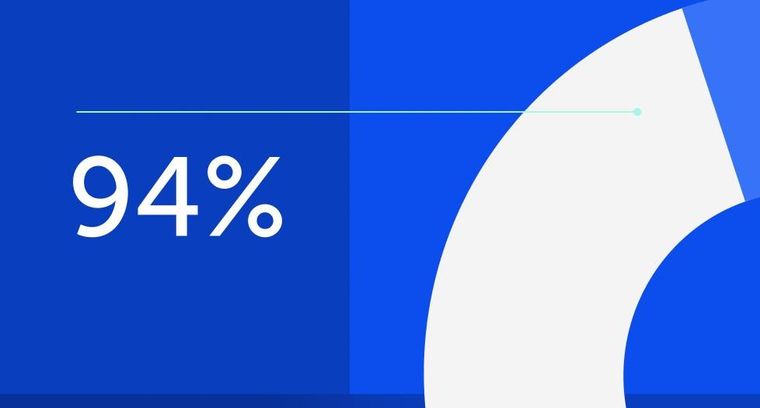
94% of researchers rate our articles as excellent or good
Learn more about the work of our research integrity team to safeguard the quality of each article we publish.
Find out more
REVIEW article
Front. Pharmacol., 22 August 2022
Sec. Experimental Pharmacology and Drug Discovery
Volume 13 - 2022 | https://doi.org/10.3389/fphar.2022.954684
This article is part of the Research TopicProgress in Pathology and Drug Development of Metabolic DiseaseView all 11 articles
The gut microbiota (GM) has received extensive attention in recent years, and its key role in the establishment and maintenance of health and in the development of diseases has been confirmed. A strong correlation between the GM and the progression of endometriosis (EMS) has been observed in emerging research. Alterations in the composition and function of the GM have been described in many studies on EMS. In contrast, the GM in the environment of EMS, especially the GM metabolites, such as bile acids and short-chain fatty acids that are related to the pathogenesis of EMS, can promote disease progression. Chenodeoxycholic acid (CDCA), as one of the primary bile acids produced in the liver, is metabolized by various enzymes derived from the GM and is critically important in maintaining intestinal homeostasis and regulating lipid and carbohydrate metabolism and innate immunity. Given that the complexity of CDCA as a signalling molecule and the interaction between the GM and EMS have not been clarified, the role of the CDCA and GM in EMS should be understood from a novel perspective. However, few articles on the relationship between CDCA and EMS have been reviewed. Therefore, we review the available and possible potential links between CDCA, the GM and EMS and put forward the hypothesis that CDCA and its derivative obeticholic acid can improve the symptoms of EMS through the GM.
Endometriosis (EMS) is an estrogen-dependent disease characterized by the planting and growth of endometrial stromal cells and gland cells outside the uterine cavity (Zondervan et al., 2020). EMS can be traditionally divided into three types, i.e., peritoneal, ovarian EMS and deep invasive EMS. Rare EMS cases of distal organs, such as thoracic EMS and cerebellar EMS, have been reported in case reports (Meggyesy et al., 2020; Chen and Li, 2021). However, the pathophysiology of EMS is still unclear. The most widely considered and accepted mechanism is the retrograde menstrual mechanism. In addition, the pathogenesis may involve factors such as coelomic metaplasia, immune alterations, stem cell theory, genetics and Mullerian duct residue (Vercellini et al., 2014; Bulun et al., 2019). Women with EMS usually suffer from chronic pain and infertility in the clinic. Given that EMS tends to be confused with digestive diseases, the diagnosis of EMS is generally not timely, thereby seriously affecting the quality of life of patients and causing huge economic losses (Surrey et al., 2020). Therefore, further elucidation of the pathological mechanism of EMS may lay the foundation for the development of new diagnostic and therapeutic drugs.
The gut microbiota (GM) exists in the human gastrointestinal tract and plays a key role in the regulation of human health and disease, and its role is no less than that of other organs in the human body (O'Hara and Shanahan, 2006). The GM is rich in species diversity and abundance, and its number is equivalent to ten times the total number of human cells. Firmicutes and Bacteroidetes are the main components of the GM and account for more than 90% of the GM composition, and Firmicutes/Bacteroidetes (F/B) is also an important regulatory factor for maintaining host homeostasis (Turnbaugh et al., 2006). F/B is usually elevated when the GM is disturbed. When host homeostasis is unregulated, the internal environment changes, leading to alterations in the structure of the GM, which in turn influences the host. Therefore, the internal environment and the GM jointly regulate the host’s homeostasis (Rooks and Garrett, 2016). The connections between the GM and the host are not limited to the intestines and involve many organs and diseases throughout the host, such as cardiovascular, nervous, respiratory, liver, autoimmunity, and other diseases (Sharon et al., 2016; Budden et al., 2017; Tang et al., 2017; Clemente et al., 2018; Ma et al., 2018).
Chenodeoxycholic acid (CDCA) is one of the main primary bile acids (BAs) in human and animal bile and is generated from the conversion of cholesterol in liver cells. Initially, CDCA was recognized as a lipid solvent and plays an indispensable role in dissolving cholesterol and maintaining enterohepatic circulation (Chenodeoxycholic acid in the dissolution, 1976). CDCA, as a signalling molecule, can also play an important role in a variety of physiological functions (Song et al., 2019). The signal transduction effect of CDCA plays a considerable role in metabolic diseases and in some inflammation-related diseases and cancers (Thomas et al., 2008; Jia et al., 2018).
Our previous study found that the level of CDCA in the intestine of EMS mice is increased (Ni et al., 1989a) and that CDCA can improve the abundance of the GM of EMS mice, which showed a high degree of similarity with normal mice (not published). However, few articles discussing the complex mechanism of CDCA in EMS in detail have been written. This article reviews the relationship between EMS and GM imbalance, the regulation of CDCA on the GM and inflammatory environment, the possible mechanism by which CDCA alleviates the symptoms of patients with EMS by improving the GM and reducing inflammation, and the potential of CDCA and its derivative obeticholic acid (OCA) in the treatment of patients with EMS.
Studies have shown that EMS changes the structure of the GM, causes an imbalance in the F/B ratio, and reduces the α and β diversity (Ni et al., 1989a; Ni et al., 1989b), which are measures of the diversity of the microbiota within a single individual and an indicator to characterize the similarities in microbial composition between individuals, respectively (Table 1). The imbalance in the GM leads to inflammation that damages the health of the intestinal epithelium and affects the health of the whole body through the downstream effects of metabolism. Decreased abundance of Lachnospiraceae and Ruminococcus in EMS has been reported in several studies (Ni et al., 1989a; Huang et al., 2021), and the two microbiotas modulate host physiology and immunity by production of short-chain fatty acids (SCFAs) (Zhang et al., 2021). SCFA, a GM metabolite, has been further demonstrated to have an ameliorative effect on EMS by inhibiting human endometriosis cell survival and lesion growth through G protein-coupled receptors, histone deacetylase and GTPase activating protein RAP1GAP (Chadchan et al., 2021). In addition, Lachnospiraceae can also promote the conversion of primary BAs to secondary BAs (Sorbara et al., 2020). Ruminococcaceae family was decreased in some reports (Yuan et al., 2018; Cao et al., 2020). Ruminococcus gnavus has been shown to produce inflammatory polysaccharides, which are closely associated with Crohn’s disease and to modulate the differentiation of T helper cells that express IL-17A (Th17 cells) through the production of specific secondary BA derivatives (Henke et al., 2019; Paik et al., 2022). Studies have reported that IL-17A plays an important role in EMS progression (Shi et al., 2022). Lactobacillus has long been used as a probiotic supplement to maintain health as well as prevent and treat diseases, but its proportion in GM elevated in EMS models (Ni et al., 1989b; Cao et al., 2020), which may be due to suppression of the differentiation of Th17 cells by Lactobacillus (Wilck et al., 2017). Furthermore, we found that the proportion of bifidobacteriaceae was elevated in some reports (Ni et al., 1989b; Yuan et al., 2018; Shan et al., 2021), and a high proportion of bifidobacteriaceae promoted the differentiation of Th17 cells (Ang et al., 2020). Furthermore, feces from mice with EMS were transplanted into antibiotic gavaged EMS mice which exhibited more severe EMS symptoms, such as an increased number of endometriotic foci (Chadchan et al., 2021). Taken together, the progression of EMS is further promoted by the disturbed GM in EMS patients or mice, the mechanisms of which are multifaceted and probably involve metabolites and Th17 cell differentiation.
