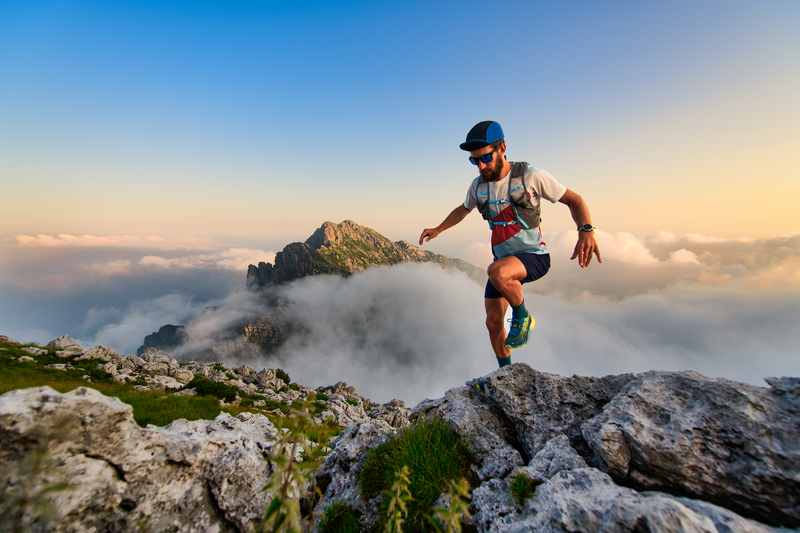
94% of researchers rate our articles as excellent or good
Learn more about the work of our research integrity team to safeguard the quality of each article we publish.
Find out more
ORIGINAL RESEARCH article
Front. Pharmacol. , 31 August 2022
Sec. Pharmacology of Infectious Diseases
Volume 13 - 2022 | https://doi.org/10.3389/fphar.2022.936295
The treatment for tuberculosis (TB), especially multidrug-resistant TB (MDR-TB), has a prolonged cycle which can last up to a year. This is partially due to the lack of effective therapies. The development of novel anti-TB drugs from the perspective of host immune regulation can provide an important supplement for conventional treatment strategies. Salidroside (SAL), a bioactive component from the Tibetan medicine Rhodiola rosea, has been used in the treatment of TB, although its mechanism remains unclear. Here, the bacteriostatic effect of SAL in vivo was first demonstrated using a zebrafish–M. marinum infection model. To further investigate the underlying mechanism, we then examined the impact of SAL on immune cell recruitment during wound and infection. Increased macrophage and neutrophil infiltrations were found both in the vicinity of the wound and infection sites after SAL treatment compared with control, which might be due to the elevated chemokine expression levels after SAL treatment. SAL treatment alone was also demonstrated to improve the survival of infected zebrafish larvae, an effect that was amplified when combining SAL treatment with isoniazid or rifampicin. Interestingly, the reduced bacterial burden and improved survival rate under SAL treatment were compromised in tnfα-deficient embryos which suggests a requirement of Tnfα signaling on the anti-mycobacterial effects of SAL. In summary, this study provides not only the cellular and molecular mechanisms for the host anti-mycobacterial effects of the Tibetan medicine SAL but also proof of concept that combined application of SAL with traditional first-line anti-TB drugs could be a novel strategy to improve treatment efficacy.
TB is still the leading infectious disease cause of death by a single pathogen (exclude the impact of COVID-19), and the spread of drug-resistant TB internationally has become an urgent problem for global public health (WHO, 2021). Multidrug-resistant TB or rifampicin-resistant TB (MDR/RR-TB) usually requires extended treatment with a failure rate of 40%, and remains a serious public health concern for developing countries, such as India, Indonesia, and China (WHO, 2021). Due to the limited therapy choices and unpleasant side effects of prolonged treatment for MDR-TB and extensively drug-resistant TB (XDR-TB), the call to develop safe and effective new anti-tuberculosis drugs is compelling.
It has been well documented that the host immune response has great impact on the outcome of TB infection (Tobin et al., 2012; Roca and Ramakrishnan, 2013; Mayer-Barber et al., 2014; Boisson-Dupuis et al., 2015; Niu et al., 2022). Thus, developing novel anti-TB drugs from the perspective of immune regulation can provide an important supplement to traditional bactericidal treatment. SAL is considered to be one of the major active compound extracted from the root of Rhodiola rosea, a natural herbal medicine in Tibet used to alleviate high-altitude sickness (Lee et al., 2013a; Lee et al., 2013b). This root has also been used to boost the immune system (Zhao et al., 2013), as an anti-inflammatory reagent (Yang et al., 2016), to treat fatigue (Ma et al., 2015) and solid cancer (Ding et al., 2020) and as an anti-aging medication (Lu et al., 2013). The application of Rhodiola rosea for pulmonary infections can be traced back to the end of the eighth century AD, and it is recorded in this Medical Canon in Four Sections (Si Bu Yi Dian) (Yutuo.Yundangongbu, 1982). Studies have shown that Rhodiola Rosea can increase the CD4/CD8 ratio in the peripheral blood of patients with tuberculosis, which might be responsible for alleviating TB symptoms after treatment (Gang, 2009). However, whether SAL also has a protective effect during mycobacterial infection and the underlying mechanism remain unclear.
The zebrafish–M. marinum infection model is ideal for the study of host immune responses against mycobacterial infection (Cronan and Tobin, 2014; Benard et al., 2016; Myllymaki et al., 2016; Ramakrishnan, 2020). Zebrafish and humans have highly conserved immune systems (Trede et al., 2004; Gomes and Mostowy, 2020), and zebrafish are the natural host for M. marinum. About 85% virulent factors in M. tb can also be found in the M. marinum genome (Stinear et al., 2008). Particularly, zebrafish has been widely accepted to be an excellent model system for high-throughput anti-tuberculosis compound screening (Dal et al., 2021). In this study, we will take the advantages of the zebrafish–M. marinum infection model to explore the effect of SAL on mycobacterial infection.
