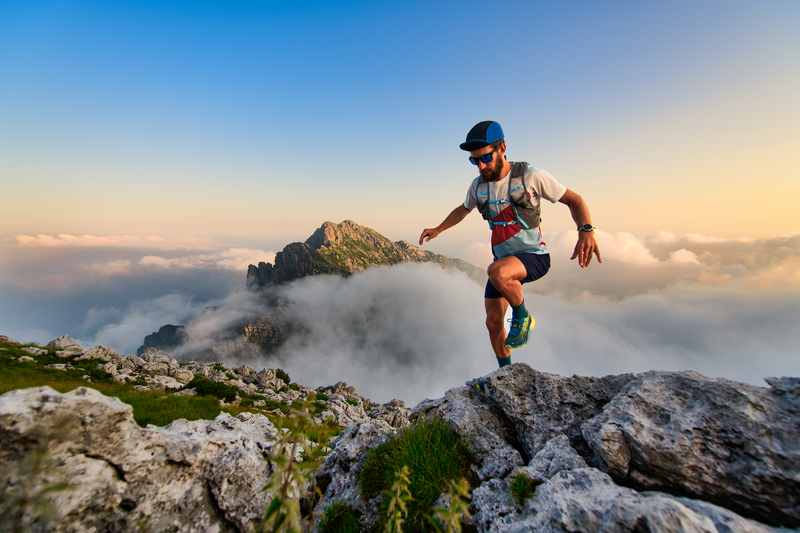
95% of researchers rate our articles as excellent or good
Learn more about the work of our research integrity team to safeguard the quality of each article we publish.
Find out more
ORIGINAL RESEARCH article
Front. Pharmacol. , 23 August 2022
Sec. Ethnopharmacology
Volume 13 - 2022 | https://doi.org/10.3389/fphar.2022.911667
This article is part of the Research Topic Treatment of Infectious Diseases with Bioactive Compounds from Medicinal Plants: Their Mechanisms and Applications View all 11 articles
Dysbiosis of respiratory microbiota is closely related to the pathophysiological processes of asthma, including airway inflammation. Previous studies have shown that Qingfei oral liquid (QF) can alleviate airway inflammation and airway hyper-responsiveness in respiratory syncytial virus-infected asthmatic mice, but its effect on the respiratory microbiota is unknown. We therefore aimed to observe the effects of QF on airway inflammation and respiratory microbiota in ovalbumin (OVA)-induced asthmatic mice. We also explored the potential mechanism of QF in reducing airway inflammation by regulating respiratory microbiota. Hematoxylin and eosin as well as periodic acid-Schiff staining were performed to observe the effects of QF on lung pathology in asthmatic mice. Cytokine levels in bronchoalveolar lavage fluid (BALF) specimens were also measured. Changes in respiratory microbiota were analyzed using 16S rRNA gene sequencing, followed by taxonomical analysis. In order to verify the metagenomic function prediction results, the expression of key proteins related to the MAPK and NOD-like receptor signaling pathways in the lung tissues were detected by immunohistochemistry. The current study found that QF had a significant anti-inflammatory effect in the airways of asthmatic mice. This is mainly attributed to a reduction in lung pathology changes and regulating cytokine levels in BALF. Analysis of the respiratory microbiota in asthmatic mice showed that the abundance of Proteobacteria at the phylum level and Pseudomonas at the genus level increased significantly and QF could significantly regulate the dysbiosis of respiratory microbiota in asthmatic mice. Metagenomic functional prediction showed that QF can downregulate the MAPK and Nod-like receptor signaling pathways. Immunohistochemical results showed that QF could downregulate the expression of p-JNK, p-P38, NLRP3, Caspase-1, and IL-1β, which are all key proteins in the signaling pathway of lung tissue. Our study therefore concluded that QF may reduce airway inflammation in asthmatic mice by regulating respiratory microbiota, and to the possibly downregulate MAPK and Nod-like receptor signaling pathways as its underlying mechanism.
Bronchial asthma is a heterogeneous disease characterized by chronic airway inflammation and airway hyper-responsiveness. The major symptoms of asthma, ranging from mild symptoms to life-threatening attacks, include coughing, wheezing, shortness of breath, and chest tightness (Licari et al., 2018). Although asthma can occur at any age, it is particularly common in children. Epidemiological investigations have shown that the global incidence of asthma in children has increased from 11.1% to 13.2% over the last 10 years (Asher et al., 2020). At present, there are still a number of children worldwide with asthma that is not effectively controlled for various reasons (Murphy et al., 2020).
Airway inflammation is considered the most important pathophysiological process in asthma. T helper (Th) lymphocytes play an important role in airway inflammation (Tumes et al., 2017). Previous studies have shown that asthma can be divided into at least two distinct molecular phenotypes based on the degree of Th2 inflammation, which have been described as Th2-high and Th2-low (Robinson et al., 2017). Th2 cells produce interleukin (IL)-4, IL-13, and IL-5, eventually leading to the accumulation of eosinophils in the lungs (Finkelman et al., 2010). In recent years, studies have shown that severely asthmatic patients with a Th2-low phenotype have been noted to display predominantly neutrophilic inflammation of their airways (Ramakrishnan et al., 2019). Moreover, Th17 cells and their cytokines (IL-17 and IL-6, among others) are implicated in the development of neutrophilic inflammation. However, CD4+CD25+ regulatory T cells and their cytokines (IL-10 and TGF-β, among others) were significantly reduced in patients with asthma (Gandhi et al., 2020).
Following the development of high-throughput sequencing technology, the relationship between changes in the human microbiota and asthma has attracted increasing attention. The respiratory microbiota, which exhibits the mucosal surface of the respiratory tract, is a direct participant in the local mucosal immunity of the respiratory system and is closely related to the occurrence and development of asthma (Budden et al., 2019). Our recent study also found that dynamic changes in the respiratory microbiota are closely associated with the progression of chronic asthma, including airway inflammation and airway remodeling (Zheng et al., 2021). Huang et al. (2015) showed that an increase in Proteobacteria abundance in the respiratory tract can promote neutrophil aggregation, which may affect the patient’s response to corticosteroid therapy. A study of the composition of nasal microbiota showed that the increased abundance of Streptococcus, Haemophilus, and Moraxella was an important risk factor for predicting wheezing in preschoolers younger than 5 years, which persisted until a school-going age (Teo et al., 2018). In a mouse model study, Prevotella was found to significantly reduce neutrophil aggregation in the lung and production of Toll-like receptor (TLR) 2-mediated pro-inflammatory cytokines, thereby reducing lung inflammation, compared with Haemophilus influenzae (Larsen et al., 2015). In addition, some respiratory microbes can also play a role in reducing lung inflammation by producing metabolites (such as short-chain fatty acids) (Segal et al., 2017a; Segal et al., 2017b). These findings indicate that alterations in respiratory microbiota can affect the phenotype and severity of airway inflammation in patients with asthma by driving local mucosal immune responses. Furthermore, it is probable that the regulation of dysbiosis of respiratory microbiota can improve airway inflammation in asthma to some extent.
