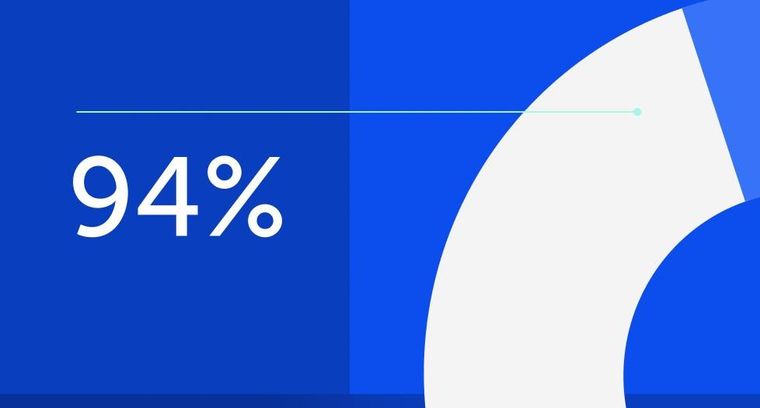
94% of researchers rate our articles as excellent or good
Learn more about the work of our research integrity team to safeguard the quality of each article we publish.
Find out more
ORIGINAL RESEARCH article
Front. Pharmacol., 28 September 2022
Sec. Respiratory Pharmacology
Volume 13 - 2022 | https://doi.org/10.3389/fphar.2022.968378
We are developing a series of thiolesters that produce an immediate and sustained reversal of the deleterious effects of opioids, such as morphine and fentanyl, on ventilation without diminishing the antinociceptive effects of these opioids. We report here the effects of systemic injections of L-cysteine methyl ester (L-CYSme) on morphine-induced changes in ventilatory parameters, arterial-blood gas (ABG) chemistry (pH, pCO2, pO2, sO2), Alveolar-arterial (A-a) gradient (i.e., the index of alveolar gas-exchange within the lungs), and antinociception in unanesthetized Sprague Dawley rats. The administration of morphine (10 mg/kg, IV) produced a series of deleterious effects on ventilatory parameters, including sustained decreases in tidal volume, minute ventilation, inspiratory drive and peak inspiratory flow that were accompanied by a sustained increase in end inspiratory pause. A single injection of L-CYSme (500 μmol/kg, IV) produced a rapid and long-lasting reversal of the deleterious effects of morphine on ventilatory parameters, and a second injection of L-CYSme (500 μmol/kg, IV) elicited pronounced increases in ventilatory parameters, such as minute ventilation, to values well above pre-morphine levels. L-CYSme (250 or 500 μmol/kg, IV) also produced an immediate and sustained reversal of the deleterious effects of morphine (10 mg/kg, IV) on arterial blood pH, pCO2, pO2, sO2 and A-a gradient, whereas L-cysteine (500 μmol/kg, IV) itself was inactive. L-CYSme (500 μmol/kg, IV) did not appear to modulate the sedative effects of morphine as measured by righting reflex times, but did diminish the duration, however, not the magnitude of the antinociceptive actions of morphine (5 or 10 mg/kg, IV) as determined in tail-flick latency and hindpaw-withdrawal latency assays. These findings provide evidence that L-CYSme can powerfully overcome the deleterious effects of morphine on breathing and gas-exchange in Sprague Dawley rats while not affecting the sedative or early stage antinociceptive effects of the opioid. The mechanisms by which L-CYSme interferes with the OR-induced signaling pathways that mediate the deleterious effects of morphine on ventilatory performance, and by which L-CYSme diminishes the late stage antinociceptive action of morphine remain to be determined.
The clinical usefulness of opioids analgesics, such as morphine and fentanyl, is compromised by their deleterious effects on breathing and alveolar gas exchange (van Dorp et al., 2007; Dahan et al., 2010; Dahan et al., 2018; Boom et al., 2012; Algera et al., 2019; Arendt, 2021). Opioid-induced respiratory depression (OIRD) can be overcome by administration of opioid receptor (OR) antagonists, such as naloxone, but these antagonists also block the analgesic and sedative actions of opioids, which may not be an issue in cases involving unexpected overdose, but which would be highly problematic in situations requiring analgesia/sedation, such as during and after surgery (Dahan et al., 2010; Dahan et al., 2018; Boom et al., 2012). Numerous classes of non-OR antagonist drugs have been proposed to combat OIRD (Dahan et al., 2010; Boom et al., 2012; van der Schier et al., 2014; Dahan et al., 2018; Algera et al., 2019; Imam et al., 2020). These drugs, many listed in Supplementary Table S1, include K + -channel blockers (Sia and Zandstra 1981), α2-adrenoceptor antagonists (Vonhof and Sirén, 1991), acetylcholinesterase inhibitors (Elmalem et al., 1991; Tsujita et al., 2007; Sakuraba et al., 2009), adenylate cyclase activators (Ballanyi et al., 1997), dopamine D1 receptor agonists (Ballanyi et al., 1997; Lalley, 2004; Lalley, 2005), phosphodiesterase inhibitors (Kasaba et al., 1997, Kimura et al., 2015), glycyl-glutamine (Owen et al., 2000), 5-HT1A-, 5-HT1A-/5-HT7- and 5-HT4-receptor agonists (Sahibzada et al., 2000; Manzke et al., 2003; Meyer et al., 2006; Dutschmann et al., 2009; Guenther et al., 2009; Manzke et al., 2011; Guenther et al., 2012; Ren et al., 2015), α-amino-3-hydroxy-5-methyl-4-isoxazole-propionic acid receptor (AMPA) agonists (ampakines) and brain targeted and allosteric AMPA receptor modulators (Ren et al., 2006; Greer and Ren, 2009; Ren et al., 2009; Lorier et al., 2010; Oertel et al., 2010; Cavalla et al., 2015; Haw et al., 2016; Dai et al., 2017; Sun et al., 2017; Dai et al., 2019; Xiao et al., 2020), the selective large conductance Ca2+-activated K+ (BK) channel blocker, GAL021 (Roozekrans et al., 2014; Golder et al., 2015; Roozekrans et al., 2015), which significantly improved alfentanil depression of ventilation in humans (Roozekrans et al., 2014; Roozekrans et al., 2015), the N-methyl-D-aspartate (NMDA) receptor antagonist, Esketamine (S-enantiomer of ketamine) (Jonkman et al., 2018), protein kinase A inhibitors, G protein-gated inwardly rectifying K+ (GIRK) channel blockers (Liang et al., 2018), microglial inhibitors (Hutchinson et al., 2008), thyrotropin releasing hormone and analogs (Boghosian et al., 2018), nicotinic receptor agonists (Ren et al., 2019, Ren et al., 2020), and KCNQ voltage-gated K+-channel blockers (Wei and Ramirez, 2019).
Most of the above OIRD reversal agents either did not enter into clinical trials, or did not progress satisfactorily in such trials (for reasons such as, lack of efficacy or unacceptable levels of side effects and/or toxicity), with a few others including ampakines and esketamine still under clinical evaluation (Dahan et al., 2018; Algera et al., 2019). As such, there is still an urgent unmet need to further develop drugs that effectively overcome OIRD by mechanisms other than direct blockade of ORs (Dahan et al., 2018; Algera et al., 2019). Recently, Algera et al. (2019) concluded that model-based drug development is needed in order to design an optimal reversal agent, which is not influenced by the kinetics of the interactions between opioid ligand and the OR, does not interfere with the analgesic actions of opioids, acts rapidly with sustained effects, and is devoid of as many adverse effects as possible. The conclusion based pharmacokinetic/pharmacodynamic data was that this ideal drug should act in the brainstem respiratory network via non-opioidergic pathways. These insights from Algera et al. (2019) are extremely valuable although issue could be taken as to whether the ideal drug should or should not directly combat OR-activated intracellular signaling processes that inhibit breathing, but not the OR-stimulated signaling pathways that elicit analgesia, and whether this ideal agent should act strictly in brainstem circuitry based on efficacy of peripherally restricted OR antagonists (Henderson et al., 2014). It is clear from Dahan et al. (2018), Algera et al. (2019) and Supplementary Table S1, that there is no unifying hypothesis as to the molecular mechanisms of action of putative ideal OIRD reversal agents.
There is compelling evidence that morphine inhibits the entry of L-cysteine into neurons via inhibition of the type 3 excitatory amino acid transporter (EEE3), resulting in subsequent changes in redox status (more oxidative environment) and/or the loss of L-cysteine participation in key signaling and metabolic pathways driving addiction (Trivedi et al., 2014; Trivedi and Deth, 2015). We hypothesize that this process may contribute to OIRD and reasoned that ethylester and methylester derivatives of cysteine and cysteine-containing compounds (i.e., thiolesters in which an ester linkage is attached to the carboxyl moiety) that can deliver the parent structure into cells may be a viable therapeutic strategy to overcome OIRD. As shown in Supplementary Table S2, there is an array of reduced (monothiol) and oxidized (disulfide) L-thiolesters that rapidly/readily enter cells within the peripheral and central nervous systems upon systemic administration or addition to in vitro preparations (references provided in Supplementary Table S2). Several L-thiolesters have been tested in disease/injury states, and for example, Henderson et al. (2016) demonstrated that γ-L-glutamyl cysteine ethylester (γ-L-GCee) decreased brain nitrosative stress in a rat traumatic brain injury model. The mechanisms by which L-thiolesters exert their biological effects are no doubt multi-factorial and may include (1) the formation of thiol adducts in the blood, such as D-glucose:L-cysteine (Wróbel et al., 1997; Szwergold, 2006; Li et al., 2015) and mixed disulfides (Wilcken and Gupta, 1979; Lash and Jones, 1985; Turell, et al., 2013), (2) direct docking of the parent L-thiolester with plasma membrane and/or intracellular proteins, such as ion-channels, receptors and enzymes that alters the activities of the proteins by mechanisms not associated with changes in redox status of the proteins (yet to be substantiated), (3) modulation of the redox status (e.g., cysteine-cystine switching) and function of plasma membrane proteins, such as Kv1.2 K+-channels (Baronas et al., 2017) and after entry into cells, redox modulation of functional intracellular proteins (Bogeski et al., 2011; Bogeski and Niemeyer, 2014; O-Uchi et al., 2014; Gamper and Ooi, 2015; Gao et al., 2017; García et al., 2018), (4) the formation of S-thiolated proteins, including S-cysteinylated, S-cysteinylglycinylated, S-glutathionylated and S-homocysteinylated proteins in plasma membranes and in cells (Winkler et al., 2007; Rossi et al., 2009; Auclair et al., 2013; Belcastro et al., 2017; Ghezzi and Chan, 2017; Bonifácio et al., 2021), (5) conversion of L-thiolesters to parent L-thiols by membrane associated esterases (Williams et al., 1985; Butterworth et al., 1993; Nishida et al., 1996; Satoh and Hosokawa, 1998; Satoh and Hosokawa, 2006; Hatfield et al., 2016) leading to increases in the intracellular levels of L-thiols, such as L-cysteine, which then enter into multiple metabolic pathways including those that generate hydrogen sulfide via the sequential actions of L-cysteine aminotransferase and cystathionine γ-lyase in central and peripheral tissues (Kimura, 2014; Kimura, 2017; Bełtowski, 2019) including the carotid bodies (Prabhakar, 2012), (6) conversion of L-thiolesters to cysteine sulfenics, sulfonics and sulfonics via cysteine dioxygenase (Yamaguchi and Hosokawa, 1987; Joseph and Maroney, 2007; Stipanuk et al., 2009; Stipanuk et al., 2011), and (7) formation of S-nitroso-L-cysteine, an endogenous S-nitrosothiol (Myers et al., 1990; Bates et al., 1991; Seckler et al., 2020) with many substantial roles in intracellular signaling cascades, (Lipton et al., 1993; Foster et al., 2009; Seth and Stamler, 2011; Stomberski et al., 2019; Gaston et al., 2020) including those controlling cardiorespiratory function (Davisson et al., 1996; Davisson et al., 1997; Ohta et al., 1997; Lipton et al., 2001; Gaston et al., 2006; Lewis et al., 2006; Gaston et al., 2020) and those involved in the attenuation of OIRD (Getsy et al., 2022a; Getsy et al., 2022b).
We are evaluating the abilities of monothiol and disulfide thiolesters to prevent and/or overcome OIRD in unanesthetized Sprague Dawley rats and reported that (1) pretreatment with L-glutathione ethylester (L-GSHee) diminished the adverse effects of fentanyl on ventilation and Alveolar-arterial (A-a) gradient (index gas alveolar exchange), but did not diminish fentanyl-induced antinociception/sedation (Jenkins et al., 2021), and (2) D-cysteine ethylester (D-CYSee) (Getsy et al., 2022c; Getsy et al., 2022d) or D-cystine diethylester (D-CYSdiee), D-cystine dimethyl ester (D-CYSdime) (Gaston et al., 2021) overcome the adverse actions of morphine on breathing and arterial blood-gas (ABG) chemistry while minimally affecting morphine antinociception/sedation. It appears that unlike OR antagonists, such as naloxone, the L-, D-thiolesters may overcome the deleterious actions of opioids by mechanisms other than direct/allosteric blockade of ORs (Gaston et al., 2021; Jenkins et al., 2021; Getsy et al., 2022a; Getsy et al., 2022b). Intravenous injections of L-cysteine ethylester (L-CYSee), but not L-cysteine, elicit an immediate and sustained reversal of the adverse effects of morphine on ABG chemistry and A-a gradient in isoflurane-anesthetized rats with a tracheal tube inserted to bypass the upper airway (nasopharynx, tongue, larynx, vocal folds), but not in rats without a tracheal tube (Mendoza et al., 2013). This finding that L-cysteine was inactive suggests that the effects of L-CYSee were due to entry of the L-thiolester into cells that initiate intracellular signaling events that overcome OIRD, but also obstruct the upper airway (e.g., collapse of the larynx, closure of the vocal folds, flaccid tongue that retracts and occludes the airway). The adverse effects of a combination of L-CYSee and morphine on the upper airway may involve the pharmacological actions of isoflurane since these effects of L-CYSee were not seen in sevoflurane-anesthetized rat (Bates et al., 1991), which is the dominant clinical gaseous anesthetic (Aoki et al., 2021; Apai et al., 2021; Liang et al., 2021). Here we extend our knowledge of L-thiolesters by describing the actions of L-CYSme on the effects of morphine in unanesthetized, unrestrained male Sprague Dawley rats. Similar to L-CYSee, L-CYSme has been widely used in studies designed to understand the effects of thiolesters on redox and metabolic processes (Supplementary Table S2). We report here that intravenous injections of L-CYSme (500 μmol/kg), but not L-cysteine (500 μmol/kg), elicit a rapid and sustained reversal of the adverse effects of 10 mg/kg of morphine on ventilatory parameters, A-a gradient and ABG chemistry while not affecting opioid antinociception/sedation. However, a distinct decrease in duration, but not maximal level of morphine-induced antinociception or sedation occurred with 5 mg/kg morphine. This pharmacological profile of L-CYSme would be advantageous in scenarios requiring immediate reversal of the deleterious effects of opioids on breathing and especially when opioids are essential for pain relief thereby making administration of popular OR antagonists a problematic situation.
All studies were carried out in strict accordance with the NIH Guide for Care and Use of Laboratory Animals (NIH Publication No. 80–23) revised in 1996, and in strict compliance with ARRIVE (Animal Research: Reporting of In Vivo Experiments) guidelines (http://www.nc3rs.org.uk/ page. asp? id = 1,357). All protocols involving the use of rats were approved by the Animal Care and Use Committees of Galleon Pharmaceuticals, Case Western Reserve University, and the University of Virginia. Adult male Sprague Dawley rats were purchased from Harlan Industries (Madison, WI, United States). After 5 days of recovery from transportation, the rats received femoral artery catheters and/or jugular vein catheters under 2–3% isoflurane anesthesia (Henderson et al., 2014; Gaston et al., 2021). The rats were given 4 days to recover from surgery before use. All femoral arterial catheters were flushed daily with a heparin solution (50 units heparin in 0.1 M, pH 7.4 phosphate-buffered saline). On the day of the study, all arterial and venous catheters were flushed with 0.3 ml of phosphate-buffered saline (0.1 M, pH 7.4) 3–4 h before commencement of the study. The pH of all stock solutions of vehicle, L-cysteine and L-CYSme were adjusted to pH of 7.2 with 0.25 M NaOH. All studies were done in a quiet room with relative humidity of 50 ± 2% and room temperature of 21.3 ± 0.2 °C. The antinociception and ventilatory/ABG chemistry experiments were performed in separate groups of rats to not compromise the ventilatory measurements. The plethysmography and antinociception recording sessions and the arterial blood sampling studies (ABG assays) were done by an investigator who injected the opioid, vehicle, L-cysteine or L-CYSme. The syringes with vehicle or test drug were made up by another investigator, such that the person performing the injections was blinded to the treatment protocol. A liquid form of (+)-morphine sulfate (10 mg/ml) was obtained from Baxter Healthcare Corporation (Deerfield, IL, United States). L-CYSme HCl powder (Product number: 410209; PubChem Substance ID: 24865699) was obtained from Sigma-Aldrich (St. Louis, MO, United States) and divided into 100 mg amounts under N2 gas and stored at 4°C. Solutions of L-CYSme (dissolved in saline and brought to pH 7.2 with 0.1 M NaOH at room temperature) were prepared immediately before injection. In every case, the data files resulting from each study were collated and analyzed by another investigator in the group blinded to the treatment protocol.
