- 1Institute of Medicinal Plant Development, Peking Union Medical College and Chinese Academy of Medical Sciences, Beijing, China
- 2Beijing Key Laboratory of Innovative Drug Discovery of Traditional Chinese Medicine (Natural Medicine) and Translational Medicine, Beijing, China
- 3Key Laboratory of Bioactive Substances and Resource Utilization of Chinese Herbal Medicine, Ministry of Education, Beijing, China
- 4Yunnan Branch, Institute of Medicinal Plant Development, Chinese Academy of Medical Sciences and Peking Union Medical College, Jinghong, China
- 5Yunnan Key Laboratory of Southern Medicine Utilization, Kunming, China
- 6College of Pharmacy, Heilongjiang University of Chinese Medicine, Harbin, China
Diabetic retinopathy (DR), a leading cause of vision loss and blindness worldwide, is caused by retinal neurovascular unit dysfunction, and its cellular pathology involves at least nine kinds of retinal cells, including photoreceptors, horizontal and bipolar cells, amacrine cells, retinal ganglion cells, glial cells (Müller cells, astrocytes, and microglia), endothelial cells, pericytes, and retinal pigment epithelial cells. Its mechanism is complicated and involves loss of cells, inflammatory factor production, neovascularization, and BRB impairment. However, the mechanism has not been completely elucidated. Drug treatment for DR has been gradually advancing recently. Research on potential drug targets relies upon clear information on pathogenesis and effective biomarkers. Therefore, we reviewed the recent literature on the cellular pathology and the diagnostic and prognostic biomarkers of DR in terms of blood, protein, and clinical and preclinical drug therapy (including synthesized molecules and natural molecules). This review may provide a theoretical basis for further DR research.
1 Introduction
Diabetic retinopathy (DR) is one of the most serious complications of diabetes mellitus and the leading cause of vision loss and blindness worldwide. The annual incidence of DR ranges from 2.2%to 12.7% due to differences in research samples across studies, such as the number of people, geographical distribution, age, and sex (Thomas R. L. et al., 2019). According to recent studies, DR is not only a diabetic microvascular complication, but also a neurodegenerative disease. Therefore, DR has recently been defined by the American Diabetes Association as a highly tissue-specific neurovascular complication of both type 1 and type 2 diabetes (Flaxel et al., 2020). In fact, the number of patients with type 2 diabetes is much higher than those with type 1 diabetes; thus, those with type 2 diabetes comprise a larger proportion of patients with DR (Flaxel et al., 2020). However, the current measure for the stages of DR is based on clinically visible retinal microvascular changes and does not include neurodegenerative lesions that might occur earlier (Abramoff et al., 2018). According to the International Council of Ophthalmology, DR can be divided into non-proliferative (NPDR) and proliferative DR (PDR), covering four overlapping stages (see Table 1 for definitions) (Wong et al., 2018; Yang et al., 2020).
In the retina, retinal neurons [photoreceptors: cones and rods, horizontal and bipolar cells, amacrine cells, and retinal ganglion cells (RGCs)], glial cells (Müller cells, astrocytes, and microglia), and blood cells (endothelial cells [ECs] and pericytes, which cooperatively form the inner BRB [iBRB]) are linked to form a vital structure called the retinal neurovascular unit, and ECs and retinal pigment epithelial (RPE) cells constitute the outer blood-retina barrier (oBRB) (Yang et al., 2020; Meng et al., 2021; Tang et al., 2022). DR is caused by retinal neurovascular unit dysfunction, including loss of cells, inflammatory factor production, neovascularization, and BRB impairment (Wang and Lo, 2018), among which inflammatory process is one of the most important features (Öhman et al., 2018). A previous study showed that diabetes jeopardizes rat retinas mainly in the outer layers because the energy metabolism activity in the outer retina is vigorous and is accompanied by a large amount of metabolic waste and mitochondrial damage (He et al., 2021). The metabolic disturbances induced by DR are mainly related to metabolism of glycolysis, polyols, tricarboxylic acid, amino acids, the urea cycle, and lipids (Xuan et al., 2020). Several observational studies have shown that dyslipidemia, mitochondrial apoptosis, and oxidative stress may be the predominant pathological changes of DR (Miller et al., 2020; Fort et al., 2021; Lin et al., 2021; Rao et al., 2021). Vascular endothelial growth factor (VEGF), HbA1c, low-density lipoprotein cholesterol, myeloperoxidase, and advanced glycation end products (AGEs) are pathogenic factors of DR. The pathogenesis of DR remains unclear, and further research is yet to be conducted.
Current therapies for DR mainly include vitreoretinal surgery, laser photocoagulation, intraocular injections of anti-VEGF agents, corticosteroids, and eye drops. Among these therapies, pan-retinal photocoagulation surgery remains the primary treatment for PDR (Flaxel et al., 2020). Additionally, in recent years, stem cell replacement therapy has been evaluated for DR, although it has significant limitations (Mathew et al., 2021b). With regard to drug treatment, in the past decade, anti-VEGF drugs have had a dramatic effect on the clinical management of DR. However, clinical trials suggest that anti–VEGF agents for DR are not effective in all patients and can even bring about complications (Fu et al., 2018b). Thus, research on novel drugs based on other targets is vital for the prevention and treatment of DR.
Therefore, we reviewed the recent literature on the cellular pathology of DR. We also evaluated the diagnostic and prognostic biomarkers of DR in terms of blood, protein, and imaging techniques. Our review also included the synthetic molecules that have been used for clinical therapy and are under clinical and preclinical investigation. Finally, we reviewed the natural molecules that are under preclinical investigation to complement the current treatment for DR.
2 Involved cells
The retina is a highly organized tissue comprising at least 10 distinct layers (Miller et al., 2020), involving photoreceptor cells, retinal ganglion cells, bipolar cells, amacrine cells, horizontal cells, glial cells, endothelial cells, pericytes, and RPE cells (Figure 1). Diabetes causes chaos among cellular interactions and loss of almost all retinal cell populations (Szabo et al., 2017). The study of the changes of various cells in DR and the interrelationship among them can help clarify the pathological mechanism of DR and identify therapeutic targets.
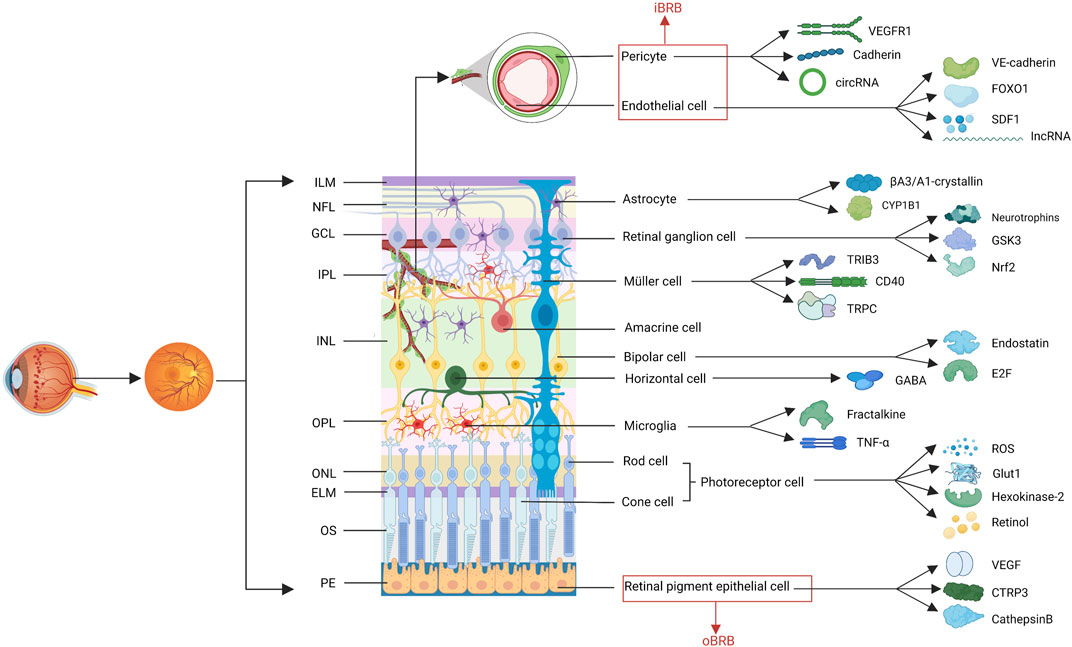
FIGURE 1. The structure of retina. The retina comprises at least 10 distinct layers, including eleven cell types involved in the progress of DR. The factors related to these cells, described in this review are shown. (ILM, internal limiting membranes; NFL, nerve fiber layer; GCL, ganglion cell layer; IPL, inner plexiform layer; INL, inner nuclear layer; OPL, outer plexiform layer; ONL, outer nuclear layer; ELM, external limiting membranes; OS, outer segments; PEL, pigment epithelium layer; iBRB, inner blood-retina barrier; oBRB, outer BRB; VEGF, vascular endothelial growth factor; VEGFR2, VEGF receptor 2; SDF1, stromal cell-derived factor 1; CYP1B1, cytochrome P450 1B1; GSK3, glucogen synthase kinase 3; Nrf2, nuclear factor erythroid 2-related factor; TRIB3, tribbles homolog 3; TRPC, transient receptor potential canonical; GABA, γ-aminobutyric acid; ROS, reactive oxygen species; Glut1, glucose transporter 1; CTRP3, C1q/TNF-related protein 3).
2.1 Photoreceptor cells
Photoreceptor cells are specialized neurons in the retina that transit light into electrical signals and rely on the cycling of 11-cisretinal. The signals are transmitted to the brain for image processing (Miller et al., 2020). Photoreceptor cells have four functional regions: the outer segment, inner segment, cell body, and synaptic terminal (Tonade and Kern, 2021). The outer segments of photoreceptor cells are the main regions of energy metabolism and glycolysis, whose long-term inhibition leads to photoreceptor degeneration (Fu et al., 2018a; Fu Z. et al., 2021; Yang T. T. et al., 2021). It is worth noting that VEGF upregulation in DR is a direct contributing factor to changes in photoreceptor function (Hu et al., 2021). The two types of photoreceptor cells are rod cells, which contain rhodopsin that facilitates vision at night, and cone cells, which contain a cone pigment and are sensitive to high light. In hyperglycemia, rhodopsin expression decreases, and rod cells are more sensitive to hyperglycemia (Yang M. et al., 2021; Song et al., 2021).
Photoreceptor cells contain more than 75% of the mitochondria of the retina (Miller et al., 2020). Dysfunction in mitochondrial oxidative phosphorylation, which generates an increase in the NADH/NAD+ redox ratio due to a decrease in NAD+ regeneration and ATP deficiency, leads to photoreceptor degeneration (Mekala et al., 2019). Additionally, the OS of DR originates from the photoreceptor cells. Mitochondria are not only producers of reactive oxygen species (ROS) but also the targets of OS. A number of ROS may accumulate and damage mitochondrial DNA, which consequently reduces the expression of regulatory proteins that eliminate ROS (Bek, 2017). Sirtuin 1, a multifunctional deacetylase that is inactivated in diabetes, protects mitochondria from the activation of mitochondria-damaging matrix metalloproteinase-9 (MMP-9) and the damage of mtDNA (Mishra et al., 2018). Furthermore, active DNA methylation plays a critical role in cytosolic ROS regulation (Duraisamy et al., 2018). This vicious cycle can be affected by epigenetic machinery in the retina (Bek, 2017; Kumari et al., 2020). Until now, current certified treatments for preventing or reversing photoceptor degeneration induced by mitochondrial dysfunction have not been found.
Hexokinase-2 (HK2), a first rate-limiting isozyme in glycolysis, plays a key role in the Warburg effect exhibited by photoreceptor cells and is required for normal rod function (Weh et al., 2020). HK2 also has non-enzymatic roles in the regulation of apoptosis by interacting with mitochondria via Akt signaling (Weh et al., 2020). Therefore, it is noteworthy that the deprivation of HK2 in rods causes inhibition of retinal glycolysis and degeneration of age-related photoreceptor cells, which leads to a progressive increase in the number and size of mitochondria in the rods (Petit et al., 2018; Zhang R. et al., 2020).
In hyperglycemia, hexokinase is saturated and photoreceptor-mediated glucose metabolism is decreased, generating the accumulation of sorbitol (a kind of polyol), which is produced from excess glucose by aldose reductase via the polyol pathway (Holoman et al., 2021). Polyol accumulation is one of the key pathological features of DR that aggravates electroretinogram defects, inflammation, and OS in the retina (Holoman et al., 2021). According to these studies, reducing polyol accumulation via glucose transporter 1 (Glut1) in photoreceptor cells can be used for DR therapy (Holoman et al., 2021). Glut1 is expressed extensively in retinal cells and is thought to be the only glucose transporter in photoreceptor cells. Retinol binding protein 3, a retinol transport protein secreted primarily by photoreceptor cells, can also combine with Glut1 to restrain Müller cells and endothelial cells from taking in glucose and inducing inflammatory cytokines (Hisashi et al., 2019). Moreover, a study implied that inhibiting Glut1 expression to restrict glucose transport will decrease retinal glucose concentrations and ameliorate DR (You et al., 2017).
Another important diabetes-associated variation in photoreceptor cells is de novo retinol lipogenesis. The main reason for this shift is the activation of fatty acid synthase by hyperglycemia (Rajagopal et al., 2021). This shift coupled with other changes in photoreceptors may make up the early pathological mechanism of angiogenesis in early DR (Rajagopal et al., 2021). Neurovascular coupling may directly be impacted by diabetes-induced lipid perturbations at photoreceptor synapses (Nippert et al., 2018). Additionally, excess retinal saturated fatty acid can be harmful to photoreceptor energy metabolism and consequently impacts vascular coupling.
Because autophagy is an essential survival mechanism in photoreceptor cells, it plays a key role in sustaining photoreceptor function by facilitating photoreceptor outer segment degradation and visual pigment recycling (Mathew et al., 2021a; Villarejo-Zori et al., 2021). Although the mechanism by which autophagy protects photoreceptors and the relationship between DR and autophagy are ambiguous, more recent studies have corroborated the importance of autophagic flux. For example, impairment of autophagy increases superoxide formation and apoptosis in 661 W cells (a cone cell line) under hyperglycemic conditions (Taki et al., 2020).
2.2 Bipolar cells
Bipolar cells can accept inputs from photoreceptors and transmit signals to retinal ganglion cells (Szabo et al., 2017; Eggers and Carreon, 2020). Endostatin, an antiangiogenic protein that exists in bipolar cells, is a naturally cleaved fragment of type XVIII collagen (Bonet et al., 2021). This protein is mainly expressed in bipolar cells and photoreceptor cells. Currently, there are few reports related to bipolar cells in DR. One study has suggested that it is highly vulnerable to high glucose levels (Wang Y. et al., 2017). In diabetic mice, the appearance of intravitreal vessels was shown to be associated with a decrease in endostatin levels in the retina (Bonet et al., 2021). In addition, high sugar levels can induce the production of reactive metabolites such as methylglyoxal (MG), which results in decreased function of bipolar cells because MG is the most reactive glycation precursor of cytotoxic end products (Schlotterer et al., 2019).
In the degenerating retina of diabetic mice, some defense mechanisms against diabetes exist in bipolar cells. Functional gene networks and pathways related to inflammation (antigen processing and presentation and/or interferon response) are commonly upregulated (Van Hove et al., 2020). Moreover, the functional gene networks and pathways related to the OS response are also commonly upregulated in bipolar cells, which is reflected in phosphorylation transcripts and an increase in transcripts correlated to ATPase-mediated proton transport (Van Hove et al., 2020). In addition, E2F transcription factors play a key role in controlling cell cycle progression, and their inactivation can rescue high glucose-induced ectopic division and cell death of bipolar cells (Wang Y. et al., 2017).
