- Department of Gastroenterology and Hepatology, Sichuan University-University of Oxford Huaxi Joint Centre for Gastrointestinal Cancer, West China Hospital, Sichuan University, Chengdu, China
Acute-on-chronic liver failure (ACLF) usually develops based on acute decompensation (AD) of cirrhosis and is characterized by intense systemic inflammation, multiple organ failure, and high short-term mortality. Validated biomarkers for the diagnosis and prognosis of ACLF remain to be clarified. Metabolomics is an emerging method used to measure low-molecular-weight metabolites and is currently frequently implemented to understand pathophysiological processes involved in disease progression, as well as to search for new diagnostic or prognostic biomarkers of various disorders. The characterization of metabolites in ACLF has recently been described via metabolomics. The role of metabolites in the pathogenesis of ACLF deserves further investigation and improvement and could be the basis for the development of new diagnostic and therapeutic strategies. In this review, we focused on the contributions of metabolomics on uncovering metabolic profiles in patients with ACLF, the key metabolic pathways that are involved in the progression of ACLF, and the potential metabolite-associated therapeutic targets for ACLF.
Introduction
Acute-on-chronic liver failure (ACLF) is a distinct disease state in patients with advanced chronic liver disease characterized by liver failure due to an acute hepatic injury in an underlying chronic liver disease with high 28-days mortality (Sarin et al., 2019). ACLF mainly progresses from acute decompensation (AD) of cirrhosis, which refers to the occurrence of ascites, encephalopathy, gastrointestinal hemorrhage, or any combination of these disorders in patients with cirrhosis (Trebicka et al., 2020). Bacterial infections, alcoholism, and chronic viral hepatitis relapse are the most common precipitating factors in half of patients with ACLF; the remaining patients do not have an identifiable trigger (Moreau et al., 2013; Shi et al., 2015). Patients with ACLF present intense systemic inflammation and multiple organ failures (liver, kidney, brain, coagulation, circulation, and respiration), leading to high short-term mortality (Sarin and Choudhury, 2016; Arroyo et al., 2020). Accumulating evidence has shown that systemic inflammation and immune paralysis are considered the main drivers of extensive tissue injury and organ failure in patients with AD who develop ACLF (Clària et al., 2016; Trebicka et al., 2019). Systemic inflammation has been reported to result mainly from the activation of innate immune cells by pathogen-associated molecular patterns (PAMPs) or damage-associated molecular patterns (DAMPs) (Fernández et al., 2018; Aizawa et al., 2020). However, the underlying mechanisms of ACLF at the tissue and cellular levels that lead to the occurrence and maintenance of organ failures remain to be elucidated. It is crucial to uncover the pathogenesis of ACLF to establish reliable biomarkers to determine the progression and/or regression of ACLF in response to a given treatment.
Metabolomics is a potent approach for measuring the products of individual protein expression, based on comprehensive monitoring of metabolites (mass <1,000 Da) in biological systems (Johnson et al., 2016). The changes at the genome or proteome level of the organism reflect the tendency for specific behaviors of biological systems, while the changes in metabolites embody the current condition of the organism (Bujak et al., 2015). Subtle changes at the genome or proteome level can be amplified due to the higher sensitivity of metabolomics. Moreover, the small molecular chemicals measured by metabolomics are downstream from the genome, transcriptome, and proteome, providing a highly integrated profile of biological status relative to other omics (Newgard, 2017). Owing to metabolome consisting of thousands of different chemical classes, metabolomic measurements are more difficult than genome or proteome. Additionally, the identification of metabolites remains a major challenge in metabolomics due to inadequate databases (Djoumbou Feunang et al., 2016; Wishart et al., 2018).
Metabolomics is mainly divided into targeted and non-targeted metabolomics. Targeted metabolomics focus on identifying and quantifying specific compound classes or metabolic pathways. Untargeted metabolomics aims to include all the metabolites (Jacob et al., 2019). Various analytical techniques are applied in both targeted and untargeted metabolomic studies. Liquid chromatography coupled with mass spectrometry (LC-MS) is widely used owing to the extensive availability and continuous advancement of instruments, while nuclear magnetic resonance (NMR) is suitable for detecting the compounds which contain the hydrogen atoms (Jang et al., 2018). By virtue of continuous advancement in sensitive analysis techniques and progressive biostatistics, metabolites in blood, urine or other body fluids can be determined and identified (Johnson et al., 2016). Changes in metabolites may appear earlier than specific symptoms in diseases. Hence, metabolomics is commonly utilized to search for new diagnostic and prognostic disease biomarkers and to gain a deeper understanding of the pathogenesis of various diseases (Gowda et al., 2008; Mamas et al., 2011).
Recently, the concept that the combined effect of cytokines/chemokines and metabolite-derived factors that act on metabotoxins contributes to systemic inflammation and tissue/organ injury of ACLF has been reinforced. It is noteworthy that metabolomics has been used in ACLF research to characterize ACLF metabolic changes and to identify metabolic signatures as prognostic indicators (McPhail et al., 2016; Moreau et al., 2020; Clària et al., 2021). These studies highlight the intrinsic biological activity of metabolites and provide new ideas for the application of metabolomics, as they can be used to identify metabolites that act as drivers or mediators of biological processes, and thus better understand their physiological role in the progression of ACLF.
In this review, we summarize the pivotal metabolic pathways involved in ACLF that have been identified by metabolomics and assess the use of metabolomics to identify biomarkers related to ACLF.
Key metabolic pathways associated with ACLF
Various metabolic pathways are altered in ACLF due to systemic inflammation, stimulating the hypothalamic-pituitary-adrenal axis and sympathetic nervous system to activate glycogenolysis, proteolysis, and lipolysis. Herein, we review several key metabolic pathways involved in the progression of ACLF (Figure 1).
