase 2 trial of a nicotinic agonist in schizophrenia. Am. J. Psychiatry 165, 1040–1047. doi:10.1176/APPI.AJP.2008.07071135
Fujii, T., Mashimo, M., Moriwaki, Y., Misawa, H., Ono, S., Horiguchi, K., et al. (2017). Expression and function of the cholinergic system in immune cells. Front. Immunol. 8, 1085. doi:10.3389/fimmu.2017.01085
Gallin, E. K., and Gallin, J. I. (1977). Interaction of chemotactic factors with human macrophages: Induction of transmembrane potential changes. J. Cell Biol. 75, 277–289. doi:10.1083/JCB.75.1.277
Gallin, E. K., and Livengood, D. R. (1980). Nonlinear current-voltage relationships in cultured macrophages. J. Cell Biol. 85, 160–165. doi:10.1083/JCB.85.1.160
Gallin, E. K. (1981). Voltage clamp studies in macrophages from mouse spleen cultures. Science 214, 458–460. doi:10.1126/SCIENCE.7291986
Ganz, T., and Nemeth, E. (2015). Iron homeostasis in host defence and inflammation. Nat. Rev. Immunol. 15, 500–510. doi:10.1038/NRI3863
Gao, Y., Hanley, P., Rinné, S., Zuzarte, M., and Daut, J. (2010). Calcium-activated K(+) channel (K(Ca)3.1) activity during Ca(2+) store depletion and store-operated Ca(2+) entry in human macrophages. Cell Calcium 48, 19–27. doi:10.1016/J.CECA.2010.06.002
Gault, L. M., Ritchie, C. W., Robieson, W. Z., Pritchett, Y., Othman, A. A., and Lenz, R. A. (2015). A phase 2 randomized, controlled trial of the α7 agonist ABT-126 in mild-to-moderate Alzheimer’s dementia. Alzheimers Dement. 1, 81–90. doi:10.1016/J.TRCI.2015.06.001
Gee, K. W., Olincy, A., Kanner, R., Johnson, L., Hogenkamp, D., Harris, J., et al. (2017). First in human trial of a type I positive allosteric modulator of alpha7-nicotinic acetylcholine receptors: Pharmacokinetics, safety, and evidence for neurocognitive effect of AVL-3288. J. Psychopharmacol. 31, 434–441. doi:10.1177/0269881117691590
Geng, J., Shi, Y., Zhang, J., Yang, B., Wang, P., Yuan, W., et al. (2021). TLR4 signalling via Piezo1 engages and enhances the macrophage mediated host response during bacterial infection. Nat. Commun. 12, 3519. doi:10.1038/s41467-021-23683-y
Gill, J. K., Savolainen, M., Young, G. T., Zwart, R., Sher, E., and Millar, N. S. (2011). Agonist activation of α7 nicotinic acetylcholine receptors via an allosteric transmembrane site. Proc. Natl. Acad. Sci. U. S. A. 108, 5867–5872. doi:10.1073/PNAS.1017975108/SUPPL_FILE/PNAS.201017975SI.PDF
Goswami, R., Arya, R. K., Sharma, S., Dutta, B., Stamov, D. R., Zhu, X., et al. (2021). Mechanosensing by TRPV4 mediates stiffness-induced foreign body response and giant cell formation. Sci. Signal. 14, eabd4077. doi:10.1126/SCISIGNAL.ABD4077
Goswami, R., Merth, M., Sharma, S., Alharbi, M. O., Aranda-Espinoza, H., Zhu, X., et al. (2017). TRPV4 calcium-permeable channel is a novel regulator of oxidized LDL-induced macrophage foam cell formation. Free Radic. Biol. Med. 110, 142–150. doi:10.1016/J.FREERADBIOMED.2017.06.004
Goyal, N., Skrdla, P., Schroyer, R., Kumar, S., Fernando, D., Oughton, A., et al. (2019). Clinical pharmacokinetics, safety, and tolerability of a novel, first-in-class TRPV4 ion Channel inhibitor, GSK2798745, in healthy and heart failure subjects. Am. J. Cardiovasc. Drugs 19, 335–342. doi:10.1007/S40256-018-00320-6
Granfeldt, A., Andersen, L. W., Vallentin, M. F., Hilberg, O., Hasselstrøm, J. B., Sørensen, L. K., et al. (2022). Senicapoc treatment in COVID-19 patients with severe respiratory insufficiency-A randomized, open-label, phase II trial. Acta Anaesthesiol. Scand. 66, 838–846. doi:10.1111/AAS.14072
Gray, M., Choy, C., Dayam, R., Ospina-Escobar, E., Somerville, A., Xiao, X., et al. (2016). Phagocytosis enhances lysosomal and bactericidal properties by activating the transcription factor TFEB. Curr. Biol. 26, 1955–1964. doi:10.1016/J.CUB.2016.05.070
Green, J. P., Swanton, T., Morris, L. v., El-Sharkawy, L. Y., Cook, J., Yu, S., et al. (2020). LRRC8A is essential for hypotonicity-but not for DAMP-induced NLRP3 inflammasome activation. Elife 9, e59704–22. doi:10.7554/ELIFE.59704
Greenberg, S., el Khoury, J., di Virgilio, F., Kaplan, E., and Silverstein, S. (1991). Ca(2+)-independent F-actin assembly and disassembly during Fc receptor-mediated phagocytosis in mouse macrophages. J. Cell Biol. 113, 757–767. doi:10.1083/JCB.113.4.757
Griffin, C. E., Kaye, A. M., Rivera Bueno, F., and Kaye, A. D. (2013). Benzodiazepine pharmacology and central nervous system–mediated effects. Ochsner J. 13, 214–223. Available at:/pmc/articles/PMC3684331/(Accessed August 9, 2022).
Grimaldi, A., D’Alessandro, G., Golia, M. T., Grössinger, E. M., di Angelantonio, S., Ragozzino, D., et al. (2016). KCa3.1 inhibition switches the phenotype of glioma-infiltrating microglia/macrophages. Cell Death Dis. 7, e2174. doi:10.1038/CDDIS.2016.73
Gu, B., Saunders, B., Jursik, C., and Wiley, J. (2010). The P2X7-nonmuscle myosin membrane complex regulates phagocytosis of nonopsonized particles and bacteria by a pathway attenuated by extracellular ATP. Blood 115, 1621–1631. doi:10.1182/BLOOD-2009-11-251744
Haig, G. M., Bain, E. E., Robieson, W. Z., Baker, J. D., and Othman, A. A. (2016a). A randomized trial to assess the efficacy and safety of ABT-126, a selective α7 nicotinic acetylcholine receptor agonist, in the treatment of cognitive impairment in schizophrenia. Am. J. Psychiatry 173, 827–835. doi:10.1176/APPI.AJP.2015.15010093
Haig, G., Wang, D., Othman, A. A., and Zhao, J. (2016b). The α7 nicotinic agonist ABT-126 in the treatment of cognitive impairment associated with schizophrenia in nonsmokers: Results from a randomized controlled phase 2b study. Neuropsychopharmacology 41, 2893–2902. doi:10.1038/NPP.2016.101
Hamanaka, K., Jian, M., Townsley, M., King, J., Liedtke, W., Weber, D., et al. (2010). TRPV4 channels augment macrophage activation and ventilator-induced lung injury. Am. J. Physiol. Lung Cell. Mol. Physiol. 299, L353–L362. doi:10.1152/AJPLUNG.00315.2009
Hanley, P., Musset, B., Renigunta, V., Limberg, S., Dalpke, A., Sus, R., et al. (2004). Extracellular ATP induces oscillations of intracellular Ca2+ and membrane potential and promotes transcription of IL-6 in macrophages. Proc. Natl. Acad. Sci. U. S. A. 101, 9479–9484. doi:10.1073/PNAS.0400733101
Haworth, A. S., and Brackenbury, W. J. (2019). Emerging roles for multifunctional ion channel auxiliary subunits in cancer. Cell Calcium 80, 125–140. doi:10.1016/J.CECA.2019.04.005
Herr, N., Bode, C., and Duerschmied, D. (2017). The effects of serotonin in immune cells. Front. Cardiovasc. Med. 4, 48. doi:10.3389/FCVM.2017.00048
Hishikawa, T., Cheung, J., Yelamarty, R., and Knutson, D. (1991). Calcium transients during Fc receptor-mediated and nonspecific phagocytosis by murine peritoneal macrophages. J. Cell Biol. 115, 59–66. doi:10.1083/JCB.115.1.59
Hulsmans, M., Clauss, S., Xiao, L., Aguirre, A. D., King, K. R., Hanley, A., et al. (2017). Macrophages facilitate electrical conduction in the heart. Cell 169, 510–522. doi:10.1016/J.CELL.2017.03.050
Imbrici, P., Camerino, D. C., and Tricarico, D. (2013). Major channels involved in neuropsychiatric disorders and therapeutic perspectives. Front. Genet. 4, 76. doi:10.3389/FGENE.2013.00076
Ince, C., Ypey, D. L., van Furth, R., and Verveen, A. A. (1983). Estimation of the membrane potential of cultured macrophages from the fast potential transient upon microelectrode entry. J. Cell Biol. 96, 796–801. doi:10.1083/JCB.96.3.796
IUPHAR/BPS (2011). IUPHAR/BPS Guide to PHARMACOLOGY. Available at: https://www.guidetopharmacology.org/.
