- 1School of Materials Science and Engineering, Hefei University of Technology, Hefei, Anhui, China
- 2Anhui Renovo Pharmaceutical Co., Ltd., Hefei, Anhui, China
- 3Department of Endocrinology, The First Affiliated Hospital of USTC, Division of Life Sciences and Medicine, University of Science and Technology of China, Hefei, Anhui, China
The morbidity and mortality of atherosclerotic cardiovascular disease (ASCVD) is increasing year by year. Cortex Moutan is a traditional Chinese medicinal herb that has been widely used for thousands of years to treat a wide variety of diseases in Eastern countries due to its heat-clearing and detoxifying effects. Paeonol is a bioactive monomer extracted from Cortex Moutan, which has anti-atherosclerotic effects. In this article, we reviewed the pharmacological effects of paeonol against experimental atherosclerosis, as well as its protective effects on vascular endothelial cells, smooth muscle cells, macrophages, platelets, and other important cell types. The pleiotropic effects of paeonol in atherosclerosis suggest that it can be a promising therapeutic agent for atherosclerosis and its complications. Large-scale randomized clinical trials are warranted to elucidate whether paeonol are effective in patients with ASCVD.
1 Introduction
The spectrum of human diseases is gradually changing with the increasing wave of industrialization and urbanization around the world. Epidemiological studies have revealed that, with the aging process of the population, environmental pollution is increasing, alongside the acquisition of bad living habits (such as excessive intake and physical inactivity), all of which contribute to chronic diseases, especially cardiovascular disease, the leading cause of global morbidity and mortality (Clark, 2013). According to World Health Organization (WHO) studies, cardiovascular disease (CVD) is responsible for more than 40% of noncommunicable disease (NCD) mortality worldwide each year (Mendis et al., 2015).
CVD is mainly caused by atherosclerosis, a chronic lipid storage and progressive inflammatory disease of the blood vessels. The development of atherosclerosis commences with endothelial dysfunction due to endothelial cell injury, which gradually leads to the retention and accumulation of LDL and its modified form in the arterial wall. The progression of atherosclerosis leads to the narrowing and hardening of the arterial lumen due to the existence of atherosclerotic plaques (Libby, 2021). The existence of atherosclerotic plaques can be asymptomatic for decades, but sometimes the vulnerable plaques can be destabilized and susceptible to rupture. The infiltration of inflammatory cells and plaque rupture, which constrict the artery lumen, promote several life-threatening diseases in the brain and heart including ischemic stroke and myocardial infarction (Libby, 2021).
Lipid-lowering has been the cornerstone of CVD. For example, statins are the first-line therapy for the therapeutic schedule of atherosclerotic cardiovascular disease (Ferraro et al., 2020). Recently, we have witnessed the emergence of other lipid-lowering therapies, such as ATP citrate lyase (ACLY) inhibitors, etc. (Feng et al., 2020). However, due to the existence of various adverse drug reactions, such as vascular restenosis (Waksman and Iantorno 2018), drug intolerance (Nicola and Ho 2019), and rhabdomyolysis (Ben Salem et al., 2020), the benefits of intensive statin therapy need to be balanced with the potential risks they may cause. To address this dilemma, natural products have been the focus of both academics and the pharmaceutical industry. It is estimated that between 1981 and 2014, about 75% of drugs approved by the drug regulatory authorities came from natural sources or chemical semi-synthetic derivatives, mainly for the treatment of various human diseases, including cancer and cardiovascular disease (Newman and Cragg, 2016). New drug discoveries from natural products have become a new exploratory path for the therapeutics of atherosclerotic cardiovascular disease.
As a widely distributed ornamental and medicinal plant all over the world, Paeonia suffruticosa has aroused great research interest. The main plants with medicinal value are P. suffruticosa Andrew (also known as Cortex Moutan) (Adki and Kulkarni 2020). Cortex Moutan is the root bark of the peony, which was widely used in ancient China for the prevention and therapeutic treatment of various diseases, such as CVD, diabetes, arthritis, and cancer (Wang et al., 2017). It is a traditional Chinese herbal medicine that has been used for thousands of years with a good safety and efficacy profile (Wu et al., 2021). We discussed the various properties and applications of paeonol in the following section.
2 Paeonol
Paeonol is a phenolic compound mainly isolated from the root bark and/or cortex of P. suffruticosa belonging to ranunculus family, the more commonly used name is Cortex Moutan, shown in Figure 1. Cortex Moutan contains a variety of bioactive components, including monoterpene glycosides, flavonoids, gallic acid derivatives, triterpenes, and particularly phenolic compounds. The primary active ingredients of Cortex Moutan include paeonol, paeoniflorin, gallic acid, and 1,2,3,4,6-penta-O-gallic acid-β-D-glucose (Wu et al., 2021). The chemical structure and 3D model of paeonol is shown in Figure 2. The relevant quality inspection standards for paeonol are specified in detail in the first part of the 2020 Edition of the Chinese Pharmacopoeia. (National Pharmacopoeia Commission, 2020), the total ash content of the cortex of medicinal materials shall not exceed 5.0% (General rule 2302), and the moisture shall not exceed 13.0% (General rule 0832 Fourth method). The pharmacopoeia stipulates that the content of paeonol (C9H10O3) should be determined by high performance liquid chromatography (HPLC, General rule 0512), and the content should not be less than 1.2%. At the same time, the pharmacopoeia stipulates that the ethanol extract should be determined by hot immersion method (General Rule 2201), and the content of alcohol-soluble extracts should not be less than 15%. To enhance the stability of paeonol, various derivatives of paeonol has been synthesized (Zhang et al., 2019).
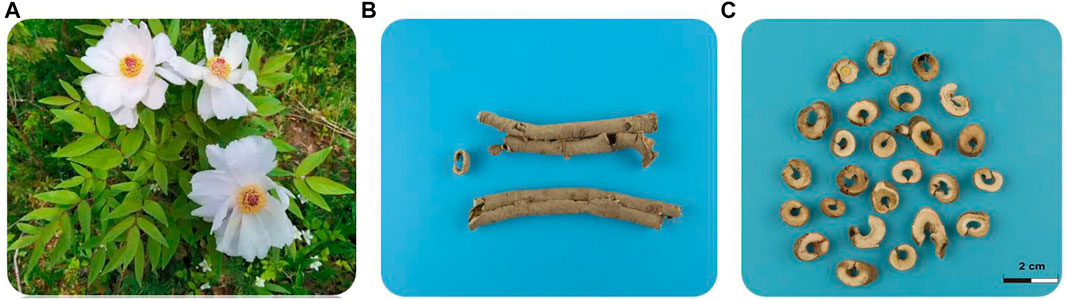
FIGURE 1. Whole plant, root bark and medicinal slices of Paeonia suffruticosa. (A) P. suffruticosa flowers in early May each year and lasts about a week. (B) Cortex Moutan, the root bark of P. suffruticosa, is rich in a variety of medicinal value substances, which can be used for the treatment of a variety of diseases. (C) Medicinal slices of Cortex Moutan.
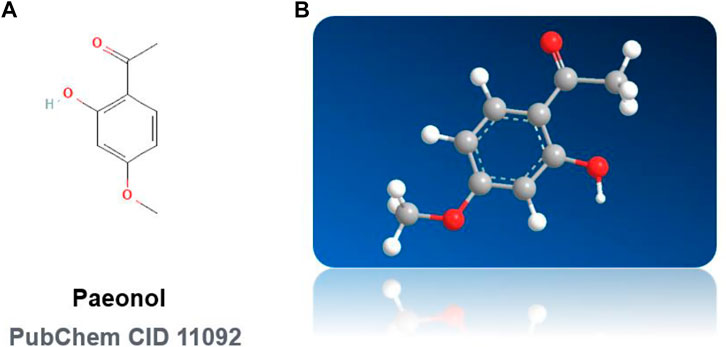
FIGURE 2. Chemical structures of paeonol. (A) Paeonol: 2D Chemical Structural Formula. (B) Paeonol: 3D Chemical Structural Formula NOTE: (Pubchem CID 11092).
2.1 Physiochemical properties of paeonol
Paeonol (1-(2-hydroxy-4-methoxyphenyl) ethanone) has a molecular weight of 166.17 g/mol and a melting point of 52.5°C. The oil-water partition coefficient and solubility of paeonol are different in phosphate buffer solutions with different pH values. Some data show that the oil-water partition coefficient is 461.97–981.17 μg/ml, the solubility is 284.06–598.23 μg/ml, and the effective passive permeability is 23.49 × 10–6 cm/s. Paeonol has an octanol/water partition coefficient of 1.98 (LogP) (Zong et al., 2017).
2.2 Pharmacokinetic properties of paeonol
It is well known that pharmacokinetic monitoring helps to assess the effectiveness and potential toxic effects of a drug and to explore mutual effects between different components. In recent years, some advanced detection methods have been used in the pharmacokinetic study of paeonol. One study has found that after the oral delivery method, paeonol was rapidly absorbed by the digestive system (mainly from the gastrointestinal tract) and rapidly distributed to vital organs and tissues (e.g., heart, brain, kidney, and liver) (Lu et al., 2018). Paeonol has a relatively short half-life (t½) and Tmax, rapid and complete first-pass metabolism, and low water solubility and high volatility, which contribute to its poor bioavailability in the body (Lu et al., 2018). Paeonol is mainly excreted by the urinary system, and its excretion is higher in urine than in feces (Gjertsen et al., 1988). Because paeonol is quickly removed from the human body, its safety can be ensured. In addition, studies have reported that the absorption of paeonol is a first-order process because it is independent of drug concentration and its absorption is better under acidic conditions. The rate of paeonol absorption is related to the rate of perfusion and is maximum in hypertonic solutions (Adki and Kulkarni, 2020).
2.3 Preparation and applications of paeonol
Based on the above-mentioned traditional and modern pharmaceutical-related research, paeonol has a lengthy history of medical applications in Northeast Asia (including China, Japan, and the Korean Peninsula). On the mainland of China, the pharmaceutical preparations of paeonol for clinical treatment date back to the 1970s (the earliest approved dosage form was an injection). At present, the dosage forms of paeonol approved by the China National Medical Products Administration (NMPA) mainly include tablets, ointments, and injections (https://www.nmpa.gov.cn/datasearch/search-result.html). According to the NMPA, the scope of indications for the instructions for paeonol drugs covers multiple fields, for instance, inflammatory or pain-related indications, including fever, headache, neuralgia, myalgia, and rheumatoid arthritis when given orally or intravenously. Topical formulations of paeonol can be used to treat skin diseases or improve skin conditions, including eczema, dermatitis, itchy skin, mosquito bites, allergic rhinitis, and colds. Furthermore, paeonol has pharmacological activities that include neuroprotective, anti-tumor, and cardiovascular protective actions along with significant anti-inflammatory properties (Zhang et al., 2019). Considering the fact that atherosclerotic cardiovascular disease is the leading cause of death worldwide, this paper focuses on evidence gleaned from experimental studies addressing paeonol’s cellular functions and mechanisms in preventing and treating atherosclerosis, including inhibitory effects on lipid accumulation, inflammatory responses, and anti-oxidation.
3 The effect of paeonol on experimental atherosclerosis
3.1 Anti-atherosclerotic effects in rabbits
Mounting evidence has suggested that the inflammatory process plays a critical role in atherosclerosis (Davis, 2005; Hansson, 2005). The inflammatory response in the blood vessel directly leads to an increase in circulating levels of inflammatory cytokines and acute-phase proteins like tumor necrosis factor (TNF)-α, interleukin (IL)-1, and C-reactive protein (CRP), which are reliable biomarkers of systemic inflammation in CVDs (Liuzzo et al., 1994; Lindahl et al., 2000) In 2009, Li et al. (2009) conducted an exploratory study to assess the anti-atherosclerotic functions of paeonol in a rabbit model of atherosclerosis by feeding rabbits a high- calorie diet for 12 weeks. The authors have shown that paeonol administration in rabbits restrains the formation and progression of atherosclerosis by inhibiting the release of inflammatory cytokines (representative inflammatory substances include: CRP, IL-1β, and TNF-α). Paeonol also significantly inhibited the nuclear translocation of nuclear factor kappa B (NF-κB, a master regulator of vascular inflammation and atherosclerosis), and inhibited lipid peroxidation, thereby significantly reduced atherosclerosis development in rabbits (Li et al., 2009).
When circulating blood rheology is abnormal, or when procoagulant substances are upregulated in atherosclerotic lesions, platelets are activated and assembled to scathing endothelium, thus initiating the coagulation cascade that leads to arterial thrombosis, vascular occlusion, and eventual life-threatening acute coronary events (Higashi et al., 2009). In addition, Shi et al. (1988) confirmed the anti-atherosclerotic effects of paeonol in rabbits fed a high-calorie diet for 6 weeks. The results showed that paeonol significantly increases the content of cyclic adenosine monophosphate (cAMP) in platelets. Paeonol improved the hemorheological parameters of rabbits by directly inhibiting platelet aggregation and blood coagulation, thereby inhibiting atherosclerosis in rabbits (Shi et al., 1988).
The above research demonstrates the therapeutic potential of paeonol for white rabbit models of AS. The main anti-atherosclerotic mechanisms of paeonol include: inhibition of platelet aggregation and blood clotting to improve blood rheological parameters; inhibits the transfer of NF-κB to the nucleus, thereby reducing the level of inflammatory factors and inhibiting lipid peroxidation.
3.2 Inhibition of atherosclerosis in quail
Dyslipidemia or high levels of triglyceride (TG) and total cholesterol (TC) are thought to play a critical role in the development of atherosclerosis (Chobanian, 1991). Elevated blood lipids reduce the fluidity of red blood cells, reduce their deformability, and increase the viscosity of whole blood and plasma. By feeding a high-calorie diet for 16 weeks, Dai et al. (1999) established atherosclerosis in a quail model. Oral administration of paeonol improved the lipoprotein profile and inhibited atherosclerosis in quail by significantly lowering the content of TC/TG/low-density lipoproteins (LDL)/very-low-density lipoproteins (VLDL)/apolipoprotein B100 and by upregulating the content of high-density lipoprotein (HDL) in serum. Overall, pheonol administration altered the ratios of HDL/TC, HDL2/HDL3, and apolipoprotein A1/apolipoprotein B100, thereby regulating the lipid profile, metabolism, and transport processes by altering lipoprotein composition. In quail, paeonol therapy decreased plaque area, prevented the production of aortic plaques, and thereby prevented atherosclerosis (Dai et al., 1999).
Studies of quail model of atherosclerosis have revealed the possibility that paeonol can significantly inhibit the level of “bad cholesterol” (represented by LDL cholesterol) in the serum, while increasing the level of “good cholesterol” (represented by HDL cholesterol), thereby changing the composition of lipoproteins, which in turn plays a critical role in regulating lipid metabolism and significantly inhibiting the occurrence and development of atherosclerosis.
3.3 Anti-atherosclerotic effect of paeonol in ApoE−/− mice
Cholesterol accumulation induced by a high-fat diet is one of the common causative factors of lipid metabolism disorders and is also a high risk factor for cardiovascular disease (Sugiyama et al., 2015). ApoE−/− mice are the classical animal model currently used to study the pathomechanisms and potentially new therapies of atherosclerosis. High-fat feeding combined with ApoE deficiency causes significant accumulation of lipids (mostly cholesterol, cholesterol crystals and cholesterol ester) in mice, leading to atherogenesis (Zhang B. et al., 2018). The anti-atherosclerotic effects of paeonol have been explored in ApoE−/− mice in several studies.