The GM promotes the progression of EMS, possibly because the GM participates in the metabolism of estrogen. β-Glucuronidase is secreted by the GM and can metabolize BA-secreted conjugated estrogen and phytestrogens to the deconjugated form (Baker et al., 2017; Ervin et al., 2019). These deconjugated and unbound forms of estrogen can enter the blood and bind to downstream estrogen receptors to regulate female reproductive development (Baker et al., 2017). The abnormal structure of the GM in EMS may promote the excessive secretion of β-glucuronidase, thereby allowing increased effective estrogen to enter the blood and bind to the estrogen receptor in the ectopic endometrial tissue. This phenomenon inhibits the antagonism of progesterone and promotes the proliferation of focal cells to further aggravate the inflammatory response (Figure 1) (Marquardt et al., 2019). Interestingly, estrogen and its derivatives have been shown to reduce the production of lipopolysaccharide (LPS) by the GM and weaken the permeability of the intestine, thereby maintaining the integrity of the intestinal epithelial barrier (Homma et al., 2005). This finding seems to contradict the ability of estrogen to promote EMS cell proliferation. One explanation is that the increased tolerance of EMS to progesterone increases local estrogen levels and promotes the proliferation of ectopic endometrial lesions (Marquardt et al., 2019).
FIGURE 1. The relationship between EMS and GM imbalance. During the progression of EMS, which can occur the imbalance of GM, the structure and the diversity of GM are altered, with the most dominant Firmicutes/Bacteroidetes (F/B) ratio being dysregulated. The disorder of GM results in the destruction of tight junction, the reduction of the expression of ZO-2 protein and further the increasing of the permeability of the intestine. Bacteria penetrate outside the intestinal lumen, activating TLR4, and lead to inflammatory responses, which may create a suitable environment for EMS onset and progression. In addition, β-glucuronidase may be increased in the intestine due to the imbalance of the GM, rendering more unconjugated estrogen that is transported to the EMS lesion location via blood vessels, binding to the estrogen receptor, further promoting the progression of EMS. EMS, endometriosis; GM, gut microbiota; F/B, Firmicutes/Bacteroidetes; TLR4, toll-like receptor 4.
The theory of menstrual retrograde has received significant attention and is widely recognized in the aetiology of EMS. Menstrual retrograde occurs in 90% of women, but only 10% of women develop EMS, which is caused by several factors. The immune system has been a hotspot that has attracted much attention. Immune dysfunction has been confirmed to be a key factor that promotes the growth of ectopic lesions after the retrograde menstruation of endometrial fragments (Symons et al., 2018; Jeljeli et al., 2020; Agostinis et al., 2021). Many iron-containing haemoglobin and reactive oxygen species (ROS) in menstrual fragments retrograde through the fallopian tube, creating an iron overload environment in the peritoneal cavity. This phenomenon promotes the proliferation and polarization of macrophages (Lousse et al., 2009; Woo et al., 2020). Recent studies have shown that the special microenvironment of EMS may promote the polarization tendency of endometrial M2 macrophages towards M1 polarization and that M2 macrophages paradoxically express proinflammatory phenotypes in the initial phase, whereas the proinflammatory phenotype of M1 macrophages is promoted when endometriotic lesions are established (Vallvé-Juanico et al., 2019). The GM has been shown to regulate extraintestinal inflammation (Karmarkar and Rock, 2013). The GM altered by EMS leads to the destruction of the tight junctions of the intestine and decreases the expression of ZO-2 protein, which further increases the permeability of the intestine (Ni et al., 1989b), resulting in the translocation and infiltration of a large number of Gram-negative bacteria outside the intestinal cavity (Meroni et al., 2019). LPS can activate macrophage Toll-like receptor 4 (TLR4) in innate immunity, producing large amounts of tumour necrosis factor-α (TNF-α) and interleukin (IL)-8 and promoting the formation of an inflammatory environment (Figure 1) (Harada et al., 2001). TNF-α and IL-8 play an essential role in endometrial tissue adhesion and the induction of angiogenesis (Nothnick, 2001). The activation of TLR4 signalling and the inflammatory environment may promote the polarization of M1 macrophages and cause them to express proinflammatory phenotypes (Wanderley et al., 2018). The ability of polarized M1 macrophages to phagocytose haemoglobin and ectopic endometrial tissue in the peritoneal cavity is inhibited, but the promotion of the growth of ectopic endometrial tissue is unrestricted. In addition, excess divalent iron ions in the peritoneal cavity enter ectopic endometrial tissue through divalent metal transporter-1, which promotes an increase in intracellular ROS and acts on NF-κB to promote the proliferation of endometrial stromal cells and inhibit apoptosis (Azuma et al., 1989). Iron may contribute to the migration abilities of human endometriotic cells via matrix metalloproteinase expression through the ROS–NF-κB pathway (Woo et al., 2020). These studies showed that the imbalance in the GM promotes the progression of EMS in such ways.
In addition, studies have found that dietary differences through the GM metabolism may also be a contributing factor to EMS. EMS patients consume more red meat, coffee and trans fat but less vegetables and omega-3 polyunsaturated fatty acids (PUFAs) (Parazzini et al., 2013). PUFAs have been confirmed to have anti-inflammatory effects in EMS mouse models (Attaman et al., 1989) and can effectively relieve pain in young women with EMS (Nodler et al., 2020). It can be inferred that the GM with EMS affects part of the host’s dietary habits and metabolizes the diet ingested by the host into products that may further promote the development of EMS. It has been reported that the content of short-chain fatty acids produced by intestinal microbial metabolism in the feces of EMS model mice is less than that of normal mice, but it can effectively prevent the progression of EMS (Chadchan et al., 2021). Therefore, EMS patients could suppress disease progression by adjusting their diet, such as eating more fish oil possessing anti-inflammatory effects and consuming beneficial bacteria that can metabolize and produce short-chain fatty acids.
In short, a close relationship between the imbalance in the GM and the pathogenesis and progression of EMS has been identified, and various complex mechanisms of the relationship urgently need to be confirmed in future studies.
CDCA is one of the main primary BAs in humans and animals. Primary BAs consist of CDCA, cholic acid and conjugated BA (CBA) (Figure 2). CDCA is conjugated with taurine or glycine to form CBA in the liver. After each meal, BAs are secreted into the upper small intestine. In the intestinal lumen, GM-mediated bile salt hydrolase (BSH) deconjugation converts CBA into unconjugated BAs. The distribution of BSH in the GM is only detected in members of the phylum Bacteroidetes, including Clostridium, Enterococcus, Bifidobacterium, and Lactobacillus (Cai et al., 2022). 7α-Dehydroxylation mediated by Clostridium and Eubacterium converts primary BAs into secondary BAs by removing the 7a/b-hydroxy group (Jia et al., 2018). Furthermore, CBA activates pancreatic lipase and forms mixed micelles with monoglycerides, cholesterol, some free fatty acids and fat-soluble vitamins to promote their absorption by intestinal cells. Unconjugated BAs and some glycine-CBAs are reabsorbed in the small intestine through passive diffusion (Dawson and Karpen, 2015). This process is called “enterohepatic circulation of BAs” and has physiological significance because it regulates the synthesis of BAs through feedback inhibition to absorb and transport cholesterol, fat and nutrients to the liver and distribute them to other organs (Hofmann, 2009).
FIGURE 2. CDCA biosynthetic and metabolic pathways. In the liver, CDCA are synthesized from cholesterol via oxidation catalyzed by CYPs, which occurs in the classical and alternative pathways. In the classical pathway, CYP7A1 converts cholesterol to 7α-hydroxycholesterol, which is then transformed into CA and CDCA through the subsequent actions by CYP8B1 and CYP27A1. The alternative pathway is initiated by CYP27A1 and produces CDCA by CYP7B1. Primary BAs CA and CDCA are amidated with glycine or taurine to form conjugated bile acids (GCA, GCDCA, TCA and TCDCA) under the actions of BACS and BAAT. When secreted into the gut after meals, conjugated bile acids are transformed into secondary BAs (LCA and DCA) via biotransformation of GM. Major microbial biotransformation reactions include deconjugation mediated by BSH, 7α-dehydroxylation and oxidation and epimerization mediated by HSDH. CYP, cytochrome P450; CA, cholic acid; GCA, glycocholic acid; GCDCA, glycochenodeoxycholic acid; TCA, taurocholic acid; TCDCA, taurochenodeoxycholic acid; BACS, bile acyl-CoA synthetase; BAAT, bile acid-CoA:amino acid N-acyltransferase; LCA, lithocholic acid; DCA, deoxycholic acid; BSH, bile salt hydrolase.