2.3 Retinal ganglion cells
RGCs, made up of 40 subtypes, are terminally differentiated neurons in the central nervous system(Thomas et al., 2017). As efferent neurons, they transfer visual information to the brain. RGCs have a limited endogenous regenerative capacity after damage; thus, apoptosis can lead to permanent vision loss. Sphingolipid rheostat, a dynamic equilibrium consisting of ceramide, sphingosine, and sphingosine-1-phosphate, has a neuroprotective effect against excitotoxic RGC death (Nakamura et al., 2021). As the most vulnerable neurons in the retina, RGCs are the most sensitive to diabetes-induced stress reactions in the early stage of nerve growth. The excitatory toxicity of glutamate increases metabolism, the caspase cascade waterfall increases apoptosis, and other mechanisms are closely related to the damage of RGCs (Fu et al., 2020). The axonal degeneration of RGCs, which disturbs axonal transport, may be the earliest event in DR pathogenesis.
Neurotrophins, including brain-derived neurotrophic factor (BDNF), nerve growth factor (NGF), and mesencephalic astrocyte-derived neurotrophic factor (MANF), are secreted growth factors that control neuronal growth, differentiation and survival and are involved in the protection against RGC injury (Gao et al., 2017; Zhang et al., 2017). BDNF can maintain synaptic plasticity and neuronal interconnections (Behl and Kotwani, 2017). However, BDNF-mediated neuroprotective actions are downregulated during hyperglycemia. In addition, the neuroprotective effect of endogenous NGF can be hindered by pro-NGF, which enhances the expression of neurotrophin receptor p75 to stimulate RGC apoptosis in DR (Elshaer and El-Remessy, 2017; Mesentier-Louro et al., 2017; Mesentier-Louro et al., 2019). Finally, MANF is a newly discovered secreted neurotrophic factor that is highly expressed in RGCs and can protect them from hypoxia-induced injury and apoptosis by preventing endoplasmic stress-mediated apoptosis in vivo and in vitro (Gao et al., 2017).
In high-fat diet -induced DR, tau hyperphosphorylation causes vision deficits and synapse loss in RGCs by destabilizing microtubule tracks, damaging microtubule-dependent synaptic targeting of cargo such as mRNA and mitochondria, and disrupting synaptic energy production in mitochondria via glucogen synthase kinase 3 (GSK3) activation (Zhu et al., 2018). In high-fat diet-induced DR mouse models, mitochondrial degeneration occurs when downregulation of β-catenin activation due to abnormal activation of GSK3β causes synaptic neurodegeneration of RGCs by suppressing ROS-scavenging enzymes, thus triggering OS-driven mitochondrial impairment (Shu XS et al., 2020).
OS is closely associated with RGC apoptosis. As a neuroprotective protein, transforming growth factor-β (TGF-β) overregulates hyperglycemia to protect RGCs from harm by activating stress response proteins and antioxidant pathways, such as aldehyde dehydrogenase 3A1, heme oxygenase-1 (HO-1), hypoxia-inducible factor (HIF) -1α, and nuclear factor erythroid 2-related factor (Nrf2) (Chen et al., 2020). Among these proteins, Nrf2 is a pivotal nuclear transcription factor that protects cells against oxidative injury. Normally, Nrf2 combines with Kelch-like erythroid-cell-derived protein with CNC homology-associated protein 1 (Keap1) as a complex to be the target of proteasomal degradation, and Nrf2 transcriptional activity is decreased in the wake of increased Keap1 levels (Radhakrishnan, 2020). Long non-coding RNA (lncRNA) is important in modulating the expression of Nrf2 in DR. The Sox2 overlapping transcript, a type of lncRNA, is a key regulator of oxidative stress in RGC damage of patients with DR (Zhang et al., 2017). Sox2 overlapping transcript knockdown can lead to the accumulation of Nrf2 protein and nuclear translocalization to combat oxidative stress by intercepting Nrf2/Keap1 contact in RGCs and activating Nrf2 and HO-1 signaling (Li et al., 2017; Liu X.-F. et al., 2018). Studies have shown that overexpression of sulfiredoxin 1 and senescence marker protein 30 may protect RGCs from high glucose (HG)-induced injury by enhancing Nrf2 expression via regulation of the Akt/glycogen synthase kinase-3β axis (Zhu F. et al., 2021; Zhang et al., 2021). Sulfiredoxin 1 is a member of the endogenous antioxidant sulfiredoxin protein family, while senescence marker protein 30 is an aging-related protein. Another important antioxidant mechanism in RGCs is the downregulation of the pleckstrin homology domain and leucine rich repeat protein phosphatase 1, which activates Nrf2/antioxidant response element -mediated transcription (Zhang X. et al., 2020). Furthermore, in high glucose treatment, Brahma-related gene 1 expression is significantly downregulated, also leading to a decline in Nrf2/HO-1 signaling (Sun et al., 2020).
2.4 Amacrine cells
Amacrine cells, made up of approximately 40 different cell types, are characterized by a wide variety of shapes, sizes, and stratification patterns, which are still under investigation. In diabetes, the immunoreactivity of AII amacrine cells appears to decrease and change, and a patchy appearance of AII amacrine cell degeneration can be observed (Szabo et al., 2017). The loss of cholinergic amacrine cells, known as starburst amacrine cells, may cause a serious decrease in the optokinetic response (Baya Mdzomba et al., 2020). In addition, dopaminergic amacrine cells degenerate in diabetic rat retinas, as revealed by transferase-mediated dUTP nick-end labeling staining (Ma et al., 2018). According to the electroretinography of oscillatory potentials, in the very early changes of the diabetic retina, the relevance of the interrelations between vascular and functional elements mainly involves the precocious involvement of amacrine cells in diabetic eyes, because amacrine cells specifically modulate the regulation in the middle retina (Midena et al., 2021a).
2.5 Horizontal cells
In horizontal cells, glucose transport into photoreceptor synapses relies on glucose transporter-2 (Yang M. et al., 2021). In a HG environment, γ-aminobutyric acid (GABA) immunoreactivity (IR) is increased in horizontal cells by decreasing GABA transporter (GAT)-1-IR and increasing GAT-3-IR to induce GABA accumulation (Carpi-Santos et al., 2017). Normally, GABA accumulation affects cytoactivity; however, in DR organotypic retinal models, HG did not affect horizontal cell viability (Yang M. et al., 2021). There are few studies on the pathological changes in horizontal cells in DR, and further tests are needed.
2.6 Glial cells
Glial cells are supporting cells of the neural retina and play an important role in the immune system of the retina (Rubsam et al., 2018). Diabetes can cause homeostatic changes in the retina that affect these glial cells; thus, glial cells promote inflammation in the retina, which is a driving force for sustaining angiogenesis in PDR (Rezzola et al., 2017). These cells can be divided into three major categories: two types of macroglia (Müller cells and astrocytes) and microglia. Microglia and astrocytes are the main sources of ROS in central nervous system chronic degenerative diseases (Mendonca et al., 2020).
2.6.1 Müller cells
Müller cells, representing 90% of retinal glia, are the main types of glial elements. They provide the structural, metabolic, and neurotrophic support necessary for the retinal layers. Endoplasmic reticulum (ER) stress in Müller cells is directly linked to retinal inflammation in DR (Yang et al., 2019). X-box binding protein 1, an effector of the unfolded protein response activated by inositol-requiring enzyme 1α, is a major transcription factor that regulates ER chaperones and ER-associated degradation (Yang et al., 2019). The activation of nucleotide-binding domain-like receptor protein-3 (NLRP3) inflammasome-mediated inflammation in Müller cells can result in BRB damage (Wei et al., 2021). Additionally, overexpression of tribbles homolog 3 (TRIB3) protein, a regulator of insulin signaling in diabetes, can reduce Müller cell viability (Pitale et al., 2021). TRIB3 is a major regulator of diabetic retinal pathophysiology and is upstream of HIF-1α, epidermal growth factor receptor, and glial fibrillary acidic protein. TRIB3 controls glucose metabolism, cytokine expression, and gliosis in retinal cells via HIF-1α-mediated Glut1 and epidermal growth factor receptor expression (Pitale et al., 2021).
Circular junctions exist between photoreceptors and Müller cells. Retinol binding protein 3, which is secreted by photoreceptors, can inhibit glucose uptake into Müller cells, leading to the decreased expression of VEGF and interleukin (IL) –6 (YokomizO et al., 2019). VEGF localizes to glial cells of the inner retina and anterior optic nerve and is expressed in the retinas and optic nerves of diabetics before retinal neovascularization (Mrugacz et al., 2021). In addition, Müller glia-derived exosomes can promote angiogenesis in DR. For example, exosome miR–9–3p promotes angiogenesis by restricting sphingosine–1-phosphate receptor (Liu et al., 2022).
Many factors can promote the inflammatory response of Müller cells, including hyperglycemia, elevated HIF–1, insulin-like growth factor 1, and CD40. HIF–1 and insulin-like growth factor 1 in the serum and vitreous body of diabetic patients activate Müller cells to form a chronic inflammatory milieu (Gaonkar et al., 2020). CD40 is an immune co-stimulatory molecule that plays a key role in Müller cells. CD40 activation can induce ATP release in Müller cells, resulting in the activation of P2X7 purinergic receptors on retinal microglia and their subsequent expression of inflammatory cytokines (Portillo et al., 2017). Furthermore, Müller cells first produce an inflammatory response in the diabetic retina andactivate signal microglia (Abcouwer, 2017).
In a study, transient receptor potential canonical (TRPC) channel, a cation channel of the transient receptor potential family, was reported to have a predominant action in Müller cells and microglia and was found to be expressed in mouse retinas in large quantities (Sachdeva et al., 2018). TRPC-mediated processes aggravating retinal neurodegeneration and vasoregression may be due to a TRPC-mediated accumulation of the reactive metabolite MG and its detoxification by Glyoxalase 1 (Sachdeva et al., 2018). However, the exact TRPC-dependent processes in DR need to be identified to understand the precise causative role of TRPCs (TRPC1-TRPC7) in DR.
2.6.2 Astrocytes
Astrocytes, which are named after their stellate shape, are located in the innermost retinal layers, and serve as a link between the retinal blood vessels and neurons. Astrocytes are also crucial for maintaining the normal function of BRB (Fresta et al., 2020b). Waves of cytosolic calcium (Ca2+) are vital for maintaining glia–astrocyte, astrocyte–astrocyte, and astrocyte–neuron communication (Shahulhameed et al., 2019). In diabetes, astrocytes are upregulated at the mRNA level and secrete various pro-inflammatory cytokines, such as IL-1β and IL-6 to amplify the inflammatory response (Rubsam et al., 2018). Hyperglycemia also significantly enhances the expression of pro-oxidants (iNOS, Nox2), nuclear translocation, activation of pNF-κB and Nrf2, and secretion of HO-1 in astrocytes (Fresta et al., 2020b).
As a lens protein expressed in astrocytes, βA3/A1-crystallin is an uncompetitive inhibitor of human protein tyrosine phosphatase 1B (PTP1B) enzyme, whereas PTP1B controls signal transducer and activator of transcription 3 phosphorylation to regulate the expression of βA3/A1-crystallin genes in astrocytes. PTP1B4 is an enzyme that links glucose metabolism and inflammation in diabetes and has a positive association with vitreous humor levels of VEGF, IL-8, and monocyte chemoattractant protein 1. Therefore, βA1-crystallin/PTP1B signaling may regulate inflammatory signaling in astrocytes under hyperglycemic stress. Moreover, βA1-crystallin is the dominant isoform of glucose metabolism in astrocytes and is essential for maintaining mitochondrial function and oxidative stress during HG stress (Ghosh et al., 2021).
Additionally, cytochrome P450 1B1 belongs to the family of heme-containing terminal oxidases, CYP450s, and engages in metabolic activation and detoxification of many compounds. The expression and activity of cytochrome P450 1B1 play an important role in resisting DR by modulating astrocytes proliferation, migration, and morphogenesis, and forming a proper fibronectin network to support retinal neurovascular function (Falero-Perez et al., 2019).
2.6.3 Microglia
Microglial cells are mononuclear phagocytes that can be regarded as tissue-resident macrophages in the retina. Microglia are also the major cell population in vitreous fibrovascular membranes. GPNMB+ (glycoprotein nonmetastatic melanoma protein B) microglia, a subpopulation of vitreous fibrovascular membranes, exhibit both profibrotic and fibrogenic properties. The profibrotic microglia and cytokines upregulated in vitreous PDR exhibit ligand-receptor interactions (Xiao et al., 2021). Additionally, the microglial population and complement system form immune defense mechanisms in the retina. During early to late changes in DR, microglia can mediate activation of the alternative complement pathway based on complement factor H and CD11b (integrin αM) gene expression to resist inflammation (Shahulhameed et al., 2020).
Microglia contact neuronal synapses and capillaries in the inner retina. There are two distinct microglial populations in the oxygen-induced retinopathy retina: activated-amoeboid phenotype microglia reside on the surface of the superficial capillary plexus of the retina, whereas resting ramified morphology microglia reside in the deep capillary plexuses (Uddin et al., 2020). Microglia can modulate vessel diameter and express vasoactive genes, likely via modulation of the local renin-angiotensin system (Mills et al., 2021). Under normal physiological conditions, retinal cells potentially restrain excessive microglial activation by secreting anti-inflammatory factors, including TGF-β2 secreted by the cones (Xiao et al., 2021). In diabetes, microglial cell morphology transitions from a resting-ramified morphology to an activated-amoeboid phenotype and inflammation-related cytokines are released (Chang et al., 2019). The activation of microglia within the PDR microenvironment involves several pathways, including interferon gamma receptor 1, chemotactic cytokine receptor 5, and CD44 signaling (Xiao et al., 2021). When cytokine-mediated inflammatory responses are highly expressed in the retina, the proliferation of microglia is increased, which shifts from an anti-inflammatory to a pro-inflammatory state (Szabo et al., 2017). In an experimental model of diabetes, activated microglial cells penetrated the basement membrane of the inner BRB (iBRB) and engulfed endothelial cells, leading to an increased number of acellular capillaries and albumin leakage, which is a significant factor in iBRB breakdown (Xie et al., 2021). Such damage to the iBRB was recently found to be preventable by inhibiting colony-stimulating factor 1-receptor (Kokona et al., 2018).
In DR, many factors are associated with the excessive activation of microglia. Fractalkine (FKN), a chemokine expressed constitutively by healthy neurons, signals microglia upon interaction with the CX3CR1 receptor. The expression of FKN declines with diabetes progression and activates microglia in the retina, resulting in an increase in IL-1β expression in microglia and astrocytes, fibrinogen deposition, and perivascular clustering of microglia (Mendiola et al., 2016; Jiang et al., 2022). Furthermore, FKN/CX3CR1 is a key signaling pathway in inducing capillary constriction, relying on microglial contact and FKN-CX3CR1-mediated up-regulation of angiotensinogen (Mills et al., 2021). MG-derived AGEs play an important role in activating microglia and accumulate in neuronal compartments of the retina during hyperglycemia (Schlotterer et al., 2019). Microglial activation is also mediated by autocrine pro-inflammatory factors, such as tumor necrosis factor-α (TNF-α) (Xiao et al., 2021). Moreover, TRIB3 mediates the increase in retinal microglia and the expression of VEGF, NF-κB, and other cytokines, thus modifying the early inflammatory response in hyperglycemia (Pitale et al., 2021).
Many inflammation-associated cytokines are expressed in microglia, including HIF-1α (Uddin et al., 2020), IL-8, TNF-α, MMP9. Pro-inflammatory markers IL-8 and MMP9 are primarily secreted from microglial cells, resulting in significant upregulation of platelet-endothelial cell adhesion molecule and VEGF-VEGF receptor 2 (VEGFR2) binding in the PDR vitreous (Shahulhameed et al., 2020). In a diabetic mouse model, the accumulation of aldose reductase accelerated VEGF protein expression and amadori-glycated albumin -induced TNF-α secretion and cell migration (Chang et al., 2019). Microglial cells are a major source of TNF-α (Xiao et al., 2021). Additionally, Nogo-A modulates phosphorylation signaling, which can increase TNF-α secretion in microglia (Baya Mdzomba et al., 2020). Nogo-A is endogenously expressed in Müller cells and RGCs. Moreover, Nogo-A is upregulated in the retina of patients with DR, and may be released into the vitreous stem from RGC lysis. Finally, hyperglycemia-induced expression of NF-κB signaling is also related to the secretion of TNF-α (Xiao et al., 2021).