One study by Song et al. (2018) found that paeonol can significantly reduce the plaque size in ApoE−/− mice, downregulate the expression of VCAM-1 and matrix metalloproteinase-9 (MMP-9), and inhibit plaque formation. At the same time, paeonol can exert an anti-inflammatory effect and protects against oxidative stress which is involved in the initiation and development of atherosclerosis (Song et al., 2018). Another study reported that paeonol induced autophagy while activating the AMP-activated protein kinase (AMPK)/mechanistic target of rapamycin (mTOR) signaling pathway, i.e., inducing AMPK phosphorylation and reducing mTOR phosphorylation, thereby inhibiting the proliferation of VSMCs, leading to suppressive effects on atherosclerosis (Wu et al., 2018). Consistently, He et al. (2021) have confirmed that paeonol can reduce the area of aortic plaques. The authors found that paeonol can significantly reduce total cholesterol (TC) and triglyceride (TG) level in the serum and liver tissues from ApoE−/− mice; Mechanistic investigations revealed that paeonol increased the content of farnesoid X receptor (FXR) antagonists (such as tauro-α-muricholic acid and tauro-β-muricholic acid, TαMCA and TβMCA), but reduced the content of FXR agonists (such as lithocholic acid, chenodeoxycholic acid, CDCA and LCA). The intestinal FXR- fibroblast growth factor 15 (FGF15) signaling pathway was also inhibited, while cholesterol 7α-hydroxylase (CYP7A1) protein expression in liver tissues was increased. The net effects of paeonol are the increase of total bile acid excretion in feces, thereby reducing cholesterol accumulation, and thus achieving its anti-atherosclerotic effect (He et al., 2021).
Liu et al. (2021) found that paeonol can induce autophagy of VSMCs via initiating the PI3K/Beclin-1 signaling pathway, thereby inhibiting the apoptosis of VSMCs and playing an anti-atherosclerotic role. Atherosclerosis is an epigenetic disease. It remains unknown whether paeonol inhibits atherosclerosis via epigenetic mechanisms. One study by Liu Y. R. et al. (2018) confirmed that paeonol inhibited the activation of STAT3 pathway in ApoE−/− mice by increasing the expression of miR-223. Likewise, Liu et al. (2022) also found that paeonol inhibited the CD40/NF-κB pro-inflammatory pathway by upregulating the expression of miR-145 in ApoE−/−mice, thereby alleviating the inflammatory response within atherosclerotic plaques.
Overall, the above-mentioned atheroprotective effects observed in ApoE−/− mice further extend the atheroprotective effects of paeonol observed in rabbit and quail atherosclerosis models. These preclinical lines of evidence in different animal models of atherosclerosis summarized in (Table 1) reinforced the confidence to develop paeonol as a promising anti-atherosclerotic drug candidate.
4 The protective effect and mechanism of paeonol
Atherosclerosis is a multifaceted vascular disease involving the dysfunction of numerous cell types, such as endothelial cells, vascular smooth muscle cells, macrophages, and platelets. Dysfunction of the above-mentioned cell types leads to endothelial injury, chronic inflammation, lipid metabolism disorders, uncontrolled oxidative stress, hyperplasia, vascular smooth muscle cell proliferation and migration, and abnormal platelet activation (Weber and Noels 2011). Paeonol has protective effects on the above-mentioned cell dysfunction. The effects of paeonol on the function of each cell type implicated in atherosclerosis will be discussed in the sections below (Figure 3).
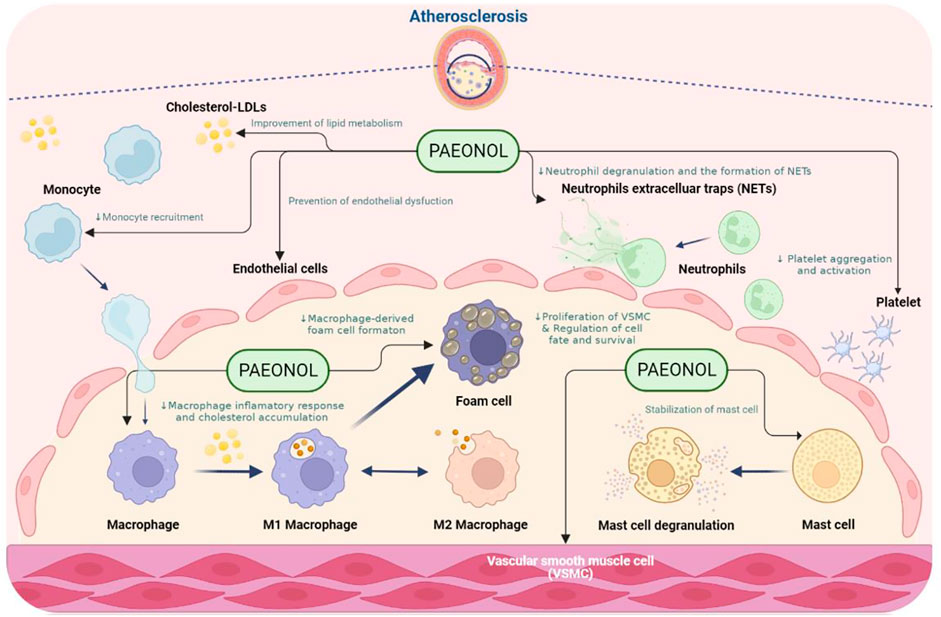
FIGURE 3. The anti-atherosclerotic effects of paeonol. NOTE: Created in BioRender.com.
4.1 Paeonol and endothelial cell function
Vascular endothelial cells constitute the inner cellular lining of arteries, veins, and capillaries, which makes them the largest distribution organs in the human body (Xu et al., 2021). It is also an integral part of the anatomical and physiological functions of every tissue and organ in the body. As the outermost barrier of blood vessels, it plays a very important role in maintaining vascular homeostasis and health (Gimbrone and Garcia-Cardena 2016). When external risk factors (such as hyperlipidemia and low/oscillatory shear stress in blood flow) damage vascular endothelial cells, the function of endothelial cells is impaired, resulting in increased secretion of intercellular adhesion molecule-1 (ICAM-1) and vascular cell adhesion molecule-1 (VCAM-1) along with a battery of other adhesion molecules and/or interleukins. Furthermore, the monocytes in the blood begin to aggregate on the surface of the damaged endothelial surface and further differentiate into macrophages. These cells further secrete monocyte chemoattractant protein-1 (MCP-1), which attracts additional monocytes and leads to the production of foam cells, which is a hallmark of atherosclerosis. The release of inflammatory factors perpetuates the local inflammatory response of blood vessels and is thus involved in the initiation and progression of atherosclerosis (Habas and Shang 2018).
In recent years, emerging studies have demonstrated the anti-atherosclerotic effects of paeonol via protective effects on endothelial cell, including inhibition of endothelial cell damage, inhibition of endothelial cell oxidative stress, inhibition of the hypersecretion of inflammatory factors, improvement in endothelial cell adhesion function, and regulation of endothelial cell survival and cell fate (Figure 4).
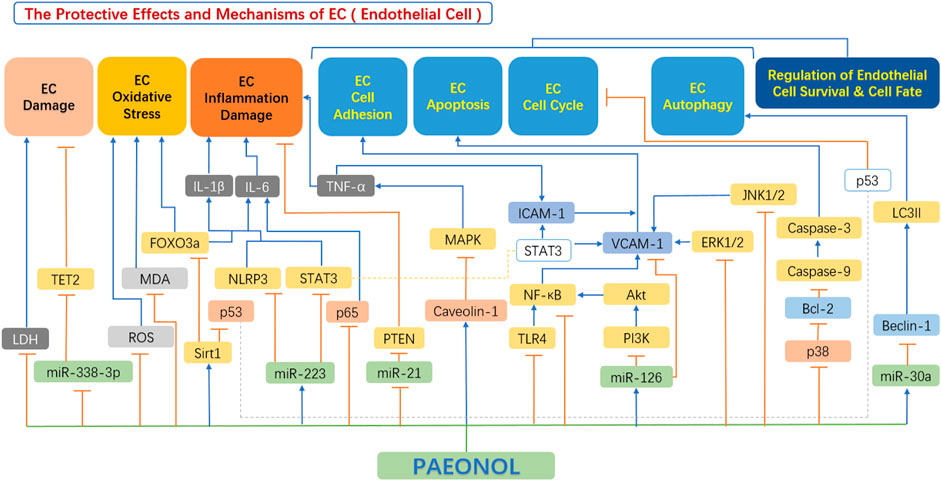
FIGURE 4. The protective effects and mechanisms of paeonol in endothelial cells. Akt, serine-threonine kinase; Bax, Bcl-2-associated X protein; Bcl-2, B-cell lymphoma-2; Beclin-1, the mammalian ortholog of yeast ATG6; ERK1/2, extracellular signal regulated kinase 1/2; FOXO3a, forkhead box transcription factor O3a; ICAM1, intercellular adhesion molecule 1; IL-1β, interleukin 1β; IL-6, interleukin 6; JNK1/2, c-Jun N-terminal kinase 1/2; LC3II, light chain 3 II; LDH, lactate dehydrogenase; MAPK, mitogen-activated protein kinase; MDA, malondialdehyde; miR-126, microRNA-126; miR-21, microRNA-21; miR-223, microRNA-223; miR-30a, microRNA-30a; miR-338-3p, microRNA-338-3p; NF-κB, the nuclear factor kB; NLRP3, NOD-like receptor 3; p38, p38 mitogen-activated protein kinase (MAPK); p53, tumor protein p53 (also known as TP53); PI3K, phosphatidylinositol-4,5-bisphosphate 3-kinase; ROS, reactive oxygen species; Sirt1, sirtuin 1; STAT3, signal transducer and activator of transcription 3; TET2, tet methyl cytosine dioxygenase 2; TLR4, toll-like receptor 4; TNF-α, tumour necrosis factor α; VCAM1, vascular cell adhesion molecule 1.
4.1.1 Inhibition of endothelial cell damage by paeonol
Vascular endothelial cell dysfunction is a primary feature of the early development of atherosclerosis (Adawi et al., 2019). Vascular endothelial cells treated with oxidized low-density lipoprotein (ox-LDL) are often used to simulate the oxidative damage of vascular endothelial cells in atherosclerosis (Zhou Z. et al., 2011). Micro-RNAs (miRNAs) are small (approximately 22 nucleotides) single strand non-coding RNAs that intercept gene expression by inhibiting transcription or accelerating the degradation of target mRNAs (Trionfini and Benigni, 2017). Studies have found an increased level of microRNA-338-3p in patients with atherosclerosis, and its overexpression accelerates ox-LDL-induced cell damage (Yin et al., 2019). Yu et al. (2020) found that paeonol could inhibit ox-LDL-induced miR-338-3p expression, by reducing Tet methylcytosine dioxygenase 2 (TET2) expression, thus regulated the development of vascular endothelial cells, and inhibited endothelial cell damage.
In addition to ox-LDL, lipopolysaccharide (LPS) is another potent pro-inflammatory stimulus that can induce the production of various inflammatory cytokines and is a widely used cellular model of inflammation-induced atherosclerosis in vitro (Mizia-Stec 2006). Hu et al. (2016) observed the protective effect of paeonol on LPS-induced vascular endothelial cells (VEC), co-cultured with smooth muscle cells (SMC). Endothelial cells produce more lactate dehydrogenase (LDH) after being stimulated by LPS, according to the researchers. In contrast, paeonol inhibited LPS-induced production of LDH, thereby significantly improved hyperpermeability and endothelial cell survival.
4.1.2 Inhibition of oxidative stress in endothelial cells by paeonol
Oxidative stress state releases excess free radicals, and intracellular reactive oxygen species (ROS), leading to endothelial dysfunction. The generation of excessive ROS interferes with endothelial nitric oxide synthase (eNOS) function, reduces the bioavailability of the vasodilator nitric oxide (NO), and increases blood pressure (Li Q. et al., 2015). At the same time, the release of ROS further exacerbates the oxidation of LDL, leading to the formation of ox-LDL, which further damages vascular endothelial cells. In the above process, the roles of malondialdehyde (MDA) and superoxide dismutase (SOD) cannot be ignored. MDA is the outcome of lipid peroxidation via the attack of ROS on polyunsaturated fatty acids on biological membranes, and SOD is an important antioxidant enzyme in the body that can defend against oxidative stress (Wei et al., 2016).
The research by Bao et al. (2013) discovered that paeonol significantly inhibited ox-LDL-induced overproduction of ROS in a dose-dependent pattern, reduced endothelial cell damage caused by oxidative stress, and protected against endothelial cell dysfunction. Studies by Kim et al. (2021) also confirmed the protective effect of paeonol in endothelial cells. Tang et al. (2021) further investigated the underlying mechanism behind the protective effect of paeonol, and their research revealed that paeonol enhanced endothelial cell viability and SOD activity. At the same time, paeonol significantly lowered the levels of ROS and MDA, inhibited the expression of acetylated- fork head box O3a (FOXO3a) in high glucose and palmitic acid (HG/HP)-treated human umbilical vein endothelial cells (HUVECs) by activating the SIRT1/FOXO3a/NF-kB pathway, thereby inhibited HG/HP-induced oxidative stress in HUVECs (Tang et al., 2021).
4.1.3 Inhibition of the secretion of inflammatory factors in endothelial cells
Inflammation is an integral part of the pathogenesis of multifarious cardiovascular diseases, including atherosclerosis (Soysal et al., 2020). Numerous studies have shown that paeonol has significant anti-inflammatory properties, which have been confirmed by other systems (Himaya et al., 2012; Zong et al., 2017). Acute events, such as infections and trauma, often lead to the results of acute inflammation. Many cytokines, such as TNF-α and IL-6, are released during this process, and monocytes and macrophages are activated, which activates or inhibits related signaling pathways and promotes monocyte adhesion to endothelial cells (Soysal et al., 2020). Monocytes reach the intima layer of the arterial vessel wall where they differentiate into macrophages, further exacerbate the release of inflammatory and pro-inflammatory substances such as IL-1, IL-18, and other pro-inflammatory cytokines. In the advanced phase of atherosclerosis, the accumulation of apoptotic cells and senescent cells further aggravates the state of inflammation (Libby et al., 2011). Various pro-inflammatory cytokines play central roles in the development of inflammation. Several studies have shown that paeonol restrain the formation and development of atherosclerosis by inhibiting the release of inflammatory cytokines, which is mainly concerned with the regulation of various inflammatory signaling pathways (Huang et al., 2008).
Some miRNAs play important regulatory roles in metabolic disorders and CVD (Zare et al., 2019). Shi et al. (2020) found that paeonol significantly increased the extent of miR-223 in plasma-derived exosomes of hyperlipidemia rats, inhibited the NOD-like receptor 3 (NLRP3) signaling pathway, and reduced the secretion of downstream inflammatory factors, thereby reducing the NLRP3 inflammasome-mediated endothelial cell inflammation. Also, based on the miR-223 target, Liu Y. R. et al. (2018) found that paeonol increased the expression of miR-223 in HUVECs after treatment with THP-1-derived exosomes and ingested exosomes, and inhibited STAT3 expression and activity in apolipoprotein E (ApoE−/−) mice. Through this pathway, the expression of STAT3 and p-STAT3 in HUVECs was reduced, followed by decreased expression of IL-1β and IL-6 (Liu Y. R. et al., 2018).