As a signalling molecule, CDCA can activate the two main BA-sensitive receptors, i.e., BA sensor farnesoid X receptor (FXR) and G protein-coupled BA receptor GPBAR1 (TGR5), and exert endocrine functions (Figure 3). TGR5 is a membrane receptor that is predicted to have 7 transmembrane domains and is a 330-amino-acid-long protein. TGR5 is widely distributed and can be expressed in a variety of organs and cell types, including the gallbladder, intestinal tract, immune cells, fat cells, muscles and nervous systems (Maruyama et al., 2002; Kawamata et al., 2003). Among the natural TGR5 agonists, CDCA is the agonist with the most affinity for TGR5 except lithocholic acid (LCA) and deoxycholic acid. The negative regulation of inflammation by TGR5 is found in macrophages in liver cells (Kupffer cells). BAs inhibit LPS-induced cytokine production by activating TGR5 in macrophages, thereby improving the inflammatory environment (Keitel et al., 2008). Therefore, the activation of the TGR5 signalling pathway can have a beneficial effect on a variety of inflammatory diseases. In intestinal inflammatory diseases, macrophages, which are essential for intestinal immune homeostasis, are the phagocytic cells that interact with microorganisms and microbial products and have a dual role in protecting the host from pathogens and regulating the mucosal response to symbiotic bacteria (Smythies et al., 2005). Inflammatory bowel disease (IBD) is caused by a disproportionate immune response to antigens in the intestinal cavity, leading to chronic inflammation of the intestine (Zhang and Li, 2014). The deletion of TGR5 easily aggravates intestinal inflammation in a mouse model of colitis, whereas the activation of TGR5 reduces the local expression of inflammatory cytokines (Cipriani et al., 2011). In contrast, the activation of TGR5 in vivo can promote the polarization of intestinal mucosa-related immune macrophages from M1 to M2, thereby reducing inflammation in mice (Biagioli et al., 2017). In addition, TGR5 agonists can induce monocytes to differentiate into IL-12 and TNF-α hyposecretory dendritic cells through the TGR5-cAMP signalling pathway (Ichikawa et al., 2012). TGR5, as an indispensable receptor in macrophages, can be activated by BAs, which can transform macrophages into an M2-like phenotype and cause macrophages to exert immunosuppressive behaviour (McMahan et al., 2013). LCA, as the most potent activator of TGR5, is metabolized by CDCA through the GM. Thus, the GM may regulate the activation of TGR5 to a certain extent. The structure of the GM changes in the EMS environment, which may result in the inability of CDCA to be effectively converted into LCA through the GM and in the downregulation of TGR5 signal efficiency. Macrophages play a key role in the progression of EMS, and M2 macrophages activated by TGR5 signalling can reduce inflammation and enhance immunosuppression. Excitingly, a study revealed that TGR5 was expressed in human endometriotic stromal cells, and INT-777, a potent agonist of TGR5, could significantly reduce the production of proinflammatory cytokines and adhesion molecules by inhibiting TNF-α, suggesting that TGR5 activators have beneficial effects against inflammation and ROS in cytokine-induced activation of endometriotic stromal cells (Lyu et al., 2019).
FIGURE 3. The potential roles of CDCA and derivatives in EMS. CDCA produced from the liver is converted into LCA, 3-oxoLCA and isoLCA after being metabolized by GM. FXR and TGR5 activated by CDCA and derivatives suppress proinflammatory cytokine expression, such as IL-1β and TNF-α in dendritic cells and macrophages. CDCA promote the release of FGF-19 by activating FXR, thereby inhibiting the production of CDCA, further forming a negative feedback regulation mechanism in normal environment. 3-oxoLCA and isoLCA inhibit the differentiation of Th17 cells expressing IL-17A upon direct binding to RORγt. Decreased levels of proinflammatory cytokines could improve EMS. Furthermore, CDCA, LCA and 3-oxoLCA modulate RORγ Tregs through the BA receptor VDR to suppress inflammation. FGF-19, fibroblast growth factor 19; RORγt, retinoic acid-related orphan receptor γt; Treg cells, regulatory T cells; VDR, vitamin D receptor.
FXR was the first BA nuclear receptor discovered, and CDCA has the highest affinity among its natural ligands (Ridlon et al., 2014). After activation, FXR can inhibit the expression of the apical sodium-dependent bile acid transporter (ASBT) and increase the expression of two transporters, i.e., organic solute transporter α (OSTα) and β (OSTβ), to reduce the uptake of BA, promote the secretion of BA in basal cells and reduce the concentration of BA in basal cells (Ding et al., 2015). Moreover, FXR is involved in intestinal immune regulation and intestinal mucosal barrier function, reduces inflammation and maintains the integrity of the intestinal epithelial barrier by regulating the degree of inflammation, maintaining the integrity and function of the intestinal wall barrier, and preventing bacterial translocation in the intestine (Gadaleta et al., 2010; Gadaleta et al., 2011a; Sorribas et al., 2019). Vavassori et al. noticed that the expression of proinflammatory cytokine mRNA in the colon of FXR-deficient mice was increased (Vavassori et al., 2009). Raybould et al. found that the activation of FXR by INT-747 can prevent DSS- and TNBS-induced intestinal inflammation, improve colitis symptoms, inhibit epithelial permeability, and reduce goblet cell loss (Raybould, 2012). Inagaki et al. proved that intestinal FXR plays a key role in limiting the overgrowth of bacteria and found that the bacteria in the intestine of mice lacking FXR overgrow, thereby increasing the permeability of the intestine (Inagaki et al., 2006). Studies have found that the activation of the NF-κB pathway leads to increased expression of proinflammatory cytokines, thereby reducing the transcription of FXR target genes and further exacerbating inflammation (Gadaleta et al., 2011b). Interestingly, unconjugated BAs, which can cause damage to liver cells at high concentrations, can regulate intestinal barrier function by activating FXR to regulate innate immunity. Therefore, an appropriate concentration of CDCA to regulate the GM is important.
Receptors that respond to CDCA and its derivatives also include vitamin D receptor (VDR) and retinoic acid-related orphan receptor γt (RORγt) (Figure 3). The antiproliferative and anti-inflammatory effects of VDR in EMS are gradually being revealed. VDR agonists reduce IL-1β- and TNFα-induced inflammatory responses and decrease metalloproteinase (MMP)-2 and MMP-9 mRNA levels in ectopic endometrial tissue (Delbandi et al., 1989). In addition, in vitro experiments also revealed that VDR activation control the inflammatory response mediated by TLR4 and TLR2 in endometrial stroma (Ghanavatinejad et al., 2021). RORγt is a key transcription factor regulating Th17 cell differentiation (Paik et al., 2022). It has been reported that Th17 cells and IL-17A secreted by Th17 cells play an important role in the progress of EMS (Shi et al., 2022). Furthermore, following direct binding of 3-oxoLCA to RORγt, a reduced differentiation of Th17 cells in the mouse intestinal lamina propria was observed (Paik et al., 2022), suggesting that CDCA derivative-regulated RORγt transcription factors could serve as a potential therapeutic strategy for EMS.
CDCA, can maintain its own metabolic function through the enterohepatic circulation pathway and can directly interact with the microbiota in the intestine as an antibacterial agent. As a signalling molecule, CDCA activates the nuclear receptor and membrane receptor, thereby improving the structure of the GM and intestinal barrier function, inhibiting the secretion of inflammatory cytokines, and reducing the inflammatory environment.