2.7 Pericytes
Pericytes are one of the types of cell that form the iBRB. Pericytes envelop the microvasculature, adhere to the abluminal surface of endothelial tubules, and are the first vascular cells affected by diabetes. The key adherens junction protein between the endothelium and pericytes is N-cadherin (Monickaraj et al., 2018). Pericyte/endothelial cell interaction is affected by Ephrin-B2 which is overexpressed in diabetes (Coucha et al., 2020). Pericyte functions in the retina include promoting endothelial sprouting, expressing VEGFR1 by pericytes, spatially restricting VEGF signaling, and maintaining the integrity of the BRB (Eilken et al., 2017). Pericytes are vital to the BRB because platelet-derived growth factor (PDGF)-B/PDGF receptor beta (PDGFRb) signaling is significant in the formation and maturation of BRB via the active recruitment of pericytes to growing retinal vessels (Park et al., 2017). PDGF-B is also an indispensable factor required for pericyte survival (Monickaraj et al., 2018). In addition, pericyte maturation, changing from a stellate shape and high proliferation to quiescent and elongated states, is necessary for vessel remodeling during angiogenesis (Figueiredo et al., 2020).
Circular RNAs (circRNAs) such as cZNF532 and cPWWP2A play an important role in regulating retinal pericyte degeneration and vascular dysfunction. For example, cZNF532 is principally produced in the cytoplasm of pericytes, and its overexpression reduces the diabetic effect on microangiopathy by acting as an miR-29a-3p sponge and inducing expression of NG2, LOXL2, and CDK2. Meanwhile, cPWWP2A acts as an miR-579 sponge that can contribute to the promotion of DR progression through upregulation of the angiopoietin-1/Occluding/Sirtuin 1 proteins (Liu C. et al., 2019; Jiang et al., 2020). Moreover, hypoxia-induced circEhmt1 in the nucleus of pericytes is a kind of circRNA that can be transferred from pericytes to endotheliocytes by exosomes, playing an important role in regulating pericyte-endotheliocyte crosstalk by mediating the NFIA/NLRP3 pathway to activate HIF signaling (Ye et al., 2021).
In early diabetic models, the total number of pericytes remained static, varying by the distribution of on-vessel versus off-vessel pericytes to form the pericyte bridge. The combination of pericytes and microvasculature is a dynamic process, and pericytes clearly move off the vessel and frequently extend and retract filopodia between vessels in time-lapse imaging of lineage-marked pericytes (Corliss et al., 2020). Pericyte detachment is a key mechanism underlying bridge cell and basement membrane bridge formation and is an essential factor that accelerates DR progression, further destabilizing retinal vascular endothelial cells (Park et al., 2017; Corliss et al., 2020). AGEs can activate Rho-kinase to mediate moesin phosphorylation at Thr558, and the resulting phospho-moesin interacts with CD44 to form the CD44 cluster, which may stimulate the migration of pericytes and subsequent pericyte detachment in microvessels (Zhang S. S. et al., 2020).
With the development of diabetic retinopathy, the loss of pericytes occurs in the retina, which causes vascular leakage due to inadequate pericyte coverage, leading to the eventual destruction of the microvasculature, while BRB permeability is increased facilitating low-density lipoprotein penetration into the retina (Park et al., 2017). Various factors influence pericyte loss through different pathways. For example, hyperglycemia-induced ER stress may lead to apoptosis and pericytes loss (Lai et al., 2017). High levels of thyroid stimulating hormone may facilitate the effect of high glucose-induced pericyte loss through thyroid stimulating hormone -receptor -dependent mitochondrial apoptosis in retinal pericytes (Lin et al., 2021). DNA methyltransferase-1 can prevent the overexpression of peroxisome proliferator-activated receptor α by mediating its methylation to increase apoptotic cells and ROS in pericytes (Zhu Y. et al., 2021). Overexpression of soluble epoxide hydrolase can initiate the loss of pericytes by generating diol 19,20-dihydroxydocosapentaenoic acid (19,20-DHDP) from docosahexaenoic acid in diabetic retina (Hu et al., 2017). Mechanistically, 19,20-DHDP can induce VE-cadherin internalization and the migration of vascular pericytes into the extravascular space, decrease N-cadherin expression, and reduce the association of cholesterol with PS1-VE-cadherin and PS1-N-cadherin complexes (Hu et al., 2017). Increased levels of cathepsin D, an aspartyl protease, can alter endothelial-pericyte interactions by decreasing N-cadherin and PDGFRb, increasing the phosphorylation of the downstream signaling protein, protein kinase C-alpha, and Ca2+/calmodulin-dependent protein kinase II (Monickaraj et al., 2018). Recent studies in C57BL/6 diabetic mouse retinas have indicated that TRIB3 can affect the formation of acellular capillaries and subsequent pericyte loss (Pitale et al., 2021). Excessive autophagy also causes stress and pericyte necrosis (Mao et al., 2017).
2.8 Endothelial cells
ECs are another main cell type found in the iBRB that form a smooth internal vascular lining and control the exchange of chemical substances between the blood and the retina. Endothelial tip cells are specialized ECs, and their ability to invade and migrate into tissues can influence sprouting angiogenesis (Figueiredo et al., 2021). The invasiveness of endothelial tip cells during angiogenesis depends on the formation of specific actin-related protein 2/3 -dependent dactylopodia, which are derived from filopodia. Actin-related protein 2/3 activation can be restricted by myosin IIA by positively regulating the maturation state of focal adhesions and negatively regulating the β-PIX/Rac1 pathway (Figueiredo et al., 2021).
In hyperglycemia, the endothelial markers CD31 and VE-cadherin are decreased in ECs, and VE-cadherin disruption leads to the loss of cell-to-cell contact in EC monolayers and mediates Sema4D/PlexinB1 to induce EC dysfunction (Portillo et al., 2017; Thomas A. A. et al., 2019; Wu et al., 2020). The increased expression of the mesenchymal markers α-smooth muscle actin, smooth muscle 22, fibroblast-specific protein 1, and vimentin in ECs demonstrates that damaged ECs transform into a mesenchymal phenotype, termed endothelial-mesenchymal transition (EMT) (Thomas A. A. et al., 2019).
As pericytes are lost, tube formation by ECs increases, which is related to endothelial angiogenesis (Ye et al., 2021). Pericyte depletion can induce forkhead transcription factor FOXO1 activation in unstable ECs, possibly through reduced Tie2-mediated PI3 kinase/Akt signaling and upregulation of angiopoietin-2, which contributes to the elevation of vascular destabilizing factors including Ang2 and VEGFR2, leading to vessels susceptible to leakage upon external VEGF-A stimulus (Park et al., 2017). Notably, pericyte loss directly leads to inflammatory responses in ECs and perivascular infiltration of macrophages, whereby macrophage-derived VEGF and placenta growth factor can also activate VEGFR2 in ECs (Ogura et al., 2017). Excess adiponectin can decrease vascular barrier function by increasing adhesion molecule-1 in ECs, enhancing VEGF-VEGFR2 signaling, and inducing EC migration via AdipoR1 and AdipoR2 pathways to mediate angiogenesis (Nishinaka et al., 2021). The hyperglycemia-mediated increase of EC-derived adhesion molecule–1 is particularly responsive to the downregulation of Nox1/4/5, which is the Nox isoform present in ECs that produces excess ROS and is overexpressed to enhance EC proliferation and tubule formation in DR (Deliyanti et al., 2020). Because of the intimate connection between pericytes and ECs, factors that impact the loss of pericytes also play a role in the loss of ECs in DR, which leads to the formation of acellular capillaries such as 19,20-DHDP and TRIB3 (Lai et al., 2017). Then, 19,20-DHDP disrupts VE-cadherin continuity in cultured ECs and increases EC permeability to dextran (Hu et al., 2017). TRIB3, which is expressed in ECs of the human diabetic retina and controls the death of ECs, has been linked to aberrant angiogenesis. In C57BL/6 diabetic mouse retinas, TRIB3 upregulates adhesion molecule-1, a cell surface glycoprotein expressed in ECs (Pitale et al., 2021).
ER stress also promotes endothelial dysfunction in DR, and the predominant inducer is chemokine SDF1 (stromal cell-derived factor), also known as CXCL12 (Lai et al., 2017). The level of SDF1α, a subtype of SDF1, is positively correlated with Nε-(carboxymethyl) lysine, a major AGE, and its expression is significantly increased in patients with diabetes. Nε-(carboxymethyl) lysine can cause retinal EC dysfunction and vascular leakage through a therapeutic targeting tumor progression locus-2/activating transcription factor-4/SDF1α-signaling pathway in DR (Lai et al., 2017).
Recent DR research has focused on lncRNAs as key regulators of EC function. For example, vascular endothelial-associated lncRNA VEAL2 competitively restrains the overactivation of protein kinase C beta 2 (PRKCB2) in ECs by binding to the DAG-binding domain to rescue the effects of PRKCB2-mediated turnover of endothelial junctional proteins, thus maintaining normal endothelial permeability (Sehgal et al., 2021). The lncRNA, MALAT1 can downregulate its expression through siRNAs to prevent a glucose-induced increase in Keap1 and modulate the transcriptional activity of Nrf2 to maintain the antioxidant defense system in DR (Radhakrishnan, 2020; Radhakrishnan and Kowluru, 2020). Both H19 and SNHG7 can prevent EMT. Overexpression of H19 restrains EMT via TGF-β1 and suppresses TGF-β signaling by intercepting the MAPK–ERK1/2 pathway, whereas SNHG7 acts via the miR-34a-5p/XBP1 axis (Thomas A. A. et al., 2019; Cao et al., 2021). However, some lncRNAs accelerate the progression of DR, such asTDRG1, which promotes microvascular cell dysfunction by mediating VEGF overexpression (Gong et al., 2019).
Additionally, circRNAs, such as circHIPK3, circCOL1A2, and circFTO are potential targets for controlling PDR and play an important role in retinal vascular dysfunction. For example, circHIPK3 increases endothelial proliferation by blocking miR-30a function (Shan et al., 2017). Moreover, circCOL1A2 and circFTO can facilitate angiogenesis during the pathological progression of DR, and their respective pathways are the miR-29b/VEGF axis and the miR-128–3p/thioredoxin-interacting protein axis (Zou et al., 2020; Guo et al., 2021). Finally, the roles of miR-29b and miR-128–3p suggest that microRNA is also significant for angiogenesis in DR.
2.9 Retinal pigment epithelial cells
The monolayer RPE cells constitute the oBRB and locate between the neuroretina and choroid. The interaction between RPE cells and microglia affects the integrity of the oBRB in DR (Jo et al., 2019). In addition, the apical microvilli of RPE cells wrap around OS of adjacent photoreceptors and provide nutritional support to photoreceptors (Mekala et al., 2019; Keeling et al., 2020).
VEGF is a vital factor for promoting angiogenesis, and its secretion in RPE cells is regulated by L-type calcium channels and pituitary adenylate cyclase-activating polypeptide (Fabian et al., 2019; Vuori et al., 2019). Pituitary adenylate cyclase-activating polypeptide is a peptide with a wide range of functions that can attenuate the levels of VEGF, endothelin-1, and angiogenin in RPE cells (Fabian et al., 2019).
HG levels can promote the migration and proliferation of RPE cells, reducing the expression of epithelial markers E-cadherin and ZO-1 and increasing the levels of mesenchymal markers vimentin and α-SMA, indicating EMT (Yang J. et al., 2021). A recent study showed that knockdown of miR-195 can inhibit EMT and RPE cell permeability (Fu S. H. et al., 2021). In addition, hyperglycemia can stimulate the production of ROS, increase the level of malondialdehyde, and reduce SOD activity in RPE cells (Zhang and He, 2019). It is worth noting that C1q/TNF-related protein 3 (CTRP3) and miR-455–5p may be new therapeutic targets for oxidative stress and apoptosis in RPE cells (Chen et al., 2019; Zhang and He, 2019). The Nrf2/HO–1 pathway is a target of CTRP3, and suppressor of cytokine signaling 3 is a direct target of miR-455–5p (Chen et al., 2019; Zhang and He, 2019). Furthermore, CTRP3 mediates iBRB compatibility and resists vascular permeability induced by DR through the AMPK-dependent occludin/claudin –5 signaling pathway (Yan et al., 2022).
Lysosome membrane permeabilization is induced in RPE cells under diabetic conditions, which leads to massive amounts of cathepsin B being released from the lysosomes into the cytosol in RPE cells under HG conditions and subsequent autophagy-lysosome pathway dysfunction (Patel et al., 2018; Feng et al., 2021). The expression of lysosome membrane permeabilization can be upregulated by overexpressed high mobility group box, a nuclear DNA-binding protein with various functions, via a cathepsin B-dependent pathway (Feng et al., 2021). Additionally, lysosome membrane permeabilization may initiate mitochondrial membrane potential changes by interacting with BCL2 family members, CYCS (cytochrome c, somatic), and ROS release, which activates the classical mitochondria-caspase pathway (Feng et al., 2021).
3 Biomarkers
The latest definition of biomarker is “a defined characteristic that is measured as an indicator of normal biological processes, pathogenic processes, or responses to an exposure or intervention, including therapeutic interventions” according to the FDA-NIH Joint Leadership Council. Sensitive, accurate, and specific detection of biomarkers is important for diagnosing and measuring the severity of DR, and provides a powerful and dynamic approach to improve our understanding of the mechanisms underlying DR (Tamhane et al., 2019). The discovery of novel biomarkers for detecting DR remains vital.
3.1 Blood
Blood is the primary source of biomarkers for DR detection. Blood metabolic biomarkers include proteins, glycoproteins, polypeptides, and amino acids, of which proteins are the most prevalent. Currently, HbA1C is the only validated systemic biomarker in DR and has been used in clinical diagnosis. Most of the research on other biomarkers is still in the preclinical basic research stage, and the more studied biomarkers of DR-angiogenesis (e.g., VEGF), inflammatory factors (e.g., IL-6, IL-8, IL-1β, IL-17A, and TNF-α), oxidative stress products (e.g., lipoperoxides and malondialdehyde), antioxidants (e.g., glutathione, glutathione peroxidase, and SOD), and apoptosis factors (e.g., Cytochrome-C) have been summarized in a number of reviews. Therefore, we have listed some of the potential biomarkers of DR that have been studied in the last 5 years (Table 2). Furthermore, individual biomarkers as well as panels of biomarkers can be used in clinical practice. In fact, the sensitivity of combinatorial biomarkers is significantly higher than that of individual biomarkers. For example, a biomarker panel consisting of 12-hydroxyeicosatetraenoic acid and 2-piperidone exhibited a faster and more accurate performance than HbA1c in diagnosing DR in vitro (Xuan et al., 2020).
The usual techniques for testing include immunoassay, western blot analysis, enzyme-linked immunosorbent assay, and radical absorbance capacity assay. However, the abundance of some biomarkers is low, requiring more sensitive techniques with lower detection limits. For example, the newly developed optoelectrokinetic bead-based immunosensing can detect the low-abundance biomarker of DR, lipocalin 1 (Wang J. C. et al., 2017). Additionally, to achieve more accurate and precise plasma quantification of biomarkers for patients with DR, high-throughput tools such as the integrated plasma proteome sample preparation system should be developed (Li et al., 2021).
Vitreous humor (VH), aqueous humor (AH), and tears can also be used to detect biomarkers. Compared to other body fluids, the collection of tears is easy and noninvasive, although there is currently no entirely efficient and universal method for its collection. More importantly, biomarker levels, such as those of TNF-α and VEGF in tears are comparable to those in the blood and increase with the severity of the disease (Nandi et al., 2021). However, tears mainly provide exact information regarding disorders in the anterior segment. For posterior segment disorders, AH and VH are the most suitable matrices for evaluating relevant biomarkers but their collection is invasive and difficult, which can cause secondary damage in diseased eyes (Tamhane et al., 2019).