MicroRNA-21 (miR-21) plays an important role in selective expression in vascular cells, mainly regulating: cell proliferation, invasion, apoptosis, and inflammation (Kumarswamy et al., 2011). Studies have shown that, in the peripheral arterial system, if acute vascular injury occurs, miR-21 is upregulated, and the knockout of miR-21 reduces neointima formation (Ji et al., 2007). Another study conducted by Liu et al. (2014) discovered that paeonol significantly reduced the expression of miR-21 induced by ox-LDL in a dose-dependent pattern by downregulating the expression of the p38 mitogen-activated protein kinase signaling pathway (p38-MAPK), PTEN, and TNF-α release and protected endothelial cells from ox-LDL-induced damage (Liu et al., 2014). Caveolae is a specialized vesicle-like pit microstructure on the endothelial cell membrane, with caveolin as the crucial structural protein. Caveolin can negatively regulate cell signaling pathways by binding and inactivating some important molecules in several intracellular signal transduction pathways (Rodriguez-Feo et al., 2008). According to Liu et al. (2020), paeonol increased the expression of aortic caveolin-1, decreased the expression of p65 protein, inhibited the activation of the NF-B pathway, and significantly reduced serum TNF-α and IL-6 levels. Collectively, paeonol reduced inflammatory responses in vascular endothelium in a rat model of atherosclerosis.
The phosphatidylinositol 3-kinase (PI3K)/serine-threonine kinase (AKT) and NF-κB signaling pathways play a significant role in LPS-induced endothelial cell damage (Sui et al., 2008). PI3K mediates the cellular inflammatory response in endothelial cells. LPS can promote the release of cellular inflammatory factors, enhance the biological activity of PI3K, and promote the activation of AKT to regulate cell function (Chen et al., 2017). Activated NF-κB translocate to the nucleus, thereby regulating the expression of many inflammation-related specific genes such as TNF and IL-6. Studies by Wenjun Hu and Zhen Zhang found that paeonol reduced the induced protein phosphorylation in the PI3K/AKT pathway and inhibited its downstream signaling pathway, downregulated the extent of an inflammatory response, and protected endothelial cells (Hu et al., 2016). Tang et al. (2021) discovered that paeonol activated the SIRT1/FOXO3a/NF-B pathway, significantly reduced TNF-α, IL-1, and IL-6 levels, and inhibited HG/HP-induced HUVEC inflammation in a dose-dependent pattern response, thus protecting endothelial cells.
4.1.4 Improve the adhesion function of endothelial cells
Cell adhesion molecules (CAMs) are membrane receptor glycoproteins that undertake very important tasks in the adhesion of cells to extracellular matrices and endothelial surfaces (Hope and Meredith, 2003). According to their structure and roles, CAMs can be divided into three main families: integrins, selectins, and the immunoglobulin superfamily. In the above classification, E-selectin, ICAM-1, and VCAM-1 play an important role in the chronic inflammatory process, and therefore have momentous relevance in CVD (Vieira et al., 2014). Selectins are binding protein molecules whose primary function is to mediate the interaction between leukocytes and endothelial cells, and subsequently, these cells form strong adhesion and migration across the endothelium. Endothelial cells express E-selectin, which is specifically and intensely stimulated by inflammatory factors like TNF-α and IL-1 and is widely expressed in the vasculature at sites of inflammation (Antoine et al., 2009).
ICAM-1 is a transmembrane glycoprotein that binds to integrins to promote the migration of leukocytes to vascular endothelial cells. With the help of ICAM-1, leukocytes adhere tightly to endothelial cells, inducing an increase in intracellular free Ca2+, thus affecting the contractility of myosin and the activation of p38 kinase (Lawson and Wolf, 2009). VCAM-1 is a type I transmembrane glycoprotein. Its main function is to facilitate the adhesion of leukocytes to endothelial cells. VCAM-1 is a member of the immunoglobulin superfamily and is mainly expressed in endothelial cells (Li et al., 2008). VCAM-1 is important in the development of atherosclerosis since it recruits monocyte adhesion molecules (Hwa et al., 2011). MCP-1 is the first human chemokine that has been discovered. It is produced by many cell types, including endothelial cells, fibroblasts, epithelial cells, smooth muscle cells, monocytes, and microglial cells (Singh et al., 2021). MCP-1 and its receptors (CCR2) undertake a very crucial function in the development of inflammatory responses and are vital for the recruitment of immune cells to the sites of inflammation (Deshmane et al., 2009).
MiRNA-126 (miR-126) is located in an intron of epidermal growth factor-like domain 7, and it can be selectively expressed in endothelial cells, which in turn plays an important role (Nikolic et al., 2010). Previous studies have shown that if miR-126 is knocked out, it can induce proliferation and migration of vascular endothelial cells, leading to vascular intimal injury and angiogenesis-dependent diseases (Bai et al., 2011). VCAM-1 is a specific target gene of miR-126, and silencing miR-126 resulted in increased VCAM-1 expression in TNF-stimulated VECs (Harris, 2008). Studies by Yuan et al. (2016) demonstrated that paeonol can upregulate the level of miRNA-126 in a concentration-dependent pattern, thus blocked the activation of the PI3K/Akt/NF-κB signaling pathway, and inhibited the expression of VCAM-1. Consequently, paeonol reduced the adhesion of rat monocytes to ox-LDL-injured VECs.
Under stress conditions, many cytokines and growth factors activate the mitogen-activated protein kinase (MAPK) pathway (Zhang and Liu 2002). In mammalian cells, the MAPK family consists of four members, including extracellular signal-regulated kinase (ERK), c-Jun N-terminal kinase (JNK), p38, and Big Map Kinase 1 (BMK1)/ERK5 (Pi et al., 2003; Wang et al., 2007). Previous studies have shown that cell adhesion is mainly connected with ERK1/2 and p38 (Huber et al., 2006). Wang et al. (2012) studied this signaling pathway and concluded that paeonol can inhibit the phosphorylation of ERK1/2, JNK1/2, and p38 by blocking the expression of VCAM-1, thereby prevented monocyte adhesion to ox-LDL-treated rat VECs. These preventive effects of paeonol were also verified by Pan and Dai (2009).
TNF-α is an important pro-inflammatory cytokine that causes vascular inflammation by activating the NF-B signaling pathway. Once NF-κB is dissociated from IκB-an inhibitor of NF-κB, it translocate from the cytoplasm to the nucleus and binds to a specific promoter region, which then transcribe VCAM-1 (Collins et al., 1995). Paeonol was discovered to inhibit the NF-κB signaling pathway in HUVECs by inhibiting VCAM-1 expression and preventing TNF-mediated monocyte infiltration (Kim et al., 2021). Also, based on the NF-κB signaling pathway, Yang et al. studied the role of toll-like receptor 4 (TLR4) in lipopolysaccharide-induced rat vascular endothelial cells. TLR4 is a transmembrane receptor that mediates inflammatory responses in endothelial cells. When TLR4 binds to its corresponding ligand, such as LPS, the canonical NF-κB signaling pathway is activated, leading to increased VCAM-1 protein expression (Sugiyama et al., 2008). However, paeonol can effectively inhibit the expression of TLR4, thereby inhibiting NF-κB and dependent pro-inflammatory signaling. These anti-inflammatory effects are reflected in reduced monocyte adhesion to rat endothelial cells (Li Y. et al., 2015). ICAM-1 molecule mediates the adhesion of leukocytes to activated endothelial cells by establishing strong bonds with their ligands and inducing strong leukocyte-specific β2 integrins such as lymphocyte function-associated antigen 1 (Chuang et al., 2004). Furthermore, in ApoE knockout mice, deletion of the ICAM-1 gene resulted in a marked reduction in the recruitment of monocytes to atherosclerotic lesions and was protective against atherosclerosis. These results highlight the significance of ICAM-1 in the development of atherosclerosis (Collins et al., 2000). Nizamutdinova et al. (2007) found that paeonol blocked p38, ERK, and NF-κB signaling pathways, reduced TNF-α-induced ICAM-1 expression in cultured HUVECs, and inhibited the adhesion of U937 cells to TNF-α-stimulated HUVECs. This result was also confirmed by the study by Zhou X. et al. (2011). Liu Y. R. et al. (2018) found that paeonol also inhibited the STAT3 signaling pathway, via not only reducing the expression of ICAM-1, but also VCAM-1 in HUVECs, thereby reduced THP-1 adhesion to HUVECs.
4.1.5 Regulation of endothelial cell survival by paeonol
A large number of studies have found that paeonol can affect the fate of endothelial cells through multiple pathways, such as apoptosis, autophagy, and premature aging. Endothelial cell apoptosis leads to plaque erosion, destabilization, and thrombosis, resulting in acute symptoms in patients with coronary heart disease (Chen et al., 2005). One study has shown that autophagy in endothelial cells plays different roles at various stages of disease (Shintani and Klionsky 2004). Activation of autophagy tends to increase the survival of VECs under stressed conditions, such as oxidative damage, cellular starvation, hypoxia, and other deleterious insults (Klionsky and Codogno 2013). When tremendous accumulation of ox-LDL occurs in endothelial cells, the secretion of a large number of inflammatory factors is increased, leading to deregulated endothelial homeostasis (Fulda and Kogel 2015). However, excessive autophagy leads to dysregulation of endothelial cells, including endothelial cell damage and death (Gatica, 2015).
Cellular senescence can be defined as an irreversible state of growth arrest in proliferative normal cells with distinct phenotypes as well as dysregulated gene expression profiles and protein function (Campisi and di Fagagna 2007). In biological redox processes, high levels of oxidative stressors, such as hydrogen peroxide (H2O2) (Higashi et al., 2009), and low levels of antioxidants are imbalanced, resulting in the formation of reactive ROS in endothelial cells over time, which can promote premature endothelial cell aging (Campisi and di Fagagna 2007). Lectin-like oxidized low-density lipoprotein receptor-1 (LOX-1) is a 50 kD type II membrane glycoprotein, discovered by Sawamura et al. (1997), and belongs to the C-type lectin family. It is expressed in different cell types such as endothelial cells, macrophages, vascular smooth muscle cells, and platelets (Sawamura et al., 1997). Numerous reports have implicated LOX-1 in the pathogenesis of atherosclerosis, including endothelial cell apoptosis and dysfunction, smooth muscle cell proliferation, platelet and monocyte adhesion, and plaque rupture (Pirillo et al., 2013). Activation of LOX-1 initiates intracellular signaling (Balzan and Lubrano 2018). As a result, LOX-1 facilitates the production of ROS, which leads to the sequential phosphorylation of a series of protein kinases, such as MAPKs.
The MAPK cascade mediates the activation of multiple transcription factors, such as NF-κB, activator protein-1 (AP-1), etc., and regulates the expression of apoptosis-related proteins, such as caspase-3 (which induces apoptosis) and B cell lymphoma-2 (Bcl-2) (which prevents apoptosis). Studies have shown that paeonol can inhibit the LOX-1/p38MAPK/NF-κB pathway in HUVECs, inhibit ROS overproduction, inhibit p38MAPK phosphorylation and nuclear translocation of NF-κB, and inhibit ox-LDL-induced caspase-3 activation, thereby prevented ox-LDL-induced endothelial cell apoptosis (Bao et al., 2013). Tang et al. (2021) revealed that paeonol inhibits the expression of caspase-3, and inhibits the HG/HP-induced HUVEC apoptosis via regulating the SIRT1/FOXO3a/NF-κB signaling pathway. Beclin-1 is a key autophagy-promoting gene that regulates the death and survival of many cell types (Zhu et al., 2009). miR-30a has been exhibited to suppress autophagy by negatively regulating the expression of its target gene-Beclin-1 (Long et al., 2013). Li et al. (2018) found that paeonol can upregulate miRNA30a expression, thus inhibit Beclin-1 and LC3-II target genes, thereby attenuate ox-LDL-induced autophagy in VECs.
With the exception of induction of autophagy and inhibition of endothelial apoptosis, activation of Sirtuin 1 (Sirt1) also protects endothelial cells against cell death by regulating cellular senescence, cell survival, and metabolism (Inoue et al., 2007). In this regard, Jamal et al. (2014) showed that paeonol can reverse the growth-arresting effects induced by H2O2, thereby restoring endothelial cell viability. At the same time, paeonol also remarkably decreased the expression levels of p53, acetyl H3K14, and H4K16 proteins, which were upregulated by H2O2. The protective effects of paeonol are partially explained by the upregulation of Sirt1 protein and its deacetylation substrates, thereby protected endothelial cells from oxidative stress-induced premature aging (Jamal et al., 2014).
4.2 Paeonol and smooth muscle cell function
Abnormal proliferation, migration, and phenotypic switch of vascular smooth muscle cells (VSMC) are prominent manifestations of VSMC dysfunction, leading to the formation of neointima and the development of atherosclerosis (Lacolley et al., 2017). In recent years, mounting studies have shown that paeonol protects against VSMC dysfunction, by inhibiting the excessive proliferation and migration of VSMC and regulating VSMC survival (Figure 5).
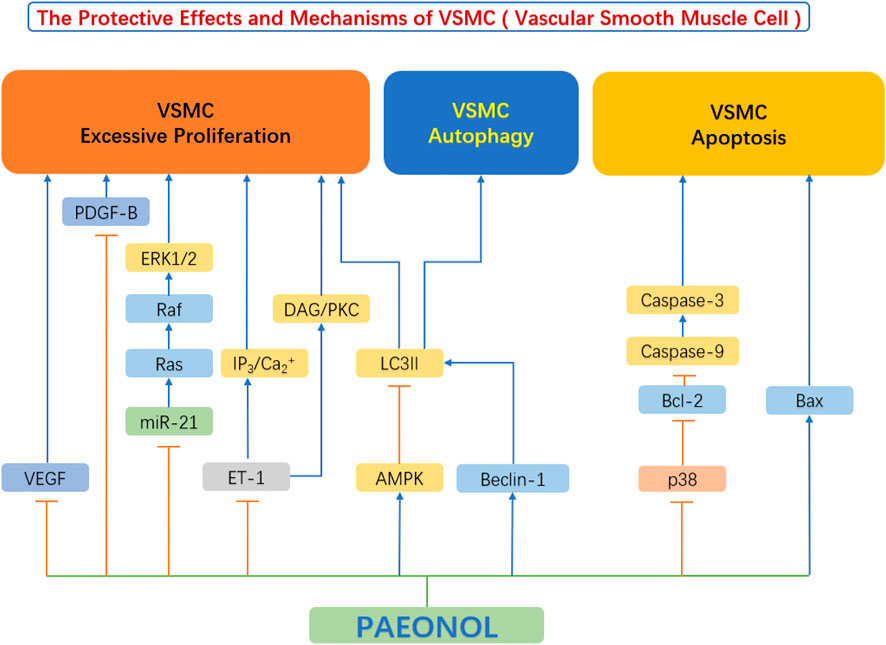
FIGURE 5. The protective effects and mechanisms of paeonol in smooth muscle cells.AMPK, AMP-activated protein kinase; Bax, Bcl-2-associated X protein; Bcl-2, B-cell lymphoma-2; Beclin-1, the mammalian ortholog of yeast ATG6; Ca, calcium; DAG, diacylglycerols; ERK1/2, extracellular signal regulated kinase 1/2; ET-1, endothelin-1; IP3, inositol 1,4,5-triphosphate; LC3II, light chain 3 II; miR-21, microRNA-21; p38, p38 mitogen-activated protein kinase (MAPK); PDGF-B, platelet derived growth factor B; PKC, protein kinase C; VEGF, vascular endothelial growth factor.
