- 1Innovation Center of Nursing Research, West China School of Nursing, Department of Gastrointestinal Surgery, National Clinical Research Center for Geriatrics, Nursing Key Laboratory of Sichuan Province, West China Hospital, Sichuan University, Chengdu, China
- 2Emergency Department, Institute of Medical Big Data, Zigong Academy of Big Data for Science and Artificial Intelligence, Zigong Fourth People’s Hospital, Zigong, China
Nicotinamide phosphoribosyltransferase (NAMPT) is the rate-limiting enzyme in the nicotinamide adenine dinucleotide (NAD) salvage pathway in mammals. It is of great significance in the metabolic homeostasis and cell survival via synthesizing nicotinamide mononucleotide (NMN) through enzymatic activities, serving as a key protein involved in the host’s defense mechanism. The NAMPT metabolic pathway connects NAD-dependent sirtuin (SIRT) signaling, constituting the NAMPT–NAD–SIRT cascade, which is validated as a strong intrinsic defense system. Neurodegenerative diseases belong to the central nervous system (CNS) disease that seriously endangers human health. The World Health Organization (WHO) proposed that neurodegenerative diseases will become the second leading cause of human death in the next two decades. However, effective drugs for neurodegenerative diseases are scant. NAMPT is specifically highly expressed in the hippocampus, which mediates cell self-renewal and proliferation and oligodendrocyte synthesis by inducing the biosynthesis of NAD in neural stem cells/progenitor cells. Owing to the active biological function of NAMPT in neurogenesis, targeting NAMPT may be a powerful therapeutic strategy for neurodegenerative diseases. This study aims to review the structure and biological functions, the correlation with neurodegenerative diseases, and treatment advance of NAMPT, aiming to provide a novel idea for targeted therapy of neurodegenerative diseases.
Introduction
Nicotinamide Phosphoribosyltransferase
The activity of NAMPT as an intracellular NAD regulator was initially reported in 1957 (Garten et al., 2015; Wang and Miao, 2015). In 1994, NAMPT was confirmed as a cytokine secreted by human peripheral blood lymphocytes (Samal et al., 1994), and it was named as the pre-B cell colony enhancing factor (PBEF) (Sun et al., 2013). Later, in 2005, NAMPT was identified to be highly expressed in visceral adipose tissues, which is closely linked with the prognosis of obese patients. Therefore, NAMPT is renamed as visfatin (Yang et al., 2006). Although NAMPT, PBEF, and visfatin have been cited in literature studies, the HUGO Gene Nomenclature Committee and the International Committee on Standardized Genetic Nomenclature for Mice both named the protein and gene of NAMPT (Fukuhara et al., 2005).
NAMPT is universally expressed in almost all organs, tissues, and cells (Yang et al., 2006), indicating the pleiotropic function in humans (Friebe et al., 2011). It is able to mediate the pathogenesis of relevant diseases by regulating inflammatory response, cell apoptosis, glucose metabolism, and oxidative stress. Therefore, NAMPT is of significance in cell metabolism, senescence, cell cycle progression, etc. Abundant homologous sequences of NAMPT exist in prokaryotes and primitive metazoans like sponges and humans (Martin et al., 2001). Both in vitro and in vivo biological activities of NAMPT have been detected (Audrito et al., 2020). In vitro biological functions of NAMPT as the regulator of NAD have been widely explored (Audrito et al., 2020). Through regulating the biosynthesis activity of NAD, NAMPT mediates the activities of NAD-dependent enzymes like acetylase (Garten et al., 2009; Koltai et al., 2010; Pavlova et al., 2015; Chen et al., 2016; Wang and Miao, 2019), poly(ADP-ribose) polymerase (Henning et al., 2018), and CD38 (Lee and Aarhus, 1991), thus influencing cell metabolism, mitochondrial biogenesis, and adaptive responses to inflammation and oxidation (Galli et al., 2013; Garten et al., 2015; Chen et al., 2016). Both NAMPT and its connection to the SIRT signaling constitute the powerful defense system against various stresses (Wang and Miao, 2015). SIRTs are a group of NAD-dependent histone deacetylases, the activation of which delays the occurrence of neurodegenerative diseases. Therefore, the role of SIRTs in neurological diseases has been well concerned. A previous study has proven that NAMPT delays senescence by improving cell resistance to oxidative stress (Wang et al., 2016).
NAMPT consists of extracellular and intracellular forms, called extracellular NAMPT (eNAMPT) and intracellular NAMPT (iNAMPT). eNAMPT serves as a growth factor, enzyme, and cytokine to exert its biological functions. It is an active protein in the extracellular space, first identified as PBEF and then thought to be preferentially secreted by visceral adipose tissue, called visfatin, which is produced by post-translational modifications of iNAMPT (Lundt and Ding, 2021). eNAMPT has been found in many tissues throughout the body, including the brain and blood. It is not only secreted by pre-B cells but also produced by many other cell types, such as fat cells, immune cells, brain cells, and cancer cells. Current studies suggest that eNAMPT maintains functional tissue homeostasis and enhances NAD, SIRT1 activity, and neural activation in the hypothalamus, and eNAMPT is also a major regulator of inflammatory networks, promoting the release of inflammatory cytokines (Yoshida et al., 2019; Quijada et al., 2021). Revollo’s studies found that eNAMPT and iNAMPT have similar enzymatic activities, and eNAMPT produced by differentiated adipocytes exhibits robust NAD biosynthetic activity that is even higher than iNAMPT (Revollo et al., 2007). However, based on the kinetic analyses of the enzyme, the research of Hara et al. revealed that eNAMPT does not participate in NMN formation under the extracellular milieus (Hara et al., 2011). Therefore, the mechanism and physiological function of the extracellular secretion of eNAMPT are still not particularly clear, and further research is needed in the later period (Garten et al., 2009; Travelli et al., 2018). iNAMPT is a pleiotropic protein that exists in the cytoplasm, nucleus, and mitochondria, especially in neurons of the hippocampus and cortex of the brain, and is also highly expressed in the brown adipose tissue, liver, and kidney (Garten et al., 2015). The cerebellum also expresses iNAMPT, especially in Purkinje cells, granule cells, and molecular layer cells (Liu et al., 2012). iNAMPT can be used as a key enzyme in catalyzing the biosynthetic pathway in the rate-limiting process of NAD, and it plays a variety of roles in energy metabolism, promoting endothelial cell proliferation, inhibiting apoptosis, and regulating vascular tone (Chen et al., 2015; Liu et al., 2021).
Neurodegenerative Diseases
Neurodegenerative diseases are a type of heterogeneous disorders (Lin and Beal, 2006a), which are the biggest health issues in the global aging (Vaquer-Alicea and Diamond, 2019). They are characterized by the gradual loss of neurons that cause severe damages (Moutinho et al., 2019). Typical neurodegenerative diseases include Alzheimer’s disease (AD), Parkinson’s disease (PD), Huntington’s disease (HD), and amyotrophic lateral sclerosis (ALS) (Dugger and Dickson, 2017). At present, significant changes like inflammatory response, oxidative stress, and protein aggregation have been detected in the nerves of patients with neurodegenerative diseases (Wang et al., 2016), in which neuroinflammation and oxidative damage are prominent features of neurodegenerative diseases (Gao and Hong, 2008). So far, great efforts have been made on controlling clinical symptoms and signs of neurodegenerative diseases, but effective therapeutic strategies are lacked, which should be urgently developed and applied to clinical practice (Silvestro et al., 2021).
Normal physiological functions of neurons are highly dependent on the mitochondrial energy metabolism. Therefore, mitochondrial dysfunction is the key event of many neurodegenerative diseases (Keating, 2008). NAD is an essential coenzyme involved in energy production and redox metabolism (Lautrup et al., 2019a), which are closely linked with the mitochondrial energy metabolism. Most NADs are not synthesized de novo. NAMPT-mediated NAD salvage pathway is the main synthesis route of NAD, while NAD biosynthesis defects result in the decline of NAD (Imai and Guarente, 2014a). Therefore, NAMPT is vital in maintaining the homeostasis of NAD. The depletion of NAD in the brain destroys the energy homeostasis in cells and causes neuron death (Wang and Miao, 2019). Neural stem cells (NSCs) are capable of producing neurons, oligodendrocytes, or astrocytes through lineage-restricted cell division. NAMPT mediates the proliferation and aggregation of NSCs (Stein and Imai, 2014). Therefore, NAMPT is particularly important for the proliferation, self-renewal, and differentiation of neural stem/progenitor cells (NSPCs) (Wang and Miao, 2019).
In the present review, we first introduced the structure and biological functions of NAMPT, as well as the close correlation between NAMPT and neurodegenerative diseases (e.g., AD and PD). Furthermore, current agonists and inhibitors targeting NAMPT that are used for the treatment of neurodegenerative diseases were summarized for their molecule sources, characteristics, and therapeutic effects; thus, providing novel ideas for the targeted therapy of neurodegenerative diseases.
Crystal Structure of Nicotinamide Phosphoribosyltransferase
The NAMPT gene locates on human chromosome 7q22 and spans 34.7 kb, which contains 11 exons and 10 introns, and produces a 2357-bp cDNA (Sommer et al., 2008; Sun et al., 2013). The NAMPT protein contains 491 amino acids with a molecular weight of 52 kDa (Sommer et al., 2008). Its structure is similar to that of the nicotinate phosphoribosyltransferase (NAPRTase) and quinolinate phosphoribosyl transferase (QAPRTase) of the hyperthermophilic archaea (Wang et al., 2006). NAMPT belongs to a dimeric class of type II phosphoribosyltransferases (Figure 1), the X-ray crystal structure of which confirms the low sequence identity (Wang et al., 2006). There are two active sites at the interface of the dimeric protein (Wang et al., 2006), where two NMN molecules bind. Through comparing active site sequences of 14 dimers in different organisms, including rats, the sequence of amino acid residues bound to the nicotinamide ring (substrate) and NMN (product) is identified as highly conserved (Luk et al., 2008).
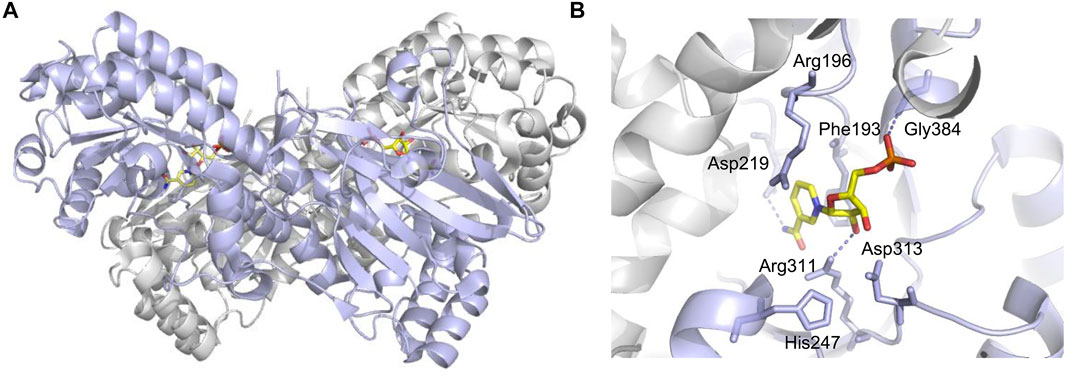
FIGURE 1. Crucial sites for NAMPT enzymatic activity (PDB code: 2H3D). (A) One monomer is purple, the other is gray, and NMN is shown in yellow stick shape. (B) Asp219 hydrogen bonded with NAM for substrate specificity. His247 is used for autophosphorylation, and dotted lines indicate hydrogen bonds.
Biological Functions of Nicotinamide Phosphoribosyltransferase
In 1957, Preiss and Handler reported that NAMPT can catalyze the synthesis of nicotinamide mononucleotide (NMN) (Preiss and Handler, 1957). It is well known that NAMPT participates in the metabolism of NAD and maintains NAD levels in cells. During the past decade, the pleiotropic effect of NAMPT has been revealed. Through regulating the biosynthesis activity of NAD, NAMPT mediates the activities of NAD-dependent enzymes like poly (ADP-ribose) polymerase (PARP), ADP-ribosyltransferase (ART), and SIRT. The connection between NAMPT–NAD and SIRT constitutes a powerful defense system against various stresses (Garten et al., 2015). In addition, the production of NMN by catalyzing nicotinamide (NAM) and 5-phosphoribosyl-1-pyrophosphate (PRPP) eventually influences the activities of NAD-dependent enzymes, and as a result, NAMPT is able to mediate cell metabolism, mitogenesis, inflammation, and oxidation (Garten et al., 2015). The regulatory effect of NAMPT on SIRT has been widely concerned (Ramsey et al., 2009). Mediated by the circadian rhythm regulators CLOCK and BMAL1 of SIRT1, NAMPT can regulate the circadian rhythm of metabolism (Galli et al., 2013).