3.2 Nucleic acids
Through immunohistochemistry, PCR, western blot analysis, high-throughput sequencing, Gene Ontology enrichment analysis, Kyoto Encyclopedia of Genes and Genomes pathway analysis, and other techniques, nucleic acid biomarkers related to DR can be explored. Several epigenetic modifications have been studied in DR, including methylation of DNA molecules, chromatin remodeling, histone modification, and non-coding RNA (ncRNA). These alterations can be prognostic, therapeutic, or diagnostic biomarkers of DR (Table 3) (Lopez-Contreras et al., 2020). Moreover, ncRNAs, including miRNAs, lncRNAs, and circRNAs, are the most studied biomarkers of DR. NcRNAs can be secreted into the body fluid to play a role in the pathomechanism of DR, and its primary pathway is executed in exosomes. Recently, exosomal nucleic acids, in particular, exosomes and their ncRNA payloads, have attracted much attention, as they may not only serve as specific biomarkers in the diagnosis of DR but also as promising therapeutic agents for the treatment of DR (Liu et al., 2020; Liu et al., 2022). In addition, ncRNAs can interact to regulate the progression of DR. For example, SNHG4 may sponge miR-200b by upregulating oxidation resistance 1, thus suppressing RPE cell apoptosis (Yu J. et al., 2021).
3.3 Imaging techniques
Imaging techniques are mainly used for the diagnosis of microangiopathy in DR, which can detect and stage DR. Slit-lamp biomicroscopy, fundus photography, optical coherence tomography (OCT), OCT angiography (OCTA), fluorescein angiography (FA), and B-scan ultrasonography are several clinical imaging tools used for detecting morphological biomarkers of DR, such as vessel density percentage, microaneurysm, and retinal venular tortuosity (Flaxel et al., 2020; Midena et al., 2021b; Chen et al., 2021). Potential imaging biomarkers for DR are listed in Table 4. These computerized imaging techniques have made enormous contributions to precision therapy. In particular, OCT examines retinal layers in a noninvasive manner using automated retinal layer segmentation software (Micera et al., 2020).
OCT, with two different scanning methods, time-domain OCT and spectral domain OCT, has been used to observe structural changes in DR and provide high-resolution imaging of the vitreoretinal interface, neurosensory retina, and subretinal space (Ishibazawa et al., 2015; Flaxel et al., 2020). OCTA is a functional extension of OCT that allows a layered view of the vascular morphology and blood flow alterations of the retina and choroid to study retinal capillary layer lesions more thoroughly (Ishibazawa et al., 2015). Although OCTA is approved by the FDA, the guidelines and indications for its use in DR screening are currently being developed. Therefore, many studies have evaluated novel imaging biomarkers based on OCTA. For example, geometric perfusion deficits are a novel OCTA biomarker based on oxygen diffusion. Compared with the vessel density percentage, geometric perfusion deficits detection is more sensitive, making analysis easier (Chen et al., 2021). Solitary, small (<30 µm) and medium level hyperreflective retinal foci, which are similar to the retinal fiber layer, may represent aggregates of activated microglial cells and serve as a biomarker of inflammation in the retina (Vujosevic et al., 2017; Midena et al., 2021b). In addition, swept-source OCTA is an improved version of OCTA that improves visualization of the vitreous and vitreoretinal interfaces, whereas technological improvements in widefield swept-source OCTA increase the field of view from 20°C to 50°C (Akiyama et al., 2018; Cui et al., 2021)
As another important diagnostic tool of DR, FA can detect primary vascular lesions (e.g., microaneurysms) and advanced vascular abnormalities (e.g., venous beading and intraretinal microvascular abnormalities) (Ishibazawa et al., 2015). However, compared to noninvasive imaging techniques, FA requires intravenous dye injection and can cause serious discomfort and stress during detection (Vujosevic et al., 2017). Furthermore, FA images are limited to two dimensions; therefore, they cannot clearly visualize the structures of layered capillary networks (Ishibazawa et al., 2015). Ultra-wide field (UWF) FA was developed based on UWF scanning laser ophthalmoscopy, which allows for the imaging of a larger area of the retina not otherwise captured (Yu et al., 2020).
4 Treatments
Compared to vitreoretinal surgery and laser photocoagulation, drug treatment is an emerging treatment for DR and has received increased attention in recent years.
4.1 Synthetic molecules
Currently, the drugs which are used in the clinical treatment of DR are all synthesized molecules. Although they cannot reverse the damage to vision caused by hyperglycemia-induced dysfunction of the retina, they do slow down the progression of DR.
In the past 10 years, anti-VEGF drugs have been used as the leading drug treatment in patients with DR. Clinical anti-VEGF drugs include ranibizumab, bevacizumab, and aflibercept (Flaxel et al., 2020; Yu H. J. et al., 2021). Ranibizumab and bevacizumab are micromolecular antibody-based drugs with a single target, whereas aflibercept is a macromolecular recombinant fusion protein that recognizes ligands of VEGF receptors 1 and 2 (Rojo Arias et al., 2020). In a recent study, aflibercept reduced the severity of retinal microvascular aberrations and significantly improved neuroretinal function (Rojo Arias et al., 2020; Yu H. J. et al., 2021). However, anti-VEGF therapy may be unsatisfactory, with some complications. First, the most serious complication of anti-VEGF injections is infectious endophthalmitis (Fu et al., 2018b). Second, long-lasting VEGF antagonism may be detrimental to the health of neurons and vascular cells because VEGF is an important neurovasculotrophic factor (Usui-Ouchi and Friedlander, 2019). Third, it may potentially increase the development of age-related macular degeneration-associated geographic atrophy which is characterized by progressive and irreversible loss of photoreceptors, RPE cells, and choriocapillaris (Usui-Ouchi and Friedlander, 2019). In addition, retinal detachment, cataract formation, and sustained elevated intraocular pressure occasionally result from VEGF therapy.
Because lipid-lowering agents have protective effects against DR progression, intravitreal corticosteroids including triamcinolone acetonide, dexamethasone, fenofibrate, omega-3 fatty acids, and statins are another important clinical drug treatment for DR (Flaxel et al., 2020; Busik, 2021). Despite their side effects of cataract progression and elevated intraocular pressure (IOP), they can be used as second-line agents for DR. However, most of the IOP caused by dexamethasone can be successfully managed using IOP-lowering medication, and no patients would require a surgery for IOP reduction (Bucolo et al., 2018). In two large randomized placebo-controlled clinical trials, fenofibrate, which is commonly used to reduce serum triacylglycerols, slowed the rate of DR progression possibly by increasing circulating hematopoietic stem/progenitor cell levels in patients with DR (Bonora et al., 2021). Furthermore, in a preclinical study, fenofibrate attenuated OS and neuroinflammation, possibly by modulating Nrf2 expression and NLRP3 inflammasome activation (Liu Q. et al., 2018).
Calcium dobesilate is the only antioxidant that is used in the clinical treatment of DR, although it is not included in clinical guidelines by the American Academy of Ophthalmology It can improve microcirculation and exert vascular-protective effects. The clinical efficacy of calcium dobesilate for DR is achieved by alleviating the high permeability of retinal vessels, which has a beneficial effect on the permeability of the BRB (Liu J. et al., 2019).
Additionally, some drugs for DR are undergoing clinical trials, such as fasudil, which is a clinically approved Rho-associated kinase inhibitor. However, owing to its short half-life, frequent repetition of intraocular injections is required, which limits further clinical development (Lebon et al., 2021). Meanwhile, we collated information on certain drugs being investigated for the treatment of DR, including anti-angiogenic, anti-vascular leakage, anti-inflammatory, anti-oxidative damage, and neuroprotective agents (Table 5). Some of these drugs play multiple roles in the treatment of DR. For example, melatonin and SZV1287 have both anti-inflammatory and antioxidant effects (Tu et al., 2021b; Tekus et al., 2021). The pathogenesis of DR is complex, therefore, multi-targeted drugs may be more effective.
4.2 Natural molecules
It is well known that natural materials have a long history with rich clinical experience in preventing and treating diseases, and these materials include plants, animals, microbes, and minerals. Natural molecules extracted from natural materials are important sources of drugs, and plants are the most commonly used natural materials. Additionally, with the development in society, people are increasingly becoming concerned about their health, and returning back to nature has become a trendy idea. Therefore, natural molecules have a great potential value. Recently, an increasing number of experimental studies have been conducted on the mechanism of natural molecules in the prevention and treatment of DR. We have compiled certain natural molecules (in preclinical studies) used for the treatment of DR in recent years (Table 6). Moreover, marine organisms serve as an emerging source of novel bioactive compounds and can be developed for the treatment of DR. For example, fucoxanthin extracted from seaweed has a protective effect on the retina by increasing catalase and reducing OS (Chiang et al., 2020).
4.3 Administration
The administration routes for synthesized molecules in preclinical studies include injection, oral, and eye drops (see Table 5). Among the injectable routes, intravitreal injection is the most commonly used clinical method for the treatment of DR. However, compared to intravitreal injection, subcutaneous injection, intraperitoneal injection, and intravenous injection are more suitable as they are noninvasive to the eyes, although they are usually only used in animal models of DR at present. Additionally, pharmacokinetic and pharmacodynamics changes with aging also represent an important aspect that needs to be considered (Leley et al., 2021).
5 Perspective
In recent years, the definition of DR has transformed from microangiopathy to highly tissue-specific neurovascular complication, and neurodegeneration in DR has become a hot research topic. However, the pathological mechanisms of DR are complex and diverse, and several potential mechanisms require further in-depth research and investigation. The spatiotemporal correlations between the currently known pathological mechanisms have not been integrally studied. Therefore, this could be a potentially important research direction for future studies. A clear sequence of DR pathogenesis in time and space could facilitate the identification of the progress and the therapeutic targets of DR.
Understanding the pathological mechanism of DR is the basis for the development of new biomarkers of this disease. The lack of effective diagnostic biomarkers for DR can lead to unsatisfactory curative treatments, and the identification of biomarkers is crucial for uncovering the underlying mechanisms of DR and making a clear classification in clinical diagnosis. More importantly, research on potential drug targets is based on clear pathogenesis and effective biomarkers. Currently, the search for biomarkers and therapeutic interventions for DR is focused on the treatment of late phases of the disease. Therefore, biomarkers and drugs for early DR (including neuropathy and NPDR) will be the key for future research. In addition, combinatorial biomarkers are worth considering, as their sensitivity is significantly higher than that of individual biomarkers.
Currently, although there are drugs already approved for DR treatment, they are not completely effective in curing DR, and most of them only decelerate disease progress. Thus, the development of new drugs remains crucial. However, pathological mechanisms of DR are complicated, and combination drug therapy with versatile targets is perhaps more effective for DR treatment.
Author contributions
JR initiated this review, collected the references, mapped figures, and drafted the manuscript. SZ revised our first draft and provided valuable comments. YP edited tables. MJ checked the spelling and grammar. JL adjusted the format. YL, XS, and GL supported the funding and revised the manuscript. All authors approved the submitted version.
Funding
This study was supported by the National Natural Science Foundation of China (No.82174034), CAMS Innovation Fund for Medical Sciences (CIFMS) (No. 2021-I2M-1–028), CAMS Innovation Fund for Medical Sciences (CIFMS) (2021-I2M-1–031), Yunnan Wang Jinhui Expert Workstation (202105AF150057), and Yunnan Provincial Science and Technology Talent and Platform Program (202105AG070011).
Conflict of interest
The authors declare that the research was conducted in the absence of any commercial or financial relationships that could be construed as a potential conflict of interest.
Publisher’s note
All claims expressed in this article are solely those of the authors and do not necessarily represent those of their affiliated organizations, or those of the publisher, the editors and the reviewers. Any product that may be evaluated in this article, or claim that may be made by its manufacturer, is not guaranteed or endorsed by the publisher.