Jara, P. I., Boric, M. P., and Sáez, J. C. (1995). Leukocytes express connexin 43 after activation with lipopolysaccharide and appear to form gap junctions with endothelial cells after ischemia-reperfusion. Proc. Natl. Acad. Sci. U. S. A. 92, 7011–7015. doi:10.1073/PNAS.92.15.7011
Jarvis, M. F., and Khakh, B. S. (2009). ATP-gated P2X cation-channels. Neuropharmacology 56, 208–215. doi:10.1016/J.NEUROPHARM.2008.06.067
Jembrek, M., and Vlainic, J. (2015). GABA receptors: Pharmacological potential and pitfalls. Curr. Pharm. Des. 21, 4943–4959. doi:10.2174/1381612821666150914121624
Jensen, B. S. (2002). BMS‐204352: A potassium channel opener developed for the treatment of stroke. CNS Drug Rev. 8, 353–360. doi:10.1111/J.1527-3458.2002.TB00233.X
Jiang, L., Salao, K., Li, H., Rybicka, J. M., Yates, R. M., Luo, X. W., et al. (2012). Intracellular chloride channel protein CLIC1 regulates macrophage function through modulation of phagosomal acidification. J. Cell Sci. 125, 5479–5488. doi:10.1242/JCS.110072
Joe, Y., Kim, H. J., Kim, S., Chung, J., Ko, M. S., Lee, W. H., et al. (2011). Tristetraprolin mediates anti-inflammatory effects of nicotine in lipopolysaccharide-stimulated macrophages. J. Biol. Chem. 286, 24735–24742. doi:10.1074/JBC.M110.204859
Jucaite, A., Öhd, J., Potter, A. S., Jaeger, J., Karlsson, P., Hannesdottir, K., et al. (2014). A randomized, double-blind, placebo-controlled crossover study of α4β 2* nicotinic acetylcholine receptor agonist AZD1446 (TC-6683) in adults with attention-deficit/hyperactivity disorder. Psychopharmacol. Berl. 231, 1251–1265. doi:10.1007/S00213-013-3116-7
Kadir, L. A., Stacey, M., and Barrett-Jolley, R. (2018). Emerging roles of the membrane potential: Action beyond the action potential. Front. Physiol. 9, 1661. doi:10.3389/fphys.2018.01661
Kantrowitz, J. T., Javitt, D. C., Freedman, R., Sehatpour, P., Kegeles, L. S., Carlson, M., et al. (2020). Double blind, two dose, randomized, placebo-controlled, cross-over clinical trial of the positive allosteric modulator at the alpha7 nicotinic cholinergic receptor AVL-3288 in schizophrenia patients. Neuropsychopharmacology 45, 1339–1345. doi:10.1038/S41386-020-0628-9
Kawashima, K., Yoshikawa, K., Fujii, Y. X., Moriwaki, Y., and Misawa, H. (2007). Expression and function of genes encoding cholinergic components in murine immune cells. Life Sci. 80, 2314–2319. doi:10.1016/J.LFS.2007.02.036
Keefe, R. S. E., Meltzer, H. A., Dgetluck, N., Gawryl, M., Koenig, G., Moebius, H. J., et al. (2015). Randomized, double-blind, placebo-controlled study of encenicline, an α7 nicotinic acetylcholine receptor agonist, as a treatment for cognitive impairment in schizophrenia. Neuropsychopharmacology 40, 3053–3060. doi:10.1038/NPP.2015.176
Kellum, J. A., Song, M., and Li, J. (2004). Science review: Extracellular acidosis and the immune response: Clinical and physiologic implications. Crit. Care 8, 331–336. doi:10.1186/CC2900
Kessler, S., Clauss, W., Günther, A., Kummer, W., and Fronius, M. (2011). Expression and functional characterization of P2X receptors in mouse alveolar macrophages. Pflugers Arch. 462, 419–430. doi:10.1007/S00424-011-0980-Z
Keystone, E. C., Wang, M. M., Layton, M., Hollis, S., and McInnes, I. B. (2012). Clinical evaluation of the efficacy of the P2X7 purinergic receptor antagonist AZD9056 on the signs and symptoms of rheumatoid arthritis in patients with active disease despite treatment with methotrexate or sulphasalazine. Ann. Rheum. Dis. 71, 1630–1635. doi:10.1136/ANNRHEUMDIS-2011-143578
Khalil, M., Babes, A., Lakra, R., Försch, S., Reeh, P., Wirtz, S., et al. (2016). Transient receptor potential melastatin 8 ion channel in macrophages modulates colitis through a balance-shift in TNF-alpha and interleukin-10 production. Mucosal Immunol. 9, 1500–1513. doi:10.1038/MI.2016.16
Kidney, J. C., Fuller, R. W., Worsdell, Y. M., Lavender, E. A., Chung, K. F., and Barnes, P. J. (1993). Effect of an oral potassium channel activator, BRL 38227, on airway function and responsiveness in asthmatic patients: Comparison with oral salbutamol. Thorax 48, 130–133. doi:10.1136/THX.48.2.130
Kiguchi, N., Kobayashi, D., Saika, F., Matsuzaki, S., and Kishioka, S. (2018). Inhibition of peripheral macrophages by nicotinic acetylcholine receptor agonists suppresses spinal microglial activation and neuropathic pain in mice with peripheral nerve injury. J. Neuroinflammation 15 (1 15), 96–13. doi:10.1186/S12974-018-1133-5
Kiguchi, N., Kobayashi, Y., Maeda, T., Tominaga, S., Nakamura, J., Fukazawa, Y., et al. (2012). Activation of nicotinic acetylcholine receptors on bone marrow-derived cells relieves neuropathic pain accompanied by peripheral neuroinflammation. Neurochem. Int. 61, 1212–1219. doi:10.1016/J.NEUINT.2012.09.001
Kim, H., Soyombo, A., Tjon-Kon-Sang, S., So, I., and Muallem, S. (2009). The Ca(2+) channel TRPML3 regulates membrane trafficking and autophagy. Traffic 10, 1157–1167. doi:10.1111/J.1600-0854.2009.00924.X
Kim, J. K., Kim, Y. S., Lee, H.-M., Jin, H. S., Neupane, C., Kim, S., et al. (2018). GABAergic signaling linked to autophagy enhances host protection against intracellular bacterial infections. Nat. Commun. 9, 4184. doi:10.1038/s41467-018-06487-5