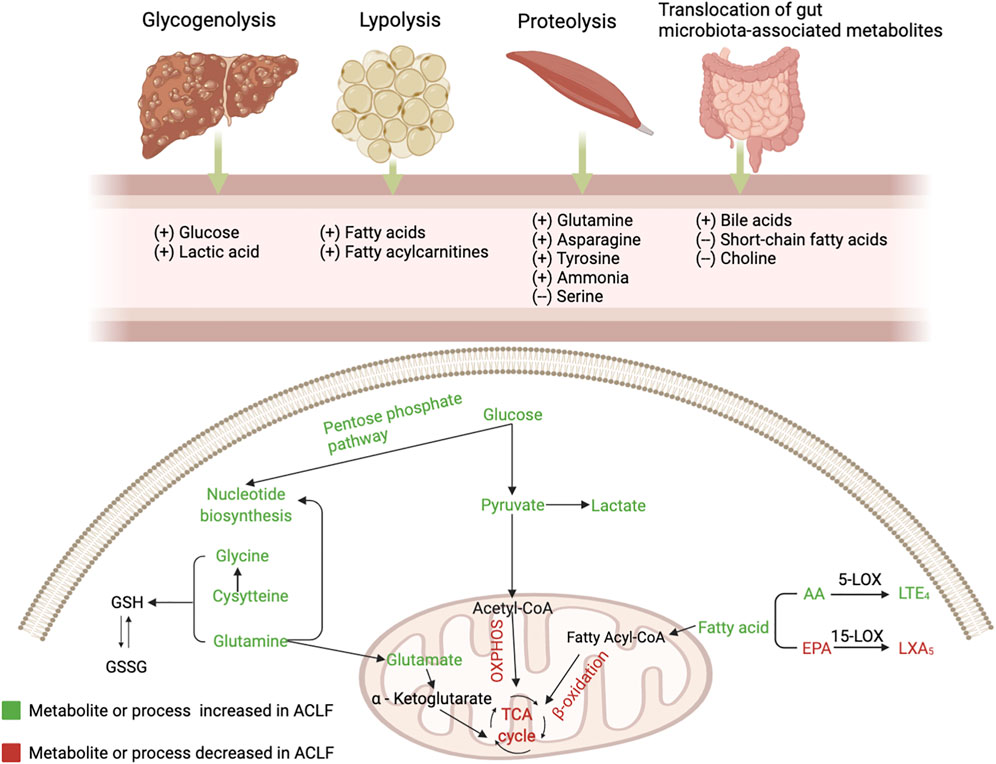
FIGURE 1. The alterations of serum metabolites and key metabolic pathways in acute-on-chronic liver failure (ACLF). Glycogenolysis (liver), proteolysis (mainly muscles), lipolysis (adipose tissue), and bacterial translocation (gut) are induced in the context of ACLF, resulting in the release of glucose, amino acids, fatty acids and gut microbiota-associated metabolites. During PAMP-induced systemic inflammation, glucose is used for promptly producing ATP through glycolysis and enters the pentose phosphate pathway that is involved in nucleotide synthesis, while mitochondrial oxidative phosphorylation (OXPHOS) is suppressed; Mitochondrial β-oxidation of FAs is inhibited and blood level of fatty acylcarnitines is increased; Increased generation and accumulation of many blood amino acid metabolites are involved in various amino acid metabolism. AA, arachidonic acid; EPA, eicosapentaenoic acid; GSH, reduced glutathione; GSSG, oxidized glutathione; LOX, lipoxygenase; LT, leukotriene; LX, lipoxin; OXPHOS, oxidative phosphorylation; TCA, tricarboxylic acid.
Inhibition of oxidative phosphorylation
The liver is the main organ that regulates various pathways controlling glucose metabolism, including glycogenesis, glycogenolysis, glycolysis, and gluconeogenesis (Nordlie et al., 1999). ACLF is characterized by changes in glucose catabolism through specific extramitochondrial pathways (Moreau et al., 2020). The main site of glucose metabolism is transferred to the cytoplasm from the mitochondria in ACLF (Zhang et al., 2021). In the context of systemic inflammation, innate and adaptive immune cells are stimulated by PAMPs or DAMPs. Glucose is distributed to immune cells to maintain energy anabolism and the immune response, which activates intracellular glycolysis pathways by activating key enzymes associated with glycolysis to rapidly generate adenosine triphosphate (ATP) (Van Wyngene et al., 2018; Zhang et al., 2021). Furthermore, mitochondrial OXPHOS is inhibited, partly due to suppression of the electron transport chain by excessive nitric oxide (Van Wyngene et al., 2018; Wang et al., 2019; Moreau et al., 2020). In addition, inhibition of OXPHOS is also associated with increased generation of reactive oxygen species (ROS) (Chan, 2006). The source of mitochondrial OXPHOS is varied, while glycolysis relies on glucose as the single energy source (Mills et al., 2017). Hence, it is less efficient at producing ATP in ACLF.
Extramitochondrial pathways
Glucose can be involved in the pentose phosphate pathway, contributing to the production of ribose and nicotinamide adenine dinucleotide phosphate (NADPH). Increased ribose promotes nucleotide synthesis, which leads to the production of inflammatory cytokines. NADPH, a hydrogen carrier, is involved in various anabolic reactions and can also produce ROS, further inhibiting mitochondrial OXPHOS (O'Neill and Pearce, 2016).
Zhang et al. (Zhang et al., 2021) indicated that extramitochondrial pathways, including glycolysis, glycogenolysis, and pentose phosphate pathway, were excessively activated in the peripheral blood mononuclear cells (PBMCs) from patients with AD and ACLF. Moreover, the nitrogen and carbon sources were utilized exceedingly in PBMCs from ACLF. Additionally, another metabolic characteristic of PBMCs from AD and ACLF patients is the impaired pyruvate decarboxylation to acetyl-CoA, a process that is intermediated by the pyruvate dehydrogenase complex (PDC) (Zhang et al., 2021). PDC, a multi-enzyme complex located in the mitochondrial matrix, plays a key role in connecting glycolysis with the tricarboxylic acid (TCA) cycle (Reed, 1981). Accordingly, the impaired PDC may partly explain the reduction in OXPHOS in ACLF (Zhang et al., 2021). Generally, disturbance of glycometabolism in leukocytes may further enhance the inflammatory response in patients with ACLF. Indeed, energy production from glycolysis can not be maintained for the long term in advanced chronic liver disease (Nishikawa et al., 2014). Thus, how energy produces remains to be elucidated with the development of ACLF.
Kynurenine pathway
Pro-inflammatory cytokines, such as interferon (INF)-γ or tumor necrosis factor (TNF)-α, induce tryptophan degradation through the kynurenine pathway, and metabolites of this pathway are involved in the pathogenesis of inflammatory diseases and cancer (Cervenka et al., 2017). It has been confirmed that kynurenine pathway (KP) activation is associated with ACLF development and is also an independent predictor of short-term death in patients with ACLF (Clària et al., 2019). Notably, the lower concentrations of kynurenate and xanthurenate of KP in patients treated with simvastatin and rifaximin indicate an inhibition of this metabolic pathway (Pose et al., 2021).
