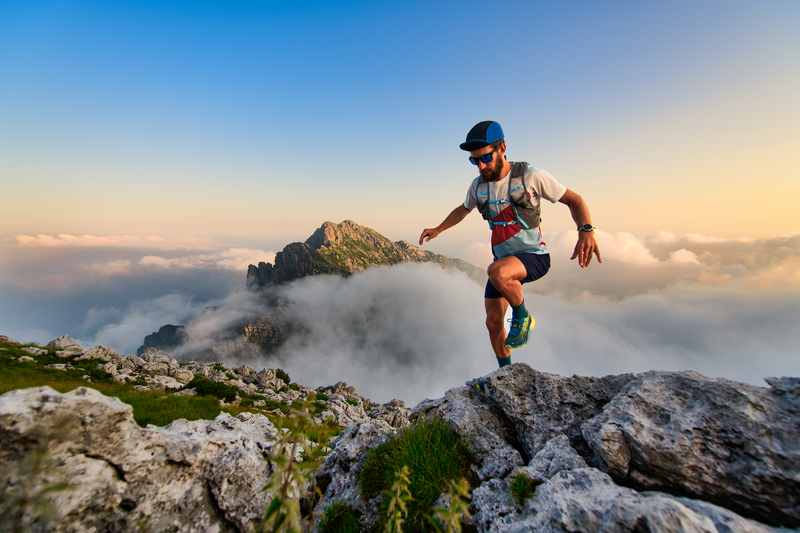
95% of researchers rate our articles as excellent or good
Learn more about the work of our research integrity team to safeguard the quality of each article we publish.
Find out more
REVIEW article
Front. Pharmacol. , 11 August 2022
Sec. Pharmacology of Anti-Cancer Drugs
Volume 13 - 2022 | https://doi.org/10.3389/fphar.2022.968061
This article is part of the Research Topic Discovery of Small Molecule Lead Compounds: a Driving Force to Unravel New Anti-Cancer Targets and Mechanisms View all 8 articles
Microtubules are the fundamental part of the cell cytoskeleton intimately involving in cell proliferation and are superb targets in clinical cancer therapy today. Microtubule stabilizers have become one of the effectively main agents in the last decades for the treatment of diverse cancers. Taccalonolides, the highly oxygenated pentacyclic steroids isolated from the genus of Tacca, are considered a class of novel microtubule-stabilizing agents. Taccalonolides not only possess a similar microtubule-stabilizing activity as the famous drug paclitaxel but also reverse the multi-drug resistance of paclitaxel and epothilone in cellular and animal models. Taccalonolides have captured numerous attention in the field of medicinal chemistry due to their variety of structures, unique mechanism of action, and low toxicity. This review focuses on the structural diversity, semi-synthesis, modification, and pharmacological activities of taccalonolides, providing bright thoughts for the discovery of microtubule-stabilizing drugs.
Natural products and their derivatives have been severed as an unsurpassed source to find microtubule-targeting drugs. Numerous natural-related microtubule-targeting agents currently in discovery or approved in preclinical or clinical are classified into two groups: microtubule-destabilizing agents (e.g., vinblastine, vincristine, colchicine, rigosertib, combretastatin A-4, ABT-751, lexibulin, and BNC105) (Taylor, 1965; Bryan, 1971; Griggs et al., 2001; Yee et al., 2005; Kremmidiotis et al., 2010; Lickliter et al., 2010; Jost et al., 2017), and microtubule-stabilizing agents (e.g., paclitaxel, docetaxel, cabazitaxel, and epothilones A and B) (Figure 1) (Bhalla et al., 1993; Jordan, 2002; Fojo and Menefee, 2007; Ojima et al., 2014), depending on their different mechanisms. Though these anti-mitotic agents have been utilized for the clinical treatment of different cancerous patients in the last decades, high toxicity, poor solubility, low oral bioavailability, and multidrug resistance render these agents less optimum for the clinical treatment of cancer (Gottesman et al., 1996; Litman et al., 2001; Zhao et al., 2016). Therefore, it remains essential to develop new microtubule-targeting agents with fewer side effects and improved activity against various classes of tumors.
The pentacyclic steroids taccalonolides are isolated from plants Tacca plantaginea (Hance) Drenth, Tacca chantrieri Andre, and Tacca paxiana. Taccalonolides are the first plant-derived microtubule-stabilizing agents except for paclitaxel. They are also the first natural steroids with microtubule-stabilizing activity (Liu et al., 2015). The previous reviews illustrated that over 120 constituents had been isolated from the genus Tacca, including 33 kinds of taccalonolides (Jiang et al., 2014; Li et al., 2014). Chen et al. and Risinger et al. summarized the biological activities of taccalonolides, as well as the challenges in formulation and supply (Risinger and Mooberry, 2010; Chen et al., 2021). Viewing the importance of the relationships between structural characteristics and biological activity, the present review is mainly focused on the classification of taccalonolides, as well as the semisynthetic taccalonolides and their synthetic routes. All the natural taccalonolides are classified into three categories according to structural characteristics and discussed in detail, along with the semisynthetic taccalonolides and their synthetic routes are summarized for the first time. The biological activities of taccalonolides in recent 6 years and the structure-activity relationships are also included.
In the early 1960s, Professor Paul Scheuer investigated the “bitter principle” of the tubers of T. leontopetaloides, a starchy food source. A compound named taccalin was purified. As an intensely bitter, this light-yellow powder had a probable tetracyclic structure (Scheuer et al., 1963). The actual structure of taccalonolides was later found to be much larger, but this pioneering work laid the groundwork for the elucidation of their structures. Up to now, 41 natural taccalonolides have been isolated from the genus Tacca (Table 1).