Kitagawa, H., Takenouchi, T., Azuma, R., Wesnes, K. A., Kramer, W. G., Clody, D. E., et al. (2003). Safety, pharmacokinetics, and effects on cognitive function of multiple doses of GTS-21 in healthy, male volunteers. Neuropsychopharmacology 28, 542–551. doi:10.1038/SJ.NPP.1300028
Koivisto, A. P., Belvisi, M. G., Gaudet, R., and Szallasi, A. (2022). Advances in TRP channel drug discovery: From target validation to clinical studies. Nat. Rev. Drug Discov. 21, 41–59. doi:10.1038/S41573-021-00268-4
Kong, X., Tang, X., Du, W., Tong, J., Yan, Y., Zheng, F., et al. (2013). Extracellular acidosis modulates the endocytosis and maturation of macrophages. Cell. Immunol. 281, 44–50. doi:10.1016/J.CELLIMM.2012.12.009
Kuebler, W. M., Jordt, S. E., and Liedtke, W. B. (2020). Urgent reconsideration of lung edema as a preventable outcome in COVID-19: Inhibition of TRPV4 represents a promising and feasible approach. Am. J. Physiology - Lung Cell. Mol. Physiology 318, L1239–L1243. doi:10.1152/AJPLUNG.00161.2020/ASSET/IMAGES/LARGE/ZH50062078670002.JPEG
Kun, J., Szitter, I., Kemény, A., Perkecz, A., Kereskai, L., Pohóczky, K., et al. (2014). Upregulation of the transient receptor potential ankyrin 1 ion channel in the inflamed human and mouse colon and its protective roles. PLoS One 9, e108164. doi:10.1371/JOURNAL.PONE.0108164
Kushnarev, M., Pirvulescu, I. P., Candido, K. D., and Knezevic, N. N. (2020). Neuropathic pain: Preclinical and early clinical progress with voltage-gated sodium channel blockers. Expert Opin. Investig. Drugs 29, 259–271. doi:10.1080/13543784.2020.1728254
Kwak, B. R., Mulhaupt, F., Veillard, N., Gros, D. B., and Mach, F. (2002). Altered pattern of vascular connexin expression in atherosclerotic plaques. Arterioscler. Thromb. Vasc. Biol. 22, 225–230. doi:10.1161/HQ0102.104125
Lang, N. N., Myles, R. C., Burton, F. L., Hall, D. P., Chin, Y. Z., Boon, N. A., et al. (2008). The vascular effects of rotigaptide in vivo in man. Biochem. Pharmacol. 76, 1194–1200. doi:10.1016/J.BCP.2008.08.022
Layhadi, J., Turner, J., Crossman, D., and Fountain, S. (2018). ATP evokes Ca 2+ responses and CXCL5 secretion via P2X 4 receptor activation in human monocyte-derived macrophages. J. Immunol. 200, 1159–1168. doi:10.4049/JIMMUNOL.1700965
Lee, Y. W., Won, C. H., Jung, K., Nam, H. J., Choi, G., Park, Y. H., et al. (2019). Efficacy and safety of PAC-14028 cream - a novel, topical, nonsteroidal, selective TRPV1 antagonist in patients with mild-to-moderate atopic dermatitis: A phase IIb randomized trial. Br. J. Dermatol. 180, 1030–1038. doi:10.1111/BJD.17455
Levy, J. A., Weiss, R. M., Dirksen, E. R., and Rosen, M. R. (1976). Possible communication between murine macrophages oriented in linear chains in tissue culture. Exp. Cell Res. 103, 375–385. doi:10.1016/0014-4827(76)90273-1
Li, B., Jie, W., Huang, L., Wei, P., Li, S., Luo, Z., et al. (2014). Nuclear BK channels regulate gene expression via the control of nuclear calcium signaling. Nat. Neurosci. 17, 1055–1063. doi:10.1038/nn.3744
Li, C., Chen, M., He, X., and Ouyang, D. (2021). A mini-review on ion fluxes that regulate NLRP3 inflammasome activation. Acta Biochim. Biophys. Sin. 53, 131–139. doi:10.1093/ABBS/GMAA155
Li, C., Levin, M., and Kaplan, D. L. (2016). Bioelectric modulation of macrophage polarization. Sci. Rep. 6, 21044. doi:10.1038/srep21044
Liedtke, W. (2006). Transient receptor potential vanilloid channels functioning in transduction of osmotic stimuli. J. Endocrinol. 191, 515–523. doi:10.1677/JOE.1.07000
Ling, M. Y., Ma, Z. Y., Wang, Y. Y., Qi, J., Liu, L., Li, L., et al. (2013). Up-regulated ATP-sensitive potassium channels play a role in increased inflammation and plaque vulnerability in macrophages. Atherosclerosis 226, 348–355. doi:10.1016/J.ATHEROSCLEROSIS.2012.11.016
Link, T., Park, U., Vonakis, B., Raben, D., Soloski, M., and Caterina, M. (2010). TRPV2 has a pivotal role in macrophage particle binding and phagocytosis. Nat. Immunol. 11, 232–239. doi:10.1038/NI.1842
Lipovsek, M., Bardy, C., Cadwell, C. R., Hadley, K., Kobak, D., and Tripathy, S. J. (2021). Patch-seq: Past, present, and future. J. Neurosci. 41, 937–946. doi:10.1523/JNEUROSCI.1653-20.2020
Liu, F., Xu, L., Nishi, M., Ichimura, A., and Takeshima, H. (2021). Enhanced Ca2+ handling in thioglycolate-elicited peritoneal macrophages. Cell Calcium 96, 102381. doi:10.1016/J.CECA.2021.102381
Liu, Q., Zhu, P., Liu, S., Tang, M., Wang, Y., Tian, Y., et al. (2019). NMAAP1 maintains M1 phenotype in macrophages through binding to IP3R and activating calcium-related signaling pathways. Protein Pept. Lett. 26, 751–757. doi:10.2174/0929866526666190503105343
Longman, S. D., and Hamilton, T. C. (1992). potassium channel activator drugs: Mechanism of action, pharmacological properties, and therapeutic potential. Med. Res. Rev. 12, 73–148. doi:10.1002/MED.2610120202
López-Muñoz, F., Ucha-Udabe, R., and Alamo, C. (2005). The history of barbiturates a century after their clinical introduction. Neuropsychiatr. Dis. Treat. 1, 329–343. Available at:/pmc/articles/PMC2424120/(Accessed August 9, 2022).