The GM of patients with EMS has changed, and this change is caused by many factors, as mentioned above. Compared with those of control patients, the α and β diversity values of the GM of patients decreased to varying degrees. The abundance values of various bacterial groups measured in different experiments also vary, probably due to discrepancies in sequencing methods and experimental details (Ni et al., 1989a; Ni et al., 1989b; Ata et al., 2019; Chadchan et al., 2019; Le et al., 2021; Svensson et al., 2021). Nevertheless, these alterations in the GM can be considered the body’s response to EMS. The anabolism of BAs is a process involving self-feedback regulation in which FXR in the intestine and liver plays an essential role. The activation of intestinal FXR can upregulate mouse fibroblast growth factor (FGF)-15 and human FGF-19 to activate liver FGF receptor 4 (FGFR4) and inhibit the activity of liver cholesterol 7α-hydroxylase, a rate-limiting enzyme for the synthesis of CDCA and BAs (Gadaleta et al., 2015; Chambers et al., 2019). CDCA can activate intestinal FXR, which inhibits the continuous synthesis of CDCA through this negative feedback mechanism. An imbalance in the F/B ratio is widely regarded as a characteristic of GM dysbiosis (Turnbaugh et al., 2006). The GM has been found to display an imbalance in the F/B ratio in EMS (Ni et al., 1989a; Ni et al., 1989b; Ata et al., 2019; Chadchan et al., 2019), which has caused a series of metabolic disorders in the body. The imbalance in the F/B ratio may prevent CDCA from being metabolized into secondary BAs by related enzymes derived from the GM, causing CDCA to accumulate in the intestine. The abnormal F/B ratio may increase the expression of tauro-β-muricholic acid, a bile salt in the intestine of mice that is an effective antagonist of FXR. Tauro-β-muricholic acid inhibits FXR activation, enhances the synthesis of CDCA in the liver and affects enterohepatic circulation. These phenomena result in an increase in the level of CDCA (Sayin et al., 2013). In addition, the probiotic Lactobacillus rhamnosus GG (LGG) is reported to inhibit the synthesis of BAs and to promote the excretion of BAs through the intestinal FXR–FGF-15 signalling pathway (Liu et al., 2020), indicating that probiotics can reverse the imbalance in the GM and BA metabolism to a certain extent. A series of experiments have discussed the close relationship between CDCA metabolism and the GM. Although the experiments are not without limitations, they also provide a feasible explanation for how EMS indirectly affects the metabolic level of CDCA after changing the structure and diversity of the GM. Overall, CDCA has a two-way effect in the intestinal tract. The positive effect of CDCA is that it acts as a signalling molecule to activate related receptors to improve inflammation and reduce BA production, and its passive effect is that it decreases secondary BAs and an excess of primary BAs in the imbalanced GM in individuals with IBD (Sokol et al., 2009), which may lead to a decrease in FXR activity and the downregulation of OSTα and OSTβ expression levels. This phenomenon causes an accumulation of BAs in liver and intestinal mucosal cells, further aggravating the inflammatory phenotype (Gadaleta et al., 2011b; Ding et al., 2015). CDCA and the GM influence each other and jointly produce a gastrointestinal disease phenotype when disrupted. Therefore, in the treatment of gastrointestinal inflammatory diseases, alternatives to CDCA should be sought to reduce its side effects.
In addition, studies have reported that ectopic endometrial cell hyperproliferation in the pelvic cavity of EMS patients as well as triggering symptoms of pelvic pain result from elevated estrogen levels (Marquardt et al., 2019). The bidirectional interaction of high levels of estrogen in the EMS with the GM has been discussed above. Bile acid metabolism has recently been shown to influence estrogen levels, which are simultaneously regulated by bile acid receptor activation and the GM. Cholestatic bile acid levels induced FXR receptor activation and downregulated expression of the hepatic sulfotransferase SULT1E1, increasing levels of estrogen in the serum of mice (Liu et al., 2018). However, another study showed that appropriate concentrations of CDCA and DCA can reduce ESR1 expression in ovarian cancer cells by almost 90% (Jin et al., 2018), indicating that CDCA may reduce proliferation by inhibiting ESR1 expression in ectopic endometrial cells through targeted effects. Furthermore, subcutaneous administration of estrogens to mice induces cholestasis (Dong et al., 2019), which partially explains the abnormal levels of bile acid metabolism in the intestine of EMS mice. However, the mechanisms linking bile acid metabolism and estrogen levels in EMS remain unclear.
Recent studies have shown that IL-17 levels are generally elevated in peritoneal fluid and serum in women with EMS (Shi et al., 2022). IL-17 further leads to proliferation, growth and invasion of endometriotic lesions and promotes immune escape of ectopic lesions and the progression of EMS by inducing M2 macrophage differentiation (Shi et al., 2022). There are several factors that can cause an elevation in IL-17 levels. Specific BA derivatives have been found to act as key players in the control of IL-17-secreting Th17 cells. Two distinct derivatives of LCA, namely, 3-oxoLCA and isoLCA, inhibited the differentiation of Th17 cells by directly binding to the key transcription factor RORγt (Hang et al., 2019; Paik et al., 2022). In addition, the GM from an array of families within the Actinobacteria and Firmicutes phyla produces 3-oxoLCA, and hydroxysteroid dehydrogenases encoded by E. lenta and R. gnavus produce 3-oxoLCA and isoLCA (Paik et al., 2022). In summary, as a metabolite of CDCA, LCA is further converted into 3-oxoLCA and isoLCA under the biotransformation of specific GM, and the two derivatives may decrease the level of IL-17 in EMS. On the other hand, isoalloLCA, another LCA derivative, increases regulatory T cell (Treg cell) differentiation via producing mitochondrial reactive oxygen species (mitoROS) that promote FOXP3 expression (Hang et al., 2019). Another study reported that CDCA and its derivatives including (LCA and 3-oxoLCA) regulate RORγ+ Treg cells through the nuclear receptor VDR (Song et al., 2020). And an imbalance in the Th17/Treg cell ratio leads to ectopic endometrial lesion survival and implantation (Shi et al., 2022). Therefore, specific primary and secondary BAs regulate the Th17/Treg cell ratio, which may influence the progression of EMS.
From the perspective of metabolism, CDCA, as a lipid solubilizer or as a signalling molecule that activates different receptors, can regulate body homeostasis and maintain metabolic balance. CDCA can dissolve cholesterol stones, reduce total cholesterol in bile, and improve glucose homeostasis and lipid and lipoprotein metabolism (Tint et al., 1986; de Aguiar Vallim et al., 2013). In patients and mice with EMS, manifestations, such as a reduction in human adipose stem cells and lipid metabolism disorders in mice, are related to abnormal lipid metabolism (Dutta et al., 2016; Zolbin et al., 2019). Therefore, CDCA may also regulate lipid metabolism to produce PUFAs to exert its anti-inflammatory effect or accelerate fat breakdown to provide the body with the necessary energy to adapt to the inflammatory response.
6-Alpha-ethyl-chenodeoxycholic acid (OCA), which was first discovered while studying FXR receptor ligands, is obtained by adding an ethyl group to the 6-position of the benzene ring of CDCA and has improved physicochemical properties (Pellicciari et al., 2002). Subsequent studies have found that the efficiency of OCA in the activation of FXR is 16–33 times that of the natural ligand CDCA (Pellicciari et al., 2002), and the efficiency in activating TGR5 is close to that of LCA as the natural ligand (Rizzo et al., 2010). In animal experiments, OCA protected against DSS-induced damage in mice, reduced the severity of the disease, and maintained the integrity of the intestinal epithelial barrier (Gadaleta et al., 2011a). At present, OCA was one of the BA receptor modulators approved by the FDA for the clinical treatment of primary biliary cholangitis and effectively reversed or reduced the degree of liver damage in 2016 (Nevens et al., 2016). In a phase III clinical trial of OCA for the treatment of nonalcoholic steatohepatitis (NASH), OCA effectively improved liver fibrosis in patients (Younossi et al., 2019). In recent years, studies have shown that OCA can change the characteristics of the GM of mice and humans and reduce the level of endogenous BAs by activating FXR (Friedman et al., 2018). A high-fat diet can induce damage to the intestinal epithelial barrier and intestinal vascular barrier in mice, leading to the translocation of intestinal bacteria or bacterial products into the blood circulation. OCA can drive the activation of β-catenin in endothelial cells to prevent damage to the intestinal epithelial and intestinal vascular barriers and the progression of NASH (Mouries et al., 2019). In addition, OCA can improve the complications associated with the metabolic disorder caused by GM-related high-fat and high-glucose diets, thereby reversing intestinal barrier dysfunction and restoring serum LPS to normal levels (Wu et al., 2021). Furthermore, the TGR5 agonist INT-777 showed therapeutic implications for EMS by mitigating the inflammatory response in human endometriotic stromal cells (Lyu et al., 2019).