Like general steroids, most of taccalonolides are based on a pentacyclic carbon framework. The unique structural feature of taccalonolides that they contain an epoxy function between C2 and C3. In the present review, taccalonolides are mainly divided into four categories according to the different positions of the lactone ring in the structures. The first category is represented by taccalonolide A (1, Figure 2) isolated from T. plantaginea and is the largest number of taccalonolides. The characteristic feature of this class is structural lactone rings linked to C23 and C26. The second category is represented by taccalonolide AT (34, Figure 3). Chemical structure of the second category is similar to the first class except for the six-membered lactone ring bearing the C15–C26 bond. The third classification is relatively small and represented by taccalonolide O (17, Figure 4). It has the hexacyclic feature and the same class of carbon skeleton with formers, characterized by a C22–C24 lactone ring. Besides, taccolanolides O (15) and P (16) (Figure 4) are classified into the last group. They have the characteristics of taccalonolide, though the partial carbon skeletons have changed.
In 1987, taccalonolides A and B (1 and 2) were firstly found in the rhizome of T. plantaginea (Chen et al., 1987). Later, nine related compounds taccalonolides D–L (4–12) were reported from the same plant. They all contain a double bond between C22 and C23 (Chen et al., 1988a; Shen et al., 2010; Shen et al., 1996; Chen et al., 1997; Muhlbauer et al., 2003). Taccalonolide N obtained from T. paxiana possessed a similar structure. Taccalonolides H and H2 (8 and 33) feature an unusual double bond at C7–C8. Unlike other taccalonolides belonging to this class, taccalonolide M (13) and taccalonolide AF (32) do not possess any double bonds. Chemical investigation of the roots of T. paxiana resulted in the isolation of five new steroidal compounds, taccalonolides R–V (18–22) (Muhlbauer et al., 2003). From the whole plants of T. plantaginea, five new pentacyclic steroids, taccalonolides W (23) and AC–AF (29–32) were isolated (Yang et al., 2008; Li et al., 2011). Taccalonolide AF (32) is the only one in this category with a C22–C23 epoxy ring and is presumably derived from the epoxidation of taccalonolides A (1). Peng et al. obtained three new taccalonolides Z (26), AA (27), and AB (28) from T. chantrieri and T. integrifolia (Peng et al., 2011), while taccalonolides AI (40) and AG (41) were reported from the roots and rhizomes of T. chantrieri (Risinger et al., 2011; Peng et al., 2014). All compounds are substituted with a five-membered lactone ring connected in positions C23 and C26, which is the important feature of taccalonolides in this class (Figure 2).
A six-membered lactone ring located in positions C15 and C26 features the characteristics of taccalonolides in this class. Taccalonolide C (3), the first example of this type, was isolated from the rhizome of T. plantaginea. Taccalonolide C (3) might derive from taccalonolide D (4): the C23–C24 lactone ring of 4 opens and then reforms a new lactone ring with C15 hydroxyl group (Chen et al., 1988b). Taccalonolides AW–AY (37–39) were isolated from the ethanolic extract of the whole plants of T. chantrieri (Ni et al., 2015). All these taccalonolides have a ketone group in position C6. Some compounds also contain a double bond in positions C21–C22, e.g., taccalonolides X (24) and AT–AV (34–36) (Figure 3) (Yang et al., 2008; Ni et al., 2015).
The last category possesses a six-membered lactone ring connected in positions C22 and C24. The representative is taccalonolide Q (17) from the rhizomes of T. subflaellata (Huang et al., 2002). Taccalonolide Y (25) was firstly isolated from the whole plants of T. plantaginea (Figure 4) (Yang et al., 2008). In addition to the common epoxy function between C2 and C3, the third type of taccalonolides also have an extra epoxy in positions C6 and C7, along with a hydroxyl group at C5.
Two novel steroidal bitter principles, taccolanolides O (15) and P (16) are isolated from the tubers of T. subflaellata (Huang et al., 2002). Differing from the above-mentioned taccolanolides, they do not contain a six-membered E ring in the structure. An epoxy group presents in positions C6 and C7.
Taccalonolides are rare in nature, which is one obstacle to developing taccalonolides into drugs. Due to complex structures, multiple fragile functional groups, and high costs of synthesis, the total synthesis of taccalonolides has not been reported until now. Semi-synthesis may solve the problem of resource shortage. There are a few semi-synthetic taccalonolides. The reported structural modifications of taccalonolides include simple hydrolysis, epoxidation, and attaching a fluorescein group at C6. So far, twenty-seven taccalonolides and their derivatives were obtained by semi-synthesis (Figures 5, 6).
An epoxidation reaction of compound 1 affords 32, which leads to a drastic increase in antiproliferative potency (Adam et al., 2004). Li et al. performed the same epoxidation reaction on taccalonolide B to generate taccalonolide AJ (42) via two-step reactions, namely hydrolysis of taccalonolide A to obtain taccalonolide B, and epoxidation of taccalonolide B to form an epoxy group in positions C22 and C23, along with a new minor compound, designated taccalonolide AO (47), was obtained (Scheme 1) (Li et al., 2011). Similarly, taccalonolide N (14) could be obtained from the hydrolysis of taccalonolide E (5). Followed by hydrolysis at C1 or lactone ring-opening and re-closing, taccalonolides AN (46) and AK (43) were yielded, respectively (Scheme 2) (Li et al., 2013). DMDO (3,3-dimethyldioxirane) is an efficient and mild reagent, which can rapidly epoxidize taccalonolides under neutral and mild conditions. Epoxidation of C22–C23 double bond in 5, 14, 18, 20, 26–28, 30, 40, and 46 by DMDO could quantitatively yield 48–57, respectively (Scheme 3) (Peng et al., 2014).