The wild-type (WT) AB, Tg (mpeg1:loxP-GFP) (Yu et al., 2017), Tg (mpeg1:loxP-DsRedx-loxP-GFP)hkz015t (Xu et al., 2016), and tnfα−/− (Xie Lingling et al., 2017) were maintained under standard conditions in the zebrafish facility of SPHCC as previously described (Wang et al., 2019). All embryos, except for survival experiments, were placed in egg water with 0.22 μM N-phenylthiourea (PTU) (Sigma-Aldrich) to prevent the formation of pigments (Wang et al., 2019).
M. marinum M strain (ATCC BAA-535, labeled with Wasabi fluorescence) was grown in the 7H9 liquid medium at 32°C as previously described (Niu et al., 2019).
Three-dpf zebrafish embryos were infected (∼250 CFU) via the duct of Cuvier by micro-injection unless otherwise indicated (Niu et al., 2022).
Isoniazid (INH) (Aladdin), rifampicin (RIF) (Sigma), and SAL (Shidande) were dissolved in egg water to make working concentrations. For the early myeloid cell development and wound response experiments, the embryos were treated with SAL 36 h before the assays. For infection with M. marinum, the embryos were treated with INH, RIF, or SAL after bacterial injection.
For this test, 3-dpf wild-type embryos were treated with different concentrations of INH and SAL. Feeding started at 5 dpf with a dry larval diet (AP100, Zeigler) twice per day, and the development or death of the embryos was monitored daily until 8 dpf.
MIC experiments were performed using a 7H10 agar plate (BD) with a serial of SAL and INH concentrations. The plates were grown at 32°C for about 8 days and then observed for colony growth (Wiegand et al., 2008).
Raw264.7 cells were cultured in 100 mm cell culture dishes, and SAL (1.6 mM) was added 36 h before infection. The Raw264.7 cells were plated in 24-well plates at 3 × 105 cells/well 12 h before infection and then infected with a single-cell suspension of green fluorescent-labeled M. marinum-Wasabi (MOI = 1). The infected Raw264.7 cells were incubated at 32°C and 5% CO2. The plates were imaged using BioTek Cytation 5. Images were taken with a ×20 objective at 2 hpi, 24 hpi, and 48 hpi.
The embryos were pretreated with 1.6 mM SAL or vehicle at 1.5 dpf. The embryos were placed in egg water with 0.02% tricaine (Sigma-Aldrich) in a sterile Petri dish, and tail fin amputation was performed using a sterile 24-gauge needle (Yan et al., 2014). RNA was extracted from the caudal fin for further analysis of cytokine expression.
For infection-induced macrophage and neutrophil recruitment assay, embryos were pre-treated with 1.6 mM SAL or vehicle 36 h before the assays. Three-dpf zebrafish embryos were anesthetized with tricaine (Sigma, 200 μg/ml) and mounted in 1% agarose for subcutaneous infection (∼300 CFU) (Niu et al., 2019). After infection, the embryos were washed into pre-warmed egg water and placed into a 28.5°C incubator immediately. The embryos were fixed at 2 hpi and then applied for macrophage and neutrophil staining assays.
The embryos were fixed for 2 h at room temperature and then washed with PBST for 4 × 5 min. The embryos were then stained with Sudan Black (Sangon Biotech) staining solution for 25 min at room temperature and washed with 70% ethanol as previously reported (Wang et al., 2019).
The antibody staining was performed as previously described (Wang et al., 2019). Briefly, the 3-dpf embryos were fixed and then stained with the goat polyclonal anti-GFP (Abcam, AB6658, 1:500) or anti-DsRed (TaKaRa, 632496, 1:500) primary antibody. The primary antibody was washed out and then stained with AF488 donkey anti-goat (Invitrogen, A11055, 1:500) or AF594 donkey anti-rabbit IgG (H + L) (YEASEN, A05121, 1:500) secondary antibodies, accordingly.
Fluorescent images were taken by Leica DMi8 microscopy. Bacterial burden was quantified by measuring the integrated fluorescent intensity of Wasabi-labeled bacteria with Fiji ImageJ software. Fluorescent-labeled macrophage areas were calculated by measuring the fluorescent area with Fiji ImageJ software.
Embryos were infected with M. marinum: Wasabi (∼250 CFU) and placed in egg water with different concentrations of SAL, INH, RIF, SAL plus INH, and SAL plus RIF. Feeding started at 5 dpf with the AP100 larval diet (Zeigler) twice per day. The numbers of dead zebrafish were recorded every day until 14 dpi.
Total RNA was extracted from zebrafish wounded caudal fins or whole embryos using TRIzol (Ambion). The PrimeScript reverse transcription kit (TaKaRa) was used for cDNA synthesis, and quantitative RT-PCR was performed using SYBR Green Supermix (Promega) to determine relative gene expression. The primer sequences (Genewiz) used are as follows: ef1a-F: 5′-CTTCTCAGGCTGACTGTGC-3′; ef1a-R: 5′-CCGCTAGCATTACCCTCC-3′; il1β-F: 5′GTACTCAAGGAGATCAGCGG-3′; il1β-R: 5′-CTCGGTGTCTTTCCTGTCCA-3′; tnfα-F: 5′-GCTGGTGATAGTGTCCAGGAG-3′; tnfα-R: 5′-TTGATTGCCCTGGGTCTTATG-3′; il6-F: 5′-CCTCAAACCTTCAGACCGCT-3′; il6-R: 5′-CTGGCTGTTTATGGCCTCCA-3′; ccl2-F: 5′-GTCTGGTGCTCTTCGCTTTC-3′; ccl2-R: 5′- TGCAGAGAAGATGCGTCGTA-3′; cxcl8-l1-F: 5′-TGTTTTCCTGGCATTTCTGACC-3′; cxcl8-l1-R: 5′-TTTACAGTGTGGGCTTGGAGGG-3′; cxcl8-l2-F:5′-CCACACACACTCCACACACA-3′; cxcl8-l2-R: 5′- CCACTGAATTGTCCTTTCATCA-3′; cxcl11a-F: 5′-ACTCAACATGGTGAAGCCAGTGCT-3′; and cxcl11a-R: 5′-CTTCAGCGTGGCTATGACTTCCAT-3′ (Yan et al., 2014; Wang et al., 2019; Sommer et al., 2021).