References
Abcouwer, S. F. (2017). Muller cell-microglia cross talk drives neuroinflammation in diabetic retinopathy. Diabetes 66 (2), 261–263. doi:10.2337/dbi16-0047
Abramoff, M. D., Fort, P. E., Han, I. C., Jayasundera, K. T., Sohn, E. H., Gardner, T. W., et al. (2018). Approach for a clinically useful comprehensive classification of vascular and neural aspects of diabetic retinal disease. Invest. Ophthalmol. Vis. Sci. 59 (1), 519–527. doi:10.1167/iovs.17-21873
Akiyama, H., Li, D., Shimoda, Y., Matsumoto, H., and Kishi, S. (2018). Observation of neovascularization of the disc associated with proliferative diabetic retinopathy using OCT angiography. Jpn. J. Ophthalmol. 62 (3), 286–291. doi:10.1007/s10384-018-0571-z
Barutta, F., Corbetta, B., Bellini, S., Guarrera, S., Matullo, G., Scandella, M., et al. (2021). MicroRNA 146a is associated with diabetic complications in type 1 diabetic patients from the EURODIAB PCS. J. Transl. Med. 19 (1), 475. doi:10.1186/s12967-021-03142-4
Baya Mdzomba, J., Joly, S., Rodriguez, L., Dirani, A., Lassiaz, P., Behar-Cohen, F., et al. (2020). Nogo-A-targeting antibody promotes visual recovery and inhibits neuroinflammation after retinal injury. Cell Death Dis. 11 (2), 101. doi:10.1038/s41419-020-2302-x
Behl, T., and Kotwani, A. (2017). Downregulated brain-derived neurotrophic factor-induced oxidative stress in the pathophysiology of diabetic retinopathy. Can. J. Diabetes 41 (2), 241–246. doi:10.1016/j.jcjd.2016.08.228
Bek, T. (2017). Mitochondrial dysfunction and diabetic retinopathy. Mitochondrion 36, 4–6. doi:10.1016/j.mito.2016.07.011
Bonet, A., Valenca, A., Mendes-Jorge, L., Casellas, A., Rodriguez-Baeza, A., Nacher, V., et al. (2021). Decreased endostatin in db/db retinas is associated with optic disc intravitreal vascularization. Exp. Eye Res. 212, 108801. doi:10.1016/j.exer.2021.108801
Bonfiglio, V., Platania, C. B. M., Lazzara, F., Conti, F., Pizzo, C., Reibaldi, M., et al. (2020). TGF-Beta serum levels in diabetic retinopathy patients and the role of anti-VEGF therapy. Int. J. Mol. Sci. 21 (24), E9558. doi:10.3390/ijms21249558
Bonora, B. M., Albiero, M., Morieri, M. L., Cappellari, R., Amendolagine, F. I., Mazzucato, M., et al. (2021). Fenofibrate increases circulating haematopoietic stem cells in people with diabetic retinopathy: A randomised, placebo-controlled trial. Diabetologia 64 (10), 2334–2344. doi:10.1007/s00125-021-05532-1
Bucolo, C., Drago, F., Maisto, R., Romano, G. L., D'Agata, V., Maugeri, G., et al. (2019). Curcumin prevents high glucose damage in retinal pigment epithelial cells through ERK1/2-mediated activation of the Nrf2/HO-1 pathway. J. Cell. Physiol. 234 (10), 17295–17304. doi:10.1002/jcp.28347
Bucolo, C., Gozzo, L., Longo, L., Mansueto, S., Vitale, D. C., Drago, F., et al. (2018). Long-term efficacy and safety profile of multiple injections of intravitreal dexamethasone implant to manage diabetic macular edema: A systematic review of real-world studies. J. Pharmacol. Sci. 138 (4), 219–232. doi:10.1016/j.jphs.2018.11.001
Busik, J. V. (2021). Lipid metabolism dysregulation in diabetic retinopathy. J. Lipid Res. 62, 100017. doi:10.1194/jlr.TR120000981
Cao, X., Xue, L. D., Di, Y., Li, T., Tian, Y. J., Song, Y., et al. (2021). MSC-derived exosomal lncRNA SNHG7 suppresses endothelial-mesenchymal transition and tube formation in diabetic retinopathy via miR-34a-5p/XBP1 axis. Life Sci. 272, 119232. doi:10.1016/j.lfs.2021.119232
Carpi-Santos, R., Maggesissi, R. S., von Seehausen, M. P., and Calaza, K. C. (2017). Retinal exposure to high glucose condition modifies the GABAergic system: Regulation by nitric oxide. Exp. Eye Res. 162, 116–125. doi:10.1016/j.exer.2017.07.010
Chen, P., Miao, Y., Yan, P., Wang, X. J., Jiang, C., Lei, Y., et al. (2019). MiR-455-5p ameliorates HG-induced apoptosis, oxidative stress and inflammatory via targeting SOCS3 in retinal pigment epithelial cells. J. Cell. Physiol. 234 (12), 21915–21924. doi:10.1002/jcp.28755
Chai, G. R., Liu, S., Yang, H. W., and Chen, X. L. (2021). Quercetin protects against diabetic retinopathy in rats by inducing heme oxygenase-1 expression. Neural Regen. Res. 16 (7), 1344–1350. doi:10.4103/1673-5374.301027
Chang, K. C., Shieh, B., and Petrash, J. M. (2019). Role of aldose reductase in diabetes-induced retinal microglia activation. Chem. Biol. Interact. 302, 46–52. doi:10.1016/j.cbi.2019.01.020
Chen, H. Y., Ho, Y. J., Chou, H. C., Liao, E. C., Tsai, Y. T., Wei, Y. S., et al. (2020). The role of transforming growth factor-beta in retinal ganglion cells with hyperglycemia and oxidative stress. Int. J. Mol. Sci. 21 (18), E6482. doi:10.3390/ijms21186482
Chen, S., Moult, E. M., Zangwill, L. M., Weinreb, R. N., and Fujimoto, J. G. (2021). Geometric perfusion deficits: A novel OCT angiography biomarker for diabetic retinopathy based on oxygen diffusion. Am. J. Ophthalmol. 222, 256–270. doi:10.1016/j.ajo.2020.09.007
Cheung, C. Y. Y., Lee, C. H., Tang, C. S., Xu, A., Au, K. W., Fong, C. H. Y., et al. (2019). Genetic regulation of pigment epithelium-derived factor (PEDF): An exome-chip association analysis in Chinese subjects with type 2 diabetes. Diabetes 68 (1), 198–206. doi:10.2337/db18-0500
Chiang, Y. F., Chen, H. Y., Chang, Y. J., Shih, Y. H., Shieh, T. M., Wang, K. L., et al. (2020). Protective effects of fucoxanthin on high glucose- and 4-hydroxynonenal (4-hne)-induced injury in human retinal pigment epithelial cells. Antioxidants 9 (12), E1176. doi:10.3390/antiox9121176
Choi, E. Y., Park, Y. W., Lee, M., Kim, M., Lee, C. S., Ahn, S. S., et al. (2021). Magnetic resonance imaging-visible perivascular spaces in the basal ganglia are associated with the diabetic retinopathy stage and cognitive decline in patients with type 2 diabetes. Front. Aging Neurosci. 13, 666495. doi:10.3389/fnagi.2021.666495
Conti, F., Lazzara, F., Romano, G. L., Platania, C. B. M., Drago, F., Bucolo, C., et al. (2021). Caffeine protects against retinal inflammation. Front. Pharmacol. 12, 824885. doi:10.3389/fphar.2021.824885
Corliss, B. A., Ray, H. C., Doty, R. W., Mathews, C., Sheybani, N., Fitzgerald, K., et al. (2020). Pericyte bridges in homeostasis and hyperglycemia. Diabetes 69 (7), 1503–1517. doi:10.2337/db19-0471
Coucha, M., Barrett, A. C., Bailey, J., Abdelghani, M., and Abdelsaid, M. (2020). Increased Ephrin-B2 expression in pericytes contributes to retinal vascular death in rodents. Vasc. Pharmacol. 131, 106761. doi:10.1016/j.vph.2020.106761
Crespo-Garcia, S., Tsuruda, P. R., Dejda, A., Ryan, R. D., Fournier, F., Chaney, S. Y., et al. (2021). Pathological angiogenesis in retinopathy engages cellular senescence and is amenable to therapeutic elimination via BCL-xL inhibition. Cell Metab. 33 (4), 818–832.e7. e817. doi:10.1016/j.cmet.2021.01.011
Cui, Y., Zhu, Y., Lu, E. S., Le, R., Lains, I., Katz, R., et al. (2021). Widefield swept-source OCT angiography metrics associated with the development of diabetic vitreous hemorrhage: A prospective study. Ophthalmology 128 (9), 1312–1324. doi:10.1016/j.ophtha.2021.02.020
Dantas da Costa, E. S. M. E., Polina, E. R., Crispim, D., Sbruzzi, R. C., Lavinsky, D., Mallmann, F., et al. (2019). Plasma levels of miR-29b and miR-200b in type 2 diabetic retinopathy. J. Cell. Mol. Med. 23 (2), 1280–1287. doi:10.1111/jcmm.14030
Deliyanti, D., Alrashdi, S. F., Touyz, R. M., Kennedy, C. R., Jha, J. C., Cooper, M. E., et al. (2020). Nox (NADPH oxidase) 1, Nox4, and Nox5 promote vascular permeability and neovascularization in retinopathy. Hypertension 75 (4), 1091–1101. doi:10.1161/HYPERTENSIONAHA.119.14100
Du, W., An, Y., He, X., Zhang, D., and He, W. (2018). Protection of kaempferol on oxidative stress-induced retinal pigment epithelial cell damage. Oxid. Med. Cell. Longev. 2018, 1610751. doi:10.1155/2018/1610751
Duraisamy, A. J., Mishra, M., Kowluru, A., and Kowluru, R. A. (2018). Epigenetics and regulation of oxidative stress in diabetic retinopathy. Invest. Ophthalmol. Vis. Sci. 59 (12), 4831–4840. doi:10.1167/iovs.18-24548
Eggers, E. D., and Carreon, T. A. (2020). The effects of early diabetes on inner retinal neurons. Vis. Neurosci. 37, E006. doi:10.1017/S095252382000005X
Eilken, H. M., Dieguez-Hurtado, R., Schmidt, I., Nakayama, M., Jeong, H. W., Arf, H., et al. (2017). Pericytes regulate VEGF-induced endothelial sprouting through VEGFR1. Nat. Commun. 8 (1), 1574. doi:10.1038/s41467-017-01738-3
Eissa, L. D., Ghobashy, W. A., and El-Azab, M. F. (2021). Inhibition of thioredoxin-interacting protein and inflammasome assembly using verapamil mitigates diabetic retinopathy and pancreatic injury. Eur. J. Pharmacol. 901, 174061. doi:10.1016/j.ejphar.2021.174061
Elshaer, S. L., and El-Remessy, A. B. (2017). Implication of the neurotrophin receptor p75NTR in vascular diseases: Beyond the eye. Expert Rev. Ophthalmol. 12 (2), 149–158. doi:10.1080/17469899.2017.1269602
Fabian, E., Reglodi, D., Horvath, G., Opper, B., Toth, G., Fazakas, C., et al. (2019). Pituitary adenylate cyclase activating polypeptide acts against neovascularization in retinal pigment epithelial cells. Ann. N. Y. Acad. Sci. 1455 (1), 160–172. doi:10.1111/nyas.14189
Falero-Perez, J., Sorenson, C. M., and Sheibani, N. (2019). Cyp1b1-deficient retinal astrocytes are more proliferative and migratory and are protected from oxidative stress and inflammation. Am. J. Physiol. Cell Physiol. 316 (6), C767–C781. doi:10.1152/ajpcell.00021.2019
Fang, M., Wan, W., Li, Q., Wan, W., Long, Y., Liu, H., et al. (2021). Asiatic acid attenuates diabetic retinopathy through TLR4/MyD88/NF-κB p65 mediated modulation of microglia polarization. Life Sci. 277, 119567. doi:10.1016/j.lfs.2021.119567
Feng, L., Liang, L., Zhang, S., Yang, J., Yue, Y., Zhang, X., et al. (2021). HMGB1 downregulation in retinal pigment epithelial cells protects against diabetic retinopathy through the autophagy-lysosome pathway. Autophagy 18 (2), 320–339. doi:10.1080/15548627.2021.1926655
Figueiredo, A. M., Barbacena, P., Russo, A., Vaccaro, S., Ramalho, D., Pena, A., et al. (2021). Endothelial cell invasion is controlled by dactylopodia. Proc. Natl. Acad. Sci. U. S. A. 118 (18), e2023829118. doi:10.1073/pnas.2023829118
Figueiredo, A. M., Villacampa, P., Dieguez-Hurtado, R., Jose Lozano, J., Kobialka, P., Cortazar, A. R., et al. (2020). Phosphoinositide 3-kinase-regulated pericyte maturation governs vascular remodeling. Circulation 142 (7), 688–704. doi:10.1161/CIRCULATIONAHA.119.042354
Flaxel, C. J., Adelman, R. A., Bailey, S. T., Fawzi, A., Lim, J. I., Vemulakonda, G. A., et al. (2020). Diabetic retinopathy preferred practice Pattern®. Ophthalmology 127 (1), P66–P145. doi:10.1016/j.ophtha.2019.09.025
Forster, R. B., Garcia, E. S., Sluiman, A. J., Grecian, S. M., McLachlan, S., MacGillivray, T. J., et al. (2021). Retinal venular tortuosity and fractal dimension predict incident retinopathy in adults with type 2 diabetes: The edinburgh type 2 diabetes study. Diabetologia 64 (5), 1103–1112. doi:10.1007/s00125-021-05388-5
Fort, P. E., Rajendiran, T. M., Soni, T., Byun, J., Shan, Y., Looker, H. C., et al. (2021). Diminished retinal complex lipid synthesis and impaired fatty acid beta-oxidation associated with human diabetic retinopathy. JCI Insight 6 (19), e152109. doi:10.1172/jci.insight.152109
Fresta, C. G., Caruso, G., Fidilio, A., Platania, C. B. M., Musso, N., Caraci, F., et al. (2020a). Dihydrotanshinone, a natural diterpenoid, preserves blood-retinal barrier integrity via P2X7 receptor. Int. J. Mol. Sci. 21 (23), 9305. doi:10.3390/ijms21239305
Fresta, C. G., Fidilio, A., Caruso, G., Caraci, F., Giblin, F. J., Leggio, G. M., et al. (2020b). A new human blood-retinal barrier model based on endothelial cells, pericytes, and astrocytes. Int. J. Mol. Sci. 21 (5), 1636. doi:10.3390/ijms21051636
Fu, S. H., Lai, M. C., Zheng, Y. Y., Sun, Y. W., Qiu, J. J., Gui, F., et al. (2021a). MiR-195 inhibits the ubiquitination and degradation of YY1 by Smurf2, and induces EMT and cell permeability of retinal pigment epithelial cells. Cell Death Dis. 12 (7), 708. doi:10.1038/s41419-021-03956-6
Fu, Y., Wang, Y., Gao, X., Li, H., and Yuan, Y. (2020). Dynamic expression of HDAC3 in db/db mouse RGCs and its relationship with apoptosis and autophagy. J. Diabetes Res. 2020, 6086780. doi:10.1155/2020/6086780
Fu, Z., Kern, T. S., Hellstrom, A., and Smith, L. E. H. (2021b). Fatty acid oxidation and photoreceptor metabolic needs. J. Lipid Res. 62, 100035. doi:10.1194/jlr.TR120000618
Fu, Z., Lofqvist, C. A., Liegl, R., Wang, Z., Sun, Y., Gong, Y., et al. (2018a). Photoreceptor glucose metabolism determines normal retinal vascular growth. EMBO Mol. Med. 10 (1), 76–90. doi:10.15252/emmm.201707966
Fu, Z., Wang, Z., Liu, C. H., Gong, Y., Cakir, B., Liegl, R., et al. (2018b). Fibroblast growth factor 21 protects photoreceptor function in type 1 diabetic mice. Diabetes 67 (5), 974–985. doi:10.2337/db17-0830
Gao, F. J., Wu, J. H., Li, T. T., Du, S. S., and Wu, Q. (2017). Identification of mesencephalic astrocyte-derived neurotrophic factor as a novel neuroprotective factor for retinal ganglion cells. Front. Mol. Neurosci. 10, 76. doi:10.3389/fnmol.2017.00076
Gao, X., Du, Y., Lau, W. B., Li, Y., Zhu, S., Ma, X. L., et al. (2021). Atg16L1 as a novel biomarker and autophagy gene for diabetic retinopathy. J. Diabetes Res. 2021, 5398645. doi:10.1155/2021/5398645
Gaonkar, B., Prabhu, K., Rao, P., Kamat, A., Rao Addoor, K., Varma, M., et al. (2020). Plasma angiogenesis and oxidative stress markers in patients with diabetic retinopathy. Biomarkers 25 (5), 397–401. doi:10.1080/1354750X.2020.1774654
Ghosh, S., Liu, H., Yazdankhah, M., Stepicheva, N., Shang, P., Vaidya, T., et al. (2021). βA1-crystallin regulates glucose metabolism and mitochondrial function in mouse retinal astrocytes by modulating PTP1B activity. Commun. Biol. 4 (1), 248. doi:10.1038/s42003-021-01763-5
Giordo, R., Nasrallah, G. K., Posadino, A. M., Galimi, F., Capobianco, G., Eid, A. H., et al. (2021). Resveratrol-elicited PKC inhibition counteracts NOX-mediated endothelial to mesenchymal transition in human retinal endothelial cells exposed to high glucose. Antioxidants 10 (2), 224. doi:10.3390/antiox10020224