Ventilatory parameters were recorded continuously in the unrestrained freely-moving rats using a whole body plethysmography system (PLY3223; Data Sciences International, St. Paul, MN) as detailed previously May et al., 2013a; May et al., 2013b; Young et al., 2013; Getsy et al., 2014; Henderson et al., 2014; Baby et al., 2018; Gaston et al., 2020; Baby S. et al., 2021; Baby S. M. et al., 2021; Getsy et al., 2021; Gaston et al., 2021; Seckler et al., 2022). The directly recorded and calculated (derived) parameters are defined in Supplementary Table S3 (Hamelmann et al., 1997; Lomask, 2006; Tsumuro et al., 2006; Quindry et al., 2016; Gaston et al., 2021). The directly recorded and derived ventilatory parameters, and the abbreviations used in this manuscript are as follows: frequency of breathing (Freq), tidal volume (TV), minute ventilation (MV), inspiratory time (Ti), expiratory time (Te), Ti/Te, end inspiratory pause (EIP), end expiratory pause (EEP), peak inspiratory flow (PIF), peak expiratory flow (PEF), PIF/PEF ratio, expiratory flow at 50% expired TV (EF50), relaxation time (RT), inspiratory drive (TV/Ti), expiratory drive (TV/Te), apneic pause [(Te/RT)-1], non-eupneic breathing index (NEBI) and NEBI corrected for Freq (NEBI/Freq). A diagram of the relationships between a few of the directly recorded parameters (adapted from Lomask, 2006) are shown in Supplementary Figure S1. On the day of the study, each rat was placed in an individual plethysmography chamber and allowed at least 60 min to acclimatize so that resting (i.e., baseline or, pre) ventilatory parameter values were accurately defined. Two sets of studies were performed. Study 1: Two groups of rats received a bolus injection of morphine (10 mg/kg, IV) and after 15 min, one group received a bolus injection of vehicle (saline) and the other group received a bolus injection of L-CYSme (500 μmol/kg, IV). The rats then received a second injection of vehicle or L-CYSme (500 μmol/kg, IV) 15 min later. Study 2: Two groups of rats received a bolus injection of morphine (10 mg/kg, IV) and after 15 min, one group received an injection of vehicle (saline) and the other group received an injection of L-cysteine (500 μmol/kg, IV). The rats then received a second injection of vehicle or L-cysteine (500 μmol/kg, IV) 15 min later. Ventilatory parameters were monitored for 60 min after the second injection of vehicle, L-cysteine or L-CYSme. The body weights of all groups of rats were similar to one another and as such, ventilatory parameters related to volumes (e.g., TV, PIF, PEF, EF50) are presented without correcting for body weight. The FinePointe (DSI) software constantly corrected digitized ventilatory values originating from the actual waveforms for alterations in chamber humidity and temperature. Pressure changes associated with the respiratory waveforms were then converted to volumes (e.g., TV, PIF, PEF, EF50) employing the algorithms of Epstein and colleagues (Epstein and Epstein, 1978; Epstein et al., 1980). Specifically, factoring in chamber humidity and temperature, cycle analyzers filtered the acquired signals, and FinePointe algorithms generated an array of box flow data that identified a waveform segment as an acceptable breath. From that data vector, the minimum and maximum values were determined and multiplied by a compensation factor provided by the selected algorithm (Epstein and Epstein, 1978; Epstein et al., 1980) thus producing TV, PIF and PEF values that were used to determine non-eupneic breathing events expressed as the non-eupneic breathing index (NEBI), reported as the percentage of non-eupneic breathing events per epoch (Getsy et al., 2014). Apneic pause was determined by the formula, (Expiratory Time/Relaxation Time)—1 (Gaston et al., 2021).
Changes in ABG chemistry values (pH, pCO2, pO2 and sO2) and A-a gradients elicited by an injection of morphine (10 mg/kg, IV) in three different sets of unanesthetized freely-moving rats (n = 9 rats per group) and after an injection of vehicle (saline, IV; 82.2 ± 0.4 days of age; 335 ± 2 g body weight), L-cysteine (500 μmol/kg, IV; 82.6 ± 0.5 days; 336 ± 3 g) or L-CYSme (500 μmol/kg, IV; 81.7 ± 0.5 days; 332 ± 2 g) were determined as detailed previously (Henderson et al., 2014; Baby et al., 2018; Baby S. et al., 2021; Gaston et al., 2021). Briefly, samples of arterial blood (100 μl) were taken 15 min before and 15 min after injection of morphine (10 mg/kg, IV). The rats then immediately received an injection of vehicle, L-cysteine or L-CYSme and blood samples were taken at 5, 15, 30 and 45 min time points. The pH, pCO2, pO2 and sO2 were determined by a Radiometer blood-gas analyzer (ABL800 FLEX). The A-a gradient defines differences between alveolar and arterial blood O2 concentrations (Stein et al., 1995; Story 1996; Henderson et al., 2014). A reduction in PaO2, without a concomitant alteration in A-a gradient is the result of hypo-ventilation, whereas a decrease in PaO2 with a concomitant elevation in A-a gradient indicates an on-going mismatch in ventilation-perfusion in alveoli (Stein et al., 1995; Story 1996; Henderson et al., 2014; Gaston et al., 2021). A-a gradient = PAO2 − PaO2, where PAO2 is the partial pressure (p) of alveolar O2 and PaO2 is pO2 in the sampled arterial blood. PAO2 = [(FiO2 x (Patm - PH2O) - (PaCO2/respiratory quotient)], where FiO2 is the fraction of O2 in inspired air; Patm is atmospheric pressure; PH2O is the partial pressure of H2O in inspired air; PaCO2 is pCO2 in arterial blood; and respiratory quotient (RQ) is the ratio of CO2 eliminated/O2 consumed. We took FiO2 of room-air to be 21% = 0.21, Patm to be 760 mmHg, and PH2O to be 47 mmHg (Gaston et al., 2021). We took the RQ value of our adult male rats to be 0.9 (Stengel et al., 2010; Chapman et al., 2012; Gaston et al., 2021; Jenkins et al., 2021).
The antinociceptive effects elicited by an injection of morphine and a subsequent injection of vehicle or D-CYSme were determined by evaluating tail-flick latency (TFL) with the use of a Tail-Flick Analgesia Meter (IITC Life Science Inc., United States) as described previously (Lewis et al., 1991; Meller et al., 1991; Carstens and Wilson, 1993; Henderson et al., 2014; Golder et al., 2015; Gaston et al., 2021; Jenkins et al., 2021). This procedure entailed a minor level of manual restraint during the positioning of the tail to apply a thermal stimulus sufficient to induce a latency of tail withdrawal of about 3.0 s in all rats. In reversal studies, baseline TFL was tested in all rats prior to any drug administration (-20 min time point). One group of rats (81.2 ± 0.9 days of age; 337 ± 2 g body weight, n = 6) received an injection of morphine (5 mg/kg, IV) and after TFL testing at + 20 min they immediately received a bolus IV injection of vehicle (saline, 100 μL/100 g body weight). A second group of rats (80.5 ± 1.5 days; 336 ± 3 g, n = 4) received a bolus injection of L-CYSme (500 μmol/kg, IV) at the +20 min time point. A third group of rats (80.7 ± 1.1 days of age; 335 ± 2 g body weight, n = 6) received an injection of morphine (10 mg/kg, IV) and after TFL testing at + 20 min they immediately received a bolus IV injection of vehicle (saline, 100 μL/100 g body weight). A fourth group of rats that had received 10 mg/kg morphine, IV (81.5 ± 1.6 days; 336 ± 3 g, n = 6) received a bolus injection of L-CYSme (500 μmol/kg, IV) at the +20 min time point. TFL was tested 20, 40, 60 , 70, 100 and 160 min post-injection of vehicle or L-CYSme. The resulting data are presented as actual TFL (sec) and as maximum possible effect (%MPE) determined by the formula, %MPE = [(post-injection TFL − baseline TFL)/(12 − baseline TFL)] x 100 (Lewis et al., 1991; Meller et al., 1991; Carstens and Wilson, 1993; Henderson et al., 2014; Golder et al., 2015; Gaston et al., 2021; Jenkins et al., 2021). In pre-treatment studies, baseline TFL was tested in all rats prior to any drug administration (−20 min time point). One group of rats (81.2 ± 0.5 days of age; 330 ± 2 g body weight, n = 9) then received a bolus IV injection of vehicle (saline, 100 μL/100 g body weight). A second group (82.0 ± 0.4 days; 334 ± 2 g, n = 9) received a bolus injection of L-CYSme (500 μmol/kg, IV). A third group (79.8 ± 0.4 days; 329 ± 3 g, n = 6) received a bolus injection of L-cysteine (500 μmol/kg, IV). TFL was then tested in the three groups 10 and 20 min after these injections (i.e., at the -10 and 0 min time points, respectively). At +20 min post-injection (time point 0 min), the rats got an injection of morphine (10 mg/kg, IV) and TFL was tested 20 , 40 , 60 , 90 , 120, 150, 180, 210, 240, 360 and 480 min post-injection. In another study, one group of rats (82.2 ± 0.4 days of age; 336 ± 3 g, n = 9) received an injection of vehicle (saline, 100 μL/100 g body weight) and a second group (82.5 ± 0.4 days; 339 ± 2 g, n = 9) received an injection of L-CYSme (500 μmol/kg, IV) and then an IV injection of a 5 mg/kg dose of morphine and TFL was tested as above. TFL data are presented as actual TFL (sec) and as maximum possible effect (%MPE) determined by the formula, %MPE = [(post-injection TFL − baseline TFL)/(12 − baseline TFL)] x 100 (Lewis et al., 1991; Meller et al., 1991; Carstens and Wilson, 1993; Henderson et al., 2014; Golder et al., 2015; Gaston et al., 2021; Jenkins et al., 2021).
The antinociceptive actions of morphine, vehicle, L-cysteine and L-CYSme were assessed by hot-plate (hind-paw withdrawal) latency (HPL) assay using the Hargreaves’s method (Hargreaves et al., 1988), as detailed previously (Baby S. et al., 2021; Gaston et al., 2021). In brief, HPL in response to a thermal stimulus was assessed using a radiant heat source (IITC, CA, United States) aimed at the plantar surface of the left hind-paw. This technique did not require restraint of the rat while positioning the thermal stimulus that was sufficient to produce paw withdrawal from the floor of the hot-plate in about 20 s (baseline values) before injection of a drug (cut-off latency was 40 s to avoid any tissue damage). Baseline HPL was tested in all three groups (−20 min time-point). One group of rats (79.7 ± 0.3 days of age; 334 ± 3 g body weight, n = 9) received an IV injection of vehicle (saline, 100 μl/100 g body weight). A second group (80.0 ± 0.3 days; 337 ± 3 g, n = 9) received an injection of L-cysteine (500 μmol/kg, IV). A third group (80.1 ± 0.3 days; 336 ± 3 g, n = 9) received an injection of L-CYSme (500 μmol/kg, IV). HPL was tested 10 and 20 min later (i.e., at the -10 and 0 min time points). At +20 min post-injection (time point 0 min), all rats received an injection of morphine (10 mg/kg, IV) and HPL was tested 15, 30, 60, 90 , 120, 180, 210, 240, 360 and 480 min post-injection. The data are presented as HPL (sec) and as “maximum possible effect” (%MPE) determined by the formula, %MPE = [(post-injection HPL − baseline HPL)/(20 − baseline HPL)] x 100.
This study evaluated the effects of bolus injections of vehicle, L-cysteine (500 μmol/kg, IV) and L-CYSme (500 μmol/kg, IV) on the duration of morphine (10 mg/kg, IV) on the modified righting reflex test. Each rat was placed in an open plastic chamber to evaluate the duration of loss of the modified righting reflex. The injection of morphine caused the rats to assume numerous types of postures, including being motionless sprawled out on their stomach on the chamber floor, lying motionless on their side, and splayed out on their stomach with the head up against the chamber wall. The duration of the sedative effects of morphine was taken as the time interval from the time of morphine injection to full recovery of the righting reflex (i.e., when rats attained/maintained a normal posture on all four legs (Ren et al., 2015; Yu et al., 2018; Ren et al., 2020; Jenkins et al., 2021). One group of rats (79.6 ± 0.3 days; 328 ± 2 g, n = 9) received an injection of morphine (10 mg/kg, IV) and after 15 min an injection of vehicle (saline). A second group (79.5 ± 0.3 days; 331 ± 3 g, n = 9) received an injection of morphine (10 mg/kg, IV) and after 15 min an injection of L-cysteine (500 μmol/kg, IV). A third group (79.4 ± 0.3 days; 327 ± 3 g, n = 9) received a bolus injection of morphine (10 mg/kg, IV) and after 15 min an injection of L-CYSme (500 μmol/kg, IV).
The directly recorded and arithmetically-derived parameters (1 min bins) were taken for statistical analyses. Pre-drug 1 min bins excluded occasional marked deviations from resting values due to abrupt movements by the rats, such as scratching. The exclusions ensured accurate determination of baseline parameters. All data are presented as mean ± SEM and were evaluated using one-way and two-way ANOVA followed by Bonferroni corrections for multiple comparisons between means using the error mean square terms from each ANOVA analysis (Wallenstein et al., 1980; Ludbrook, 1998; McHugh, 2011) as detailed previously (Getsy et al., 2021). A p < 0.05 value denoted the initial level of statistical significance that was modified according to the number of comparisons between means as described by Wallenstein et al. (1980). The modified t-statistic is t = (mean group 1 - mean group 2)/[s x (1/n1 + 1/n2)1/2] where s2 = the mean square within groups term from the ANOVA (the square root of this value is used in the modified t-statistic formula) and n1 and n2 are the number of rats in each group under comparison. Based on an elementary inequality called Bonferroni’s inequality, a conservative critical value for modified t-statistics obtained from tables of t-distribution using a significance level of P/m, where m is the number of comparisons between groups to be performed (Winer, 1971). The degrees of freedom are those for the mean square for within group variation from the ANOVA table. In the majority of situations, the critical Bonferroni value cannot be found in conventional tables of the t- distribution but can be approximated from tables of the normal curve by t* = z + (z + z3)/4n, with n being the degrees of freedom and z being the critical normal curve value for P/m (Wallenstein et al., 1980; Ludbrook, 1998; McHugh, 2011). Wallenstein et al. (1980) first demonstrated that the Bonferroni procedure is preferable for general use since it is easy to apply, has the widest range of applications, and because it provides critical values that are lower than those of other procedures when the investigator can limit the number of comparisons (and will be slightly larger than those of other procedures if many comparisons are made. As mentioned, a value of p < 0.05 was taken as the initial level of statistical significance (Wallenstein et al., 1980; Ludbrook, 1998; McHugh, 2011 and statistical analyses were performed with the aid of GraphPad Prism software (GraphPad Software, Inc., La Jolla, CA).
The ages, body weights, and baseline (pre) ventilatory parameter values of the rats before the administration of morphine (10 mg/kg, IV) are shown in Supplementary Table S4. There were no between group differences for any of the parameters (p > 0.05, for all comparisons). A summary of the total responses produced by the injection of morphine (10 mg/kg, IV) during the 15 min prior to the rats receiving injections of vehicle or L-CYSme are described in Supplementary Table S5. The injection of morphine elicited pronounced decreases in TV, MV, PIF, PIF/PEF and inspiratory and expiratory drives that were accompanied by substantial increases in Ti, Te, Ti/Te, EIP, EEP, apneic pause, NEBI and NEBI/Freq. There were no sustained changes in Freq, Te, PEF, EF50 or RT. Figure 1 summarizes the minute-by-minute values for Freq, TV and MV recorded before, after the injection of morphine (10 mg/kg, IV) and subsequent injections of vehicle or L-CYSme (500 μmol/kg, IV). As seen in Panel A, the injection of morphine elicited a transient increase in Freq that was followed by a relatively minor decrease in Freq in both groups that had recovered towards baseline values at the time (15 min post-injection of morphine) that vehicle or L-CYSme was injected. The two injections of vehicle given 15 min apart elicited negligible responses (Panel A). Panel A also shows that the first injection of L-CYSme elicited a relatively small but sustained increase in Freq, whereas the second injection elicited a pronounced elevation in Freq that gradually declined towards resting values over the following 60 min. As can be seen in Panel B, the injection of morphine elicited a rapid, substantial, and sustained decrease in TV in both groups of rats and this decrease in TV remained 15 min post-administration of morphine when the injections of vehicle or L-CYSme were given The injections of vehicle did not immediately change TV, which gradually recovered to pre-morphine levels toward the end of the recording period (90 min post-morphine). As also seen in Panel B, the first injection of L-CYSme elicited a prompt and sustained reversal of the deleterious effects of morphine on TV (to values equivalent to those prior to administration of morphine), whereas the second injection elicited a remarkable elevation in TV that had not fully recovered to baseline values after 60 min. As can be seen in Panel C, the above changes in Freq and TV translated into sustained morphine-induced decreases in MV and a prompt and sustained reversal by L-CYSme of the deleterious effects of morphine on MV.
FIGURE 1. Frequency of breathing Panel (A), tidal volume Panel (B) and minute ventilation Panel (C) prior to (Pre), following the injection of morphine (10 mg/kg, IV), and then two injections of vehicle or L-cysteine methyl ester (L-CYSme, 500 μmol/kg, IV) given 15 min apart, in freely-moving rats. The data are presented as mean ± SEM. There were 6 rats in each group.