GraphPad Prism 8.0.2 was applied for statistical analyses. The results are shown as mean ± SEM. Differences were analyzed by using the two-tailed student’s t-test for comparisons between two groups, and a one-way/two-way ANOVA for multiple groups. Zebrafish mortality and survival data were analyzed using the log rank (Mantel–Cox) test (Dong et al., 2021). Statistical significance was indicated as follows: *, p < 0.05; **, p < 0.01; ***, p < 0.001; and ns, not statistically significant (p > 0.05).
Because toxic side effects often appear after prolonged administration of anti-TB drugs (Nunn et al., 2019), the toxicity of SAL on zebrafish embryos was first assessed using INH as the control. Based on previous reports and our preliminary results (Dharra et al., 2017; Reingewertz et al., 2020), we first selected the ranges of 0.075–1.2 mM and 0.8–12.8 mM for toxicity testing, respectively. A dose-dependent toxicity effect can be observed with concentrations of INH above 0.6 mM, while treatment with SAL showed no death or deformity phenotypes (Figures 1A,B; Supplementary Table S1). These results indicate that SAL treatment (≤12.8 mM) has no toxic effects on zebrafish embryonic development.
FIGURE 1. SAL has no obvious teratogenic effect on the growth and development of zebrafish. (A) Representative photos for zebrafish embryos exposed to different concentrations of INH and SAL (4 dpt). (B) Mortality rates of zebrafish embryos exposed to different concentrations of INH and SAL from 0 to 8 dpf. A and B; n = 20 per group. This is one representative data from three independent repeats.
In order to study the impact of SAL on mycobacterial infection, we first tested whether SAL has direct anti-mycobacterial activity in vitro. M. marinum was cultured on 7H10 agar plates containing different concentrations of INH and SAL for about 8 days to determine the MIC (Figures 2A,B). The results showed the INH MIC to be 0.01875 mM, similar to that reported previously (Reingewertz et al., 2020). However, SAL showed no direct anti-mycobacterial activity regardless of concentration. The in vivo anti-mycobacterial activity of SAL was next assessed using a zebrafish–M. marinum infection model. INH (≥0.0375 mM) showed significant dose-dependent bactericidal activity. However, SAL treatment only showed a slight but significant decrease in green fluorescence intensity (Figures 2C,D; Supplementary Figure S1). The anti-mycobacterium effect of SAL was also confirmed by a rodent macrophage infection model (Figures 2E,F). Together, these data suggest SAL may convey bacteriostatic activity via the host immune system.
FIGURE 2. SAL has bacteriostatic activity in vivo but not in vitro. (A) Images showing the growth of M. marinum on 7H10 plates containing different concentrations of INH and SAL after 8 days incubation. (B) Statistics of the colony number in (A). (C) Representative images for the distribution of M. marinum (Wasabi) in different groups of zebrafish at 72 hpi. Scale bar: 200 μm. (D) Statistics of integrated green fluorescence intensity in (C). Values are shown as mean ± s.e.m. One-way ANOVA for comparison at the same time point. Two-way ANOVA for co-operation at different time points. (E) M. marinum-Wasabi-infected RAW cells with or without SAL treatment. (F) Statistics of relative fluorescence units (RFU) of total (extracellular and intracellular) bacteria in (E). A and B are one representative data from three independent repeats. C and D are one representative data from two independent repeats. E and F are one representative data from three independent repeats.
To confirm our hypothesis that SAL inhibits bacterial proliferation via host immune regulation, we further investigated the impact of SAL on immune system development and wound response. No significant difference was observed in neutrophil and macrophage numbers between SAL-treated and control groups (Figures 3A–D), which suggests that SAL treatment does not have a major effect on early myeloid development. We then looked into the effects of SAL on the immune response during non-infectious injury. Interestingly, the numbers of neutrophils and macrophages recruited to wound sites in the SAL treatment group were significantly higher than those in the untreated group (Figures 3E–G). We further found that the expression of pro-inflammatory cytokines and chemokines was increased in the SAL-treated group (Figures 3H,I). Consistently, an increased macrophage and neutrophil infiltration was also observed after SAL treatment (Figure 3J–L). Our data suggest that SAL does not impact general myeloid development but can promote innate immune cell recruitment in response to injury and infection.