Lu, Y., Wang, X. M., Yang, P., Han, L., Wang, Y. Z., Zheng, Z. H., et al. (2018). Effect of gap junctions on RAW264.7 macrophages infected with H37Rv. Med. (United States) 97, e12125. doi:10.1097/MD.0000000000012125
Lv, Z., Xu, X., Sun, Z., Yang, Y. X., Guo, H., Li, J., et al. (2021). TRPV1 alleviates osteoarthritis by inhibiting M1 macrophage polarization via Ca2+/CaMKII/Nrf2 signaling pathway. Cell Death Dis. 12, 504. doi:10.1038/S41419-021-03792-8
Ma, S., Dubin, A. E., Zhang, Y., Mousavi, S. A. R., Wang, Y., Coombs, A. M., et al. (2021). A role of PIEZO1 in iron metabolism in mice and humans. Cell 184, 969–982. doi:10.1016/J.CELL.2021.01.024
Ma, S., and Wang, D. H. (2021). Knockout of Trpa1 exacerbates renal ischemia-reperfusion injury with classical activation of macrophages. Am. J. Hypertens. 34, 110–116. doi:10.1093/AJH/HPAA162
Mackenzie, A., Chirakkal, H., and North, R. (2003). Kv1.3 potassium channels in human alveolar macrophages. Am. J. Physiol. Lung Cell. Mol. Physiol. 285, L862–L868. doi:10.1152/AJPLUNG.00095.2003
MacKenzie, A., Wilson, H., Kiss-Toth, E., Dower, S., North, R., and Surprenant, A. (2001). Rapid secretion of interleukin-1beta by microvesicle shedding. Immunity 15, 825–835. doi:10.1016/S1074-7613(01)00229-1
Maehara, T., Matsumoto, K., Horiguchi, K., Kondo, M., Iino, S., Horie, S., et al. (2015). Therapeutic action of 5-HT3 receptor antagonists targeting peritoneal macrophages in post-operative ileus. Br. J. Pharmacol. 172, 1136–1147. doi:10.1111/BPH.13006
Majno, G., and Palade, G. E. (1961). Studies on inflammation. 1. The effect of histamine and serotonin on vascular permeability: An electron microscopic study. J. Biophys. Biochem. Cytol. 11, 571–605. doi:10.1083/JCB.11.3.571
Malik, M., Jividen, K., Padmakumar, V. C., Cataisson, C., Li, L., Lee, J., et al. (2012). Inducible NOS-induced chloride intracellular channel 4 (CLIC4) nuclear translocation regulates macrophage deactivation. Proc. Natl. Acad. Sci. U. S. A. 109, 6130–6135. doi:10.1073/PNAS.1201351109
Mariathasan, S., Weiss, D., Newton, K., McBride, J., O’Rourke, K., Roose-Girma, M., et al. (2006). Cryopyrin activates the inflammasome in response to toxins and ATP. Nature 440, 228–232. doi:10.1038/NATURE04515
Masuda, T., Iwamoto, S., Yoshinaga, R., Tozaki-Saitoh, H., Nishiyama, A., Mak, T., et al. (2014). Transcription factor IRF5 drives P2X4R+-reactive microglia gating neuropathic pain. Nat. Commun. 5, 3771. doi:10.1038/NCOMMS4771
Mauler, F., Hinz, V., Horváth, E., Schuhmacher, J., Hofmann, H., Wirtz, S., et al. (2004). Selective intermediate-/small-conductance calcium-activated potassium channel (KCNN4) blockers are potent and effective therapeutics in experimental brain oedema and traumatic brain injury caused by acute subdural haematoma. Eur. J. Neurosci. 20, 1761–1768. doi:10.1111/J.1460-9568.2004.03615.X
McLeod, J. F., Leempoels, J. M., Peng, S. X., Dax, S. L., Myers, L. J., and Golder, F. J. (2014). GAL-021, a new intravenous BKCa-channel blocker, is well tolerated and stimulates ventilation in healthy volunteers. Br. J. Anaesth. 113, 875–883. doi:10.1093/BJA/AEU182
McNeil, P., Swanson, J., Wright, S., Silverstein, S., and Taylor, D. (1986). Fc-receptor-mediated phagocytosis occurs in macrophages without an increase in average [Ca++]i. J. Cell Biol. 102, 1586–1592. doi:10.1083/JCB.102.5.1586
Miller, F., Björnsson, M., Svensson, O., and Karlsten, R. (2014). Experiences with an adaptive design for a dose-finding study in patients with osteoarthritis. Contemp. Clin. Trials 37, 189–199. doi:10.1016/J.CCT.2013.12.007
Montero, M. C., Campo, M., Bono, M., Simon, M. V., Guerrero, J., and Lagos, N. (2020). Neosaxitoxin inhibits the expression of inflammation markers of the M1 phenotype in macrophages. Mar. Drugs 18, E283. doi:10.3390/MD18060283
Montgomery, J., Ghatnekar, G. S., Grek, C. L., Moyer, K. E., and Gourdie, R. G. (2018). Connexin 43-based therapeutics for dermal wound healing. Int. J. Mol. Sci. 19, E1778. doi:10.3390/IJMS19061778
Moran, M. M., and Szallasi, A. (2018). Targeting nociceptive transient receptor potential channels to treat chronic pain: Current state of the field. Br. J. Pharmacol. 175, 2185–2203. doi:10.1111/BPH.14044
Moran, M. M. (2018). TRP channels as potential drug targets. Annu. Rev. Pharmacol. Toxicol. 58, 309–330. doi:10.1146/ANNUREV-PHARMTOX-010617-052832
Mosser, D. M., Hamidzadeh, K., and Goncalves, R. (2020). Macrophages and the maintenance of homeostasis. Cell. Mol. Immunol. 18, 579–587. doi:10.1038/s41423-020-00541-3
Mukherjee, A., Mukherjee, D. C., Prakash, C., Coutinho, A., Moller, A., Immunol, S. J., et al. (1983). Action potentials in macrophages derived from human monocytes. Science 219 (1979), 991–993. doi:10.1126/SCIENCE.6823563
Muñoz-Planillo, R., Kuffa, P., Martínez-Colón, G., Smith, B., Rajendiran, T., and Núñez, G. (2013). K+ efflux is the common trigger of NLRP3 inflammasome activation by bacterial toxins and particulate matter. Immunity 38, 1142–1153. doi:10.1016/J.IMMUNI.2013.05.016
Muraki, K., Iwata, Y., Katanosaka, Y., Ito, T., Ohya, S., Shigekawa, M., et al. (2003). TRPV2 is a component of osmotically sensitive cation channels in murine aortic myocytes. Circ. Res. 93, 829–838. doi:10.1161/01.RES.0000097263.10220.0C
Murray, P. J., and Wynn, T. A. (2011). Protective and pathogenic functions of macrophage subsets. Nat. Rev. Immunol. 11, 723–737. doi:10.1038/nri3073
Nagasawa, M., and Kojima, I. (2012). Translocation of calcium-permeable TRPV2 channel to the podosome: Its role in the regulation of podosome assembly. Cell Calcium 51, 186–193. doi:10.1016/J.CECA.2011.12.012
Nagasawa, M., Nakagawa, Y., Tanaka, S., and Kojima, I. (2007). Chemotactic peptide fMetLeuPhe induces translocation of the TRPV2 channel in macrophages. J. Cell. Physiol. 210, 692–702. doi:10.1002/JCP.20883
Naik, S. K., Pattanaik, K., Eich, J., Sparr, V., Hauptmann, M., Kalsdorf, B., et al. (2020). Differential roles of the calcium ion Channel TRPV4 in host responses to Mycobacterium tuberculosis early and late in infection. iScience 23, 101206. doi:10.1016/J.ISCI.2020.101206
Nascimento Da Conceicao, V., Sun, Y., Ramachandran, K., Chauhan, A., Raveendran, A., Venkatesan, M., et al. (2021). Resolving macrophage polarization through distinct Ca2+ entry channel that maintains intracellular signaling and mitochondrial bioenergetics. iScience 24, 103339. doi:10.1016/J.ISCI.2021.103339
Nguyen, T. N., Siddiqui, G., Veldhuis, N. A., and Poole, D. P. (2022). Diverse roles of TRPV4 in macrophages: A need for unbiased profiling. Front. Immunol. 12, 5918. doi:10.3389/fimmu.2021.828115
Ni, L., Fang, P., Hu, Z., Zhou, H., Chen, J., Wang, F., et al. (2018). Identification and function of acid-sensing ion channels in RAW 264.7 macrophage cells. Curr. Med. Sci. 38, 436–442. doi:10.1007/S11596-018-1897-Y