In recent years, an increasing number of studies have found that Traditional Chinese Medicine (TCM) compounds play a role in asthma prevention and treatment (Tsang et al., 2018; Cui et al., 2020). Qingfei oral liquid (QF), founded by Wang Shouchuan, a famous professor at Nanjing University of Chinese Medicine in China, has been used for the prevention and treatment of asthma in children for decades, and has achieved satisfactory results. It contains TCM concoction of Ephedra sinica Stapf [Ephedraceae], Prunus armeniaca var. Armeniaca [Rosaceae], and Scutellaria baicalensis Georgi [Lamiaceae], among others. A previous study by our team found that QF can reduce airway inflammation and airway hyper-responsiveness in respiratory syncytial virus (RSV)-infected asthmatic mice (Jing et al., 2020; Yu et al., 2021), but its effect on respiratory microbiota has not been observed. In view of this, the aim of the current study was to investigate the anti-inflammatory activities of QF and its effect on respiratory microbiota in mice with ovalbumin (OVA)-induced asthma and to explore the possible mechanism of QF in alleviating airway inflammation by regulating respiratory microbiota. The purpose of this study was to provide new knowledge for the prevention and treatment of asthma and provide a theoretical basis for the effective intervention of QF.
We obtained OVA from Sigma-Aldrich Chemical Co. (grade V; St. Louis, MO, United States). Mouse IL-4, IL-6, IL-10, and IL-17A ELISA kits and Imject Alum were purchased from Thermo Fisher Scientific (Rockford, IL, United States). Mouse total IgE and OVA-specific IgE ELISA kits were purchased from BioLegend (San Diego, CA, United States). Antibodies against NLRP3, Caspase-1, and IL-1β were obtained from Abcam Co. (Cambridge, MA, United States). Antibodies against p-P38, and p-JNK were obtained from CST Co. (Boston, MA, United States).
We obtained QF from the Chinese Pharmacy of Longhua Hospital, Shanghai University of Traditional Chinese Medicine. The standard dose of QF was 91 g (weight of total herb mixtures) and the composition of it was presented in Table 1. The extraction and quality control of QF were performed by the Department of Pharmacology at the Shanghai University of Traditional Chinese Medicine and Shanghai Fudan Fuda Science & Technology Co., Ltd. (Shanghai, China).
Samples were analyzed by suspending 100 mg of each compound in 10 ml of 50% methanol aqueous solution in a 15 ml centrifugal tube. The tube was then sonicated for 30 min, whereafter 1 ml of supernatant was centrifuged at 14,000 rpm for 5 min. Following centrifugation, the supernatant was filtered through a 0.22 µm microporous membrane, and placed into sample vials for UHPLC-MS/MS analysis. Control samples were processed under the same conditions.
The LC conditions were as follows. Column: ACQUITY UPLC HSS T3 column (2.1 × 100 mm, 1.8 μm); Column temperature: 35°C; Injection volume: 10 μl; Flow rate: 0.3 ml/min; Mobile Phase A: (deionized water with 0.1% formic acid); Phase B (acetonitrile with 0.1% formic acid). Specific gradient elution conditions were as follows: 0 min, 0% B; 10 min, 30% B; 25 min, 40% B; 30 min, 50% B; 40 min, 70% B; 45 min, 100% B; 60 min, 100% B; 60.5 min, 0% B and 70 min, 0% B.
The MS conditions were as follows. Q Exactive Orbitrap high resolution mass spectrometry was used to analyze sample. The detection mode was full MS-DDMS2, and positive and negative ion modes were scanned. Acquisition range: 100–1,200 Da, MS1 resolution: 70,000, MS2 resolution: 17,500, capillary voltage: ± 3.2 kV, capillary temp: 320°C, Aux gas heater temp: 350°C, sheath gas flow rate: 40 L/min, auxiliary gas flow rate: 15 L/min, AGC target: 1e6, TopN: 5, Full MS-ddMS2 NCE: 30, 40, 50.
Female BALB/c mice (n = 36) aged 4–6 weeks and weighing 17.5–20.5 g were obtained from Shanghai Sippr-BK Laboratory Animal Co., Ltd. (Shanghai, China). All mice were maintained in a specific-pathogen-free grade animal room under controlled conditions with a 12 h light/dark cycle at a temperature of 24 ± 2°C with a relative appropriate humidity. Animal experiments were conducted in accordance with institutional guidelines for animal research. The Center for Laboratory Animals, Shanghai Children’s Hospital, School of Medicine, Shanghai Jiao Tong University, Shanghai, China authorized this experimental protocol (approval no. 2017Y003).
A mouse model of OVA-induced asthma was constructed as previously described, with slight modifications (Temelkovski et al., 1998). First, 36 mice were divided into two groups: control (n = 6) and OVA (n = 30). Mice in the OVA group were sensitized by intraperitoneal injection of 200 µl of a solution composed of 20 µg OVA dissolved in 100 µl sterile saline and 100 µl Imject Alum (containing 4 mg aluminum hydroxide (net weight)) on days 0, 7, and 14. Then, 30 mice from the OVA group were randomly divided into five groups: OVA group (no treatment, n = 6), OVA + QFH group (high-dose QF, n = 6), OVA + QFM group (medium-dose QF, n = 6), OVA + QFL group (low-dose QF, n = 6), and OVA + BUD group (budesonide aerosol inhalation, n = 6). For the OVA challenge, the mice were exposed to OVA aerosol (2.5% w/v) OVA solution in sterile saline administered using a PARI PRONEB Ultra compressor (Pari Proneb, Midlothian, WA, United States) for 30 min on days 21–24 and 28–31. The mice in the OVA + QFH group, the OVA + QFM group and the OVA + QFL group were given 1.82 g/d, 0.91 g/d and 0.455 g/d QF (Herbs dry weight), respectively by intragastric administration 30 min before OVA challenge. One hour prior to the OVA challenge, mice in the OVA + BUD group were nebulized with budesonide (1 mg budesonide in 3 ml normal saline) for 30 min. Mice in the OVA group (no treatment) were administered an equivalent volume of distilled water intragastrical, followed by nebulization with normal saline for 30 min before OVA challenge. Mice in the control group were injected intraperitoneally with saline and an Imject Alum emulsion and then exposed to an aerosol of sterile saline without OVA, according to the same schedule. The mice were sacrificed within 24 h of final nebulization. After the mice were euthanized, nasal lavage fluid (NLF), bronchoalveolar lavage fluid (BALF), and left lower lung tissue were collected and preserved.
First, we ligated the left lower lung and removed it after complete bronchoalveolar lavage. The lungs were harvested and infused with 4% paraformaldehyde for 24 h. Sections (4 μm-thick) were embedded in paraffin and then subjected to hematoxylin and eosin (H&E) and periodic acid-Schiff (PAS) staining to evaluate airway inflammation and mucus production in the lung tissues. Pathological changes were observed under a light microscope at ×200 magnification. To grade the extent of lung inflammation and goblet cell hyperplasia, a semiquantitative scoring system was used as previously described (Kujur et al., 2015; Kubo et al., 2019).
To observe the expression levels of related proteins in lung tissues, ligated left lung tissues were fixed with 4% paraformaldehyde and embedded in paraffin. Sections (4 μm-thick) were deparaffinized and rehydrated. The sections were pretreated with 0.3% H2O2 in methanol for 15 min to quench the endogenous peroxide activity and boiled at 100°C for 20 min in a 10% citrate buffer to unmask the antigens. Sections were incubated in primary antibodies (anti-NLRP3 1:200, anti-Caspase-1 1:1,000, and anti-IL-1β 1:500, anti-p-P38 1:200, and anti-p-JNK 1:100) at 4°C overnight and stained with HRP-labelled anti-rabbit IgG (1:1,000). After washing, DAB substrate was applied to the sections. Representative images of each slide were acquired at ×200 magnification for morphometric and comparative analysis.