Ketone bodies and the ammonia pathway
Amino acids act as nutrients and intracellular signaling molecules, regulating key metabolic pathways that are essential for cellular activity (Baker, 2009). In the context of systemic inflammation, substantial inflammation-linked molecules and acute phase proteins are produced by activated leukocytes and hepatocytes, respectively (Moreau et al., 2013; Clària et al., 2016). The production of these molecules involved in the inflammatory response may be based on the mobilization of a large number of proteinogenic amino acids from skeletal muscle (Zaccherini et al., 2021). Ketone bodies, as fuel for tissues and organs in the body, are produced from fatty acids and ketogenic amino acids (Ganeshan et al., 2019). In ACLF, the production of ketone bodies in the liver relies on the catabolism of ketogenic amino acids due to the inhibition of fatty acid β oxidation (Ganeshan et al., 2019; Moreau et al., 2020). Furthermore, the transsulfuration pathway, a part of the methionine cycle that is involved in one-carbon metabolism, is activated—contributing to the synthesis of glutathione to resist systemic oxidative stress in ACLF (Sanderson et al., 2019).
Circulating ammonia, mainly derived from amino acids through phosphate-activated glutaminase, is increased in decompensation and ACLF (Sawhney et al., 2016). Portosystemic shunts and reduced activity of the urea cycle weaken ammonia removal capacities, leading to hyperammonaemia (Wright et al., 2011). Elevated ammonia not only causes hepatic encephalopathy but is also associated with death and organ failures (Poh and Chang, 2012; Shalimar et al., 2019). Ammonia can serve as a cytotoxic product by changing the cell membrane potential and Pondus Hydrogenii (PH) and producing several free radicals, which leads to mitochondrial dysfunction and aggravates organ failure (Rose et al., 2005; Dasarathy et al., 2017). Furthermore, hyperammonemia can aggravate portal pressure by stellate cell contraction and can directly impair neutrophil phagocytosis, contributing to systemic inflammation (Shawcross et al., 2008; Jalan et al., 2016).
Fatty acylcarnitines and the sphingolipids pathway
Immune-metabolic dysregulation and inflammation can be mediated by changes in lipid composition (Ertunc and Hotamisligil, 2016). Levels of a large series of fatty acids are increased in plasma due to lipolysis in adipose tissue due to systemic inflammation (Van Wyngene et al., 2018). The liver plays a key role in the synthesis of endogenous lipids (Eisenberg and Levy, 1975). Accordingly, suppression of serum lipid levels is largely attributed to the disturbance of liver function.
There is a reduction in fatty acids (FAs) catabolism through mitochondrial β-oxidation in ACLF(25). Increased levels of fatty acylcarnitines in plasma indicate that mitochondrial β-oxidation is inhibited in peripheral organs in ACLF, partly resulting from the suppression of translocation of fatty acylcarnitines into the mitochondrial matrix mediated by the peroxisome proliferator-activated receptor (PPAR)-α (Ganeshan et al., 2019; Moreau et al., 2020). Ultimately, a large amount of FAs, which cannot be dissimilated via β-oxidation, together with ROS produced by systemic inflammation, causes mitochondrial damage, which contributes to the progression of extrahepatic organ failure (Moreau et al., 2020). Sphingolipids are basic components of cytomembranes and are involved in immunomodulation and maintenance of cell survival (Hannun and Obeid, 2018). Reduced intermediates involved in sphingolipid synthesis and downregulated genes coding for enzymes involved in sphingolipid biosynthesis partly explain the inhibition of the sphingolipid pathway in ACLF (Clària et al., 2021). Paradoxically, a multicenter study from the Chinese Group for the Study of Severe Hepatitis B (COSSH) demonstrated that sphingolipid metabolic pathways were upregulated in PBMCs from acute hepatitis B virus-related acute-on-chronic liver failure (HBV-ACLF), which could be partly explained by different etiologies (Li et al., 2021).
Bile acids pathway
Increased serum bile acid levels indicate deterioration of liver clearance and the formation of portosystemic shunting (Krähenbühl and Reichen, 1988). Bile acids can exert vasoactive effects and induce splanchnic vasodilation through stimulation of the G protein-coupled bile acid receptor one and can contribute to the development of portal hypertension, which further aggravates the translocation of components of the intestinal flora components translocation (Thomas et al., 1991; Fryer et al., 2014). In addition, bile acids directly alter the gut barrier function by downregulating the nuclear receptor farnesoid-X-receptor (FXR) (Sorribas et al., 2019). Furthermore, bile acids can stimulate the generation of inflammatory cytokines directly in hepatocytes during cholestasis by activating inflammatory pathways, involved in the progress of ACLF (Allen et al., 2011; Horvatits et al., 2017).
Short-chain fatty acids pathway
Short-chain fatty acids (SCFAs), gut microbiota-derived metabolites that participate in maintaining the integrity of intestinal mucosal barrier and host’s immune response, are markedly reduced in advanced stages of liver disease, while decreased SCFAs are inversely correlated with the severity of portal hypertension, systemic inflammation, and the prevalence of decompensating events (Juanola et al., 2019). Furthermore, reduced SCFAs can affect energy metabolism in decompensated cirrhosis because fewer SCFAs are transformed into acetyl coenzyme A to produce ATP (Crawford et al., 2009). The microbial metabolites of phenylalanine and tyrosine, which engage the aryl hydrocarbon receptor (AhR) and promote interleukin (IL)-22 secretion, and contribute to local immunization, were reported to be reduced in patients who developed ACLF, which manifested a relative immunodeficiency (Gao et al., 2018; Bajaj et al., 2020).
Biomarkers associated with the development of ACLF based on metabonomics
Recent investigations have determined the alteration of metabolites in patients with ACLF and identified several fingerprints shaped by various metabolites in ACLF (Table 1). Herein, we summarize various key metabolites which are identified to be robust biomarkers for ACLF development (Figure 2).