In addition to the intracellular functions, extracellular functions of NAMPT have been highlighted in recent years. Induced by pathogen-derived lipopolysaccharide (LPS) and host-derived inflammatory stimuli (e.g., leptin, TNF-α, IL-1β, and IL-6), NAMPT is able to mediate inflammatory response (Busso et al., 2008; Audrito et al., 2020; Wang et al., 2021). It is reported that NAMPT serves as an adipokine to regulate insulin secretion in pancreatic β-cells (Imai and Yoshino, 2013). The immune response can be influenced by NAMPT, which inhibits apoptosis of immune cells like neutrophils and macrophages (Travelli et al., 2018). Although the mechanism underlying extracellular functions of NAMPT has not been clearly demonstrated (Carbone et al., 2017), its potential as a therapeutic target has been highlighted due to vital physiological functions (Figure 2).
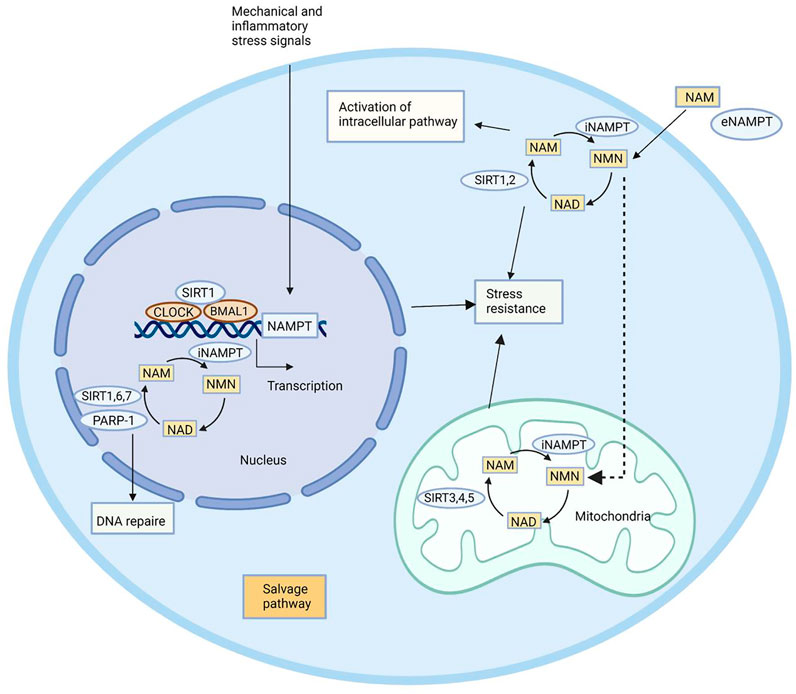
FIGURE 2. Biological functions of NAMPT. NAMPT plays its role by maintaining intracellular NAD levels and recovering NAM, which is produced by NAD-dependent enzymes PARP-1, SIRT1, SIRT6, and SIRT7 in the nucleus, SIRT1 and SIRT2 in the cytoplasm, and SIRT3, SIRT4, and SIRT5 in the mitochondria. NAMPT expression is induced by circadian regulators CLOCK and BMAL1, which are complex with SIRT1. NAMPT affects a remedial pathway that can resist stress and repair DNA. In addition to enzyme activity, NAMPT can also play an extracellular role in helping NAM produce NMN.
The Close Correlation Between Nicotinamide Phosphoribosyltransferase and Neurodegenerative Diseases
Neurodegenerative diseases are caused by the apoptosis of neurons in the brain and spinal cord, resulting in dysfunctions with the disease aggravation (Dugger and Dickson, 2017). Damaged nerves caused by neurodegenerative diseases not only affect existing neurons but also impair the endogenous repair mechanism for cell renewal and differentiation (Wang et al., 2016). Neurodegenerative diseases are mainly associated with oxidative stress, mitochondrial dysfunction, excitotoxicity, and inflammation (Richards et al., 2018; Tofaris and Buckley, 2018). Among them, oxidative stress is considered as a vital pathogenic factor that induces the proliferation, reduction of self-renewal and differentiation, mitochondrial dysfunction, and downregulation of NAD and NAMPT levels in neurodegenerative disease cases (Lugert et al., 2010). NAD is a classic coenzyme involved in energy production (ATP) and redox metabolism, which can be generated de novo from tryptophan or recovered from NAM through the NAMPT-dependent salvage pathway (Rajman et al., 2018). Due to the high energy consumption and ATP synthesis rate, rapid consumption of NAD in the brain inevitably inhibits the production of ATP, destroys the energy homeostasis, and ultimately leads to neuron death (Lautrup et al., 2019a). PARPs (PAPR1 as the most analyzed), CD38 (a transmembrane enzyme), and SIRT are examined as NAD-dependent enzymes (Imai and Guarente, 2014a). The protective effect of NAD relies on SIRTs (Imai and Guarente, 2014a; Verdin, 2015). Among them, SIRT1 is the most analyzed member of SIRTs, which is responsive to the change of intracellular NAD level under various stresses owing to the deacylase activity (Wang et al., 2011). Since NAMPT is the rate-limiting enzyme of the NAD salvage pathway in mammals, it can regulate neurodegenerative diseases by maintaining NAD homeostasis, cell metabolism, and mitochondrial function.
Nicotinamide Phosphoribosyltransferase and Alzheimer’s Disease
AD is the most common neurodegenerative disease. The prevalence of AD significantly increases with age, which mainly affects the elderly. Typical manifestations of AD include memory loss that is linked with the loss of neurons in the hippocampus and others linked with frontal lobe functions. It is generally considered that the extracellular amyloid plaques like amyloid β (Aβ) (Tagliavini et al., 1988), neurofibrillary tangles (NFT) formed by hyperphosphorylated tau protein, and loss of neurons and synapses are the main pathogenic mechanisms of AD (Lin and Beal, 2006a).
So far, only five therapeutic strategies for cognitive impairment in AD cases have been approved in the United States, including the cholinesterase inhibitors (donepezil, galantamine, and rivastigmine), NMDA receptor antagonist (memantine) (Cummings et al., 2014), and the fixed-dose combination of donepezil and memantine for moderate and severe AD (Cummings et al., 2019). However, they are only effective for alleviating clinical symptoms of AD, rather than cure or prevention. At present, multiple new drugs for AD have been analyzed, including flavonoids and rapamycin (Aso and Ferrer, 2013; Maher, 2019).
It is reported that cytokines involved in mitogenesis (e.g., NRF1, NRF2, and TFAM) are associated with neurodegenerative diseases like AD. Therefore, mitochondrial dysfunction can affect the disease condition of AD. Intervention in mitochondrial levels can improve Aβ-induced degeneration and dysfunction (Golpich et al., 2017b). Impaired mitogenesis is considered as a potential factor for AD-induced mitochondrial dysfunction (Sheng et al., 2012). Therefore, to enhance mitogenesis may be a potential pharmacological strategy for the treatment of AD. iNAMPT is important in neurodegenerative diseases through mediating mitochondria to produce energy. In addition, neuroinflammation, oxidative stress, and cholinergic neuron damage also induce neurodegeneration (Kwon and Koh, 2020a). A recent study has shown that overactivation of the immune proteasome (IP) triggers neuroinflammation and the death of neurons (Sonninen et al., 2020). Through suppressing inflammatory response and oxidative stress, iNAMPT is functionally involved in neurodegenerative diseases.
Nicotinamide Phosphoribosyltransferase and Parkinson’s Disease
PD is the second most common neurodegenerative disease in the world, which seriously affects the normal life of middle-aged and elderly patients (Jankovic and Tan, 2020a). The necrosis of dopaminergic neurons in the substantia nigra of the midbrain is the typical pathological feature of PD. The exact pathogenic mechanism of PD, however, has not been fully elucidated (Tomas-Camardiel et al., 2004; Kalia and Lang, 2015). It is generally believed that mitochondrial dysfunction, oxidative stress, chronic inflammation, and abnormal protein folding are main causes leading to PD (Pajares et al., 2020). Latest studies have shown that increased activation of microglia in the substantia nigra of PD patients is responsible for inflammatory response (Kwon and Koh, 2020a).
Currently approved PD drugs by the U.S. Food and Drug Administration (FDA) mainly include dopaminergic antiparkinsonism agents [e.g., aromatic l-amino acid decarboxylase (AADC) inhibitors, catechol-O-methyltransferase (COMT) inhibitors, monoamine oxidase B (MAO-B) inhibitors, dopamine transporter (DAT) inhibitors, and dopamine receptor (DR) agonists] (Elsworth, 2020), 5-hydroxytryptamine (5-HT) antiparkinsonism agents [e.g., serotonin 2A (5-HT2A) receptors and serotonin 2C (5-HT2C) receptor antagonists], and cholinergic drugs [e.g., muscarinic acetylcholine receptor (mAChR) antagonists and N-methyl-D-aspartic acid (NMDA) antagonists] (Hayes, 2019; Jankovic and Tan, 2020a). However, they only significantly alleviate symptoms of PD patients in a short period, while the long-term use may result in gradually aggravated autonomic dysfunction and some ineffective or insensitive symptoms (De Virgilio et al., 2016). Therefore, it is necessary to develop new drugs with less adverse events and better therapeutic efficacy.
The significant reduction of ATP and creatine phosphate (PCr) in the core–shell polymers indicates mitochondrial dysfunction of striatal dopaminergic neurons in the early and late stages of PD (Hattingen et al., 2009). Mitochondrial dysfunction affects the respiratory chain, leading to the decreased production of ATP and increased production of free radicals (Golpich et al., 2017b). Free radicals directly inhibit the mitochondrial respiratory chain, which further leads to oxidative damage (Weise et al., 2005). iNAMPT can maintain cell metabolism, and in turn affects mitochondrial function. Studies found that mitochondrial dysfunction is a key driver of PD. Increasing NAD by nicotinamide nucleoside (NR), the precursor of NAD, significantly improved the mitochondrial function of neurons in patients. iNAMPT can utilize NAD biosynthesis enzymes NR kinase 1 (NRK1) to synthesize NAD from NAD precursors (Schondorf et al., 2018). Great efforts have been made on exploring the therapeutic potential of NSCs in PD (Zhang et al., 2017). A growing number of evidence has shown that NSCs suffer cell senescence under various stresses (Zeng et al., 2021). iNAMPT is particularly important for the proliferation, self-renewal, and differentiation of NSPCs. The NAMPT agonist P7C3-A20 is able to protect mature hippocampal neurons and substantia nigra dopaminergic neurons in the PD model (Vazquez-Rosa et al., 2020). Therefore, targeting iNAMPT would become a novel research direction for the treatment of PD.
Nicotinamide Phosphoribosyltransferase and Ischemic Stroke
Ischemic stroke is the second leading cause of death in the world, which is the most common cause of permanent disability in adults (O'Donnell et al., 2010; Orellana-Urzua et al., 2020). Previous studies mainly analyzed the oxidative stress and inflammation in ischemic stroke cases, and relevant neuroprotective drugs based on these mechanisms have been developed (Maida et al., 2020). However, they have not been applied to clinical practice due to inappropriate selection of animal models, severe adverse events, and individualized differences (Wu et al., 2020).
Currently, ischemic stroke can only be treated with intravenous tissue plasminogen activator (t-PA) and endovascular treatment (Adeoye et al., 2011; Dirnagl and Endres, 2014). However, they are limited by the narrow range of indications, production of highly harmful reactive oxygen species (ROS), and oxidative stress, leading to brain damages (Orellana-Urzua et al., 2020). In addition, oxidative stress also causes cell apoptosis, autophagy, and necrosis in the brain (Orellana-Urzua et al., 2020). Although ischemic stroke has been extensively analyzed, the effective treatment is lacked and requires further explorations.
The interruption of cerebral arteries causes oxygen and glucose deprivation (OGD), which not only induces changes in blood vessels but also affects neuronal and glial function (Khoshnam et al., 2017). iNAMPT in the brain is mainly expressed in neurons and NSCs, which is responsible for regulating the nerve function (Wang et al., 2011; Stein and Imai, 2014). It is reported that the mean plasma eNAMPT level in ischemic stroke patients is two to eight times higher than that of non-stroke subjects (Kadoglou et al., 2014). Mitochondrial dysfunction is considered as one of the clinical signs of ischemic stroke, and therefore, targeting mitochondria may be a promising therapeutic strategy for ischemic stroke (He et al., 2020a). NAD is important in maintaining cell energy and mitogenesis. Its level decreases in the hypoxic environment after ischemic stroke due to insufficient blood supply. It is confirmed that upregulation of iNAMPT is favorable to protect from stroke (Jing et al., 2014; Wang and Miao, 2019). The connection of the NAMPT–NAD axis and SIRT forms a powerful defense system against various stresses (Wang and Miao, 2015). A growing number of in vitro and in vivo studies have demonstrated the neuroprotective effect of iNAMPT on stroke (Wang and Miao, 2015). Therefore, the role of NAMPT in cerebral ischemic injury has been well concerned, and it is expected to be applied to the treatment of ischemic stroke.