Gong, Q., Dong, W., Fan, Y., Chen, F., Bian, X., Xu, X., et al. (2019). LncRNA TDRG1-mediated overexpression of VEGF aggravated retinal microvascular endothelial cell dysfunction in diabetic retinopathy. Front. Pharmacol. 10, 1703. doi:10.3389/fphar.2019.01703
Gong, X., Draper, C. S., Allison, G. S., Marisiddaiah, R., and Rubin, L. P. (2017). Effects of the macular carotenoid lutein in human retinal pigment epithelial cells. Antioxidants 6 (4), 100. doi:10.3390/antiox6040100
Gonzalez-Correa, J. A., Rodriguez-Perez, M. D., Marquez-Estrada, L., Lopez-Villodres, J. A., Reyes, J. J., Rodriguez-Gutierrez, G., et al. (2018). Neuroprotective effect of hydroxytyrosol in experimental diabetic retinopathy: Relationship with cardiovascular biomarkers. J. Agric. Food Chem. 66 (3), 637–644. doi:10.1021/acs.jafc.7b05063
Greco, M., Chiefari, E., Accattato, F., Corigliano, D. M., Arcidiacono, B., Mirabelli, M., et al. (2020). MicroRNA-1281 as a novel circulating biomarker in patients with diabetic retinopathy. Front. Endocrinol. 11, 528. doi:10.3389/fendo.2020.00528
Guo, J., Xiao, F., Ren, W., Zhu, Y., Du, Q., Li, Q., et al. (2021). Circular ribonucleic acid circFTO promotes angiogenesis and impairs blood-retinal barrier via targeting the miR-128-3p/thioredoxin interacting protein Axis in diabetic retinopathy. Front. Mol. Biosci. 8, 685466. doi:10.3389/fmolb.2021.685466
Hainsworth, D. P., Gangula, A., Ghoshdastidar, S., Kannan, R., and Upendran, A. (2020). Diabetic retinopathy screening using a gold nanoparticle-based paper strip assay for the at-home detection of the urinary biomarker 8-hydroxy-2'-deoxyguanosine. Am. J. Ophthalmol. 213, 306–319. doi:10.1016/j.ajo.2020.01.032
He, M., Long, P., Chen, T., Li, K., Wei, D., Zhang, Y., et al. (2021). ALDH2/SIRT1 contributes to type 1 and type 2 diabetes-induced retinopathy through depressing oxidative stress. Oxid. Med. Cell. Longev. 2021, 1641717. doi:10.1155/2021/1641717
Y., Hisashi, M., Y., Park, K., Clermont, A. C., Hernandez, S. L., Fickweiler, W., et al. (2019). Retinol binding protein 3 is increased in the retina of patients with diabetes resistant to diabetic retinopathy. Sci. Transl. Med. 11 (499), eaau6627. doi:10.1126/scitranslmed.aau6627
Holoman, N. C., Aiello, J. J., Trobenter, T. D., Tarchick, M. J., Kozlowski, M. R., Makowski, E. R., et al. (2021). Reduction of Glut1 in the neural retina but not the RPE alleviates polyol accumulation and normalizes early characteristics of diabetic retinopathy. J. Neurosci. 41 (14), 3275–3299. doi:10.1523/jneurosci.2010-20.2021
Hu, J., Dziumbla, S., Lin, J., Bibli, S. I., Zukunft, S., de Mos, J., et al. (2017). Inhibition of soluble epoxide hydrolase prevents diabetic retinopathy. Nature 552 (7684), 248–252. doi:10.1038/nature25013
Hu, J., Zhu, M., Li, D., Wu, Q., and Le, Y.-Z. (2021). VEGF as a direct functional regulator of photoreceptors and contributing factor to diabetes-induced alteration of photoreceptor function. Biomolecules 11 (7), 988. doi:10.3390/biom11070988
Huang, J., Yi, Q., You, Y., Chen, Y., Niu, T., Li, Y., et al. (2021). Curcumin suppresses oxidative stress via regulation of ROS/NF-κB signaling pathway to protect retinal vascular endothelial cell in diabetic retinopathy. Mol. Cell. Toxicol. 17 (3), 367–376. doi:10.1007/s13273-021-00144-7
Iban-Arias, R., Lisa, S., Mastrodimou, N., Kokona, D., Koulakis, E., Iordanidou, P., et al. (2018). The synthetic microneurotrophin BNN27 affects retinal function in rats with streptozotocin-induced diabetes. Diabetes 67 (2), 321–333. doi:10.2337/db17-0391
Inanc, M., Tekin, K., Kiziltoprak, H., Ozalkak, S., Doguizi, S., Aycan, Z., et al. (2019). Changes in retinal microcirculation precede the clinical onset of diabetic retinopathy in children with type 1 diabetes mellitus. Am. J. Ophthalmol. 207, 37–44. doi:10.1016/j.ajo.2019.04.011
Ishibazawa, A., Nagaoka, T., Takahashi, A., Omae, T., Tani, T., Sogawa, K., et al. (2015). Optical coherence tomography angiography in diabetic retinopathy: A prospective pilot study. Am. J. Ophthalmol. 160 (1), 35–44. doi:10.1016/j.ajo.2015.04.021
Jiang, M., Xie, H., Zhang, C., Wang, T., Tian, H., Lu, L., et al. (2022). Enhancing fractalkine/CX3CR1 signalling pathway can reduce neuroinflammation by attenuating microglia activation in experimental diabetic retinopathy. J. Cell. Mol. Med. 26 (4), 1229–1244. doi:10.1111/jcmm.17179
Jiang, Q., Liu, C., Li, C. P., Xu, S. S., Yao, M. D., Ge, H. M., et al. (2020). Circular RNA-ZNF532 regulates diabetes-induced retinal pericyte degeneration and vascular dysfunction. J. Clin. Invest. 130 (7), 3833–3847. doi:10.1172/JCI123353
Jo, D. H., Yun, J.-H., Cho, C. S., Kim, J. H., Kim, J. H., Cho, C.-H., et al. (2019). Interaction between microglia and retinal pigment epithelial cells determines the integrity of outer blood-retinal barrier in diabetic retinopathy. Glia 67 (2), 321–331. doi:10.1002/glia.23542
Keeling, E., Chatelet, D. S., Tan, N. Y. T., Khan, F., Richards, R., Thisainathan, T., et al. (2020). 3D-Reconstructed retinal pigment epithelial cells provide insights into the anatomy of the outer retina. Int. J. Mol. Sci. 21 (21), 8408. doi:10.3390/ijms21218408
Kokona, D., Ebneter, A., Escher, P., and Zinkernagel, M. S. (2018). Colony-stimulating factor 1 receptor inhibition prevents disruption of the blood-retina barrier during chronic inflammation. J. Neuroinflammation 15 (1), 340. doi:10.1186/s12974-018-1373-4
Kolibabka, M., Dietrich, N., Klein, T., and Hammes, H. P. (2018). Anti-angiogenic effects of the DPP-4 inhibitor linagliptin via inhibition of VEGFR signalling in the mouse model of oxygen-induced retinopathy. Diabetologia 61 (11), 2412–2421. doi:10.1007/s00125-018-4701-4
Kumari, N., Karmakar, A., and Ganesan, S. K. (2020). Targeting epigenetic modifications as a potential therapeutic option for diabetic retinopathy. J. Cell. Physiol. 235 (3), 1933–1947. doi:10.1002/jcp.29180
Lai, D. W., Lin, K. H., Sheu, W. H., Lee, M. R., Chen, C. Y., Lee, W. J., et al. (2017). TPL2 (therapeutic targeting tumor progression locus-2)/ATF4 (activating transcription factor-4)/sdf1α (chemokine stromal cell-derived factor-α) Axis suppresses diabetic retinopathy. Circ. Res. 121 (6), e37–e52. doi:10.1161/CIRCRESAHA.117.311066
Lebon, C., Neubauer, H., Berdugo, M., Delaunay, K., Markert, E., Becker, K., et al. (2021). Evaluation of an intravitreal rho-associated kinase inhibitor depot formulation in a rat model of diabetic retinopathy. Pharmaceutics 13 (8), 1105. doi:10.3390/pharmaceutics13081105
Leley, S. P., Ciulla, T. A., and Bhatwadekar, A. D. (2021). Diabetic retinopathy in the aging population: A perspective of pathogenesis and treatment. Clin. Interv. Aging 16, 1367–1378. doi:10.2147/CIA.S297494
Li, C. P., Wang, S. H., Wang, W. Q., Song, S. G., and Liu, X. M. (2017). Long noncoding RNA-Sox2OT knockdown alleviates diabetes mellitus-induced retinal ganglion cell (RGC) injury. Cell. Mol. Neurobiol. 37 (2), 361–369. doi:10.1007/s10571-016-0380-1
Li, T., Xu, Y., Shi, Y., Chen, J., Lin, S., Zhu, J., et al. (2020a). Genome-wide analysis of DNA methylation identifies S100A13 as an epigenetic biomarker in individuals with chronic (≥ 30 years) type 2 diabetes without diabetic retinopathy. Clin. Epigenetics 12 (1), 77. doi:10.1186/s13148-020-00871-z
Li, Y., Alhendi, A. M. N., Yeh, M.-C., Elahy, M., Santiago, F. S., Deshpande, N. P., et al. (2020b). Thermostable small-molecule inhibitor of angiogenesis and vascular permeability that suppresses a pERK-FosB/ΔFosB–VCAM-1 axis. Sci. Adv. 6 (31), eaaz7815. doi:10.1126/sciadv.aaz7815
Li, Y., Yuan, H., Dai, Z., Zhang, W., Zhang, X., Zhao, B., et al. (2021). Integrated proteomic sample preparation with combination of on-line high-abundance protein depletion, denaturation, reduction, desalting and digestion to achieve high throughput plasma proteome quantification. Anal. Chim. Acta 1154, 338343. doi:10.1016/j.aca.2021.338343
Lin, D., Qin, R., and Guo, L. (2021). Thyroid stimulating hormone aggravates diabetic retinopathy through the mitochondrial apoptotic pathway. J. Cell. Physiol. 237 (1), 868–880. doi:10.1002/jcp.30563
Liu, C., Ge, H. M., Liu, B. H., Dong, R., Shan, K., Chen, X., et al. (2019a). Targeting pericyte-endothelial cell crosstalk by circular RNA-cPWWP2A inhibition aggravates diabetes-induced microvascular dysfunction. Proc. Natl. Acad. Sci. U. S. A. 116 (15), 7455–7464. doi:10.1073/pnas.1814874116
Liu, J., Jiang, F., Jiang, Y., Wang, Y., Li, Z., Shi, X., et al. (2020). Roles of exosomes in ocular diseases. Int. J. Nanomedicine 15, 10519–10538. doi:10.2147/IJN.S277190
Liu, J., Li, S., and Sun, D. (2019b). Calcium dobesilate and micro-vascular diseases. Life Sci. 221, 348–353. doi:10.1016/j.lfs.2019.02.023
Liu, Q., Zhang, F., Zhang, X., Cheng, R., Ma, J. X., Yi, J., et al. (2018a). Fenofibrate ameliorates diabetic retinopathy by modulating Nrf2 signaling and NLRP3 inflammasome activation. Mol. Cell. Biochem. 445 (1-2), 105–115. doi:10.1007/s11010-017-3256-x
Liu, X.-F., Zhou, D.-D., Xie, T., Hao, J.-L., Malik, T. H., Lu, C.-B., et al. (2018b). The Nrf2 signaling in retinal ganglion cells under oxidative stress in ocular neurodegenerative diseases. Int. J. Biol. Sci. 14 (9), 1090–1098. doi:10.7150/ijbs.25996
Liu, Y., Yang, Q., Fu, H., Wang, J., Yuan, S., Li, X., et al. (2022). Muller glia-derived exosomal miR-9-3p promotes angiogenesis by restricting sphingosine-1-phosphate receptor S1P1 in diabetic retinopathy. Mol. Ther. Nucleic Acids 27, 491–504. doi:10.1016/j.omtn.2021.12.019
Lopez-Contreras, A. K., Martinez-Ruiz, M. G., Olvera-Montano, C., Robles-Rivera, R. R., Arevalo-Simental, D. E., Castellanos-Gonzalez, J. A., et al. (2020). Importance of the use of oxidative stress biomarkers and inflammatory profile in aqueous and vitreous humor in diabetic retinopathy. Antioxidants 9 (9), 891. doi:10.3390/antiox9090891
Luo, Y., Dong, X., Lu, S., Gao, Y., Sun, G., Sun, X., et al. (2021). Gypenoside XVII alleviates early diabetic retinopathy by regulating Muller cell apoptosis and autophagy in db/db mice. Eur. J. Pharmacol. 895, 173893. doi:10.1016/j.ejphar.2021.173893
Lv, J., Bao, S., Liu, T., Wei, L., Wang, D., Ye, W., et al. (2020). Sulforaphane delays diabetes-induced retinal photoreceptor cell degeneration. Cell Tissue Res. 382 (3), 477–486. doi:10.1007/s00441-020-03267-w
Ma, M., Xu, Y., Xiong, S., Zhang, J., Gu, Q., Ke, B., et al. (2018). Involvement of ciliary neurotrophic factor in early diabetic retinal neuropathy in streptozotocin-induced diabetic rats. Eye 32 (9), 1463–1471. doi:10.1038/s41433-018-0110-7
Malaguarnera, G., Gagliano, C., Giordano, M., Salomone, S., Vacante, M., Bucolo, C., et al. (2014). Homocysteine serum levels in diabetic patients with non proliferative, proliferative and without retinopathy. Biomed. Res. Int. 2014, 191497. doi:10.1155/2014/191497
Mao, X. B., You, Z. P., Wu, C., and Huang, J. (2017). Potential suppression of the high glucose and insulin-induced retinal neovascularization by Sirtuin 3 in the human retinal endothelial cells. Biochem. Biophys. Res. Commun. 482 (2), 341–345. doi:10.1016/j.bbrc.2016.11.065
Mat Nor, M. N., Rupenthal, I. D., Green, C. R., and Acosta, M. L. (2020). Connexin hemichannel block using orally delivered tonabersat improves outcomes in animal models of retinal disease. Neurotherapeutics 17 (1), 371–387. doi:10.1007/s13311-019-00786-5
Mathew, B., Chennakesavalu, M., Sharma, M., Torres, L. A., Stelman, C. R., Tran, S., et al. (2021a). Autophagy and post-ischemic conditioning in retinal ischemia. Autophagy 17 (6), 1479–1499. doi:10.1080/15548627.2020.1767371
Mathew, B., Torres, L. A., Gamboa Acha, L., Tran, S., Liu, A., Patel, R., et al. (2021b). Uptake and distribution of administered bone marrow mesenchymal stem cell extracellular vesicles in retina. Cells 10 (4), 730. doi:10.3390/cells10040730
Mei, X., Zhou, L., Zhang, T., Lu, B., Sheng, Y., Ji, L., et al. (2018). Chlorogenic acid attenuates diabetic retinopathy by reducing VEGF expression and inhibiting VEGF-mediated retinal neoangiogenesis. Vasc. Pharmacol. 101, 29–37. doi:10.1016/j.vph.2017.11.002
Mekala, N. K., Kurdys, J., Depuydt, M. M., Vazquez, E. J., and Rosca, M. G. (2019). Apoptosis inducing factor deficiency causes retinal photoreceptor degeneration. The protective role of the redox compound methylene blue. Redox Biol. 20, 107–117. doi:10.1016/j.redox.2018.09.023
Mendiola, A. S., Garza, R., Cardona, S. M., Mythen, S. A., Lira, S. A., Akassoglou, K., et al. (2016). Fractalkine signaling attenuates perivascular clustering of microglia and fibrinogen leakage during systemic inflammation in mouse models of diabetic retinopathy. Front. Cell. Neurosci. 10, 303. doi:10.3389/fncel.2016.00303
Mendonca, H. R., Carpi-Santos, R., da Costa Calaza, K., and Blanco Martinez, A. M. (2020). Neuroinflammation and oxidative stress act in concert to promote neurodegeneration in the diabetic retina and optic nerve: galectin-3 participation. Neural Regen. Res. 15 (4), 625–635. doi:10.4103/1673-5374.266910
Meng, C., Gu, C., He, S., Su, T., Lhamo, T., Draga, D., et al. (2021). Pyroptosis in the retinal neurovascular unit: New insights into diabetic retinopathy. Front. Immunol. 12, 763092. doi:10.3389/fimmu.2021.763092
Mesentier-Louro, L. A., De Nicolo, S., Rosso, P., De Vitis, L. A., Castoldi, V., Leocani, L., et al. (2017). Time-dependent nerve growth factor signaling changes in the rat retina during optic nerve crush-induced degeneration of retinal ganglion cells. Int. J. Mol. Sci. 18 (1), E98. doi:10.3390/ijms18010098
Mesentier-Louro, L. A., Rosso, P., Carito, V., Mendez-Otero, R., Santiago, M. F., Rama, P., et al. (2019). Nerve growth factor role on retinal ganglion cell survival and axon regrowth: Effects of ocular administration in experimental model of optic nerve injury. Mol. Neurobiol. 56 (2), 1056–1069. doi:10.1007/s12035-018-1154-1