After euthanasia of the mice by cervical dislocation, the lungs were washed four times with sterile saline, using endotracheal intubation to the lower respiratory tract (LRT) (0.5 ml per round) to obtain samples for BALF. Then, a sterile leather hose was reinserted to rinse the nasal cavity with normal saline (0.5 ml per round) for 3–4 times and to collect the NLF. Both BALF and NLF were filtered once with a 0.22 µm filter. The filtered BALF was centrifuged at 1,000 g for 15 min, and the supernatants were collected for cytokine detection. A filter membrane was used for DNA extraction. All specimens were stored at −80°C.
All BALF specimens were collected as described in Section 2.5. The levels of total IgE and OVA-specific IgE, IL-4, IL-6, IL-17A, and IL-10 in BALF were measured using ELISA kits according to the manufacturer’s instructions.
To explore the effect of QF on the respiratory microbiota of asthmatic mice, 16S rRNA gene sequencing was performed using NLF and BALF specimens from the control, OVA, OVA + QFM, and OVA + BUD groups. Total genomic DNA was extracted using the OMEGA Soil DNA Kit (Omega Bio-Tek, Norcross, GA, United States), according to the manufacturer’s instructions, and stored at −80°C until needed for analysis. The forward primer 338F (5-ACTCCTACGGGAGGCAGCA-3) and reverse primer 806R (5-GGACTACHVGGGTWTCTAAT-3) were used to amplify the V3–V4 region of the bacterial 16S rRNA genes. After the individual quantification step, the amplicons were pooled in equal quantities and paired-end sequencing (2 × 300 bp) was performed using Illumina MiSeq (Illumina, San Diego, CA, United States) at Shanghai Personal Biotechnology Co., Ltd. (Shanghai, China). The procedures for DNA extraction, PCR amplification, and sequencing were based on previous studies[10] of our team.
Microbiome bioinformatic analysis was performed using QIIME 2 2020.11 with minor modifications (Hall and Beiko, 2018). Briefly, raw sequence data were demultiplexed using the demux plugin, followed by primer cutting using the Cutadapt plugin. The sequences were subjected to quality filtering, denoising, and merging, and chimeras were removed using the DADA2 plugin (Callahan et al., 2016). Non-singleton amplicon sequence variants were used to construct a phylogeny using FastTree (Price et al., 2009). We used the q2-diversity plugin to compute different α diversity metrics using Pielou’s evenness indices and β diversity metrics using weighted UniFrac distance matrices. Principal coordinates analysis (PCoA) with weighted UniFrac distance matrices was used to study community composition. Taxonomy was assigned using a naive Bayes classifier pre-trained on the Greengenes 13_8_99% OTUs 16S rRNA gene full-length sequences and the q2-feature-classifier plugin (Bokulich et al., 2018). The bar chart of the composition of respiratory microbiota was completed using Wekemo Bioincloud (https://www.bioincloud.tech). Linear discriminant analysis effect size (LEfSe) (Segata et al., 2011) was used to detect differentially abundant taxa across groups using default parameters. The PICRUSt software package (Langille et al., 2013) was used for metagenomic functional prediction analysis. Kyoto Encyclopedia of Genes and Genomes (KEGG) pathways (Kanehisa et al., 2004) were used to identify metagenomic contents. LEfSe and PICRUSt analyses were performed using resources available at http://huttenhower.sph.harvard.edu/galaxy/. The STAMP software (Parks et al., 2014) was used to analyze the predicted metagenome and identify pathways associated with airway inflammation.
The expression levels of cytokines and related proteins were compared by analysis of variance using GraphPad Prism 8.0, and SPSS 24.0. The differences between the four groups were tested using one-way analysis of variance (ANOVA), and the variance between the two groups was compared using an Tukey’s multiple comparisons test. The Kruskal–Wallis test was used to estimate intergroup differences in α diversity metrics, β diversity metrics, and LEfSe analysis. The Wilcoxon test was used to compare subclasses. The predicted metagenome was analyzed using White’s non-parametric t-test for comparisons between the two groups. Statistical tests used in the study were two-sided, and a p value ≤0.05 was considered to indicate statistical significance.
The QF extract was analyzed using UHPLC-MS/MS. The TIC in the positive and negative ion modes of the QF extract are shown in Figures 1A,B, respectively. Analysis of the top 20 compounds sorted by MS response values. The source of the active QF component was based on the Chinese Pharmacopoeia 2015 edition, the literature search was based on PubMed, and the compound data search was based on PubChem, Chemical Book, and SciFinder databases. The information on the top 20 compounds is presented in Table 2. The TIC of the blank samples are shown in Supplementary Figure S1.
FIGURE 1. Total ion chromatography (TIC) of the extract of Qingfei oral liquid (QF). (A) TIC of the extract of QF in positive ion mode; (B) Total ion chromatography of the extract of QF in negative ion mode.
To investigate the effect of QF on airway inflammation in OVA-induced asthmatic mice, lungs were stained with H&E and PAS. Compared to control mice, OVA-challenged mice showed obvious inflammatory cell infiltration around the small airways, bronchial wall thickening, and constriction (Figures 2A,B). Treatment of OVA-challenged mice with QF and budesonide significantly alleviated airway inflammation. In the budesonide and QFM group, the number of inflammatory cells infiltrating the airway was significantly lower than in the OVA group, as was the airway wall thickness and the inflammation score. For OVA-challenged mice treated with QF, medium-dose QF had the best effect (Figures 2A,B). PAS staining showed that the number of mucus-secreting goblet cells increased in OVA-challenged mice compared to that in control mice (Figures 2A,C). The budesonide and QF groups significantly reduced airway mucus secretion when compared to the OVA group at different doses, but there was no significant difference in the PAS score between the treatment groups. We can deduce that QF and budesonide alleviated these pathological changes. These results showed that QF can significantly alleviate airway inflammation in the lung pathology of asthmatic mice.
FIGURE 2. Effect of Qingfei oral liquid (QF) on pulmonary pathology in ovalbumin (OVA)-induced asthmatic mice. (A) Representative hematoxylin and eosin (H&E) staining were performed to observe airway inflammation in asthmatic mice (200×; the black arrows indicate the aggregation of inflammatory cells, and the black vertical line indicates the airway wall thickness). Periodic acid-Schiff (PAS) staining indicating the mucus-producing goblet cells around the small airways (200×; similar to the purple area indicated by the black arrow). (B) Inflammation scores are based on H&E staining. (C) The PAS scores indicating the mucus-producing goblet cells around the small airways. Data are expressed as mean ± standard deviation (SD) (one-way ANOVA followed by Tukey’s test). n = 6, △p ≤ 0.05 vs. the OVA group, △△p ≤ 0.01 vs. the OVA group; #p ≤ 0.05 vs. the budesonide (BUD) group, ##p ≤ 0.01 vs. the BUD group, ns: no difference.