Ninomiya, Y., Tanuma, S., and Tsukimoto, M. (2017). Differences in the effects of four TRPV1 channel antagonists on lipopolysaccharide-induced cytokine production and COX-2 expression in murine macrophages. Biochem. Biophys. Res. Commun. 484, 668–674. doi:10.1016/J.BBRC.2017.01.173
Numata, T., Shimizu, T., and Okada, Y. (2007). TRPM7 is a stretch- and swelling-activated cation channel involved in volume regulation in human epithelial cells. Am. J. Physiol. Cell Physiol. 292, C460–C467. doi:10.1152/AJPCELL.00367.2006
Okada, Y., Reinach, P. S., Shirai, K., Kitano, A., Kao, W. W. Y., Flanders, K. C., et al. (2011). TRPV1 involvement in inflammatory tissue fibrosis in mice. Am. J. Pathol. 178, 2654–2664. doi:10.1016/J.AJPATH.2011.02.043
Olincy, A., Harris, J. G., Johnson, L. L., Pender, V., Kongs, S., Allensworth, D., et al. (2006). Proof-of-concept trial of an alpha7 nicotinic agonist in schizophrenia. Arch. Gen. Psychiatry 63, 630–638. doi:10.1001/ARCHPSYC.63.6.630
Ortega, F. J., Gimeno-Bayon, J., Espinosa-Parrilla, J. F., Carrasco, J. L., Batlle, M., Pugliese, M., et al. (2012). ATP-dependent potassium channel blockade strengthens microglial neuroprotection after hypoxia-ischemia in rats. Exp. Neurol. 235, 282–296. doi:10.1016/J.EXPNEUROL.2012.02.010
Papavlassopoulos, M., Stamme, C., Thon, L., Adam, D., Hillemann, D., Seydel, U., et al. (2006). MaxiK blockade selectively inhibits the lipopolysaccharide-induced i?b-α/NF-κB signaling pathway in macrophages. J. Immunol. 177, 4086–4093. doi:10.4049/JIMMUNOL.177.6.4086
Park, C. W., Kim, B. J., Lee, Y. W., Won, C., Park, C. O., Chung, B. Y., et al. (2022). Asivatrep, a TRPV1 antagonist, for the topical treatment of atopic dermatitis: Phase 3, randomized, vehicle-controlled study (CAPTAIN-AD). J. Allergy Clin. Immunol. 149, 1340–1347.e4. e4. doi:10.1016/J.JACI.2021.09.024
Park, S. A., Lee, Y. C., Ma, T. Z., Park, J. A., Han, M. K., Lee, H. H., et al. (2006). hKv1.5 channels play a pivotal role in the functions of human alveolar macrophages. Biochem. Biophys. Res. Commun. 346, 567–571. doi:10.1016/J.BBRC.2006.05.149
Pelegrin, P., and Surprenant, A. (2006). Pannexin-1 mediates large pore formation and interleukin-1beta release by the ATP-gated P2X7 receptor. EMBO J. 25, 5071–5082. doi:10.1038/SJ.EMBOJ.7601378
Peng, Y., Lu, K., Li, Z., Zhao, Y., Wang, Y., Hu, B., et al. (2014). Blockade of Kv1.3 channels ameliorates radiation-induced brain injury. Neuro. Oncol. 16, 528–539. doi:10.1093/NEUONC/NOT221
Penner, R., and Fleig, A. (2007). The Mg2+ and Mg(2+)-nucleotide-regulated channel-kinase TRPM7. Handb. Exp. Pharmacol. 179, 313–328. doi:10.1007/978-3-540-34891-7_19
Pétrilli, V., Papin, S., Dostert, C., Mayor, A., Martinon, F., and Tschopp, J. (2007). Activation of the NALP3 inflammasome is triggered by low intracellular potassium concentration. Cell Death Differ. 14, 1583–1589. doi:10.1038/SJ.CDD.4402195
Pi, J., Li, T., Liu, J., Su, X., Wang, R., Yang, F., et al. (2014). Detection of lipopolysaccharide induced inflammatory responses in RAW264.7 macrophages using atomic force microscope. Micron 65, 1–9. doi:10.1016/J.MICRON.2014.03.012
Piccini, A., Carta, S., Tassi, S., Lasiglié, D., Fossati, G., and Rubartelli, A. (2008). ATP is released by monocytes stimulated with pathogen-sensing receptor ligands and induces IL-1beta and IL-18 secretion in an autocrine way. Proc. Natl. Acad. Sci. U. S. A. 105, 8067–8072. doi:10.1073/PNAS.0709684105
Plesch, E., Chen, C. C., Butz, E., Rosato, A. S., Krogsaeter, E. K., Yinan, H., et al. (2018). Selective agonist of TRPML2 reveals direct role in chemokine release from innate immune cells. Elife 7, e39720. doi:10.7554/ELIFE.39720
Porvaznik, M., and MacVittie, T. J. (1979). Detection of gap junctions between the progeny of a canine macrophage colony-forming cell in vitro. J. Cell Biol. 82, 555–564. doi:10.1083/JCB.82.2.555
Priest, B. T., and McDermott, J. S. (2015). Cardiac ion channels. Channels 9, 352–359. doi:10.1080/19336950.2015.1076597
Purves, D., Augustine, G. J., Fitzpatrick, D., Katz, L. C., LaMantia, A.-S., McNamara, J. O., et al. (2001). Channels and transporters. Available at: https://www.ncbi.nlm.nih.gov/books/NBK11010/(Accessed May 18, 2021).
Py, B., Jin, M., Desai, B., Penumaka, A., Zhu, H., Kober, M., et al. (2014). Caspase-11 controls interleukin-1β release through degradation of TRPC1. Cell Rep. 6, 1122–1128. doi:10.1016/J.CELREP.2014.02.015
Ramond, E., Jamet, A., Coureuil, M., and Charbit, A. (2019). Pivotal role of mitochondria in macrophage response to bacterial pathogens. Front. Immunol. 01, 2461. doi:10.3389/FIMMU.2019.02461
Ramsey, I. S., Ruchti, E., Kaczmarek, J. S., and Clapham, D. E. (2009). Hv1 proton channels are required for high-level NADPH oxidase-dependent superoxide production during the phagocyte respiratory burst. Proc. Natl. Acad. Sci. U. S. A. 106, 7642–7647. doi:10.1073/PNAS.0902761106
Raza, S., Robertson, K. A., Lacaze, P. A., Page, D., Enright, A. J., Ghazal, P., et al. (2008). A logic-based diagram of signalling pathways central to macrophage activation. BMC Syst. Biol. 2, 36–15. doi:10.1186/1752-0509-2-36
Riazanski, V., Gabdoulkhakova, A., Boynton, L., Eguchi, R., Deriy, L., Hogarth, D., et al. (2015). TRPC6 channel translocation into phagosomal membrane augments phagosomal function. Proc. Natl. Acad. Sci. U. S. A. 112, E6486–E6495. doi:10.1073/PNAS.1518966112
Rodjakovic, D., Salm, L., and Beldi, G. (2021). Function of connexin-43 in macrophages. Int. J. Mol. Sci. 22, 1412–12. doi:10.3390/IJMS22031412
Roozekrans, M., van der Schrier, R., Okkerse, P., Hay, J., McLeod, J. F., and Dahan, A. (2014). Two studies on reversal of opioid-induced respiratory depression by BK-channel blocker GAL021 in human volunteers. Anesthesiology 121, 459–468. doi:10.1097/ALN.0000000000000367
Samie, M., Wang, X., Zhang, X., Goschka, A., Li, X., Cheng, X., et al. (2013). A TRP channel in the lysosome regulates large particle phagocytosis via focal exocytosis. Dev. Cell 26, 511–524. doi:10.1016/J.DEVCEL.2013.08.003
Sampieri, A., Santoyo, K., Asanov, A., and Vaca, L. (2018). Association of the IP3R to STIM1 provides a reduced intraluminal calcium microenvironment, resulting in enhanced store-operated calcium entry. Sci. Rep. 8, 13252. doi:10.1038/s41598-018-31621-0
Sanjai Kumar, P., Nayak, T. K., Mahish, C., Sahoo, S. S., Radhakrishnan, A., De, S., et al. (2021). Inhibition of transient receptor potential vanilloid 1 (TRPV1) channel regulates chikungunya virus infection in macrophages. Arch. Virol. 166, 139–155. doi:10.1007/S00705-020-04852-8
Santoni, G., Cardinali, C., Morelli, M., Santoni, M., Nabissi, M., and Amantini, C. (2015). Danger- and pathogen-associated molecular patterns recognition by pattern-recognition receptors and ion channels of the transient receptor potential family triggers the inflammasome activation in immune cells and sensory neurons. J. Neuroinflammation 12, 21. doi:10.1186/S12974-015-0239-2