Intestinal dysbiosis destroys normal immune function and leads to chronic inflammation by changing the spectrum of immune cells and promoting the release of inflammatory cytokines. This chronic inflammatory state creates an ideal environment conducive to increased tissue adhesion and angiogenesis, which may lead to the development of EMS. Moreover, EMS can induce changes in the structure of the GM and possibly increase the abundance of potentially pathogenic species, which may cause a vicious cycle between intestinal dysbiosis and EMS. Abnormal estrogen metabolism and changes in the levels of macrophages and inflammatory cytokines provide a possible explanation for the vicious cycle between EMS and intestinal dysbiosis, but several unknowns related to the GM remain. CDCA can regulate the intestinal immune system and GM through its action as a signalling molecule. In this review, we propose the possibility of CDCA and its derivatives as targeted therapeutic drugs for EMS and theoretically discuss the role of CDCA in EMS via the biotransformation of the GM and BA receptors. Several potential approaches that CDCA and its derivatives to treatment of EMS are amelioration of the disordered GM by activating BA receptors and regulation of Th17/Treg cells by CDCA derivatives binding to RORγt and promoting mitoROS production. Furthermore, CDCA can regulate the intestinal inflammatory environment and the GM, which may further improve the activity of β-glucosidase that restores normal levels of estrogen. In addition, effort is still needed in the future to determine the “core” GM that plays a central role in EMS and to clarify the mechanism underlying the relationship between BAs/BA receptor agonists and EMS.
Conceptualization, CY and ZN; visualization, YL, KW, and JD; writing—original draft preparation, YL, KW and JD; writing—review and editing, YL, KW, JD and ZN; supervision, SS and CY. All authors have read and agreed to the published version of the manuscript.
This research was funded by National Natural Science Foundation of China (82074206, 82004408) and Technology Innovation Action Plan of Shanghai Science and Technology Commission (No. 21Y21920500).
The authors declare that the research was conducted in the absence of any commercial or financial relationships that could be construed as a potential conflict of interest.
All claims expressed in this article are solely those of the authors and do not necessarily represent those of their affiliated organizations, or those of the publisher, the editors and the reviewers. Any product that may be evaluated in this article, or claim that may be made by its manufacturer, is not guaranteed or endorsed by the publisher.
Agostinis, C., Zorzet, S., Balduit, A., Zito, G., Mangogna, A., Macor, P., et al. (2021). The inflammatory feed-forward loop triggered by the complement component C3 as a potential target in endometriosis. Front. Immunol. 12, 693118. doi:10.3389/fimmu.2021.693118
Ang, Q. Y., Alexander, M., Newman, J. C., Tian, Y., Cai, J., Upadhyay, V., et al. (2020). Ketogenic diets alter the gut microbiome resulting in decreased intestinal Th17 cells. Cell. 181 (6), 1263–1275. e16. doi:10.1016/j.cell.2020.04.027
Ata, B., Yildiz, S., Turkgeldi, E., Brocal, V. P., Dinleyici, E. C., Moya, A., et al. (2019). The endobiota study: Comparison of vaginal, cervical and gut microbiota between women with stage 3/4 endometriosis and healthy controls. Sci. Rep. 9 (1), 2204. doi:10.1038/s41598-019-39700-6
Attaman, J. A., Stanic, A. K., Kim, M., Lynch, M. P., Rueda, B. R., and Styer, A. K. (2014). The anti-inflammatory impact of omega-3 polyunsaturated Fatty acids during the establishment of endometriosis-like lesions. Am. J. Reprod. Immunol. 72 (4), 392–402. doi:10.1111/aji.12276
Azuma, Y., Taniguchi, F., Nakamura, K., Nagira, K., Khine, Y. M., Kiyama, T., et al. (2017). Lipopolysaccharide promotes the development of murine endometriosis-like lesions via the nuclear factor-kappa B pathway. Am. J. Reprod. Immunol. 77 (4). doi:10.1111/aji.12631
Baker, J. M., Al-Nakkash, L., and Herbst-Kralovetz, M. M. (2017). Estrogen-gut microbiome axis: Physiological and clinical implications. Maturitas 103, 45–53. doi:10.1016/j.maturitas.2017.06.025
Biagioli, M., Carino, A., Cipriani, S., Francisci, D., Marchianò, S., Scarpelli, P., et al. (2017). The bile acid receptor GPBAR1 regulates the M1/M2 phenotype of intestinal macrophages and activation of GPBAR1 rescues mice from murine colitis. J. Immunol. 199 (2), 718–733. doi:10.4049/jimmunol.1700183
Budden, K. F., Gellatly, S. L., Wood, D. L., Cooper, M. A., Morrison, M., Hugenholtz, P., et al. (2017). Emerging pathogenic links between microbiota and the gut-lung axis. Nat. Rev. Microbiol. 15 (1), 55–63. doi:10.1038/nrmicro.2016.142
Bulun, S. E., Yilmaz, B. D., Sison, C., Miyazaki, K., Bernardi, L., Liu, S., et al. (2019). Endometriosis. Endocr. Rev. 40 (4), 1048–1079. doi:10.1210/er.2018-00242
Cai, J., Sun, L., and Gonzalez, F. J. (2022). Gut microbiota-derived bile acids in intestinal immunity, inflammation, and tumorigenesis. Cell. Host Microbe 30 (3), 289–300. doi:10.1016/j.chom.2022.02.004
Cao, Y., Jiang, C., Jia, Y., Xu, D., and Yu, Y. (2020). Letrozole and the traditional Chinese medicine, shaofu zhuyu decoction, reduce endometriotic disease progression in rats: A potential role for gut microbiota. Evid. Based. Complement. Altern. Med. 2020, 3687498. doi:10.1155/2020/3687498
Chadchan, S. B., Cheng, M., Parnell, L. A., Yin, Y., Schriefer, A., Mysorekar, I. U., et al. (2019). Antibiotic therapy with metronidazole reduces endometriosis disease progression in mice: A potential role for gut microbiota. Hum. Reprod. 34 (6), 1106–1116. doi:10.1093/humrep/dez041
Chadchan, S. B., Popli, P., Ambati, C. R., Tycksen, E., Han, S. J., Bulun, S. E., et al. (2021). Gut microbiota-derived short-chain fatty acids protect against the progression of endometriosis. Life Sci. Alliance 4 (12), e202101224. doi:10.26508/lsa.202101224
Chambers, K. F., Day, P. E., Aboufarrag, H. T., and Kroon, P. A. (2019). Polyphenol effects on cholesterol metabolism via bile acid biosynthesis, CYP7A1: A review. Nutrients 11 (11), E2588. doi:10.3390/nu11112588
Chen, M. L., and Li, C. Y. (2021). Thoracic endometriosis. N. Engl. J. Med. 385 (19), e65. doi:10.1056/NEJMicm2105508
Chenodeoxycholic acid in the dissolution of gallstones. Nutr. Rev. 1976;34(1):20–21. doi:10.1111/j.1753-4887.1976.tb05664.x
Cipriani, S., Mencarelli, A., Chini, M. G., Distrutti, E., Renga, B., Bifulco, G., et al. (2011). The bile acid receptor GPBAR-1 (TGR5) modulates integrity of intestinal barrier and immune response to experimental colitis. PLoS One 6 (10), e25637. doi:10.1371/journal.pone.0025637
Clemente, J. C., Manasson, J., and Scher, J. U. (2018). The role of the gut microbiome in systemic inflammatory disease. Bmj 360, j5145. doi:10.1136/bmj.j5145