As shown in Figure 2 (Panel A), the injection morphine elicited a transient decrease in Ti that was followed by sustained increases in Ti in both groups of rats. The two injections of vehicle elicited minor changes from the increase in Ti seen after morphine injection, whereas the first and especially the second injection of L-CYSme elicited substantial decreases in Ti. As seen in Panel B, morphine elicited a transient increase in Te that was followed by a sustained decrease in Te. The subsequent injections of vehicle did not produce noticeable responses, whereas the second injection of L-CYSme in particular elicited a substantial decrease in Te that remained lower than values in vehicle-treated rats for about 25 min. As shown in Panel C, the Ti/Te ratio fell markedly initially after injection of morphine, but rose substantially and remained elevated above baseline in both groups of rats. The injections of vehicle produced negligible responses, whereas the second but not first injection of L-CYSme caused a noticeable and sustained decrease in Ti/Te values in comparison to those in the vehicle-treated rats. As can be seen in Figure 3 (Panel A), the injection of morphine elicited a sustained increase in EIP in both groups of rats and that the injections of vehicle elicited negligible responses. However, the first injection of L-CYSme elicited substantial decreases in EIP that had returned to the sustained increase in EIP seen 15 min post morphine injection by the time the second injection was given. The second injection of L-CYSme produced a profound and long-lasting decrease in EIP. As can seen in Panel B, morphine elicited a pronounced transient increase in EEP that was followed by a sustained decrease in EEP. The two injections of L-CYSme produced trivial changes in EEP and did not alter the sustained decrease in EEP. Figure 4 (Panel A) demonstrates that morphine elicited a transient increase followed by a pronounced and sustained decrease in PIF, and that the injections of vehicle elicited minimal responses. In contrast, the injections of L-CYSme elicited pronounced and sustained increases in PIF with the first injection reversing the effects of morphine back to baseline values, and the second injection elevating PIF to values considerably above baseline values. As seen in Panel B, morphine elicited a transient reduction in PEF that had resolved by the time the injections of vehicle or L-CYSme were administered. The two injections of vehicle produced trivial changes in EEP, whereas the two injections of L-CYSme elicited pronounced and sustained increases in PEF to levels well above baseline values. Taken together, these changes in PIF and PEF resulted in morphine eliciting sustained decreases in PIF/PEF with the second injection of L-CYSme causing an increase towards baseline values, however this increase was not sustained over the 60 min recovery period. As seen in Figure 5 (Panel A), the injection of morphine elicited a transient increase that EF50 that was followed by a minor, but sustained, elevation in EF50 in the rats that received the two injections of vehicle. The two injections of L-CYSme elicited pronounced and sustained increases in EF50. As seen in Panel B, morphine elicited a transient decrease in RT that was followed by a sustained decrease in rats that received the two injections of vehicle. The second injection of L-CYSme elicited a pronounced further decrease in RT that had resolved by 60 min post-injection to values near the vehicle-treated rats. As summarized in Panel C, morphine elicited a transient but pronounced increase in apneic pause and the injections of both vehicle and L-CYSme elicited trivial responses. As shown in Figure 6, the injection of morphine elicited a prompt and sustained decrease in inspiratory drive (Panel A) and a pronounced, but relatively transient reduction in expiratory drive (Panel B). The injections of vehicle elicited trivial responses whereas the injections of L-CYSme produced pronounced and sustained increases in inspiratory and expiratory drives. As summarized in Figure 7, the injection of morphine elicited a transient increase in NEBI (Panel A) and NEBI/Freq (Panel B) that was followed by a sustained decrease in both parameters. Injections of L-CYSme elicited trivial responses and did not alter the time-course of morphine-induced changes in NEBI or NEBI/Freq observed in vehicle-injected rats. Figure 8 summarizes the total (cumulative) changes in ventilatory parameters recorded over the 15 min period after the first injection of vehicle or L-CYSme (expressed as %change from Pre values). The first injection of L-CYSme caused a sustained reversal of the deleterious effects of morphine on TV, MV, Ti, EIP and inspiratory drive (InspD). The first injection of L-CYSme also resulted in a minor increase in Freq and substantial increases in PEF and expiratory drive (ExpD), but did not alter the morphine-induced decreases in Te, EEP, PIF/PEF, NEBI or NEBI/Freq or the morphine-induced increases in Ti/Te. Figure 9 shows the total (cumulative) changes in ventilatory parameters recorded over the 60 min period after the second injection of vehicle or L-CYSme (%change from Pre values). The second injection of L-CYSme overcame the morphine-induced decreases in TV, MV, PIF and inspiratory drive (InspD) and slightly increased the morphine-induced decease in NEBI/Freq but not NEBI. The second injection of L-CYSme augmented the morphine-induced increase in EF50 and caused a substantial increase in Freq, PEF and expiratory drive (ExpD). The second injection of L-CYSme did not alter the morphine-induced decreases on Te, EEP, PIF/PEF or the lack of effects of morphine on apneic pause (AP).
FIGURE 2. Inspiratory time Panel (A), expiratory time Panel (B) and inspiratory time/expiratory time (Ti/Te) Panel (C) prior to (Pre), following the injection of morphine (10 mg/kg, IV), and then two injections of vehicle or L-cysteine methyl ester (L-CYSme, 500 μmol/kg, IV) given 15 min apart, in freely-moving rats. The data are shown as mean ± SEM. There were 6 rats in each group.
FIGURE 3. End inspiratory pause Panel (A) and end expiratory pause Panel (B) prior to (Pre), following the injection of morphine (10 mg/kg, IV), and then two injections of vehicle or L-cysteine methyl ester (L-CYSme, 500 μmol/kg, IV) given 15 min apart, in freely-moving rats. The data are presented as mean ± SEM. There were 6 rats in each group.
FIGURE 4. Peak inspiratory flow (Panel (A), peak expiratory flow (Panel (B) and peak inspiratory flow/peak expiratory flow (PIF/PEF) (Panel (C) prior to (Pre), following the injection of morphine (10 mg/kg, IV), and then two injections of vehicle or L-cysteine methyl ester (L-CYSme, 500 μmol/kg, IV) given 15 min apart, in freely-moving rats. The data are presented as mean ± SEM. There were 6 rats in each group.
FIGURE 5. Expiratory flow at 50% of expired tidal volume (EF50) Panel (A), relaxation time Panel (B) and apneic pause Panel (C) prior to (Pre), following the injection of morphine (10 mg/kg, IV), and then two injections of vehicle or L-cysteine methyl ester (L-CYSme, 500 μmol/kg, IV) given 15 min apart, in freely-moving rats. The data are presented as mean ± SEM. There were 6 rats in each group.
FIGURE 6. Inspiratory drive (Panel (A) and expiratory drive (Panel (B) prior to (Pre), following the injection of morphine (10 mg/kg, IV), and then two injections of vehicle or L-cysteine methyl ester (L-CYSme, 500 μmol/kg, IV) given 15 min apart, in freely-moving rats. The data are presented as mean ± SEM. There were 6 rats in each group.
FIGURE 7. Non-eupneic breathing (NEB) index Panel (A) and NEB index/frequency of breathing (Panel (B) prior to (Pre), following the injection of morphine (10 mg/kg, IV), and then two injections of vehicle or L-cysteine methyl ester (L-CYSme, 500 μmol/kg, IV) given 15 min apart, in freely-moving rats. The data are presented as mean ± SEM. There were 6 rats in each group.
FIGURE 8. Cumulative changes in ventilatory parameters recorded over the 15-min period following the first injection of vehicle or L-cysteine methyl ester (L-CYSme, 500 μmol/kg, IV) in rats that had been injected with morphine (10 mg/kg, IV). Panel (A) frequency of breathing (Freq), tidal volume (TV), minute ventilation (MV), inspiratory time (Ti), expiratory time (Te), Ti/Te, end inspiratory pause (EIP), and end expiratory pause (EEP). Panel (B) peak inspiratory flow (PIF), peak expiratory flow (PEF), PIF/PEF, relaxation time (RT), expiratory flow at 50% of expired tidal volume (EF50), apneic pause (AP), inspiratory drive (InspD, TV/Ti), expiratory drive (ExpD, TV/Te), non-eupneic breathing index (NEBI), and NEBI/freq (NEBI/F). Data are presented as mean ± SEM. There were 6 rats in each group. *p < 0.05, significant difference from Pre values. †p < 0.05, L-CYSme versus vehicle.
FIGURE 9. Cumulative changes in ventilatory parameters recorded over the 60-min period that followed the second injection of vehicle or L-cysteine methyl ester (L-CYSme, 500 μmol/kg, IV) in rats injected with morphine (10 mg/kg, IV). Panel (A) frequency of breathing (Freq), tidal volume (TV), minute ventilation (MV), inspiratory time (Ti), expiratory time (Te), Ti/Te, end inspiratory pause (EIP), and end expiratory pause (EEP). Panel (B) peak inspiratory flow (PIF), peak expiratory flow (PEF), PIF/PEF, relaxation time (RT), expiratory flow at 50% of expired tidal volume (EF50), apneic pause (AP), inspiratory drive (InspD, TV/Ti), expiratory drive (ExpD, TV/Te), non-eupneic breathing index (NEBI), and NEBI/freq (NEBI/F). Data are presented as mean ± SEM. There were 6 rats in each group. *p < 0.05 significant difference from Pre values. †p < 0.05, significant difference between L-CYSme versus vehicle.
The effects of L-cysteine or L-CYSme on morphine changes in ABG chemistry and A-a gradient are shown in Figure 10 summarizes the recorded ABG values (pH, pCO2, pO2 and sO2) before and after injection of morphine (10 mg/kg, IV) and subsequent injection of vehicle, L-cysteine (500 μmol/kg, IV) or L-CYSme (500 μmol/kg, IV) in three separate groups of unanesthetized rats. The values denoted M15 to M60 are the times post-morphine injection and the values denoted D0 to D45 reflect the times following injection of vehicle, L-cysteine or L-CYSme. Panel A shows that the injection of morphine elicited substantial and equivalent decreases in arterial blood pH in the three groups of rats and that the injection of L-CYSme, but not L-cysteine, elicited a prompt and sustained reversal of the acidosis. Panel B shows that injection of morphine produced a substantial and equivalent increase in pCO2 in the three treatment groups and that L-CYSme, but not L-cysteine, caused an immediate and sustained reversal of the hypercapnia. Panels C and D demonstrate that morphine produced sustained decreases in pO2 and sO2, respectively and that L-CYSme, but not L-cysteine, produced a prompt and sustained reversal of the hypoxemic status of the arterial blood. As summarized in Figure 11, the injection of morphine (10 mg/kg, IV) produced substantial and equivalent increases in A-a gradient values (index of diminished alveolar gas-exchange in the lungs) in the three groups of rats. As can be seen, the injection of L-CYSme (500 μmol/kg, IV), but not the injection of L-cysteine (500 μmol/kg, IV), produced an immediate and sustained reversal of this deleterious effect of morphine. The arithmetic changes in ventilatory parameters presented in Supplementary Table S6 reinforce all of the above conclusions.
FIGURE 10. Values of pH Panel (A), pCO2 Panel (B), pO2 Panel (C) and sO2 Panel (D) before (Pre) and after injection of morphine (10 mg/kg, IV) in three separate groups of freely-moving rats followed by injection of vehicle (VEH, saline), L-cysteine (500 μmol/kg, IV) or L-cysteine methyl ester (L-CYSme, 500 μmol/kg, IV). The terms M15, M30, M30, M45 and M60 denote 15, 30, 45 and 60 min after injection of morphine. The terms D0, D15, D30 and D45 denote 0, 15, 30 and 45 min after injection of drug (vehicle, L-Cysteine or L-CYSme). The data are prsented as mean ± SEM. There were 9 rats in each group. *p < 0.05, significant change from Pre values. †p < 0.05, significant change between L-CYSme versus vehicle.
FIGURE 11. Alveolar-arterial (A-a) gradient values before (Pre) and after injection of morphine (10 mg/kg, IV) in three groups of freely-moving rats followed by injection of vehicle (VEH, saline), L-Cysteine (500 μmol/kg, IV) or L-cysteine methyl ester (L-CYSme, 500 μmol/kg, IV). The terms M15, M30, M45 and M60 denote 15, 30, 45 and 60 min after injection of morphine. The terms D0, D15, D30 and D45 denote 0, 15, 30 and 45 min after injection of drug (vehicle, L-Cysteine or L-CYSme). The data are mean ± SEM. There were 9 rats in each group. *p < 0.05, significant change from Pre values. †p < 0.05, significant change between L-CYSme versus vehicle.
As seen in Figure 12, the duration of the antinociceptive effects of morphine (TFL studies) were minimally reduced by the injection of L-CYSme (500 μmol/kg, IV) with differences only occurring at the 180 min time point. As seen in Supplementary Table S7, the injection of L-cysteine (500 μmol/kg, IV) elicited a transient decrease in TFL, whereas injections of L-CYSme (500 μmol/kg, IV) elicited a transient increase in TFL and HPL. All responses were evident 10 min after administration of L-cysteine or L-CYSme but not after 20 min, the time point when morphine (10 mg/kg, IV) was injected. Supplementary Figure S2 summarizes the magnitudes and durations of the antinociceptive effects of 5 mg/kg (Panels A and C) or 10 mg/kg (Panels B and D) doses of morphine as assessed by TFL assay in rats pre-treated with vehicle or L-CYSme (500 μmol/kg, IV). The duration of antinociception elicited by 10 mg/kg of morphine was greater than that elicited by the 5 mg/kg dose in the vehicle-treated rats. The duration, but not the magnitude, of the antinociceptive actions of morphine were diminished in L-CYSme-treated rats. The loss of antinociception began 120 min after injection of 5 mg/kg of morphine and 210 min after administration of the 10 mg/kg dose of the opioid. As summarized in Supplementary Figure S3, pre-treatment with L-CYSme (500 μmol/kg, IV) also reduced the duration, but not magnitude, of the antinociceptive actions of morphine (10 mg/kg, IV) as assessed by HPL assay. Again, the loss of antinociception began 120 min after the injection of 5 mg/kg dose of morphine and 210 min after injection of 10 mg/kg of the opioid. In contrast, neither the magnitude nor duration of antinociceptive actions of the 5 and 10 mg/kg doses of morphine were affected by pre-treatment with L-cysteine (Supplementary Figure S4).
FIGURE 12. Changes in tail-flick latency values elicited by an intravenous injection of vehicle (VEH, saline) or L-cysteine methyl ester (L-CYSme, 500 μmol/kg, IV) given 20 min after the injection of morphine at 5 mg/kg Panel (A) or 10 mg/kg Panel (B) in freely-moving rats. Panels (C,D) display the data as maximum possible effect (%MPE). The data are shown as mean ± SEM. There were 6 rats in each group. *p < 0.05, significant change from Pre. †p < 0.05, significant change between L-CYSme versus vehicle.
The observed behaviors in each group of rats that received injections of morphine plus injections of vehicle, L-cysteine or L-CYSme were not obviously different to one another. More specifically, the injection of morphine elicited a relatively rapid (within 2–3 min) sedative effect in all of the rats that consisted as an almost complete loss of mobility and unusual body postures (see descriptions in Material and Methods). The full return of the modified righting-reflex in vehicle-treated rats (74.3 ± 11.5 min, n = 9), L-cysteine (500 μmol/kg, IV)-treated rats (69.8 ± 8.5 min, n = 9) and L-CYSme (500 μmol/kg, IV)-treated rats (91.0 ± 11.2 min, n = 9) were similar to one another (p > 0.05, for all between-group comparisons).
The changes in ventilatory parameters elicited by a 10 mg/kg intravenous dose of morphine were consistent with those of our previous reports (May et al., 2013a; May et al., 2013b; Young et al., 2013; Baby et al., 2018; Baby S. M. et al., 2021; Gaston et al., 2021; Getsy et al., 2022d). Morphine elicited a transient reduction in Freq that was not truly reflective of its effects on respiratory timing because it elicited a sustained increase in Ti (i.e., a longer inspiratory duration), but a pronounced decrease in Te (i.e., a shorter expiratory duration). Moreover, morphine caused a long-lasting increase in EIP, whereas it elicited a transient increase followed by a sustained decrease in EEP. The ability of morphine to lengthen Ti while marginally affecting Te (Fone and Wilson, 1986; Kasaba et al., 1997; Chevillard et al., 2009) or the shortening of Te (May et al., 2013a; May et al., 2013b; Young et al., 2013; Baby et al., 2018; Baby S. M. et al., 2021; Gaston et al., 2021) is well-known, as is the propensity of opioids to differentially affect EIP and EEP (May et al., 2013a; May et al., 2013b; Henderson et al., 2013; Young et al., 2013; Henderson et al., 2014; Baby et al., 2018; Baby S. M. et al., 2021; Gaston et al., 2021). Mechanisms of action and brain structures, including nucleus tractus solitarius (Hassen et al., 1982; Li et al., 1996; Iniushkin, 1997; Zhuang et al., 2012), parabrachial nucleus/Kölliker-Fuse nucleus (Eguchi et al., 1987; Lalley et al., 2014; Bachmutsky et al., 2020; Varga et al., 2020), and pre-Bötzinger complex (Bachmutsky et al., 2020; Varga et al., 2020), by which morphine and fentanyl affect inspiratory and expiratory timing have been investigated. It is evident that the qualitative and quantitative responses elicited by opioids, such as morphine, fentanyl and remifentanil, on Ti and Te are very much dose-dependent (Li et al., 1996; Henderson et al., 2013; Henderson et al., 2014; Palkovic et al., 2020; Palkovic et al., 2021; Palkovic et al., 2022). However, the sites and mechanisms by which opioids exert their differential effects on EIP and EEP have received only limited attention (Henderson et al., 2014; Bachmutsky et al., 2020). Studies in anesthetized rats and in vitro preparations found that depression of carotid body chemoreceptor afferent reflexes participates in morphine-induced reduction in Freq, whereas morphine (10 mg/kg)-induced reduction in Freq is enhanced in unanesthetized Sprague Dawley rats with bilateral carotid sinus nerve transection (Baby et al., 2018), suggesting that morphine augments chemoreflex activity in unanesthetized rats. Morphine elicited long-lasting decreases in TV, MV and PIF and RT, a long-lasting increase in EF50, a transient decrease in PEF, a substantial, but transient, increase in apneic pause, and an initial increase and then a sustained decrease in NEBI (May et al., 2013a; May et al., 2013b; Young et al., 2013; Baby et al., 2018; Baby S. M. et al., 2021; Gaston et al., 2021; Getsy et al., 2022d). This multi-directional pattern of ventilatory responses points to the disparate role of OR signaling mechanisms in the control of breathing. How quickly effective opioid concentrations reach the multiple target sites will obviously play a major role in the temporal and dose-dependent effects of opioids ventilation. expression. This rate will depend on the (1) plasma concentration, (2) lipophilicity/ability to cross the blood brain barrier (fentanyl crosses the blood-brain barrier faster than morphine), (3) diffusion time to neurons of interest, and (4) synaptic concentrations required to affect different neuron subtypes (Haji et al., 2003). Moreover, Haji et al. (2003) provided compelling evidence that morphine depresses respiratory neuronal activity through two different intracellular mechanisms, both of which are elicited by μ-ORs.