FIGURE 3. SAL promotes the recruitment of innate immune cells to the wound and infection sites. (A) Images of whole body SB staining of 3-dpf embryos. (B) Fluorescent images of 3-dpf Tg (mpeg1:loxP-GFP) embryos. A and B Scale bar: 200 μm. (C) Statistics of the number of SB+ neutrophils in A. (D) Quantification of total macrophage fluorescent area in B. (E) Schematic view of tail amputation experiment and the tissue for RNA extraction (upper panel); the recruitment of SB+ neutrophils to wound (left panel); and the recruitment of mpeg1:loxp:GFP+ macrophages to wound (right panel). Scale bar: 50 μm. (F) Statistics of the number of SB+ neutrophils in E. (G) Statistics of the number of macrophages in E. (H) Expression of various cytokines in the tail region at 2 hpa. (I) Expression of macrophage and neutrophil recruitment-related chemokines in the tail region at 2 hpa. (J) Images of the SB+ neutrophils (left panel) and mpeg1:LRLG+ macrophages (right panel) infiltration to infection sites. Scale bar: 50 μm. (K) Statistics of the number of SB+ neutrophils in J. (L) Statistics of the number of macrophages in J. A–G, J–L: n = 20 per group; H and I: n = 30 per sample. This is one representative data from three independent biological replicates.
Due to the different anti-mycobacterial mechanisms of each drug, we speculate that the combination of SAL and first-line anti-TB drugs may improve anti-mycobacterial efficacy. Bacterial burdens and survival curves were monitored after single or combined treatments. Consistent with the in vivo bacteriostatic activity, SAL treatment could only significantly improve the survival rates (Figure 4A). However, the bacterial fluorescence intensity did not show a significant difference between INH only and combined treatment groups (Figures 4B,C). Excitingly, although the combination of INH and SAL improved the survival rates as compared with INH treatment alone, regardless of the INH concentration, this improvement was only significant in low-concentration INH-treated groups (Figure 4A). A similar effect on survival rates was also observed when zebrafish embryos were treated with RIF and SAL (Supplementary Figure S2). These results imply that the improvement in bacterial clearance and increase in survival by the combined application of first-line anti-TB therapies with the traditional Tibetan medicine SAL may be due to their distinct anti-mycobacterial mechanisms.
FIGURE 4. Combined treatment of SAL and INH significantly improve the survival of zebrafish during mycobacterial infection. (A) Survival rates of M. marinum-infected zebrafish embryos treated with various concentrations of INH and SAL. n = 20 per group. (B) Representative images for the distribution of M. marinum (Wasabi) in different groups of zebrafish embryos at 72 hpi. Scale bar: 200 μm. (C) Statistics of integrated green fluorescence intensity in (B). One-way ANOVA was applied for the comparison. (D) Expression of two potent pro-inflammatory cytokines at 12 hpi. n = 30 per sample. A and D: data are representative of three independent biological replicates. B and C data are representative of two independent repeats.
The success of the host anti-tuberculosis response depends on tight control of the expression of pro-inflammatory cytokines such as TNFα and IL1β (Cooper et al., 2011). Thus, we examined RNA expression of these potent cytokines in infected zebrafish embryos after SAL treatment and observed a significant increase in tnfα and il1β compared to controls (Figure 4D). These findings suggest that the potential mechanism of SAL treatment against mycobacterial infection is inducing upregulation of pro-inflammatory cytokines.
Because Tnfα is a potent pro-inflammatory cytokine associated with mycobacterial infection, we tested whether the SAL-mediated protective effect required Tnfα signaling. Interestingly, both the differences between treatments in bacterial fluorescence intensity and the survival rates were diminished in tnfα-deficient embryos (Figures 5A–C), indicating that Tnfα signaling is required for SAL-mediated protection during mycobacterium infection.
FIGURE 5. Tnfα is required for SAL-mediated protective effects during mycobacterial infection. (A) Representative images for the distribution of M. marinum (Wasabi) in different groups of zebrafish embryos at 72 hpi. Scale bar: 200 μm. (B) Statistics of normalized green fluorescence intensity in A. One-way ANOVA was applied for the comparison. (C) Survival rates of M. marinum-infected zebrafish tnfα−/− embryos treated with various concentrations of INH and SAL. n = 20 per group. A, B, and C: data are representative of three independent repeats.
TB is one of the major infectious diseases endangering public health. Given the long treatment cycle and continual outbreaks of drug-resistant TB, there is an urgent need for the development of novel anti-TB therapies. SAL is a prominent bio-active extract of Rhodiola rosea and has been reported to play an important role in treating many immune system-related diseases (Qiu and Jia, 2014; Zheng et al., 2015; Hao et al., 2019). However, research concerning the application of SAL for the treatment of TB is limited. In this study, we used a zebrafish–M. marinum infection model to study the impact of SAL on mycobacterial infection. Our data show that while SAL has no direct anti-mycobacterial activity in vitro, it can significantly inhibit the bacterial proliferation in vivo and increase the survival of M. marinum-infected zebrafish. Excitingly, the combination of SAL and INH achieved better protective effects in controlling bacterial burden and improving the survival curve than either treatment alone. We have also shown that SAL can regulate immune cell recruitment during wound response and infection. The increased expression of pro-inflammatory cytokines and innate immune cell infiltration during infection could be the mechanisms of action for SAL’s protective effects during mycobacterial infection in vivo.
SAL has been reported to play a role in boosting the immune system. For example, it has been reported that adding SAL to the diet can enhance resistance against Aeromonas hydrophila infection in crucian carp (Carassius auratus) (Yang et al., 2020). Similarly, Navita Sharma et al. (2016) reported that SAL exerts its antiviral activity by increasing the expression of RNA helicases such as RIG-I, thereby initiating a downstream signaling cascade that induces upregulation of IRF-3 and IRF-7. However, the underlying molecular mechanisms for the SAL’s anti-infection role remain unclear. In this study, we first found that SAL is able to promote the recruitment of neutrophils and macrophages to the wound and infection sites and then demonstrated a positive regulatory role of SAL for the expression of pro-inflammatory cytokines during mycobacterial infection. Considering that zebrafish embryos only preserve the innate immune system, we can only dissect the impact of SAL on the innate immune system. Whether SAL also has a regulatory effect on adaptive immunity is worth further exploration.