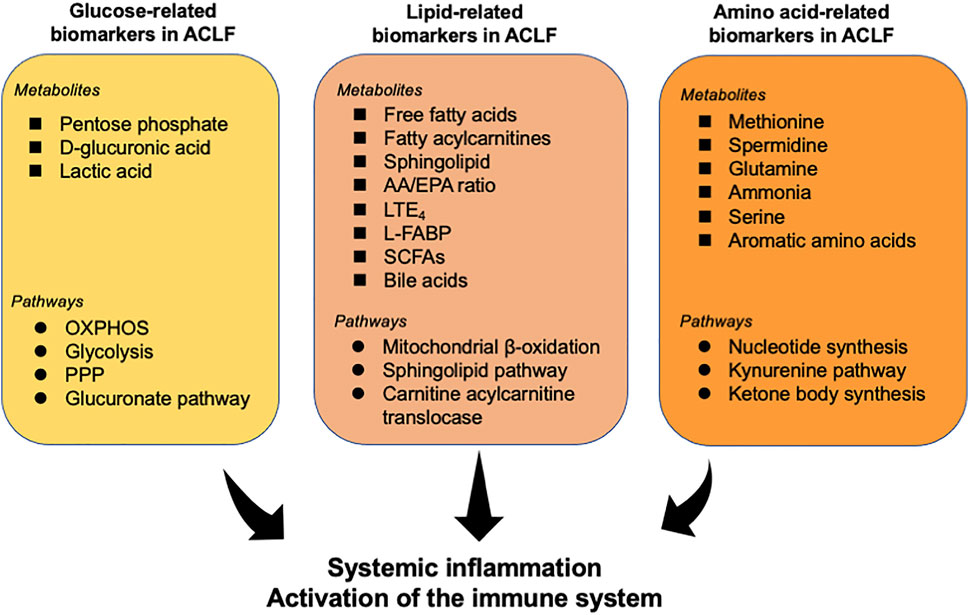
FIGURE 2. Overview of metabolites and related pathways which have been identified to be robust biomarkers for ACLF. Various metabolites and pathways associated with glycometabolism, lipid metabolism, and amino acid metabolism have been determined to be powerful indicators for ACLF prognosis. These metabolites may be a trigger for the activation of the immune system and systemic inflammation, contributing to the progress of ACLF. AA, arachidonic acid; EPA, eicosapentaenoic acid; L-FABP, liver fatty acid binding protein; LTE4, leukotriene E4; OXPHOS, oxidative phosphorylation; PPP, pentose phosphate pathway; Short-chain fatty acids (SCFAs).
Biomarkers related to glucose metabolism
In the context of systemic inflammation, ACLF is characterized by a disturbance of energy metabolism. Moreau et al. (Moreau et al., 2020) identified the alterations of serum metabolites associated with glucose metabolism in patients with ACLF through serum metabolomics. In particular, pentose phosphate, as an intermediate of the pentose phosphate pathway, was identified to increase 362-fold in ACLF (which was the strongest alteration in this contrast), 64-fold in acute decompensation, and 16-fold in compensated cirrhosis (CC), compared to healthy subjects (HS), in accordance with previous discoveries in immunometabolism-associated inflammation (O'Neill and Pearce, 2016). The level of d-glucuronic acid increases in ACLF, indicating the increased activity of the glucuronate pathway, which can be connected to the pentose phosphate pathway. Additionally, 4-hydroxy-3-methoxyphenylglycol sulfate, a metabolic product of norepinephrine that can be a stimulant of glycolysis, and blood levels of lactic acid, the key product of glycolysis, also increase in patients with ACLF (Moreau et al., 2020).
Biomarkers related to the metabolism of amino acids
Skeletal muscle is the largest reservoir of amino acids in the body (Davis and Fiorotto, 2009). High levels of amino acids and peptides likely reflect increased proteolysis and have been associated with a high catabolic status characteristic of inflammatory conditions and sepsis (Hotamisligil, 2017). Similarly, intense proteolysis acids (e.g., tyrosine, asparagine, lysine, methionine, and isoleucine) were increased in serum from patients with ACLF (Moreau et al., 2020; Pose et al., 2021).
Amino acid-related metabolites were associated with intense systemic inflammation and oxidative stress in ACLF and were correlated with organ system failures and severity (Zaccherini et al., 2021). A cluster including 38 metabolites with a remarkably significant association with ACLF was identified via metabonomics analysis combined with bioinformatics analysis and several metabolites of the cluster were amino acids or amino acid derivatives (e.g., saccharopine and N6, N6, N6-trimethyl-l-lysine, the intermediary metabolites in the degradation of lysine) (Moreau et al., 2020). Furthermore, the metabolism of aromatic amino acids, arginine, and benzoates has also been identified to be correlated with the development of ACLF and death (Bajaj et al., 2020).
Biomarkers related to lipid metabolism
In addition to the alternation of the sphingolipid pathway above-mentioned, bioinformatics analysis of untargeted lipidomics indicated that sphingolipids are the best markers to distinguish acute decompensation from healthy controls and that reduced sphingolipids are associated with the severity of the disease (Clària et al., 2021). A previous investigation revealed that lower serum levels of sphingolipids are associated with worse survival in patients with alcoholic cirrhosis and that impaired sphingolipid biosynthesis is associated with undernutrition in hospitalized patients with AD (Grammatikos et al., 2015; Rachakonda et al., 2019). Furthermore, serum extracellular vesicles loaded with sphingolipids have been shown to predict death in decompensated cirrhosis (Sehrawat et al., 2021). Cholesteryl ester (CE) can accumulate in the adrenal cortex and can be used for the biosynthesis of steroid hormones (Kraemer et al., 2013). Therefore, reduced CE levels could be related to adrenal failure in patients with ACLF (Acevedo et al., 2013). López-Vicario et al. (López-Vicario et al., 2020) uncovered that patients with ACLF had an increased ratio between arachidonic acid and eicosapentaenoic acid, which is an indicator of systemic inflammation (Simopoulos, 2002). Notably, two lipid molecules leukotriene E4 (LTE4) and 12-hydroxyheptadecatrienoic acid (HHT) were identified to distinguish ACLF from AD. Additionally, it has been proved elevated urinary liver fatty acid binding protein (L-FABP) may suggest the activation of inflammatory pathways through lipid mediators and is correlated with multiorgan dysfunction and serves as a promising biomarker to predict mortality in patients with ACLF (Juanola et al., 2022).