Total IgE, OVA-specific IgE, IL-4, IL-6, IL-17A, and IL-10 levels in BALF were measured using ELISA (Figure 3). Compared with the control group, the OVA group had significantly higher levels of total IgE (Figure 3A), OVA-specific IgE (Figure 3B), IL-4 (Figure 3C), IL-6 (Figure 3D), and IL-17A (Figure 3E), but lower levels of IL-10 (Figure 3F). Similar to the OVA-challenged mice treated with budesonide, the levels of total IgE, OVA-specific IgE, IL-4, IL-6, and IL-17A in the BALF of OVA-challenged mice treated with QF were significantly decreased (Figures 3A–E). Both QF and budesonide-treated mice showed increased levels of IL-10 to some extent (Figure 2F). In addition, QF was less effective than budesonide at reducing the levels of total IgE, OVA-specific IgE, and IL-4 (p < 0.05). QFM showed no significant difference in reducing IL-6 levels or increasing IL-10 levels when compared to budesonide (p > 0.05), but significantly improved in reducing IL-17A levels (p < 0.05). The effect of QF on reducing IL-6, IL-4, and IL-17A levels was especially significant in mice treated with intermediate doses of QF. These results suggest that QF regulates cytokine levels in the BALF of OVA-induced asthmatic mice.
FIGURE 3. Effects of Qingfei oral liquid (QF) on IgE and inflammatory cytokines in bronchoalveolar lavage fluid (BALF) of asthmatic mice. BALF samples from different groups were collected to detect the levels of (A) total IgE, (B) Ovalbumin (OVA)-specific IgE, (C) IL-4, (D) IL-6, (E) IL-17A, and (F) IL-10 using ELISA. Data are expressed as mean ± standard deviation (SD) and were performed with one-way ANOVA followed by Tukey’s tests. n = 6, △p ≤ 0.05 vs. the OVA group, △△p ≤ 0.01 vs. the OVA group; #p ≤ 0.05 vs. the budesonide (BUD) group, ##p ≤ 0.01 vs. the BUD group; ◆p ≤ 0.05 vs. the QFM group, ◆◆p ≤ 0.01 vs. the QFM group; ns: no difference; line over bars indicates that all groups are include.
Since the mid-dose QF had the best anti-airway inflammation effect, this study further compared the differences in respiratory microbiota among the control, OVA, OVA + QFM (QFM), and OVA + BUD (BUD) groups. The shape of the alpha rarefaction curve indicated that the sequencing depth was sufficient (Figures 4A,B). The α diversity represents the diversity of microbial groups in the model, and the β diversity analysis indicated the microbial diversity in different groups of mice. The upper respiratory tract (URT) microbiota (NLF samples) of the OVA-induced mice had a significantly higher α diversity (p < 0.05) than that of the control mice, as determined using Pielou’s evenness (Figure 4C). The URT microbiota of OVA-challenged mice treated with QF or budesonide did not differ significantly from that of the untreated OVA-challenged mice. However, there was no significant difference in the Pielou’s evenness indices of the LRT microbiota (BALF samples) among the control, OVA, QFM, and BUD groups (p > 0.05) (Figure 4D).
FIGURE 4. Effects of Qingfei oral liquid (QF) on the diversity of the respiratory microbiota in ovalbumin (OVA)-induced asthmatic mice. (A) The alpha rarefaction curve in nasal lavage fluid (NLF) microbiota. (B) The alpha rarefaction curve in bronchoalveolar lavage fluid (BALF) microbiota. (C) α diversity analysis (using the Pielou’s evenness) of the NLF microbiota. (D) α diversity analysis (using the Pielou’s evenness) of the BALF microbiota. (E) PCoA plot showing the β diversity of NLF microbiota (p = 0.001). (F) PCoA plot showing the β diversity of BALF microbiota (p = 0.001). PCoA of all samples using weighted UniFrac distance. PCoA, principal coordinates analysis. (n = 5 in each group).
The weighted UniFrac distance was used to evaluate β-diversity among the different groups. The scatter plot based on the PCoA scores indicated a clear separation of community composition between the control and OVA groups. PCoA showed that the β-diversity of the respiratory microbiota of OVA-induced mice treated with QF was significantly different from that of untreated OVA-challenged mice (Figures 4E,F). These results indicate that QF can affect the diversity of the respiratory microbiota in OVA-induced asthmatic mice.
To understand the effect of QF on the composition of the respiratory microbiota in OVA-induced asthmatic mice, we analyzed the microbial composition at the phylum and genus levels. At the phylum level, Actinobacteria was predominant in the control group, whereas Proteobacteria was the most abundant phylum in the OVA group (in both URT and LRT samples). Fortunately, Proteobacteria were significantly reduced in OVA-induced asthmatic mice treated with QF and budesonide, and Actinobacteria were significantly increased in OVA-induced asthmatic mice treated with QF (Figures 5A,B).
FIGURE 5. Effects of Qingfei oral liquid (QF) on the composition of respiratory microbiota in ovalbumin (OVA)-induced asthmatic mice. (A) The composition of nasal lavage fluid (NLF) microbiota at the phylum level. (B) The composition of bronchoalveolar lavage fluid (BALF) microbiota at the phylum level. (C) The composition of NLF microbiota at the genus level. (D) The composition of the BALF microbiota at the genus level. (n = 5 in each group; only the top 10 legends with high abundance are shown).
At the genus level, Micrococcaceae was most abundant in the control group, whereas Pseudomonas and Cupriavidus were more abundant in the OVA group (Figures 5C,D). In asthmatic mice treated with QF and budesonide, the proportion of Pseudomonas decreased significantly. Cupriavidus decreased significantly in asthmatic mice treated with budesonide, but there was no significant difference between QF and untreated asthmatic mice.
To further identify the effect of QF on the composition of the microbiota in OVA-induced asthmatic mice, we analyzed the different abundances of bacterial communities using LEfSe (Figure 6). Analysis of microbial composition at the genus level using LEfSe revealed that among the URT and LRT microbiota, Pseudomonas, Cupriavidus and many other genera from Proteobacteria were present in the OVA group, Bacilli from Firmicutes in the QFM group, and Bacillus from Firmicutes in the BUD group. These results indicate that QF can regulate the composition of respiratory microbiota in asthmatic mice to some extent.
FIGURE 6. Different abundances of bacterial communities in the respiratory samples, as indicated in LEfSe analysis. The differences are indicated by the color of over-represented taxa: green indicating ovalbumin (OVA)-induced mice, blue indicating Qingfei oral liquid medium concentration (QFM) treated mice, and red indicating budesonide (BUD) treated mice. (A) Different abundances of bacterial communities in the upper respiratory tract (URT) (nasal lavage fluid (NLF) samples) with LDA scores >2.5. (B) Different abundances of bacterial communities in the lower respiratory tract (LRT) (bronchoalveolar lavage fluid (BALF) samples) with LDA scores >3.0. The circles represent phylogenetic levels from phylum (innermost circle) to genera (outermost circle). n = 5 in each group; adjusted p values ≤0.05.
To understand whether alterations in the respiratory microbiota contribute to airway inflammation in asthmatic mice, we performed bacterial metagenomic function prediction analyses using the PICRUSt program. We explored the differences in the bacterial metagenomic functional prediction among the different groups at KEGG Level 3 (Figure 7).
FIGURE 7. Effects of Qingfei oral liquid (QF) on metagenomic functional prediction of the respiratory microbiota in ovalbumin (OVA)-induced asthmatic mice. Bacterial metagenomic functional categories were derived from Level 3 KEGG pathways. (A) Control group vs. the OVA group for the nasal lavage fluid (NLF) microbiota. (B) Control group vs. the OVA group in bronchoalveolar lavage fluid (BALF) microbiota. (C) BALF microbiota of the OVA group vs. the QF medium (QFM) concentration group. Gene functions with significant differences (corrected p-value ≤ 0.05, White’s non-parametric t test in STAMP) and parts of the pathways associated with asthma are shown.