L-CYSme (500 μmol/kg) elicited a rapid reversal of the adverse effects of morphine (10 mg/kg) on ventilation in unanesthetized rats. L-CYSme overcame the effects of morphine on TV, MV, Ti, EIP, inspiratory and expiratory drives, elicited pronounced increases in Freq (at a time when the initial depressant effects of, morphine on Freq had mostly subsided), augmented morphine-induced decreases in Te, and augmented morphine-induced increases in EF50, but did not affect morphine-induced decreases in EEP, RT, NEBI or NEBI/Freq. The first injection of L-CYSme overcame most of the deleterious effects of morphine, whereas the second injection often produced dramatic changes in ventilation. These findings add to knowledge of L-CYSme, including the oxidation of L-CYSme to L-cystine dimethylester (Krämer and Schmidt, 1984), the formation of mixed disulfides with lipoic acid (Ishii et al., 2010), alterations in mechanical properties of dipalmitoyl-phosphatidyl-choline in plasma membranes (Arias et al., 2019), hydrogen bonding to chloride ions (Mosier-Boss and Lieberman, 2005), interactions with myoglobin (Shimizu et al., 1976), redox reduction of cytochrome C (Engman et al., 1994), altered stress-induced changes in cardiac intracellular Ca2+ (Fukui et al., 1994), formation of or one-electron reduction of S-nitrosothiols (Liu et al., 1994; Manoj et al., 2006; Hu and Ho, 2011), regulation of aminotransferases (Pagani et al., 1994), non-enzymatic isomerization of 9-cis-retinoic acid (Shih et al., 1997), disassembly/reassembly of [2Fe-2S] clusters in redox-regulated transcription factor SoxR (Ding and Demple, 1998), the ability to act as substrates for or be oxidized by peroxidases (Svensson, 1988a; Svensson, 1988b; Svensson et al., 1993; Burner et al., 1999), potentiation of glucose-induced insulin release (Ammon et al., 1986), the ability to reduce mucus viscosity (Yanaura et al., 1982), the ability to enter into lungs (Hobbs et al., 1993; Hobbs et al., 1998; Lalley, 2004), and the ability to reduce pulmonary edema (Lailey et al., 1991). We have not determined mechanisms by which L-CYSme exerts its effects against morphine although the lack of immediate effects of L-CYSme on the analgesic/sedative effect of morphine suggest that unlike OR antagonists (van Dorp et al., 2007; Dahan et al., 2010; Boom et al., 2012; Dahan et al., 2018; Algera et al., 2019), the L-thiolester does not block ORs by competitive or allosteric binding. Moreover, establishing that L-cysteine (500 μmol/kg, IV) did not modify any of the actions of morphine suggests that intracellular entry is key to the activity of L-CYSme and that upon entry it exerts its effects as the thiolester itself, or de-esterification to L-cysteine and formation of bioactive L-cysteine derivatives, such as hydrogen sulfide (Wu et al., 2009; Yin et al., 2016; Paul et al., 2018), cysteine-sulfenic, -sulfinic and -sulphonic acids (Yamaguchi and Hosokawa, 1987; Joseph and Maroney, 2007; Stipanuk et al., 2009; Stipanuk et al., 2011) and the S-nitrosothiol, S-nitroso-L-cysteine (Myers et al., 1990; Bates et al., 1991; Foster et al., 2009; Seth and Stamler, 2011; Gaston et al., 2020; Seckler et al., 2020). The lack of effect of L-cysteine may also be due to the ability of morphine to inhibit L-cysteine uptake into neurons via inhibition of excitatory amino acid transporter type 3 (Trivedi et al., 2014; Trivedi and Deth, 2015) although it is unknown whether morphine affects other L-cysteine up-take systems (Tunnicliff, 1994; Shanker and Aschner, 2001; Aoyama et al., 2008; Albrecht and Zielińsk, 2019), including excitatory amino acid transporters 1 and 2 (Zerangue and Kavanaugh, 1996; Chen and Swanson, 2003; Himi et al., 2003; Trivedi et al., 2014; Trivedi and Deth, 2015; Albrecht and Zielińsk, 2019), large neutral amino acid transporters (Simmons-Willis et al., 2002; Nemoto et al., 2003; Li and Whorton, 2007; Granillo et al., 2008), band three protein-anion transport system (Young et al., 1981; Tunnicliff, 1994) and high affinity Na+-dependent glutamate transporters (Hayes et al., 2005).
With respect to where L-CYSme may act to overcome the deleterious effects of morphine on ventilatory parameters, intravenous injection (10 mg/kg, 100 μCi/kg) of 35S-L-CYSme (Wepierre et al., 1964) or 35S-L-CYSee (Servin et al., 1988) resulted in rapid appearance in all tissues, organs and blood. At 5 min, 35S-L-CYSme and 35S-L-CYSee were highest in lungs, kidneys, chest-wall muscle, intestines and brain (only 35S-L-CYSee studied in the brain). Low levels of 35S-L-CYSme and 35S-L-CYSee were observed in blood, likely reflecting rapid dispersal of L-thiolesters into tissues. After 60 min, 35S-L-CYSme and 35S-L-CYSee were heavily seen in lungs, chest-wall, liver, kidneys and intestines, but diminished in brain with a few regions showing strong labeling. In contrast, intravenous injection (10 mg/kg, 100 μCi/kg) of 35S-L-cysteine resulted in intense labeling in kidneys and liver, but not lungs, chest-wall muscle or brain (Servin et al., 1988). As such, L-CYSme may enter central and peripheral structures that mediate the effects of morphine on breathing (Pattinson, 2008; Boom et al., 2012; Henderson et al., 2013; Henderson et al., 2014; Palkovic et al., 2020) thereby overcoming signaling events eliciting OIRD. A second injection of L-CYSme (500 μmol/kg) elicited pronounced/sustained effects on breathing including rises in Freq, TV and MV. These effects contrast those seen with D-CYSee since injection of the D-thiolester (500 μmol/kg) caused an immediate and sustained reversal of the effects of morphine (10 mg/kg) on ventilation, but a second injection elicited minor additional responses (Getsy et al., 2022d). It would appear that higher levels of L-CYSme activate functional proteins and/or enter into metabolic pathways not available to D-CYSee. The findings with L-CYSme add to evidence regarding the efficacy of reduced (monosulfide) thiolesters, such as L-GSHee (Jenkins et al., 2021) and D-CYSee (Getsy et al., 2022c; Getsy et al., 2022d), and oxidized (disulfide) thiolesters, such as D-CYSdiee and D-CYSdime (Gaston et al., 2021) in preventing/overcoming the deleterious actions of morphine or fentanyl on ventilation. The possibility that the mechanisms of action of L-CYSme involves formation of S-nitrosyl forms of L-CYSme and/or L-cysteine is supported by our findings that the ability of morphine or fentanyl to adversely affect ventilation, ABG chemistry and A-a gradient are markedly reduced in rats receiving continuous intravenous infusions of SNO-L-cysteine (Getsy et al., 2022a; Getsy et al., 2022b), whereas the adverse effects of fentanyl (morphine not studied to date) are augmented after inhibition of nitric oxide synthase (Seckler et al., 2022). It is well-studied that SNO-L-cysteine regulates many intracellular signaling cascades (Lipton et al., 1993; Foster et al., 2009; Seth and Stamler, 2011; Stomberski et al., 2019; Gaston et al., 2020), including cardiorespiratory control systems (Davisson et al., 1996; Davisson et al., 1997; Ohta et al., 1997; Lipton et al., 2001; Lewis et al., 2006; Gaston et al., 2020) and those involved in attenuation of OIRD (Getsy et al., 2022a; Getsy et al., 2022b).
Consistent with previous findings (Baby et al., 2018; Baby S. M. et al., 2021; Gaston et al., 2021; Getsy et al., 2022a; Getsy et al., 2022c; Getsy et al., 2022d), morphine (10 mg/kg, IV) elicited pronounced and long-lasting adverse changes in ABG chemistry in unanesthetized rats that consisted of a decrease in arterial blood pH, an increase in pCO2, and decreases in pO2 and sO2, responses consistent with morphine hypoventilation. These changes in ABG chemistry were associated with sustained increases in A-a gradient, suggesting that morphine adversely affected alveolar gas-exchange (Gaston et al., 2021; Getsy et al., 2022a; Getsy et al., 2022c; Getsy et al., 2022d). The elevation in A-a gradient could signify atelectasis (i.e., alveolar collapse) due to hypoventilation, but may also involve more complicated effects on surfactant status/alveolar fluid clearance, which if adversely affected would impair alveolar gas-exchange, despite the morphine-induced decrease in TV. The principal end-result of any therapeutic being developed to overcome OIRD must be restoration of normal ABG chemistry, and so the ability of the therapeutic to drive breathing should not be compromised by untoward effects on other functions driving breathing or gas-exchange. The second important set of findings was that a single injection of L-CYSme (500 μmol/kg, IV), but not L-cysteine (500 μmol/kg, IV), elicited an immediate and sustained reversal of the adverse effects of a 10 mg/kg dose of morphine on ABG chemistry and A-a gradient. As such, the ability of L-CYSme to overcome the effects of morphine on ventilation are not compromised by untoward effects on the upper airway and/or gas exchange as produced by L-CYSee in isoflurane-anesthetized rats (Mendoza et al., 2013), remembering that the co-administration of L-CYSee and morphine does not adversely affect the upper airway in rats anesthetized with sevoflurane (Bates et al., 1991 unpublished findings). Although the abilities of L-CYSme, D-CYSee (Getsy et al., 2022c; Getsy et al., 2022d) and L-CYSee (Mendoza et al., 2013) to overcome the effects of morphine on ventilation and alveolar gas-exchange may involve supply of intracellular reducing equivalents, we found that the powerful reducing/cell-permeable L-thiolester, N-acetyl-L-cysteine methylester (L-NACme) (Tsikas et al., 2018), had minor effects on the ability of morphine to depress ventilation (Gaston et al., 2021). The rapid conversion of L-NACme to L-cysteine in cells (Lailey and Upshall, 1994) argues that the beneficial actions of L-CYSme on morphine OIRD are not due to provision of reducing equivalents in cells or entry of L-cysteine into metabolic pathways. Rather, these findings suggest that the efficacy of L-CYSme, L-GSHee (Jenkins et al., 2021), D-CYSee (Getsy et al., 2022c; Getsy et al., 2022d) and D-cystine di(m)ethylester (Gaston et al., 2021) may involve the intracellular actions of the thiolester moieties themselves. Our findings that intravenous Tempol, a scavenger of free-radicals/superoxide anion, attenuates OIRD elicited by morphine or fentanyl (Baby S. et al., 2021; Baby S. M. et al., 2021) points to the importance of redox mechanisms in opioid actions and possibly the ability of thiolesters to overcome OIRD. The effectiveness of L-CYSme to overcome the adverse effects of morphine in unanesthetized rats and in sevoflurane-anesthetized rats, the most clinically-relevant gaseous anesthetic (Aoki et al., 2021; Apai et al., 2021; Liang et al., 2021), supports the necessity of further studies with L-CYSme in pre-clinical models of OIRD, such as dogs (Walker et al., 1967; Lemmens et al., 2008) or goats (Hubbard et al., 1992; Heard et al., 1996) that bridge potential clinical trials studies in humans.
Intravenous doses of 5 or 10 mg/kg of morphine elicited robust antinociception for over 180 and 240 min in TFL and HPL assays. The antinociception actions of morphine were reduced 160 min post-L-CYSme (180 min post-morphine) as compared to vehicle controls. The 500 μmol/kg dose of L-CYSme in naïve rats increased TFL and HPL at 10 min but not at 20 min. Because no behaviors occurred upon injection, L-CYSme may have elicited short-lived antinociception. The antinociceptive effects of morphine given after L-CYSme were also diminished in duration (evident 2.5 h after 5 mg/kg of morphine and 3.5 h after 10 mg/kg morphine). In contrast, 500 μmol/kg of L-cysteine did not affect TFL, HPL or the actions of morphine. L-cysteine enters cells by several uptake systems (Young et al., 1981; Tunnicliff, 1994; Zerangue and Kavanaugh, 1996; Shanker and Aschner, 2001; Simmons-Willis et al., 2002; Chen and Swanson, 2003; Himi et al., 2003; Nemoto et al., 2003; Hayes et al., 2005; Li and Whorton, 2007; Aoyama et al., 2008; Granillo et al., 2008; Trivedi et al., 2014; Trivedi and Deth, 2015; Albrecht and Zielińsk, 2019). As such, any effects of L-cysteine may have occurred before the 10 min testing time or not enough L-cysteine entered into cells affecting antinociceptive processing. The effects of microinjections of L-cysteine, D-cysteine and L- and D-cystine into the hindpaw (Todorovic et al., 2001; Todorovic et al., 2004; Nelson et al., 2005; Pathirathna et al., 2006; Nelson et al., 2010; Todorovic and Jevtovic-Todorovic, 2014), and sensory cell bodies in dorsal root ganglia (Jagodic et al., 2007) and thalamus (Joksovic et al., 2006) on antinociception in rats have been studied. L-cysteine was pronociceptive, whereas L-cystine was antinociceptive (Todorovic et al., 2001; Todorovic et al., 2004; Nelson et al., 2005; Joksovic et al., 2006; Pathirathna et al., 2006; Jagodic et al., 2007; Nelson et al., 2010; Todorovic and Jevtovic-Todorovic, 2014). Direct intra-dermal microinjections of L-cysteine into the peripheral receptive field (ventral side) of the right hind paw of rats elicited a dose/time-dependent hyperalgesia that was prevented by a neuroactive steroid, 3-βOH, which blocks voltage-dependent T-type Ca2+ channels (Pathirathna et al., 2006). Moreover, L-cysteine caused hyperalgesia upon microinjection into the thalamus via activation of CaV3.2 (Joksovic et al., 2006). The lack of effects of L-cysteine in the present study may be due to lack of rapid/sufficient entry into cells, whereas L-CYSme antinociception may involve extracellular/intracellular actions of the L-thiolester moiety. The initial antinociception responses elicited by 5 and 10 mg/kg morphine were not reduced by L-CYSme suggesting that the L-thiolester did not block ORs in central and/or peripheral pathways that elicit opioid antinociception (Connor and Christie, 1999; Williams et al., 2013; Henderson et al., 2014). However, the decrease in the duration of the antinociceptive actions of morphine by L-CYSme suggests that this L-thiolester does enter these particular pathways to gradually establish intracellular processes that countermand morphine antinociception. L-CYSme may exert antinociceptive actions as the L-thiolester whose effects are counterbalanced by de-esterification to L-cysteine, which as its levels rise, gradually overcomes morphine antinociception by activating voltage-dependent T-type Ca2+ channels (Joksovic et al., 2006; Pathirathna et al., 2006). S-nitroso-L-cysteine diminishes T-type Ca2+ channel activity upon microinjection into the thalamus of rats (Joksovic et al., 2007; Lee et al., 2013). Therefore, L-CYSme may be S-nitrosylated upon entry into nociceptive-antinociceptive pathways to promote morphine antinociception. As the levels of S-nitroso-L-CYSme decrease over time and those of L-cysteine increase, the nociceptive effects of L-cysteine would dominate those of SNO-L-CYSme thereby reducing morphine antinociception.
A limitation of the present study is that we have not tested the efficacy of lower doses of L-CYSme on morphine-induced OIRD. Lower doses may prove effective against OIRD and have less effect on the duration of morphine antinociception. Our evidence that 500 μmol/kg of L-CYSme overcomes the adverse effects of morphine on breathing, ABG chemistry and gas-exchange in alveoli, should be extended to include whether 100 or 250 μmol/kg doses overcome morphine OIRD. Synthetic opioids are playing a major role in the current opioid crisis (Arendt, 2021; Deo et al., 2021) and further investigation needs to include whether L-CYSme can overcome the adverse actions of high potency opioids, such as fentanyl. Another limitation of this study is the lack of data about the efficacy of L- or D-thiolesters in preventing/reversing OIRD in female rats, especially since opioids exert qualitatively and quantitatively different ventilatory responses in females compared to males (Dahan et al., 1998; Hosseini et al., 2011). Yet another limitation is that full dose-response studies with L-CYSme or other L-,D-thiolesters have not been performed that would allow determination of a maximally effective dose, maximal duration of action, and also potentially limiting side-effects in unanesthetized rats. At present, it is unknown whether the different magnitudes of effects of L-CYSme compared to the thiolester, D-CYSee, for example, depend on drug kinetics rather than on efficacy.
Additionally, the lack of understanding about the molecular mechanisms by which L-CYSme affects morphine- and/or fentanyl-induced OIRD also presents a limitation to this study. Aside from possible direct interactions with yet to be determined functional proteins, potential mechanisms of action of L-CYSme may involve (1) direct binding to putative L-, D-cysteine binding protein, a myristoylated alanine-rich C-kinase substrate (Semenza et al., 2021), (2) interruption of OR-β-arrestin-coupled cell signaling processes to spare the antinociceptive G-protein-dependent actions of morphine (Schmid et al., 2017; Grim et al., 2020), and/or (3) potential conversion of L-CYSme to S-nitroso-L-CYSme or S-nitroso-L-cysteine, by S-nitrosylation of the sulfur atom in L-thiolesters via processes requiring nitric oxide synthase (Perissinotti et al., 2005; Hess and Stamler, 2012; Stomberski et al., 2019; Seckler et al., 2022), which may act in a similar way to the intracellular penetrating L-thiolester, S-nitroso-L-cysteine ethyl ester (Clancy et al., 2001). To test the possibility that L-CYSme elicits the production of S-nitrosylated versions of the L-thiolester, we are determining whether intravenous injections of L-CYSme increase production of S-nitrosylated species in blood, peripheral tissues, and brain via the use of an ultra-sensitive capacitive sensor (Seckler et al., 2017) and whether such injections of L-CYSme increase expression of NADPH diaphorase in the brain and peripheral structures, on the basis that NADPH diaphorase visualizes free S-nitrosothiols and S-nitrosylated proteins in aldehyde-treated tissue (Seckler et al., 2020). It is well-known that S-nitrosothiols, such as S-nitroso-L-cysteine and S-nitroso-L-glutathione, play important roles in ventilatory control processes within the brainstem, circulating red blood cells, and peripheral structures, such as the carotid bodies (Gaston et al., 2001; Lipton et al., 2001; Palmer et al., 2013; Gaston et al., 2014; Palmer et al., 2015; Gaston et al., 2020). The ability of morphine or fentanyl to depress breathing and adversely affect ABG chemistry is substantially reduced in rats receiving intravenous infusion of S-nitroso-L-cysteine (Getsy et al., 2022a; Getsy et al., 2022b). The efficacy of S-nitroso-L-cysteine adds to our knowledge regarding the ability of L-S-nitrosothiols to profoundly affect cardiorespiratory control systems (Davisson et al., 1996; Lewis et al., 1996; Travis et al., 1996; Davisson et al., 1997; Ohta et al., 1997; Travis et al., 1997; Gaston et al., 2001; Lewis et al., 2005; Lewis et al., 2006; Gaston et al., 2020). We are currently determining the degree that systemic injections of L-CYSme generate S-nitrosothiols within key cardiorespiratory control brain structures, including the nucleus tractus solitarius, retrotrapezoid nucleus, Kölliker-Fuse nucleus, pre-Bötzinger complex, and key peripheral structures, such as the carotid bodies, diaphragm, chest-wall, and larynx, employing sensor technology (Seckler et al., 2017) and NADPH diaphorase histochemistry (Seckler et al., 2020). To address our lack of understanding of the temporal distribution of L-CYSme in blood and tissues, we are doing pharmacokinetics studies using liquid chromatography-mass spectrometry (Altawallbeh et al., 2019) to determine temporal distributions of L-CYSme in blood, peripheral structures, cerebrospinal fluid, and brain structures relevant to expression of OIRD, including the medulla oblongata, pons and medial prefrontal cortex. We are also testing whether L-CYSme ameliorates the latent adverse effects of morphine on ventilatory responses to hypoxic and hypoxic-hypercapnic (May et al., 2013a; May et al., 2013b) exposures. An important concern is whether the efficacies of OIRD reversal agents in rats will translate into therapies that overcome OIRD in humans. Many such OIRD reversal agents that have demonstrated efficacy in rats lacked such efficacy in human clinical trials (Dahan et al., 2018; Algera et al., 2019). A true limitation also pertains to our lack of understanding as to how L-CYSme reduces the duration of morphine antinociception. The potential mechanisms of action of L-CYSme described above provide us with starting points to explore the mechanisms by which these affects of L-CYSme are exerted.