Biomarkers related to intestinal microbial metabolites
The gut and liver interact through the gut-liver axis and tight bidirectional links through the biliary tract, portal vein, and systemic circulation (Tripathi et al., 2018). Translocation of components or metabolites of the gut microbiota—facilitated by intestinal dysbiosis, increased intestinal permeability and portal hypertension—is a key driver of the development and progression of ACLF (Ridlon et al., 2015; Trebicka et al., 2021). Gut microbiota-associated metabolites can be detected in the serum by blood metabonomics and there are significant changes in the level and composition of metabolites produced by the intestinal microbiota in patients with ACLF (Bajaj et al., 2020; Moreau et al., 2020). The levels of metabolites involved in choline metabolism and most aromatic amino acids decreased in ACLF, while the levels of metabolites associated with tyrosine, secondary bile acids, and benzoate are increased. Of these, 4-methoxyphenol sulfate, which belongs to tyrosine metabolism, is the most correlated with ACLF and is also associated with inpatient death. However, aromatic amino acid metabolites were higher in those who died compared to survivors (Bajaj et al., 2020). Gut microbial metabolites may be valuable biomarkers to identify patients at risk of decompensation and ACLF. However, these signatures are not consistent, and more research is needed.
Metabolites as potential therapeutic targets for ACLF
With the advent and evolution of metabolomics technologies, the discovery of active metabolites that can change cell physiology has grown rapidly. The active metabolites can regulate phenotypes and pathophysiological processes via interaction with the key proteins or enzymes (Rinschen et al., 2019). Recent investigations have indicated the potential therapeutic benefits of exogenous supplementation of several active metabolites in different liver diseases (Masubuchi et al., 2011; Cai et al., 2016). Mitochondrial dysfunction mediates metabolic disorders in leukocytes in patients with ACLF and may be involved in the progression of organ failure (Zhang et al., 2021). Furthermore, two break points of the TCA cycle in PBMCs were identified: one is the higher utilization of α-KG inducing a reduced utilization of upstream intermediates, including isocitrate, cis-aconitate and citrate; and the other is the higher utilization of fumarate leading to a lower utilization of succinate (Zhang et al., 2021). Therefore, supplementation of the two metabolites may replenish the impaired TCA cycle and attenuate the development of ACLF.
Conclusion and future directions
This review described the role of metabolomics in investigations aimed at determining biomarkers correlated with the pathophysiology of ACLF. Regarding the high short-term mortality of ACLF, metabolomics should be earlier performed in patients with decompensated cirrhosis, especially in patients presenting with acute variceal bleeding, ascites, or hepatic encephalopathy, to identify individuals at high risk of developing ACLF via target metabolomics, and to enable early preventive interventions. Metabolomics approaches have provided insightful evidence on altered metabolites and metabolic pathways in ACLF. Overall, studies have shown variations in glucose metabolism, amino acid metabolism, several aspects of lipid metabolism, and intestinal microbial metabolism; and identified the association between serum metabolites and the pathogenesis of ACLF. Furthermore, future studies should validate the biomarkers and metabolic pathways reported in the progression of ACLF and explore potential therapeutic targets associated with metabolites for ACLF. It is promising that metabolomics can provide clinical tools for precision therapy for ACLF in the future.
Author contributions
All authors listed have made a substantial, direct, and intellectual contribution to the work and approved it for publication.
Funding
The project is supported by 1·3·5 project for disciplines of excellence—Clinical Research Incubation Project, West China Hospital, Sichuan University (2019HXFH055); Project for Science and Technology Department of Sichuan Province (2020YFH0089).
Conflict of interest
The authors declare that the research was conducted in the absence of any commercial or financial relationships that could be construed as a potential conflict of interest.
Publisher’s note
All claims expressed in this article are solely those of the authors and do not necessarily represent those of their affiliated organizations, or those of the publisher, the editors and the reviewers. Any product that may be evaluated in this article, or claim that may be made by its manufacturer, is not guaranteed or endorsed by the publisher.
Abbreviations
ACLF, acute-on-chronic liver failure; PAMPs, pathogen-associated molecular patterns; DAMPs, damage-associated molecular patterns.
References
Acevedo, J., Fernández, J., Prado, V., Silva, A., Castro, M., Pavesi, M., et al. (2013). Relative adrenal insufficiency in decompensated cirrhosis: Relationship to short-term risk of severe sepsis, hepatorenal syndrome, and death. Hepatology 58, 1757–1765. doi:10.1002/hep.26535
Aizawa, S., Brar, G., and Tsukamoto, H. (2020). Cell death and liver disease. Gut Liver 14, 20–29. doi:10.5009/gnl18486
Allen, K., Jaeschke, H., and Copple, B. L. (2011). Bile acids induce inflammatory genes in hepatocytes: a novel mechanism of inflammation during obstructive cholestasis. Am. J. Pathol. 178, 175–186. doi:10.1016/j.ajpath.2010.11.026
Arroyo, V., Moreau, R., and Jalan, R. (2020). Acute-on-Chronic liver failure. N. Engl. J. Med. 382, 2137–2145. doi:10.1056/NEJMra1914900