Nicotinamide Phosphoribosyltransferase and Amyotrophic Lateral Sclerosis
ALS is a genetic neurodegenerative disease involving the upper motor neurons (brain, brain stem, and spinal cord), lower motor neurons (cranial nucleus and anterior horn cells), and the trunk, limbs, and head and face muscles they control (van Es et al., 2017). The main symptoms of ALS include muscle weakness and spasm, fasciculation and atrophy, and others include cognitive impairment, dysphonia, and salivation (Hardiman et al., 2017a). ALS affects most of the muscles including the respiratory muscles, and patients die within 3–5 years of onset due to respiratory failure (Niedermeyer et al., 2019). At present, the complicated mechanism of ALS has not been fully elucidated. Mitochondrial dysfunction, excitotoxicity, oxidative stress, metabolic disorders, and neuroinflammation are considered as potential pathological factors (Hardiman et al., 2017a; Chia et al., 2018).
ALS can be intervened by pharmacological and non-pharmacological methods. So far, more than 50 drugs with different mechanisms have been analyzed for the treatment of ALS, but only riluzole and edaravone are available in the market. Riluzole is the first drug for ALS approved by the FDA (Kiernan et al., 2011; Hardiman et al., 2017a). After 18-months treatment with riluzole, the survival of ALS patients is prolonged for 3 months, which is the only drug providing the survival benefit of about 3 months (Hobson and Mcdermott, 2016). Edaravone has been approved by the FDA as an antioxidant that alleviates the early-stage onset and disease progression of ALS. Symptomatic treatment drugs for muscle spasms like baclofen and tizanidine, both of which are muscle relaxants, are available (van Es et al., 2017). Nevertheless, most symptomatic treatments for ALS have not been tested in randomized controlled trials, and they are mainly assessed based on the management of other diseases (van Es et al., 2017). It is necessary to develop novel drugs with the high efficacy and safety for ALS.
Primary astrocytes isolated from mice overexpressing mutant human superoxide dismutase 1 (hSOD1) can induce the death of motor neurons (Harlan et al., 2016; Obrador et al., 2021). The increased mitochondrial level of NAD in astrocytes of ALS cases enhances the resistance to oxidative stress and reverses the toxicity of co-cultured motor neurons (Obrador et al., 2021). iNAMPT is the rate-limiting enzyme of the NAD salvage pathway, and its overexpression upregulates mitochondrial level of NAD in astrocytes. Therefore, iNAMPT may be a potential therapeutic target to prevent astrocyte-mediated motor neuron death in ALS cases.
Nicotinamide Phosphoribosyltransferase and Huntington’s Disease
HD is a progressive autosomal dominant inherited neurodegenerative disease that seriously affects the quality of life (Walker, 2007). The main symptoms of HD include mental disorders, chorea, dystonia, and cognitive impairment (Walker, 2007). It is caused by an increase in trinucleotide (CAG) in the HTT gene that causes repeated polyglutamine sequence in the Huntingtin protein. Mutant Huntingtin further results in neuronal dysfunction and death via regulating cellular protein, transcription, mitochondria, and synaptic function (Harlan et al., 2019). At present, supportive and symptomatic treatment is preferred to HD patients and effective therapeutic strategies to improve the disease are lacked (Jimenez-Sanchez et al., 2017).
Mitochondrial disorders and oxidative stress are the main cellular characteristics of HD (Mccolgan and Tabrizi, 2018). Increased proliferation of cells expressing PCNA in the subventricular zone (SVZ) of HD patients after death indicates the accelerated neurogenesis (Liu and Song, 2016). SIRT1 is a NAD-dependent lysine deacetylase, and its overexpression is able to alleviate mutant Huntingtin (mHTT) toxicity and peripheral defects in the transgenic N171-82Q mice, showing a neuroprotective effect (Naia et al., 2017). The activity of SIRT1 can be regulated by iNAMPT by mediating the biosynthetic activity of NAD. Abundant evidence has proven the therapeutic potential of iNAMPT in HD. How iNAMPT regulates HD mouse models and induced pluripotent stem cells (iPSCs) derived from HD patients’ needs thorough analyses in the future.
Currently, there are no drugs that can prevent neuron death in neurodegenerative diseases. Owing to the regulatory effects on cell metabolism, mitochondrial dysfunction, inflammatory response, and oxidative stress, NAMPT has been extensively analyzed in the treatment of cancers, obesity, and other diseases (Revollo et al., 2007; Sampath et al., 2015; Zhang H. et al., 2019). However, its application in the treatment of neurodegenerative diseases has been rarely reported. So far, the significance of NAMPT in the proliferation, self-renewal, and differentiation of NSPCs has been reported (Wang et al., 2016). It is believed that NAMPT can be a promising therapeutic strategy for neurodegenerative diseases.
Current Progress of Nicotinamide Phosphoribosyltransferase-Based Drugs
Nicotinamide Phosphoribosyltransferase Inhibitors in Clinical Stage
In 2002, the first nanomolar inhibitor FK866 (compound 1, also known as APO866 and WK175) appeared. Then, Hasmann reported the inhibition and antitumor effects of FK866 on NAMPT (Hasmann and Schemainda, 2003). Subsequently, the research studies showed that FK866 and nicotinamide competed for the same site, and FK866 had a higher affinity for the site (Ki = 0.3 nM) than niacinamide, the natural substrate of NAMPT (Km = 2 µM). Early studies have shown that FK866 can significantly reduce intracellular NAD levels, resulting in delayed apoptosis of human leukemia cells and death of HEPG2 hepatoma cells (Wosikowski et al., 2002). The X-ray crystal structure of FK866 and NAMPT complex further promoted the research and development of NAMPT inhibitors (Khan et al., 2006). Currently, the NAMPT inhibitor FK866 had completed phase II trials (NCT00435084, NCT00432107, and NCT00431912). In addition, CHS828 (compound 2, also known as GMX1778) with a similar structure had been shown to inhibit intracellular NAD synthesis (Olesen et al., 2008). Pharmacological studies demonstrated that NAMPT was the main biological target for the cytotoxic activity of GMX1778 and that GMX1778 was a competitive inhibitor (Watson et al., 2009). However, its clinical application had been limited by the problems such as short half-life, thrombocytopenia, and gastrointestinal toxicity. Finally, CHS828 was withdrawn in phase I trials (NCT00003979) (Hovstadius et al., 2002). To overcome the pharmacokinetic and solubility problems observed in early clinical trials of CHS828, a water-soluble prodrug of CHS828, EB1627, later renamed GMX1777 (compound 3), was synthesized and it could be administered intravenously. EB1627 showed synergistic antitumor activity in mice when combined with etoposide (Binderup et al., 2005). Unfortunately, GMX1777 was withdrawn in phase I trials (NCT00457574) and terminated in phase II (NCT00724841).
Recently, Korotchkina et al. identified a small molecule, OT-82 (compound 4), characterized by pyrazole groups, that showed no cardiac, neurological, or retinal toxicity compared to other NAMPT inhibitors. Research showed strong dependence of neoplastic cells of hematopoietic origin on NAMPT; therefore, OT-82 may be used for hematological malignancies treatment. OT-82 is enrolled in a phase I clinical trial (NCT03921879) to evaluate patients for lymphoma (Korotchkina et al., 2020). KPT9274 (compound 5) was a dual inhibitor of p21-activated kinase (PAK4) and NAMPT, and the IC50 of KPT9274 was about 120 nM (Figure 3). Moreover, KPT9274 showed strong cytotoxic activity against B-ALL cells. KPT-9274 was being evaluated in a phase I clinical trial in solid tumors and lymphomas (NCT02702492) (Abu et al., 2016). It is currently evaluated in phase I trials enrolling patients with advanced solid tumors or non-Hodgkin’s lymphoma acute myeloid leukemia (NCT04281420 and NCT04914845).
Small-Molecule Inhibitors of Nicotinamide Phosphoribosyltransferase
Based on the phase II NAMPT inhibitor FK866, Zhang et al. explored the structure–activity relationship and obtained the derivative compound 6 containing diaryl as a new NAMPT inhibitor (IC50 = 5.08 nM) (Zhang K. et al., 2019). Colombano et al. used click chemistry for compound optimization and structure–activity relationship studies. Representative compound 7 (GPP78), a triazole-based NAMPT inhibitor, reduced the IC50 of cellular NAD by 3.0 nM. But there were defects of low water solubility and metabolic instability (Colombano et al., 2010). On this basis, Travelli et al. reconstructed the tail group of inhibitors and obtained a novel triazoli–nicotinamide phosphate ribotransferase (NAMPT) inhibitor compound 8, which was the most characteristic compound in the series, with an IC50 of 3.8 nM. It was active in vitro and in vivo (t1/2 = 1.89 h) and could significantly reduce the damage of colitis model (Travelli et al., 2019). With the introduction of profitable N, N-dialkyl methylamine groups, we found an experimental/pharmacological tool that specifically targets the extracellular cytokine eNAMPT inhibitor compound 9 (IC50 = 13.6 nM), which could not cross the plasma membrane, and can play a role in cell biology. In addition, the author stumbled upon compound 10 (IC50 = 3.4 nM) that crossed the plasma membrane and was cytotoxic, effectively reducing the growth of triple negative breast cancer in mice without retinal and cardiotoxicity (Travelli et al., 2017) (Figure 4).
Using high-throughput screening, Wang et al. identified a non-fluorescent compound F671-0003 (compound 11) and a fluorescent compound M049-0244 (compound 12), which showed excellent in vitro activity (IC50 = 85 and 170 nM, respectively) and anti-proliferation activity of HepG2 cells (IC50 = 1.69 and 1.95 μM, respectively). M049-0244 is an antitumor lead compound and a fluorescent probe for living cell imaging NAMPT. The pharmacokinetic characteristics and in vivo distribution of these inhibitors remained to be further studied (Wang et al., 2015). Based on silico screening and low-throughput screening, Takeuchi et al. found that the representative compound AS1604498 (compound 13) was the most effective inhibitor with an IC50 of 44 nM, which could be used as a hit compound to develop a new treatment for NAMPT (Takeuchi et al., 2014).
Curtin et al. identified a variety of isoindoline analogs by using crystallographic-driven structure-based drug design and structure–activity relationship optimization. Representative compounds 14 and 15 (A-1293201) had strong anti-tumor proliferation activity, with IC50 values of 24 and 56 nM against PC-3 (human prostate cancer) cells, respectively (Figure 5). Both had good oral bioavailability with F values of 100 and 59%, respectively (Curtin et al., 2017). X-ray crystallography results showed that 15 isoindoline urea was partially involved in important pi-stacking interactions and hydrogen bonding and hydrophobic interactions. The authors confirmed that isoindoline urea compounds were the first known effective nonphosphate ribonucleic NAMPT inhibitors compared to FK866 (Wilsbacher et al., 2017).
Zheng et al. obtained a series of 1H-pyrazolo [3,4-b] pyridine-containing inhibitors through reasonable structure design. Representative compound 16 effectively inhibited NAMPT with an IC50 value of 6.1 nM and showed good anti-proliferation activity against A2780 cells with an IC50 value of 4.3 nM. In addition, compound 16 showed encouraging in vivo efficacy in a mouse xenograft tumor model derived from the A2780 cell line (Zheng et al., 2013a). In the same year, this group designed a class of effective amide inhibitors containing azazindole components. Representative compound 17 (GNE-617) significantly inhibited NAMPT with an IC50 value of 5 nM and inhibited A2780 cell proliferation with an IC50 value of 2 nM. The crystal structure of compound 17 and NAMPT complex revealed hydrogen bonding between amide NH and Asp219 and water-mediated hydrogen bonding between amide carbonyl group and Ser275(Zheng et al., 2013b). Subsequently, Zak et al. optimized and obtained compound 18, which improved the time-dependent inhibition (TDI) of cytochrome P450 CYP3A4 subtype and the deficiency of low water solubility (Zak et al., 2015).
Compared with previous NAMPT inhibitors containing urea and amide, the 2, 3-dihydro-1H-pyrrole [3, 4-C] pyridine-derived compound 19 optimized by Dragovich et al. showed improved water solubility and strong NAMPT inhibitory activity (IC50 = 11 nM). PC-3 cell proliferation was effectively inhibited (IC50 = 36 nM) (Dragovich et al., 2013). Zak et al. found that compound 19 inhibited cytochrome P450 CYP2C9 subtype effectively (IC50 = 0.08 μM), which may cause unnecessary drug–drug interactions. Further optimization of pharmacodynamics and ADME properties led to the discovery of compound 20. Compound 20 was an effective intracellular NAMPT inhibitor (IC50 = 5.5 nM) and had minimal inhibition on CYP2C9 (IC50 > 10 μM) (Zak et al., 2016). Gunzner-toste et al. synthesized and optimized compound 21 that did not inhibit CYP2C9, significantly inhibiting NAMPT activity (IC50 = 3 nM) and inhibiting A2780 cell proliferation with an IC50 value of 70 nM (Gunzner-Toste et al., 2013). Giannetti et al. used surface plasmon resonance (SPR) method to conduct extensive segment-based screening and structural design. Compound 22 containing trans-2-(pyridine-3-yl) cyclopropane-carboxamide is a representative NAMPT inhibitor (IC50 = 5.1 nM) (Figure 6). Encouraging in vivo efficacy was demonstrated in HT-1080 mouse xenograft tumor models (Giannetti et al., 2014).