This study demonstrates that systemic injection of L-CYSme overcame the adverse effects of morphine on breathing, A-a gradient, and ABG chemistry in unanesthetized, unrestrained male Sprague Dawley rats. L-CYSme did not diminish the duration of morphine sedation, whereas it did decrease the duration of morphine antinociception. Since L-cysteine did not exert similar effects to those of L-CYSme, we propose that L-CYSme may overcome the deleterious effects of morphine on ventilation by mechanisms other than direct antagonism of ORs by processes including reversal of OR-initiated intracellular signaling processes and/or by increasing the activity of neurons that were not directly affected by opioids, but which contribute excitatory drive to the respiratory pattern generator. It is possible that administration of L-CYSme may result in a gradual appearance of compounds that overcome the effects of morphine or act as algesic substances. We have not investigated these possibilities, but know that thiols, such as mercaptoethanol, form conjugates with the minor morphine metabolite, morphinone (Yamano et al., 1985). Whether conjugate formation or potential ability of L-thiolesters to augment enzymatic conversion of morphine to major metabolites, such as morphine-3-glucuronide (Blomqvist et al., 2020), contribute to how L-CYSme overcomes the adverse effects of morphine is unknown. It is likely that the ability of L-CYSme to enter the brain and spinal cord likely overcomes the effect of morphine on breathing although peripheral mechanisms may also be involved since peripherally-restricted OR antagonists reduce OIRD (Lewanowitsch and Irvine, 2002; Lewanowitsch et al., 2006; Henderson et al., 2014). Our results with L-CYSme add to knowledge about the efficacy of L,D-thiolesters, such as L-GSHee (Jenkins et al., 2021), D-CYSee (Getsy et al., 2022c; Getsy et al., 2022d), D-cystine di(m)ethylester (Gaston et al., 2021) and the free radical-superoxide anion scavenger, Tempol, against OIRD (Baby S. et al., 2021; Baby S. M. et al., 2021).
The raw data supporting the conclusions of this article will be made available by the authors, without undue reservation.
The animal study was reviewed and approved by the Animal Care and Use Committees of Galleon Pharmaceuticals, Case Western Reserve University, and the University of Virginia.
The study was originated and constructed by SB, JB, BG, Y-HH, and SL. All experiments were performed by PG, WM, SB, CW, and RG. The data were collated and statistically analyzed by TL, SB, PG, CW, JB and SL. The figures and tables were prepared by SB, PG, FC and SL. All authors contributed to the writing of the original version of the manuscript and the revision of the final document that was submitted for publication.
These studies were funded partially by an NIH/NIDA grant (U01DA051373, Optimization of Novel Thiolesters as a Therapeutic Strategy for Combating Opioid Overdoses and Abuse) to SL. In addition, SL received funding for this study from Galleon Pharmaceuticals, Inc.
The authors wish to thank the staff at the animal care facilities at Galleon Pharmaceuticals, Inc., the University of Virginia and Case Western Reserve University for their expert and most caring technical assistance. The authors also wish to thank David Kalergis (CEO, Atelerix Life Sciences) for providing perspectives related to clinical importance of the findings.
The co-author, SB, was employed by Galleon Pharmaceuticals, Inc. The leadership of Galleon Pharmaceuticals was not directly involved in this study as a commercial entity. Only the principal scientists of Galleon Pharmaceuticals were involved in study design, collection, analysis, interpretation of data, writing of this article and the decision to submit it for publication.
The remaining authors declare that the research was conducted in the absence of any commercial or financial relationships that could be construed as a potential conflict of interest.
All claims expressed in this article are solely those of the authors and do not necessarily represent those of their affiliated organizations, or those of the publisher, the editors and the reviewers. Any product that may be evaluated in this article, or claim that may be made by its manufacturer, is not guaranteed or endorsed by the publisher.
The Supplementary Material for this article can be found online at: https://www.frontiersin.org/articles/10.3389/fphar.2022.968378/full#supplementary-material
ABG, arterial blood-gas chemistry; A-a gradient, Alveolar-arterial gradient; BK channel, large conductance Ca2+-activated K+ channel; D-CYSdiee, D-cystine diethylester; D-CYSdime, D-cystine dimethyl ester; EIP, end inspiratory pause; EEP, end expiratory pause; EF50, expiratory flow at 50% expired TV; D-CYSee, D-cysteine ethylester; EAA1-3, type 1–3 excitatory amino acid transporters; freq, frequency of breathing; L-CYSme, L-cysteine methylester; L-CYSee, L-cysteine ethylester; y-L-GCee, L-glutamyl cysteine ethylester; L-GSHee, L-glutathione ethylester; MV, minute ventilation; NEBI, non-eupneic breathing index; OR, opioid receptor; PIF, peak inspiratory flow; PEF, peak expiratory flow; RT, relaxation time; TV, tidal volume; Ti, inspiratory time; Te, expiratory time.
Albrecht, J., and Zielińska, M. (2019). Exchange-mode glutamine transport across CNS cell membranes. Neuropharmacology 161, 107560. doi:10.1016/j.neuropharm.2019.03.003
Algera, M. H., Kamp, J., van der Schrier, R., van Velzen, M., Niesters, M., Aarts, L., et al. (2019). Opioid-induced respiratory depression in humans: A review of pharmacokinetic-pharmacodynamic modelling of reversal. Br. J. Anaesth. 122, e168–e179. doi:10.1016/j.bja.2018.12.023
Altawallbeh, G., Smith, L., Lewis, S. J., Authier, S., Bujold, K., Gaston, B., et al. (2019). Pharmacokinetic study of Sudaxine in dog plasma using novel LC-MS/MS method. Drug Test. Anal. 11, 403–410. doi:10.1002/dta.2507
Ammon, H. P., Hehl, K. H., Enz, G., Setiadi-Ranti, A., and Verspohl, E. J. (1986). Cysteine analogues potentiate glucose-induced insulin release in vitro. Diabetes 35, 1390–1396. doi:10.2337/diab.35.12.1390
Aoki, N., Suwa, T., Kawashima, H., Tajika, A., Sunada, N., Shimizu, T., et al. (2021). Sevoflurane in electroconvulsive therapy: A systematic review and meta-analysis of randomised trials. J. Psychiatr. Res. 141, 16–25. doi:10.1016/j.jpsychires.2021.06.030
Aoyama, K., Watabe, M., and Nakaki, T. (2008). Regulation of neuronal glutathione synthesis. J. Pharmacol. Sci. 108, 227–238. doi:10.1254/jphs.08r01cr
Apai, C., Shah, R., Tran, K., and Pandya Shah, S. (2021). Anesthesia and the developing brain: A review of sevoflurane-induced neurotoxicity in pediatric populations. Clin. Ther. 43, 762–778. doi:10.1016/j.clinthera.2021.01.024
Arendt, F. (2021). The opioid-overdose crisis and fentanyl: The role of online information seeking via internet search engines. Health Commun. 36, 1148–1154. doi:10.1080/10410236.2020.1748820
Arias, J. M., Díaz, S. B., Ben Altabef, A., Dupuy, F. G., and Ben AltAbef, A. (2019). Interaction of cysteine and its derivatives with monolayers of dipalmitoylphosphatidylcholine. Colloids Surf. B Biointerfaces 184, 110548. doi:10.1016/j.colsurfb.2019.110548
Auclair, J. R., Brodkin, H. R., D'Aquino, J. A., Petsko, G. A., Ringe, D., and Agar, J. N. (2013). Structural consequences of cysteinylation of Cu/Zn-superoxide dismutase. Biochemistry 52, 6145–6150. doi:10.1021/bi400613h
Baby, S., Gruber, R., Discala, J., Puskovic, V., Jose, N., Cheng, F., et al. (2021). Systemic administration of Tempol attenuates the cardiorespiratory depressant effects of fentanyl. Front. Pharmacol. 12, 690407. doi:10.3389/fphar.2021.690407
Baby, S. M., Discala, J. F., Gruber, R., Getsy, P. M., Cheng, F., Damron, D. S., et al. (2021). Tempol reverses the negative effects of morphine on arterial blood-gas chemistry and tissue oxygen saturation in freely-moving rats. Front. Pharmacol. 12, 749084. doi:10.3389/fphar.2021.749084
Baby, S. M., Gruber, R. B., Young, A. P., MacFarlane, P. M., Teppema, L. J., and Lewis, S. J. (2018). Bilateral carotid sinus nerve transection exacerbates morphine-induced respiratory depression. Eur. J. Pharmacol. 834, 17–29. doi:10.1016/j.ejphar.2018.07.018
Bachmutsky, I., Wei, X. P., Kish, E., and Yackle, K. (2020). Opioids depress breathing through two small brainstem sites. Elife 9, e52694. doi:10.7554/eLife.52694
Ballanyi, K., Lalley, P. M., Hoch, B., and Richter, D. W. (1997). cAMP-dependent reversal of opioid- and prostaglandin-mediated depression of the isolated respiratory network in newborn rats. J. Physiol. 504, 127–134. doi:10.1111/j.1469-7793.1997.127bf.x
Baronas, V. A., Yang, R. Y., and Kurata, H. T. (2017). Extracellular redox sensitivity of Kv1.2 potassium channels. Sci. Rep. 7, 9142. doi:10.1038/s41598-017-08718-z
Bates, J. N., Harrison, D. G., Myers, P. R., and Minor, R. L. (1991). EDRF: Nitrosylated compound or authentic nitric oxide. Basic Res. Cardiol. 86, 17–26. doi:10.1007/978-3-642-72461-9_3
Belcastro, E., Gaucher, C., Corti, A., Leroy, P., Lartaud, I., and Pompella, A. (2017). Regulation of protein function by S-nitrosation and S-glutathionylation: Processes and targets in cardiovascular pathophysiology. Biol. Chem. 398, 1267–1293. doi:10.1515/hsz-2017-0150
Bełtowski, J. (2019). Synthesis, metabolism, and signaling mechanisms of hydrogen sulfide: An overview. Methods Mol. Biol. 2007, 1–8. doi:10.1007/978-1-4939-9528-8_1
Blomqvist, K. J., Viisanen, H., Ahlström, F. H. G., Jokinen, V., Sidorova, Y. A., Suleymanova, I., et al. (2020). Morphine-3-glucuronide causes antinociceptive cross-tolerance to morphine and increases spinal substance P expression. Eur. J. Pharmacol. 875, 173021. doi:10.1016/j.ejphar.2020.173021
Bogeski, I., Kappl, R., Kummerow, C., Gulaboski, R., Hoth, M., and Niemeyer, B. A. (2011). Redox regulation of calcium ion channels: Chemical and physiological aspects. Cell. Calcium 50, 407–423. doi:10.1016/j.ceca.2011.07.006
Bogeski, I., and Niemeyer, B. A. (2014). Redox regulation of ion channels. Antioxid. Redox Signal. 21, 859–862. doi:10.1089/ars.2014.6019
Boghosian, J. D., Luethy, A., and Cotton, J. F. (2018). Intravenous and intratracheal thyrotropin releasing hormone and its analog taltirelin reverse opioid-induced respiratory depression in isoflurane anesthetized rats. J. Pharmacol. Exp. Ther. 366, 105–112. doi:10.1124/jpet.118.248377
Bonifácio, V. D. B., Pereira, S. A., Serpa, J., and Vicente, J. B. (2021). Cysteine metabolic circuitries: Druggable targets in cancer. Br. J. Cancer 124, 862–879. doi:10.1038/s41416-020-01156-1
Boom, M., Niesters, M., Sarton, E., Aarts, L., Smith, T. W., and Dahan, A. (2012). Non-analgesic effects of opioids: Opioid-induced respiratory depression. Curr. Pharm. Des. 18, 5994–6004. doi:10.2174/138161212803582469
Burner, U., Jantschko, W., and Obinger, C. (1999). Kinetics of oxidation of aliphatic and aromatic thiols by myeloperoxidase compounds I and II. FEBS Lett. 443, 290–296. doi:10.1016/s0014-5793(98)01727-x
Butterworth, M., Upshall, D. G., and Cohen, G. M. (1993). A novel role for carboxylesterase in the elevation of cellular cysteine by esters of cysteine. Biochem. Pharmacol. 46, 1131–1137. doi:10.1016/0006-2952(93)90460-e
Carstens, E., and Wilson, C. (1993). Rat tail flick reflex: Magnitude measurement of stimulus-response function, suppression by morphine and habituation. J. Neurophysiol. 70, 630–639. doi:10.1152/jn.1993.70.2.630
Cavalla, D., Chianelli, F., Korsak, A., Hosford, P. S., Gourine, A. V., and Marina, N. (2015). Tianeptine prevents respiratory depression without affecting analgesic effect of opiates in conscious rats. Eur. J. Pharmacol. 761, 268–272. doi:10.1016/j.ejphar.2015.05.067
Chapman, C. D., Dono, L. M., French, M. C., Weinberg, Z. Y., Schuette, L. M., and Currie, P. J. (2012). Paraventricular nucleus anandamide signaling alters eating and substrate oxidation. Neuroreport 23, 425–429. doi:10.1097/WNR.0b013e32835271d1
Chen, Y., and Swanson, R. A. (2003). The glutamate transporters EAAT2 and EAAT3 mediate cysteine uptake in cortical neuron cultures. J. Neurochem. 84, 1332–1339. doi:10.1046/j.1471-4159.2003.01630.x
Chevillard, L., Mégarbane, B., Risède, P., and Baud, F. J. (2009). Characteristics and comparative severity of respiratory response to toxic doses of fentanyl, methadone, morphine, and buprenorphine in rats. Toxicol. Lett. 191, 327–340. doi:10.1016/j.toxlet.2009.09.017
Clancy, R., Cederbaum, A. I., and Stoyanovsky, D. A. (2001). Preparation and properties of S-nitroso-L-cysteine ethyl ester, an intracellular nitrosating agent. J. Med. Chem. 44, 2035–2038. doi:10.1021/jm000463f
Connor, M., and Christie, M. D. (1999). Opioid receptor signalling mechanisms. Clin. Exp. Pharmacol. Physiol. 26, 493–499. doi:10.1046/j.1440-1681.1999.03049.x
Dahan, A., Aarts, L., and Smith, T. W. (2010). Incidence, reversal, and prevention of opioid-induced respiratory depression. Anesthesiology 112, 226–238. doi:10.1097/ALN.0b013e3181c38c25
Dahan, A., Sarton, E., Teppema, L., and Olievier, C. (1998). Sex-related differences in the influence of morphine on ventilatory control in humans. Anesthesiology 88, 903–913. doi:10.1097/00000542-199804000-00009
Dahan, A., van der Schrier, R., Smith, T., Aarts, L., van Velzen, M., and Niesters, M. (2018). Averting opioid-induced respiratory depression without affecting analgesia. Anesthesiology 128, 1027–1037. doi:10.1097/ALN.0000000000002184
Dai, W., Gao, X., Xiao, D., Li, Y. L., Zhou, X. B., Yong, Z., et al. (2019). The impact and mechanism of a novel allosteric AMPA receptor modulator LCX001 on protection against respiratory depression in rodents. Front. Pharmacol. 10, 105. doi:10.3389/fphar.2019.00105
Dai, W., Xiao, D., Gao, X., Zhou, X. B., Fang, T. Y., Yong, Z., et al. (2017). A brain-targeted ampakine compound protects against opioid-induced respiratory depression. Eur. J. Pharmacol. 809, 122–129. doi:10.1016/j.ejphar.2017.05.025
Davisson, R. L., Travis, M. D., Bates, J. N., Johnson, A. K., and Lewis, S. J. (1997). Stereoselective actions of S-nitrosocysteine in central nervous system of conscious rats. Am. J. Physiol. 272, H2361–H2368. doi:10.1152/ajpheart.1997.272.5.H2361
Davisson, R. L., Travis, M. D., Bates, J. N., and Lewis, S. J. (1996). Hemodynamic effects of L- and D-S-nitrosocysteine in the rat. Stereoselective S-nitrosothiol recognition sites. Circ. Res. 79, 256–262. doi:10.1161/01.res.79.2.256
Deo, V. S., Gilson, T. P., Kaspar, C., and Singer, M. E. (2021). The fentanyl phase of the opioid epidemic in Cuyahoga County, Ohio, United States. J. Forensic Sci. 66, 926–933. doi:10.1111/1556-4029.14665
Ding, H., and Demple, B. (1998). Thiol-mediated disassembly and reassembly of [2Fe-2S] clusters in the redox-regulated transcription factor SoxR. Biochemistry 37, 17280–17286. doi:10.1021/bi980532g