Bajaj, J. S., Reddy, K. R., O'Leary, J. G., Vargas, H. E., Lai, J. C., Kamath, P. S., et al. (2020). Serum levels of metabolites produced by intestinal microbes and lipid moieties independently associated with acute-on-chronic liver failure and death in patients with cirrhosis. Gastroenterology 159, 1715–1730. doi:10.1053/j.gastro.2020.07.019
Baker, D. H. (2009). Advances in protein-amino acid nutrition of poultry. Amino Acids 37, 29–41. doi:10.1007/s00726-008-0198-3
Bujak, R., Struck-Lewicka, W., Markuszewski, M. J., and Kaliszan, R. (2015). Metabolomics for laboratory diagnostics. J. Pharm. Biomed. Anal. 113, 108–120. doi:10.1016/j.jpba.2014.12.017
Cai, X., Bao, L., Wang, N., Xu, M., Mao, R., and Li, Y. (2016). Dietary nucleotides supplementation and liver injury in alcohol-treated rats: A metabolomics investigation. Molecules 21, 435. doi:10.3390/molecules21040435
Cervenka, I., Agudelo, L. Z., and Ruas, J. L. (2017). Kynurenines: Tryptophan's metabolites in exercise, inflammation, and mental health. Science 357, eaaf9794. doi:10.1126/science.aaf9794
Chan, D. C. (2006). Mitochondria: dynamic organelles in disease, aging, and development. Cell 125, 1241–1252. doi:10.1016/j.cell.2006.06.010
Clària, J., Curto, A., Moreau, R., Colsch, B., López-Vicario, C., Lozano, J. J., et al. (2021). Untargeted lipidomics uncovers lipid signatures that distinguish severe from moderate forms of acutely decompensated cirrhosis. J. Hepatol. 75, 1116–1127. doi:10.1016/j.jhep.2021.06.043
Clària, J., Moreau, R., Fenaille, F., Amorós, A., Junot, C., Gronbaek, H., et al. (2019). Orchestration of tryptophan-kynurenine pathway, acute decompensation, and acute-on-chronic liver failure in cirrhosis. Hepatology 69, 1686–1701. doi:10.1002/hep.30363
Clària, J., Stauber, R. E., Coenraad, M. J., Moreau, R., Jalan, R., Pavesi, M., et al. (2016). Systemic inflammation in decompensated cirrhosis: Characterization and role in acute-on-chronic liver failure. Hepatology 64, 1249–1264. doi:10.1002/hep.28740
Crawford, P. A., Crowley, J. R., Sambandam, N., Muegge, B. D., Costello, E. K., Hamady, M., et al. (2009). Regulation of myocardial ketone body metabolism by the gut microbiota during nutrient deprivation. Proc. Natl. Acad. Sci. U. S. A. 106, 11276–11281. doi:10.1073/pnas.0902366106
Dasarathy, S., Mookerjee, R. P., Rackayova, V., Rangroo Thrane, V., Vairappan, B., Ott, P., et al. (2017). Ammonia toxicity: from head to toe? Metab. Brain Dis. 32, 529–538. doi:10.1007/s11011-016-9938-3
Davis, T. A., and Fiorotto, M. L. (2009). Regulation of muscle growth in neonates. Curr. Opin. Clin. Nutr. Metab. Care 12, 78–85. doi:10.1097/MCO.0b013e32831cef9f
Djoumbou Feunang, Y., Eisner, R., Knox, C., Chepelev, L., Hastings, J., Owen, G., et al. (2016). ClassyFire: automated chemical classification with a comprehensive, computable taxonomy. J. Cheminform. 8, 61. doi:10.1186/s13321-016-0174-y
Ertunc, M. E., and Hotamisligil, G. S. (2016). Lipid signaling and lipotoxicity in metaflammation: indications for metabolic disease pathogenesis and treatment. J. Lipid Res. 57, 2099–2114. doi:10.1194/jlr.R066514
Fernández, J., Acevedo, J., Wiest, R., Gustot, T., Amoros, A., Deulofeu, C., et al. (2018). Bacterial and fungal infections in acute-on-chronic liver failure: prevalence, characteristics and impact on prognosis. Gut 67, 1870–1880. doi:10.1136/gutjnl-2017-314240
Fryer, R. M., Ng, K. J., Nodop Mazurek, S. G., Patnaude, L., Skow, D. J., Muthukumarana, A., et al. (2014). G protein-coupled bile acid receptor 1 stimulation mediates arterial vasodilation through a K(Ca)1.1 (BK(Ca))-dependent mechanism. J. Pharmacol. Exp. Ther. 348, 421–431. doi:10.1124/jpet.113.210005
Ganeshan, K., Nikkanen, J., Man, K., Leong, Y. A., Sogawa, Y., Maschek, J. A., et al. (2019). Energetic trade-offs and hypometabolic states promote disease tolerance. Cell 177, 399–413. doi:10.1016/j.cell.2019.01.050
Gao, J., Xu, K., Liu, H., Liu, G., Bai, M., Peng, C., et al. (2018). Impact of the gut microbiota on intestinal immunity mediated by tryptophan metabolism. Front. Cell. Infect. Microbiol. 8, 13. doi:10.3389/fcimb.2018.00013
Gowda, G. A., Zhang, S., Gu, H., Asiago, V., Shanaiah, N., and Raftery, D. (2008). Metabolomics-based methods for early disease diagnostics. Expert Rev. Mol. Diagn. 8, 617–633. doi:10.1586/14737159.8.5.617
Grammatikos, G., Ferreiròs, N., Waidmann, O., Bon, D., Schroeter, S., Koch, A., et al. (2015). Serum sphingolipid variations associate with hepatic decompensation and survival in patients with cirrhosis. PLoS One 10, e0138130. doi:10.1371/journal.pone.0138130
Hannun, Y. A., and Obeid, L. M. (2018). Sphingolipids and their metabolism in physiology and disease. Nat. Rev. Mol. Cell Biol. 19, 175–191. doi:10.1038/nrm.2017.107
Horvatits, T., Drolz, A., Roedl, K., Rutter, K., Ferlitsch, A., Fauler, G., et al. (2017). Serum bile acids as marker for acute decompensation and acute-on-chronic liver failure in patients with non-cholestatic cirrhosis. Liver Int. 37, 224–231. doi:10.1111/liv.13201
Hotamisligil, G. S. (2017). Inflammation, metaflammation and immunometabolic disorders. Nature 542, 177–185. doi:10.1038/nature21363
Jacob, M., Lopata, A. L., Dasouki, M., and Abdel Rahman, A. M. (2019). Metabolomics toward personalized medicine. Mass Spectrom. Rev. 38, 221–238. doi:10.1002/mas.21548
Jalan, R., De Chiara, F., Balasubramaniyan, V., Andreola, F., Khetan, V., Malago, M., et al. (2016). Ammonia produces pathological changes in human hepatic stellate cells and is a target for therapy of portal hypertension. J. Hepatol. 64, 823–833. doi:10.1016/j.jhep.2015.11.019