Subsequently, compound 23, which was designed by Dragovich et al. based on the fragment, exerted anti-proliferation effect on A2780 cells through NAMPT-mediated mechanism (NAMPT, IC50 = 0.019 μM; A2780, IC50 = 0.12 μM). The crystal structure showed that a hydrogen bond was observed between the amide NH portion of compound 23 and the Asp219 residue, and the water-mediated hydrogen bond was formed with two oxygen atoms in the terminal phenylsulfonamide portion (Dragovich et al., 2014). In addition, Zheng et al., through virtual screening and reasonable structural design, found that 3-pyridyl group was the preferred substitution group for inhibitor terminal, and determined that a urea moiety was the best ligand. The optimal compound 24 exhibited excellent biochemical and cellular potency (NAMPT, IC50 = 7 nM; A2780, IC50 = 32 nM) and reasonable PK properties in mice with a t1/2 of 1.3 h and oral bioavailability of 26% (Zheng et al., 2013c). In 2014, the author also designed a new class of cyanoguanidine-containing inhibitors containing sulfone through molecular modeling and structure–activity relationship studies. The IC50 value of representative compound 25 to NAMPT was 4.4 nM (Zheng et al., 2014).
The pharmacophore-based virtual screening study by Ozgencil et al. identified compounds GF4 (compound 26) and GF8 (compound 27) as new urea-typed inhibitors of NAMPT with IC50 values of 2.15 and 7.31 μM, respectively, which also showed cytotoxicity against HepG2 cells (Figure 7). The IC50 values were 15.2 and 24.28 μM, respectively (Ozgencil et al., 2020).
Palacios et al. obtained compound 28 through phenotypic screening and structure-based drug design driven by crystallography, and compound 28 had good in vivo characteristics (t1/2 = 90 min). In addition, the author found that the (S, S) cyclopropylcarboxamide and the (S)-N-1-phenylethylamide were key functional groups that enhanced the activity of NAMPT inhibitors (Palacios et al., 2018). Through rational drug design, the author also found that unsubstituted pyridine provided an optimal mix of cellular potency and ADME properties, resulting in a highly potent NAMPT inhibitor 29, which effectively inhibited A2780 cell proliferation with an IC50 value of 0.7 nM (Palacios et al., 2019).
Based on virtual screening and rational structural design, a highly selective NAMPT inhibitor LSN3154567 (compound 30) was identified, which could not cause significant retinal and hematological toxicity in rodents, but still retained strong potency (IC50 = 3.1 nM) (Zhao et al., 2017). Lameijer et al., using a novel prodrug strategy of photoactivated chemotherapy (PACT), obtained two water-soluble ruthenium complexes (compounds 31 and 32) that were inherently inactive. Compounds 31 and 32 had an 18-fold increase in inhibitory effect when exposed to red light (Lameijer et al., 2017). Chemical genome analysis was an effective method to elucidate the target and mechanism of compounds. Estoppey et al. pioneered the use of CRISPR/Cas9 chemogenomic profiling to identify LB-60-OF61 (compound 33) with an IC50 of NAMPT about 15 nM (Estoppey et al., 2017). Lockman et al. found that 3-pyridyl formamide substituent was critical to NAMPT activity and cytotoxicity. The best compound 34 showed nanomolar NAMPT inhibition with an IC50 value of 0.3 nM (Lockman et al., 2010). Compound 35 was shown to be an inhibitor of NAMPT (IC50 = 242.7 nM) by virtual screening based on structure and ligand, and showed a protective effect when tested in axonal cell models, suggesting that inhibition of NAMPT may be an effective treatment for neurodegenerative diseases (Clark et al., 2016) (Figure 8).
Dual-Target Inhibitors
Given the efficacy of drug combinations, and in order to overcome the pharmacokinetic problems associated with the simultaneous use of two drugs, the strategy pursued in drug development is the synthesis of hybrid molecules that exhibit two different and well-balanced mechanisms of action. With the efforts of pharmaceutical researchers, many NAMPT inhibitors with dual modes of action have made breakthroughs.
Stf-31 (compound 36) developed by Kraus et al. has a dual mode of action and was an inhibitor of NAMPT and glucose transporter (GLUT), while the low expression level of NAMPT may be conducive to NAMPT-specific inhibition of the drug (Kraus et al., 2018). Through structure-based drug design, chemical synthesis, and biological analysis, Dong et al. successfully identified a highly efficient dual inhibitor of NAMPT and histone deacetylase (HDAC). Compound 37 showed good and balanced activities against NAMPT (IC50 = 31 nM) and HDAC1 (IC50 = 55 nM). The crystal structure showed the binding patterns of compound 37 with NAMPT and HDAC1, including π−π stacking and hydrogen bond interactions. In addition, it could effectively induce apoptosis and autophagy leading to cell death and had shown excellent in vivo antitumor effect in HCT116 xenograft model (Dong et al., 2017). Chen et al. in the same group also designed a class of novel NAMPT and HDAC dual inhibitors by pharmacophore fusion method, in which the representative compound 38 was highly active against the two targets (NAMPT, IC50 = 15 nM; HDAC1, IC50 = 2 nM) (Figure 9) (Chen et al., 2018).
eNicotinamide Phosphoribosyltransferase-Neutralizing Antibody and Nicotinamide Phosphoribosyltransferasei Antibody–Drug Conjugates
Compared to traditional small-molecule NAMPT inhibitors, antibody–drug conjugates (ADCs) can selectively target cancer cells through antibodies binding to cancer-specific cell surface markers, thereby sparing normal cells from systemic NAD depletion. The dose-toxicity problems of small molecule inhibitors in clinical trials may be solved by ADCs comprising NAMPT inhibitors (NAMPTi–ADCs).
Targeting eNAMPT rather than inhibiting iNAMPT is expected to lead to safer and more effective treatments. The receptor-binding mode may be a reasonable mechanism for mediating eNAMPT cytokine-like activity. At present, toll-like receptor 4 (TLR4) is considered as putative eNAMPT receptors. eNAMPT, as a key innate immune regulator and potent damage-associated molecular pattern protein, activates an inflammatory cascade by linking TLR4 (Camp et al., 2015). Garcia’s group’s studies proved that NAMPT was strongly expressed in invasive prostate cancer tissues, especially plasma eNAMPT was significantly increased in patients with extra-prostatic invasion. eNAMPT enhanced the aggressiveness of the tumor (Sun et al., 2020). eNAMPT-neutralized humanized monoclonal antibody (ALT-100 mAb) was used to achieve effective therapeutic effect in preclinical prostate cancer (PCa) orthotopic xenotransplantation model. ALT-100 mAb not only inhibited NFκB phosphorylation and signal transduction in PCa cells in vivo and in vitro but also reduced PCa proliferation, local invasion, and distal metastasis, further inducing tumor necrosis (Sun et al., 2021). In acute respiratory distress syndrome, eNAMPT-neutralizing antibody [polyclonal antibody (pAb) or mAb] can eliminate eNAMPT-induced activation of the TLR4 pathway and disruption of the endothelial cell barrier. Intravenous administration of eNAMPT-neutralizing antibody significantly reduces the severity of acute inflammatory lung injury in mice. Therefore, eNAMPT neutralizing humanized monoclonal antibody can be used as a highly effective targeted biotherapy (Quijada et al., 2021).
eNAMPT-neutralizing antibodies are endowed with a therapeutic potential in inflammatory bowel disease. Colombo et al. reported a monoclonal antibody (C269) that neutralizes the eNAMPT cytokine-like activity while sparing the enzymatic activity. It was demonstrated that serum eNAMPT may be a predictor of anti-TNF response and that neutralization of C269 interrupts cytokines and cellular circuits that promote inflammatory bowel disease pathology in the absence of enzyme inhibition (Colombo et al., 2020). IgG-8 is a non-conjugated NAMPTi-ADC that has significantly improved toxicological characteristics. ADC delivery may provide the best form of NAMPT inhibition. ADCs have a long circulating half-life in vivo and may expose the drug to continuous antigen-positive target cells (Neumann et al., 2018).
Nicotinamide Phosphoribosyltransferase Agonists in Development
Pieper and colleagues used in vivo screening to identify the first activator of NAMPT, P7C3 (compound 39), with good pharmacokinetic properties and appropriate bioavailability and half-life. P7C3 protects newborn neurons from death, as well as existing mature neurons from dying in neurodegenerative diseases (Pieper et al., 2010). P7C3-A20 (compound 40), a derivative of P7C3, also prevented neuronal cell death and increased the number of new neurons (Wang et al., 2016) and up-regulated brain NAD level during ischemic stress, playing a neuroprotective role in neurodegenerative diseases (Vazquez-Rosa et al., 2020). In 2021, Wang et al. obtained an effective NAMPT activator NAT-5r (compound 41) through high-throughput screening and structural optimization. NAT-5r showed a KD value of 132 nM and an EC50 value of 2.6 μM. This is the first study on the structure–activity relationship of NAMPT activator based on the co-crystal structure (Wang et al., 2022). NAT-5r can increase intracellular NAD levels and induce subsequent metabolic and transcriptional reprogramming. In addition, it effectively protects cultured cells from FK866-mediated toxicity. Strong neuroprotective efficacy in mouse models of chemotherapy-induced peripheral neuropathy without any apparent toxicity demonstrated the potential of NAMPT activator in treating neurodegenerative diseases or diseases associated with decreased NAD levels (Yao et al., 2022).
Gardell et al. obtained SBI-797812 (compound 42) (EC50 = 0.279 μM) from a high-throughput screening of NAMPT activator and hit-to-lead campaign, which was structurally similar to active site-directed NAMPT inhibitors. The binding of these inhibitors to NAMPT could be blocked. The pyridine nitrogen was shifted from position 4 in SBI-797812 to position 2 or 3 to change the compound from NAMPT activator to inhibitor (Gardell et al., 2019). The resulting compound 43 showed more efficient NAMPT activation (EC50 = 0.023 μM) and improved oral bioavailability (Pinkerton et al., 2021). DS68702229 (compound 44) was identified as a potent NAMPT activator (EC50 = 0.046 μM) that increases cellular NAD+ levels and is a promising anti-obesity drug candidate (Akiu et al., 2021a). Optimized by high-throughput screening, the same research group found pyridine-4-methyl urea derivative with double-ring core structure. SAR study proved that reducing logD was beneficial to reducing CYP inhibition, which resulted in compound 45 (Figure 10), which had the problem of low membrane permeability and still needed further optimization (Akiu et al., 2021b).
Previous studies mainly focus on the anti-cancer functions of NAMPT inhibitors, while their potential neuroprotective effect has been recently highlighted. They can improve neurodegenerative disease-induced cognitive impairment. Therefore, NAMPT inhibitors are novel candidates for the treatment of neurodegenerative diseases. At present, the research on small molecule compounds to activate NAMPT is initiated in the infant stage, which requires in-depth explorations in the future, thus benefiting people who suffer from neurodegenerative diseases.
Summary and Prospect
Globally, millions of people are affected by neurodegenerative diseases. Current therapeutic strategies can only alleviate or control clinical symptoms and signs of neurodegenerative diseases, rather than cure them. Abundant in vivo and in vitro evidence has proven the role of NAMPT in the self-renewal and proliferation of neurons. The NAMPT–NAD–SIRT cascade, which is validated as a strong intrinsic defense system, fights against energy consumption and neuron death in neurodegenerative disease cases. Therefore, we believed that NAMPT is a very promising therapeutic target for neurodegenerative diseases.
Very latest, great efforts have been made on clarifying biological functions of NAMPT, especially the extracellular functions. Meanwhile, research on developing NAMPT agonists and inhibitors has achieved satisfactory outcomes. Small-molecule NAMPT inhibitors remarkably eliminate tumor lesions in a short period by downregulating NAD. However, effective NAMPT agonists or inhibitors in the application of neurodegenerative diseases are very scant. It is urgent and essential to clearly elucidate the mechanism underlying the neuroprotective effect of NAMPT and to develop more effective NAMPT-target drugs with less adverse events for neurodegenerative diseases.
Author Contributions
All authors listed have made a substantial, direct, and intellectual contribution to the work and approved it for publication.
Funding
This work was supported by the National Natural Science Foundation of China (Grant 22177083), Sichuan Science and Technology Program (Grant 2019YFS0003), Open Foundation of Artificial Intelligence Key Laboratory of Sichuan Province (Grant 2020RYY03), and West China Nursing Discipline Development Special Fund Project, Sichuan University (Grant HXHL21011).