Dutschmann, M., Waki, H., Manzke, T., Simms, A. E., Pickering, A. E., Richter, D. W., et al. (2009). The potency of different serotonergic agonists in counteracting opioid evoked cardiorespiratory disturbances. Philos. Trans. R. Soc. Lond. B Biol. Sci. 364, 2611–2623. doi:10.1098/rstb.2009.0076
Eguchi, K., Tadaki, E., Simbulan, D., and Kumazawa, T. (1987). Respiratory depression caused by either morphine microinjection or repetitive electrical stimulation in the region of the nucleus parabrachialis of cats. Pflugers Arch. 409, 367–373. doi:10.1007/BF00583790
Elmalem, E., Chorev, M., and Weinstock, M. (1991). Antagonism of morphine-induced respiratory depression by novel anticholinesterase agents. Neuropharmacology 30, 1059–1064. doi:10.1016/0028-3908(91)90134-w
Engman, L., Tunek, A., Hallberg, M., and Hallberg, A. (1994). Catalytic effects of glutathione peroxidase mimetics on the thiol reduction of cytochrome c. Chem. Biol. Interact. 93, 129–137. doi:10.1016/0009-2797(94)90092-2
Epstein, M. A., and Epstein, R. A. (1978). A theoretical analysis of the barometric method for measurement of tidal volume. Respir. Physiol. 32, 105–120. doi:10.1016/0034-5687(78)90103-2
Epstein, R. A., Epstein, M. A., Haddad, G. G., and Mellins, R. B. (1980). Practical implementation of the barometric method for measurement of tidal volume. J. Appl. Physiol. Respir. Environ. Exerc. Physiol. 49, 1107–1115. doi:10.1152/jappl.1980.49.6.1107
Fone, K. C., and Wilson, H. (1986). The effects of alfentanil and selected narcotic analgesics on the rate of action potential discharge of medullary respiratory neurones in anaesthetized rats. Br. J. Pharmacol. 89, 67–76. doi:10.1111/j.1476-5381.1986.tb11121.x
Foster, M. W., Hess, D. T., and Stamler, J. S. (2009). Protein S-nitrosylation in health and disease: A current perspective. Trends Mol. Med. 15, 391–404. doi:10.1016/j.molmed.2009.06.007
Fukui, K., Kaneda, M., Takahashi, E., Washio, M., and Doi, K. (1994). Protective effects of sulfhydryl compounds on HOCl-induced intracellular Ca2+ increase in single rat ventricular myocytes. J. Mol. Cell. Cardiol. 26, 455–461. doi:10.1006/jmcc.1994.1056
Gamper, N., and Ooi, L. (2015). Redox and nitric oxide-mediated regulation of sensory neuron ion channel function. Antioxid. Redox Signal. 22, 486–504. doi:10.1089/ars.2014.5884
Gao, L., González-Rodríguez, P., Ortega-Sáenz, P., and López-Barneo, J. (2017). Redox signaling in acute oxygen sensing. Redox Biol. 12, 908–915. doi:10.1016/j.redox.2017.04.033
García, I. E., Sánchez, H. A., Martínez, A. D., and Retamal, M. A. (2018). Redox-mediated regulation of connexin proteins; focus on nitric oxide. Biochim. Biophys. Acta. Biomembr. 1860, 91–95. doi:10.1016/j.bbamem.2017.10.006
Gaston, B., Baby, S. M., May, W. J., Young, A. P., Grossfield, A., Bates, J. N., et al. (2021). D-Cystine di(m)ethyl ester reverses the deleterious effects of morphine on ventilation and arterial blood gas chemistry while promoting antinociception. Sci. Rep. 11, 10038. doi:10.1038/s41598-021-89455-2
Gaston, B., May, W. J., Sullivan, S., Yemen, S., Marozkina, N. V., Palmer, L. A., et al. (2014). Essential role of hemoglobin beta-93-cysteine in post-hypoxia facilitation of breathing in conscious mice. J. Appl. Physiol. 116, 1290–1299. doi:10.1152/japplphysiol.01050.2013
Gaston, B., Singel, D., Doctor, A., and Stamler, J. S. (2006). S-nitrosothiol signaling in respiratory biology. Am. J. Respir. Crit. Care Med. 173, 1186–1193. doi:10.1164/rccm.200510-1584PP
Gaston, B., Smith, L., Bosch, J., Seckler, J., Kunze, D., Kiselar, J., et al. (2020). Voltage-gated potassium channel proteins and stereoselective S-nitroso-l-cysteine signaling. JCI Insight 5, e134174. doi:10.1172/jci.insight.134174
Getsy, P. M., Baby, S. M., Gruber, R. B., Gaston, B., Lewis, T. H. J., Grossfield, A., et al. (2022b). S-nitroso-L-cysteine stereoselectively blunts the negative effects of fentanyl on breathing while augmenting fentanyl antinociception in freely-moving rats. Front. Pharmacol. 13, 892307. doi:10.3389/fphar.2022.892307
Getsy, P. M., Baby, S. M., May, W. J., Young, A. P., Gaston, B., Hodges, M. R., et al. (2022c). D-Cysteine ethyl ester reverses the deleterious effects of morphine on breathing in freely-moving rats while not negating the antinociceptive effects of the opioid. Front. Physiol. 13, 883329. doi:10.3389/fphar.2022.883329
Getsy, P. M., Coffee, G. A., and Lewis, S. J. (2020). The role of carotid sinus nerve input in the hypoxic-hypercapnic ventilatory response in juvenile rats. Front. Physiol. 11, 613786. doi:10.3389/fphys.2020.613786
Getsy, P. M., Davis, J., Coffee, G. A., May, W. J., Palmer, L. A., Strohl, K. P., et al. (2014). Enhanced non-eupneic breathing following hypoxic, hypercapnic or hypoxic-hypercapnic gas challenges in conscious mice. Respir. Physiol. Neurobiol. 204, 147–159. doi:10.1016/j.resp.2014.09.006
Getsy, P. M., Sundararajan, S., May, W. J., von Schill, G. C., McLaughlin, D. K., Palmer, L. A., et al. (2021). Short-term facilitation of breathing upon cessation of hypoxic challenge is impaired in male but not female endothelial NOS knock-out mice. Sci. Rep. 11, 18346. doi:10.1038/s41598-021-97322-3
Getsy, P. M., Young, A. P., Gaston, B., Bates, J. N., Baby, S. M., Seckler, J. M., et al. (2022a). S-Nitroso-L-Cysteine stereoselectively blunts the negative effects of morphine on breathing and arterial blood gas chemistry while promoting analgesia. Front. Pharmacol. 153, 113436. doi:10.1016/j.biopha.2022.113436
Getsy, P. M., Young, A. P., Grossfield, A., Seckler, J. M., Wilson, C. G., Gaston, B., et al. (2022d). D-Cysteine ethylester and D-cystine dimethylester reverse the deleterious effects of morphine on arterial blood-gas chemistry and Alveolar-arterial gradient in anesthetized rats. Resp. Physiol. Neurobiol. 302, 103912. doi:10.1016/j.resp.2022.103912
Ghezzi, P., and Chan, P. (2017). Redox proteomics applied to the thiol secretome. Antioxid. Redox Signal. 26, 299–312. doi:10.1089/ars.2016.6732
Golder, F. J., Dax, S., Baby, S. M., Gruber, R., Hoshi, T., Ideo, C., et al. (2015). Identification and characterization of GAL-021 as a novel breathing control modulator. Anesthesiology 123, 1093–1104. doi:10.1097/ALN.0000000000000844
Granillo, O. M., Brahmajothi, M. V., Li, S., Whorton, A. R., Mason, S. N., McMahon, T. J., et al. (2008). Pulmonary alveolar epithelial uptake of S-nitrosothiols is regulated by L-type amino acid transporter. Am. J. Physiol. Lung Cell. Mol. Physiol. 295, L38–L43. doi:10.1152/ajplung.00280.2007
Greer, J. J., and Ren, J. (2009). Ampakine therapy to counter fentanyl-induced respiratory depression. Respir. Physiol. Neurobiol. 168, 153–157. doi:10.1016/j.resp.2009.02.011
Grim, T. W., Acevedo-Canabal, A., and Bohn, L. M. (2020). Toward directing opioid receptor signaling to refine opioid therapeutics. Biol. Psychiatry 87, 15–21. doi:10.1016/j.biopsych.2019.10.020
Guenther, U., Manzke, T., Wrigge, H., Dutschmann, M., Zinserling, J., Putensen, C., et al. (2009). The counteraction of opioid-induced ventilatory depression by the serotonin 1A-agonist 8-OH-DPAT does not antagonize antinociception in rats in situ and in vivo. Anesth. Analg. 108, 1169–1176. doi:10.1213/ane.0b013e318198f828
Guenther, U., Theuerkauf, N. U., Huse, D., Boettcher, M. F., Wensing, G., Putensen, C., et al. (2012). Selective 5-HT1A-R-agonist repinotan prevents remifentanil-induced ventilatory depression and prolongs antinociception. Anesthesiology 116, 56–64. doi:10.1097/ALN.0b013e31823d08fa
Haji, A., Okazaki, M., Ohi, Y., Yamazaki, H., and Takeda, R. (2003). Biphasic effects of morphine on bulbar respiratory neuronal activities in decerebrate cats. Neuropharmacology 45, 368–379. doi:10.1016/s0028-3908(03)00154-0
Hamelmann, E., Schwarze, J., Takeda, K., Oshiba, A., Larsen, G. L., Irvin, C. G., et al. (1997). Noninvasive measurement of airway responsiveness in allergic mice using barometric plethysmography. Am. J. Respir. Crit. Care Med. 156, 766–775. doi:10.1164/ajrccm.156.3.9606031
Hargreaves, K., Dubner, R., Brown, F., Flores, C., and Joris, J. (1988). A new and sensitive method for measuring thermal nociception in cutaneous hyperalgesia. Pain 32, 77–88. doi:10.1016/0304-3959(88)90026-7
Hassen, A. H., Feuerstein, G., Pfeiffer, A., and Faden, A. I. (1982). Delta versus mu receptors: Cardiovascular and respiratory effects of opiate agonists microinjected into nucleus tractus solitarius of cats. Regul. Pept. 4, 299–309. doi:10.1016/0167-0115(82)90140-9
Hatfield, M. J., Umans, R. A., Hyatt, J. L., Edwards, C. C., Wierdl, M., Tsurkan, L., et al. (2016). Carboxylesterases: General detoxifying enzymes. Chem. Biol. Interact. 259, 327–331. doi:10.1016/j.cbi.2016.02.011
Haw, A. J., Meyer, L. C., Greer, J. J., and Fuller, A. (2016). Ampakine CX1942 attenuates opioid-induced respiratory depression and corrects the hypoxaemic effects of etorphine in immobilized goats (Capra hircus). Vet. Anaesth. Analg. 43, 528–538. doi:10.1111/vaa.12358
Hayes, D., Wiessner, M., Rauen, T., and McBean, G. J. (2005). Transport of L-[14C]cystine and L-[14C]cysteine by subtypes of high affinity glutamate transporters over-expressed in HEK cells. Neurochem. Int. 46, 585–594. doi:10.1016/j.neuint.2005.03.001
Heard, D. J., Nichols, W. W., Buss, D., and Kollias, G. V. (1996). Comparative cardiopulmonary effects of intramuscularly administered etorphine and carfentanil in goats. Am. J. Vet. Res. 57, 87–96.
Henderson, F., May, W. J., Gruber, R. B., Discala, J. F., Puskovic, V., Young, A. P., et al. (2014). Role of central and peripheral opiate receptors in the effects of fentanyl on analgesia, ventilation and arterial blood-gas chemistry in conscious rats. Respir. Physiol. Neurobiol. 191, 95–105. doi:10.1016/j.resp.2013.11.005
Henderson, F., May, W. J., Gruber, R. B., Young, A. P., Palmer, L. A., Gaston, B., et al. (2013). Low-dose morphine elicits ventilatory excitant and depressant responses in conscious rats: Role of peripheral μ-opioid receptors. Open J. Mol. Integr. Physiol. 3, 111–124. doi:10.4236/ojmip.2013.33017
Henderson, M., Rice, B., Sebastian, A., Sullivan, P. G., King, C., Robinson, R. A., et al. (2016). Neuroproteomic study of nitrated proteins in moderate traumatic brain injured rats treated with gamma glutamyl cysteine ethyl ester administration post injury: Insight into the role of glutathione elevation in nitrosative stress. Proteomics. Clin. Appl. 10, 1218–1224. doi:10.1002/prca.201600004
Hess, D. T., and Stamler, J. S. (2012). Regulation by S-nitrosylation of protein post-translational modification. J. Biol. Chem. 287, 4411–4418. doi:10.1074/jbc.R111.285742
Himi, T., Ikeda, M., Yasuhara, T., Nishida, M., and Morita, I. (2003). Role of neuronal glutamate transporter in the cysteine uptake and intracellular glutathione levels in cultured cortical neurons. J. Neural Transm. 110, 1337–1348. doi:10.1007/s00702-003-0049-z
Hobbs, M. J., Butterworth, M., Cohen, G. M., and Upshall, D. G. (1993). Structure-activity relationships of cysteine esters and their effects on thiol levels in rat lung in vitro. Biochem. Pharmacol. 45, 1605–1612. doi:10.1016/0006-2952(93)90301-c
Hobbs, M. J., Williams, N. E., Patel, S. K., and Upshall, D. G. (1998). Elevation of endogenous nucleophiles in rat lung by cysteine and glutathione esters in vitro. Biochem. Pharmacol. 55, 1573–1584. doi:10.1016/s0006-2952(97)00674-6
Hosseini, M., Taiarani, Z., Hadjzadeh, M. A., Salehabadi, S., Tehranipour, M., and Alaei, H. A. (2011). Different responses of nitric oxide synthase inhibition on morphine-induced antinociception in male and female rats. Pathophysiology 18, 143–149. doi:10.1016/j.pathophys.2010.05.004
Hu, T. M., and Ho, S. C. (2011). Similarity and dissimilarity of thiols as anti-nitrosative agents in the nitric oxide-superoxide system. Biochem. Biophys. Res. Commun. 404, 785–789. doi:10.1016/j.bbrc.2010.12.059
Hubbard, J. W., Locke, K. W., Forster, H. V., Brice, A. G., Pan, L. G., Lowry, T. F., et al. (1992). Cardiorespiratory effects of the novel opioid analgesic HP 736 in the anesthetized dog and conscious goat. J. Pharmacol. Exp. Ther. 260, 1268–1277.
Hutchinson, M. R., Northcutt, A. L., Chao, L. W., Kearney, J. J., Zhang, Y., Berkelhammer, D. L., et al. (2008). Minocycline suppresses morphine-induced respiratory depression, suppresses morphine-induced reward, and enhances systemic morphine-induced analgesia. Brain Behav. Immun. 22, 1248–1256. doi:10.1016/j.bbi.2008.07.008
Iniushkin, A. N. (1997). Respiratory and hemodynamic responses to microinjections of opioids into the solitary tract nucleus. Ross. Fiziol. Zh. Im. I. M. Sechenova 83, 112–121.
Ishii, T., Wakabayashi, M., Mori, T., and Nakayama, T. (2010). A new method for the detection and characterization of alpha-lipoic acid mixed disulphides. Free Radic. Res. 44, 403–409. doi:10.3109/10715760903536331
Imam, M. Z., Kuo, A., and Smith, M. T. (2020). Countering opioid-induced respiratory depression by non-opioids that are respiratory stimulants. F1000Res 9, F1000 Faculty Rev-91. doi:10.12688/f1000research.21738.1
Jagodic, M. M., Pathirathna, S., Nelson, M. T., Mancuso, S., Joksovic, P. M., Rosenberg, E. R., et al. (2007). Cell-specific alterations of T-type calcium current in painful diabetic neuropathy enhance excitability of sensory neurons. J. Neurosci. 27, 3305–3316. doi:10.1523/JNEUROSCI.4866-06.2007
Jenkins, M. W., Khalid, F., Baby, S. M., May, W. J., Young, A. P., Bates, J. N., et al. (2021). Glutathione ethyl ester reverses the deleterious effects of fentanyl on ventilation and arterial blood-gas chemistry while prolonging fentanyl-induced analgesia. Sci. Rep. 11, 6985. doi:10.1038/s41598-021-86458-x
Joksovic, P. M., Doctor, A., Gaston, B., and Todorovic, S. M. (2007). Functional regulation of T-type calcium channels by s-nitrosothiols in the rat thalamus. J. Neurophysiol. 97, 2712–2721. doi:10.1152/jn.00926.2006
Joksovic, P. M., Nelson, M. T., Jevtovic-Todorovic, V., Patel, M. K., Perez-Reyes, E., Campbell, K. P., et al. (2006). CaV3.2 is the major molecular substrate for redox regulation of T-type Ca2+ channels in the rat and mouse thalamus. J. Physiol. 574, 415–430. doi:10.1113/jphysiol.2006.110395
Jonkman, K., van Rijnsoever, E., Olofsen, E., Aarts, L., Sarton, E., van Velzen, M., et al. (2018). Esketamine counters opioid-induced respiratory depression. Br. J. Anaesth. 120, 1117–1127. doi:10.1016/j.bja.2018.02.021
Joseph, C. A., and Maroney, M. J. (2007). Cysteine dioxygenase: Structure and mechanism. Chem. Commun. 32, 3338–3349. doi:10.1039/b702158e
Kasaba, T., Takeshita, M., and Takasaki, M. (1997). The effects of caffeine on the respiratory depression by morphine. Masui. 46, 1570–1574.