Micera, A., Balzamino, B. O., Di Zazzo, A., Dinice, L., Bonini, S., Coassin, M., et al. (2020). Biomarkers of neurodegeneration and precision therapy in retinal disease. Front. Pharmacol. 11, 601647. doi:10.3389/fphar.2020.601647
Midena, E., Torresin, T., Longhin, E., Midena, G., Pilotto, E., Frizziero, L., et al. (2021a). Early microvascular and oscillatory potentials changes in human diabetic retina: Amacrine cells and the intraretinal neurovascular crosstalk. J. Clin. Med. 10 (18), 4035. doi:10.3390/jcm10184035
Midena, E., Torresin, T., Velotta, E., Pilotto, E., Parrozzani, R., Frizziero, L., et al. (2021b). OCT hyperreflective retinal foci in diabetic retinopathy: A semi-automatic detection comparative study. Front. Immunol. 12, 613051. doi:10.3389/fimmu.2021.613051
Miller, D. J., Cascio, M. A., and Rosca, M. G. (2020). Diabetic retinopathy: The role of mitochondria in the neural retina and microvascular disease. Antioxidants 9 (10), 905. doi:10.3390/antiox9100905
Mills, S. A., Jobling, A. I., Dixon, M. A., Bui, B. V., Vessey, K. A., Phipps, J. A., et al. (2021). Fractalkine-induced microglial vasoregulation occurs within the retina and is altered early in diabetic retinopathy. Proc. Natl. Acad. Sci. U. S. A. 118 (51), e2112561118. doi:10.1073/pnas.2112561118
Mishra, M., Duraisamy, A. J., and Kowluru, R. A. (2018). Sirt1: A guardian of the development of diabetic retinopathy. Diabetes 67 (4), 745–754. doi:10.2337/db17-0996
Monickaraj, F., McGuire, P., and Das, A. (2018). Cathepsin D plays a role in endothelial-pericyte interactions during alteration of the blood-retinal barrier in diabetic retinopathy. FASEB J. 32 (5), 2539–2548. doi:10.1096/fj.201700781RR
Mrugacz, M., Bryl, A., and Zorena, K. (2021). Retinal vascular endothelial cell dysfunction and neuroretinal degeneration in diabetic patients. J. Clin. Med. 10 (3), 458. doi:10.3390/jcm10030458
Nakamura, N., Honjo, M., Yamagishi, R., Kurano, M., Yatomi, Y., Watanabe, S., et al. (2021). Neuroprotective role of sphingolipid rheostat in excitotoxic retinal ganglion cell death. Exp. Eye Res. 208, 108623. doi:10.1016/j.exer.2021.108623
Nandi, S. K., Singh, D., Upadhay, J., Gupta, N., Dhiman, N., Mittal, S. K., et al. (2021). Identification of tear-based protein and non-protein biomarkers: Its application in diagnosis of human diseases using biosensors. Int. J. Biol. Macromol. 193, 838–846. doi:10.1016/j.ijbiomac.2021.10.198
Nippert, A. R., Biesecker, K. R., and Newman, E. A. (2018). Mechanisms mediating functional hyperemia in the brain. Neuroscientist 24 (1), 73–83. doi:10.1177/1073858417703033
Nishinaka, A., Nakamura, S., Tanaka, M., Masuda, T., Inoue, Y., Yamamoto, T., et al. (2021). Excess adiponectin in eyes with progressive ocular vascular diseases. FASEB J. 35 (2), e21313. doi:10.1096/fj.202001740RR
Noh, M., Zhang, H., Kim, H., Park, S., Kim, Y.-M., Kwon, Y.-G., et al. (2021). Primaquine diphosphate, a known antimalarial drug, blocks vascular leakage acting through junction stabilization. Front. Pharmacol. 12, 695009. doi:10.3389/fphar.2021.695009
Ogura, S., Kurata, K., Hattori, Y., Takase, H., Ishiguro-Oonuma, T., Hwang, Y., et al. (2017). Sustained inflammation after pericyte depletion induces irreversible blood-retina barrier breakdown. JCI Insight 2 (3), e90905. doi:10.1172/jci.insight.90905
Öhman, T., Tamene, F., Göös, H., Loukovaara, S., and Varjosalo, M. (2018). Systems pathology analysis identifies neurodegenerative nature of age-related vitreoretinal interface diseases. Aging Cell 17 (5), e12809. doi:10.1111/acel.12809
Opdenakker, G., and Abu El-Asrar, A. (2019). Metalloproteinases mediate diabetes-induced retinal neuropathy and vasculopathy. Cell. Mol. Life Sci. 76 (16), 3157–3166. doi:10.1007/s00018-019-03177-3
Park, D. Y., Lee, J., Kim, J., Kim, K., Hong, S., Han, S., et al. (2017). Plastic roles of pericytes in the blood-retinal barrier. Nat. Commun. 8, 15296. doi:10.1038/ncomms15296
Park, W., Kim, J., Choi, S., Kim, T., Park, M., Kim, S., et al. (2021). Human plasminogen-derived N-acetyl-Arg-Leu-Tyr-Glu antagonizes VEGFR-2 to prevent blood-retinal barrier breakdown in diabetic mice. Biomed. Pharmacother. 134, 111110. doi:10.1016/j.biopha.2020.111110
Patel, S., Homaei, A., El-Seedi, H. R., and Akhtar, N. (2018). Cathepsins: Proteases that are vital for survival but can also be fatal. Biomed. Pharmacother. 105, 526–532. doi:10.1016/j.biopha.2018.05.148
Petit, L., Ma, S., Cipi, J., Cheng, S. Y., Zieger, M., Hay, N., et al. (2018). Aerobic glycolysis is essential for normal rod function and controls secondary cone death in retinitis pigmentosa. Cell Rep. 23 (9), 2629–2642. doi:10.1016/j.celrep.2018.04.111
Pitale, P. M., Saltykova, I. V., Adu-Agyeiwaah, Y., Calzi, S. L., Satoh, T., Akira, S., et al. (2021). Tribbles homolog 3 mediates the development and progression of diabetic retinopathy. Diabetes 70 (8), 1738–1753. doi:10.2337/db20-1268
Platania, C. B. M., Fidilio, A., Lazzara, F., Piazza, C., Geraci, F., Giurdanella, G., et al. (2018). Retinal protection and distribution of curcumin in vitro and in vivo. Front. Pharmacol. 9, 670. doi:10.3389/fphar.2018.00670
Portillo, J. C., Lopez Corcino, Y., Miao, Y., Tang, J., Sheibani, N., Kern, T. S., et al. (2017). CD40 in retinal muller cells induces P2X7-dependent cytokine expression in macrophages/microglia in diabetic mice and development of early experimental diabetic retinopathy. Diabetes 66 (2), 483–493. doi:10.2337/db16-0051
Radhakrishnan, R., and Kowluru, R. A. (2020). Long noncoding RNA MALAT1 and regulation of the antioxidant defense system in diabetic retinopathy. Diabetes 70 (1), 227–239. doi:10.2337/db20-0375
Radhakrishnan, R. K. R. (2020). 574-P: Antioxidant defence and long noncoding RNA in diabetic retinopathy. Diabetes 69, 574. doi:10.2337/db20-574-P
Rajagopal, R., Sylvester, B., Zhang, S., Adak, S., Wei, X., Bowers, M., et al. (2021). Glucose-mediated de novo lipogenesis in photoreceptors drives early diabetic retinopathy. J. Biol. Chem. 297 (3), 101104. doi:10.1016/j.jbc.2021.101104
Rao, H., Jalali, J. A., Johnston, T. P., and Koulen, P. (2021). Emerging roles of dyslipidemia and hyperglycemia in diabetic retinopathy: Molecular mechanisms and clinical perspectives. Front. Endocrinol. 12, 620045. doi:10.3389/fendo.2021.620045
Rezzola, S., Corsini, M., Chiodelli, P., Cancarini, A., Nawaz, I. M., Coltrini, D., et al. (2017). Inflammation and N-formyl peptide receptors mediate the angiogenic activity of human vitreous humour in proliferative diabetic retinopathy. Diabetologia 60 (4), 719–728. doi:10.1007/s00125-016-4204-0
Rojo Arias, J. E., Economopoulou, M., Juarez Lopez, D. A., Kurzbach, A., Au Yeung, K. H., Englmaier, V., et al. (2020). VEGF-Trap is a potent modulator of vasoregenerative responses and protects dopaminergic amacrine network integrity in degenerative ischemic neovascular retinopathy. J. Neurochem. 153 (3), 390–412. doi:10.1111/jnc.14875
Rubsam, A., Parikh, S., and Fort, P. E. (2018). Role of inflammation in diabetic retinopathy. Int. J. Mol. Sci. 19 (4), E942. doi:10.3390/ijms19040942
Russo, R., Varano, G. P., Adornetto, A., Nazio, F., Tettamanti, G., Girardello, R., et al. (2018). Rapamycin and fasting sustain autophagy response activated by ischemia/reperfusion injury and promote retinal ganglion cell survival. Cell Death Dis. 9, 981. doi:10.1038/s41419-018-1044-5
Sachdeva, R., Schlotterer, A., Schumacher, D., Matka, C., Mathar, I., Dietrich, N., et al. (2018). TRPC proteins contribute to development of diabetic retinopathy and regulate glyoxalase 1 activity and methylglyoxal accumulation. Mol. Metab. 9, 156–167. doi:10.1016/j.molmet.2018.01.003
Schafer, C. M., Gurley, J. M., Kurylowicz, K., Lin, P. K., Chen, W., Elliott, M. H., et al. (2020). An inhibitor of endothelial ETS transcription factors promotes physiologic and therapeutic vessel regression. Proc. Natl. Acad. Sci. U. S. A. 117 (42), 26494–26502. doi:10.1073/pnas.2015980117
Schlotterer, A., Kolibabka, M., Lin, J., Acunman, K., Dietrich, N., Sticht, C., et al. (2019). Methylglyoxal induces retinopathy-type lesions in the absence of hyperglycemia: Studies in a rat model. FASEB J. 33 (3), 4141–4153. doi:10.1096/fj.201801146RR
Sehgal, P., Mathew, S., Sivadas, A., Ray, A., Tanwar, J., Vishwakarma, S., et al. (2021). LncRNA VEAL2 regulates PRKCB2 to modulate endothelial permeability in diabetic retinopathy. Embo J. 40 (15), e107134. doi:10.15252/embj.2020107134
Shahulhameed, S., Swain, S., Jana, S., Chhablani, J., Ali, M. J., Pappuru, R. R., et al. (2019). A robust model system for retinal hypoxia: Live imaging of calcium dynamics and gene expression studies in primary human mixed retinal culture. Front. Neurosci. 13, 1445. doi:10.3389/fnins.2019.01445
Shahulhameed, S., Vishwakarma, S., Chhablani, J., Tyagi, M., Pappuru, R. R., Jakati, S., et al. (2020). A systematic investigation on complement pathway activation in diabetic retinopathy. Front. Immunol. 11, 154. doi:10.3389/fimmu.2020.00154
Shan, K., Liu, C., Liu, B. H., Chen, X., Dong, R., Liu, X., et al. (2017). Circular noncoding RNA HIPK3 mediates retinal vascular dysfunction in diabetes mellitus. Circulation 136 (17), 1629–1642. doi:10.1161/CIRCULATIONAHA.117.029004
Shu, X., Hu, Y., Huang, C., and Wei, N. (2021). Nimbolide ameliorates the streptozotocin-induced diabetic retinopathy in rats through the inhibition of TLR4/NF-kappa B signaling pathway. Saudi J. Biol. Sci. 28 (8), 4255–4262. doi:10.1016/j.sjbs.2021.06.039
Shu Xs, Z. H., Huang, X., Yang, Y., Wang, D., Zhang, Y., Zhang, W., et al. (2020). Loss of β-catenin via activated GSK3β causes diabetic retinalneurodegeneration by instigating a vicious cycle of oxidativestress-driven mitochondrial impairment. Aging (Albany NY) 12 (13), 13437–13462.
Shu, X., Zhang, Y., Li, M., Huang, X., Yang, Y., Zeng, J., et al. (2019). Topical ocular administration of the GLP-1 receptor agonist liraglutide arrests hyperphosphorylated tau-triggered diabetic retinal neurodegeneration via activation of GLP-1R/Akt/GSK3β signaling. Neuropharmacology 153, 1–12. doi:10.1016/j.neuropharm.2019.04.018
Siddiqui, M. K., Kennedy, G., Carr, F., Doney, A. S. F., Pearson, E. R., Morris, A. D., et al. (2018). Lp-PLA2 activity is associated with increased risk of diabetic retinopathy: A longitudinal disease progression study. Diabetologia 61 (6), 1344–1353. doi:10.1007/s00125-018-4601-7
Simo, R., Bogdanov, P., Ramos, H., Huerta, J., Simo-Servat, O., Hernandez, C., et al. (2021). Effects of the topical administration of semaglutide on retinal neuroinflammation and vascular leakage in experimental diabetes. Biomedicines 9 (8), 926. doi:10.3390/biomedicines9080926
Song, S., Bao, S., Zhang, C., Zhang, J., Lv, J., Li, X., et al. (2021). Stimulation of AMPK prevents diabetes-induced photoreceptor cell degeneration. Oxid. Med. Cell. Longev. 2021, 5587340. doi:10.1155/2021/5587340
Stravalaci, M., Ferrara, M., Pathak, V., Davi, F., Bottazzi, B., Mantovani, A., et al. (2021). The long pentraxin PTX3 as a new biomarker and pharmacological target in age-related macular degeneration and diabetic retinopathy. Front. Pharmacol. 12, 811344. doi:10.3389/fphar.2021.811344
Sun, W., Yu, J., and Kang, Q. (2020). Upregulation of heme oxygenase-1 by Brahma-related gene 1 through Nrf2 signaling confers protective effect against high glucose-induced oxidative damage of retinal ganglion cells. Eur. J. Pharmacol. 875, 173038. doi:10.1016/j.ejphar.2020.173038
Szabo, K., Enzsoly, A., Dekany, B., Szabo, A., Hajdu, R. I., Radovits, T., et al. (2017). Histological evaluation of diabetic neurodegeneration in the retina of zucker diabetic fatty (ZDF) rats. Sci. Rep. 7, 8891. doi:10.1038/s41598-017-09068-6
Taki, K., Horie, T., Kida, T., Mimura, M., Ikeda, T., Oku, H., et al. (2020). Impairment of autophagy causes superoxide formation and caspase activation in 661 W cells, a cell line for cone photoreceptors, under hyperglycemic conditions. Int. J. Mol. Sci. 21 (12), E4240. doi:10.3390/ijms21124240
Tamhane, M., Cabrera-Ghayouri, S., Abelian, G., and Viswanath, V. (2019). Review of biomarkers in ocular matrices: Challenges and opportunities. Pharm. Res. 36 (3), 40. doi:10.1007/s11095-019-2569-8
Tang, L., Zhang, C., Lu, L., Tian, H., Liu, K., Luo, D., et al. (2022). Melatonin maintains inner blood–retinal barrier by regulating microglia via inhibition of PI3K/Akt/Stat3/NF-κB signaling pathways in experimental diabetic retinopathy. Front. Immunol. 13, 831660. doi:10.3389/fimmu.2022.831660
Tawfik, A. M., Mohamed, R., Alhusban, S., Chu, S. M., Khalil, A. Q., Bartolli, M., et al. (2019). 594-P: Homocysteine as a predictor for diabetic retinopathy risk and management. Diabetes 68 (1), 594. doi:10.2337/db19-594-P
Tekus, V., Horvath, A. I., Cseko, K., Szabadfi, K., Kovacs-Valasek, A., Danyadi, B., et al. (2021). Protective effects of the novel amine-oxidase inhibitor multi-target drug SZV 1287 on streptozotocin-induced beta cell damage and diabetic complications in rats. Biomed. Pharmacother. 134, 111105. doi:10.1016/j.biopha.2020.111105
Thomas, A. A., Biswas, S., Feng, B., Chen, S., Gonder, J., Chakrabarti, S., et al. (2019a). lncRNA H19 prevents endothelial-mesenchymal transition in diabetic retinopathy. Diabetologia 62 (3), 517–530. doi:10.1007/s00125-018-4797-6
Thomas, C. N., Berry, M., Logan, A., Blanch, R. J., and Ahmed, Z. (2017). Caspases in retinal ganglion cell death and axon regeneration. Cell Death Discov. 3, 17032. doi:10.1038/cddiscovery.2017.32
Thomas, R. L., Halim, S., Gurudas, S., Sivaprasad, S., and Owens, D. R. (2019b). IDF diabetes atlas: A review of studies utilising retinal photography on the global prevalence of diabetes related retinopathy between 2015 and 2018. Diabetes Res. Clin. Pract. 157, 107840. doi:10.1016/j.diabres.2019.107840
Ting, K. K., Zhao, Y., Shen, W., Coleman, P., Yam, M., Chan-Ling, T., et al. (2019). Therapeutic regulation of VE-cadherin with a novel oligonucleotide drug for diabetic eye complications using retinopathy mouse models. Diabetologia 62 (2), 322–334. doi:10.1007/s00125-018-4770-4