Santoni, G., Morelli, M. B., Amantini, C., Nabissi, M., Santoni, M., and Santoni, A. (2020). Involvement of the TRPML mucolipin channels in viral infections and anti-viral innate immune responses. Front. Immunol. 1, 739. doi:10.3389/FIMMU.2020.00739
Santoni, G., Morelli, M. B., Amantini, C., Santoni, M., Nabissi, M., Marinelli, O., et al. (2018). Immuno-transient receptor potential ion channels”: The role in monocyte- and macrophage-mediated inflammatory responses. Front. Immunol. 0, 1273. doi:10.3389/FIMMU.2018.01273
Schein, S. J., Colombini, M., and Finkelstein, A. (1976). Reconstitution in planar lipid bilayers of a voltage-dependent anion-selective channel obtained from paramecium mitochondria. J. Membr. Biol. 30, 99–120. doi:10.1007/BF01869662
Scheraga, R., Abraham, S., Grove, L., Southern, B., Crish, J., Perelas, A., et al. (2020a). TRPV4 protects the lung from bacterial pneumonia via MAPK molecular pathway switching. J. Immunol. 204, 1310–1321. doi:10.4049/JIMMUNOL.1901033
Scheraga, R., Abraham, S., Niese, K., Southern, B., Grove, L., Hite, R., et al. (2016). TRPV4 mechanosensitive ion Channel regulates lipopolysaccharide-stimulated macrophage phagocytosis. J. Immunol. 196, 428–436. doi:10.4049/JIMMUNOL.1501688
Scheraga, R. G., Southern, B. D., Grove, L. M., and Olman, M. A. (2020b). The role of TRPV4 in regulating innate immune cell function in lung inflammation. Front. Immunol. 11, 1211. doi:10.3389/FIMMU.2020.01211
Schilling, T., Miralles, F., and Eder, C. (2014). TRPM7 regulates proliferation and polarisation of macrophages. J. Cell Sci. 127, 4561–4566. doi:10.1242/JCS.151068
Seidel, M. F., Fiebich, B. L., Ulrich-Merzenich, G., Candelario-Jalil, E., Koch, F.-W., and Vetter, H. (2008). Serotonin mediates PGE2 overexpression through 5-HT2A and 5-HT3 receptor subtypes in serum-free tissue culture of macrophage-like synovial cells. Rheumatol. Int. 28 (10 28), 1017–1022. doi:10.1007/S00296-008-0564-1
Selezneva, A., Gibb, A. J., and Willis, D. (2022). The nuclear envelope as a regulator of immune cell function. Front. Immunol. 0, 840069. doi:10.3389/FIMMU.2022.840069
Selezneva, A., Yoshida, M., Gibb, A., and Willis, D. (2021). Nuclear BK channels regulate CREB phosphorylation in RAW264.7 macrophages. Pharmacol. Rep. 73, 881–890. doi:10.1007/s43440-021-00229-z
Serafini, N., Dahdah, A., Barbet, G., Demion, M., Attout, T., Gautier, G., et al. (2012). The TRPM4 channel controls monocyte and macrophage, but not neutrophil, function for survival in sepsis. J. Immunol. 189, 3689–3699. doi:10.4049/JIMMUNOL.1102969
Sharma, D., Tiwari, B. K., Mehto, S., Antony, C., Kak, G., Singh, Y., et al. (2016). Suppression of protective responses upon activation of L-type voltage gated calcium channel in macrophages during Mycobacterium bovis BCG infection. PLOS ONE 11, e0163845. doi:10.1371/JOURNAL.PONE.0163845
Shaw, P., and Feske, S. (2012). Regulation of lymphocyte function by ORAI and STIM proteins in infection and autoimmunity. J. Physiol. 590, 4157–4167. doi:10.1113/JPHYSIOL.2012.233221
Shen, C., Chen, J., Lee, Y., Hassan, M., Kim, S., Choi, E., et al. (2018). mTOR- and SGK-mediated connexin 43 expression participates in lipopolysaccharide-stimulated macrophage migration through the iNOS/src/FAK AxisJ. Immunol, 201. 2986–2997. doi:10.4049/JIMMUNOL.1700954
Shi, S., Pang, C., Guo, S., Chen, Y., Ma, B., Qu, C., et al. (2020). Recent progress in structural studies on TMEM16A channel. Comput. Struct. Biotechnol. J. 18, 714–722. doi:10.1016/J.CSBJ.2020.03.015
Shim, A. H. R., Tirado-Lee, L., and Prakriya, M. (2015). Structural and functional mechanisms of CRAC channel regulation. J. Mol. Biol. 427, 77–93. doi:10.1016/J.JMB.2014.09.021
Shiratsuchi, H., Kouatli, Y., Yu, G. X., Marsh, H. M., and Basson, M. D. (2009). Propofol inhibits pressure-stimulated macrophage phagocytosis via the GABAA receptor and dysregulation of p130cas phosphorylation. Am. J. Physiol. Cell Physiol. 296, 1400–1410. doi:10.1152/ajpcell.00345.2008
Shunmugam, S. R., Sugihara, C., Freemantle, N., Round, P., Furniss, S., and Sulke, N. (2018). A double-blind, randomised, placebo-controlled, cross-over study assessing the use of XEN-D0103 in patients with paroxysmal atrial fibrillation and implanted pacemakers allowing continuous beat-to-beat monitoring of drug efficacy. J. Interv. Card. Electrophysiol. 51, 191–197. doi:10.1007/S10840-018-0318-2
Simon-Chica, A., Fernández, M. C., Wülfers, E. M., Lother, A., Hilgendorf, I., Seemann, G., et al. (2021). Novel insights into the electrophysiology of murine cardiac macrophages: Relevance of voltage-gated potassium channels. Cardiovasc. Res. 118, 798–813. doi:10.1093/CVR/CVAB126
Solanki, S., Dube, P., Tano, J., Birnbaumer, L., and Vazquez, G. (2014). Reduced endoplasmic reticulum stress-induced apoptosis and impaired unfolded protein response in TRPC3-deficient M1 macrophages. Am. J. Physiol. Cell Physiol. 307, C521–C531. doi:10.1152/AJPCELL.00369.2013
Solle, M., Labasi, J., Perregaux, D., Stam, E., Petrushova, N., Koller, B., et al. (2001). Altered cytokine production in mice lacking P2X(7) receptors. J. Biol. Chem. 276, 125–132. doi:10.1074/JBC.M006781200
Spix, B., Butz, E. S., Chen, C. C., Rosato, A. S., Tang, R., Jeridi, A., et al. (2022). Lung emphysema and impaired macrophage elastase clearance in mucolipin 3 deficient mice. Nat. Commun. 13 (1 13), 318–18. doi:10.1038/s41467-021-27860-x
Staats, K. A., Humblet-Baron, S., Bento-Abreu, A., Scheveneels, W., Nikolaou, A., Deckers, K., et al. (2016). Genetic ablation of IP 3 receptor 2 increases cytokines and decreases survival of SOD1 G93A mice. Hum. Mol. Genet. 25, 3491–3499. doi:10.1093/hmg/ddw190
Ständer, S., Augustin, M., Roggenkamp, D., Blome, C., Heitkemper, T., Worthmann, A. C., et al. (2017). Novel TRPM8 agonist cooling compound against chronic itch: Results from a randomized, double-blind, controlled, pilot study in dry skin. J. Eur. Acad. Dermatol. Venereol. 31, 1064–1068. doi:10.1111/JDV.14041
Stewart, G. M., Johnson, B. D., Sprecher, D. L., Reddy, Y. N. V., Obokata, M., Goldsmith, S., et al. (2020). Targeting pulmonary capillary permeability to reduce lung congestion in heart failure: A randomized, controlled pilot trial. Eur. J. Heart Fail. 22, 1641–1645. doi:10.1002/EJHF.1809
Stock, T. C., Bloom, B. J., Wei, N., Ishaq, S., Park, W., Wang, X., et al. (2012). Efficacy and safety of CE-224, 535, an antagonist of P2X7 receptor, in treatment of patients with rheumatoid arthritis inadequately controlled by methotrexate. J. Rheumatol. 39, 720–727. doi:10.3899/JRHEUM.110874
Strøbæk, D., Brown, D., Jenkins, D., Chen, Y.-J., Coleman, N., Ando, Y., et al. (2013). NS6180, a new KCa3.1 channel inhibitor prevents T-cell activation and inflammation in a rat model of inflammatory bowel disease. Br. J. Pharmacol. 168, 432–444. doi:10.1111/J.1476-5381.2012.02143.X