Kimura, H. (2017). Hydrogen sulfide and polysulfide signaling. Antioxid. Redox Signal. 27, 619–621. doi:10.1089/ars.2017.7076
Kimura, H. (2014). The physiological role of hydrogen sulfide and beyond. Nitric Oxide 41, 4–10. doi:10.1016/j.niox.2014.01.002
Kimura, S., Ohi, Y., and Haji, A. (2015). Blockade of phosphodiesterase 4 reverses morphine-induced ventilatory disturbance without loss of analgesia. Life Sci. 127, 32–38. doi:10.1016/j.lfs.2015.02.006
Krämer, E., and Schmidt, A. (1984). Oxidation of cysteine to cystine by membrane fractions of Chlorella fusca. Planta 160, 235–241. doi:10.1007/BF00402860
Lailey, A. F., Hill, L., Lawston, I. W., Stanton, D., and Upshall, D. G. (1991). Protection by cysteine esters against chemically induced pulmonary oedema. Biochem. Pharmacol. 42, S47–S54. doi:10.1016/0006-2952(91)90391-h
Lailey, A. F., and Upshall, D. G. (1994). Thiol levels in rat bronchio-alveolar lavage fluid after administration of cysteine esters. Hum. Exp. Toxicol. 13, 776–780. doi:10.1177/096032719401301106
Lalley, P. M. (2005). D1-dopamine receptor agonists prevent and reverse opiate depression of breathing but not antinociception in the cat. Am. J. Physiol. Regul. Integr. Comp. Physiol. 289, R45–R51. doi:10.1152/ajpregu.00868.2004
Lalley, P. M. (2004). Dopamine1 receptor agonists reverse opioid respiratory network depression, increase CO2 reactivity. Respir. Physiol. Neurobiol. 139, 247–262. doi:10.1016/j.resp.2003.10.007
Lalley, P. M., Pilowsky, P. M., Forster, H. V., and Zuperku, E. J. (2014). CrossTalk opposing view: The pre-Botzinger complex is not essential for respiratory depression following systemic administration of opioid analgesics. J. Physiol. 592, 1163–1166. doi:10.1113/jphysiol.2013.258830
Lash, L. H., and Jones, D. P. (1985). Distribution of oxidized and reduced forms of glutathione and cysteine in rat plasma. Arch. Biochem. Biophys. 240, 583–592. doi:10.1016/0003-9861(85)90065-7
Lee, J., Nelson, M. T., Rose, K. E., and Todorovic, S. M. (2013). Redox mechanism of S-nitrosothiol modulation of neuronal CaV3.2 T-type calcium channels. Mol. Neurobiol. 48, 274–280. doi:10.1007/s12035-013-8493-8
Lemmens, S., Stienen, P. J., Jaramillo, L. G., Doornenbal, A., and Hellebrekers, L. J. (2008). The cardiorespiratory effects of a fentanyl infusion following acepromazine and glycopyrrolate in dogs. Tijdschr. Diergeneeskd. 133, 888–895.
Lewanowitsch, T., and Irvine, R. J. (2002). Naloxone methiodide reverses opioid-induced respiratory depression and analgesia without withdrawal. Eur. J. Pharmacol. 445, 61–67. doi:10.1016/s0014-2999(02)01715-6
Lewanowitsch, T., Miller, J. H., and Irvine, R. J. (2006). Reversal of morphine, methadone and heroin induced effects in mice by naloxone methiodide. Life Sci. 78, 682–688. doi:10.1016/j.lfs.2005.05.062
Lewis, S. J., Hoque, A., and Bates, J. N. (2005). Differentiation of L- and D-S-nitrosothiol recognition sites in vivo. J. Cardiovasc. Pharmacol. 46, 660–671. doi:10.1097/01.fjc.0000181714.94827.5d
Lewis, S. J., Meller, S. T., Brody, M. J., and Gebhart, G. F. (1991). Reduced nociceptive effects of intravenous serotonin (5-HT) in the spontaneously hypertensive rat. Clin. Exp. Hypertens. A 13, 849–857. doi:10.3109/10641969109042089
Lewis, S. J., Owen, J. R., and Bates, J. N. (2006). S-nitrosocysteine elicits hemodynamic responses similar to those of the Bezold-Jarisch reflex via activation of stereoselective recognition sites. Eur. J. Pharmacol. 531, 254–258. doi:10.1016/j.ejphar.2005.11.027
Lewis, S. J., Travis, M. D., and Bates, J. N. (1996). Stereoselective S-nitrosocysteine recognition sites in rat brain. Eur. J. Pharmacol. 312, R3–R5. doi:10.1016/0014-2999(96)00607-3
Lipton, A. J., Johnson, M. A., Macdonald, T., Lieberman, M. W., Gozal, D., and Gaston, B. (2007). S-nitrosothiols signal the ventilatory response to hypoxia. Nature 413, 171–174. doi:10.1038/35093117
Li, S., and Whorton, A. R. (2007). Functional characterization of two S-nitroso-L-cysteine transporters, which mediate movement of NO equivalents into vascular cells. Am. J. Physiol. Cell. Physiol. 292, C1263–C1271. doi:10.1152/ajpcell.00382.2006
Li, W. M., Sato, A., Sato, Y., and Schmidt, R. F. (1996). Morphine microinjected into the nucleus tractus solitarius and rostral ventrolateral medullary nucleus enhances somatosympathetic A- and C- reflexes in anesthetized rats. Neurosci. Lett. 221, 53–56. doi:10.1016/s0304-3940(96)13286-9
Li, Y., Su, L., Li, F., Wang, C., Yuan, D., Chen, J., et al. (2015). Acute and sub-chronic toxicity of glucose-cysteine Maillard reaction products in Sprague-Dawley rats. Food Chem. Toxicol. 80, 271–276. doi:10.1016/j.fct.2015.03.021
Liang, T. Y., Peng, S. Y., Ma, M., Li, H. Y., Wang, Z., and Chen, G. (2021). Protective effects of sevoflurane in cerebral ischemia reperfusion injury: A narrative review. Med. Gas. Res. 11, 152–154. doi:10.4103/2045-9912.318860
Liang, X., Yong, Z., and Su, R. (2018). Inhibition of protein kinase A and GIRK channel reverses fentanyl-induced respiratory depression. Neurosci. Lett. 677, 14–18. doi:10.1016/j.neulet.2018.04.029
Lipton, A. J., Johnson, M. A., Macdonald, T., Lieberman, M. W., Gozal, D., and Gaston, B. (2001). S-nitrosothiols signal the ventilatory response to hypoxia. Nature 413, 171–174. doi:10.1038/35093117
Lipton, S. A., Choi, Y. B., Pan, Z. H., Lei, S. Z., Chen, H. S., Sucher, N. J., et al. (1993). A redox-based mechanism for the neuroprotective and neurodestructive effects of nitric oxide and related nitroso-compounds. Nature 364, 626–632. doi:10.1038/364626a0
Liu, X., Gillespie, J. S., and Martin, W. (1994). Non-adrenergic, non-cholinergic relaxation of the bovine retractor penis muscle: Role of S-nitrosothiols. Br. J. Pharmacol. 111, 1287–1295. doi:10.1111/j.1476-5381.1994.tb14885.x
Lomask, M. (2006). Further exploration of the Penh parameter. Exp. Toxicol. Pathol. 57, 13–20. doi:10.1016/j.etp.2006.02.014
Lorier, A. R., Funk, G. D., and Greer, J. J. (2010). Opiate-induced suppression of rat hypoglossal motoneuron activity and its reversal by ampakine therapy. PLoS One 5, e8766. doi:10.1371/journal.pone.0008766
Ludbrook, J. (1998). Multiple comparison procedures updated. Clin. Exp. Pharmacol. Physiol. 25, 1032–1037. doi:10.1111/j.1440-1681.1998.tb02179.x
Manoj, V. M., Mohan, H., Aravind, U. K., and Aravindakumar, C. T. (2006). One-electron reduction of S-nitrosothiols in aqueous medium. Free Radic. Biol. Med. 41, 1240–1246. doi:10.1016/j.freeradbiomed.2006.06.025
Manzke, T., Guenther, U., Ponimaskin, E. G., Haller, M., Dutschmann, M., Schwarzacher, S., et al. (2003). 5-HT4a receptors avert opioid-induced breathing depression without loss of analgesia. Science 301, 226–229. doi:10.1126/science.1084674
Manzke, T., Niebert, M., Koch, U. R., Caley, A., Vogelgesang, S., Bischoff, A. M., et al. (2011). Serotonin receptor 1A-modulated dephosphorylation of glycine receptor α3: A new molecular mechanism of breathing control for compensation of opioid-induced respiratory depression without loss of analgesia. Schmerz 25, 272–281. doi:10.1007/s00482-011-1044-1
May, W. J., Gruber, R. B., Discala, J. F., Puskovic, V., Henderson, F., Palmer, L. A., et al. (2013b). Morphine has latent deleterious effects on the ventilatory responses to a hypoxic challenge. Open J. Mol. Integr. Physiol. 3, 166–180. doi:10.4236/ojmip.2013.34022
May, W. J., Henderson, F., Gruber, R. B., Discala, J. F., Young, A. P., Bates, J. N., et al. (2013a). Morphine has latent deleterious effects on the ventilatory responses to a hypoxic-hypercapnic challenge. Open J. Mol. Integr. Physiol. 3, 134–145. doi:10.4236/ojmip.2013.33019
McHugh, M. L. (2011). Multiple comparison analysis testing in ANOVA. Biochem. Med. Zagreb. 21, 203–209. doi:10.11613/bm.2011.029
Meller, S. T., Lewis, S. J., Brody, M. J., and Gebhart, G. F. (1991). The peripheral nociceptive actions of intravenously administered 5-HT in the rat requires dual activation of both 5-HT2 and 5-HT3 receptor subtypes. Brain Res. 561, 61–68. doi:10.1016/0006-8993(91)90749-l
Mendoza, J., Passafaro, R., Baby, S., Young, A. P., Bates, J. N., Gaston, B., et al. (2013). L-Cysteine ethyl ester reverses the deleterious effects of morphine on, arterial blood-gas chemistry in tracheotomized rats. Respir. Physiol. Neurobiol. 189, 136–143. doi:10.1016/j.resp.2013.07.007
Meyer, L. C., Fuller, A., and Mitchell, D. (2006). Zacopride and 8-OH-DPAT reverse opioid-induced respiratory depression and hypoxia but not catatonic immobilization in goats. Am. J. Physiol. Regul. Integr. Comp. Physiol. 290, R405–R413. doi:10.1152/ajpregu.00440.2005
Mosier-Boss, P. A., and Lieberman, S. H. (2005). The role of hydrogen bonding in the selectivity of L-cysteine methyl ester (CYSM) and L-cysteine ethyl ester (CYSE) for chloride ion. Spectrochim. Acta. A Mol. Biomol. Spectrosc. 61, 845–854. doi:10.1016/j.saa.2004.06.011
Myers, P. R., Minor, R. L., Guerra, R., Bates, J. N., and Harrison, D. G. (1990). Vasorelaxant properties of the endothelium-derived relaxing factor more closely resemble S-nitrosocysteine than nitric oxide. Nature 345, 161–163. doi:10.1038/345161a0
Nelson, M. T., Joksovic, P. M., Perez-Reyes, E., and Todorovic, S. M. (2005). The endogenous redox agent L-cysteine induces T-type Ca2+ channel-dependent sensitization of a novel subpopulation of rat peripheral nociceptors. J. Neurosci. 25, 8766–8775. doi:10.1523/JNEUROSCI.2527-05.2005
Nelson, M. T., Milescu, L. S., Todorovic, S. M., and Scroggs, R. S. (2010). A modeling study of T-type Ca2+ channel gating and modulation by L-cysteine in rat nociceptors. Biophys. J. 98, 197–206. doi:10.1016/j.bpj.2009.10.007
Nemoto, T., Shimma, N., Horie, S., Saito, T., Okuma, Y., Nomura, Y., et al. (2003). Involvement of the system L amino acid transporter on uptake of S-nitroso-L-cysteine, an endogenous S-nitrosothiol, in PC12 cells. Eur. J. Pharmacol. 458, 17–24. doi:10.1016/s0014-2999(02)02699-7
Nishida, K., Ohta, Y., Ito, M., Nagamura, Y., Kitahara, S., Fujii, K., et al. (1996). Conversion of gamma-glutamylcysteinylethyl ester to glutathione in rat hepatocytes. Biochim. Biophys. Acta 1313, 47–53. doi:10.1016/0167-4889(96)00054-7
O-Uchi, J., Ryu, S. Y., Jhun, B. S., Hurst, S., and Sheu, S. S. (2014). Mitochondrial ion channels/transporters as sensors and regulators of cellular redox signaling. Antioxid. Redox Signal. 21, 987–1006. doi:10.1089/ars.2013.5681
Oertel, B. G., Felden, L., Tran, P. V., Bradshaw, M. H., Angst, M. S., Schmidt, H., et al. (2010). Selective antagonism of opioid-induced ventilatory depression by an ampakine molecule in humans without loss of opioid analgesia. Clin. Pharmacol. Ther. 87, 204–211. doi:10.1038/clpt.2009.194
Ohta, H., Bates, J. N., Lewis, S. J., and Talman, W. T. (1997). Actions of S-nitrosocysteine in the nucleus tractus solitarii are unrelated to release of nitric oxide. Brain Res. 746, 98–104. doi:10.1016/s0006-8993(96)01188-2
Owen, M. D., Unal, C. B., Callahan, M. F., Trivedi, K., York, C., and Millington, W. R. (2000). Glycyl-glutamine inhibits the respiratory depression, but not the antinociception, produced by morphine. Am. J. Physiol. Regul. Integr. Comp. Physiol. 279, R1944–R1948. doi:10.1152/ajpregu.2000.279.5.R1944
Pagani, R., Leoncini, R., Terzuoli, L., Pizzichini, M., and Marinello, E. (1994). The regulation of alanine and aspartate aminotransferase by different aminothiols and by vitamin B-6 derivatives. Biochim. Biophys. Acta 1204, 250–256. doi:10.1016/0167-4838(94)90015-9
Palkovic, B., Callison, J. J., Marchenko, V., Stuth, E. A. E., Zuperku, E. J., and Stucke, A. G. (2021). Dose-dependent respiratory depression by remifentanil in the rabbit parabrachial nucleus/kölliker-fuse complex and pre-bötzinger complex. Anesthesiology 135, 649–672. doi:10.1097/ALN.0000000000003886
Palkovic, B., Cook-Snyder, D., Callison, J. J., Langer, T. M., Nugent, R., Stuth, E. A. E., et al. (2022). Contribution of the caudal medullary raphe to opioid induced respiratory depression. Respir. Physiol. Neurobiol. 299, 103855. doi:10.1016/j.resp.2022.103855
Palkovic, B., Marchenko, V., Zuperku, E. J., Stuth, E. A. E., and Stucke, A. G. (2020). Multi-level regulation of opioid-induced respiratory depression. Physiol. (Bethesda) 35, 391–404. doi:10.1152/physiol.00015.2020
Palmer, L. A., deRonde, K., Brown-Steinke, K., Gunter, S., Jyothikumar, V., Forbes, M. S., et al. (2015). Hypoxia-induced changes in protein S-nitrosylation in female mouse brainstem. Am. J. Respir. Cell. Mol. Biol. 52, 37–45. doi:10.1165/rcmb.2013-0359OC
Palmer, L. A., May, W. J., deRonde, K., Brown-Steinke, K., Bates, J. N., Gaston, B., et al. (2013). Ventilatory responses during and following exposure to a hypoxic challenge in conscious mice deficient or null in S-nitrosoglutathione reductase. Respir. Physiol. Neurobiol. 185, 571–581. doi:10.1016/j.resp.2012.11.009
Pathirathna, S., Covey, S. M., Todorovic, D. F., and Jevtovic-Todorovic, V. (2006). Differential effects of endogenous cysteine analogs on peripheral thermal nociception in intact rats. Pain 125, 53–64. doi:10.1016/j.pain.2006.04.024
Pattinson, K. T. (2008). Opioids and the control of respiration. Br. J. Anaesth. 100, 747–758. doi:10.1093/bja/aen094
Paul, B. D., Sbodio, J. I., and Snyder, S. H. (2018). Cysteine metabolism in neuronal redox homeostasis. Trends Pharmacol. Sci. 39, 513–524. doi:10.1016/j.tips.2018.02.007
Perissinotti, L. L., Turjanski, A. G., Estrin, D. A., and Doctorovich, F. (2005). Transnitrosation of nitrosothiols: Characterization of an elusive intermediate. J. Am. Chem. Soc. 127, 486–487. doi:10.1021/ja044056v
Prabhakar, N. R. (2012). Hydrogen sulfide (H2S): A physiologic mediator of carotid body response to hypoxia. Adv. Exp. Med. Biol. 758, 109–113. doi:10.1007/978-94-007-4584-1_15
Quindry, J. C., Ballmann, C. G., Epstein, E. E., and Selsby, J. T. (2016). Plethysmography measurements of respiratory function in conscious unrestrained mice. J. Physiol. Sci. 66, 157–164. doi:10.1007/s12576-015-0408-1
Ren, J., Ding, X., Funk, G. D., and Greer, J. J. (2009). Ampakine CX717 protects against fentanyl-induced respiratory depression and lethal apnea in rats. Anesthesiology 110, 1364–1370. doi:10.1097/ALN.0b013e31819faa2a
Ren, J., Ding, X., and Greer, J. J. (2015). 5-HT1A receptor agonist Befiradol reduces fentanyl-induced respiratory depression, analgesia, and sedation in rats. Anesthesiology 122, 424–434. doi:10.1097/ALN.0000000000000490
Ren, J., Ding, X., and Greer, J. J. (2019). Activating α4β2 nicotinic acetylcholine receptors alleviates fentanyl-induced respiratory depression in rats. Anesthesiology 130, 1017–1031. doi:10.1097/ALN.0000000000002676
Ren, J., Ding, X., and Greer, J. J. (2020). Countering opioid-induced respiratory depression in male rats with nicotinic acetylcholine receptor partial agonists varenicline and ABT 594. Anesthesiology 132, 1197–1211. doi:10.1097/ALN.0000000000003128
Ren, J., Poon, B. Y., Tang, Y., Funk, G. D., and Greer, J. J. (2006). Ampakines alleviate respiratory depression in rats. Am. J. Respir. Crit. Care Med. 174, 1384–1391. doi:10.1164/rccm.200606-778OC
Roozekrans, M., Olofsen, E., van der Schrier, R., van Gerven, J., Peng, S., McLeod, J., et al. (2015). Reversal of opioid-induced respiratory depression by BK-channel blocker GAL021: A pharmacokinetic-pharmacodynamic modeling study in healthy volunteers. Clin. Pharmacol. Ther. 97, 641–649. doi:10.1002/cpt.99
Roozekrans, M., van der Schrier, R., Okkerse, P., Hay, J., McLeod, J. F., and Dahan, A. (2014). Two studies on reversal of opioid-induced respiratory depression by BK-channel blocker GAL021 in human volunteers. Anesthesiology 121, 459–468. doi:10.1097/ALN.0000000000000367
Rossi, R., Giustarini, D., Milzani, A., and Dalle-Donne, I. (2009). Cysteinylation and homocysteinylation of plasma protein thiols during ageing of healthy human beings. J. Cell. Mol. Med. 13, 3131–3140. doi:10.1111/j.1582-4934.2008.00417.x
Sahibzada, N., Ferreira, M., Wasserman, A. M., Taveira-DaSilva, A. M., and Gillis, R. A. (2000). Reversal of morphine-induced apnea in the anesthetized rat by drugs that activate 5-hydroxytryptamine1A receptors. J. Pharmacol. Exp. Ther. 292, 704–713.