Dawson, P. A., and Karpen, S. J. (2015). Intestinal transport and metabolism of bile acids. J. Lipid Res. 56 (6), 1085–1099. doi:10.1194/jlr.R054114
de Aguiar Vallim, T. Q., Tarling, E. J., and Edwards, P. A. (2013). Pleiotropic roles of bile acids in metabolism. Cell. Metab. 17 (5), 657–669. doi:10.1016/j.cmet.2013.03.013
Delbandi, A. A., Mahmoudi, M., Shervin, A., and Zarnani, A. H. (2016). 1, 25-dihydroxy vitamin D3 modulates endometriosis-related features of human endometriotic stromal cells. Am. J. Reprod. Immunol. 75 (4), 461–473. doi:10.1111/aji.12463
Ding, L., Yang, L., Wang, Z., and Huang, W. (2015). Bile acid nuclear receptor FXR and digestive system diseases. Acta Pharm. Sin. B 5 (2), 135–144. doi:10.1016/j.apsb.2015.01.004
Dong, R., Wang, J., Gao, X., Wang, C., Liu, K., Wu, J., et al. (2019). Yangonin protects against estrogen-induced cholestasis in a farnesoid X receptor-dependent manner. Eur. J. Pharmacol. 857, 172461. doi:10.1016/j.ejphar.2019.172461
Dutta, M., Anitha, M., Smith, P. B., Chiaro, C. R., Maan, M., Chaudhury, K., et al. (2016). Metabolomics reveals altered lipid metabolism in a mouse model of endometriosis. J. Proteome Res. 15 (8), 2626–2633. doi:10.1021/acs.jproteome.6b00197
Ervin, S. M., Li, H., Lim, L., Roberts, L. R., Liang, X., Mani, S., et al. (2019). Gut microbial β-glucuronidases reactivate estrogens as components of the estrobolome that reactivate estrogens. J. Biol. Chem. 294 (49), 18586–18599. doi:10.1074/jbc.RA119.010950
Friedman, E. S., Li, Y., Shen, T. D., Jiang, J., Chau, L., Adorini, L., et al. (2018). FXR-dependent modulation of the human small intestinal microbiome by the bile acid derivative obeticholic acid. Gastroenterology 155 (6), 1741–1752. e5. doi:10.1053/j.gastro.2018.08.022
Gadaleta, R. M., Cariello, M., Sabbà, C., and Moschetta, A. (2015). Tissue-specific actions of FXR in metabolism and cancer. Biochim. Biophys. Acta 1851 (1), 30–39. doi:10.1016/j.bbalip.2014.08.005
Gadaleta, R. M., Oldenburg, B., Willemsen, E. C., Spit, M., Murzilli, S., Salvatore, L., et al. (2011). Activation of bile salt nuclear receptor FXR is repressed by pro-inflammatory cytokines activating NF-κB signaling in the intestine. Biochim. Biophys. Acta 1812 (8), 851–858. doi:10.1016/j.bbadis.2011.04.005
Gadaleta, R. M., van Erpecum, K. J., Oldenburg, B., Willemsen, E. C., Renooij, W., Murzilli, S., et al. (2011). Farnesoid X receptor activation inhibits inflammation and preserves the intestinal barrier in inflammatory bowel disease. Gut 60 (4), 463–472. doi:10.1136/gut.2010.212159
Gadaleta, R. M., van Mil, S. W., Oldenburg, B., Siersema, P. D., Klomp, L. W., and van Erpecum, K. J. (2010). Bile acids and their nuclear receptor FXR: Relevance for hepatobiliary and gastrointestinal disease. Biochim. Biophys. Acta 1801 (7), 683–692. doi:10.1016/j.bbalip.2010.04.006
Ghanavatinejad, A., Rashidi, N., Mirahmadian, M., Rezania, S., Mosalaei, M., Ghasemi, J., et al. (2021). Vitamin D3 controls TLR4- and TLR2-mediated inflammatory responses of endometrial cells. Gynecol. Obstet. Investig. 86 (1-2), 139–148. doi:10.1159/000513590
Hang, S., Paik, D., Yao, L., Kim, E., Trinath, J., Lu, J., et al. (2019). Bile acid metabolites control T(H)17 and T(reg) cell differentiation. Nature 576 (7785), 143–148. doi:10.1038/s41586-019-1785-z
Harada, T., Iwabe, T., and Terakawa, N. (2001). Role of cytokines in endometriosis. Fertil. Steril. 76 (1), 1–10. doi:10.1016/s0015-0282(01)01816-7
Henke, M. T., Kenny, D. J., Cassilly, C. D., Vlamakis, H., Xavier, R. J., and Clardy, J. (2019). Ruminococcus gnavus, a member of the human gut microbiome associated with Crohn's disease, produces an inflammatory polysaccharide. Proc. Natl. Acad. Sci. U. S. A. 116 (26), 12672–12677. doi:10.1073/pnas.1904099116
Hofmann, A. F. (2009). The enterohepatic circulation of bile acids in mammals: Form and functions. Front. Biosci. 14, 2584–2598. doi:10.2741/3399
Homma, H., Hoy, E., Xu, D. Z., Lu, Q., Feinman, R., and Deitch, E. A. (2005). The female intestine is more resistant than the male intestine to gut injury and inflammation when subjected to conditions associated with shock states. Am. J. Physiol. Gastrointest. Liver Physiol. 288 (3), G466–G472. doi:10.1152/ajpgi.00036.2004
Huang, L., Liu, B., Liu, Z., Feng, W., Liu, M., Wang, Y., et al. (2021). Gut microbiota exceeds cervical microbiota for early diagnosis of endometriosis. Front. Cell. Infect. Microbiol. 11, 788836. doi:10.3389/fcimb.2021.788836
Ichikawa, R., Takayama, T., Yoneno, K., Kamada, N., Kitazume, M. T., Higuchi, H., et al. (2012). Bile acids induce monocyte differentiation toward interleukin-12 hypo-producing dendritic cells via a TGR5-dependent pathway. Immunology 136 (2), 153–162. doi:10.1111/j.1365-2567.2012.03554.x
Inagaki, T., Moschetta, A., Lee, Y. K., Peng, L., Zhao, G., Downes, M., et al. (2006). Regulation of antibacterial defense in the small intestine by the nuclear bile acid receptor. Proc. Natl. Acad. Sci. U. S. A. 103 (10), 3920–3925. doi:10.1073/pnas.0509592103
Jeljeli, M., Riccio, L. G. C., Chouzenoux, S., Moresi, F., Toullec, L., Doridot, L., et al. (2020). Macrophage immune memory controls endometriosis in mice and humans. Cell. Rep. 33 (5), 108325. doi:10.1016/j.celrep.2020.108325
Jia, W., Xie, G., and Jia, W. (2018). Bile acid-microbiota crosstalk in gastrointestinal inflammation and carcinogenesis. Nat. Rev. Gastroenterol. Hepatol. 15 (2), 111–128. doi:10.1038/nrgastro.2017.119
Jin, Q., Noel, O., Nguyen, M., Sam, L., and Gerhard, G. S. (2018). Bile acids upregulate BRCA1 and downregulate estrogen receptor 1 gene expression in ovarian cancer cells. Eur. J. Cancer Prev. 27 (6), 553–556. doi:10.1097/CEJ.0000000000000398
Karmarkar, D., and Rock, K. L. (2013). Microbiota signalling through MyD88 is necessary for a systemic neutrophilic inflammatory response. Immunology 140 (4), 483–492. doi:10.1111/imm.12159
Kawamata, Y., Fujii, R., Hosoya, M., Harada, M., Yoshida, H., Miwa, M., et al. (2003). A G protein-coupled receptor responsive to bile acids. J. Biol. Chem. 278 (11), 9435–9440. doi:10.1074/jbc.M209706200
Keitel, V., Donner, M., Winandy, S., Kubitz, R., and Häussinger, D. (2008). Expression and function of the bile acid receptor TGR5 in Kupffer cells. Biochem. Biophys. Res. Commun. 372 (1), 78–84. doi:10.1016/j.bbrc.2008.04.171
Le, N., Cregger, M., Brown, V., Loret de Mola, J., Bremer, P., Nguyen, L., et al. (2021). Association of microbial dynamics with urinary estrogens and estrogen metabolites in patients with endometriosis. PLoS One 16 (12), e0261362. doi:10.1371/journal.pone.0261362
Liu, X., Xue, R., Yang, C., Gu, J., Chen, S., and Zhang, S. (2018). Cholestasis-induced bile acid elevates estrogen level via farnesoid X receptor-mediated suppression of the estrogen sulfotransferase SULT1E1. J. Biol. Chem. 293 (33), 12759–12769. doi:10.1074/jbc.RA118.001789