Conflict of Interest
The authors declare that the research was conducted in the absence of any commercial or financial relationships that could be construed as a potential conflict of interest.
Publisher’s Note
All claims expressed in this article are solely those of the authors and do not necessarily represent those of their affiliated organizations, or those of the publisher, the editors, and the reviewers. Any product that may be evaluated in this article, or claim that may be made by its manufacturer, is not guaranteed or endorsed by the publisher.
References
Abu Aboud, O., Chen, C. H., Senapedis, W., Baloglu, E., Argueta, C., and Weiss, R. H. (2016). Dual and Specific Inhibition of NAMPT and PAK4 by KPT-9274 Decreases Kidney Cancer Growth. Mol. Cancer Ther. 15 (9), 2119–2129. doi:10.1158/1535-7163.MCT-16-0197
Adeoye, O., Hornung, R., Khatri, P., and Kleindorfer, D. (2011). Recombinant Tissue-type Plasminogen Activator Use for Ischemic Stroke in the United States: a Doubling of Treatment Rates over the Course of 5 Years. Stroke 42 (7), 1952–1955. doi:10.1161/STROKEAHA.110.612358
Akiu, M., Tsuji, T., Iida, K., Sogawa, Y., Terayama, K., Yokoyama, M., et al. (2021a). Discovery of DS68702229 as a Potent, Orally Available NAMPT (Nicotinamide Phosphoribosyltransferase) Activator. Chem. Pharm. Bull. (Tokyo) 69 (11), 1110–1122. doi:10.1248/cpb.c21-00700
Akiu, M., Tsuji, T., Sogawa, Y., Terayama, K., Yokoyama, M., Tanaka, J., et al. (2021b). Discovery of 1-[2-(1-Methyl-1h-Pyrazol-5-Yl)-[1,2,4]triazolo[1,5-A]pyridin-6-Yl]-3-(pyridin-4-Ylmethyl)urea as a Potent NAMPT (Nicotinamide Phosphoribosyltransferase) Activator with Attenuated CYP Inhibition. Bioorg. Med. Chem. Lett. 43, 128048. doi:10.1016/j.bmcl.2021.128048
Aso, E., and Ferrer, I. (2013). It May Be Possible to Delay the Onset of Neurodegenerative Diseases with an Immunosuppressive Drug (Rapamycin). Expert Opin. Biol. Ther. 13 (9), 1215–1219. doi:10.1517/14712598.2013.799129
Audrito, V., Messana, V. G., and Deaglio, S. (2020). NAMPT and NAPRT: Two Metabolic Enzymes with Key Roles in Inflammation. Front. Oncol. 10, 358. doi:10.3389/fonc.2020.00358
Binderup, E., Björkling, F., Hjarnaa, P. V., Latini, S., Baltzer, B., Carlsen, M., et al. (2005). EB1627: a Soluble Prodrug of the Potent Anticancer Cyanoguanidine CHS828. Bioorg. Med. Chem. Lett. 15 (10), 2491–2494. doi:10.1016/j.bmcl.2005.03.064
Busso, N., Karababa, M., Nobile, M., Rolaz, A., Van Gool, F., Galli, M., et al. (2008). Pharmacological Inhibition of Nicotinamide Phosphoribosyltransferase/visfatin Enzymatic Activity Identifies a New Inflammatory Pathway Linked to NAD. PLoS One 3 (5), e2267. doi:10.1371/journal.pone.0002267
Camp, S. M., Ceco, E., Evenoski, C. L., Danilov, S. M., Zhou, T., Chiang, E. T., et al. (2015). Unique Toll-like Receptor 4 Activation by NAMPT/PBEF Induces NFκB Signaling and Inflammatory Lung Injury. Sci. Rep. 5, 13135. doi:10.1038/srep13135
Carbone, F., Liberale, L., Bonaventura, A., Vecchiè, A., Casula, M., Cea, M., et al. (2017). Regulation and Function of Extracellular Nicotinamide Phosphoribosyltransferase/Visfatin. Compr. Physiol. 7 (2), 603–621. doi:10.1002/cphy.c160029
Chen, H., Wang, S., Zhang, H., Nice, E. C., and Huang, C. (2016). Nicotinamide Phosphoribosyltransferase (Nampt) in Carcinogenesis: New Clinical Opportunities. Expert Rev. Anticancer Ther. 16 (8), 827–838. doi:10.1080/14737140.2016.1190649
Chen, W., Dong, G., Wu, Y., Zhang, W., Miao, C., and Sheng, C. (2018). Dual NAMPT/HDAC Inhibitors as a New Strategy for Multitargeting Antitumor Drug Discovery. ACS Med. Chem. Lett. 9 (1), 34–38. doi:10.1021/acsmedchemlett.7b00414
Chen, X., Zhao, S., Song, Y., Shi, Y., Leak, R. K., and Cao, G. (2015). The Role of Nicotinamide Phosphoribosyltransferase in Cerebral Ischemia. Curr. Top. Med. Chem. 15 (21), 2211–2221. doi:10.2174/1568026615666150610142234
Clark, D. E., Waszkowycz, B., Wong, M., Lockey, P. M., Adalbert, R., Gilley, J., et al. (2016). Application of Virtual Screening to the Discovery of Novel Nicotinamide Phosphoribosyltransferase (NAMPT) Inhibitors with Potential for the Treatment of Cancer and Axonopathies. Bioorg. Med. Chem. Lett. 26 (12), 2920–2926. doi:10.1016/j.bmcl.2016.04.039
Colombano, G., Travelli, C., Galli, U., Caldarelli, A., Chini, M. G., Canonico, P. L., et al. (2010). A Novel Potent Nicotinamide Phosphoribosyltransferase Inhibitor Synthesized via Click Chemistry. J. Med. Chem. 53 (2), 616–623. doi:10.1021/jm9010669
Colombo, G., Clemente, N., Zito, A., Bracci, C., Colombo, F. S., Sangaletti, S., et al. (2020). Neutralization of Extracellular NAMPT (Nicotinamide Phosphoribosyltransferase) Ameliorates Experimental Murine Colitis. J. Mol. Med. Berl. 98 (4), 595–612. doi:10.1007/s00109-020-01892-0
Cummings, J. L., Morstorf, T., and Zhong, K. (2014). Alzheimer's Disease Drug-Development Pipeline: Few Candidates, Frequent Failures. Alzheimers Res. Ther. 6 (4), 37. doi:10.1186/alzrt269
Cummings, J. L., Tong, G., and Ballard, C. (2019). Treatment Combinations for Alzheimer's Disease: Current and Future Pharmacotherapy Options. J. Alzheimers Dis. 67 (3), 779–794. doi:10.3233/JAD-180766
Curtin, M. L., Heyman, H. R., Clark, R. F., Sorensen, B. K., Doherty, G. A., Hansen, T. M., et al. (2017). SAR and Characterization of Non-substrate Isoindoline Urea Inhibitors of Nicotinamide Phosphoribosyltransferase (NAMPT). Bioorg. Med. Chem. Lett. 27 (15), 3317–3325. doi:10.1016/j.bmcl.2017.06.018
De Virgilio, A., Greco, A., Fabbrini, G., Inghilleri, M., Rizzo, M. I., Gallo, A., et al. (2016). Parkinson's Disease: Autoimmunity and Neuroinflammation. Autoimmun. Rev. 15 (10), 1005–1011. doi:10.1016/j.autrev.2016.07.022
Dirnagl, U., and Endres, M. (2014). Found in Translation: Preclinical Stroke Research Predicts Human Pathophysiology, Clinical Phenotypes, and Therapeutic Outcomes. Stroke 45 (5), 1510–1518. doi:10.1161/STROKEAHA.113.004075
Dong, G., Chen, W., Wang, X., Yang, X., Xu, T., Wang, P., et al. (2017). Small Molecule Inhibitors Simultaneously Targeting Cancer Metabolism and Epigenetics: Discovery of Novel Nicotinamide Phosphoribosyltransferase (NAMPT) and Histone Deacetylase (HDAC) Dual Inhibitors. J. Med. Chem. 60 (19), 7965–7983. doi:10.1021/acs.jmedchem.7b00467
Dragovich, P. S., Bair, K. W., Baumeister, T., Ho, Y. C., Liederer, B. M., Liu, X., et al. (2013). Identification of 2,3-Dihydro-1h-Pyrrolo[3,4-C]pyridine-Derived Ureas as Potent Inhibitors of Human Nicotinamide Phosphoribosyltransferase (NAMPT). Bioorg. Med. Chem. Lett. 23 (17), 4875–4885. doi:10.1016/j.bmcl.2013.06.090
Dragovich, P. S., Zhao, G., Baumeister, T., Bravo, B., Giannetti, A. M., Ho, Y. C., et al. (2014). Fragment-based Design of 3-Aminopyridine-Derived Amides as Potent Inhibitors of Human Nicotinamide Phosphoribosyltransferase (NAMPT). Bioorg. Med. Chem. Lett. 24 (3), 954–962. doi:10.1016/j.bmcl.2013.12.062
Dugger, B. N., and Dickson, D. W. (2017). Pathology of Neurodegenerative Diseases. Cold Spring Harb. Perspect. Biol. 9 (7). doi:10.1101/cshperspect.a028035
Elsworth, J. D. (2020). Parkinson's Disease Treatment: Past, Present, and Future. J. Neural Transm. (Vienna) 127 (5), 785–791. doi:10.1007/s00702-020-02167-1
Estoppey, D., Hewett, J. W., Guy, C. T., Harrington, E., Thomas, J. R., Schirle, M., et al. (2017). Identification of a Novel NAMPT Inhibitor by CRISPR/Cas9 Chemogenomic Profiling in Mammalian Cells. Sci. Rep. 7, 42728. doi:10.1038/srep42728
Friebe, D., Neef, M., Kratzsch, J., Erbs, S., Dittrich, K., Garten, A., et al. (2011). Leucocytes Are a Major Source of Circulating Nicotinamide Phosphoribosyltransferase (NAMPT)/pre-B Cell Colony (PBEF)/visfatin Linking Obesity and Inflammation in Humans. Diabetologia 54 (5), 1200–1211. doi:10.1007/s00125-010-2042-z
Fukuhara, A., Matsuda, M., Nishizawa, M., Segawa, K., Tanaka, M., Kishimoto, K., et al. (2005). Visfatin: A Protein Secreted by Visceral Fat that Mimics the Effects of Insulin. Science 307, 426–430. doi:10.1126/science.1097243
Galli, U., Travelli, C., Massarotti, A., Fakhfouri, G., Rahimian, R., Tron, G. C., et al. (2013). Medicinal Chemistry of Nicotinamide Phosphoribosyltransferase (NAMPT) Inhibitors. J. Med. Chem. 56 (16), 6279–6296. doi:10.1021/jm4001049
Gao, H. M., and Hong, J. S. (2008). Why Neurodegenerative Diseases Are Progressive: Uncontrolled Inflammation Drives Disease Progression. Trends Immunol. 29 (8), 357–365. doi:10.1016/j.it.2008.05.002
Gardell, S. J., Hopf, M., Khan, A., Dispagna, M., Hampton Sessions, E., Falter, R., et al. (2019). Boosting NAD(+) with a Small Molecule that Activates NAMPT. Nat. Commun. 10 (1), 3241. doi:10.1038/s41467-019-11078-z
Garten, A., Petzold, S., Körner, A., Imai, S., and Kiess, W. (2009). Nampt: Linking NAD Biology, Metabolism and Cancer. Trends Endocrinol. Metab. 20 (3), 130–138. doi:10.1016/j.tem.2008.10.004
Garten, A., Schuster, S., Penke, M., Gorski, T., de Giorgis, T., and Kiess, W. (2015). Physiological and Pathophysiological Roles of NAMPT and NAD Metabolism. Nat. Rev. Endocrinol. 11 (9), 535–546. doi:10.1038/nrendo.2015.117
Giannetti, A. M., Zheng, X., Skelton, N. J., Wang, W., Bravo, B. J., Bair, K. W., et al. (2014). Fragment-based Identification of Amides Derived from Trans-2-(pyridin-3-yl)cyclopropanecarboxylic Acid as Potent Inhibitors of Human Nicotinamide Phosphoribosyltransferase (NAMPT). J. Med. Chem. 57 (3), 770–792. doi:10.1021/jm4015108
Golpich, M., Amini, E., Mohamed, Z., Azman Ali, R., Mohamed Ibrahim, N., and Ahmadiani, A. (2017b). Mitochondrial Dysfunction and Biogenesis in Neurodegenerative Diseases: Pathogenesis and Treatment. CNS Neurosci. Ther. 23 (1), 5–22. doi:10.1111/cns.12655
Gunzner-Toste, J., Zhao, G., Bauer, P., Baumeister, T., Buckmelter, A. J., Caligiuri, M., et al. (2013). Discovery of Potent and Efficacious Urea-Containing Nicotinamide Phosphoribosyltransferase (NAMPT) Inhibitors with Reduced CYP2C9 Inhibition Properties. Bioorg. Med. Chem. Lett. 23 (12), 3531–3538. doi:10.1016/j.bmcl.2013.04.040
Hara, N., Yamada, K., Shibata, T., Osago, H., and Tsuchiya, M. (2011). Nicotinamide Phosphoribosyltransferase/visfatin Does Not Catalyze Nicotinamide Mononucleotide Formation in Blood Plasma. PLoS One 6 (8), e22781. doi:10.1371/journal.pone.0022781
Hardiman, O., Al-Chalabi, A., Chio, A., Corr, E. M., Logroscino, G., Robberecht, W., et al. (2017a). Amyotrophic Lateral Sclerosis. Nat. Rev. Dis. Prim. 3, 17071. doi:10.1038/nrdp.2017.71
Harlan, B. A., Pehar, M., Killoy, K. M., and Vargas, M. R. (2019). Enhanced SIRT6 Activity Abrogates the Neurotoxic Phenotype of Astrocytes Expressing ALS-Linked Mutant SOD1. FASEB J. 33 (6), 7084–7091. doi:10.1096/fj.201802752R
Harlan, B. A., Pehar, M., Sharma, D. R., Beeson, G., Beeson, C. C., and Vargas, M. R. (2016). Enhancing NAD+ Salvage Pathway Reverts the Toxicity of Primary Astrocytes Expressing Amyotrophic Lateral Sclerosis-Linked Mutant Superoxide Dismutase 1 (SOD1). J. Biol. Chem. 291 (20), 10836–10846. doi:10.1074/jbc.M115.698779
Hasmann, M., and Schemainda, I. (2003). FK866, a Highly Specific Noncompetitive Inhibitor of Nicotinamide Phosphoribosyltransferase, Represents a Novel Mechanism for Induction of Tumor Cell Apoptosis. Cancer Res. 63 (21), 7436–7442.