4.2.1 Inhibition of proliferation of vascular smooth muscle cells
Vascular endothelial cell injury and dysfunction is the leading cause of atherosclerosis development. The subsequent event following endothelial injury is the aberrant proliferation and migration of VSMC (Qin and Liu 2007). They release a large number of cytokines/chemokines/growth factors in response to endothelial injury, including Vascular Endothelial Growth Factor (VEGF), basic Fibroblast Growth Factor (bFGF), Transforming Growth Factor-β (TGF-β), Platelet Derived Growth Factor (PDGF), and others, all of which play important roles in regulating the proliferation and migration of VSMC by acting as mitogens (Laddha and Kulkarni 2019). These growth factors bind to VSMC membrane receptors and trigger the phosphorylation of downstream receptors of VEGF or PDGF-B pathways (Labrecque et al., 2005), such as ERK1/2, leading to increased VSMC proliferation and intimal thickening (Ortmann et al., 2011). Chen et al. (2014) found that paeonol can reduce the release of VEGF and PDGF-B from vascular endothelial cells. At the same time, the authors also found that paeonol can directly inhibit the Ras-Raf-ERK1/2 signaling pathway and inhibit the upregulation of Ras, Raf, and ERK phosphorylation in VSMCs (Chen et al., 2014). Further research by Wu et al. found that paeonol can also decrease the expression of endothelin-1 (ET-1), block IP3/Ca2+ and DAG/PKC signaling pathways, thereby inhibit vascular smooth muscle cell hyperproliferation (Wu et al., 2015).
Zhang et al. found that paeonol inhibited theERK1/2 signaling pathway and reduced the hypoxia-induced expression of cyclin A and cyclin D. By doing so, paeonol prevented the proliferation of VSMC by arresting the cell cycle at G0/G1 phase (Zhang et al., 2018). Previous studies have exhibited that AMP-dependent protein kinase (AMPK) is involved in the endothelial protective effects mediated by paeonol (Choy et al., 2016). Similarly, Wu et al. (2018) showed that the above mechanism also exists in VSMCs. Paeonol can activate the AMPK/mechanistic target of rapamycin (mTOR) signaling pathway, thereby inhibit VSMC proliferation (Wu et al., 2018).
4.2.2 Regulation of smooth muscle cell fate and survival
It is well established that the apoptosis of VSMCs most often triggers plaque rupture and its sequelae (Grootaert et al., 2018). Apoptosis of VSMCs promotes multiple characteristics of vulnerable plaques, by increasing necrotic core, thinning of the fibrous cap, and infiltration of macrophages into the fibrous cap (Harrison et al., 2019). Furthermore, VSMC apoptosis leads to calcification and vascular inflammation (Dong et al., 2019).
There is evidence that high concentration of ox-LDL induce apoptosis of VSMCs and ultimately promote the formation of atherosclerosis (Ding et al., 2013). Caspase-9 plays a crucial role in the endogenous apoptotic pathway as well as caspase-3 activation (Yin et al., 2011). Meng et al. (2015) showed that paeonol can promote the upregulation of Bax in smooth muscle cells and the downregulation of Bcl-2 to induce apoptosis of vascular smooth muscle cells, thereby effectively inhibit the proliferation of VSMCs. The P38 MAPK signaling pathway plays an important role in regulating apoptosis (Gaundar and Bendall 2010), and its downstream proteins p53 and caspase-3 are closely related to apoptosis (Muller and Vousden 2014). Liu Y. et al. (2018) have shown that paeonol can inhibit the activation of the p38 MAPK signaling pathway in VSMCs, thereby reduced the level of apoptosis-related proteins, and finally inhibited the apoptosis of VSMCs. In terms of the important role of apoptosis of smooth muscle cells in driving plaque vulnerability, paeonol has the potential to stabilize vulnerable plaques (Liu Y. et al., 2018).
Unlike apoptosis, autophagy is a defense mechanism that removes unnecessary and harmful cytoplasmic components through autophagosomes. VSMC autophagy is crucial in the development of atherosclerosis (Tai et al., 2016). Autophagy plays a key protective role in maintaining cellular homeostasis and alleviating atherosclerosis progression (Liao et al., 2012). Upregulation of VSMC autophagy in the early stages of atherosclerosis helps to promote a quiescent cellular phenotype, reduces proliferation, and inhibits fibrosis (Wei et al., 2013). This process was shown to protect plaque cells from oxidative damage and maintain plaque stability (Hughes et al., 2020). In advanced stages of atherosclerosis, however, autophagy is insufficient to clear damaged mitochondria, and an intrinsic apoptotic pathway may occur (Ryter et al., 2010). In other words, autophagy abnormalities in VSMCs disrupt cellular homeostasis and cause cellular proliferation, which leads to the development or acceleration of atherosclerosis (Grootaert et al., 2015). Studies have shown that the phosphatidylinositol-3 kinase (PI3K)-Beclin-1 pathway is one of the main signaling pathways regulating autophagy (Jamuna et al., 2019). PI3Ks are thought to regulate autophagosome formation after forming a complex with Beclin-1 (Wang et al., 2020). The study by Liu et al. (2021) showed that paeonol significantly increased the level of LC3II protein in ox-LDL-induced injured VSMCs, decreased p62 and cleaved caspase-3 protein levels, and increased the number of autophagosomes of ox-LDL-injured vascular smooth muscle cells, which promotes autophagy. At the same time, the authors found that paeonol can significantly activate the PI3K/Beclin-1 signaling pathway, induce autophagy in VSMCs, and ultimately inhibit ox-LDL-induced apoptosis of vascular smooth muscle cells (Liu et al., 2021). Research by Wu et al. (2018) also showed similar results.
4.3 Paeonol and macrophage function
A large number of recent studies have found that paeonol has a protective effect on macrophages, which is achieved by inhibiting macrophage M1 polarization, regulating macrophage inflammation and macrophage-derived foam cell formation (Figure 6).
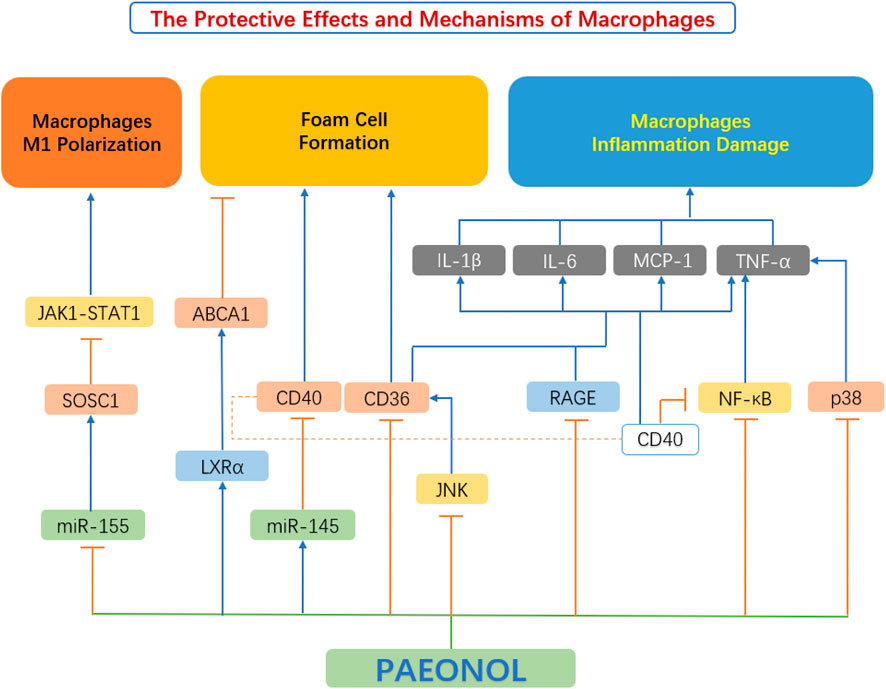
FIGURE 6. The protective effects and mechanisms of paeonol on macrophage functions. ABCA1, ATP binding cassette transporter A1; CD36, scavenger receptors; CD40, cluster of differentiation 40; IL-1β, interleukin 1β; IL-6, interleukin 6; JAK1-STAT1, Janus kinase 1-signal transducer and activator of transcription 1; JNK, c-Jun N-terminal kinase; LXRα, liver X receptor α; MCP-1, monocyte chemoattractant protein-1; miR-145, microRNA-145; miR-155, microRNA-155; NF-κB, the nuclear factor kB; P38, p38 mitogen-activated protein kinase (MAPK); RAGE, advanced glycation end products; SOCS1, suppressor of cytokine signaling 1; TNF-α, tumour necrosis factor α.
4.3.1 Inhibition of macrophage polarization
Mounting studies have shown that the polarization of macrophages from the M1 to M2 subtype is related to the activation of Janus kinase-signal transducer and activator of the transcription (JAK-STAT) pathway. Activation of the JAK1-STAT1 pathway leads to an increase in the phosphorylation of key mediators in the JAK1-STAT1 pathway. After phosphorylation, STAT1 translocate to the nucleus, leading to the M1-type polarization of macrophages. The downstream Suppressor of Cytokine Signaling1 (SOCS1) protein is also a negative feedback regulator of this pathway. Elevation of SOCS1 inhibits the JAK1-STAT1 pathway (Feng et al., 2019; Liang et al., 2017). miR-155 is a pro-inflammatory miRNA that is highly expressed in M1-type macrophages. SOCS1 is also a target gene of miR-155. When miR-155 is elevated, the expression of SOCS1 is inhibited (Moore et al., 2013; Xu, 2016). Sun et al. stimulated RAW264.7 macrophages with LPS and IFN to establish an M1-type polarization model, then tested the effect of paeonol on macrophage polarization. It was found that paeonol reduces the expression of CD86, F4/80, IL-6, TNF-α, miR-155, and the activation of the JAK1-STAT1 pathway. Paeonol significantly downregulated the phosphorylation level of proteins involved in JAK1-STAT1 and upregulated SOCS1 protein expression. The concerted actions of paeonol reduced the polarization of RAW264.7 macrophages to M1 subtype (Sun et al., 2020).
4.3.2 Regulation of macrophage-derived foam cell formation
Foam cell formation is chiefly caused by impaired cholesterol efflux or unbounded uptake of ox-LDL within macrophages (Tsai et al., 2010). Paeonol regulates lipid homeostasis by inhibiting lipid uptake and promoting cholesterol efflux in macrophages. Macrophage express a variety of scavenger receptors (SR), for example, SR-A and cluster of differentiation 36 (CD36), which are chiefly responsible for ox-LDL uptake (Zhou et al., 2013). In contrast, intracellular cholesterol efflux is primarily achieved through reverse cholesterol transporters (RCTs), mediated by ATP-binding cassette transporters a1 (ABCA1), ABCG11 and SR-BI (Saeed et al., 2012). Two of the most important factors in limiting foam cell development are scavenger receptor-mediated ox-LDL internalization and RCT-mediated cholesterol efflux. (Zhao et al., 2012).
Studies by Li X. Y. et al. (2015) showed that paeonol can reduce the expression of CD36 in RAW264.7 macrophages, thereby inhibiting the uptake of ox-LDL by macrophages, and resulting in reduced foam cell formation. Meanwhile, paeonol can also increase the expression of ABCA1 in macrophages, leading to enhanced RCT (Li X. Y. et al., 2015). Zhao et al. (2013) further investigated the mechanism by which paeonol increased the expression of ABCA1. The study found that paeonol induces ABCA1 expression via an LXR-dependent mechanism. By activating LXR, paeonol promoted cholesterol efflux from macrophages and reduced foam cell formation (Zhao et al., 2013).
4.3.3 Inhibition of inflammatory responses in macrophages
Advanced oxidation protein products (AOPPs) are credible markers of oxidative stress and systemic inflammation, originally described in uremic subjects by Witko-Sarsat et al. (1999). AOPPs, function as a class of potential pro-inflammatory mediators in various cell types, especially macrophages, through the production of pro-inflammatory cytokines and adhesion molecules (Kishimoto et al., 2010). Studies by Ping et al. (2014) have shown that paeonol can reduce the expression level of inflammatory factors in macrophages, induced by AOPP, and promote the expression of receptors for advanced glycation end products (RAGE) and CD36 protein. Activation of the RAGE-mediated and CD36-mediated signaling pathways reduces the expression of inflammatory factors and inhibits the secretion of inflammatory factors by macrophages (Ping et al., 2014).
Jin et al. (2016) explored the effect of paeonol on the MAPK/ERK/p38 signaling pathway in macrophages and found that paeonol can dose-dependently inhibit LPS-induced phosphorylation of JNK, ERK 1/2 and p38 MAPKs, thereby attenuating the production of inflammatory factors, and improving macrophage survival.
4.4 Anti-platelet functions of paeonol
Platelets are enucleated cells produced by megakaryocytes in the bone marrow and lungs (Lefrancais et al., 2017). Platelets are proverbially activated and recruited to impaired endothelial cells due to abnormal circulating rheology or upregulation of pro-coagulant substances in atherosclerotic lesions. The initiation of the coagulation cascade leads to arterial thrombosis and vascular occlusion. This kind of acute coronary artery disease can seriously endanger the patient’s life. (Kishimoto et al., 2010). Studies by Koo et al. (2010) elucidated that paeonol and its analogs have beneficial effects on thrombosis by directly inhibiting platelet aggregation and blood coagulation. Hirai et al. (1983) discovered that paeonol inhibited ADP-induced and collagen-induced platelet aggregation in vitro in a dose-dependent pattern. The mechanism of reducing platelet aggregation may be attributed to the reduction of thromboxane synthesis, along with the improvement of hemorheological parameters (Hirai et al., 1983). The anti-platelet actions of paeonol were confirmed by Bai (2015). Another anti-thrombotic mechanism of paeonol may be related to the increase of nitric oxide (NO) and prostacyclin I2 (PGI2), which are antagonists of platelet activity. While endothelin ET-1 and thromboxane A2 (TXA2) are agonists of platelet activation and aggregation, paeonol can reduce the content of ET-1 and TXA2 in the diabetic rats’ serum, and inhibit the activation and aggregation of platelets (Min et al., 2007). Therefore, paeonol is considered to be a protective agent for reducing thrombotic events (Figure 7).
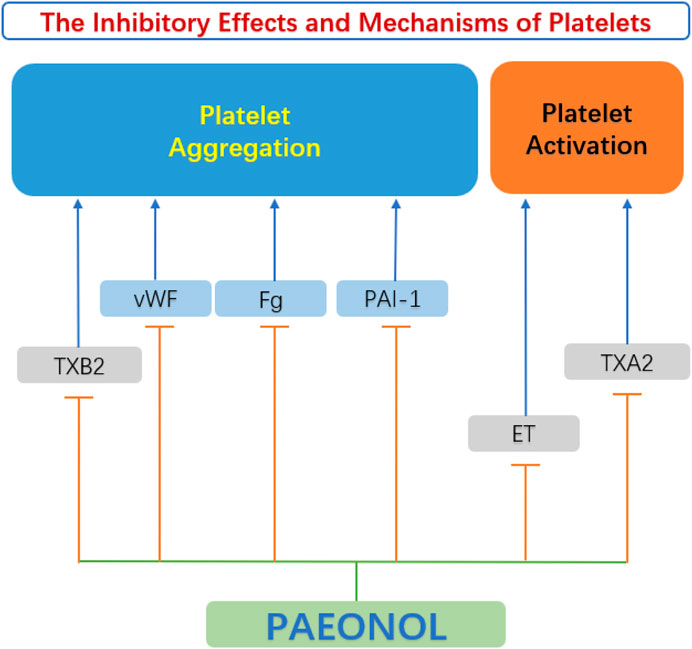
FIGURE 7. Anti-platelet function of paeonol. ET, endothelin; Fg, fibrinogen; PAI-1, plasminogen activator inhibitor 1 gene; TXA2, thromboxane A2; TXB2, thromboxane B2; vWF, von Willebrand factor.
4.5 Other protective effects and mechanisms
In addition to the relevant functions mentioned above, paeonol also has many other protective effects, which play an important role in the occurrence and development of AS (Figure 8).