Taccalonolides cannot dissociate from tubulin and be detected after binding. The biochemical and pharmacokinetic analyses are limited by the covalent bonding. Inspired by cell-permeable taxane-based probes, Du et al. reported the fluorogenic taccalonolide probes that maintain the native biological properties of 42, allowing for more detailed evaluations of the uptake, target binding, and distribution of these compounds in vitro and in vivo (Du et al., 2019; Du et al., 2020). When the fluorescein moiety links with the taccalonolide skeleton by an amide bond or ester bond in C6 position, their microtubule-binding and -stabilizing activities will not be compromised. Totally, eleven fluorogenic taccalonolide probes (58–68) were obtained (Figure 6). Among them, dipivaloyl-protected compound 66 is the most potent irreversible fluorogenic microtubule probe. The synthetic routes of 65 and 66 are shown in Scheme 4. Apart from the amide-linked fluorescein tag in C6 position, one more ester linker exists in their structures. The reduction of taccalonolide B (2) by NaBH3CN results in the stereospecific formation of 6S–OH derivative, which is ideally suited for esterification. Du et al. also synthesized the fluorescein derivatives with an alkyne linker. The derivatives were covalently bonded via “click” chemistry to generate the intermediate 77. Epoxidation of C22–C23 double bond in 77 affords 68 (Scheme 5).
The structural diversity of taccalonolides leads to the diversity of biological activity, which mainly manifests in microtubule-stabilizing, cytotoxic activity, antitumor, drug-sensitive, and multidrug-resistant cell lines. According to the biological activities in vitro and in vivo, Risinger et al. clearly summarized the biological activities of taccalonolides before 2010 (Risinger and Mooberry, 2010). The current review concerns the biological activities of taccalonolides from 2010 (Table 2). Notably, the research on the mechanism of taccalonolides that made a breakthrough is focused.
The unique microtubule stabilization mechanism of taccalonolides is imparted by the covalent bonding of the C22–C23 epoxy moiety to tubulin, which causes the density of interphase microtubules to increase, G2/M cell accumulation, Bcl-2 phosphorylation, and initiation of apoptosis (Risinger and Mooberry, 2010; Peng et al., 2011; Cao et al., 2018). Thus, as novel microtubule stabilizers, taccalonolides could circumvent clinically relevant forms of drug resistance. Moreover, taccalonolides could enhance the extent of tubulin polymerization without affecting the time required to initiate tubulin polymerization, which made them distinct from other microtubule stabilizers (Peng et al., 2014). In the past few years, many investigations have been performed on the microtubule-stabilizing activity of taccalonolides. Taccalonolides with C23–C26 lactone ring (e.g., taccalonolides A and E) are proved to have moderate or strong microtubule-stabilizing effects on different human cancer cell lines (e.g., HeLa, SCC4, and A-10 embryonic aortic smooth muscle cells).
Risinger et al. reported that compound 42 stabilized the plus ends of microtubules more effectively than paclitaxel. The increased resistance of microtubule plus ends to catastrophe may play a role in the observed inability of taccalonolide-induced asters to coalesce during mitosis (Risinger et al., 2014). However, in recent years, more researchers focused on the relative contribution of key tubulin residues and taccalonolide moieties for drug-target interaction. The high-resolution crystallographic data showed the M-loop of 42 was in an unordered conformation. Meanwhile, hydrogen-deuterium exchange experiments indicated that taccalonolides did not promote M-loop stabilization (Risinger et al., 2013a; Wang et al., 2017; Balaguer et al., 2019). Wang et al. determined the 2.05 Å crystal structure of the 42-tubulin complex. The structure revealed that C22–C23 epoxy group of 42 is covalently bound to β-tubulin D226. With a binding of 42, the M-loop underwent a conformational shift to facilitate tubulin polymerization (Wang et al., 2017). The contact area between taccalonolides and β-tubulin may play a role in microtubule-stabilizing activity. Du et al. found the fluorescein moiety of 67 occupied an adjacent binding pocket on β-tubulin close to the M-loop, affording additional interactions with β-tubulin residues via hydrophobic interactions, H bonds, and/or salt bridges. It is suggested the enhanced ability of 61 and 67 to promote microtubule stabilization could be attributed to improving binding affinity to β-tubulin afforded by these additional contacts (Du et al., 2020). These studies firstly reveal the mechanism of the only steroidal natural products, which could bind to the β-subunit of microtubules from the perspective of molecules, atoms, and chemical bonds.
Both natural taccalonolides (e.g., 1, 5, and 32) and semi-synthetic taccalonolides (e.g., 42, 55, and 66) have good cytotoxic activity in vitro (e.g., HeLa, HepG2, and Huh7 cells) and antitumor activity in vivo (e.g., MDA-MB-231 and -435 breast cancer xenograft model). The present review will discuss the progress of cytotoxic and antitumor activities of taccalonolides in recent 6 years.
When HepG2 and Huh7 cells were treated with 1, the expression of apoptosis-associate protein Bax was up-regulated, whereas Bcl-2 was down-regulated. It was indicated compound 1 could improve the cytotoxicity of sorafenib in hepatocellular cancer by inhibiting the activation of the sonic hedgehog pathway (Tian and He, 2020). Compound 66, reported by Du et al. represented a cell-permeable, fluorogenic probe that combined the potent antiproliferative activities of 42 with excellent fluorescence properties. In HeLa or SK-OV-3 cells, the direct drug-fluorophore conjugation in 66 led to GI50 values of 30–50 nM (Du et al., 2020).
Compounds 1, 42, and taccabulin A were combined to evaluate the synergistic antiproliferative effects in MDA-MB-435 and HeLa cancer cell lines. The result showed the CI values were between 0.65 and 0.85. Moreover, the synergy of 42 and taccabulin A was more obvious. The study was the precedent for the combination of microtubule-stabilizing and -destabilizing small molecules in combination chemotherapy (Risinger et al., 2013b). The antitumor action in vivo may relate to half-life. Compound 42 exhibited excellent and highly persistent antitumor effects when directly acting on tumors. However, it was no antitumor effects when administered systemically, probably due to the short half-life (8.1 min) in vivo (Risinger et al., 2017).