In this study, we have observed significantly increased expression of pro-inflammatory cytokines after SAL treatment during infection, including tnfα and il1β. However, we did not observe a similar increase pattern during a wound response. It is possible that, compared with the impact of SAL on mycobacterial infection, which is a systematic response, the induced cytokine response to a localized tail amputation is too trivial to be detected. Similar findings were observed while studying the disease resistance role of SAL in common carp (Cyprinus carpio) and rainbow trout (Oncorhynchus mykiss) (Baba et al., 2018; Zemheri-Navruz et al., 2019). However, the research results of Guan et al. (2011) showed that SAL significantly reduces the production of TNFα, IL1β, and IL6 in the serum of mice stimulated by LPS. This inconsistency may be due to the different animal models chosen and the inflammatory stimuli applied. Thus, whether SAL plays distinct roles in different disease models requires further verification.
By utilizing tnfα-deficient zebrafish, we proved that the protective effect of SAL during M. marinum infection requires tnfα signaling (Figure 5C). We still cannot exclude the possibility that il1β, another significantly increased mycobacterial infection-related cytokine, also plays a role in SAL-mediated protection, which is worth further exploration in the future. Interestingly, there is no significant difference in the bacterial burden during the early stage of infection between tnfα-deficient embryos and wild-type controls, which is also consistent with previous reports (Figure 5B) (Clay et al., 2008; Xie Lingling et al., 2017). It is probably due to the dual role of tnfα on host control of infection and tissue damage (Tobin et al., 2010; Roca and Ramakrishnan, 2013). In an osteoarthritis rat model, it was found that SAL can regulate the inflammatory and immune responses of the rats through the NF-κB pathway (Gao et al., 2020). This suggests that SAL may act on host anti-mycobacterial responses via the NF-κB–Tnfα axis. Interestingly, the impact of SAL on the anti-mycobacterial responses does not work in a simple dose-dependent manner. No significant improvement in the anti-mycobacterial effect was further observed upon increasing the dose, once the concentration of SAL reaches the effective concentration. It suggests that a more complicated regulatory mechanism may exist. A balance in TNFα signaling has also been suggested as a requirement for successful control of mycobacterial proliferation and granuloma formation both in zebrafish and mouse models (Roach et al., 2002; Tobin et al., 2010; Dorhoi and Kaufmann, 2014). Whether SAL has an impact on granuloma formation also deserves further study.
The most widely used first-line therapy for TB is a combination of isoniazid, rifampin, pyrazinamide, and ethambutol, which mainly focuses on bacterial control. However, this treatment cycle is long and often results in treatment-induced drug resistance and severe side effects. Application of traditional Chinese medicine for TB shifts the focus on the immune regulation of the host (Choi et al., 2015). Thus, the combined application of traditional Chinese medicine and conventional anti-TB drugs may have the advantage of a shorter treatment cycle and avoiding therapy-induced drug resistance. In this study, we have applied fluorescent-labeled M. marinum to carry out serial observations of bacterial burdens both in zebrafish and RAW264.7 macrophage infection models. Compared with conventional plating and counting for the CFU method, fluorescence-based methods can greatly reduce the time and experimental resource costs (Takaki et al., 2012; Cortesia et al., 2017; Hodges et al., 2018). Excitingly, the combined usage of SAL and INH showed the most promising therapeutic results in this study. Interestingly, we did not observe a reduction of bacterial burden in the SAL and INH combined treatment group compared with the INH only group, which may be due to the time point we chose for detecting bacterial burden. A reduced bacterial burden in the combined treatment group might be detected at a later stage of infection. Notably, we have also explored the protective effect of the combined application of SAL and RIF in the revised article and found a similar effect to that produced by the treatment of SAL and INH. In summary, our results provide a proof of concept that the combination of conventional anti-TB and traditional Chinese medicine is a superior treatment strategy.
In summary, SAL was proven to play an anti-infection role in a zebrafish–M. marinum infection model. We have also shown that SAL treatment can regulate innate immune cell recruitment and upregulate the expression of pro-inflammatory genes. We further demonstrated, by using a tnfα-deficient mutant zebrafish model, that the protective role of SAL requires Tnfα signaling. Our data provide proof of concept that immune-regulating traditional Tibetan medicine could be a potent tool in anti-TB drug development.
The original contributions presented in the study are included in the article/Supplementary Material; further inquiries can be directed to the corresponding authors.
Zebrafish were maintained under standard conditions that comply with the guidelines for ethical review of laboratory animal welfare (GB/T35823-2018). The animal study was reviewed and approved (2019-A016-01) by Shanghai Public Health Clinical Center SPHCC of Fudan University. Written informed consent was obtained from the owners for the participation of their animals in this study.
SH, SZ, and BY designed and supervised the whole project. SH, HF, and BY wrote the original draft. HF, BS, MY, HL, JY, JL, SL, ZC, JZ, and LX performed the experiments. SH, HF, BS, MY, HL, JY, JL, ZC, and LX performed the data analysis. All co-authors performed the review and editing of the final version of the manuscript.
This work was supported by the National Natural Science Foundation of China (81801977 to BY; 81871613 to SZ), the Shanghai “Science and Technology Innovation Action Plan” Medical Innovation Research Special Project (22Y11920500 to BY), the Outstanding Youth Training Program of Shanghai Municipal Health Commission (2018YQ54 to BY), the Shanghai Sailing Program (18YF1420400 to BY), and the Natural Science Foundation of Xizang (Tibet) Autonomous Region [No. XZ2019ZR G-37(z)].