Liu, Y., Chen, K., Li, F., Gu, Z., Liu, Q., He, L., et al. (2020). Probiotic Lactobacillus rhamnosus GG prevents liver fibrosis through inhibiting hepatic bile acid synthesis and enhancing bile acid excretion in mice. Hepatology 71 (6), 2050–2066. doi:10.1002/hep.30975
Lousse, J. C., Defrère, S., Van Langendonckt, A., Gras, J., González-Ramos, R., Colette, S., et al. (2009). Iron storage is significantly increased in peritoneal macrophages of endometriosis patients and correlates with iron overload in peritoneal fluid. Fertil. Steril. 91 (5), 1668–1675. doi:10.1016/j.fertnstert.2008.02.103
Lyu, D., Tang, N., Wang, J., Zhang, Y., Chang, J., Liu, Z., et al. (2019). TGR5 agonist INT-777 mitigates inflammatory response in human endometriotic stromal cells: A therapeutic implication for endometriosis. Int. Immunopharmacol. 71, 93–99. doi:10.1016/j.intimp.2019.02.044
Ma, C., Han, M., Heinrich, B., Fu, Q., Zhang, Q., Sandhu, M., et al. (2018). Gut microbiome-mediated bile acid metabolism regulates liver cancer via NKT cells. Science 360 (6391), eaan5931. doi:10.1126/science.aan5931
Marquardt, R. M., Kim, T. H., Shin, J. H., and Jeong, J. W. (2019). Progesterone and estrogen signaling in the endometrium: What goes wrong in endometriosis? Int. J. Mol. Sci. 20 (15), E3822. doi:10.3390/ijms20153822
Maruyama, T., Miyamoto, Y., Nakamura, T., Tamai, Y., Okada, H., Sugiyama, E., et al. (2002). Identification of membrane-type receptor for bile acids (M-BAR). Biochem. Biophys. Res. Commun. 298 (5), 714–719. doi:10.1016/s0006-291x(02)02550-0
McMahan, R. H., Wang, X. X., Cheng, L. L., Krisko, T., Smith, M., El Kasmi, K., et al. (2013). Bile acid receptor activation modulates hepatic monocyte activity and improves nonalcoholic fatty liver disease. J. Biol. Chem. 288 (17), 11761–11770. doi:10.1074/jbc.M112.446575
Meggyesy, M., Friese, M., Gottschalk, J., and Kehler, U. (2020). Case report of cerebellar endometriosis. J. Neurol. Surg. A Cent. Eur. Neurosurg. 81 (4), 372–376. doi:10.1055/s-0040-1701622
Meroni, M., Longo, M., and Dongiovanni, P. (2019). Alcohol or gut microbiota: Who is the guilty? Int. J. Mol. Sci. 20 (18), E4568. doi:10.3390/ijms20184568
Mouries, J., Brescia, P., Silvestri, A., Spadoni, I., Sorribas, M., Wiest, R., et al. (2019). Microbiota-driven gut vascular barrier disruption is a prerequisite for non-alcoholic steatohepatitis development. J. Hepatol. 71 (6), 1216–1228. doi:10.1016/j.jhep.2019.08.005
Nevens, F., Andreone, P., Mazzella, G., Strasser, S. I., Bowlus, C., Invernizzi, P., et al. (2016). A placebo-controlled trial of obeticholic acid in primary biliary cholangitis. N. Engl. J. Med. 375 (7), 631–643. doi:10.1056/NEJMoa1509840
Ni, Z., Ding, J., Zhao, Q., Cheng, W., Yu, J., Zhou, L., et al. (2021). Alpha-linolenic acid regulates the gut microbiota and the inflammatory environment in a mouse model of endometriosis. Am. J. Reprod. Immunol. 86 (4), e13471. doi:10.1111/aji.13471
Ni, Z., Sun, S., Bi, Y., Ding, J., Cheng, W., Yu, J., et al. (2020). Correlation of fecal metabolomics and gut microbiota in mice with endometriosis. Am. J. Reprod. Immunol. 84 (6), e13307. doi:10.1111/aji.13307
Nodler, J. L., DiVasta, A. D., Vitonis, A. F., Karevicius, S., Malsch, M., Sarda, V., et al. (2020). Supplementation with vitamin D or ω-3 fatty acids in adolescent girls and young women with endometriosis (SAGE): A double-blind, randomized, placebo-controlled trial. Am. J. Clin. Nutr. 112 (1), 229–236. doi:10.1093/ajcn/nqaa096
Nothnick, W. B. (2001). Treating endometriosis as an autoimmune disease. Fertil. Steril. 76 (2), 223–231. doi:10.1016/s0015-0282(01)01878-7
O'Hara, A. M., and Shanahan, F. (2006). The gut flora as a forgotten organ. EMBO Rep. 7 (7), 688–693. doi:10.1038/sj.embor.7400731
Paik, D., Yao, L., Zhang, Y., Bae, S., D'Agostino, G. D., Zhang, M., et al. (2022). Human gut bacteria produce Τ(Η)17-modulating bile acid metabolites. Nature 603 (7903), 907–912. doi:10.1038/s41586-022-04480-z
Parazzini, F., Viganò, P., Candiani, M., and Fedele, L. (2013). Diet and endometriosis risk: A literature review. Reprod. Biomed. Online 26 (4), 323–336. doi:10.1016/j.rbmo.2012.12.011
Pellicciari, R., Fiorucci, S., Camaioni, E., Clerici, C., Costantino, G., Maloney, P. R., et al. (2002). 6alpha-ethyl-chenodeoxycholic acid (6-ECDCA), a potent and selective FXR agonist endowed with anticholestatic activity. J. Med. Chem. 45 (17), 3569–3572. doi:10.1021/jm025529g
Raybould, H. E. (2012). Gut microbiota, epithelial function and derangements in obesity. J. Physiol. 590 (3), 441–446. doi:10.1113/jphysiol.2011.222133
Ridlon, J. M., Kang, D. J., Hylemon, P. B., and Bajaj, J. S. (2014). Bile acids and the gut microbiome. Curr. Opin. Gastroenterol. 30 (3), 332–338. doi:10.1097/MOG.0000000000000057
Rizzo, G., Passeri, D., De Franco, F., Ciaccioli, G., Donadio, L., Rizzo, G., et al. (2010). Functional characterization of the semisynthetic bile acid derivative INT-767, a dual farnesoid X receptor and TGR5 agonist. Mol. Pharmacol. 78 (4), 617–630. doi:10.1124/mol.110.064501
Rooks, M. G., and Garrett, W. S. (2016). Gut microbiota, metabolites and host immunity. Nat. Rev. Immunol. 16 (6), 341–352. doi:10.1038/nri.2016.42
Sayin, S. I., Wahlström, A., Felin, J., Jäntti, S., Marschall, H. U., Bamberg, K., et al. (2013). Gut microbiota regulates bile acid metabolism by reducing the levels of tauro-beta-muricholic acid, a naturally occurring FXR antagonist. Cell. Metab. 17 (2), 225–235. doi:10.1016/j.cmet.2013.01.003
Shan, J., Ni, Z., Cheng, W., Zhou, L., Zhai, D., Sun, S., et al. (2021). Gut microbiota imbalance and its correlations with hormone and inflammatory factors in patients with stage 3/4 endometriosis. Arch. Gynecol. Obstet. 304 (5), 1363–1373. doi:10.1007/s00404-021-06057-z
Sharon, G., Sampson, T. R., Geschwind, D. H., and Mazmanian, S. K. (2016). The central nervous system and the gut microbiome. Cell. 167 (4), 915–932. doi:10.1016/j.cell.2016.10.027
Shi, J. L., Zheng, Z. M., Chen, M., Shen, H. H., Li, M. Q., and Shao, J. (2022). IL-17: An important pathogenic factor in endometriosis. Int. J. Med. Sci. 19 (4), 769–778. doi:10.7150/ijms.71972
Smythies, L. E., Sellers, M., Clements, R. H., Mosteller-Barnum, M., Meng, G., Benjamin, W. H., et al. (2005). Human intestinal macrophages display profound inflammatory anergy despite avid phagocytic and bacteriocidal activity. J. Clin. Investig. 115 (1), 66–75. doi:10.1172/JCI19229
Sokol, H., Seksik, P., Furet, J. P., Firmesse, O., Nion-Larmurier, I., Beaugerie, L., et al. (2009). Low counts of Faecalibacterium prausnitzii in colitis microbiota. Inflamm. Bowel Dis. 15 (8), 1183–1189. doi:10.1002/ibd.20903
Song, M., Ye, J., Zhang, F., Su, H., Yang, X., He, H., et al. (2019). Chenodeoxycholic acid (CDCA) protects against the lipopolysaccharide-induced impairment of the intestinal epithelial barrier function via the FXR-MLCK pathway. J. Agric. Food Chem. 67 (32), 8868–8874. doi:10.1021/acs.jafc.9b03173