The multidrug resistance of cancer cells is evolved by multiple mechanisms, including overexpression of P-glycoprotein (Pgp), multidrug resistance protein 7 (MRP7), and βIII isotype of tubulin (Morris and Fornier, 2008). Unlike paclitaxel, taccalonolides are not substrates of Pgp. Taccalonolides exhibit in vivo antitumor efficacy in both drug-sensitive and resistant tumor models. They are still against paclitaxel-resistant tumor cells (Tinley et al., 2003; Risinger et al., 2008). Ola et al. found taccalonolides substituted with isovalerate in position C7 or C15, along with epoxy group connected at C22–C23, showed effective and highly persistent antitumor activity in paclitaxel-resistant xenograft model when administered intratumorally, without associated toxicity (Ola et al., 2018). Compounds 1 and 5 completely inhibited cell proliferation and induced cell death in drug-sensitive cell lines SK-OV-3 and MDA-MB-435. These two compounds also induced cytotoxicity in the drug-resistant cell line NCI/ADR (Tinley et al., 2003). Both compound 1 with a total dose of 38 mg/kg, and compound 5 with 86 mg/kg, showed 91% growth inhibition on the Pgp overexpressing Mam17/ADR model, indicating they have excellent antitumor activity in vivo. Besides, 5 might be better tolerated than 1 (Risinger et al., 2008).
MRP7 expression induces drug resistance in non-small-cell lung cancer cell lines on paclitaxel treatment. MRP7 expression levels are correlated with both paclitaxel accumulation and sensitivity. Under the treatment of 1, 2, 5, and 14, HEK-MRP7-C17 and HEK-MRP7-C18 cell lines were more sensitive compared with the control cells (HEK-pcDNA3). Whether MRP7 was overexpressed, compounds 1, 2, 5, and 14 had similar potency in HEK293 cells (Risinger et al., 2008). The result suggested that the ability of taccalonolides to circumvent MRP7-mediated efflux may provide a significant advantage for the treatment of cancer.
Except for Pgp and MRP7 overexpression, βIII-tubulin isotype expression is a main clinical determinant of resistance to tubulin-target therapy. Dose-response curves suggested that wild-type βIII cells (a HeLa-derived cell line that ectopically expresses the human βIII-tubulin gene) were 4.7-fold resistant to paclitaxel as compared with the HeLa cell line. But the βIII-expressing cell line reproducibly showed sensitivity to 2, 5, and 14, suggesting that incorporation of βIII isotype tubulin into mitotic spindles does not confer resistance to taccalonolides (Risinger et al., 2008). Moreover, IC50 of 42 in HeLa and HeLa βIII-tubulin overexpressing paclitaxel-resistant cells was 6.2 and 9.6 nM, respectively (Balaguer et al., 2019). These results suggested that covalent bonding to tubulin facilitates overcoming βIII-tubulin-mediated drug resistance.
Studying the structure–activity relationship of taccalonolides would be beneficial to designing and synthesizing derivatives as a new generation of antitumor agents with improved physical, chemical, and biological properties. Therefore, we summarize the SAR of taccalonolides, hoping to help identify specific structural moieties crucial for potent biological activities, as well as those that impede optimal cellular effects (Figure 7).
The epoxy function in positions C22 and C23 is critical for the potent antitumor effects of the naturally occurring epoxy-taccalonolides. The presence of C22–C23 epoxy group can restore the activity of taccalonolides that are reduced by the C6 ketone (Peng et al., 2014; Danielsson et al., 2017). Compounds containing C22–23 epoxies showed no antiproliferative, cytotoxic, or microtubule bundling activities in cells and did not directly interact with tubulin in biochemical assays (Du et al., 2020). When C22–C23 double bond of 1 and 2 was epoxidized into 32 and 42, respectively, the antiproliferative activity dramatically increased by 234–743 folds, indicating the epoxidation of C22–C23 double bond significantly enhanced the potency of taccalonolides (Li et al., 2011; Wang et al., 2017; Sanchez-Murcia et al., 2019). After C22–C23 epoxidation, 56 and 57 also showed modest increases of 2–4 folds, and 52 and 53 showed relatively modest 7 and 2 folds increases in potency, respectively (Peng et al., 2014). Surprisingly, 29, which differed from 1 only by an additional α-hydroperoxyl group at C20, had no antiproliferative or microtubule-stabilizing activities at a concentration up to 50 µM. It was indicated that α-hydroperoxyl group was not optimal for bioactivity and substituent at this site was important (Li et al., 2011). And 43 and 47 have an obvious structural rearrangement in C20–C23 region, but antiproliferative activity or microtubule-stabilizing effects were not observed at concentrations up to 50 µM (Li et al., 2013). In summary, the results highlight the importance of substituents at C20–C23 of taccalonolides.
Modifications in position C1 could significantly affect the potency of taccalonolides. Compound 20 with an isovalerate group in position C1 has better antiproliferative potency than compound 18 substituted with an acetoxy group in the same position. The antiproliferative potency of 20 is 38-folds of 18 (Peng et al., 2011). Replacement of the acetoxy group at C1 in 44 with an isovalerate group generates 45. Similarly, 17-folds increasement in the potency of 45 was observed (Li et al., 2013). Hydrolysis of the acetoxy group at C1 or C15, or retention of the isovalerate group at C1 could significantly improve the biological activity. Modifications at C1 enhanced activity were linked to the data of the crystal structure. Peng et al. reported that 55 showed an even higher potency than 42 (Peng et al., 2014). The bulky isovalerate group at C1 of 55 was well-positioned into a hydrophobic pocket surrounded by L217, L230, L275, and F272, which might be the reason for the higher potency than 42 (Wang et al., 2017). These data emphasize the importance of the group in C1 position on the activity of taccalonolides.