Sakuraba, S., Tsujita, M., Arisaka, H., Takeda, J., Yoshida, K., and Kuwana, S. (2009). Donepezil reverses buprenorphine-induced central respiratory depression in anesthetized rabbits. Biol. Res. 42, 469–475./S0716-97602009000400008.
Satoh, T., and Hosokawa, M. (2006). Structure, function and regulation of carboxylesterases. Chem. Biol. Interact. 162, 195–211. doi:10.1016/j.cbi.2006.07.001
Satoh, T., and Hosokawa, M. (1998). The mammalian carboxylesterases: From molecules to functions. Annu. Rev. Pharmacol. Toxicol. 38, 257–288. doi:10.1146/annurev.pharmtox.38.1.257
Schmid, C. L., Kennedy, N. M., Ross, N. C., Lovell, K. M., Yue, Z., Morgenweck, J., et al. (2017). Bias factor and therapeutic window correlate to predict safer opioid analgesics. Cell. 171, 1165–1175. e13. doi:10.1016/j.cell.2017.10.035
Seckler, J. M., Grossfield, A., May, W. J., Getsy, P. M., and Lewis, S. J. (2022). Nitrosyl factors play a vital role in the ventilatory depressant effects of fentanyl in unanesthetized rats. Biomed. Pharmacother. 146, 112571. doi:10.1016/j.biopha.2021.112571
Seckler, J. M., Meyer, N. M., Burton, S. T., Bates, J. N., Gaston, B., and Lewis, S. J. (2017). Detection of trace concentrations of S-nitrosothiols by means of a capacitive sensor. PLoS One 12, grime0187149. doi:10.1371/journal.pone.0187149
Seckler, J. M., Shen, J., Lewis, T. H. J., Abdulameer, M. A., Zaman, K., Palmer, L. A., et al. (2020). NADPH diaphorase detects S-nitrosylated proteins in aldehyde-treated biological tissues. Sci. Rep. 10, 21088. doi:10.1038/s41598-020-78107-6
Semenza, E. R., Harraz, M. M., Abramson, E., Malla, A. P., Vasavda, C., Gadalla, M. M., et al. (2021). D-cysteine is an endogenous regulator of neural progenitor cell dynamics in the mammalian brain. Proc. Natl. Acad. Sci. U. S. A. 118, e2110610118. doi:10.1073/pnas.2110610118
Servin, A. L., Goulinet, S., and Renault, H. (1988). Pharmacokinetics of cysteine ethyl ester in rat. Xenobiotica. 18, 839–847. doi:10.3109/00498258809041722
Seth, D., and Stamler, J. S. (2011). The SNO-proteome: Causation and classifications. Curr. Opin. Chem. Biol. 15, 129–136. doi:10.1016/j.cbpa.2010.10.012
Shanker, G., and Aschner, M. (2001). Identification and characterization of uptake systems for cystine and cysteine in cultured astrocytes and neurons: Evidence for methylmercury-targeted disruption of astrocyte transport. J. Neurosci. Res. 66, 998–1002. doi:10.1002/jnr.10066
Shih, T. W., Lin, T. H., Shealy, Y. F., and Hill, D. L. (1997). Nonenzymatic isomerization of 9-cis-retinoic acid catalyzed by sulfhydryl compounds. Drug Metab. Dispos. 25, 27–32.
Shimizu, T., Nozawa, T., and Hatano, M. (1976). Magnetic circular dichroism of myoglobin-thiolate complexes. Biochim. Biophys. Acta 434, 126–136. doi:10.1016/0005-2795(76)90042-8
Sia, R. L., and Zandstra, D. F. (1981). 4-Aminopyridine reversal of fentanyl-induced respiratory depression in normocapnic and hypercapnic patients. Br. J. Anaesth. 53, 373–379. doi:10.1093/bja/53.4.373
Simmons-Willis, T. A., Koh, A. S., Clarkson, T. W., and Ballatori, N. (2002). Transport of a neurotoxicant by molecular mimicry: The methylmercury-L-cysteine complex is a substrate for human L-type large neutral amino acid transporter (LAT) 1 and LAT2. Biochem. J. 367, 239–246. doi:10.1042/BJ20020841
Stein, P. D., Goldhaber, S. Z., and Henry, J. W. (1995). Alveolar-arterial oxygen gradient in the assessment of acute pulmonary embolism. Chest 107, 139–143. doi:10.1378/chest.107.1.139
Stengel, A., Coskun, T., Goebel, M., Wang, L., Craft, L., Alsina-Fernandez, J., et al. (2010). Central injection of the stable somatostatin analog ODT8-SST induces a somatostatin2 receptor-mediated orexigenic effect: Role of neuropeptide Y and opioid signaling pathways in rats. Endocrinology 151, 4224–4235. doi:10.1210/en.2010-0195
Stipanuk, M. H., Simmons, C. R., Karplus, P. A., and Dominy, J. E. (2011). Thiol dioxygenases: Unique families of cupin proteins. Amino Acids 41, 91–102. doi:10.1007/s00726-010-0518-2
Stipanuk, M. H., Ueki, I., Dominy, J. E., Simmons, C. R., and Hirschberger, L. L. (2009). Cysteine dioxygenase: A robust system for regulation of cellular cysteine levels. Amino Acids 37, 55–63. doi:10.1007/s00726-008-0202-y
Stomberski, C. T., Hess, D. T., and Stamler, J. S. (2019). Protein S-nitrosylation: Determinants of specificity and enzymatic regulation of S-Nitrosothiol-Based signaling. Antioxid. Redox Signal. 30, 1331–1351. doi:10.1089/ars.2017.7403
Story, D. A. (1996). Alveolar oxygen partial pressure, alveolar carbon dioxide partial pressure, and the alveolar gas equation. Anesthesiology 84, 1011. doi:10.1097/00000542-199604000-00036
Sun, Y., Liu, K., Martinez, E., Dale, J., Huang, D., and Wang, J. (2017). AMPAkines and morphine provide complementary analgesia. Behav. Brain Res. 334, 1–5. doi:10.1016/j.bbr.2017.07.020
Svensson, B. E. (1988a). Abilities of peroxidases to catalyse peroxidase-oxidase oxidation of thiols. Biochem. J. 256, 757–762. doi:10.1042/bj2560757
Svensson, B. E., Gräslund, A., Ström, G., Moldeus, P., and Graslund, A. (1993). Thiols as peroxidase substrates. Free Radic. Biol. Med. 14, 167–175. doi:10.1016/0891-5849(93)90007-h
Svensson, B. E. (1988b). Thiols as myeloperoxidase-oxidase substrates. Biochem. J. 253, 441–449. doi:10.1042/bj2530441
Szwergold, B. S. (2006). Alpha-thiolamines such as cysteine and cysteamine act as effective transglycating agents due to formation of irreversible thiazolidine derivatives. Med. Hypotheses 66, 698–707. doi:10.1016/j.mehy.2005.10.029
Todorovic, S. M., Jevtovic-Todorovic, V., Meyenburg, A., Mennerick, S., Perez-Reyes, E., Romano, C., et al. (2001). Redox modulation of T-type calcium channels in rat peripheral nociceptors. Neuron 31, 75–85. doi:10.1016/s0896-6273(01)00338-5
Todorovic, S. M., and Jevtovic-Todorovic, V. (2014). Redox regulation of neuronal voltage-gated calcium channels. Antioxid. Redox Signal. 21, 880–891. doi:10.1089/ars.2013.5610
Todorovic, S. M., Meyenburg, A., and Jevtovic-Todorovic, V. (2004). Redox modulation of peripheral T-type Ca2+ channels in vivo: Alteration of nerve injury-induced thermal hyperalgesia. Pain 109, 328–339. doi:10.1016/j.pain.2004.01.026
Travis, M. D., Davisson, R. L., Bates, J. N., and Lewis, S. J. (1997). Hemodynamic effects of L- and D-S-nitroso-beta, beta-dimethylcysteine in rats. Am. J. Physiol. 273, H1493–H1501. doi:10.1152/ajpheart.1997.273.3.H1493
Travis, M. D., Stoll, L. L., Bates, J. N., and Lewis, S. J. (1996). L- and D-S-nitroso-beta, beta-dimethylcysteine differentially increase cGMP in cultured vascular smooth muscle cells. Eur. J. Pharmacol. 318, 47–53. doi:10.1016/s0014-2999(96)00719-4
Trivedi, M. S., and Deth, R. (2015). Redox-based epigenetic status in drug addiction: A potential contributor to gene priming and a mechanistic rationale for metabolic intervention. Front. Neurosci. 8, 444. doi:10.3389/fnins.2014.00444
Trivedi, M., Shah, J., Hodgson, N., Byun, H. M., and Deth, R. (2014). Morphine induces redox-based changes in global DNA methylation and retrotransposon transcription by inhibition of excitatory amino acid transporter type 3-mediated cysteine uptake. Mol. Pharmacol. 85, 747–757. doi:10.1124/mol.114.091728
Tsikas, D., Schwedhelm, K. S., Surdacki, A., Giustarini, D., Rossi, R., Kukoc-Modun, L., et al. (2018). S-Nitroso-N-acetyl-L-cysteine ethyl ester (SNACET) and N-acetyl-L-cysteine ethyl ester (NACET)-Cysteine-based drug candidates with unique pharmacological profiles for oral use as NO, H2S and GSH suppliers and as antioxidants: Results and overview. J. Pharm. Anal. 8, 1–9. doi:10.1016/j.jpha.2017.12.003
Tsujita, M., Sakuraba, S., Kuribayashi, J., Hosokawa, Y., Hatori, E., Okada, Y., et al. (2007). Antagonism of morphine-induced central respiratory depression by donepezil in the anesthetized rabbit. Biol. Res. 40, 339–346. doi:10.4067/S0716-97602007000400008
Tsumuro, T., Alejandra Hossen, M., Kishi, Y., Fujii, Y., and Kamei, C. (2006). Nasal congestion model in Brown Norway rats and the effects of some H1-antagonists. Int. Immunopharmacol. 6, 759–763. doi:10.1016/j.intimp.2005.11.009
Tunnicliff, G. (1994). Amino acid transport by human erythrocyte membranes. Comp. Biochem. Physiol. Comp. Physiol. 108, 471–478. doi:10.1016/0300-9629(94)90329-8
Turell, L., Radi, R., and Alvarez, B. (2013). The thiol pool in human plasma: The central contribution of albumin to redox processes. Free Radic. Biol. Med. 65, 244–253. doi:10.1016/j.freeradbiomed.2013.05.050
van der Schier, R., Roozekrans, M., van Velzen, M., Dahan, A., and Niesters, M. (2014). Opioid-induced respiratory depression: Reversal by non-opioid drugs. F1000Prime Rep. 6, 79. doi:10.12703/P6-79
van Dorp, E., Yassen, A., and Dahan, A. (2007). Naloxone treatment in opioid addiction: The risks and benefits. Expert Opin. Drug Saf. 6, 125–132. doi:10.1517/14740338.6.2.125
Varga, A. G., Reid, B. T., Kieffer, B. L., and Levitt, E. S. (2020). Differential impact of two critical respiratory centres in opioid-induced respiratory depression in awake mice. J. Physiol. 598, 189–205. doi:10.1113/JP278612
Vonhof, S., and Sirén, A. L. (1991). Reversal of μ-opioid-mediated respiratory depression by α2-adrenoceptor antagonism. Life Sci. 49, 111–119. doi:10.1016/0024-3205(91)90024-6
Walker, J. E., Hoff, H. E., Huggins, R. A., and Deavers, S. I. (1967). Influence of morphine on central control of respiration and circulation in the dog. Arch. Int. Pharmacodyn. Ther. 170, 216–228.
Wallenstein, S., Zucker, C. L., and Fleiss, J. L. (1980). Some statistical methods useful in circulation research. Circ. Res. 47, 1–9. doi:10.1161/01.res.47.1.1
Wei, A. D., and Ramirez, J. M. (2019). Presynaptic mechanisms and KCNQ potassium channels modulate opioid depression of respiratory drive. Front. Physiol. 10, 1407. doi:10.3389/fphys.2019.01407
Wepierre, J., Laprand, J., Garcet, S., and Laurre, M. (1964). Etude de la repartition et de l’elimination de l’ester methylique de la cysteine 35S chez la Souris et le Rat. Therapie 19, 187–200.
Wilcken, D. E., and Gupta, V. J. (1979). Cysteine-homocysteine mixed disulphide: Differing plasma concentrations in normal men and women. Clin. Sci. 57, 211–215. doi:10.1042/cs0570211
Williams, F. M. (1985). Clinical significance of esterases in man. Clin. Pharmacokinet. 10, 392–403. doi:10.2165/00003088-198510050-00002
Williams, J. T., Ingram, S. L., Henderson, G., Chavkin, C., von Zastrow, M., Schulz, S., et al. (2013). Regulation of μ-opioid receptors: Desensitization, phosphorylation, internalization, and tolerance. Pharmacol. Rev. 65, 223–254. doi:10.1124/pr.112.005942
Winer, B. J. (1971). Statistical principles of experimental design. Tokyo: McGraw-Hill Book Co., Kogakusha Ltd., 752–809.
Winkler, A., Kutchan, T. M., and Macheroux, P. (2007). 6-S-cysteinylation of bi-covalently attached FAD in berberine bridge enzyme tunes the redox potential for optimal activity. J. Biol. Chem. 282, 24437–24443. doi:10.1074/jbc.M703642200
Wróbel, M., Ubuka, T., Yao, W. B., and Abe, T. (1997). Effect of glucose-cysteine adduct on cysteine desulfuration in Guinea pig tissues. Physiol. Chem. Phys. Med. NMR 29, 11–14.
Xiao, D., Xie, F., Xu, X., and Zhou, X. (2020). The impact and mechanism of ampakine CX1739 on protection against respiratory depression in rats. Future Med. Chem. 12, 2093–2104. doi:10.4155/fmc-2020-0256
Wu, L., Yang, W., Jia, X., Yang, G., Duridanova, D., Cao, K., et al. (2009). Pancreatic islet overproduction of H2S and suppressed insulin release in Zucker diabetic rats. Lab. Invest. 89, 59–67. doi:10.1038/labinvest.2008.109
Yamaguchi, K., and Hosokawa, Y. (1987). Cysteine dioxygenase. Methods Enzymol. 143, 395–403. doi:10.1016/0076-6879(87)43069-3
Yamano, S., Kageura, E., Ishida, T., and Toki, S. (1985). Purification and characterization of Guinea pig liver morphine 6-dehydrogenase. J. Biol. Chem. 260, 5259–5264. doi:10.1016/s0021-9258(18)89015-9
Yanaura, S., Takeda, H., and Misawa, M. (1982). Behavior of mucus glycoproteins of tracheal secretory cells following L-cysteine methyl ester treatment. J. Pharmacobiodyn. 5, 603–610. doi:10.1248/bpb1978.5.603
Yin, J., Ren, W., Yang, G., Duan, J., Huang, X., Fang, R., et al. (2016). L-Cysteine metabolism and its nutritional implications. Mol. Nutr. Food Res. 60, 134–146. doi:10.1002/mnfr.201500031
Young, A. P., Gruber, R. B., Discala, J. F., May, W. J., Palmer, L. A., and Lewis, S. J. (2013). Co-activation of μ- and δ-opioid receptors elicits tolerance to morphine-induced ventilatory depression via generation of peroxynitrite. Resp. Physiol. Neurobiol. 186, 255–264. doi:10.1016/j.resp.2013.02.028
Young, J. D., Jones, S. E. M., and Ellory, J. C. (1981). Amino acid transport via the red cell anion transport system. Biochim. Biophys. Acta 645, 157–160. doi:10.1016/0005-2736(81)90524-1
Yu, C., Yuan, M., Yang, H., Zhuang, X., and Li, H. (2018). P-glycoprotein on blood-brain barrier plays a vital role in fentanyl brain exposure and respiratory toxicity in rats. Toxicol. Sci. 164, 353–362. doi:10.1093/toxsci/kfy093
Zerangue, N., and Kavanaugh, M. P. (1996). Interaction of L-cysteine with a human excitatory amino acid transporter. J. Physiol. 493, 419–423. doi:10.1113/jphysiol.1996.sp021393
Keywords: L-cysteine methylester, morphine, ventilatory depression, arterial blood-gas chemistry, Sprague Dawley rats
Citation: Getsy PM, Baby SM, May WJ, Bates JN, Ellis CR, Feasel MG, Wilson CG, Lewis THJ, Gaston B, Hsieh Y-H and Lewis SJ (2022) L-cysteine methyl ester overcomes the deleterious effects of morphine on ventilatory parameters and arterial blood-gas chemistry in unanesthetized rats. Front. Pharmacol. 13:968378. doi: 10.3389/fphar.2022.968378
Received: 13 June 2022; Accepted: 22 August 2022;
Published: 28 September 2022.
Edited by:
Albert Dahan, Leiden University Medical Center (LUMC), NetherlandsReviewed by:
Rutger Martijn Van Der Schrier, Leiden University Medical Center (LUMC), NetherlandsCopyright © 2022 Getsy, Baby, May, Bates, Ellis, Feasel, Wilson, Lewis, Gaston, Hsieh and Lewis. This is an open-access article distributed under the terms of the Creative Commons Attribution License (CC BY). The use, distribution or reproduction in other forums is permitted, provided the original author(s) and the copyright owner(s) are credited and that the original publication in this journal is cited, in accordance with accepted academic practice. No use, distribution or reproduction is permitted which does not comply with these terms.
*Correspondence: Paulina M. Getsy, cHhnNTVAY2FzZS5lZHU=
†Present address: Santhosh M. Baby, Translational Sciences Treatment Discovery, Galvani Bioelectronics, Inc., Collegeville, PA, United States
Disclaimer: All claims expressed in this article are solely those of the authors and do not necessarily represent those of their affiliated organizations, or those of the publisher, the editors and the reviewers. Any product that may be evaluated in this article or claim that may be made by its manufacturer is not guaranteed or endorsed by the publisher.
Research integrity at Frontiers
Learn more about the work of our research integrity team to safeguard the quality of each article we publish.