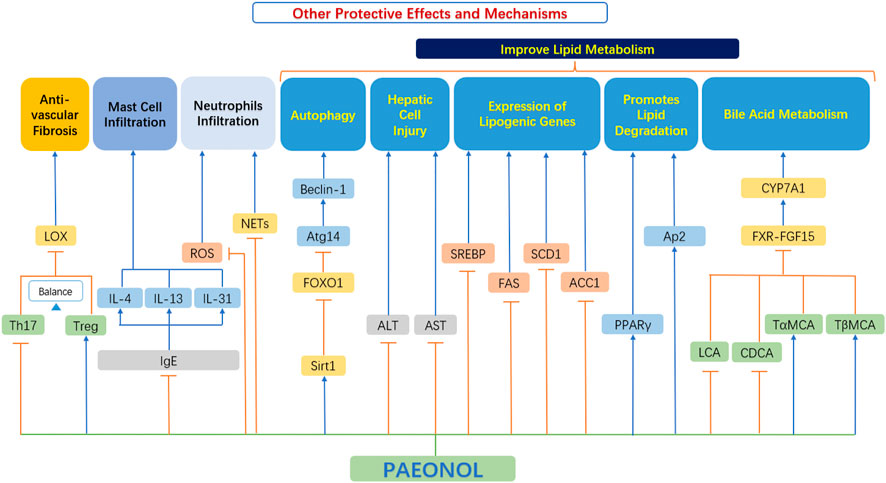
FIGURE 8. Other protective effects and mechanisms of paeonol. ACC1, acetyl-CoA carboxylase-1; ALT, alanine aminotransferase; Ap2, adipocyte protein 2; AST, aspartate aminotransferase; Atg14, autophagy related 14; Beclin-1, the mammalian ortholog of yeast ATG6; CDCA, chenodeoxycholic acid; CYP7A1, cholesterol 7α-hydroxylase; FAS, fatty acid synthase; FOXO1, forkhead box transcription factor O1; FXR, farnesoid X receptor; FGF15, fibroblast growth factor 15; IgE, immunoglobulin E; IL-4, interleukin 4; IL-13, interleukin 13; IL-31, interleukin 31; LOX, lysyl oxidase; NETs, neutrophils extracellular traps; PPAR-γ, peroxisome proliferator-activated receptor γ; ROS, reactive oxygen species; SCD1, stearoyl-CoA desaturase 1; Sirt1, sirtuin 1; SREBP, sterol regulatory element-binding protein; TαMCA, tauro-α-muricholic acid; TβMCA, tauro-β-muricholic acid; Th17, T helper type 17 cell; Treg, Regulatory T-cells.
4.5.1 Anti-fibrotic effects
Th17 cells and Treg cells both play vital roles in regulating vascular fibrosis by adjusting the expression of collagen and MMPs (Slobodin and Rimar 2017). However, a reduction in gut microbiota diversity leads to a skewed Treg/Th17 balance towards Th17 cells, which contrariwise exacerbates vascular fibrosis. Short-chain fatty acids (SCFAs) have been demonstrated to promote Treg cell differentiation and suppress inflammatory responses (Liu et al., 2020).
Shi et al. (2021) found that paeonol selectively upregulated the proportion of Treg cells and downregulated the proportion of Th17 cells in the spleen of mice. Paeonol improved the Treg/Th17 balance while indirectly downregulated the protein expression level of LOX and fibrosis-related markers (MMP-2/9 and collagen I/III), thereby reduced vascular fibrosis in a gut microbiota-dependent manner (Shi et al., 2021).
4.5.2 Improvement of lipid metabolism
Dong et al. (2020) showed that paeonol activates the SIRT1-FoxO1-ATG14 signaling pathway, promotes SIRT1-FoxO1-ATG14-dependent autophagy, prevents lipid metabolism dysfunction in palmitate-induced HepG2. Hu et al. (2010) explored the beneficial effects of paeonol on alcoholic steatohepatitis in mice. Relevant studies have found that paeonol can significantly reduce the serum transaminase level in a model of alcoholic liver injury, and reduce the severity of hepatocyte injury, steatosis, and inflammatory cell infiltration. At the same time, paeonol significantly decreased the mRNA expression of hepatic lipogenesis genes and reduced hepatic lipogenesis (Hu et al., 2010). This also proves that paeonol has some potential for improving lipid metabolism.
Their studies (Li X. Y. et al., 2015) showed that paeonol can inhibit adipogenesis [inhibiting triglyceride (TG) synthesis and the expression of adipogenesis transcription factors in adipocytes], and at the same time, paeonol can promote lipid degradation in adipocytes, which in turn improves lipid metabolism.
4.5.3 Stabilization of mast cells
Mast cells are primarily known for their role in host defense system and their involvement in allergies. Recently, a lot of evidence has proved that mast cells have a significant impact on cardiovascular diseases, especially on the occurrence and development of atherosclerotic plaques. Activated mast cells release growth factors, histamines and chemokines. This in turn leads to a series of cardiovascular adverse events, such as matrix degradation, apoptosis, and recruitment of inflammatory cells (Bot et al., 2015). Therefore, therapeutically, intervening in mast cell activation pathways may be more beneficial than blocking the role of individual mast cell mediator (Bot, 2021).
Kim et al. (2004) found that paeonol significantly inhibited the release of histamine by mast cells, exhibited dose-dependent inhibition of TNF-α, inhibited the production of immunoglobulin E (IgE) effectively, and downregulated the expression of IL-4 in cells, thus playing an anti-atherosclerosis role. Lee et al. (2008) also confirmed these functions. Further studies by Meng et al. (2019) found that paeonol can inhibit the level of IgE and inflammatory cytokines, decrease thymic stromal lymphopoietin, IL-4, IL-13, IL-31 and histamine, regulate T helper (Th) cell subset (Th1/Th2) ratio, and effectively inhibit mast cell infiltration, thus playing an effective protective role.
Thus, mast cell stabilization effects by paeonol appears to be a promising novel mechanism of paeonol in suppressing atherosclerosis.
4.5.4 Regulation of neutrophils and neutrophils extracellular traps
Neutrophils are the main players in the pathophysiology of inflammatory diseases not only by secreting reactive oxygen species (ROS) and tissue-damaging cytotoxic enzymes, but also by releasing vital immunomodulatory cytokines and chemokines (Cross et al., 2020). There is growing evidence supporting those neutrophils are important actors in plaque progression (Döring et al., 2015). During the development of atherosclerosis, various stimulants such as platelets and chemokines are produced successively (Van Avondt et al., 2019). When neutrophils are affected, they release cytoplasmic and nuclear material, forming a network of extracellular structures that form neutrophils extracellular traps (NETs). NETs further promote inflammation and progression of atherosclerosis (Josefs et al., 2020).
The study by Chou showed that inhibition of neutrophil infiltration may be an important mechanism for paeonol to achieve anti-inflammatory effects (Chou, 2003). Fu et al. (2012) also proved the above effects. Cross et al. (2020) have recently completed a clinical trial showing that the novel drug APPA which components are apocynin (AP) and paeonol (PA) downregulates the level of ROS and neutrophil degranulation and reduces of neutrophil extracellular traps formation. In addition, APPA attenuates the expression of cytokine stimulated genes and inhibited the cell signaling induced by TNF-α and granulocyte-macrophage colony-stimulating factor (GM-CSF). All these evidence indicate a new therapeutic notion, that is, to use paeonol to intervene the progress of neutrophils and NETs (Cross et al., 2020). It should be noted that this trial is a compound preparation (APPA), so the precise effect of paeonol needs to be further verified experimentally and clinically.
5 Summary and outlook
Paeonol, a naturally-occurring and biologically active compound found in P. suffruticosa, has been demonstrated experimentally to be a promising potential therapeutic drug for the treatment of cardiovascular diseases. Paeonol has protective effects on endothelial cells, smooth muscle cells, macrophages, and platelets in the initiation and progression of atherosclerosis. These protective effects are attributed to multifactorial effects such as anti-inflammatory, anti-oxidative, anti-apoptotic, and lipid metabolism control, which are all based on separate pathways.
These pharmacological effects of paeonol indicate that it has great potential for clinical application in the prevention and treatment of atherosclerosis. We examined the drug similarity of paeonol through SwissADME website (Daina et al., 2017), and the parameters including Lipinski, Ghose, Veber, Egan, and Muegge, except for Muegge, all the other results showed that paeonol had good drug-likeness. Nevertheless, the pharmacokinetic properties of paeonol have not been well characterized. Various new dosage forms of paeonol have been developed to overcome the shortcomings of its poor solubility and instability, and to improve its oral bioavailability and drug retention in the body (Adki and Kulkarni, 2020). These efforts provided reliable technical support for paeonol in practice.
In addition, our literature review found that the atheroprotective effects of paeonol have only been addressed in rabbits, quails and ApoE knockout mice, thus lacking efficient studies in large animal models, such as rabbits and monkeys. In addition, clinical data for paeonol in patients with CVD is still insufficient. We searched the NIH clinical trial database (clinicaltrials.gov) and did not find any clinical trials using paeonol as an active ingredient for atherosclerotic diseases. Hence, large-scale randomized, placebo-controlled, and double-blinded clinical trials are urgently needed to assess the efficacy and safety of paeonol.
As summarized in this review, the molecular targets of paeonol are very complex, and the relationship (synergy and antagonism) between different targets and signaling pathways cannot be clearly explained. This urgently requires us to use systems biology methods to deeply study the specific molecular targets of paeonol for instance, the use of photoactive probes to label metformin, and then identify PEN2 as the direct target of metformin (Ma et al., 2022).
At the same time, the pathophysiology of severe acute respiratory syndrome coronavirus 2 (SARS-CoV-2) is characterized by the overproduction of inflammatory cytokines (such as IL-6 and TNF-α) leading to systemic inflammation and multiple organ dysfunction syndrome, including acute and chronic effects on the cardiovascular system (AlShahrani et al., 2021). In addition, preliminary studies indicate that vascular endothelial cells can be infected by SARS-CoV-2, and evidence of widespread endothelial injury and inflammation is found in severe patients with COVID-19 (Nagele et al., 2020). Paeonol could be a possible medication to alleviate endothelial dysfunction in COVID-19 patients due to its inhibitory effect on the expression/secretion of inflammatory cytokines.
In addition to the several major cell types mentioned above, there are many other types of cells that profoundly influence the occurrence, development, and outcome of atherosclerosis, such as immune cells (neutrophils, T/B lymphocyte cells, etc.) (Aguilar-Ballester, et al., 2020) and blood cells (red blood cells, etc.) (Blum, 2009). However, our literature review shows that there are few studies of the effect of paeonol on these cell types, and the relevant mechanisms of action and the targets and signaling pathways involved are not clear. It is well worthwhile to carry out relevant research work in the future.
Later stages of atherosclerotic progression may lead to arterial blockage, followed by myocardial hypoperfusion. This continued no-reflow may lead to severe hospitalization and death. The study of Ma et al. (2016) showed that paeonol could significantly reduce the area of myocardial infarction and no-reflow, and improve local myocardial perfusion and cardiac function, showing a potential cardiac protective effect.
In summary, paeonol exerts pleiotropic effects in different stages of atherosclerosis and may represent a promising drug candidate in pharmacotherapies of cardiovascular diseases.
Author contributions
Conceptualization: WY and SX. Write: WY. Revise: WY, SX, II, and NA.
Funding
This study was supported by Anhui Provincial Key Research and Development Program (Grant No. 202104j07020051) and Anhui Province Science Fund for Distinguished Young Scholars (Grant No. 2208085J08).
Conflict of interest
WY was employed by Anhui Renovo Pharmaceutical Co., Ltd.
The remaining authors declare that the research was conducted in the absence of any commercial or financial relationships that could be construed as a potential conflict of interest.
Publisher’s note
All claims expressed in this article are solely those of the authors and do not necessarily represent those of their affiliated organizations, or those of the publisher, the editors and the reviewers. Any product that may be evaluated in this article, or claim that may be made by its manufacturer, is not guaranteed or endorsed by the publisher.
References
Adawi, M., Pastukh, N., Saaida, G., Sirchan, R., Watad, A., Blum, A., et al. (2019). Inhibition of endothelial progenitor cells may explain the high cardiovascular event rate in patients with rheumatoid arthritis. Qjm-an Int. J. Med. 112 (2), 161. doi:10.1093/qjmed/hcy204
Adki, K. M., and Kulkarni, Y. A. (2020). Chemistry, pharmacokinetics, pharmacology and recent novel drug delivery systems of paeonol. Life Sci. 250, 117544. doi:10.1016/j.lfs.2020.117544
Aguilar-Ballester, M., Herrero-Cervera, A., Vinué, Á., Martínez-Hervás, S., and González-Navarro, H. (2020). Impact of cholesterol metabolism in immune cell function and atherosclerosis. Nutrients 12 (7), E2021. doi:10.3390/nu12072021
AlShahrani, I., Hosmani, J., Shankar, V. G., AlShahrani, A., Togoo, R. A., Yassin, S. M., et al. (2021). COVID-19 and cardiovascular system-a comprehensive review. Rev. Cardiovasc. Med. 22 (2), 343–351. doi:10.31083/j.rcm2202041
Antoine, M., Tag, C. G., Gressner, A. M., Hellerbrand, C., and Kiefer, P.(2009). Expression of E-selectin ligand-1 (CFR/ESL-1) on hepatic stellate cells: implications for leukocyte extravasation and liver metastasis. Oncol. Rep. 21 (2), 357–362. doi:10.3892/or_00000230
Bai, Q. (2015). Influence of endothelial function and blood rheology in diabetic rats by paeonol and tanshinol. Chin. J. Exp. Traditional Med. Formulae 21 (3), 110–113.