Compound 9 differs from 1 in the positions of C6 and C7: the ketone and hydroxy groups are located in positions C6 and C7 of 1, respectively, while they are opposite of 9. The difference resulted in a 9.25-folds decrease in antiproliferative potency of 9. However, compound 30 contained a C5–C6 enol group and a C7 ketone is more effective than 1, suggesting that the double bond plays a role in retaining antiproliferative activity. Compared with 1, 33 possesses a double bond in positions of C7 and C8, leading to the potency increased by 7.4 times, which illustrates that the α, β-unsaturated ketone at C7–C9 might have the best potency. In addition, when a hydroxyl group was added to C7 of 1 to form the rare geminal diol compound 31, the potency also unchanged (Li et al., 2011). The effect of C5 hydroxyl group on the efficacy of taccalonolides is complicated. Compared with 14, the C5 hydroxyl group in 44 caused a 4-fold decrease in the effectiveness (Li et al., 2013). However, compared to 1, the potency of 26 contained a C5 hydroxyl group was increased 44 times (Peng et al., 2011).
The C6 metabolite of 1 decreased the potency, demonstrating the importance of the C6 ketone (Peng et al., 2014). Furthermore, the study showed improvement of antiproliferative activities could be achieved for taxane-based probes by replacement of a β-alanine linker with a shorter glycine linker (Lee et al., 2017). Du et al. synthesized the dipivaloyl-protected taccalonolide probe 66 featuring direct conjugation of the fluorescein moiety with the taccalonalide skeleton by an amide bond. Indeed, the antiproliferative potency of 66 was a 50-folds improvement as compared to 63 and <10-folds difference as compared to the untagged 42 against HeLa and SK-OV-3 cell lines (Du et al., 2020). The IC50 value of 68 was determined to be 2.5 ± 0.1 µM. This represented a 600-fold decrease in cellular potency as compared to 42. Additionally, the generation of a functional C6 taccalonolide probe provides a proof of principle for utilizing C6 as a site for conjugation of the taccalonolides for targeted drug delivery (Du et al., 2019).
Taccalonolides modified at C7 or C15 positions were easy to hydrolysis in aqueous solutions, and modification at C25 resulted in the disappearance of biological activity (Ola et al., 2018). Hydrolysis of the C15-acetoxy group in 1 or 5 affords 2 or 14, respectively. The activity of 2 was 3.1 times higher than that of 1, while 14 was 6 times higher than that of 5 in HeLa cells, indicating hydrolysis of acetate in C15 position increase the efficacy (Li et al., 2013). The presence of acetoxy in C11 position showed little effect on the efficacy of taccalonolides. Compound 1 differs from 5 in only one substituent: it contains an acetoxy group instead of a hydroxyl group at C11. However, their activities were similar to each other. Similarly, the potency of 2 and 14 show no obvious difference. The taccalonolides contain six-membered lactones connected in positions C15 and C24 that have poor antitumor activities, which is illustrated by the results of 34 and 39. These two compounds exhibit no antiproliferative activity against various cancer cell lines (Ni et al., 2015).
Drugs that affect microtubule dynamics, including the taxanes and vinca alkaloids, have been a mainstay in the treatment of leukemias and solid tumors for decades (Risinger et al., 2009). As lead compounds with microtubule targeting activities, the structural and biological diversity of taccalonolides provide the directions to develop new antitumor drugs, especially for solid tumors. In addition to paclitaxel-like microtubule-stabilizing activity, taccalonolides exhibit circumvention of paclitaxel resistance, stronger antiproliferation in vitro, and antitumor activity in vivo. Among them, taccalonolides A and E are more prominent with better microtubule-stabilizing activity and dose-dependent effects on cell cycle distribution and microtubules. Moreover, circumvention of Pgp-mediated drug resistance in vivo by them was exciting. These two compounds belong to C23–C26 lactone ring class. Compared with other types of taccalonolides, their better activity suggests more attention should be paid to these C23–C26 lactone ring classes of taccalonolides to find lead compounds with better activity.
Microtubule stabilizers have been confirmed to play important roles in clinical cancer therapy. They would be more and more widely used in clinics for cancer treatments. As new microtubule stabilizers with multiple structures, taccalonolides possess unique mechanisms of stabilizing microtubules like paclitaxel, along with weaker toxicity than colchicine, as well as better drug resistance than paclitaxel. With SAR of taccalonolides being revealed, new lead compounds from taccalonolides used to replace paclitaxel-resistant microtubule stabilizers would be gradually developed. Moreover, it will refocus attention on the source of anticancer drugs to natural products to promote the development of drugs based on natural active products if taccalonolides-like compounds would have been developed into anticancer drugs.
L-LZ, GL, and YL initiated and designed the project. FG and J-BX contributed to collecting and arranging the documents. Y-FD and YL coordinated the project and wrote the article. All authors reviewed the manuscript.
We are grateful to NSFC (31870329) and the Department of Education of Sichuan Province, China (No. 16ZA0290), for the financial support.
The authors declare that the research was conducted in the absence of any commercial or financial relationships that could be construed as a potential conflict of interest.
All claims expressed in this article are solely those of the authors and do not necessarily represent those of their affiliated organizations, or those of the publisher, the editors, and the reviewers. Any product that may be evaluated in this article, or claim that may be made by its manufacturer, is not guaranteed or endorsed by the publisher.