The authors thank Dr. C. Niu and Dr. Z. Wen for sharing the tnfα−/−, Tg (mpeg1:loxP-GFP), and Tg (mpeg1:loxP-DsRedx-loxP-GFP) zebrafish strains and Dr. L. Ramakrishnan for sharing M. marinum strains.
The authors declare that the research was conducted in the absence of any commercial or financial relationships that could be construed as a potential conflict of interest.
All claims expressed in this article are solely those of the authors and do not necessarily represent those of their affiliated organizations, or those of the publisher, the editors, and the reviewers. Any product that may be evaluated in this article, or claim that may be made by its manufacturer, is not guaranteed or endorsed by the publisher.
The Supplementary Material for this article can be found online at: https://www.frontiersin.org/articles/10.3389/fphar.2022.936295/full#supplementary-material
Baba, E., Acar, U., Yilmaz, S., Zemheri, F., and Ergun, S. (2018). Dietary olive leaf (Olea europea L.) extract alters some immune gene expression levels and disease resistance to Yersinia ruckeri infection in rainbow trout Oncorhynchus mykiss. Fish. Shellfish Immunol. 79, 28–33. doi:10.1016/j.fsi.2018.04.063
Benard, E. L., Rougeot, J., Racz, P. I., Spaink, H. P., and Meijer, A. H. (2016). Transcriptomic approaches in the zebrafish model for tuberculosis-insights into host- and pathogen-specific determinants of the innate immune response. Adv. Genet. 95, 217–251. doi:10.1016/bs.adgen.2016.04.004
Boisson-Dupuis, S., Bustamante, J., El-Baghdadi, J., Camcioglu, Y., Parvaneh, N., El Azbaoui, S., et al. (2015). Inherited and acquired immunodeficiencies underlying tuberculosis in childhood. Immunol. Rev. 264, 103–120. doi:10.1111/imr.12272
Choi, B. B., Choi, J. H., Park, S. R., Kim, J. Y., Hong, J. W., and Kim, G. C. (2015). Scutellariae radix induces apoptosis in chemoresistant SCC-25 human tongue squamous carcinoma cells. Am. J. Chin. Med. 43, 167–181. doi:10.1142/S0192415X15500111
Clay, H., Volkman, H. E., and Ramakrishnan, L. (2008). Tumor necrosis factor signaling mediates resistance to mycobacteria by inhibiting bacterial growth and macrophage death. Immunity 29, 283–294. doi:10.1016/j.immuni.2008.06.011
Cooper, A. M., Mayer-Barber, K. D., and Sher, A. (2011). Role of innate cytokines in mycobacterial infection. Mucosal Immunol. 4, 252–260. doi:10.1038/mi.2011.13
Cortesia, C., Bello, T., Lopez, G., Franzblau, S., De Waard, J., and Takiff, H. (2017). Use of green fluorescent protein labeled non-tuberculous mycobacteria to evaluate the activity quaternary ammonium compound disinfectants and antibiotics. Braz. J. Microbiol. 48, 151–158. doi:10.1016/j.bjm.2016.09.009
Cronan, M. R., and Tobin, D. M. (2014). Fit for consumption: Zebrafish as a model for tuberculosis. Dis. Model. Mech. 7, 777–784. doi:10.1242/dmm.016089
Dal, N. K., Speth, M., Johann, K., Barz, M., Beauvineau, C., Wohlmann, J., et al. (2021). The zebrafish embryo as an in vivo model for screening nanoparticle-formulated lipophilic anti-tuberculosis compounds. Dis. Model Mech. 15, dmm049147. doi:10.1242/dmm.049147
Dharra, R., Talwar, S., Singh, Y., Gupta, R., Cirillo, J. D., Pandey, A. K., et al. (2017). Rational design of drug-like compounds targeting Mycobacterium marinum MelF protein. PLoS One 12, e0183060. doi:10.1371/journal.pone.0183060
Ding, S. Y., Wang, M. T., Dai, D. F., Peng, J. L., and Wu, W. L. (2020). Salidroside induces apoptosis and triggers endoplasmic reticulum stress in human hepatocellular carcinoma. Biochem. Biophys. Res. Commun. 527, 1057–1063. doi:10.1016/j.bbrc.2020.05.066
Dong, W., Nie, X., Zhu, H., Liu, Q., Shi, K., You, L., et al. (2021). Mycobacterial fatty acid catabolism is repressed by FdmR to sustain lipogenesis and virulence. Proc. Natl. Acad. Sci. U. S. A. 118, e2019305118. doi:10.1073/pnas.2019305118
Dorhoi, A., and Kaufmann, S. H. (2014). Tumor necrosis factor alpha in mycobacterial infection. Semin. Immunol. 26, 203–209. doi:10.1016/j.smim.2014.04.003
Gang, Y. (2009). Recent effect of rhodiola capsule in adjuvant treatment of tuberculosis. Qinghai, China: Qinghai Medical Journal.