Tonade, D., and Kern, T. S. (2021). Photoreceptor cells and RPE contribute to the development of diabetic retinopathy. Prog. Retin. Eye Res. 83, 100919. doi:10.1016/j.preteyeres.2020.100919
Torres-Cuevas, I., Millan, I., Asensi, M., Vento, M., Oger, C., Galano, J. M., et al. (2021). Analysis of lipid peroxidation by UPLC-MS/MS and retinoprotective effects of the natural polyphenol pterostilbene. Antioxidants 10 (2), 168. doi:10.3390/antiox10020168
Tu, Y., Li, L., Zhu, L., Guo, Y., Du, S., Zhang, Y., et al. (2021a). Geniposide attenuates hyperglycemia-induced oxidative stress and inflammation by activating the Nrf2 signaling pathway in experimental diabetic retinopathy. Oxid. Med. Cell. Longev. 2021, 9247947. doi:10.1155/2021/9247947
Tu, Y., Song, E., Wang, Z., Ji, N., Zhu, L., Wang, K., et al. (2021b). Melatonin attenuates oxidative stress and inflammation of Muller cells in diabetic retinopathy via activating the Sirt1 pathway. Biomed. Pharmacother. 137, 111274. doi:10.1016/j.biopha.2021.111274
Uddin, M. I., Kilburn, T. C., Duvall, C. L., and Penn, J. S. (2020). Visualizing HIF-1α mRNA in a subpopulation of bone marrow-derived cells to predict retinal neovascularization. ACS Chem. Biol. 15 (11), 3004–3012. doi:10.1021/acschembio.0c00662
Usui-Ouchi, A., and Friedlander, M. (2019). Anti-VEGF therapy: Higher potency and long-lasting antagonism are not necessarily better. J. Clin. Invest. 129 (8), 3032–3034. doi:10.1172/JCI129862
Van Hove, I., De Groef, L., Boeckx, B., Modave, E., Hu, T. T., Beets, K., et al. (2020). Single-cell transcriptome analysis of the Akimba mouse retina reveals cell-type-specific insights into the pathobiology of diabetic retinopathy. Diabetologia 63 (10), 2235–2248. doi:10.1007/s00125-020-05218-0
Villarejo-Zori, B., Jimenez-Loygorri, J. I., Zapata-Munoz, J., Bell, K., and Boya, P. (2021). New insights into the role of autophagy in retinal and eye diseases. Mol. Asp. Med. 82, 101038. doi:10.1016/j.mam.2021.101038
Vujosevic, S., Bini, S., Torresin, T., Berton, M., Midena, G., Parrozzani, R., et al. (2017). Hyperreflective retinal spots in normal and diabetic eyes: B-scan and en face spectral domain optical coherence tomography evaluation. RETINA 37 (6), 1092–1103. doi:10.1097/iae.0000000000001304
Vuori, N., Sandholm, N., Kumar, A., Hietala, K., Syreeni, A., Forsblom, C., et al. (2019). CACNB2 is a novel susceptibility gene for diabetic retinopathy in type 1 diabetes. Diabetes 68 (11), 2165–2174. doi:10.2337/db19-0130
Wang, H., Li, J., Zhong, P., Wang, S., Zhang, L., Yang, R., et al. (2019). Blocking CXCR3 with AMG487 ameliorates the blood-retinal barrier disruption in diabetic mice through anti-oxidative. Life Sci. 228, 198–207. doi:10.1016/j.lfs.2019.04.016
Wang, J. C., Ku, H. Y., Chen, T. S., and Chuang, H. S. (2017a). Detection of low-abundance biomarker lipocalin 1 for diabetic retinopathy using optoelectrokinetic bead-based immunosensing. Biosens. Bioelectron. 89 (2), 701–709. doi:10.1016/j.bios.2016.11.014
Wang, N., Wang, L., Zhang, C., Tan, H. Y., Zhang, Y., Feng, Y., et al. (2021). Berberine suppresses advanced glycation end products-associated diabetic retinopathy in hyperglycemic mice. Clin. Transl. Med. 11 (11), e569. doi:10.1002/ctm2.569
Wang, W., and Lo, A. C. Y. (2018). Diabetic retinopathy: Pathophysiology and treatments. Int. J. Mol. Sci. 19 (6), E1816. doi:10.3390/ijms19061816
Wang, X., Chen, W., Lao, W., and Chen, Y. (2022). Silencing LncRNA PVT1 reverses high glucose-induced regulation of the high expression of PVT1 in HRMECs by targeting miR-128-3p. Horm. Metab. Res. 54 (2), 119–125. doi:10.1055/a-1730-5091
Wang, Y., Zhou, Y., Xiao, L., Zheng, S., Yan, N., Chen, D., et al. (2017b). E2f1 mediates high glucose-induced neuronal death in cultured mouse retinal explants. Cell Cycle 16 (19), 1824–1834. doi:10.1080/15384101.2017.1361070
Weh, E., Lutrzykowska, Z., Smith, A., Hager, H., Pawar, M., Wubben, T. J., et al. (2020). Hexokinase 2 is dispensable for photoreceptor development but is required for survival during aging and outer retinal stress. Cell Death Dis. 11 (6), 422. doi:10.1038/s41419-020-2638-2
Wei, J., Xiang, X.-H., Tang, Y., Qin, D.-L., Wu, J.-M., Yu, C.-L., et al. (2021). Lychee seed polyphenol protects blood-retinal barrier by increasing tight joint proteins and inhibiting the activation of TLR4/MYD88/NF-kappa B-mediated NLRP3 inflammasome. Food Agric. Immunol. 32 (1), 516–539. doi:10.1080/09540105.2021.1968352
Wong, T. Y., Sun, J., Kawasaki, R., Ruamviboonsuk, P., Gupta, N., Lansingh, V. C., et al. (2018). Guidelines on diabetic eye care: The international Council of Ophthalmology recommendations for screening, follow-up, referral, and treatment based on resource settings. Ophthalmology 125 (10), 1608–1622. doi:10.1016/j.ophtha.2018.04.007
Wu, J.-h., Li, Y.-n., Chen, A.-q., Hong, C.-d., Zhang, C.-l., Wang, H.-l., et al. (2020). Inhibition of Sema4D/PlexinB1 signaling alleviates vascular dysfunction in diabetic retinopathy. EMBO Mol. Med. 12 (2), e10154. doi:10.15252/emmm.201810154
Xiao, Y., Hu, X., Fan, S., Zhong, J., Mo, X., Liu, X., et al. (2021). Single-cell transcriptome profiling reveals the suppressive role of retinal neurons in microglia activation under diabetes mellitus. Front. Cell Dev. Biol. 9, 680947. doi:10.3389/fcell.2021.680947
Xie, H., Zhang, C., Liu, D., Yang, Q., Tang, L., Wang, T., et al. (2021). Erythropoietin protects the inner blood-retinal barrier by inhibiting microglia phagocytosis via Src/Akt/cofilin signalling in experimental diabetic retinopathy. Diabetologia 64 (1), 211–225. doi:10.1007/s00125-020-05299-x
Xuan, Q., Ouyang, Y., Wang, Y., Wu, L., Li, H., Luo, Y., et al. (2020). Multiplatform metabolomics reveals novel serum metabolite biomarkers in diabetic retinopathy subjects. Adv. Sci. 7 (22), 2001714. doi:10.1002/advs.202001714
Yan, Z., Wang, C., Meng, Z., Gan, L., Guo, R., Liu, J., et al. (2022). C1q/TNF-Related protein 3 prevents diabetic retinopathy via AMPK-dependent stabilization of blood-retinal barrier tight junctions. Cells 11 (5), 779. doi:10.3390/cells11050779
Yang, J., Chen, C., McLaughlin, T., Wang, Y., Le, Y. Z., Wang, J. J., et al. (2019). Loss of X-box binding protein 1 in Muller cells augments retinal inflammation in a mouse model of diabetes. Diabetologia 62 (3), 531–543. doi:10.1007/s00125-018-4776-y
Yang, J., Yang, K., Meng, X., Liu, P., Fu, Y., Wang, Y., et al. (2021a). Silenced SNHG1 inhibited epithelial-mesenchymal transition and inflammatory response of ARPE-19 cells induced by high glucose. J. Inflamm. Res. 14, 1563–1573. doi:10.2147/jir.S299010
Yang, M., Chen, Y., Vagionitis, S., Kortvely, E., Ueffing, M., Schmachtenberg, O., et al. (2021b). Expression of glucose transporter-2 in murine retina: Evidence for glucose transport from horizontal cells to photoreceptor synapses. J. Neurochem. 160 (2), 283–296. doi:10.1111/jnc.15533
Yang, S., Zhang, J., and Chen, L. (2020). The cells involved in the pathological process of diabetic retinopathy. Biomed. Pharmacother. 132, 110818. doi:10.1016/j.biopha.2020.110818
Yang, T. T., Li, H., and Dong, L. J. (2021c). Role of glycolysis in retinal vascular endothelium, glia, pigment epithelium, and photoreceptor cells and as therapeutic targets for related retinal diseases. Int. J. Ophthalmol. 14 (9), 1302–1309. doi:10.18240/ijo.2021.09.02
Ye, L., Guo, H., Wang, Y., Peng, Y., Zhang, Y., Li, S., et al. (2021). Exosomal circEhmt1 released from hypoxia-pretreated pericytes regulates high glucose-induced microvascular dysfunction via the NFIA/NLRP3 pathway. Oxid. Med. Cell. Longev. 2021, 8833098. doi:10.1155/2021/8833098
Ying, Y., Zhang, Y. L., Ma, C. J., Li, M. Q., Tang, C. Y., Yang, Y. F., et al. (2019). Neuroprotective effects of ginsenoside Rg1 against hyperphosphorylated tau-induced diabetic retinal neurodegeneration via activation of IRS-1/akt/gsk3β signaling. J. Agric. Food Chem. 67 (30), 8348–8360. doi:10.1021/acs.jafc.9b02954
YokomizO, H., Park, K., Clermont, A., Maeda, Y., Fickweiler, W., Ishikado, A., et al. (2019). 597-P: Retinol binding protein 3 in diabetic retinopathy: Mechanisms of protective activity. Diabetes 68 (1), 597. doi:10.2337/db19-597-P
You, Z.-P., Zhang, Y.-L., Shi, K., Shi, L., Zhang, Y.-Z., Zhou, Y., et al. (2017). Suppression of diabetic retinopathy with GLUT1 siRNA. Sci. Rep. 7, 7437. doi:10.1038/s41598-017-07942-x
Yu, B., Xiao, M., Yang, F., Xiao, J., Zhang, H., Su, L., et al. (2021a). MicroRNA-431-5p encapsulated in serum extracellular vesicles as a biomarker for proliferative diabetic retinopathy. Int. J. Biochem. Cell Biol. 135, 105975. doi:10.1016/j.biocel.2021.105975
Yu, C. G., Yuan, S. S., Yang, L. Y., Ke, J., Zhang, L. J., Lang, J. N., et al. (2018). Angiopoietin-like 3 is a potential biomarker for retinopathy in type 2 diabetic patients. Am. J. Ophthalmol. 191, 34–41. doi:10.1016/j.ajo.2018.03.040
Yu, G., Aaberg, M. T., Patel, T. P., Iyengar, R. S., Powell, C., Tran, A., et al. (2020). Quantification of retinal nonperfusion and neovascularization with ultrawidefield fluorescein angiography in patients with diabetes and associated characteristics of advanced disease. JAMA Ophthalmol. 138 (6), 680–688. doi:10.1001/jamaophthalmol.2020.1257
Yu, H. J., Ehlers, J. P., Sevgi, D. D., Hach, J., O'Connell, M., Reese, J. L., et al. (2021b). Real-time photographic- and fluorescein angiographic-guided management of diabetic retinopathy: Randomized PRIME trial outcomes. Am. J. Ophthalmol. 226, 126–136. doi:10.1016/j.ajo.2021.01.024
Yu, J., Qin, M., Li, J., and Cui, S. (2021c). LncRNA SNHG4 sponges miR-200b to inhibit cell apoptosis in diabetic retinopathy. Arch. Physiol. Biochem., 1–6. doi:10.1080/13813455.2021.1900873
Yun, J. H., Jeong, H. S., Kim, K. J., Han, M. H., Lee, E. H., Lee, K., et al. (2018). β-Adrenergic receptor agonists attenuate pericyte loss in diabetic retinas through Akt activation. FASEB J. 32 (5), 2324–2338. doi:10.1096/fj.201700570RR
Zhang, J., and He, J. (2019). CTRP3 inhibits high glucose-induced oxidative stress and apoptosis in retinal pigment epithelial cells. Artif. Cells Nanomed. Biotechnol. 47 (1), 3758–3764. doi:10.1080/21691401.2019.1666864
Zhang, J., Liu, R., Kuang, H. Y., Gao, X. Y., and Liu, H. L. (2017). Protective treatments and their target retinal ganglion cells in diabetic retinopathy. Brain Res. Bull. 132, 53–60. doi:10.1016/j.brainresbull.2017.05.007
Zhang, L., Zhu, T., He, F., and Li, X. (2021). Senescence marker protein 30 (SMP30) protects against high glucose-induced apoptosis, oxidative stress and inflammatory response in retinal ganglion cells by enhancing Nrf2 activation via regulation of Akt/GSK-3β pathway. Int. Immunopharmacol. 101 (2), 108238. doi:10.1016/j.intimp.2021.108238
Zhang, R., Shen, W., Du, J., and Gillies, M. C. (2020a). Selective knockdown of hexokinase 2 in rods leads to age-related photoreceptor degeneration and retinal metabolic remodeling. Cell Death Dis. 11 (10), 885. doi:10.1038/s41419-020-03103-7
Zhang, S. S., Hu, J. Q., Liu, X. H., Chen, L. X., Chen, H., Guo, X. H., et al. (2020b). Role of moesin phosphorylation in retinal pericyte migration and detachment induced by advanced glycation endproducts. Front. Endocrinol. 11, 603450. doi:10.3389/fendo.2020.603450
Zhang, X., Lu, Y., He, N., and Wang, F. (2020c). Downregulation of PHLPP1 ameliorates high glucose-evoked injury in retinal ganglion cells by attenuating apoptosis and oxidative stress through enhancement of Nrf2 activation. Exp. Cell Res. 397 (2), 112344. doi:10.1016/j.yexcr.2020.112344
Zhang, X. Z., Tu, W. J., Wang, H., Zhao, Q., Liu, Q., Sun, L., et al. (2018). Circulating serum fatty acid-binding protein 4 levels predict the development of diabetic retinopathy in type 2 diabetic patients. Am. J. Ophthalmol. 187, 71–79. doi:10.1016/j.ajo.2017.12.022
Zhou, L., Xu, Z., Oh, Y., Gamuyao, R., Lee, G., Xie, Y., et al. (2021). Myeloid cell modulation by a GLP-1 receptor agonist regulates retinal angiogenesis in ischemic retinopathy. JCI Insight 6 (23), e93382. doi:10.1172/jci.insight.93382
Zhu, F., Shao, J., Tian, Y., and Xu, Z. (2021a). Sulfiredoxin-1 protects retinal ganglion cells from high glucose-induced oxidative stress and inflammatory injury by potentiating Nrf2 signaling via the Akt/GSK-3β pathway. Int. Immunopharmacol. 101 (2), 108221. doi:10.1016/j.intimp.2021.108221
Zhu, H., Zhang, W., Zhao, Y., Shu, X., Wang, W., Wang, D., et al. (2018). GSK3-mediated tau hyperphosphorylation triggers diabetic retinal neurodegeneration by disrupting synaptic and mitochondrial functions. Mol. Neurodegener. 13, 62. doi:10.1186/s13024-018-0295-z
Zhu, Y., Wang, X., Zhou, X., Ding, L., Liu, D., Xu, H., et al. (2021b). DNMT1-mediated PPAR alpha methylation aggravates damage of retinal tissues in diabetic retinopathy mice. Biol. Res. 54 (1), 25. doi:10.1186/s40659-021-00347-1
Zhu, Z., Duan, P., Song, H., Zhou, R., and Chen, T. (2021c). Downregulation of Circular RNA PSEN1 ameliorates ferroptosis of the high glucose treated retinal pigment epithelial cells via miR-200b-3p/cofilin-2 axis. Bioengineered 12 (2), 12555–12567. doi:10.1080/21655979.2021.2010369
Keywords: diabetic retinopathy, cells, potential drug targets, biomarkers, drug therapy
Citation: Ren J, Zhang S, Pan Y, Jin M, Li J, Luo Y, Sun X and Li G (2022) Diabetic retinopathy: Involved cells, biomarkers, and treatments. Front. Pharmacol. 13:953691. doi: 10.3389/fphar.2022.953691
Received: 26 May 2022; Accepted: 06 July 2022;
Published: 09 August 2022.
Edited by:
Paola Bagnoli, University of Pisa, ChinaCopyright © 2022 Ren, Zhang, Pan, Jin, Li, Luo, Sun and Li. This is an open-access article distributed under the terms of the Creative Commons Attribution License (CC BY). The use, distribution or reproduction in other forums is permitted, provided the original author(s) and the copyright owner(s) are credited and that the original publication in this journal is cited, in accordance with accepted academic practice. No use, distribution or reproduction is permitted which does not comply with these terms.
*Correspondence: Yun Luo, ly20040423@126.com; Xiaobo Sun , sun_xiaobo163@163.com; Guang Li, lhbg311@hotmail.com