Adam, W., Saha-Möller, C. R., and Zhao, C. G. (2004). Dioxirane epoxidation of alkenes. ChemInform 61, 219–290. doi:10.1002/chin.200407298
Balaguer, F. D., Muhlethaler, T., Estevez-Gallego, J., Calvo, E., Gimenez-Abian, J. F., Risinger, A. L., et al. (2019). Crystal structure of the cyclostreptin-tubulin adduct: Implications for tubulin activation by taxane-site ligands. Int. J. Mol. Sci. 20, 1392. doi:10.3390/ijms20061392
Bhalla, K., Ibrado, A. M., Tourkina, E., Tang, C., Mahoney, M. E., Huang, Y., et al. (1993). Taxol induces internucleosomal DNA fragmentation associated with programmed cell death in human myeloid-leukemia cells. Leukemia 7, 563–568. doi:10.1016/1040-8428(93)90022-V
Bryan, J. (1971). Vinblastine and microtubules. I. Induction and isolation of crystals from sea urchin oocytes. Exp. Cell Res. 66, 129–136. doi:10.1016/S0014-4827(71)80020-4
Buey, R. M., Barasoain, I., Jackson, E., Meyer, A., Giannakakou, P., Paterson, I., et al. (2005). Microtubule interactions with chemically diverse stabilizing agents: Thermodynamics of binding to the paclitaxel site predicts cytotoxicity. Chem. Biol. 12, 1269–1279. doi:10.1016/j.chembiol.2005.09.010
Cao, Y. N., Zheng, L. L., Wang, D., Liang, X. X., Gao, F., Zhou, X. L., et al. (2018). Recent advances in microtubule-stabilizing agents. Eur. J. Med. Chem. 143, 806–828. doi:10.1016/j.ejmech.2017.11.062
Chen, X. Y., Winstead, A., Yu, H. T., and Peng, J. N. (2021). Taccalonolides: A novel class of microtubule-stabilizing anticancer agents. Cancers 13, 920. doi:10.3390/cancers13040920
Chen, Z. L., Shen, J. H., Gao, Y. S., and Wichtl, M. (1997). Five taccalonolides from Tacca plantaginea. Planta Med. 63, 40–43. doi:10.1055/s-2006-957600
Chen, Z. L., Wang, B. D., and Chen, M. Q. (1987). Steroidal bitter principles from Tacca plantaginea structures of taccalonolide A and B. Tetrahedron Lett. 28, 1673–1675. doi:10.1016/S0040-4039(00)95391-7
Chen, Z. L., Wang, B. D., and Chen, M. Q. (1988b). Study on the bitter principles from Tacca plants: Structures of taccalonolides A and B. Acta chimi. Sin. 6, 251–256. doi:10.1002/cjoc.19880060311
Chen, Z. L., Wang, B. D., and Shen, J. H. (1988a). Taccalonolide C and D, two pentacyclic steroids of Tacca plantaginea. Phytochemistry 27, 2999–3001. doi:10.1016/0031-9422(88)80711-8
Danielsson, J., Sun, D. X., Chen, X. Y., Risinger, A. L., Mooberry, S. L., Sorensen, E. J., et al. (2017). A stereocontrolled annulation of the taccalonolide epoxy lactone onto the molecular framework of trans-androsterone. Org. Lett. 19, 4892–4895. doi:10.1021/acs.orglett.7b02349
Dike, V. T., Vihiior, B., Bosha, J. A., Yin, T. M., Ebiloma, G. U., de Koning, H. P., et al. (2016). Antitrypanosomal activity of a novel taccalonolide from the tubers of Tacca leontopetaloides. Phytochem. Anal. 27, 217–221. doi:10.1002/pca.2619
Du, L., Risinger, A. L., Yee, S. S., Ola, A. R. B., Zammiello, C. L., Cichewicz, R. H., et al. (2019). Identification of C-6 as a new site for linker conjugation to the taccalonolide microtubule stabilizers. J. Nat. Prod. 82, 583–588. doi:10.1021/acs.jnatprod.8b01036
Du, L., Yee, S. S., Ramachandran, K., and Risinger, A. L. (2020). Elucidating target specificity of the taccalonolide covalent microtubule stabilizers employing a combinatorial chemical approach. Nat. Commun. 11, 654. doi:10.1038/s41467-019-14277-w
Fojo, T., and Menefee, M. (2007). Mechanisms of multidrug resistance: The potential role of microtubule-stabilizing agents. Ann. Oncol. 18, V3–V8. doi:10.1093/annonc/mdm172
Gottesman, M. M., Pastan, I., and Ambudkar, S. V. (1996). P-Glycoprotein and multidrug resistance. Curr. Opin. Genet. Dev. 6, 610–617. doi:10.1016/S0959-437X(96)80091-8
Griggs, J., Brindle, K. M., Metcalfe, J. C., Hill, S. A., Smith, G. A., Beauregard, D. A., et al. (2001). Potent anti-metastatic activity of combretastatin-A4. Int. J. Oncol. 19, 821–825. doi:10.3892/ijo.19.4.821
Huang, Y., Liu, J. K., Muhlbauer, A., and Henkel, T. (2002). Three novel taccalonolides from the tropical plant Tacca subflaellata. Helv. Chim. Acta., 85, 25532–25588. doi:10.1002/1522-2675(200208)85:8<2553::AID-HLCA2553>3.0.CO;2-8
Jiang, J. H., Yang, H. M., Wang, Y. L., and Chen, Y. G. (2014). Phytochemical and pharmacological studies of the genus Tacca: A review. Trop. J. Pharm. Res. 13, 635. doi:10.4314/tjpr.v13i4.23
Jordan, M. A. (2002). Mechanism of action of antitumor drugs that interact with microtubules and tubulin. Curr. Med. Chem. Anticancer. Agents 2, 1–17. doi:10.2174/1568011023354290
Jost, M., Chen, Y. W., Gilbert, L. A., Horlbeck, M. A., Krenning, L., Menchon, G., et al. (2017). Combined CRISPRi/a-based chemical genetic screens reveal that rigosertib is A microtubule-destabilizing agent. Mol. Cell 68, 210–223. doi:10.1016/j.molcel.2017.09.012