In both URT and LRT samples, the MAPK signaling pathway-yeast, NOD-like receptor signaling pathway, antigen processing and presentation, bacterial secretion system, and replication, recombination, and repair proteins were significantly upregulated in the OVA group compared to the control group (Figures 7A,B). Among these pathways, MAPK signaling pathway-yeast and NOD-like receptor signaling pathway have been shown to be closely associated with airway inflammation. In LRT samples, MAPK signaling pathway-yeast and Nod-like receptor signaling pathway were significantly downregulated in QF treated asthmatic mice, but no similar changes were found in URT samples (Figure 7C). Therefore, we speculated that the underlying mechanism of QF to improve airway inflammation in OVA-induced asthmatic mice by regulating the respiratory microbiota may be related to the down-regulation of MAPK signaling pathway-yeast and NOD-like receptor signaling pathway.
Metagenomic functional prediction showed that QF could downregulate the MAPK and Nod-like receptor signaling pathways related to airway inflammation. MAPK is an important transmitter of signals from the cell surface to the nucleus. Three distinct MAPK pathways have been described: p38 MAPK pathway, c-Jun amino-terminal kinase (JNK) pathway, and extracellular signal-regulated kinases (ERKs or p42/44 MAPK pathway). The JNK and p38 MAPK pathways, known as stress-activated protein kinases, respond to inflammatory and environmental physical insults, whereas the ERK pathway is activated by mitogenic and proliferative stimuli (Khorasanizadeh et al., 2017; Manley et al., 2019). The Nod-like receptor family pyrin domain-containing protein 3 (NLRP3) inflammasome is an important component of innate immunity that can recognize intracellular pathogens or injury-related molecular patterns and mediate the processing, maturation, and release of a variety of cytokines, including Il-1β, are involved in the regulation of the occurrence and development of various inflammatory diseases. The NLRP3 inflammasome is mainly composed of NLRP3, apoptosis-associated speck-like protein containing CARD (ASC) and caspase-1 (Gritsenko et al., 2020; Louvrier et al., 2020).
Therefore, the expression levels of p-P38, p-JNK and NLRP3, Caspase-1, IL-1β in the lung tissues were measured using Immunohistochemistry to confirm the prediction results (Figure 8). Compared with the control mice, the expression levels of p-P38 and p-JNK were significantly increased in the lung tissues of OVA-induced asthmatic mice but significantly decreased in the lung tissues of asthmatic mice treated with QF (Figure 8A). The effect of QF on reducing p-JNK expression levels was less efficient than BUD treatment (p < 0.01), whereas the effect on reducing p-P38 expression levels was not significantly different from BUD (p > 0.05; Figure 8C). Similarly, compared to the control mice, mice with OVA-induced asthma showed a significantly higher expression of NLRP3, Caspase-1, IL-1β in the lung tissues, and the expression of these key proteins was significantly decreased in asthmatic mice treated with QF (Figure 8B). When compared to BUD treatment, QF had more effect on lowering NLRP3, Caspase-1 and IL-1β expression levels (Figure 8D). These results further confirmed the metagenomic functional prediction of QF in the respiratory microbiota.
FIGURE 8. Effects of Qingfei oral liquid (QF) on the expression of key proteins related to MAPK and NOD-like receptor signal pathway. (A) Representative immunohistochemistry staining of p-P38 and p-JNK (200×, Brown particles represent positive protein expression). (B) Representative immunohistochemistry staining of NLRP3, caspase-1, and IL-1β (precursor IL-1 β and cleaved IL-1 β) (200×, Brown particles represent positive protein expression). (C) The positive area of p-P38 and p-JNK (%). (D) The positive area of NLRP3, caspase-1, and IL-1β (%). Each bar represents the mean ± standard deviation (SD). For all of these key proteins, there were significant differences between the control and the OVA groups (p ≤ 0.01). (n = 6, △p ≤ 0.05 vs. the OVA group, △△p ≤ 0.01 vs. the OVA group; #p ≤ 0.05 vs. the budesonide (BUD) group, ##p ≤ 0.01 vs. the BUD group; ns: no difference; one-way ANOVA followed by Tukey’s test).
In the present study, we explored the anti-inflammatory activities of QF and its influence on respiratory microbiota in mice with OVA-induced asthma. We found that the downregulation of MAPK and Nod-like receptor signaling pathways may be the underlying mechanism of QF which alleviates airway inflammation in asthma by regulating respiratory microbiota. Furthermore, we also identified and analyzed bioactive compounds in QF, including baicalin, wogonoside, amygdalin, cryptotanshinone, polydatin, emodin, resveratrol, and baicalein, among others, using UHPLC-MS/MS. Previous studies have shown that these compounds have anti-inflammatory (He et al., 2020), anti-oxidation (Nagappan et al., 2019), anti-allergic, spasmolytic, immune response inhibition (Bui et al., 2017; Son et al., 2021) and other effects.
Our study found that QF can significantly reduce the aggregation of inflammatory cells around the small airway, thickening of the bronchial wall, and secretion of mucus in the lung tissue of OVA-induced asthmatic mice, and also reduce the levels of IgE, IL-4, IL-6, and IL-17A in BALF. The best effect was delivered by QF at a medium dose. QF has been widely used in the treatment of pediatric respiratory diseases in China and has been approved by the Chinese National Drug administration (Lin et al., 2021). Children aged about 6 years old should take one dose (crude drug 91 g) daily, which is equivalent to the medium dose of mice according to the conversion method of body surface area between mice and human. In our study, the high-dose and low-dose groups were set up respectively, that is, the dose of the medium-dose group was doubled, and the dose of the medium-dose group was halved. The high-dose group was set to see if increasing the dose would lead to better results. The experimental results showed that the high dose group could not improve the clinical efficacy in OVA-induced asthmatic mouse models. From the composition of Qingfei oral liquid, there are some herbs that affect the metabolism of bacteria. High dose of QF might affect some beneficial bacteria in respiratory tract, so the effect is reduced. It is suggested that there is no need to increase the dose of QF in clinical application to improve the efficacy. Previous research by other members of the research team found that QF alleviated asthma exacerbation by decreasing the levels of IL-4, IL-6, and IL-13 in the serum and inflammatory cells in the lung tissue of RSV-infected asthmatic mice (Zhou et al., 2018; Yu et al., 2021). In this study, QF was comparable to budesonide in reducing IL-6 and IL-17A and increasing IL-10 levels, but not as effective as budesonide in reducing IgE and IL-4 levels. Numerous previous studies (Kuo et al., 2017; Lambrecht et al., 2019; Hinks et al., 2021) have shown that eosinophilic asthma is mainly characterized by IgE and Th2 cytokines (IL-4, IL-5, etc.), whereas neutrophil asthma is mainly characterized by Th17 cytokines (IL-17, IL-6, etc.), which are involved in the development of steroid-resistant asthma (Nabe, 2020). In this study, a mouse model of OVA-induced asthma was dominated by eosinophil inflammatory infiltration. This is based on the increased the total IgE, OVA-specific IgE, and IL-4 levels as shown in Figure 3, and the highest proportion of eosinophils in BALF in Supplementary Figure S2. Therefore, the mouse model of OVA-induced asthma is mainly Th2-driven inflammation in this study. Interestingly, genetic predisposition and microbial metabolites appear to influence T cell differentiation plasticity. Several recent studies have linked the pathogenesis of asthma caused by IgE and IL-4 to a TH17-dependent mechanism. LPS stimulation promotes the transition from Th2-derived airway eosinophil inflammation to Th17-derived neutrophil inflammation in an ovalbumin allergy mouse model of asthma (Zhao et al., 2017), and IgE-related polymorphisms affect asthma TH 17 gene expression (Worth et al., 2018). Furthermore, IL4R variants linked to allergic asthma were discovered to exacerbate airway inflammation by promoting regulatory T cell conversion to TH17-like cells (Massoud et al., 2016). Numerous studies have shown that allergic asthma can progress to IL-17-mediated neutrophilic asthma. Even so, as the inflammation persisted, neutrophil inflammation mediated by Th17 cells and cytokines began to play a role in the asthma process, due to the persistence of inflammation, neutrophil inflammation mediated by Th17 cell and cytokines also began to participate in the process of asthma. In our previous study (Zheng et al., 2021), dynamic observation of OVA-induced asthmatic mouse models showed that the 2-week phase of nebulization was the transition from eosinophilic inflammation to neutrophil inflammation and the initiation of small airway remodeling, so we selected the asthmatic mouse model during this critical transition period for our study. Many previous studies (Lönnkvist et al., 2001; Wenzel et al., 2016) have shown that Budesonide has a unique advantage for eosinophil asthma. In our asthmatic mouse model, it was found that Budesonide had a stronger inhibitory effect on eosinophilic inflammation than Qingfei oral Liquid (QF), but Traditional Chinese Medicine (TCM) compounds QF had a stronger inhibitory effect on IL-17 induced neutrophil inflammation than Budesonide. These results suggest that QF may have some advantages in the treatment of neutrophil asthma.