Suadicani, S., Brosnan, C., and Scemes, E. (2006). P2X7 receptors mediate ATP release and amplification of astrocytic intercellular Ca2+ signaling. J. Neurosci. 26, 1378–1385. doi:10.1523/JNEUROSCI.3902-05.2006
Sui, J., Zhang, C., Fang, X., Wang, J., Li, Y., Wang, J., et al. (2020). Dual role of Ca2+-activated Cl− channel transmembrane member 16A in lipopolysaccharide-induced intestinal epithelial barrier dysfunction in vitro. Cell Death Dis. 11 (5 11), 404–412. doi:10.1038/s41419-020-2614-x
Sun, H., Jiang, J., Gong, L., Li, X., Yang, Y., Luo, Y., et al. (2019). Voltage-gated sodium channel inhibitor reduces atherosclerosis by modulating monocyte/macrophage subsets and suppressing macrophage proliferation. Biomed. Pharmacother. 120, 109352. doi:10.1016/J.BIOPHA.2019.109352
Sun, L., Hua, Y., Vergarajauregui, S., Diab, H. I., and Puertollano, R. (2015). Novel role of TRPML2 in the regulation of the innate immune response. J. Immunol. 195, 4922–4932. doi:10.4049/JIMMUNOL.1500163
Sun, X., Xu, M., Cao, Q., Huang, P., Zhu, X., and Dong, X.-P. (2020). A lysosomal K+ channel regulates large particle phagocytosis by facilitating lysosome Ca2+ release. Sci. Rep. 10, 1038. doi:10.1038/s41598-020-57874-2
Tano, J., Solanki, S., Lee, R., Smedlund, K., Birnbaumer, L., and Vazquez, G. (2014). Bone marrow deficiency of TRPC3 channel reduces early lesion burden and necrotic core of advanced plaques in a mouse model of atherosclerosis. Cardiovasc. Res. 101, 138–144. doi:10.1093/CVR/CVT231
Tarcha, E. J., Olsen, C. M., Probst, P., Peckham, D., Muñoz-Elías, E. J., Kruger, J. G., et al. (2017). Safety and pharmacodynamics of dalazatide, a Kv1.3 channel inhibitor, in the treatment of plaque psoriasis: A randomized phase 1b trial. PLoS One 12, e0180762. doi:10.1371/JOURNAL.PONE.0180762
Thompson, A. J. (2013). Recent developments in 5-HT3 receptor pharmacology. Trends Pharmacol. Sci. 34, 100–109. doi:10.1016/J.TIPS.2012.12.002
Timmers, M., Ravenstijn, P., Xi, L., Triana-Baltzer, G., Furey, M., van Hemelryck, S., et al. (2018). Clinical pharmacokinetics, pharmacodynamics, safety, and tolerability of JNJ-54175446, a brain permeable P2X7 antagonist, in a randomised single-ascending dose study in healthy participants. J. Psychopharmacol. 32, 1341–1350. doi:10.1177/0269881118800067
Toyama, K., Wulff, H., Chandy, K., Azam, P., Raman, G., Saito, T., et al. (2008). The intermediate-conductance calcium-activated potassium channel KCa3.1 contributes to atherogenesis in mice and humans. J. Clin. Invest. 118, 3025–3037. doi:10.1172/JCI30836
Tsai, K., Chang, H., and Wu, S. (2013). The inhibition of inwardly rectifying K+ channels by memantine in macrophages and microglial cells. Cell. Physiol. biochem. 31, 938–951. doi:10.1159/000350112
Tsoyi, K., Jang, H. J., Kim, J. W., Chang, H. K., Lee, Y. S., Pae, H. O., et al. (2011). Stimulation of Alpha7 nicotinic acetylcholine receptor by nicotine attenuates inflammatory response in macrophages and improves survival in experimental model of sepsis through heme oxygenase-1 induction. Antioxid. Redox Signal. 14, 2057–2070. doi:10.1089/ARS.2010.3555
Ulmann, L., Hirbec, H., and Rassendren, F. (2010). P2X4 receptors mediate PGE2 release by tissue-resident macrophages and initiate inflammatory pain. EMBO J. 29, 2290–2300. doi:10.1038/EMBOJ.2010.126
Umar Shinge, S. A., Zhang, D., Din, A. U., Yu, F., and Nie, Y. M. (2022). Emerging Piezo1 signaling in inflammation and atherosclerosis; a potential therapeutic target. Int. J. Biol. Sci. 18, 923–941. doi:10.7150/IJBS.63819
Vaeth, M., Zee, I., Concepcion, A. R., Maus, M., Shaw, P., Portal-Celhay, C., et al. (2015). Ca2+ signaling but not store-operated Ca2+ entry is required for the function of macrophages and dendritic cells. J. Immunol. 195, 1202–1217. doi:10.4049/JIMMUNOL.1403013
Vaiciuleviciute, R., Kalvaityte, U., Bernotiene, E., and Mobasheri, A. (2021). ion Channel modulators for treatment-resistant rheumatoid arthritis: Focus on inflammation. BIOELECTRICITY 3, 243–248. doi:10.1089/bioe.2021.0038
Valle, B. K., and Lemberg, L. (1991). Update on thrombolytic therapy in acute myocardial infarction. Heart Lung. 20, 526–530. Available at: https://europepmc.org/article/MED/1819251 (Accessed August 9, 2022).
Vazquez, G., Solanki, S., Dube, P., Smedlund, K., and Ampem, P. (2016). On the roles of the transient receptor potential canonical 3 (TRPC3) channel in endothelium and macrophages: Implications in atherosclerosis. Adv. Exp. Med. Biol. 898, 185–199. doi:10.1007/978-3-319-26974-0_9
Venkatachalam, K., and Montell, C. (2007). TRP channels. Annu. Rev. Biochem. 76, 387–417. doi:10.1146/ANNUREV.BIOCHEM.75.103004.142819
Vernon-Wilson, E. F., Dé Ric Auradé, F., Tian, L., Rowe, I. C. M., Shipston, M. J., Savill, J., et al. (2007). CD31 delays phagocyte membrane repolarization to promote efficient binding of apoptotic cells. J. Leukoc. Biol. 82, 1278–1288. doi:10.1189/jlb.0507283
Vicente, R., Escalada, A., Coma, M., Fuster, G., Sánchez-Tilló, E., López-Iglesias, C., et al. (2003). Differential voltage-dependent K+ channel responses during proliferation and activation in macrophages. J. Biol. Chem. 278, 46307–46320. doi:10.1074/JBC.M304388200
Vicente, R., Escalada, A., Villalonga, N., Texido, L., Roura-Ferrer, M., Martín-Satué, M., et al. (2006). Association of Kv1.5 and Kv1.3 contributes to the major voltage-dependent K+ channel in macrophages. J. Biol. Chem. 281, 37675–37685. doi:10.1074/JBC.M605617200
Vicente, R., Villalonga, N., Calvo, M., Escalada, A., Solsona, C., Soler, C., et al. (2008). Kv1.5 association modifies Kv1.3 traffic and membrane localization. J. Biol. Chem. 283, 8756–8764. doi:10.1074/JBC.M708223200
Villalonga, N., David, M., Biejanska, J., Vicente, R., Comes, N., Valenzuela, C., et al. (2010). Immunomodulation of voltage-dependent K+ channels in macrophages: Molecular and biophysical consequences. J. Gen. Physiol. 135, 135–147. doi:10.1085/JGP.200910334
Villalonga, N., Escalada, A., Vicente, R., Sánchez-Tilló, E., Celada, A., Solsona, C., et al. (2007). Kv1.3/Kv1.5 heteromeric channels compromise pharmacological responses in macrophages. Biochem. Biophys. Res. Commun. 352, 913–918. doi:10.1016/J.BBRC.2006.11.120
Walker, J. R., Novick, P. A., Parsons, W. H., McGregor, M., Zablocki, J., Pande, V. S., et al. (2012). Marked difference in saxitoxin and tetrodotoxin affinity for the human nociceptive voltage-gated sodium channel (Nav1.7) [corrected]. Proc. Natl. Acad. Sci. U. S. A. 109, 18102–18107. doi:10.1073/PNAS.1206952109
Wang, H., Yu, M., Ochani, M., Amella, C. A., Tanovic, M., Susarla, S., et al. (2002). Nicotinic acetylcholine receptor alpha7 subunit is an essential regulator of inflammation. Nature 421, 384–388. doi:10.1038/nature01339