Kremmidiotis, G., Leske, A. F., Lavranos, T. C., Beaumont, D., Gasic, J., Hall, A., et al. (2010). BNC105: A novel tubulin polymerization inhibitor that selectively disrupts tumor vasculature and displays single-agent antitumor efficacy. Mol. Cancer Ther. 9, 1562–1573. doi:10.1158/1535-7163.MCT-09-0815
Lee, M. M., Gao, Z., and Peterson, B. R. (2017). Synthesis of A Fluorescent analogue of paclitaxel that selectively binds microtubules and sensitively detects efflux by P-glycoprotein. Angew. Chem. Int. Ed. Engl. 56, 6927–6931. doi:10.1002/anie.201703298
Li, J., Peng, J. N., Risinger, A. L., and Mooberry, S. L. (2013). Hydrolysis reactions of the taccalonolides reveal structure-activity relationships. J. Nat. Prod. 76, 1369–1375. doi:10.1021/np400435t
Li, J., Risinger, A. L., and Mooberry, S. L. (2014). Taccalonolide microtubule stabilizers. Bioorg. Med. Chem. 22, 5091–5096. doi:10.1016/j.bmc.2014.01.012
Li, J., Risinger, A. L., Peng, J. N., Chen, Z. L., Hu, L. H., Mooberry, S. L., et al. (2011). Potent taccalonolides, AF and AJ, inform significant structure-activity relationships and tubulin as the binding site of these microtubule stabilizers. J. Am. Chem. Soc. 133, 19064–19067. doi:10.1021/ja209045k
Lickliter, J. D., Francesconi, A. B., Smith, G., Burge, M., Coulthard, A., Rose, S., et al. (2010). Phase I trial of CYT997, A novel cytotoxic and vascular-disrupting agent. Br. J. Cancer 103, 597–606. doi:10.1038/sj.bjc.6605841
Litman, T., Druley, T. E., Stein, W. D., and Bates, S. E. (2001). From MDR to MXR: New understanding of multidrug resistance systems, their properties and clinical significance. Cell. Mol. Life Sci. 58, 931–959. doi:10.1007/PL00000912
Liu, Z. H., Yan, H., and Liu, H. Y. (2015). Chemical constituents and their bioactivities of plants of taccaceae. Chem. Biodivers. 12, 221–238. doi:10.1002/cbdv.201300353
Morris, P. G., and Fornier, M. N. (2008). Microtubule active agents: Beyond the taxane frontier. Clin. Cancer Res. 14, 7167–7172. doi:10.1158/1078-0432.CCR-08-0169
Muhlbauer, A., Seip, S., Nowak, A., and Tran, V. S. (2003). Five novel taccalonolides from the roots of the Vietnamese plant Tacca paxiana. Helv. Chim. Acta 86, 2065–2072. doi:10.1002/hlca.200390162
Ni, G., Zhang, H. Z., Fu, N. J., Zhang, L. L., Wang, M. C., Chen, J., et al. (2015). Cytotoxic taccalonolides and withanolides from Tacca chantrieri. Planta Med. 81, 247–256. doi:10.1055/s-0034-1396203
Ojima, I., Kumar, K., Awasthi, D., and Vineberg, J. G. (2014). Drug discovery targeting cell division proteins, microtubules and FtsZ. Bioorg. Med. Chem. 22, 5060–5077. doi:10.1016/j.bmc.2014.02.036
Ola, A. R. B., Risinger, A. L., Du, L., Zammiello, C. L., Peng, J. N., Cichewicz, R. H., et al. (2018). Taccalonolide microtubule stabilizers generated using semisynthesis define the effects of mono acyloxy moieties at C-7 or C-15 and disubstitutions at C-7 and C-25. J. Nat. Prod. 81, 579–593. doi:10.1021/acs.jnatprod.7b00967
Peng, J. N., Risinger, A. L., Li, J., and Mooberry, S. L. (2014). Synthetic reactions with rare taccalonolides reveal the value of C-22, 23 epoxidation for microtubule stabilizing potency. J. Med. Chem. 57, 6141–6149. doi:10.1021/jm500619j
Peng, J., Risinger, A. L., Fest, G. A., Jackson, E. M., Helms, G., Polin, L. A., et al. (2011). Identification and biological activities of new taccalonolide microtubule stabilizers. J. Med. Chem. 54, 6117–6124. doi:10.1021/jm200757g
Risinger, A. L., Giles, F. J., and Mooberry, S. L. (2009). Microtubule dynamics as A target in oncology. Cancer Treat. Rev. 35, 255–261. doi:10.1016/j.ctrv.2008.11.001
Risinger, A. L., Jackson, E. M., Polin, L. A., Helms, G. L., LeBoeuf, D. A., Joe, P. A., et al. (2008). The taccalonolides; microtubule stabilizers that circumvent clinically relevant taxane resistance mechanisms. Cancer Res. 68, 8881–8888. doi:10.1158/0008-5472.CAN-08-2037
Risinger, A. L., Li, J., Bennett, M. J., Rohena, C. C., Peng, J., Schriemer, D. C., et al. (2013a). Taccalonolide binding to tubulin imparts microtubule stability and potent in vivo activity. Cancer Res. 73, 6780–6792. doi:10.1158/0008-5472.CAN-13-1346
Risinger, A. L., Li, J., Du, L., Benavides, R., Robles, A. J., Cichewicz, R. H., et al. (2017). Pharmacokinetic analysis and in vivo antitumor efficacy of taccalonolides AF and AJ. J. Nat. Prod. 80, 409–414. doi:10.1021/acs.jnatprod.6b00944
Risinger, A. L., Li, J., Peng, J., Chen, Z., and Mooberry, S. L. (2011). P3-16-17: The identification of novel microtubule stabilizing taccalonolides. Cancer Res. 71, 3. doi:10.1158/0008-5472.SABCS11-P3-16-17
Risinger, A. L., and Mooberry, S. L. (2011). Cellular studies reveal mechanistic differences between taccalonolide A and paclitaxel. Cell Cycle 10, 2162–2171. doi:10.4161/cc.10.13.16238
Risinger, A. L., and Mooberry, S. L. (2010). Taccalonolides: Novel microtubule stabilizers with clinical potential. Cancer Lett. 291, 14–19. doi:10.1016/j.canlet.2009.09.020