Jang, C., Chen, L., and Rabinowitz, J. D. (2018). Metabolomics and isotope tracing. Cell 173, 822–837. doi:10.1016/j.cell.2018.03.055
Johnson, C. H., Ivanisevic, J., and Siuzdak, G. (2016). Metabolomics: beyond biomarkers and towards mechanisms. Nat. Rev. Mol. Cell Biol. 17, 451–459. doi:10.1038/nrm.2016.25
Juanola, A., Graupera, I., Elia, C., Piano, S., Solé, C., Carol, M., et al. (2022). Urinary L-FABP is a promising prognostic biomarker of ACLF and mortality in patients with decompensated cirrhosis. J. Hepatol. 76, 107–114. doi:10.1016/j.jhep.2021.08.031
Juanola, O., Ferrusquía-Acosta, J., García-Villalba, R., Zapater, P., Magaz, M., Marín, A., et al. (2019). Circulating levels of butyrate are inversely related to portal hypertension, endotoxemia, and systemic inflammation in patients with cirrhosis. Faseb J. 33, 11595–11605. doi:10.1096/fj.201901327R
Kraemer, F. B., Khor, V. K., Shen, W. J., and Azhar, S. (2013). Cholesterol ester droplets and steroidogenesis. Mol. Cell. Endocrinol. 371, 15–19. doi:10.1016/j.mce.2012.10.012
Krähenbühl, S., and Reichen, J. (1988). Canalicular bile flow and bile salt secretion are maintained in rats with liver cirrhosis. Further evidence for the intact cell hypothesis. J. Hepatol. 7, 63–71. doi:10.1016/s0168-8278(88)80507-5
Li, J., Liang, X., Jiang, J., Yang, L., Xin, J., Shi, D., et al. (2021). PBMC transcriptomics identifies immune-metabolism disorder during the development of HBV-ACLF. Gut 71, 163–175. doi:10.1136/gutjnl-2020-323395
López-Vicario, C., Checa, A., Urdangarin, A., Aguilar, F., Alcaraz-Quiles, J., Caraceni, P., et al. (2020). Targeted lipidomics reveals extensive changes in circulating lipid mediators in patients with acutely decompensated cirrhosis. J. Hepatol. 73, 817–828. doi:10.1016/j.jhep.2020.03.046
Mamas, M., Dunn, W. B., Neyses, L., and Goodacre, R. (2011). The role of metabolites and metabolomics in clinically applicable biomarkers of disease. Arch. Toxicol. 85, 5–17. doi:10.1007/s00204-010-0609-6
Masubuchi, Y., Nakayama, J., and Sadakata, Y. (2011). Protective effects of exogenous glutathione and related thiol compounds against drug-induced liver injury. Biol. Pharm. Bull. 34, 366–370. doi:10.1248/bpb.34.366
McPhail, M. J. W., Shawcross, D. L., Lewis, M. R., Coltart, I., Want, E. J., Antoniades, C. G., et al. (2016). Multivariate metabotyping of plasma predicts survival in patients with decompensated cirrhosis. J. Hepatol. 64, 1058–1067. doi:10.1016/j.jhep.2016.01.003
Mills, E. L., Kelly, B., and O'Neill, L. A. J. (2017). Mitochondria are the powerhouses of immunity. Nat. Immunol. 18, 488–498. doi:10.1038/ni.3704
Moreau, R., Clària, J., Aguilar, F., Fenaille, F., Lozano, J. J., Junot, C., et al. (2020). Blood metabolomics uncovers inflammation-associated mitochondrial dysfunction as a potential mechanism underlying ACLF. J. Hepatol. 72, 688–701. doi:10.1016/j.jhep.2019.11.009
Moreau, R., Jalan, R., Gines, P., Pavesi, M., Angeli, P., Cordoba, J., et al. (2013). Acute-on-chronic liver failure is a distinct syndrome that develops in patients with acute decompensation of cirrhosis. Gastroenterology 144, 1426–1429. doi:10.1053/j.gastro.2013.02.042
Newgard, C. B. (2017). Metabolomics and metabolic diseases: Where do we stand? Cell Metab. 25, 43–56. doi:10.1016/j.cmet.2016.09.018
Nishikawa, T., Bellance, N., Damm, A., Bing, H., Zhu, Z., Handa, K., et al. (2014). A switch in the source of ATP production and a loss in capacity to perform glycolysis are hallmarks of hepatocyte failure in advance liver disease. J. Hepatol. 60, 1203–1211. doi:10.1016/j.jhep.2014.02.014
Nordlie, R. C., Foster, J. D., and Lange, A. J. (1999). Regulation of glucose production by the liver. Annu. Rev. Nutr. 19, 379–406. doi:10.1146/annurev.nutr.19.1.379
O'Neill, L. A., and Pearce, E. J. (2016). Immunometabolism governs dendritic cell and macrophage function. J. Exp. Med. 213, 15–23. doi:10.1084/jem.20151570
Poh, Z., and Chang, P. E. (2012). A current review of the diagnostic and treatment strategies of hepatic encephalopathy. Int. J. Hepatol. 2012, 480309. doi:10.1155/2012/480309
Pose, E., Solà, E., Lozano, J. J., Juanola, A., Sidorova, J., Zaccherini, G., et al. (2021). Treatment with simvastatin and rifaximin restores the plasma metabolomic profile in patients with decompensated cirrhosis. Hepatol. Commun. 6, 1100–1112. doi:10.1002/hep4.1881
Rachakonda, V., Argemi, J., Borhani, A. A., Bataller, R., Tevar, A., and Behari, J. (2019). Reduced serum sphingolipids constitute a molecular signature of malnutrition in hospitalized patients with decompensated cirrhosis. Clin. Transl. Gastroenterol. 10, e00013. doi:10.14309/ctg.0000000000000013
Reed, L. J. (1981). Regulation of mammalian pyruvate dehydrogenase complex by a phosphorylation-dephosphorylation cycle. Curr. Top. Cell. Regul. 18, 95–106. doi:10.1016/b978-0-12-152818-8.50012-8
Ridlon, J. M., Kang, D. J., Hylemon, P. B., and Bajaj, J. S. (2015). Gut microbiota, cirrhosis, and alcohol regulate bile acid metabolism in the gut. Dig. Dis. 33, 338–345. doi:10.1159/000371678
Rinschen, M. M., Ivanisevic, J., Giera, M., and Siuzdak, G. (2019). Identification of bioactive metabolites using activity metabolomics. Nat. Rev. Mol. Cell Biol. 20, 353–367. doi:10.1038/s41580-019-0108-4
Rose, C., Kresse, W., and Kettenmann, H. (2005). Acute insult of ammonia leads to calcium-dependent glutamate release from cultured astrocytes, an effect of pH. J. Biol. Chem. 280, 20937–20944. doi:10.1074/jbc.M412448200
Sanderson, S. M., Gao, X., Dai, Z., and Locasale, J. W. (2019). Methionine metabolism in health and cancer: a nexus of diet and precision medicine. Nat. Rev. Cancer 19, 625–637. doi:10.1038/s41568-019-0187-8