Wang, Q., Chen, K., Zhang, F., Peng, K., Wang, Z., Yang, D., et al. (2020). TRPA1 regulates macrophages phenotype plasticity and atherosclerosis progression. Atherosclerosis 301, 44–53. doi:10.1016/J.ATHEROSCLEROSIS.2020.04.004
Wang, Y., Li, N., Zhang, X., and Horng, T. (2021). Mitochondrial metabolism regulates macrophage biology. J. Biol. Chem. 297, 100904. doi:10.1016/J.JBC.2021.100904
Westphalen, K., Gusarova, G. A., Islam, M. N., Subramanian, M., Cohen, T. S., Prince, A. S., et al. (2014). Sessile alveolar macrophages communicate with alveolar epithelium to modulate immunity. Nature 506 (506), 7489503–7489506. doi:10.1038/nature12902
Williams, A. J., Lee, T. H., Vyse, T., Chiew, F., Cochrane, G. M., Williams, A. J., et al. (1990). Attenuation of nocturnal asthma by cromakalim. Lancet 336, 334–336. doi:10.1016/0140-6736(90)91877-D
Winchester, W. J., Gore, K., Glatt, S., Petit, W., Gardiner, J. C., Conlon, K., et al. (2014). Inhibition of TRPM8 channels reduces pain in the cold pressor test in humans. J. Pharmacol. Exp. Ther. 351, 259–269. doi:10.1124/JPET.114.216010
Wu, L., Wu, G., Akhavan Sharif, M., Baker, A., Jia, Y., Fahey, F., et al. (2012). The voltage-gated proton channel Hv1 enhances brain damage from ischemic stroke. Nat. Neurosci. 15, 565–573. doi:10.1038/NN.3059
Wu, X., Lv, J., Zhang, S., Yi, X., Xu, Z., Zhi, Y., et al. (2021). ML365 inhibits TWIK2 channel to block ATP-induced NLRP3 inflammasome. Acta Pharmacol. Sin. 2021, 992–1000. doi:10.1038/s41401-021-00739-9
Wynn, T. A., Chawla, A., and Pollard, J. W. (2013). Macrophage biology in development, homeostasis and disease. Nature 496, 445–455. doi:10.1038/nature12034
Xu, R., Li, C., Wu, Y., Shen, L., Ma, J., Qian, J., et al. (2017). Role of KCa3.1 channels in macrophage polarization and its relevance in atherosclerotic plaque instability. Arterioscler. Thromb. Vasc. Biol. 37, 226–236. doi:10.1161/ATVBAHA.116.308461
Xu, S., Liu, B., Yin, M., Koroleva, M., Mastrangelo, M., Ture, S., et al. (2016). A novel TRPV4-specific agonist inhibits monocyte adhesion and atherosclerosis. Oncotarget 7, 37622–37635. doi:10.18632/ONCOTARGET.9376
Yamamoto, S., Shimizu, S., Kiyonaka, S., Takahashi, N., Wajima, T., Hara, Y., et al. (2008). TRPM2-mediated Ca2+influx induces chemokine production in monocytes that aggravates inflammatory neutrophil infiltration. Nat. Med. 14, 738–747. doi:10.1038/NM1758
Yamashiro, K., Sasano, T., Tojo, K., Namekata, I., Kurokawa, J., Sawada, N., et al. (2010). Role of transient receptor potential vanilloid 2 in LPS-induced cytokine production in macrophages. Biochem. Biophys. Res. Commun. 398, 284–289. doi:10.1016/J.BBRC.2010.06.082
Yang, J. M., Li, F., Liu, Q., Rüedi, M., Wei, E. T., Lentsman, M., et al. (2017). A novel TRPM8 agonist relieves dry eye discomfort. BMC Ophthalmol. 17, 101. doi:10.1186/S12886-017-0495-2
Yang, X., Wang, G., Cao, T., Zhang, L., Ma, Y., Jiang, S., et al. (2019). Large-conductance calcium-activated potassium channels mediate lipopolysaccharide-induced activation of murine microglia. J. Biol. Chem. 294, 12921–12932. doi:10.1074/jbc.RA118.006425
Yoshida, M. (2017). Role of BK channels in macrophage activation. PhD thesis. London: University College London.
Yoshikawa, H., Kurokawa, M., Ozaki, N., Nara, K., Atou, K., Takada, E., et al. (2006). Nicotine inhibits the production of proinflammatory mediators in human monocytes by suppression of I-kappaB phosphorylation and nuclear factor-kappaB transcriptional activity through nicotinic acetylcholine receptor alpha7. Clin. Exp. Immunol. 146, 116–123. doi:10.1111/J.1365-2249.2006.03169.X
Zamponi, G. W., Striessnig, J., Koschak, A., and Dolphin, A. C. (2015). The physiology, pathology, and pharmacology of voltage-gated calcium channels and their future therapeutic potential. Pharmacol. Rev. 67, 821–870. doi:10.1124/PR.114.009654
Zhang, B., Vogelzang, A., Miyajima, M., Sugiura, Y., Wu, Y., Chamoto, K., et al. (2021). B cell-derived GABA elicits IL-10+ macrophages to limit anti-tumour immunity. Nature 599 (7885 599), 471–476. doi:10.1038/s41586-021-04082-1
Zhang, J., Shipston, M. J., and Brown, S. B. (2010). A role for potassium permeability in the recognition, clearance, and anti-inflammatory effects of apoptotic cells. Mol. Neurobiol. 42, 17–24. doi:10.1007/s12035-010-8127-3
Zhen, J., Chen, W., Zhao, L., Zang, X., and Liu, Y. (2019). A negative Smad2/miR-9/ANO1 regulatory loop is responsible for LPS-induced sepsis. Biomed. Pharmacother. 116, 109016. doi:10.1016/J.BIOPHA.2019.109016
Zheng, F., Tao, Y., Liu, J., Geng, Z., Wang, Y., Wang, Y., et al. (2021). KCa3.1 inhibition of macrophages suppresses inflammatory response leading to endothelial damage in a cell model of kawasaki disease. J. Inflamm. Res. 14, 719–735. doi:10.2147/JIR.S297131
Zhong, Z., Zhai, Y., Liang, S., Mori, Y., Han, R., Sutterwala, F., et al. (2013). TRPM2 links oxidative stress to NLRP3 inflammasome activation. Nat. Commun. 4, 1611. doi:10.1038/NCOMMS2608
Zhou, X., Ye, Y., Sun, Y., Li, X., Wang, W., Privratsky, B., et al. (2015a). Transient receptor potential channel 1 deficiency impairs host defense and proinflammatory responses to bacterial infection by regulating protein kinase cα signaling. Mol. Cell. Biol. 35, 2729–2739. doi:10.1128/MCB.00256-15
Zhou, Y., Wong, C. O., Cho, K. J., van der Hoeven, D., Liang, H., Thakur, D. P., et al. (2015b). SIGNAL TRANSDUCTION. Membrane potential modulates plasma membrane phospholipid dynamics and K-Ras signaling. Science 349, 873–876. doi:10.1126/SCIENCE.AAA5619
Zhu, H., Wang, Y., He, Y., and Yu, W. (2022). Inflammation-mediated macrophage polarization induces TRPV1/TRPA1 heteromers in endometriosis. Am. J. Transl. Res. 14, 3066–3078. Available at: https://pmc/articles/PMC9185066/ (Accessed August 2, 2022).
Keywords: macrophage, ion channels, immune signalling, innate immunity, membrane potential (ΔΨ), inflammation
Citation: Selezneva A, Gibb AJ and Willis D (2022) The contribution of ion channels to shaping macrophage behaviour. Front. Pharmacol. 13:970234. doi: 10.3389/fphar.2022.970234
Received: 15 June 2022; Accepted: 15 August 2022;
Published: 07 September 2022.
Edited by:
Daniel Yakubovich, Ariel University, IsraelReviewed by:
Marina Quartu, University of Cagliari, ItalyRishov Goswami, Dana–Farber Cancer Institute, United States
Copyright © 2022 Selezneva, Gibb and Willis. This is an open-access article distributed under the terms of the Creative Commons Attribution License (CC BY). The use, distribution or reproduction in other forums is permitted, provided the original author(s) and the copyright owner(s) are credited and that the original publication in this journal is cited, in accordance with accepted academic practice. No use, distribution or reproduction is permitted which does not comply with these terms.
*Correspondence: Dean Willis, ZGVhbi53aWxsaXNAdWNsLmFjLnVr