Our previous studies found that changes in respiratory microbiota are closely related to the pathophysiological processes of asthma, including airway inflammation (Zheng et al., 2021). Therefore, we proposed the following hypothesis: QF alleviates airway inflammation by regulating the respiratory microbiota of asthmatic mice. We found that QF and budesonide had no significant effect on the α-diversity of the respiratory microbiota in asthmatic mice, but both could affect the β-diversity of the respiratory microbiota to some extent. Since QF can alter the β-diversity of the respiratory microbiota composition in asthmatic mice, we further explored its effect on the composition of the respiratory microbiota. At the phylum level, compared to normal mice, the abundance of Proteobacteria in asthmatic mice was significantly increased, while that of Actinobacteria was significantly decreased. Previous studies have shown a significant increase in Proteobacteria in patients with asthma, especially in severe or steroid-resistant asthma (Li et al., 2017; Taylor et al., 2018). Actinobacteria were found to be more abundant in the normal population and in patients with eosinophilic asthma (Li et al., 2017; Hufnagl et al., 2020). Surprisingly, QF significantly reduced the abundance of Proteobacteria and slightly increased the abundance of Actinobacteria in the asthmatic mice. This may be one of the mechanisms through which QF reduces neutrophil inflammation. At the genus level, the abundance of Pseudomonas was significantly higher in asthmatic mice than in normal mice, while QF significantly reduced the abundance of Pseudomonas. Ferri et al. (2020) showed that the presence of Pseudomonas in sputum is an important risk factor for the persistent and frequent exacerbation of asthma. These results suggest that QF can regulate the composition of the respiratory microbiota in asthmatic mice.
Therefore, what effect does QF have on the host by regulating the dysbiosis of the respiratory microbiota in asthmatic mice? We explored the differences in bacterial metagenomic functional predictions. The results showed that the “MAPK signaling pathway-yeast” and “NOD-like receptor signaling pathway” related to airway inflammation were significantly upregulated in asthmatic mice, and QF significantly downregulated these two signaling pathways in the LRT. The three subfamilies of MAPKs have been involved in the pathogenesis of asthma, and JNK and P-38, in particular, are more closely associated with asthmatic airway inflammation, which can cause high expression of downstream factors such as IL-6, IL-1β, and IL-4 and IL-5, respectively (Dong et al., 2002; Pahl et al., 2002; Khorasanizadeh et al., 2017). The phosphorylation states and/or activities of all three MAPK members are upregulated in animal models of asthma (Dong et al., 2002). Inhibitors of JNK and P-38 both can significantly reduce airway inflammation in asthmatic patients (Bhavsar et al., 2010; Khorasanizadeh et al., 2017). NOD-like receptors (NLRs; nucleotide-binding oligomerization do-main-like receptors) represent a class of widespread, sophisticated signaling regulators, including more than 20 members have been reported (Liu et al., 2019). NLRP3 belongs to the NLRP subfamily, which is an inflammatory protein complex composed of the intracellular innate immune receptor NLRP3, adaptor protein ASC, and protease caspase-1 (Guo et al., 2015). They can help the body recognize endogenous and exogenous abnormal substances and release the inflammatory factors IL-1β and IL-18 (Tang et al., 2019). Previous studies have shown that elevated caspase-1 and IL-1β can be detected in mouse models of asthma, and increased IL-18 can also be detected in the sputum of asthmatic patients and is associated with asthma severity (Harada et al., 2009; Liao et al., 2015).
In order to verify the metagenomic function prediction results, this study further explored the expression of key proteins related to MAPK and NOD-like receptor signaling pathways in the lung tissues. The expression levels of p-P38 and p-JNK were significantly increased in the lung tissues of asthmatic mice but significantly decreased in the lung tissues of asthmatic mice treated with QF. Similarly, mice with OVA-induced asthma showed a significantly higher expression of NLRP3, Caspase-1, IL-1β in lung tissues, and QF can significantly downregulate the expression levels of these proteins. These results suggest that the potential mechanism of QF to alleviate airway inflammation in asthma by regulating respiratory microbiota may be related to downregulation of MAPK and NOD-like receptor signaling pathways. However, there were still many limitations to our research. For example, the in vivo sample size is relatively small. In addition, more studies are needed to confirm the strong link between QF in reducing airway inflammation and regulating respiratory microbiota.
In conclusion, we observed the anti-airway inflammation effect of QF in OVA-induced asthmatic mice, and for the first time, we explored the effect of QF on the composition of the respiratory microbiota in asthmatic mice. Our study found that QF can regulate the composition of respiratory microbiota in asthmatic mice, and metagenomic function prediction suggests that QF can downregulate MAPK and NOD-like receptor signaling pathways that are significantly upregulated in asthmatic mice. Immunohistochemical results showed that QF could downregulate the expression of p-JNK, p-P38, NLRP3, Caspase-1, and IL-1β, which are the key proteins in the signaling pathways of lung tissue. This study provides a theoretical basis for effective use of QF in asthmatic airway inflammation.
The data presented in the study are deposited in the NCBI repository, accession number PRJNA730096 and SRP320140.
The animal study was reviewed and approved by the Center for Laboratory Animals, Shanghai Children’s Hospital, School of Medicine. Shanghai Jiao Tong University, Shanghai, China.
LH and SG conceived and designed the study, critically revised the manuscript, and were responsible for the funding. JZ, QW, LZ, YZ, and MW completed the animal experiments, acquired and interpreted the data, and drafted and critically revised the manuscript. All the authors have read and approved the final manuscript.
This study was financially supported by two grants from the National Natural Science Foundation of China (Nos. 81774365 and 81973902).