Sarin, S. K., and Choudhury, A. (2016). Acute-on-chronic liver failure: terminology, mechanisms and management. Nat. Rev. Gastroenterol. Hepatol. 13, 131–149. doi:10.1038/nrgastro.2015.219
Sarin, S. K., Choudhury, A., Sharma, M. K., Maiwall, R., Al Mahtab, M., Rahman, S., et al. (2019). Acute-on-chronic liver failure: consensus recommendations of the asian pacific association for the study of the liver (APASL): an update. Hepatol. Int. 13, 353–390. doi:10.1007/s12072-019-09946-3
Sawhney, R., Holland-Fischer, P., Rosselli, M., Mookerjee, R. P., Agarwal, B., and Jalan, R. (2016). Role of ammonia, inflammation, and cerebral oxygenation in brain dysfunction of acute-on-chronic liver failure patients. Liver Transpl. 22, 732–742. doi:10.1002/lt.24443
Sehrawat, T. S., Arab, J. P., Liu, M., Amrollahi, P., Wan, M., Fan, J., et al. (2021). Circulating extracellular vesicles carrying sphingolipid cargo for the diagnosis and dynamic risk profiling of alcoholic hepatitis. Hepatology 73, 571–585. doi:10.1002/hep.31256
Shalimar, , Sheikh, M. F., Mookerjee, R. P., Agarwal, B., Acharya, S. K., and Jalan, R. (2019). Prognostic role of ammonia in patients with cirrhosis. Hepatology 70, 982–994. doi:10.1002/hep.30534
Shawcross, D. L., Wright, G. A., Stadlbauer, V., Hodges, S. J., Davies, N. A., Wheeler-Jones, C., et al. (2008). Ammonia impairs neutrophil phagocytic function in liver disease. Hepatology 48, 1202–1212. doi:10.1002/hep.22474
Shi, Y., Yang, Y., Hu, Y., Wu, W., Yang, Q., Zheng, M., et al. (2015). Acute-on-chronic liver failure precipitated by hepatic injury is distinct from that precipitated by extrahepatic insults. Hepatology 62, 232–242. doi:10.1002/hep.27795
Simopoulos, A. P. (2002). The importance of the ratio of omega-6/omega-3 essential fatty acids. Biomed. Pharmacother. 56, 365–379. doi:10.1016/s0753-3322(02)00253-6
Sorribas, M., Jakob, M. O., Yilmaz, B., Li, H., Stutz, D., Noser, Y., et al. (2019). FXR modulates the gut-vascular barrier by regulating the entry sites for bacterial translocation in experimental cirrhosis. J. Hepatol. 71, 1126–1140. doi:10.1016/j.jhep.2019.06.017
Thomas, S. H., Joh, T., and Benoit, J. N. (1991). Role of bile acids in splanchnic hemodynamic response to chronic portal hypertension. Dig. Dis. Sci. 36, 1243–1248. doi:10.1007/bf01307516
Trebicka, J., Amoros, A., Pitarch, C., Titos, E., Alcaraz-Quiles, J., Schierwagen, R., et al. (2019). Addressing profiles of systemic inflammation across the different clinical phenotypes of acutely decompensated cirrhosis. Front. Immunol. 10, 476. doi:10.3389/fimmu.2019.00476
Trebicka, J., Bork, P., Krag, A., and Arumugam, M. (2021). Utilizing the gut microbiome in decompensated cirrhosis and acute-on-chronic liver failure. Nat. Rev. Gastroenterol. Hepatol. 18, 167–180. doi:10.1038/s41575-020-00376-3
Trebicka, J., Fernandez, J., Papp, M., Caraceni, P., Laleman, W., Gambino, C., et al. (2020). The PREDICT study uncovers three clinical courses of acutely decompensated cirrhosis that have distinct pathophysiology. J. Hepatol. 73, 842–854. doi:10.1016/j.jhep.2020.06.013
Tripathi, A., Debelius, J., Brenner, D. A., Karin, M., Loomba, R., Schnabl, B., et al. (2018). The gut-liver axis and the intersection with the microbiome. Nat. Rev. Gastroenterol. Hepatol. 15, 397–411. doi:10.1038/s41575-018-0011-z
Van Wyngene, L., Vandewalle, J., and Libert, C. (2018). Reprogramming of basic metabolic pathways in microbial sepsis: therapeutic targets at last? EMBO Mol. Med. 10, e8712. doi:10.15252/emmm.201708712
Wang, A., Luan, H. H., and Medzhitov, R. (2019). An evolutionary perspective on immunometabolism. Science 363, eaar3932. doi:10.1126/science.aar3932
Wishart, D. S., Feunang, Y. D., Marcu, A., Guo, A. C., Liang, K., Vázquez-Fresno, R., et al. (2018). HMDB 4.0: the human metabolome database for 2018. Nucleic Acids Res. 46, D608–d617. doi:10.1093/nar/gkx1089
Wright, G., Noiret, L., Olde Damink, S. W., and Jalan, R. (2011). Interorgan ammonia metabolism in liver failure: the basis of current and future therapies. Liver Int. 31, 163–175. doi:10.1111/j.1478-3231.2010.02302.x
Zaccherini, G., Aguilar, F., Caraceni, P., Clària, J., Lozano, J. J., Fenaille, F., et al. (2021). Assessing the role of amino acids in systemic inflammation and organ failure in patients with ACLF. J. Hepatol. 74, 1117–1131. doi:10.1016/j.jhep.2020.11.035
Keywords: ACLF (acute-on-chronic liver failure), OxPhos, systemic inflammation, metabolomics, metabolites
Citation: Liu G, Wang X, Fan X and Luo X (2022) Metabolomics profiles in acute-on-chronic liver failure: Unveiling pathogenesis and predicting progression. Front. Pharmacol. 13:953297. doi: 10.3389/fphar.2022.953297
Received: 26 May 2022; Accepted: 28 July 2022;
Published: 19 August 2022.
Edited by:
Pedro Miguel Rodrigues, Biodonostia Health Research Institute (IIS Biodonostia), SpainReviewed by:
André Simão, Research Institute for Medicines (iMed.ULisboa), PortugalElisa Pinto, University of Padua, Italy
Copyright © 2022 Liu, Wang, Fan and Luo. This is an open-access article distributed under the terms of the Creative Commons Attribution License (CC BY). The use, distribution or reproduction in other forums is permitted, provided the original author(s) and the copyright owner(s) are credited and that the original publication in this journal is cited, in accordance with accepted academic practice. No use, distribution or reproduction is permitted which does not comply with these terms.
*Correspondence: Xuefeng Luo, bHVvX3h1ZWZlbmdAd2Noc2N1LmNu
†These authors have contributed equally to this work and share first authorship