Hattingen, E., Magerkurth, J., Pilatus, U., Mozer, A., Seifried, C., Steinmetz, H., et al. (2009). Phosphorus and Proton Magnetic Resonance Spectroscopy Demonstrates Mitochondrial Dysfunction in Early and Advanced Parkinson's Disease. Brain 132 (Pt 12), 3285–3297. doi:10.1093/brain/awp293
He, Z., Ning, N., Zhou, Q., Khoshnam, S. E., and Farzaneh, M. (2020a). Mitochondria as a Therapeutic Target for Ischemic Stroke. Free Radic. Biol. Med. 146, 45–58. doi:10.1016/j.freeradbiomed.2019.11.005
Henning, R. J., Bourgeois, M., and Harbison, R. D. (2018). Poly(ADP-ribose) Polymerase (PARP) and PARP Inhibitors: Mechanisms of Action and Role in Cardiovascular Disorders. Cardiovasc. Toxicol. 18 (6), 493–506. doi:10.1007/s12012-018-9462-2
Hobson, E. V., and Mcdermott, C. J. (2016). Supportive and Symptomatic Management of Amyotrophic Lateral Sclerosis. Nat. Rev. Neurol. 12 (9), 526–538. doi:10.1038/nrneurol.2016.111
Hovstadius, P., Larsson, R., Jonsson, E., Skov, T., Kissmeyer, A. M., Krasilnikoff, K., et al. (2002). A Phase I Study of CHS 828 in Patients with Solid Tumor Malignancy. Clin. Cancer Res. 8 (9), 2843–2850.
Imai, S., and Guarente, L. (2014a). NAD+ and Sirtuins in Aging and Disease. Trends Cell Biol. 24 (8), 464–471. doi:10.1016/j.tcb.2014.04.002
Imai, S., and Yoshino, J. (2013). The Importance of NAMPT/NAD/SIRT1 in the Systemic Regulation of Metabolism and Ageing. Diabetes Obes. Metab. 15 Suppl 3 (Suppl. 3), 26–33. doi:10.1111/dom.12171
Jankovic, J., and Tan, E. K. (2020a). Parkinson's Disease: Etiopathogenesis and Treatment. J. Neurol. Neurosurg. Psychiatry 91 (8), 795–808. doi:10.1136/jnnp-2019-322338
Jimenez-Sanchez, M., Licitra, F., Underwood, B. R., and Rubinsztein, D. C. (2017). Huntington's Disease: Mechanisms of Pathogenesis and Therapeutic Strategies. Cold Spring Harb. Perspect. Med. 7 (7). doi:10.1101/cshperspect.a024240
Jing, Z., Xing, J., Chen, X., Stetler, R. A., Weng, Z., Gan, Y., et al. (2014). Neuronal NAMPT Is Released after Cerebral Ischemia and Protects against White Matter Injury. J. Cereb. Blood Flow. Metab. 34 (10), 1613–1621. doi:10.1038/jcbfm.2014.119
Kadoglou, N. P., Fotiadis, G., Lambadiari, V., Maratou, E., Dimitriadis, G., and Liapis, C. D. (2014). Serum Levels of Novel Adipokines in Patients with Acute Ischemic Stroke: Potential Contribution to Diagnosis and Prognosis. Peptides 57, 12–16. doi:10.1016/j.peptides.2014.04.008
Kalia, L. V., and Lang, A. E. (2015). Parkinson's Disease. Lancet 386 (9996), 896–912. doi:10.1016/S0140-6736(14)61393-3
Keating, D. J. (2008). Mitochondrial Dysfunction, Oxidative Stress, Regulation of Exocytosis and Their Relevance to Neurodegenerative Diseases. J. Neurochem. 104 (2), 298–305. doi:10.1111/j.1471-4159.2007.04997.x
Khan, J. A., Tao, X., and Tong, L. (2006). Molecular Basis for the Inhibition of Human NMPRTase, a Novel Target for Anticancer Agents. Nat. Struct. Mol. Biol. 13 (7), 582–588. doi:10.1038/nsmb1105
Khoshnam, S. E., Winlow, W., and Farzaneh, M. (2017). The Interplay of MicroRNAs in the Inflammatory Mechanisms Following Ischemic Stroke. J. Neuropathol. Exp. Neurol. 76 (7), 548–561. doi:10.1093/jnen/nlx036
Koltai, E., Szabo, Z., Atalay, M., Boldogh, I., Naito, H., Goto, S., et al. (2010). Exercise Alters SIRT1, SIRT6, NAD and NAMPT Levels in Skeletal Muscle of Aged Rats. Mech. Ageing Dev. 131 (1), 21–28. doi:10.1016/j.mad.2009.11.002
Korotchkina, L., Kazyulkin, D., Komarov, P. G., Polinsky, A., Andrianova, E. L., Joshi, S., et al. (2020). OT-82, a Novel Anticancer Drug Candidate that Targets the Strong Dependence of Hematological Malignancies on NAD Biosynthesis. Leukemia 34 (7), 1828–1839. doi:10.1038/s41375-019-0692-5
Kraus, D., Reckenbeil, J., Veit, N., Kuerpig, S., Meisenheimer, M., Beier, I., et al. (2018). Targeting Glucose Transport and the NAD Pathway in Tumor Cells with STF-31: a Re-evaluation. Cell Oncol. (Dordr) 41 (5), 485–494. doi:10.1007/s13402-018-0385-5
Kwon, H. S., and Koh, S. H. (2020a). Neuroinflammation in Neurodegenerative Disorders: the Roles of Microglia and Astrocytes. Transl. Neurodegener. 9 (1), 42. doi:10.1186/s40035-020-00221-2
Lameijer, L. N., Ernst, D., Hopkins, S. L., Meijer, M. S., Askes, S. H. C., Le Dévédec, S. E., et al. (2017). A Red-Light-Activated Ruthenium-Caged NAMPT Inhibitor Remains Phototoxic in Hypoxic Cancer Cells. Angew. Chem. Int. Ed. Engl. 56 (38), 11549–11553. doi:10.1002/anie.201703890
Lautrup, S., Sinclair, D. A., Mattson, M. P., and Fang, E. F. (2019a). NAD(+) in Brain Aging and Neurodegenerative Disorders. Cell Metab. 30 (4), 630–655. doi:10.1016/j.cmet.2019.09.001
Lee, H. C., and Aarhus, R. (1991). ADP-ribosyl Cyclase: an Enzyme that Cyclizes NAD+ into a Calcium-Mobilizing Metabolite. Cell Regul. 2 (3), 203–209. doi:10.1091/mbc.2.3.203
Lin, M. T., and Beal, M. F. (2006a). Mitochondrial Dysfunction and Oxidative Stress in Neurodegenerative Diseases. Nature 443 (7113), 787–795. doi:10.1038/nature05292
Liu, H., and Song, N. (2016). Molecular Mechanism of Adult Neurogenesis and its Association with Human Brain Diseases. J. Cent. Nerv. Syst. Dis. 8, 5–11. doi:10.4137/JCNSD.S32204
Liu, J., Che, X., You, J., Zhang, G., Zhao, R., Fu, J., et al. (2021). Intracellular Nampt Impairs Esophageal Squamous Cell Carcinoma Neo-Adjuvant Chemotherapy Response Independent of eNampt. Am. J. Transl. Res. 13 (3), 1411–1421.