Song, X., Sun, X., Oh, S. F., Wu, M., Zhang, Y., Zheng, W., et al. (2020). Microbial bile acid metabolites modulate gut RORγ(+) regulatory T cell homeostasis. Nature 577 (7790), 410–415. doi:10.1038/s41586-019-1865-0
Sorbara, M. T., Littmann, E. R., Fontana, E., Moody, T. U., Kohout, C. E., Gjonbalaj, M., et al. (2020). Functional and genomic variation between human-derived isolates of Lachnospiraceae reveals inter- and intra-species diversity. Cell. Host Microbe 28 (1), 134–146. doi:10.1016/j.chom.2020.05.005
Sorribas, M., Jakob, M. O., Yilmaz, B., Li, H., Stutz, D., Noser, Y., et al. (2019). FXR modulates the gut-vascular barrier by regulating the entry sites for bacterial translocation in experimental cirrhosis. J. Hepatol. 71 (6), 1126–1140. doi:10.1016/j.jhep.2019.06.017
Surrey, E., Soliman, A. M., Trenz, H., Blauer-Peterson, C., and Sluis, A. (2020). Impact of endometriosis diagnostic delays on healthcare resource utilization and costs. Adv. Ther. 37 (3), 1087–1099. doi:10.1007/s12325-019-01215-x
Svensson, A., Brunkwall, L., Roth, B., Orho-Melander, M., and Ohlsson, B. (2021). Associations between endometriosis and gut microbiota. Reprod. Sci. 28 (8), 2367–2377. doi:10.1007/s43032-021-00506-5
Symons, L. K., Miller, J. E., Kay, V. R., Marks, R. M., Liblik, K., Koti, M., et al. (2018). The immunopathophysiology of endometriosis. Trends Mol. Med. 24 (9), 748–762. doi:10.1016/j.molmed.2018.07.004
Tang, W. H., Kitai, T., and Hazen, S. L. (2017). Gut microbiota in cardiovascular health and disease. Circ. Res. 120 (7), 1183–1196. doi:10.1161/CIRCRESAHA.117.309715
Thomas, C., Pellicciari, R., Pruzanski, M., Auwerx, J., and Schoonjans, K. (2008). Targeting bile-acid signalling for metabolic diseases. Nat. Rev. Drug Discov. 7 (8), 678–693. doi:10.1038/nrd2619
Tint, G. S., Salen, G., and Shefer, S. (1986). Effect of ursodeoxycholic acid and chenodeoxycholic acid on cholesterol and bile acid metabolism. Gastroenterology 91 (4), 1007–1018. doi:10.1016/0016-5085(86)90708-0
Turnbaugh, P. J., Ley, R. E., Mahowald, M. A., Magrini, V., Mardis, E. R., and Gordon, J. I. (2006). An obesity-associated gut microbiome with increased capacity for energy harvest. Nature 444 (7122), 1027–1031. doi:10.1038/nature05414
Vallvé-Juanico, J., Santamaria, X., Vo, K. C., Houshdaran, S., and Giudice, L. C. (2019). Macrophages display proinflammatory phenotypes in the eutopic endometrium of women with endometriosis with relevance to an infectious etiology of the disease. Fertil. Steril. 112 (6), 1118–1128. doi:10.1016/j.fertnstert.2019.08.060
Vavassori, P., Mencarelli, A., Renga, B., Distrutti, E., and Fiorucci, S. (2009). The bile acid receptor FXR is a modulator of intestinal innate immunity. J. Immunol. 183 (10), 6251–6261. doi:10.4049/jimmunol.0803978
Vercellini, P., Viganò, P., Somigliana, E., and Fedele, L. (2014). Endometriosis: Pathogenesis and treatment. Nat. Rev. Endocrinol. 10 (5), 261–275. doi:10.1038/nrendo.2013.255
Wanderley, C. W., Colón, D. F., Luiz, J. P. M., Oliveira, F. F., Viacava, P. R., Leite, C. A., et al. (2018). Paclitaxel reduces tumor growth by reprogramming tumor-associated macrophages to an M1 profile in a TLR4-dependent manner. Cancer Res. 78 (20), 5891–5900. doi:10.1158/0008-5472.CAN-17-3480
Wilck, N., Matus, M. G., Kearney, S. M., Olesen, S. W., Forslund, K., Bartolomaeus, H., et al. (2017). Salt-responsive gut commensal modulates T(H)17 axis and disease. Nature 551 (7682), 585–589. doi:10.1038/nature24628
Woo, J. H., Choi, Y. S., and Choi, J. H. (2020). Iron-Storage protein ferritin is upregulated in endometriosis and iron overload contributes to a migratory phenotype. Biomedicines 8 (11), E454. doi:10.3390/biomedicines8110454
Wu, L., Han, Y., Zheng, Z., Zhu, S., Chen, J., Yao, Y., et al. (2021). Obeticholic acid inhibits anxiety via alleviating gut microbiota-mediated microglia accumulation in the brain of high-fat high-sugar diet mice. Nutrients 13 (3), 940. doi:10.3390/nu13030940
Younossi, Z. M., Ratziu, V., Loomba, R., Rinella, M., Anstee, Q. M., Goodman, Z., et al. (2019). Obeticholic acid for the treatment of non-alcoholic steatohepatitis: Interim analysis from a multicentre, randomised, placebo-controlled phase 3 trial. Lancet 394 (10215), 2184–2196. doi:10.1016/S0140-6736(19)33041-7
Yuan, M., Li, D., Zhang, Z., Sun, H., An, M., and Wang, G. (2018). Endometriosis induces gut microbiota alterations in mice. Hum. Reprod. 33 (4), 607–616. doi:10.1093/humrep/dex372
Zhang, J., Feng, F., and Zhao, M. (2021). Glycerol monocaprylate modulates gut microbiota and increases short-chain fatty acids production without adverse effects on metabolism and inflammation. Nutrients 13 (5), 1427. doi:10.3390/nu13051427
Zhang, Y. Z., and Li, Y. Y. (2014). Inflammatory bowel disease: Pathogenesis. World J. Gastroenterol. 20 (1), 91–99. doi:10.3748/wjg.v20.i1.91
Zolbin, M. M., Mamillapalli, R., Nematian, S. E., Goetz, T. G., and Taylor, H. S. (2019). Adipocyte alterations in endometriosis: Reduced numbers of stem cells and microRNA induced alterations in adipocyte metabolic gene expression. Reprod. Biol. Endocrinol. 17 (1), 36. doi:10.1186/s12958-019-0480-0
Keywords: chenodeoxycholic acid, gut microbiota, endometriosis, inflammation, bile acids
Citation: Li Y, Wang K, Ding J, Sun S, Ni Z and Yu C (2022) Influence of the gut microbiota on endometriosis: Potential role of chenodeoxycholic acid and its derivatives. Front. Pharmacol. 13:954684. doi: 10.3389/fphar.2022.954684
Received: 27 May 2022; Accepted: 27 July 2022;
Published: 22 August 2022.
Edited by:
Ping Zhou, Chinese Academy of Medical Sciences and Peking Union Medical College, ChinaReviewed by:
Tao Yang, Guizhou University of Traditional Chinese Medicine, ChinaCopyright © 2022 Li, Wang, Ding, Sun, Ni and Yu. This is an open-access article distributed under the terms of the Creative Commons Attribution License (CC BY). The use, distribution or reproduction in other forums is permitted, provided the original author(s) and the copyright owner(s) are credited and that the original publication in this journal is cited, in accordance with accepted academic practice. No use, distribution or reproduction is permitted which does not comply with these terms.
*Correspondence: Zhexin Ni, bml6eHpnQDE2My5jb20=; Chaoqin Yu, Y2hxeXU4MUAxNjMuY29t
†These authors have contributed equally to this work
Disclaimer: All claims expressed in this article are solely those of the authors and do not necessarily represent those of their affiliated organizations, or those of the publisher, the editors and the reviewers. Any product that may be evaluated in this article or claim that may be made by its manufacturer is not guaranteed or endorsed by the publisher.
Research integrity at Frontiers
Learn more about the work of our research integrity team to safeguard the quality of each article we publish.