We are grateful to Xiao-Yong Fan (TB Center, Shanghai Emerging and Reemerging Infectious Disease Institute, Shanghai) for his help with the experimental techniques.
The authors declare that the research was conducted in the absence of any commercial or financial relationships that could be construed as a potential conflict of interest.
All claims expressed in this article are solely those of the authors and do not necessarily represent those of their affiliated organizations, or those of the publisher, the editors and the reviewers. Any product that may be evaluated in this article, or claim that may be made by its manufacturer, is not guaranteed or endorsed by the publisher.
The Supplementary Material for this article can be found online at: https://www.frontiersin.org/articles/10.3389/fphar.2022.911667/full#supplementary-material
SUPPLEMENTARY FIGURE S1 | Total ion chromatography (TIC) of the blank samples. TIC of the blank samples in positive ion mode; (B) Total ion chromatography of the blank samples in negative ion mode.
SUPPLEMENTARY FIGURE S2 | The leukocyte cell type counts in BALF samples. The BALF was centrifuged and the precipitated cells were counted after staining by Wright-Giemsa. Data are expressed as the mean ± standard deviation (SD) and were performed with one-way ANOVA followed by Tukey’s tests. (n = 6, △p ≤ 0.05 vs. the OVA group, △△p ≤ 0.01 vs. the OVA group; #p ≤ 0.05 vs. the budesonide (BUD) group, ##p ≤ 0.01 vs. the BUD group; ◆p ≤ 0.05 vs. the QFM group, ◆◆p ≤ 0.01 vs the QFM group; ns: no difference; line over bars indicates that all groups are include.).
Asher, M. I., García-Marcos, L., Pearce, N. E., and Strachan, D. P. (2020). Trends in worldwide asthma prevalence. Eur. Respir. J. 56, 2002094. doi:10.1183/13993003.02094-2020
Bhavsar, P., Khorasani, N., Hew, M., Johnson, M., and Chung, K. F. (2010). Effect of p38 MAPK inhibition on corticosteroid suppression of cytokine release in severe asthma. Eur. Respir. J. 35, 750–756. doi:10.1183/09031936.00071309
Bokulich, N. A., Dillon, M. R., Bolyen, E., Kaehler, B. D., Huttley, G. A., Caporaso, J. G., et al. (2018). q2-sample-classifier: machine-learning tools for microbiome classification and regression. J. Open Res. Softw. 3, 934. doi:10.21105/joss.00934
Budden, K. F., Shukla, S. D., Rehman, S. F., Bowerman, K. L., Keely, S., Hugenholtz, P., et al. (2019). Functional effects of the microbiota in chronic respiratory disease. Lancet. Respir. Med. 7, 907–920. doi:10.1016/s2213-2600(18)30510-1
Bui, T. T., Piao, C. H., Song, C. H., Lee, C. H., Shin, H. S., Chai, O. H., et al. (2017). Baicalein, wogonin, and Scutellaria baicalensis ethanol extract alleviate ovalbumin-induced allergic airway inflammation and mast cell-mediated anaphylactic shock by regulation of Th1/Th2 imbalance and histamine release. Anat. Cell Biol. 50, 124–134. doi:10.5115/acb.2017.50.2.124
Callahan, B. J., Mcmurdie, P. J., Rosen, M. J., Han, A. W., Johnson, A. J., Holmes, S. P., et al. (2016). DADA2: high-resolution sample inference from Illumina amplicon data. Nat. Methods 13, 581–583. doi:10.1038/nmeth.3869
Cui, F., Shi, C. L., Zhou, X. J., Wen, W., Gao, X. P., Wang, L. Y., et al. (2020). Lycium barbarum polysaccharide extracted from lycium barbarum leaves ameliorates asthma in mice by reducing inflammation and modulating gut microbiota. J. Med. Food 23, 699–710. doi:10.1089/jmf.2019.4544
Dong, C., Davis, R. J., and Flavell, R. A. (2002). MAP kinases in the immune response. Annu. Rev. Immunol. 20, 55–72. doi:10.1146/annurev.immunol.20.091301.131133
Ferri, S., Crimi, C., Campisi, R., Cacopardo, G., Paoletti, G., Puggioni, F., et al. (2020). Impact of asthma on bronchiectasis severity and risk of exacerbations. J. Asthma 59, 469–475. doi:10.1080/02770903.2020.1857395
Finkelman, F. D., Hogan, S. P., Hershey, G. K., Rothenberg, M. E., and Wills-Karp, M. (2010). Importance of cytokines in murine allergic airway disease and human asthma. J. Immunol. 184, 1663–1674. doi:10.4049/jimmunol.0902185
Gandhi, G. R., Leão, G. C. S., Calisto, V., Vasconcelos, A. B. S., Almeida, M. L. D., Quintans, J. S. S., et al. (2020). Modulation of interleukin expression by medicinal plants and their secondary metabolites: a systematic review on anti-asthmatic and immunopharmacological mechanisms. Phytomedicine 70, 153229. doi:10.1016/j.phymed.2020.153229
Gritsenko, A., Green, J. P., Brough, D., and Lopez-Castejon, G. (2020). Mechanisms of NLRP3 priming in inflammaging and age related diseases. Cytokine Growth Factor Rev. 55, 15–25. doi:10.1016/j.cytogfr.2020.08.003
Guo, H., Callaway, J. B., and Ting, J. P. (2015). Inflammasomes: mechanism of action, role in disease, and therapeutics. Nat. Med. 21, 677–687. doi:10.1038/nm.3893
Hall, M., and Beiko, R. G. (2018). 16S rRNA gene analysis with QIIME2. Methods Mol. Biol. 1849, 113–129. doi:10.1007/978-1-4939-8728-3_8
Harada, M., Obara, K., Hirota, T., Yoshimoto, T., Hitomi, Y., Sakashita, M., et al. (2009). A functional polymorphism in IL-18 is associated with severity of bronchial asthma. Am. J. Respir. Crit. Care Med. 180, 1048–1055. doi:10.1164/rccm.200905-0652OC
He, X. Y., Wu, L. J., Wang, W. X., Xie, P. J., Chen, Y. H., Wang, F., et al. (2020). Amygdalin - a pharmacological and toxicological review. J. Ethnopharmacol. 254, 112717. doi:10.1016/j.jep.2020.112717
Hinks, T. S. C., Levine, S. J., and Brusselle, G. G. (2021). Treatment options in type-2 low asthma. Eur. Respir. J. 57, 2000528. doi:10.1183/13993003.00528-2020
Huang, Y. J., Nariya, S., Harris, J. M., Lynch, S. V., Choy, D. F., Arron, J. R., et al. (2015). The airway microbiome in patients with severe asthma: associations with disease features and severity. J. Allergy Clin. Immunol. 136, 874–884. doi:10.1016/j.jaci.2015.05.044
Hufnagl, K., Pali-Schöll, I., Roth-Walter, F., and Jensen-Jarolim, E. (2020). Dysbiosis of the gut and lung microbiome has a role in asthma. Semin. Immunopathol. 42, 75–93. doi:10.1007/s00281-019-00775-y
Jing, X., Yan, W., Zeng, H., and Cheng, W. (2020). Qingfei oral liquid alleviates airway hyperresponsiveness and mucus hypersecretion via TRPV1 signaling in RSV-infected asthmatic mice. Biomed. Pharmacother. 128, 110340. doi:10.1016/j.biopha.2020.110340