Liu, L. Y., Wang, F., Zhang, X. Y., Huang, P., Lu, Y. B., Wei, E. Q., et al. (2012). Nicotinamide Phosphoribosyltransferase May Be Involved in Age-Related Brain Diseases. PLoS One 7 (10), e44933. doi:10.1371/journal.pone.0044933
Lockman, J. W., Murphy, B. R., Zigar, D. F., Judd, W. R., Slattum, P. M., Gao, Z. H., et al. (2010). Analogues of 4-[(7-Bromo-2-Methyl-4-Oxo-3h-Quinazolin-6-Yl)methylprop-2-Ynylamino]-N-(3-Pyridylmethyl)benzamide (CB-30865) as Potent Inhibitors of Nicotinamide Phosphoribosyltransferase (Nampt). J. Med. Chem. 53 (24), 8734–8746. doi:10.1021/jm101145b
Lugert, S., Basak, O., Knuckles, P., Haussler, U., Fabel, K., Götz, M., et al. (2010). Quiescent and Active Hippocampal Neural Stem Cells with Distinct Morphologies Respond Selectively to Physiological and Pathological Stimuli and Aging. Cell Stem Cell 6 (5), 445–456. doi:10.1016/j.stem.2010.03.017
Luk, T., Malam, Z., and Marshall, J. C. (2008). Pre-B Cell Colony-Enhancing Factor (PBEF)/visfatin: a Novel Mediator of Innate Immunity. J. Leukoc. Biol. 83 (4), 804–816. doi:10.1189/jlb.0807581
Lundt, S., and Ding, S. (2021). NAD(+) Metabolism and Diseases with Motor Dysfunction. Genes (Basel) 12 (11). doi:10.3390/genes12111776
Maher, P. (2019). The Potential of Flavonoids for the Treatment of Neurodegenerative Diseases. Int. J. Mol. Sci. 20 (12). doi:10.3390/ijms20123056
Maida, C. D., Norrito, R. L., Daidone, M., Tuttolomondo, A., and Pinto, A. (2020). Neuroinflammatory Mechanisms in Ischemic Stroke: Focus on Cardioembolic Stroke, Background, and Therapeutic Approaches. Int. J. Mol. Sci. 21 (18). doi:10.3390/ijms21186454
Martin, P. R., Shea, R. J., and Mulks, M. H. (2001). Identification of a Plasmid-Encoded Gene from Haemophilus Ducreyi Which Confers NAD Independence. J. Bacteriol. 183 (4), 1168–1174. doi:10.1128/JB.183.4.1168-1174.2001
Mccolgan, P., and Tabrizi, S. J. (2018). Huntington's Disease: a Clinical Review. Eur. J. Neurol. 25 (1), 24–34. doi:10.1111/ene.13413
Moutinho, M., Codocedo, J. F., Puntambekar, S. S., and Landreth, G. E. (2019). Nuclear Receptors as Therapeutic Targets for Neurodegenerative Diseases: Lost in Translation. Annu. Rev. Pharmacol. Toxicol. 59, 237–261. doi:10.1146/annurev-pharmtox-010818-021807
Naia, L., Rosenstock, T. R., Oliveira, A. M., Oliveira-Sousa, S. I., Caldeira, G. L., Carmo, C., et al. (2017). Comparative Mitochondrial-Based Protective Effects of Resveratrol and Nicotinamide in Huntington's Disease Models. Mol. Neurobiol. 54 (7), 5385–5399. doi:10.1007/s12035-016-0048-3
Neumann, C. S., Olivas, K. C., Anderson, M. E., Cochran, J. H., Jin, S., Li, F., et al. (2018). Targeted Delivery of Cytotoxic NAMPT Inhibitors Using Antibody-Drug Conjugates. Mol. Cancer Ther. 17 (12), 2633–2642. doi:10.1158/1535-7163.MCT-18-0643
Niedermeyer, S., Murn, M., and Choi, P. J. (2019). Respiratory Failure in Amyotrophic Lateral Sclerosis. Chest 155 (2), 401–408. doi:10.1016/j.chest.2018.06.035
Obrador, E., Salvador-Palmer, R., López-Blanch, R., Dellinger, R. W., and Estrela, J. M. (2021). NAD(+) Precursors and Antioxidants for the Treatment of Amyotrophic Lateral Sclerosis. Biomedicines 9 (8). doi:10.3390/biomedicines9081000
Olesen, U. H., Christensen, M. K., Björkling, F., Jäättelä, M., Jensen, P. B., Sehested, M., et al. (2008). Anticancer Agent CHS-828 Inhibits Cellular Synthesis of NAD. Biochem. Biophys. Res. Commun. 367 (4), 799–804. doi:10.1016/j.bbrc.2008.01.019
Orellana-Urzúa, S., Rojas, I., Líbano, L., and Rodrigo, R. (2020). Pathophysiology of Ischemic Stroke: Role of Oxidative Stress. Curr. Pharm. Des. 26 (34), 4246–4260. doi:10.2174/1381612826666200708133912
Ozgencil, F., Eren, G., Ozkan, Y., Guntekin-Ergun, S., and Cetin-Atalay, R. (2020). Identification of Small-Molecule Urea Derivatives as Novel NAMPT Inhibitors via Pharmacophore-Based Virtual Screening. Bioorg Med. Chem. 28 (1), 115217. doi:10.1016/j.bmc.2019.115217
Pajares, M., I Rojo, A., Manda, G., Boscá, L., and Cuadrado, A. (2020). Inflammation in Parkinson's Disease: Mechanisms and Therapeutic Implications. Cells 9 (7). doi:10.3390/cells9071687
Palacios, D. S., Meredith, E., Kawanami, T., Adams, C., Chen, X., Darsigny, V., et al. (2018). Structure Based Design of Nicotinamide Phosphoribosyltransferase (NAMPT) Inhibitors from a Phenotypic Screen. Bioorg. Med. Chem. Lett. 28 (3), 365–370. doi:10.1016/j.bmcl.2017.12.037
Palacios, D. S., Meredith, E. L., Kawanami, T., Adams, C. M., Chen, X., Darsigny, V., et al. (2019). Scaffold Morphing Identifies 3-Pyridyl Azetidine Ureas as Inhibitors of Nicotinamide Phosphoribosyltransferase (NAMPT). ACS Med. Chem. Lett. 10 (11), 1524–1529. doi:10.1021/acsmedchemlett.9b00325
Pavlová, T., Novák, J., and Bienertová-Vašků, J. (2015). The Role of Visfatin (PBEF/Nampt) in Pregnancy Complications. J. Reprod. Immunol. 112, 102–110. doi:10.1016/j.jri.2015.09.004
Pieper, A. A., Xie, S., Capota, E., Estill, S. J., Zhong, J., Long, J. M., et al. (2010). Discovery of a Proneurogenic, Neuroprotective Chemical. Cell 142 (1), 39–51. doi:10.1016/j.cell.2010.06.018
Pinkerton, A. B., Sessions, E. H., Hershberger, P., Maloney, P. R., Peddibhotla, S., Hopf, M., et al. (2021). Optimization of a Urea-Containing Series of Nicotinamide Phosphoribosyltransferase (NAMPT) Activators. Bioorg. Med. Chem. Lett. 41, 128007. doi:10.1016/j.bmcl.2021.128007
Preiss, J., and Handler, P. (1957). Enzymatic Synthesis of Nicotinamide Mononucleotide. J. Biol. Chem. 225 (2), 759–770. doi:10.1016/s0021-9258(18)64875-6
Quijada, H., Bermudez, T., Kempf, C. L., Valera, D. G., Garcia, A. N., Camp, S. M., et al. (2021). Endothelial eNAMPT Amplifies Pre-clinical Acute Lung Injury: Efficacy of an eNAMPT-Neutralising Monoclonal Antibody. Eur. Respir. J. 57 (5). doi:10.1183/13993003.02536-2020
Rajman, L., Chwalek, K., and Sinclair, D. A. (2018). Therapeutic Potential of NAD-Boosting Molecules: The In Vivo Evidence. Cell Metab. 27 (3), 529–547. doi:10.1016/j.cmet.2018.02.011
Ramsey, K. M., Yoshino, J., Brace, C. S., Abrassart, D., Kobayashi, Y., Marcheva, B., et al. (2009). Circadian Clock Feedback Cycle through NAMPT-Mediated NAD+ Biosynthesis. Science 324 (5927), 651–654. doi:10.1126/science.1171641
Revollo, J. R., Körner, A., Mills, K. F., Satoh, A., Wang, T., Garten, A., et al. (2007). Nampt/PBEF/Visfatin Regulates Insulin Secretion in Beta Cells as a Systemic NAD Biosynthetic Enzyme. Cell Metab. 6 (5), 363–375. doi:10.1016/j.cmet.2007.09.003
Richards, R. I., Robertson, S. A., and Kastner, D. L. (2018). Neurodegenerative Diseases Have Genetic Hallmarks of Autoinflammatory Disease. Hum. Mol. Genet. 27 (R2), R108–R118. doi:10.1093/hmg/ddy139
Samal, B., Sun, Y., Stearns, G., Xie, C., Suggs, S., and Mcniece, I. (1994). Cloning and Characterization of the cDNA Encoding a Novel Human Pre-B-cell Colony-Enhancing Factor. Mol. Cell. Biol. 14 (2), 1431–1437. doi:10.1128/mcb.14.2.1431-1437.1994
Sampath, D., Zabka, T. S., Misner, D. L., O'Brien, T., and Dragovich, P. S. (2015). Inhibition of Nicotinamide Phosphoribosyltransferase (NAMPT) as a Therapeutic Strategy in Cancer. Pharmacol. Ther. 151, 16–31. doi:10.1016/j.pharmthera.2015.02.004
Schöndorf, D. C., Ivanyuk, D., Baden, P., Sanchez-Martinez, A., De Cicco, S., Yu, C., et al. (2018). The NAD+ Precursor Nicotinamide Riboside Rescues Mitochondrial Defects and Neuronal Loss in iPSC and Fly Models of Parkinson's Disease. Cell Rep. 23 (10), 2976–2988. doi:10.1016/j.celrep.2018.05.009
Sheng, B., Wang, X., Su, B., Lee, H. G., Casadesus, G., Perry, G., et al. (2012). Impaired Mitochondrial Biogenesis Contributes to Mitochondrial Dysfunction in Alzheimer's Disease. J. Neurochem. 120 (3), 419–429. doi:10.1111/j.1471-4159.2011.07581.x
Silvestro, S., Sindona, C., Bramanti, P., and Mazzon, E. (2021). A State of the Art of Antioxidant Properties of Curcuminoids in Neurodegenerative Diseases. Int. J. Mol. Sci. 22 (6), 3168. doi:10.3390/ijms22063168
Sommer, G., Garten, A., Petzold, S., Beck-Sickinger, A. G., Blüher, M., Stumvoll, M., et al. (2008). Visfatin/PBEF/Nampt: Structure, Regulation and Potential Function of a Novel Adipokine. Clin. Sci. (Lond) 115 (1), 13–23. doi:10.1042/CS20070226
Sonninen, T. M., Goldsteins, G., Laham-Karam, N., Koistinaho, J., and Lehtonen, Š. (2020). Proteostasis Disturbances and Inflammation in Neurodegenerative Diseases. Cells 9 (10). doi:10.3390/cells9102183
Stein, L. R., and Imai, S. (2014). Specific Ablation of Nampt in Adult Neural Stem Cells Recapitulates Their Functional Defects during Aging. EMBO J. 33 (12), 1321–1340. doi:10.1002/embj.201386917
Sun, B. L., Sun, X., Casanova, N., Garcia, A. N., Oita, R., Algotar, A. M., et al. (2020). Role of Secreted Extracellular Nicotinamide Phosphoribosyltransferase (eNAMPT) in Prostate Cancer Progression: Novel Biomarker and Therapeutic Target. EBioMedicine 61, 103059. doi:10.1016/j.ebiom.2020.103059
Sun, B. L., Tang, L., Sun, X., Garcia, A. N., Camp, S. M., Posadas, E., et al. (2021). A Humanized Monoclonal Antibody Targeting Extracellular Nicotinamide Phosphoribosyltransferase Prevents Aggressive Prostate Cancer Progression. Pharm. (Basel) 14 (12). doi:10.3390/ph14121322
Sun, Z., Lei, H., and Zhang, Z. (2013). Pre-B Cell Colony Enhancing Factor (PBEF), a Cytokine with Multiple Physiological Functions. Cytokine Growth Factor Rev. 24, 433–442. doi:10.1016/j.cytogfr.2013.05.006
Tagliavini, F., Giaccone, G., Frangione, B., and Bugiani, O. (1988). Preamyloid Deposits in the Cerebral Cortex of Patients with Alzheimer's Disease and Nondemented Individuals. Neurosci. Lett. 93 (2-3), 191–196. doi:10.1016/0304-3940(88)90080-8
Takeuchi, M., Niimi, T., Masumoto, M., Orita, M., Yokota, H., and Yamamoto, T. (2014). Discovery of a Novel Nicotinamide Phosphoribosyl Transferase (NAMPT) Inhibitor via In Silico Screening. Biol. Pharm. Bull. 37 (1), 31–36. doi:10.1248/bpb.b13-00495
Tofaris, G. K., and Buckley, N. J. (2018). Convergent Molecular Defects Underpin Diverse Neurodegenerative Diseases. J. Neurol. Neurosurg. Psychiatry 89 (9), 962–969. doi:10.1136/jnnp-2017-316988
Tomás-Camardiel, M., Rite, I., Herrera, A. J., de Pablos, R. M., Cano, J., Machado, A., et al. (2004). Minocycline Reduces the Lipopolysaccharide-Induced Inflammatory Reaction, Peroxynitrite-Mediated Nitration of Proteins, Disruption of the Blood-Brain Barrier, and Damage in the Nigral Dopaminergic System. Neurobiol. Dis. 16 (1), 190–201. doi:10.1016/j.nbd.2004.01.010
Travelli, C., Aprile, S., Mattoteia, D., Colombo, G., Clemente, N., Scanziani, E., et al. (2019). Identification of Potent Triazolylpyridine Nicotinamide Phosphoribosyltransferase (NAMPT) Inhibitors Bearing a 1,2,3-triazole Tail Group. Eur. J. Med. Chem. 181, 111576. doi:10.1016/j.ejmech.2019.111576
Travelli, C., Aprile, S., Rahimian, R., Grolla, A. A., Rogati, F., Bertolotti, M., et al. (2017). Identification of Novel Triazole-Based Nicotinamide Phosphoribosyltransferase (NAMPT) Inhibitors Endowed with Antiproliferative and Antiinflammatory Activity. J. Med. Chem. 60 (5), 1768–1792. doi:10.1021/acs.jmedchem.6b01392
Travelli, C., Colombo, G., Mola, S., Genazzani, A. A., and Porta, C. (2018). NAMPT: A Pleiotropic Modulator of Monocytes and Macrophages. Pharmacol. Res. 135, 25–36. doi:10.1016/j.phrs.2018.06.022
van Es, M. A., Hardiman, O., Chio, A., Al-Chalabi, A., Pasterkamp, R. J., Veldink, J. H., et al. (2017). Amyotrophic Lateral Sclerosis. Lancet 390 (10107), 2084–2098. doi:10.1016/S0140-6736(17)31287-4
Vaquer-Alicea, J., and Diamond, M. I. (2019). Propagation of Protein Aggregation in Neurodegenerative Diseases. Annu. Rev. Biochem. 88, 785–810. doi:10.1146/annurev-biochem-061516-045049
Vázquez-Rosa, E., Shin, M. K., Dhar, M., Chaubey, K., Cintrón-Pérez, C. J., Tang, X., et al. (2020). P7C3-A20 Treatment One Year after TBI in Mice Repairs the Blood-Brain Barrier, Arrests Chronic Neurodegeneration, and Restores Cognition. Proc. Natl. Acad. Sci. U. S. A. 117 (44), 27667–27675. doi:10.1073/pnas.2010430117
Verdin, E. (2015). NAD(+) in Aging, Metabolism, and Neurodegeneration. Science 350 (6265), 1208–1213. doi:10.1126/science.aac4854
Walker, F. O. (2007). Huntington's Disease. Lancet 369 (9557), 218–228. doi:10.1016/S0140-6736(07)60111-1
Wang, L., Liu, M., Zu, Y., Yao, H., Wu, C., Zhang, R., et al. (2022). Optimization of NAMPT Activators to Achieve In Vivo Neuroprotective Efficacy. Eur. J. Med. Chem. 236, 114260. doi:10.1016/j.ejmech.2022.114260
Wang, P., and Miao, C. Y. (2015). NAMPT as a Therapeutic Target against Stroke. Trends Pharmacol. Sci. 36 (12), 891–905. doi:10.1016/j.tips.2015.08.012
Wang, P., Xu, T. Y., Guan, Y. F., Tian, W. W., Viollet, B., Rui, Y. C., et al. (2011). Nicotinamide Phosphoribosyltransferase Protects against Ischemic Stroke through SIRT1-dependent Adenosine Monophosphate-Activated Kinase Pathway. Ann. Neurol. 69 (2), 360–374. doi:10.1002/ana.22236
Wang, S. N., and Miao, C. Y. (2019). Targeting NAMPT as a Therapeutic Strategy against Stroke. Stroke Vasc. Neurol. 4 (2), 83–89. doi:10.1136/svn-2018-000199
Wang, S. N., Xu, T. Y., Li, W. L., and Miao, C. Y. (2016). Targeting Nicotinamide Phosphoribosyltransferase as a Potential Therapeutic Strategy to Restore Adult Neurogenesis. CNS Neurosci. Ther. 22 (6), 431–439. doi:10.1111/cns.12539
Wang, T., Zhang, X., Bheda, P., Revollo, J. R., Imai, S., and Wolberger, C. (2006). Structure of Nampt/PBEF/visfatin, a Mammalian NAD+ Biosynthetic Enzyme. Nat. Struct. Mol. Biol. 13 (7), 661–662. doi:10.1038/nsmb1114
Wang, X., Xu, T. Y., Liu, X. Z., Zhang, S. L., Wang, P., Li, Z. Y., et al. (2015). Discovery of Novel Inhibitors and Fluorescent Probe Targeting NAMPT. Sci. Rep. 5, 12657. doi:10.1038/srep12657
Wang, Y. Y., Hung, A. C., Lo, S., and Yuan, S. F. (2021). Adipocytokines Visfatin and Resistin in Breast Cancer: Clinical Relevance, Biological Mechanisms, and Therapeutic Potential. Cancer Lett. 498, 229–239. doi:10.1016/j.canlet.2020.10.045
Watson, M., Roulston, A., Bélec, L., Billot, X., Marcellus, R., Bédard, D., et al. (2009). The Small Molecule GMX1778 Is a Potent Inhibitor of NAD+ Biosynthesis: Strategy for Enhanced Therapy in Nicotinic Acid Phosphoribosyltransferase 1-deficient Tumors. Mol. Cell. Biol. 29 (21), 5872–5888. doi:10.1128/MCB.00112-09
Weise, J., Engelhorn, T., Dörfler, A., Aker, S., Bähr, M., and Hufnagel, A. (2005). Expression Time Course and Spatial Distribution of Activated Caspase-3 after Experimental Status Epilepticus: Contribution of Delayed Neuronal Cell Death to Seizure-Induced Neuronal Injury. Neurobiol. Dis. 18 (3), 582–590. doi:10.1016/j.nbd.2004.10.025
Wilsbacher, J. L., Cheng, M., Cheng, D., Trammell, S. A. J., Shi, Y., Guo, J., et al. (2017). Discovery and Characterization of Novel Nonsubstrate and Substrate NAMPT Inhibitors. Mol. Cancer Ther. 16 (7), 1236–1245. doi:10.1158/1535-7163.MCT-16-0819
Wosikowski, K., Mattern, K., Schemainda, I., Hasmann, M., Rattel, B., and Löser, R. (2002). WK175, a Novel Antitumor Agent, Decreases the Intracellular Nicotinamide Adenine Dinucleotide Concentration and Induces the Apoptotic Cascade in Human Leukemia Cells. Cancer Res. 62 (4), 1057–1062.