Gao, H., Peng, L., Li, C., Ji, Q., and Li, P. (2020). Salidroside alleviates cartilage degeneration through NF-κB pathway in osteoarthritis rats. Drug Des. devel. Ther. 14, 1445–1454. doi:10.2147/DDDT.S242862
Gomes, M. C., and Mostowy, S. (2020). The case for modeling human infection in zebrafish. Trends Microbiol. 28, 10–18. doi:10.1016/j.tim.2019.08.005
Guan, S., Feng, H., Song, B., Guo, W., Xiong, Y., Huang, G., et al. (2011). Salidroside attenuates LPS-induced pro-inflammatory cytokine responses and improves survival in murine endotoxemia. Int. Immunopharmacol. 11, 2194–2199. doi:10.1016/j.intimp.2011.09.018
Hao, X., Yuan, J., and Dong, H. (2019). Salidroside prevents diabetesinduced cognitive impairment via regulating the Rho pathway. Mol. Med. Rep. 19, 678–684. doi:10.3892/mmr.2018.9621
Hodges, H. L., Brown, R. A., Crooks, J. A., Weibel, D. B., and Kiessling, L. L. (2018). Imaging mycobacterial growth and division with a fluorogenic probe. Proc. Natl. Acad. Sci. U. S. A. 115, 5271–5276. doi:10.1073/pnas.1720996115
Lee, S. Y., Li, M. H., Shi, L. S., Chu, H., Ho, C. W., and Chang, T. C. (2013a). Rhodiola crenulata extract alleviates hypoxic pulmonary edema in rats. Evid. Based. Complement. Altern. Med. 2013, 718739. doi:10.1155/2013/718739
Lee, S. Y., Shi, L. S., Chu, H., Li, M. H., Ho, C. W., Lai, F. Y., et al. (2013b). Rhodiola crenulata and its bioactive components, salidroside and tyrosol, reverse the hypoxia-induced reduction of plasma-membrane-associated Na, K-ATPase expression via inhibition of ROS-AMPK-PKC xi pathway. Evid. Based. Complement. Altern. Med. 2013, 284150. doi:10.1155/2013/284150
Lu, L., Yuan, J., and Zhang, S. (2013). Rejuvenating activity of salidroside (SDS): Dietary intake of SDS enhances the immune response of aged rats. Age (Dordr) 35, 637–646. doi:10.1007/s11357-012-9394-x
Ma, C., Hu, L., Tao, G., Lv, W., and Wang, H. (2015). An UPLC-MS-based metabolomics investigation on the anti-fatigue effect of salidroside in mice. J. Pharm. Biomed. Anal. 105, 84–90. doi:10.1016/j.jpba.2014.11.036
Mayer-Barber, K. D., Andrade, B. B., Oland, S. D., Amaral, E. P., Barber, D. L., Gonzales, J., et al. (2014). Host-directed therapy of tuberculosis based on interleukin-1 and type I interferon crosstalk. Nature 511, 99–103. doi:10.1038/nature13489
Myllymaki, H., Bauerlein, C. A., and Ramet, M. (2016). The zebrafish breathes new life into the study of tuberculosis. Front. Immunol. 7, 196. doi:10.3389/fimmu.2016.00196
Niu, L., Luo, G., Liang, R., Qiu, C., Yang, J., Xie, L., et al. (2022). Negative regulator nlrc3-like maintain the balanced innate immune response during mycobacterial infection in zebrafish. Front. Immunol. 13, 893611. doi:10.3389/fimmu.2022.893611
Niu, L., Wang, C., Zhang, K., Kang, M., Liang, R., Zhang, X., et al. (2019). Visualization of macrophage lytic cell death during mycobacterial infection in zebrafish embryos via intravital microscopy. J. Vis. Exp. 1, 1. doi:10.3791/60698
Nunn, A. J., Phillips, P. P. J., Meredith, S. K., Chiang, C. Y., Conradie, F., Dalai, D., et al. (2019). A trial of a shorter regimen for rifampin-resistant tuberculosis. N. Engl. J. Med. 380, 1201–1213. doi:10.1056/NEJMoa1811867
Qiu, X., and Jia, J. (2014). Research advances on TCM anti-tumor effects and the molecular mechanisms. J. Cancer Res. Ther. 10 (1), 8–13. doi:10.4103/0973-1482.139744
Ramakrishnan, L. (2020). Mycobacterium tuberculosis pathogenicity viewed through the lens of molecular Koch's postulates. Curr. Opin. Microbiol. 54, 103–110. doi:10.1016/j.mib.2020.01.011
Reingewertz, T. H., Meyer, T., Mcintosh, F., Sullivan, J., Meir, M., Chang, Y. F., et al. (2020). Differential sensitivity of mycobacteria to isoniazid is related to differences in KatG-mediated enzymatic activation of the drug. Antimicrob. Agents Chemother. 64, e01899–19. doi:10.1128/AAC.01899-19
Roach, D. R., Bean, A. G., Demangel, C., France, M. P., Briscoe, H., and Britton, W. J. (2002). TNF regulates chemokine induction essential for cell recruitment, granuloma formation, and clearance of mycobacterial infection. J. Immunol. 168, 4620–4627. doi:10.4049/jimmunol.168.9.4620
Roca, F. J., and Ramakrishnan, L. (2013). TNF dually mediates resistance and susceptibility to mycobacteria via mitochondrial reactive oxygen species. Cell 153, 521–534. doi:10.1016/j.cell.2013.03.022
Sharma, N., Mishra, K. P., and Ganju, L. (2016). Salidroside exhibits anti-dengue virus activity by upregulating host innate immune factors. Arch. Virol. 161, 3331–3344. doi:10.1007/s00705-016-3034-1
Sommer, F., Ortiz Zacari As, N. V., Heitman, L. H., and Meijer, A. H. (2021). Inhibition of macrophage migration in zebrafish larvae demonstrates in vivo efficacy of human CCR2 inhibitors. Dev. Comp. Immunol. 116, 103932. doi:10.1016/j.dci.2020.103932
Stinear, T. P., Seemann, T., Harrison, P. F., Jenkin, G. A., Davies, J. K., Johnson, P. D., et al. (2008). Insights from the complete genome sequence of Mycobacterium marinum on the evolution of Mycobacterium tuberculosis. Genome Res. 18, 729–741. doi:10.1101/gr.075069.107
Takaki, K., Cosma, C. L., Troll, M. A., and Ramakrishnan, L. (2012). An in vivo platform for rapid high-throughput antitubercular drug discovery. Cell Rep. 2, 175–184. doi:10.1016/j.celrep.2012.06.008
Tobin, D. M., Roca, F. J., Oh, S. F., Mcfarland, R., Vickery, T. W., Ray, J. P., et al. (2012). Host genotype-specific therapies can optimize the inflammatory response to mycobacterial infections. Cell 148, 434–446. doi:10.1016/j.cell.2011.12.023
Tobin, D. M., Vary, J. C., Ray, J. P., Walsh, G. S., Dunstan, S. J., Bang, N. D., et al. (2010). The lta4h locus modulates susceptibility to mycobacterial infection in zebrafish and humans. Cell 140, 717–730. doi:10.1016/j.cell.2010.02.013
Trede, N. S., Langenau, D. M., Traver, D., Look, A. T., and Zon, L. I. (2004). The use of zebrafish to understand immunity. Immunity 20, 367–379. doi:10.1016/s1074-7613(04)00084-6
Wang, T., Yan, B., Lou, L., Lin, X., Yu, T., Wu, S., et al. (2019). Nlrc3-like is required for microglia maintenance in zebrafish. J. Genet. Genomics 46, 291–299. doi:10.1016/j.jgg.2019.06.002
Wiegand, I., Hilpert, K., and Hancock, R. E. (2008). Agar and broth dilution methods to determine the minimal inhibitory concentration (MIC) of antimicrobial substances. Nat. Protoc. 3, 163–175. doi:10.1038/nprot.2007.521
Xie Lingling, W. F., Wang, D., Zhao, C., Peng, G., and Chen, N. (2017). Application of tumor necrosis factor α mutant zebrafish in Mycobacterium marinum infection. J. Microbes Infect. 12, 156–163.