Bai, Y. Y., Bai, X., Wang, Z. Y., Zhang, X. F., Ruan, C. G., Miao, J. C., et al. (2011). MicroRNA-126 inhibits ischemia-induced retinal neovascularization via regulating angiogenic growth factors. Exp. Mol. Pathol. 91 (1), 471–477. doi:10.1016/j.yexmp.2011.04.016
Balzan, S., and Lubrano, V. (2018). LOX-1 receptor: A potential link in atherosclerosis and cancer. Life Sci. 198, 79–86. doi:10.1016/j.lfs.2018.02.024
Bao, M. H., Zhang, Y. W., and Zhou, H. H. (2013). Paeonol suppresses oxidized low-density lipoprotein induced endothelial cell apoptosis via activation of LOX-1/p38MAPK/NF-kappa B pathway. J. Ethnopharmacol. 146 (2), 543–551. doi:10.1016/j.jep.2013.01.019
Ben Salem, C., Sahnoun, D., Slim, R., Ben Fradj, F., and Aouani, C. (2020). Atorvastatin and sildenafil interaction-induced rhabdomyolysis. Ann. Pharmacother. 54 (10), 1047–1048. doi:10.1177/1060028020919933
Blum, A. (2009). The possible role of red blood cell microvesicles in atherosclerosis. Eur. J. Intern. Med. 20 (2), 101–105. doi:10.1016/j.ejim.2008.06.001
Bot, I., Shi, G. P., and Kovanen, P. T. (2015). Mast cells as effectors in atherosclerosis. Arterioscler. Thromb. Vasc. Biol. 35 (2), 265–271. doi:10.1161/ATVBAHA.114.303570
Bot, I. (2021). The mast cell: A novel actor in cardiac microvessel dysfunction. Arterioscler. Thromb. Vasc. Biol. 41 (4), 1337–1338. doi:10.1161/ATVBAHA.121.316043
Campisi, J., and di Fagagna, F. D. (2007). Cellular senescence: When bad things happen to good cells. Nat. Rev. Mol. Cell. Biol. 8 (9), 729–740. doi:10.1038/nrm2233
Chen, F., Eriksson, P., Kimura, T., Herzfeld, I., and Valen, G. (2005). Apoptosis and angiogenesis are induced in the unstable coronary atherosclerotic plaque. Coron. Artery Dis. 16 (3), 191–197. doi:10.1097/00019501-200505000-00009
Chen, J. J., Dai, M., and Wang, Y. Q. (2014). Paeonol inhibits proliferation of vascular smooth muscle cells stimulated by high glucose via ras-raf-ERK1/2 signaling pathway in coculture model. Evidence-Based Complementary Altern. Med. 9, 1. doi:10.1155/2014/484269
Chen, L., Liu, P., Feng, X., and Ma, C. (2017). Salidroside suppressing LPS-induced myocardial injury by inhibiting ROS-mediated PI3K/Akt/mTOR pathway in vitro and in vivo. J. Cell. Mol. Med. 21 (12), 3178–3189. doi:10.1111/jcmm.12871
Chobanian, A. V (1991). Single risk factor intervention may be inadequate to inhibit atherosclerosis progression when hypertension and hypercholesterolemia coexist. Hypertension 18 (2), 130–131. doi:10.1161/01.hyp.18.2.130
Chou, T. C. (2003). Anti-inflammatory and analgesic effects of paeonol in carrageenan-evoked thermal hyperalgesia. Br. J. Pharmacol. 139 (6), 1146–1152. doi:10.1038/sj.bjp.0705360
Choy, K. W., Mustafa, M. R., Lau, Y. S., Liu, J., Murugan, D., Lau, C. W., et al. (2016). Paeonol protects against endoplasmic reticulum stress-induced endothelial dysfunction via AMPK/PPAR delta signaling pathway. Biochem. Pharmacol. 116, 51–62. doi:10.1016/j.bcp.2016.07.013
Chuang, K. P., Huang, Y. F., Hsu, Y. L., Liu, H. S., Chen, H. C., Shieh, C. C., et al. (2004). Ligation of lymphocyte function-associated antigen-1 on monocytes decreases very late antigen-4-mediated adhesion through a reactive oxygen species-dependent pathway. Blood 104 (13), 4046–4053. doi:10.1182/blood-2004-05-1822
Clark, H. (2013). NCDs: A challenge to sustainable human development. Lancet381 (9866), 510–5111. doi:10.1016/S0140-6736(13)60058-6
Collins, R. G., Velji, R., Guevara, N. V., Hicks, M. J., Chan, L., Beaudet, A. L., et al. (2000). P-Selectin or intercellular adhesion molecule (ICAM)-1 deficiency substantially protects against atherosclerosis in apolipoprotein E-deficient mice. J. Exp. Med. 191 (1), 189–194. doi:10.1084/jem.191.1.189
Collins, T., Read, M. A., Neish, A. S., Whitley, M. Z., Thanos, D., and Maniatis, T.(1995) Transcriptional regulation of endothelial cell adhesion molecules: NF-kappa B and cytokine-inducible enhancers. FASEB j, 9 (10), 899-909. doi:10.1096/fasebj.9.10.7542214
Cross, A. L., Hawkes, J., Wright, H. L., Moots, R. J., and Edwards, S. W. (2020). APPA (apocynin and paeonol) modulates pathological aspects of human neutrophil function, without supressing antimicrobial ability, and inhibits TNFα expression and signalling. Inflammopharmacology 28 (5), 1223–1235. doi:10.1007/s10787-020-00715-5
Dai, M., Zhi, X., Peng, D., and Liu, Q. (1999). [Inhibitory effect of paeonol on experimental atherosclerosis in quails]. Zhongguo Zhong Yao Za Zhi 24 (8), 488–490. [Article in Chinese].
Daina, A., Michielin, O., and Zoete, V. (2017). SwissADME: A free web tool to evaluate pharmacokinetics, drug-likeness and medicinal chemistry friendliness of small molecules. Sci. Rep. 7 (1), 42717. doi:10.1038/srep42717
Deshmane, S. L., Kremlev, S., Amini, S., and Sawaya, B. E. (2009). Monocyte chemoattractant protein-1 (MCP-1): An overview. J. Interferon Cytokine Res. 29 (6), 313–326. doi:10.1089/jir.2008.0027
Ding, Z. F., Liu, S. J., Wang, X. W., Khaidakov, M., Dai, Y., Mehta, J. L., et al. (2013). Oxidant stress in mitochondrial DNA damage, autophagy and inflammation in atherosclerosis. Sci. Rep. 3, 1077. doi:10.1038/srep01077
Dong, Y., Chen, H. W., Gao, J. L., Liu, Y. M., Li, J., Wang, J., et al. (2019). Molecular machinery and interplay of apoptosis and autophagy in coronary heart disease. J. Mol. Cell. Cardiol. 136, 27–41. doi:10.1016/j.yjmcc.2019.09.001
Dong, Z. M., Xie, X. M., Sun, Y., Wu, H. F., and Dai, M. (2020). Paeonol prevents lipid metabolism dysfunction in palmitic acid -induced HepG2 injury through promoting SIRT1-FoxO1-ATG14-dependent autophagy. Eur. J. Pharmacol. 880, 173145. doi:10.1016/j.ejphar.2020.173145
Döring, Y., Drechsler, M., Soehnlein, O., and Weber, C. (2015). Neutrophils in atherosclerosis: From mice to man. Arterioscler. Thromb. Vasc. Biol. 35 (2), 288–295. doi:10.1161/ATVBAHA.114.303564
Feng, L. L., Sun, Y., Song, P. P., Xu, L. S., Wu, X. X., Wu, X. F., et al. (2019). Seselin ameliorates inflammation via targeting Jak2 to suppress the proinflammatory phenotype of macrophages. Br. J. Pharmacol. 176 (2), 317–333. doi:10.1111/bph.14521
Feng, X., Zhang, L., Xu, S., and Shen, A. Z. (2020). ATP-citrate lyase (ACLY) in lipid metabolism and atherosclerosis: An updated review. Prog. Lipid Res. 77, 101006. doi:10.1016/j.plipres.2019.101006
Ferraro, R., Latina, J. M., Alfaddagh, A., Michos, E. D., Blaha, M. J., Jones, S. R., et al. (2020). Evaluation and management of patients with stable Angina: Beyond the ischemia paradigm JACC state-of-the-art review. J. Am. Coll. Cardiol. 76 (19), 2252–2266. doi:10.1016/j.jacc.2020.08.078
Fu, P. K., Wu, C. L., Tsai, T. H., and Hsieh, C. L. (2012). Anti-inflammatory and anticoagulative effects of paeonol on LPS-induced acute lung injury in rats. Evid. Based. Complement. Altern. Med. 2012, 837513. doi:10.1155/2012/837513
Fulda, S., and Kogel, D. (2015). Cell death by autophagy: Emerging molecular mechanisms and implications for cancer therapy. Oncogene 34 (40), 5105–5113. doi:10.1038/onc.2014.458
Gatica, D., Chiong, M., Lavandero, S., and Klionsky, D. J. (2015). Molecular mechanisms of autophagy in the cardiovascular system. Circ. Res. 116 (7), 456–467. doi:10.1161/circresaha.114.303788
Gaundar, S. S., and Bendall, L. J. (2010). The potential and limitations of p38MAPK as a drug target for the treatment of hematological malignancies. Curr. Drug Targets 11 (7), 823–833. doi:10.2174/138945010791320854
Gimbrone, M. A., and Garcia-Cardena, G. (2016). Endothelial cell dysfunction and the pathobiology of atherosclerosis. Circ. Res. 118 (4), 620–636. doi:10.1161/CIRCRESAHA.115.306301
Gjertsen, F. B., Solheim, E., and Scheline, R. R. (1988). Metabolism of aromatic plant ketones in rats: acetovanillone and paeonol. Xenobiotica 18 (2), 225–34. doi:10.3109/00498258809041658
Grootaert, M. O., da Costa Martins, P. A., Bitsch, N., Pintelon, I., De Meyer, G. R., Martinet, W., et al. (2015). Defective autophagy in vascular smooth muscle cells accelerates senescence and promotes neointima formation and atherogenesis. Autophagy 11 (11), 2014–2032. doi:10.1080/15548627.2015.1096485
Grootaert, M. O. J., Moulis, M., Roth, L., Martinet, W., Vindis, C., Bennett, M. R., et al. (2018). Vascular smooth muscle cell death, autophagy and senescence in atherosclerosis. Cardiovasc. Res. 114 (4), 622–634. doi:10.1093/cvr/cvy007
Habas, K., and Shang, L. J. (2018). Alterations in intercellular adhesion molecule 1 (ICAM-1) and vascular cell adhesion molecule 1 (VCAM-1) in human endothelial cells. Tissue Cell. 54, 139–143. doi:10.1016/j.tice.2018.09.002
Hansson, G. K. (2005). Inflammation, atherosclerosis, and coronary artery disease. N. Engl. J. Med. 352 (16), 1685–1695. doi:10.1056/NEJMra043430
Harris, T. A., Yamakuchi, M., Ferlito, M., Mendell, J. T., and Lowenstein, C. J. (2008). MicroRNA-126 regulates endothelial expression of vascular cell adhesion molecule 1. Proc. Natl. Acad. Sci. U. S. A. 105 (5), 1516–1521. doi:10.1073/pnas.0707493105
Harrison, O. J., Visan, A. C., Moorjani, N., Modi, A., Salhiyyah, K., Torrens, C., et al. (2019). Defective notch signaling drives increased vascular smooth muscle cell apoptosis and contractile differentiation in bicuspid aortic valve aortopathy: A review of the evidence and future directions. Trends cardiovasc. Med. 29 (2), 61–68. doi:10.1016/j.tcm.2018.06.008
He, H., Huang, H., Shi, X., Liu, L., Liu, Y., Wu, H., et al. (2021). Paeonol regulates bile acid metabolism in ApoE-/- mice and reduces atherosclerosis. Chin. J. Arteriosclerosis 29, 123–128.
Higashi, Y., Noma, K., Yoshizumi, M., and Kihara, Y. (2009). Endothelial function and oxidative stress in cardiovascular diseases. Circ. J. 73 (3), 411–418. doi:10.1253/circj.cj-08-1102
Himaya, S. W., Ryu, B., Qian, Z. J., and Kim, S. K. (2012).Paeonol from Hippocampus kuda Bleeler suppressed the neuro-inflammatory responses in vitro via NF-κB and MAPK signaling pathways. Toxicol. in Vitro 26(6), 878–887. doi:10.1016/j.tiv.2012.04.022
Hirai, A., Terano, T., Hamazaki, T., Sajiki, J., Saito, H., Tahara, K., et al. (1983). Studies on the mechanism of antiaggregatory effect of Moutan Cortex. Thromb. Res. 31 (1), 29–40. doi:10.1016/0049-3848(83)90005-1
Hope, S. A., and Meredith, I. T. (2003). Cellular adhesion molecules and cardiovascular disease. Part I. Their expression and role in atherogenesis. Intern. Med. J. 33 (8), 380–386. doi:10.1046/j.1444-0903.2003.00378.x
Hu, S. L., Shen, G., Zhao, W. G., Wang, F., Jiang, X. D., Huang, D. B., et al. (2010). Paeonol, the main active principles of Paeonia moutan, ameliorates alcoholic steatohepatitis in mice. J. Ethnopharmacol. 128 (1), 100–106. doi:10.1016/j.jep.2009.12.034
Hu, W.-J., Zhang, Z., and Dai, M. (2016). Paeonol affects proliferation activity of rat vasular endothelial cells induced by lipopolysaccharide and co-cultured with smooth muscle cells via inhibiting pathway of PI3K/AKT-NF-κB signaling. Zhongguo Zhong yao za Zhi. 41 (12), 2298–2302. doi:10.4268/cjcmm20161221
Huang, H., Chang, E. J., Lee, Y., Kim, J. S., Kang, S. S., Kim, H. H., et al. (2008). A genome-wide microarray analysis reveals anti-inflammatory target genes of paeonol in macrophages. Inflamm. Res. 57 (4), 189–198. doi:10.1007/s00011-007-7190-3
Huber, J., Furnkranz, A., Bochkov, V. N., Patricia, M. K., Lee, H., Hedrick, C. C., et al. (2006). Specific monocyte adhesion to endothelial cells induced by oxidized phospholipids involves activation of cPLA(2) and lipoxygenase. J. Lipid Res. 47 (5), 1054–1062. doi:10.1194/jlr.M500555-JLR200
Hughes, W. E., Beyer, A. M., and Gutterman, D. D. (2020). Vascular autophagy in health and disease. Basic Res. Cardiol. 115 (4), 41. doi:10.1007/s00395-020-0802-6
Hwa, J. S., Mun, L., Kim, H. J., Seo, H. G., Lee, J. H., Kwak, J. H., et al. (2011). Genipin Selectively Inhibits TNF-α-activated VCAM-1 But Not ICAM-1 Expression by Upregulation of PPAR-γ in Human Endothelial Cells. Korean J Physiol Pharmacol 15 (3), 157–162. doi:10.4196/kjpp.2011.15.3.157
Inoue, T., Hiratsuka, M., Osaki, M., and Oshimura, M. (2007). The molecular biology of mammalian SIRT proteins - SIRT2 in cell cycle regulation. Cell. Cycle 6 (9), 1011–1018. doi:10.4161/cc.6.9.4219
Jamal, J., Mustafa, M. R., and Wong, P. F. (2014). Paeonol protects against premature senescence in endothelial cells by modulating Sirtuin 1 pathway. J. Ethnopharmacol. 154 (2), 428–436. doi:10.1016/j.jep.2014.04.025
Jamuna, S., Ashokkumar, R., Sadullah, M. S. S., and Devaraj, S. N. (2019). Oligomeric proanthocyanidins and epigallocatechin gallate aggravate autophagy of foam cells through the activation of Class III PI3K/Beclin1-complex mediated cholesterol efflux. Biofactors 45 (5), 763–773. doi:10.1002/biof.1537
Ji, R. R., Cheng, Y. H., Yue, J. M., Yang, J., Liu, X. J., Chen, H., et al. (2007). MicroRNA expression signature and antisense-mediated depletion reveal an essential role of microRNA in vascular neointimal lesion formation. Circ. Res. 100 (11), 1579–1588. doi:10.1161/CIRCRESAHA.106.141986
Jin, X., Wang, J., Xia, Z. M., Shang, C. H., Chao, Q. L., Liu, Y. R., et al. (2016). Anti-inflammatory and anti-oxidative activities of paeonol and its metabolites through blocking MAPK/ERK/p38 signaling pathway. Inflammation 39 (1), 434–446. doi:10.1007/s10753-015-0265-3
Josefs, T., Barrett, T. J., Brown, E. J., Quezada, A., Wu, X., Voisin, M., et al. (2020). Neutrophil extracellular traps promote macrophage inflammation and impair atherosclerosis resolution in diabetic mice. JCI Insight 5 (7), 134796. doi:10.1172/jci.insight.134796