Wu, Q., Yan, R., and Sun, J. (2020). Probing the Drug Delivery Strategies in Ischemic Stroke Therapy. Drug Deliv. 27 (1), 1644–1655. doi:10.1080/10717544.2020.1850918
Yang, H., Lavu, S., and Sinclair, D. A. (2006). Nampt/PBEF/Visfatin: a Regulator of Mammalian Health and Longevity? Exp. Gerontol. 41, 718–726. doi:10.1016/j.exger.2006.06.003
Yao, H., Liu, M., Wang, L., Zu, Y., Wu, C., Li, C., et al. (2022). Discovery of Small-Molecule Activators of Nicotinamide Phosphoribosyltransferase (NAMPT) and Their Preclinical Neuroprotective Activity. Cell Res. 32, 570–584. doi:10.1038/s41422-022-00651-9
Yoshida, M., Satoh, A., Lin, J. B., Mills, K. F., Sasaki, Y., Rensing, N., et al. (2019). Extracellular Vesicle-Contained eNAMPT Delays Aging and Extends Lifespan in Mice. Cell Metab. 30 (2), 329–e5. e5. doi:10.1016/j.cmet.2019.05.015
Zak, M., Liederer, B. M., Sampath, D., Yuen, P. W., Bair, K. W., Baumeister, T., et al. (2015). Identification of Nicotinamide Phosphoribosyltransferase (NAMPT) Inhibitors with No Evidence of CYP3A4 Time-dependent Inhibition and Improved Aqueous Solubility. Bioorg. Med. Chem. Lett. 25 (3), 529–541. doi:10.1016/j.bmcl.2014.12.026
Zak, M., Yuen, P. W., Liu, X., Patel, S., Sampath, D., Oeh, J., et al. (2016). Minimizing CYP2C9 Inhibition of Exposed-Pyridine NAMPT (Nicotinamide Phosphoribosyltransferase) Inhibitors. J. Med. Chem. 59 (18), 8345–8368. doi:10.1021/acs.jmedchem.6b00697
Zeng, M., Wei, T. F., Chen, C., Shen, C., Gao, T. Y., Xie, X., et al. (2021). Nicotinamide Phosphoribosyltransferase Inhibitor Ameliorates Mouse Aging-Induced Cognitive Impairment. J. Cereb. Blood Flow. Metab. 41 (10), 2510–2523. doi:10.1177/0271678X211006291
Zhang, H., Zhang, N., Liu, Y., Su, P., Liang, Y., Li, Y., et al. (2019a). Epigenetic Regulation of NAMPT by NAMPT-AS Drives Metastatic Progression in Triple-Negative Breast Cancer. Cancer Res. 79 (13), 3347–3359. doi:10.1158/0008-5472.CAN-18-3418
Zhang, K., Ni, Y., Chen, J., Tu, Z., Wu, X., Chen, D., et al. (2019b). Discovery of Trans-3-(pyridin-3-yl)acrylamide-derived Sulfamides as Potent Nicotinamide Phosphoribosyltransferase (NAMPT) Inhibitors for the Potential Treatment of Cancer. Bioorg. Med. Chem. Lett. 29 (12), 1502–1506. doi:10.1016/j.bmcl.2019.04.013
Zhang, Q., Chen, W., Tan, S., and Lin, T. (2017). Stem Cells for Modeling and Therapy of Parkinson's Disease. Hum. Gene Ther. 28 (1), 85–98. doi:10.1089/hum.2016.116
Zhao, G., Green, C. F., Hui, Y. H., Prieto, L., Shepard, R., Dong, S., et al. (2017). Discovery of a Highly Selective NAMPT Inhibitor that Demonstrates Robust Efficacy and Improved Retinal Toxicity with Nicotinic Acid Coadministration. Mol. Cancer Ther. 16 (12), 2677–2688. doi:10.1158/1535-7163.MCT-16-0674
Zheng, X., Bair, K. W., Bauer, P., Baumeister, T., Bowman, K. K., Buckmelter, A. J., et al. (2013a). Identification of Amides Derived from 1H-Pyrazolo[3,4-B]pyridine-5-Carboxylic Acid as Potent Inhibitors of Human Nicotinamide Phosphoribosyltransferase (NAMPT). Bioorg. Med. Chem. Lett. 23 (20), 5488–5497. doi:10.1016/j.bmcl.2013.08.074
Zheng, X., Bauer, P., Baumeister, T., Buckmelter, A. J., Caligiuri, M., Clodfelter, K. H., et al. (2013b). Structure-based Discovery of Novel Amide-Containing Nicotinamide Phosphoribosyltransferase (Nampt) Inhibitors. J. Med. Chem. 56 (16), 6413–6433. doi:10.1021/jm4008664
Zheng, X., Bauer, P., Baumeister, T., Buckmelter, A. J., Caligiuri, M., Clodfelter, K. H., et al. (2013c). Structure-based Identification of Ureas as Novel Nicotinamide Phosphoribosyltransferase (Nampt) Inhibitors. J. Med. Chem. 56 (12), 4921–4937. doi:10.1021/jm400186h
Zheng, X., Baumeister, T., Buckmelter, A. J., Caligiuri, M., Clodfelter, K. H., Han, B., et al. (2014). Discovery of Potent and Efficacious Cyanoguanidine-Containing Nicotinamide Phosphoribosyltransferase (Nampt) Inhibitors. Bioorg. Med. Chem. Lett. 24 (1), 337–343. doi:10.1016/j.bmcl.2013.11.006
Glossary
5-HT 5-Hydroxytryptamine
5-HT2A Serotonin 2A
5-HT2C Serotonin 2C
Aβ Amyloid β-protein
AADC Aromatic amino acid decarboxylase
AD Alzheimer’s disease
ADCs Antibody–drug conjugates
ALS Amyotrophic lateral sclerosis
BMAL1 Aryl hydrocarbon receptor translocator-like protein 1
CAG Canadian Association of Gastroenterology
CLOCK Circadian locomoter output cycles protein kaput
COMT Catechol-O-methyltransferase
DAT Dopamine transporter
DR Dopamine receptor
eNAMPT Extracellular nicotinamide phosphoribosyltransferase
FDA Food and Drug Administration
GLUT Glucose transporter
HD Huntington’s disease
HDAC Histone deacetylase
hSOD 1 Human superoxide dismutase 1
IL Interleukin
iNAMPT Intracellular nicotinamide phosphoribosyltransferase
IP Immune proteasome
iPSC Induced pluripotent stem cell
LPS Lipopolysaccharide
mAb Monoclonal antibody
mAChR Muscarinic acetylcholine receptor
MAO-B Monoamine oxidase B
mHTT Mutant Huntingtin
NAD Nicotinamide adenine dinucleotide
NAM Nicotinamide
NAMPT Nicotinamide phosphoribosyltransferase
NAPRTase Nicotinic acid phosphoribosyltransferase
NMDA N-methyl-D-aspartic acid
NMN Nicotinamide mononucleotide
NR Nicotinamide nucleoside
NRF Nuclear respiratory factor
NRK1 Nicotinamide nucleoside kinase 1
NSCs Neural stem cells
NSPCs Neural stem/progenitor cells
OGD Oxygen–glucose-deprivation
pAb Polyclonal antibody
PACT Photo activated chemotherapy
PAK4 p21-activated kinase
PARP Poly(ADP-ribose) polymerase
PBEF Pre-B-cell colony-enhancing factor
PC-3 Human prostate cancer
PCa Prostate cancer
PD Parkinson’s disease
PRPP 5′-Phosphoribosyl-1-pyrophosphate
QAPRTase Quinolinic acid phosphoribosyltransferase
ROS Reactive oxygen species
SIRT Sirtuin
SPR Surface plasmon resonance
SVZ Subventricular zone
TDI Time-dependent inhibition
TFAM Mitochondrial transcription factor A
TLR4 Toll-like receptor 4
TNF Tumour necrosis factor.
Keywords: nicotinamide phosphoribosyltransferase, nicotinamide adenine dinucleotide, neurodegenerative diseases, inhibitors, agonists
Citation: Zhu Y, Xu P, Huang X, Shuai W, Liu L, Zhang S, Zhao R, Hu X and Wang G (2022) From Rate-Limiting Enzyme to Therapeutic Target: The Promise of NAMPT in Neurodegenerative Diseases. Front. Pharmacol. 13:920113. doi: 10.3389/fphar.2022.920113
Received: 19 April 2022; Accepted: 08 June 2022;
Published: 12 July 2022.
Edited by:
Armando A Genazzani, University of Eastern Piedmont, ItalyReviewed by:
Ambra A Grolla, University of Eastern Piedmont, ItalyJulia Skokowa, University of Tübingen, Germany
Copyright © 2022 Zhu, Xu, Huang, Shuai, Liu, Zhang, Zhao, Hu and Wang. This is an open-access article distributed under the terms of the Creative Commons Attribution License (CC BY). The use, distribution or reproduction in other forums is permitted, provided the original author(s) and the copyright owner(s) are credited and that the original publication in this journal is cited, in accordance with accepted academic practice. No use, distribution or reproduction is permitted which does not comply with these terms.
*Correspondence: Rui Zhao, emhhb3J1aTAxMjJAMTI2LmNvbQ==; Xiuying Hu, aHV4aXV5aW5nQHNjdS5lZHUuY24=; Guan Wang, Z3VhbjgwNzlAMTYzLmNvbQ==
†These authors have contributed equally to this work and share first authorship