Xu, J., Wang, T., Wu, Y., Jin, W., and Wen, Z. (2016). Microglia colonization of developing zebrafish midbrain is promoted by apoptotic neuron and lysophosphatidylcholine. Dev. Cell 38, 214–222. doi:10.1016/j.devcel.2016.06.018
Yan, B., Han, P., Pan, L., Lu, W., Xiong, J., Zhang, M., et al. (2014). IL-1β and reactive oxygen species differentially regulate neutrophil directional migration and Basal random motility in a zebrafish injury-induced inflammation model. J. Immunol. 192, 5998–6008. doi:10.4049/jimmunol.1301645
Yang, D. W., Kang, O. H., Lee, Y. S., Han, S. H., Lee, S. W., Cha, S. W., et al. (2016). Anti-inflammatory effect of salidroside on phorbol-12-myristate-13-acetate plus A23187-mediated inflammation in HMC-1 cells. Int. J. Mol. Med. 38, 1864–1870. doi:10.3892/ijmm.2016.2781
Yang, K., Qi, X., He, M., Song, K., Luo, F., Qu, X., et al. (2020). Dietary supplementation of salidroside increases immune response and disease resistance of crucian carp (Carassius auratus) against Aeromonas hydrophila. Fish. Shellfish Immunol. 106, 1–7. doi:10.1016/j.fsi.2020.07.054
Yu, T., Guo, W., Tian, Y., Xu, J., Chen, J., Li, L., et al. (2017). Distinct regulatory networks control the development of macrophages of different origins in zebrafish. Blood 129, 509–519. doi:10.1182/blood-2016-07-727651
Zemheri-Navruz, F., Acar, U., and Yilmaz, S. (2019). Dietary supplementation of olive leaf extract increases haematological, serum biochemical parameters and immune related genes expression level in common carp (Cyprinus carpio) juveniles. Fish. Shellfish Immunol. 89, 672–676. doi:10.1016/j.fsi.2019.04.037
Zhao, X., Lu, Y., Tao, Y., Huang, Y., Wang, D., Hu, Y., et al. (2013). Salidroside liposome formulation enhances the activity of dendritic cells and immune responses. Int. Immunopharmacol. 17, 1134–1140. doi:10.1016/j.intimp.2013.10.016
Keywords: mycobacterium, salidroside, zebrafish, innate immunity, neutrophil, macrophage, Tnfα
Citation: He S, Fan H, Sun B, Yang M, Liu H, Yang J, Liu J, Luo S, Chen Z, Zhou J, Xia L, Zhang S and Yan B (2022) Tibetan medicine salidroside improves host anti-mycobacterial response by boosting inflammatory cytokine production in zebrafish. Front. Pharmacol. 13:936295. doi: 10.3389/fphar.2022.936295
Received: 05 May 2022; Accepted: 29 July 2022;
Published: 31 August 2022.
Edited by:
Stefan Oehlers, Technology and Research (A∗STAR), SingaporeReviewed by:
Elinor Hortle, Royal Prince Alfred Hospital, AustraliaCopyright © 2022 He, Fan, Sun, Yang, Liu, Yang, Liu, Luo, Chen, Zhou, Xia, Zhang and Yan. This is an open-access article distributed under the terms of the Creative Commons Attribution License (CC BY). The use, distribution or reproduction in other forums is permitted, provided the original author(s) and the copyright owner(s) are credited and that the original publication in this journal is cited, in accordance with accepted academic practice. No use, distribution or reproduction is permitted which does not comply with these terms.
*Correspondence: Shumei He, shumeihe2021@sina.com; Shulin Zhang, shulinzhang@sjtu.edu.cn; Bo Yan, bo.yan@shphc.org.cn
†These authors have contributed equally to this work
Disclaimer: All claims expressed in this article are solely those of the authors and do not necessarily represent those of their affiliated organizations, or those of the publisher, the editors and the reviewers. Any product that may be evaluated in this article or claim that may be made by its manufacturer is not guaranteed or endorsed by the publisher.
Research integrity at Frontiers
Learn more about the work of our research integrity team to safeguard the quality of each article we publish.