Kim, M. J., Kang, H. H., Seo, Y. J., Kim, K. M., Kim, Y. J., Jung, S. K., et al. (2021). Paeonia lactiflora root extract and its components reduce biomarkers of early atherosclerosis via anti-inflammatory and antioxidant effects in vitro and in vivo. Antioxidants 10 (10), 1507. doi:10.3390/antiox10101507
Kim, S. H., Kim, S. A., Park, M. K., Kim, S. H., Park, Y. D., Na, H. J., et al. (2004). Paeonol inhibits anaphylactic reaction by regulating histamine and TNF-alpha. Int. Immunopharmacol. 4 (2), 279–287. doi:10.1016/j.intimp.2003.12.013
Kishimoto, Y., Tani, M., Uto-Kondo, H., Iizuka, M., Saita, E., Sone, H., et al. (2010). Astaxanthin suppresses scavenger receptor expression and matrix metalloproteinase activity in macrophages. Eur. J. Nutr. 49 (2), 119–126. doi:10.1007/s00394-009-0056-4
Klionsky, D. J., and Codogno, P. (2013). The mechanism and physiological function of macroautophagy. J. Innate Immun. 5 (5), 427–433. doi:10.1159/000351979
Koo, Y. K., Kim, J. M., Koo, J. Y., Kang, S. S., Bae, K., Kim, Y. S., et al. (2010). Platelet anti-aggregatory and blood anti-coagulant effects of compounds isolated from Paeonia lactiflora and Paeonia suffruticosa. Pharmazie 65 (8), 624–628. doi:10.1691/ph.2010.9870
Kumarswamy, R., Volkmann, I., and Thum, T. (2011). Regulation and function of miRNA-21 in health and disease. RNA Biol. 8 (5), 706–713. doi:10.4161/rna.8.5.16154
Labrecque, L., Lamy, S., Chapus, A., Mihoubi, S., Durocher, Y., Cass, B., et al. (2005). Combined inhibition of PDGF and VEGF receptors by ellagic acid, a dietary-derived phenolic compound. Carcinogenesis 26 (4), 821–826. doi:10.1093/carcin/bgi024
Lacolley, P., Regnault, V., Segers, P., and Laurent, S. (2017). Vascular smooth muscle cells and arterial stiffening: Relevance in development, aging, and disease. Physiol. Rev. 97 (4), 1555–1617. doi:10.1152/physrev.00003.2017
Laddha, A. P., and Kulkarni, Y. A. (2019). VEGF and FGF-2: Promising targets for the treatment of respiratory disorders. Respir. Med. 156, 33–46. doi:10.1016/j.rmed.2019.08.003
Lawson, C., and Wolf, S. (2009). ICAM-1 signaling in endothelial cells. Pharmacol. Rep. 61 (1), 22–32. doi:10.1016/s1734-1140(09)70004-0
Lee, B., Shin, Y. W., Bae, E. A., Han, S. J., Kim, J. S., Kang, S. S., et al. (2008). Antiallergic effect of the root of Paeonia lactiflora and its constituents paeoniflorin and paeonol. Arch. Pharm. Res. 31 (4), 445–450. doi:10.1007/s12272-001-1177-6
Lefrancais, E., Ortiz-Munoz, G., Caudrillier, A., Mallavia, B., Liu, F. C., Sayah, D. M., et al. (2017). The lung is a site of platelet biogenesis and a reservoir for haematopoietic progenitors. Nature 544 (7648), 105–109. doi:10.1038/nature21706
Libby, P., Ridker, P. M., and Hansson, G. K. (2011). Progress and challenges in translating the biology of atherosclerosis. Nature 473 (7347), 317–325. doi:10.1038/nature10146
Li, C., Yang, L., Wu, H. F., and Dai, M. (2018). Paeonol inhibits oxidized low-density lipoprotein-induced vascular endothelial cells autophagy by upregulating the expression of miRNA-30a. Front. Pharmacol. 9, 95. doi:10.3389/fphar.2018.00095
Li, H. K., Dai, M., and Jia, W. (2009). Paeonol attenuates high-fat-diet-induced atherosclerosis in rabbits by anti-inflammatory activity. Planta Med. 75 (1), 7–11. doi:10.1055/s-0028-1088332
Li, Q., Youn, J. Y., and Cai, H. (2015). Mechanisms and consequences of endothelial nitric oxide synthase dysfunction in hypertension. J. Hypertens. 33 (6), 1128–1136. doi:10.1097/HJH.0000000000000587
Li, X. Y., Zhou, Y. D., Yu, C., Yang, H., Zhang, C. Z., Ye, Y., et al. (2015). Paeonol suppresses lipid accumulation in macrophages via upregulation of the ATP-binding cassette transporter A1 and downregulation of the cluster of differentiation 36. Int. J. Oncol. 46 (2), 764–774. doi:10.3892/ijo.2014.2757
Li, Y., Dai, M., and Chen, P. (2015). Effects of paeonol on lipopolysaccharide-induced release of vascular endothelial cell adhesion molecule-1 and tumor necrosis factor-alpha and tlr4/nf-κb signaling pathway in rat vascular endothelial cells. J. Anhui Univ. Chin. Med. 34 (1), 46–50. doi:10.3969/j.issn.2095-7246.2015.01.016
Li, W., Ishihara, K., Yokota, T., Nakagawa, T., Koyama, N., Jin, J., et al. (2008). Reduced alpha4beta1 integrin/VCAM-1 interactions lead to impaired pre-B cell repopulation in alpha 1, 6-fucosyltransferase deficient mice. Glycobiology 18 (1), 114–124. doi:10.1093/glycob/cwm107
Liang, Y. B., Tang, H., Chen, Z. B., Zeng, L. J., Wu, J. G., Yang, W., et al. (2017). Downregulated SOCS1 expression activates the JAK1/STAT1 pathway and promotes polarization of macrophages into M1 type. Mol. Med. Rep. 16 (5), 6405–6411. doi:10.3892/mmr.2017.7384
Liao, X., Sluimer, J. C., Wang, Y., Subramanian, M., Brown, K., Pattison, J. S, et al. (2012). Macrophage autophagy plays a protective role in advanced atherosclerosis. Cell Metab 15 (4), 545-53. doi:10.1016/j.cmet.2012.01.022
Libby, P. (2021). The changing landscape of atherosclerosis. Nature 592 (7855), 524–533. doi:10.1038/s41586-021-03392-8
Liu, L., Jiang, T., Yang, Y., Shi, X., Liu, Y., Wu, H., et al. (2022). Paeonol inhibits inflammatory response of foam cells in atherosclerotic mice by regulating miR-145 Based on the Cluster of Differentiation 40/Nuclear Factor-kappa b pathway. J. Anhui Univ. Chin. Med. 41, 68–74. doi:10.3969/j.issn.2095-7246.2022.02.015
Liu, Y. R., Chen, J. J., and Dai, M. (2014). Paeonol protects rat vascular endothelial cells from ox-LDL-induced injury in vitro via downregulating microRNA-21 expression and TNF-α release. Acta Pharmacol Sin. 35 (4), 483–488. doi:10.1038/aps.2013.190
Liu, Y. J., Tang, B., Wang, F. C., Tang, L., Lei, Y. Y., Luo, Y., et al. (2020). Parthenolide ameliorates colon inflammation through regulating Treg/Th17 balance in a gut microbiota-dependent manner. Theranostics 10 (12), 5225–5241. doi:10.7150/thno.43716
Liu, Y. R., Li, C., Wu, H. F., Xie, X. M., Sun, Y., Dai, M., et al. (2018). Paeonol attenuated inflammatory response of endothelial cells via stimulating monocytes-derived exosomal MicroRNA-223. Front. Pharmacol. 9, 1105. doi:10.3389/fphar.2018.01105
Liu, Y. R., Song, A. W., Wu, H. F., Sun, Y., and Dai, M. (2021). Paeonol inhibits apoptosis of vascular smooth muscle cells via up-regulation of autophagy by activating class III PI3K/Beclin-1 signaling pathway. Life Sci. 264, 118714. doi:10.1016/j.lfs.2020.118714
Liu, Y., Wu, H., and Dai, M. (2018). Effects of paeonol on the release of tumor necrosis factor-αfrom vascular endothelial cells with lipopolysaccharide-induced injury and the apoptosis of vascular smooth muscle cells in a Co-culture system and its influence on the p38MAPK signaling pathway. J. Anhui Univ. Chin. Med. 37, 65–71. doi:10.3969/j.issn.2095-7246.2018.04.018
Lindahl, B., Toss, H., Siegbahn, A., Venge, P., and Wallentin, L. (2000) Markers of myocardial damage and inflammation in relation to long-term mortality in unstable coronary artery disease. FRISC Study Group. Fragmin during Instability in Coronary Artery Disease. N. Engl. J. Med. 343 (16), 1139-1147. doi:10.1056/NEJM200010193431602
Liuzzo, G., Biasucci, L. M., Gallimore, J. R., Grillo, R. L., Rebuzzi, A. G., Pepys, M. B., Maseri, A., et al. (1994) The prognostic value of C-reactive protein and serum amyloid a protein in severe unstable angina. N. Engl. J. Med. 331 (7), 417-424. doi:10.1056/NEJM199408183310701
Long, G. W., Wang, F., Li, H. P., Yin, Z. W., Sandip, C., Lou, Y., et al. (2013). Circulating miR-30a, miR-126 and let-7b as biomarker for ischemic stroke in humans. BMC Neurol. 13, 178. doi:10.1186/1471-2377-13-178
Lu, L., Qin, Y., Chen, C., and Guo, X. (2018). Beneficial effects exerted by paeonol in the management of atherosclerosis. Oxid Med. Cell Longev. 1098617. doi:10.1155/2018/1098617
Ma, L., Chuang, C. C., Weng, W., Zhao, L., Zheng, Y., Zhang, J., et al. (2016). Paeonol protects rat heart by improving regional blood perfusion during No-reflow. Front. Physiol. 7, 298. doi:10.3389/fphys.2016.00298
Ma, T., Tian, X., Zhang, B. D., Li, M. Q., Wang, Y., Yang, C. Y., et al. (2022). Low-dose metformin targets the lysosomal AMPK pathway through PEN2. Nature 603 (7899), 159–165. doi:10.1038/s41586-022-04431-8
Mendis, S., Davis, S., and Norrving, B. (2015). Organizational update the world health organization global status report on noncommunicable diseases 2014; one more landmark step in the combat against stroke and vascular disease. Stroke 46 (5), E121–E122. doi:10.1161/STROKEAHA.115.008097
Meng, L., Xu, W. D., Guo, L. H., Ning, W. Q., and Zeng, X. D. (2015). Paeonol inhibits the proliferation, invasion, and inflammatory reaction induced by TNF-alpha in vascular smooth muscle cells. Cell. biochem. Biophys. 73 (2), 495–503. doi:10.1007/s12013-015-0686-5
Meng, Y., Liu, Z., Zhai, C., Di, T., Zhang, L., Zhang, L., et al. (2019). Paeonol inhibits the development of 1-chloro-2, 4-dinitrobenzene-induced atopic dermatitis via mast and T cells in BALB/c mice. Mol. Med. Rep. 19 (4), 3217–3229. doi:10.3892/mmr.2019.9985
Min, C.-Y., Liu, H.-Q., Zhan, F., and Qiu, W.-Z. (2007). Effect of cortex moutan on PGI2, TXA2, ET and NO in diabetic rats. Zhong yao Cai. 30 (6), 687–690. doi:10.13863/j.issn1001-4454.2007.06.029
Mizia-Stec, K. (2006) Cytokines and adhesive molecules in detection of endothelial dysfunction. Pharmacol. Rep.58, 21–32.
Moore, C. S., Rao, V. T. S., Durafourt, B. A., Bedell, B. J., Ludwin, S. K., Bar-Or, A., et al. (2013). miR-155 as a multiple sclerosis-relevant regulator of myeloid cell polarization. Ann. Neurol. 74 (5), 709–720. doi:10.1002/ana.23967
Muller, P. A. J., and Vousden, K. H. (2014). Mutant p53 in cancer: New functions and therapeutic opportunities. Cancer Cell. 25 (3), 304–317. doi:10.1016/j.ccr.2014.01.021
Nagele, M. P., Haubner, B., Tanner, F. C., Ruschitzka, F., and Flammer, A. J. (2020). Endothelial dysfunction in COVID-19: Current findings and therapeutic implications. Atherosclerosis 314, 58–62. doi:10.1016/j.atherosclerosis.2020.10.014
National Pharmacopoeia Commission (2020). Chinese pharmacopoeia Part I. Beijing: China Medical Science and Technology Press, 179.
Newman, D. J., and Cragg, G. M. (2016). Natural products as sources of new drugs from 1981 to 2014. J. Nat. Prod. 79 (3), 629–661. doi:10.1021/acs.jnatprod.5b01055
Nicola, H., and Ho, K. M. (2019). Aspirin resistance incidence and associations between aspirin effect and outcomes in cardiac surgery. Ann. Thorac. Surg. 108 (6), 1815–1821. doi:10.1016/j.athoracsur.2019.04.114
Nikolic, I., Plate, K.-H., and Schmidt, M. H. H. (2010). EGFL7 meets miRNA-126: An angiogenesis alliance. J. Angiogenes. Res. 2 (1), 9. doi:10.1186/2040-2384-2-9
Nizamutdinova, I. T., Oh, H. M., Min, Y. N., Park, S. H., Lee, M. J., Kim, J. S., et al. (2007). Paeonol suppresses intercellular adhesion molecule-1 expression in tumor necrosis factor-alpha-stimulated human umbilical vein endothelial cells by blocking p38, ERK and nuclear factor-kappaB signaling pathways. Int. Immunopharmacol. 7 (3), 343–350. doi:10.1016/j.intimp.2006.11.004
Ortmann, J., Veit, M., Zingg, S., Di Santo, S., Traupe, T., Yang, Z. J., et al. (2011). Estrogen receptor-alpha but not -beta or GPER inhibits high glucose-induced human VSMC proliferation: Potential role of ROS and ERK. J. Clin. Endocrinol. Metab. 96 (1), 220–228. doi:10.1210/jc.2010-0943
Pan, L. L., and Dai, M. (2009). Paeonol from Paeonia suffruticosa prevents TNF-alpha-induced monocytic cell adhesion to rat aortic endothelial cells by suppression of VCAM-1 expression. Phytomedicine 16 (11), 1027–1032. doi:10.1016/j.phymed.2009.04.003
Pi, X. C., Yan, C., Zheng, Q. L., Tai, L. K., and Berk, B. C. (2003). Big mitogen-activated protein kinase (BMK1)/ERK5 protects endothelial cells from apoptosis. Faseb J. 17 (5), A1242. doi:10.1161/01.RES.0000112406.27800.6F
Ping, M., Xiao, W., Mo, L. Q., Xiao, X. Y., Song, S. L., Tang, W. J., et al. (2014). Paeonol attenuates advanced oxidation protein product-induced oxidative stress injury in THP-1 macrophages. Pharmacology 93 (5-6), 286–295. doi:10.1159/000363577
Pirillo, A., Norata, G. D., and Catapano, A. L. (2013). LOX-1, OxLDL, and atherosclerosis. Mediat. Inflamm. 2013, 152786. doi:10.1155/2013/152786
Qin, C. C., and Liu, Z. H. (2007). In atherogenesis, the apoptosis of endothelial cell itself could directly induce over-proliferation of smooth muscle cells. Med. Hypotheses 68 (2), 275–277. doi:10.1016/j.mehy.2006.07.037
Rodriguez-Feo, J. A., Hellings, W. E., Moll, F. L., De Vries, J., van Middelaar, B. J., Algra, A., et al. (2008). Caveolin-1 influences vascular protease activity and is a potential stabilizing factor in human atherosclerotic disease. Plos One 3 (7), e2612. doi:10.1371/journal.pone.0002612
Ryter, S. W., Lee, S. J., Smith, A., and Choi, A. M. (2010). Autophagy in vascular disease. Proc Am Thorac Soc7 (1), 40–7. doi:10.1513/pats.200909-100JS
Saeed, O., Otsuka, F., Polavarapu, R., Karmali, V., Weiss, D., Davis, T., et al. (2012). Pharmacological suppression of hepcidin increases macrophage cholesterol efflux and reduces foam cell formation and atherosclerosis. Arterioscler. Thromb. Vasc. Biol. 32 (2), 299–307. doi:10.1161/ATVBAHA.111.240101
Sawamura, T., Kume, N., Aoyama, T., Moriwaki, H., Hoshikawa, H., Aiba, Y., et al. (1997). An endothelial receptor for oxidized low-density lipoprotein. Nature 386 (6620), 73–77. doi:10.1038/386073a0
Shi, L., Fan, P. S., Fang, J. X., and Han, Z. X. (1988). Inhibitory effects of paeonol on experimental atherosclerosis and platelet aggregation of rabbit. Zhongguo yao li xue Bao. 9 (6), 555–558.