Risinger, A. L., Peng, J. N., Rohena, C. C., Aguilar, H. R., Frantz, D. E., Mooberry, S. L., et al. (2013b). The bat flower: A source of microtubule-destabilizing and -stabilizing compounds with synergistic antiproliferative actions. J. Nat. Prod. 76, 1923–1929. doi:10.1021/np4005079
Risinger, A. L., Riffle, S. M., Lopus, M., Jordan, M. A., Wilson, L., Mooberry, S. L., et al. (2014). The taccalonolides and paclitaxel cause distinct effects on microtubule dynamics and aster formation. Mol. Cancer 13, 41. doi:10.1186/1476-4598-13-41
Risingera, A. L., Natarajan, M., Thomas, C. R., and Mooberry, S. L. (2011). The taccalonolides, novel microtubule stabilizers, and gamma-radiation have additive effects on cellular viability. Cancer Lett. 307, 104–111. doi:10.1016/j.canlet.2011.03.022
Rohena, C. C., Peng, J. N., Johnson, T. A., Crews, P., and Mooberry, S. L. (2013). Chemically diverse microtubule stabilizing agents initiate distinct mitotic defects and dysregulated expression of key mitotic kinases. Biochem. Pharmacol. 85, 1104–1114. doi:10.1016/j.bcp.2013.01.030
Sanchez-Murcia, P. A., Mills, A., Cortes-Cabrera, A., and Gago, F. (2019). Unravelling the covalent binding of zampanolide and taccalonolide AJ to a minimalist representation of a human microtubule. J. Comput. Aided. Mol. Des. 33, 627–644. doi:10.1007/s10822-019-00208-w
Scheuer, P. J., Swanholm, C. E., Madamba, L. A., and Hudgins, W. R. (1963). Constituents of Tacca leontopetaloides. Lloydia 26, 133–140.
Shen, J. H., Chen, Z. J., and Gao, Y. S. (1996). Taccalonolides from Tacca plantaginea. Phytochemistry 42, 891–893. doi:10.1016/0031-9422(95)00972-8
Shen, J. H., Chen, Z. L., and Gao, Y. S. (1991). The pentacyclic steroidal constituents of Tacca plantaginea: taccalonolide E and F. Chin. J. Chem. 9, 92–94. doi:10.1002/cjoc.19910090113
Taylor, E. W. (1965). The mechanism of colchicine inhibition of mitosis. I. Kinetics of inhibition and the binding of H3-colchicine. J. Cell Biol. 25 (Suppl. L), 145–160. doi:10.1083/jcb.25.1.145
Tian, H., and He, Z. K. (2020). Anti-hepatoma effect of taccalonolide A through suppression of sonic hedgehog pathway. Artif. Cells Nanomed. Biotechnol. 48, 939–947. doi:10.1080/21691401.2020.1773484
Tinley, T. L., Randall-Hlubek, D. A., Leal, R. M., Jackson, E. M., Cessac, J. W., Quada, J. C., et al. (2003). Taccalonolides E and A: Plant-derived steroids with microtubule-stabilizing activity. Cancer 97, 3133–3275. doi:10.1002/cncr.11380
Wang, Y. X., Yu, Y. M., Li, G. B., Li, S. A., Wu, C. Y., Gigant, B., et al. (2017). Mechanism of microtubule stabilization by taccalonolide AJ. Nat. Commun. 8, 15787. doi:10.1038/ncomms15787
Yang, J. Y., Zhao, R. H., Chen, C. X., Ni, W., Teng, F., Hao, X. J., et al. (2008). Taccalonolides W-Y, three new pentacyclic steroids from Tacca plantaginea. Helv. Chim. Acta 91, 1077–1082. doi:10.1002/hlca.200890116
Yee, K. W. L., Hagey, A., Verstovsek, S., Cortes, J., Garcia-Manero, G., O'Brien, S. M., et al. (2005). Phase 1 study of ABT-751, a novel microtubule inhibitor, in patients with refractory hematologic malignancies. Clin. Cancer Res. 11, 6615–6624. doi:10.1158/1078-0432.CCR-05-0650
Keywords: taccalonolides, microtubule-stabilizer, structural classification, antitumor, pharmacological mechanism
Citation: Li Y, Du Y-F, Gao F, Xu J-B, Zheng L-L, Liu G and Lei Y (2022) Taccalonolides: Structure, semi-synthesis, and biological activity. Front. Pharmacol. 13:968061. doi: 10.3389/fphar.2022.968061
Received: 13 June 2022; Accepted: 04 July 2022;
Published: 11 August 2022.
Edited by:
Yan Zhang, Shenyang Pharmaceutical University, ChinaCopyright © 2022 Li, Du, Gao, Xu, Zheng, Liu and Lei. This is an open-access article distributed under the terms of the Creative Commons Attribution License (CC BY). The use, distribution or reproduction in other forums is permitted, provided the original author(s) and the copyright owner(s) are credited and that the original publication in this journal is cited, in accordance with accepted academic practice. No use, distribution or reproduction is permitted which does not comply with these terms.
*Correspondence: Ling-Li Zheng, emhlbmdsaW5nbGlAY21jLmVkdS5jbg==; Gang Liu, bGl1Z2FuZ2N5ZnlAMTYzLmNvbQ==; Yu Lei, aGV5eW91MTIzMzFAMTYzLmNvbQ==
Disclaimer: All claims expressed in this article are solely those of the authors and do not necessarily represent those of their affiliated organizations, or those of the publisher, the editors and the reviewers. Any product that may be evaluated in this article or claim that may be made by its manufacturer is not guaranteed or endorsed by the publisher.
Research integrity at Frontiers
Learn more about the work of our research integrity team to safeguard the quality of each article we publish.