Shi, X. Y., Huang, H. W., Zhou, M., Liu, Y. R., Wu, H. F., Dai, M., et al. (2021). Paeonol attenuated vascular fibrosis through regulating Treg/Th17 balance in a gut microbiota-dependent manner. Front. Pharmacol. 12, 12. doi:10.3389/fphar.2021.765482
Shi, X. Y., Xie, X. M., Sun, Y., He, H., Huang, H. W., Liu, Y. R., et al. (2020). Paeonol inhibits NLRP3 mediated inflammation in rat endothelial cells by elevating hyperlipidemic rats plasma exosomal miRNA-223. Eur. J. Pharmacol. 885, 173473. doi:10.1016/j.ejphar.2020.173473
Shintani, T., and Klionsky, D. J. (2004). Autophagy in health and disease: A double-edged sword. Science 306 (5698), 990–995. doi:10.1126/science.1099993
Singh, S., Anshita, D., and Ravichandiran, V. (2021). MCP-1: Function, regulation, and involvement in disease. Int. Immunopharmacol. 101, 107598. doi:10.1016/j.intimp.2021.107598
Slobodin, G., and Rimar, D. (2017). Regulatory T cells in systemic sclerosis: A comprehensive review. Clin. Rev. Allergy Immunol. 52 (2), 194–201. doi:10.1007/s12016-016-8563-6
Song, A., Wu, H., and Dai, M. (2018). Paeonol attenuates progression of atherosclerotic lesion formation through lipid regulation, anti-inflammatory and antioxidant activities. J. Chin. Pharm. Sci. 27, 565–575. doi:10.5246/jcps.2018.08.057
Sugiyama, D., Okamura, T., Watanabe, M., Higashiyama, A., Okuda, N., Nakamura, Y., et al. (2015). Risk of hypercholesterolemia for cardiovascular disease and the population attributable fraction in a 24-year Japanese cohort study. J. Atheroscler. Thromb. 22 (1), 95–107. doi:10.5551/jat.25908
Sugiyama, K., Muroi, M., and Tanamoto, K. (2008). A novel TLR4-binding peptide that inhibits LPS-induced activation of NF-kappaB and in vivo toxicity. Eur. J. Pharmacol. 594 (1-3), 152–156. doi:10.1016/j.ejphar.2008.07.037
Sui, L., Wang, J., and Li, B. M. (2008). Role of the phosphoinositide 3-kinase-Akt-mammalian target of the rapamycin signaling pathway in long-term potentiation and trace fear conditioning memory in rat medial prefrontal cortex. Learn. Mem. 15 (10), 762–776. doi:10.1101/lm.1067808
Sun, Y., Liu, L., Shi, X.-Y., He, H., Huang, H.-W., Dai, M., et al. (2020). Paeonol inhibits macrophage M1 polarization by down-regulating miR-155/JAK1-STAT1 pathway. Zhongguo Zhong yao za Zhi. 45 (9), 2158–2164. doi:10.19540/j.cnki.cjcmm.20200210.409
Soysal, P., Arik, F., Smith, L., Jackson, S. E., and Isik, A. T. (2020). Inflammation, frailty and cardiovascular disease. Adv. Exp. Med. Biol.1216, 55–64. doi:10.1007/978-3-030-33330-0_7
Tai, S., Hu, X. Q., Peng, D. Q., Zhou, S. H., and Zheng, X. L. (2016). The roles of autophagy in vascular smooth muscle cells. Int. J. Cardiol. 211, 1–6. doi:10.1016/j.ijcard.2016.02.128
Tang, H. Q., Li, K. M., Zhang, S. T., Lan, H. Q., Liang, L. L., Huang, C. H., et al. (2021). Inhibitory effect of paeonol on apoptosis, oxidative stress, and inflammatory response in human umbilical vein endothelial cells induced by high glucose and palmitic acid induced through regulating SIRT1/FOXO3a/NF-kappa B pathway. J. Interferon Cytokine Res. 41 (3), 111–124. doi:10.1089/jir.2019.0236
Trionfini, P., and Benigni, A. (2017). MicroRNAs as master regulators of glomerular function in health and disease. J. Am. Soc. Nephrol. 28 (6), 1686–1696. doi:10.1681/ASN.2016101117
Tsai, J. Y., Su, K. H., Shyue, S. K., Kou, Y. R., Yu, Y. B., Hsiao, S. H., et al. (2010). EGb761 ameliorates the formation of foam cells by regulating the expression of SR-A and ABCA1: Role of haem oxygenase-1. Cardiovasc. Res. 88 (3), 415–423. doi:10.1093/cvr/cvq226
Van Avondt, K., Maegdefessel, L., and Soehnlein, O. (2019). Therapeutic targeting of neutrophil extracellular traps in atherogenic inflammation. Thromb. Haemost. 119 (4), 542–552. doi:10.1055/s-0039-1678664
Vieira, R. A. L., de Freitas, R. N., and Volp, A. C. P. (2014). Adhesion molecules and chemokines: Relation to anthropometric, body composition, biochemical and dietary variables. Nutr. Hosp. 30 (2), 223–236. doi:10.3305/nh.2014.30.2.7416
Waksman, R., and Iantorno, M. (2018). Refractory in-stent restenosis: Improving outcomes by standardizing our approach. Curr. Cardiol. Rep. 20 (12), 140. doi:10.1007/s11886-018-1076-6
Wang, J., Zhou, J. Y., and Wu, G. S. (2007). ERK-dependent MKP-1-mediated cisplatin resistance in human ovarian cancer cells. Cancer Res. 67 (24), 11933–11941. doi:10.1158/0008-5472.CAN-07-5185
Wang, X. C., Jiang, Y. J., Zhu, L., Cao, L., Xu, W., Rahman, S. U., et al. (2020). Autophagy protects PC12 cells against deoxynivalenol toxicity via the Class III PI3K/beclin 1/Bcl-2 pathway. J. Cell. Physiol. 235 (11), 7803–7815. doi:10.1002/jcp.29433
Wang, Y. Q., Dai, M., Zhong, J. C., and Yin, D. K. (2012). Paeonol inhibits oxidized low density lipoprotein-induced monocyte adhesion to vascular endothelial cells by inhibiting the mitogen activated protein kinase pathway. Biol. Pharm. Bull. 35 (5), 767–772. doi:10.1248/bpb.35.767
Wang, Z. Q., He, C. N., Peng, Y., Chen, F. H., and Xiao, P. G. (2017). Origins, phytochemistry, pharmacology, analytical methods and safety of cortex moutan (paeonia suffruticosa Andrew): A systematic review. Molecules 22 (6), E946. doi:10.3390/molecules22060946
Weber, C., and Noels, H. (2011). Atherosclerosis: Current pathogenesis and therapeutic options. Nat. Med. 17 (11), 1410–1422. doi:10.1038/nm.2538
Wei, L. F., Zhang, H. M., Wang, S. S., Jing, J. J., Zheng, Z. C., Gao, J. X., et al. (2016). Changes of MDA and SOD in brain tissue after secondary brain injury with seawater immersion in rats. Turk. Neurosurg. 26 (3), 384–288. doi:10.5137/1019-5149.JTN.8265-13.1
Wei, Y. M., Li, X., Xu, M., Abais, J. M., Chen, Y., Riebling, C. R., Boini, K. M., Li, P. L., and Zhang, Y. (2013). Enhancement of autophagy by simvastatin through inhibition of Rac1-mTOR signaling pathway in coronary arterial myocytes. Cell. Physiol. Biochem. 31 (6), 925-37. doi:10.1159/000350111
Witko-Sarsat, V., Nguyen-Khoa, T., Jungers, P., Drueke, T. B., and Descamps-Latscha, B. (1999). Advanced oxidation protein products as a novel molecular basis of oxidative stress in uraemia. Nephrol. Dial. Transpl. 14, 76–78. doi:10.1093/ndt/14.suppl_1.76
Wu, H. F., Song, A. W., Hu, W. J., and Dai, M. (2018). The anti-atherosclerotic effect of paeonol against vascular smooth muscle cell proliferation by up-regulation of autophagy via the AMPK/mTOR signaling pathway. Front. Pharmacol. 8, 948. doi:10.3389/fphar.2017.00948
Wu, H., Su, X., and Dai, M. (2015). Paeonol inhibits over proliferation of VSMCs in ox-LDL-injured co-cultured system by suppression of ET-1 expression. Lat. Am. J. Pharm. 34, 1858–1865.
Wu, M., Yu, Z. L., Li, X. Y., Zhang, X. N., Wang, S. Z., Yang, S. J., et al. (2021). Paeonol for the treatment of atherosclerotic cardiovascular disease: A pharmacological and mechanistic overview. Front. Cardiovasc. Med. 8, 690116. doi:10.3389/fcvm.2021.690116
Xu, F., Kang, Y., Zhang, H., Piao, Z., Yin, H., Diao, R., et al. (2016). Akt1-mediated regulation of macrophage polarization in a murine model of Staphylococcus aureus pulmonary infection. J. Infect. Dis. 208 (3), 528–538. doi:10.1093/infdis/jit177
Xu, S., Ilyas, I., Little, P. J., Li, H., Kamato, D., Zheng, X., et al. (2021). Endothelial dysfunction in atherosclerotic cardiovascular diseases and beyond: From mechanism to pharmacotherapies. Pharmacol. Rev. 73 (3), 924–967. doi:10.1124/pharmrev.120.000096
Yin, J., Hou, X. H., and Yang, S. B. (2019). microRNA-338-3p promotes ox-LDL-induced endothelial cell injury through targeting BAMBI and activating TGF-/Smad pathway. J. Cell. Physiol. 234 (7), 11577–11586. doi:10.1002/jcp.27814
Yin, S. L., Wang, R., Zhou, F., Zhang, H., and Jing, Y. K. (2011). Bcl-xL is a dominant antiapoptotic protein that inhibits homoharringtonine-induced apoptosis in leukemia cells. Mol. Pharmacol. 79 (6), 1072–1083. doi:10.1124/mol.110.068528
Yu, Y. F., Yan, R., Chen, X. Z., Sun, T., and Yan, J. F. (2020). Paeonol suppresses the effect of ox-LDL on mice vascular endothelial cells by regulating miR-338-3p/TET2 axis in atherosclerosis. Mol. Cell. Biochem. 475 (1-2), 127–135. doi:10.1007/s11010-020-03865-w
Yuan, X. S., Chen, J. J., and Dai, M. (2016). Paeonol promotes microRNA-126 expression to inhibit monocyte adhesion to ox-LDL-injured vascular endothelial cells and block the activation of the PI3K/Akt/NF-kappa B pathway. Int. J. Mol. Med. 38 (6), 1871–1878. doi:10.3892/ijmm.2016.2778
Zare, N., Eskandari, N., Mehrzad, V., and Javanmard, S. H. (2019). The expression level of hsa-miR-146a-5p in plasma-derived exosomes of patients with diffuse large B-cell lymphoma. J. Res. Med. Sci. 24, 10. doi:10.4103/jrms.JRMS_507_18
Zhang, B., Ma, Y., and Xiang, C. (2018). SIRT2 decreases atherosclerotic plaque formation in low-density lipoprotein receptor-deficient mice by modulating macrophage polarization. Biomed. Pharmacother. 97, 1238–1242. doi:10.1016/j.biopha.2017.11.061
Zhang, L., Li, D. C., and Lin, L. F. (2019). Paeonol: Pharmacological effects and mechanisms of action. Int. Immunopharmacol. 72, 413–421. doi:10.1016/j.intimp.2019.04.033
Zhang, W., and Liu, H. T. (2002). MAPK signal pathways in the regulation of cell proliferation in mammalian cells. Cell. Res. 12 (1), 9–18. doi:10.1038/sj.cr.7290105
Zhang., L. X., Ma, C., Gu, R., Zhang, M., Wang, X. Y., Yang, L., et al. (2018). Paeonol regulates hypoxia-induced proliferation of pulmonary artery smooth muscle cells via EKR 1/2 signalling. Eur. J. Pharmacol. 834, 257–265. doi:10.1016/j.ejphar.2018.07.017
Zhao, J.-F., Jim Leu, S.-J., Shyue, S.-K., Su, K.-H., Wei, J., Lee, T.-S., et al. (2013). Novel effect of paeonol on the formation of foam cells: Promotion of lxrα-ABCA1–dependent cholesterol efflux in macrophages. Am. J. Chin. Med. 41 (05), 1079–1096. doi:10.1142/S0192415X13500730
Zhao, J. F., Ching, L. C., Huang, Y. C., Chen, C. Y., Chiang, A. N., Kou, Y. R., et al. (2012). Molecular mechanism of curcumin on the suppression of cholesterol accumulation in macrophage foam cells and atherosclerosis. Mol. Nutr. Food Res. 56 (5), 691–701. doi:10.1002/mnfr.201100735
Zhou, F. H., Pan, Y. Y., Huang, Z. Y., Jia, Y. H., Zhao, X. S., Chen, Y. Y., et al. (2013). Visfatin induces cholesterol accumulation in macrophages through up-regulation of scavenger receptor-A and CD36. Cell. Stress Chaperones 18 (5), 643–652. doi:10.1007/s12192-013-0417-z
Zhou, X., Niu, C., Cao, K., and Xu, Q. (2011). Paeonol reduces expression of adhesion molecules in HUVECs induced by hyperlipidemic serum via inhibiting the pathway of NF - KB signaling. Chin. J. Pathophysiol. 27 (2), 249–253. doi:10.3969/j.issn.1000-4718.2011.02-008
Zhou, Z., Subramanian, P., Sevilmis, G., Globke, B., Soehnlein, O., Karshovska, E., et al. (2011). Lipoprotein-derived lysophosphatidic acid promotes atherosclerosis by releasing CXCL1 from the endothelium. Eur. Heart J. 32, 517. doi:10.1016/j.cmet.2011.02.016
Zhu, H., Wu, H., Liu, X. P., Li, B. A., Chen, Y., Ren, X. C., et al. (2009). Regulation of autophagy by a beclin 1-targeted microRNA, miR-30a, in cancer cells. Autophagy 5 (6), 816–823. doi:10.4161/auto.9064
Keywords: atherosclerosis, cardiovascular disease, endothelial cell, macrophage, paeonol, platelet, smooth muscle cell
Citation: Yu W, Ilyas I, Aktar N and Xu S (2022) A review on therapeutical potential of paeonol in atherosclerosis. Front. Pharmacol. 13:950337. doi: 10.3389/fphar.2022.950337
Received: 22 May 2022; Accepted: 05 July 2022;
Published: 04 August 2022.
Edited by:
Yi-Hung Chen, China Medical University, TaiwanReviewed by:
Hongfei Wu, Anhui University of Chinese Medicine, ChinaPin-Kuei Fu, Taichung Veterans General Hospital, Taiwan
Copyright © 2022 Yu, Ilyas, Aktar and Xu. This is an open-access article distributed under the terms of the Creative Commons Attribution License (CC BY). The use, distribution or reproduction in other forums is permitted, provided the original author(s) and the copyright owner(s) are credited and that the original publication in this journal is cited, in accordance with accepted academic practice. No use, distribution or reproduction is permitted which does not comply with these terms.
*Correspondence: Wei Yu, eXV3ZWk1MjIxM0AxNjMuY29t; Suowen Xu, c3h1MTk4NEB1c3RjLmVkdS5jbg==