Kanehisa, M., Goto, S., Kawashima, S., Okuno, Y., and Hattori, M. (2004). The KEGG resource for deciphering the genome. Nucleic Acids Res. 32, D277–D280. doi:10.1093/nar/gkh063
Khorasanizadeh, M., Eskian, M., Gelfand, E. W., and Rezaei, N. (2017). Mitogen-activated protein kinases as therapeutic targets for asthma. Pharmacol. Ther. 174, 112–126. doi:10.1016/j.pharmthera.2017.02.024
Kubo, F., Ariestanti, D. M., Oki, S., Fukuzawa, T., Demizu, R., Sato, T., et al. (2019). Loss of the adhesion G-protein coupled receptor ADGRF5 in mice induces airway inflammation and the expression of CCL2 in lung endothelial cells. Respir. Res. 20, 11. doi:10.1186/s12931-019-0973-6
Kujur, W., Gurram, R. K., Haleem, N., Maurya, S. K., and Agrewala, J. N. (2015). Caerulomycin A inhibits Th2 cell activity: a possible role in the management of asthma. Sci. Rep. 5, 15396. doi:10.1038/srep15396
Kuo, C. S., Pavlidis, S., Loza, M., Baribaud, F., Rowe, A., Pandis, I., et al. (2017). T-helper cell type 2 (Th2) and non-Th2 molecular phenotypes of asthma using sputum transcriptomics in U-BIOPRED. Eur. Respir. J. 49, 1602135. doi:10.1183/13993003.02135-2016
Lambrecht, B. N., Hammad, H., and Fahy, J. V. (2019). The cytokines of asthma. Immunity 50, 975–991. doi:10.1016/j.immuni.2019.03.018
Langille, M. G., Zaneveld, J., Caporaso, J. G., Mcdonald, D., Knights, D., Reyes, J. A., et al. (2013). Predictive functional profiling of microbial communities using 16S rRNA marker gene sequences. Nat. Biotechnol. 31, 814–821. doi:10.1038/nbt.2676
Larsen, J. M., Musavian, H. S., Butt, T. M., Ingvorsen, C., Thysen, A. H., Brix, S., et al. (2015). Chronic obstructive pulmonary disease and asthma-associated Proteobacteria, but not commensal Prevotella spp., promote Toll-like receptor 2-independent lung inflammation and pathology. Immunology 144, 333–342. doi:10.1111/imm.12376
Li, N., Qiu, R., Yang, Z., Li, J., Chung, K. F., Zhong, N., et al. (2017). Sputum microbiota in severe asthma patients: relationship to eosinophilic inflammation. Respir. Med. 131, 192–198. doi:10.1016/j.rmed.2017.08.016
Liao, Z., Xiao, H. T., Zhang, Y., Tong, R. S., Zhang, L. J., Bian, Y., et al. (2015). IL-1β: a key modulator in asthmatic airway smooth muscle hyper-reactivity. Expert Rev. Respir. Med. 9, 429–436. doi:10.1586/17476348.2015.1063422
Licari, A., Brambilla, I., Marseglia, A., De Filippo, M., Paganelli, V., Marseglia, G. L., et al. (2018). Difficult vs. Severe asthma: definition and limits of asthma control in the pediatric population. Front. Pediatr. 6, 170. doi:10.3389/fped.2018.00170
Lin, L., An, L., Chen, H., Feng, L., Lu, M., Liu, Y., et al. (2021). Integrated network Pharmacology and lipidomics to reveal the inhibitory effect of Qingfei oral liquid on excessive autophagy in RSV-induced lung inflammation. Front. Pharmacol. 12, 777689. doi:10.3389/fphar.2021.777689
Liu, P., Lu, Z., Liu, L., Li, R., Liang, Z., Shen, M., et al. (2019). NOD-like receptor signaling in inflammation-associated cancers: from functions to targeted therapies. Phytomedicine 64, 152925. doi:10.1016/j.phymed.2019.152925
Lönnkvist, K., Hellman, C., Lundahl, J., Halldén, G., and Hedlin, G. (2001). Eosinophil markers in blood, serum, and urine for monitoring the clinical course in childhood asthma: impact of budesonide treatment and withdrawal. J. Allergy Clin. Immunol. 107 (5), 812–817. doi:10.1067/mai.2001.114246
Louvrier, C., Assrawi, E., El Khouri, E., Melki, I., Copin, B., Bourrat, E., et al. (2020). NLRP3-associated autoinflammatory diseases: phenotypic and molecular characteristics of germline versus somatic mutations. J. Allergy Clin. Immunol. 145, 1254–1261. doi:10.1016/j.jaci.2019.11.035
Manley, G. C. A., Parker, L. C., and Zhang, Y. (2019). Emerging regulatory roles of dual-specificity phosphatases in inflammatory airway disease. Int. J. Mol. Sci. 20, E678. doi:10.3390/ijms20030678
Massoud, A. H., Charbonnier, L. M., Lopez, D., Pellegrini, M., Phipatanakul, W., Chatila, T. A., et al. (2016). An asthma-associated IL4R variant exacerbates airway inflammation by promoting conversion of regulatory T cells to TH17-like cells. Nat. Med. 22 (9), 1013–1022. doi:10.1038/nm.4147
Murphy, K. R., Hong, J. G., Wandalsen, G., Larenas-Linnemann, D., El Beleidy, A., Zaytseva, O. V., et al. (2020). Nebulized inhaled corticosteroids in asthma treatment in children 5 Years or younger: a systematic review and global expert analysis. J. Allergy Clin. Immunol. Pract. 8, 1815–1827. doi:10.1016/j.jaip.2020.01.042
Nabe, T. (2020). Steroid-resistant asthma and neutrophils. Biol. Pharm. Bull. 43, 31–35. doi:10.1248/bpb.b19-00095
Nagappan, A., Kim, J. H., Jung, D. Y., and Jung, M. H. (2019). Cryptotanshinone from the salvia miltiorrhiza bunge attenuates ethanol-induced liver injury by activation of AMPK/SIRT1 and Nrf2 signaling pathways. Int. J. Mol. Sci. 21, E265. doi:10.3390/ijms21010265
Pahl, A., Zhang, M., Kuss, H., Szelenyi, I., and Brune, K. (2002). Regulation of IL-13 synthesis in human lymphocytes: implications for asthma therapy. Br. J. Pharmacol. 135, 1915–1926. doi:10.1038/sj.bjp.0704656
Parks, D. H., Tyson, G. W., Hugenholtz, P., and Beiko, R. G. (2014). Stamp: statistical analysis of taxonomic and functional profiles. Bioinformatics 30, 3123–3124. doi:10.1093/bioinformatics/btu494
Price, M. N., Dehal, P. S., and Arkin, A. P. (2009). FastTree: computing large minimum evolution trees with profiles instead of a distance matrix. Mol. Biol. Evol. 26, 1641–1650. doi:10.1093/molbev/msp077
Ramakrishnan, R. K., Al Heialy, S., and Hamid, Q. (2019). Role of IL-17 in asthma pathogenesis and its implications for the clinic. Expert Rev. Respir. Med. 13, 1057–1068. doi:10.1080/17476348.2019.1666002
Robinson, D., Humbert, M., Buhl, R., Cruz, A. A., Inoue, H., Korom, S., et al. (2017). Revisiting type 2-high and type 2-low airway inflammation in asthma: current knowledge and therapeutic implications. Clin. Exp. Allergy 47, 161–175. doi:10.1111/cea.12880
Segal, L. N., Clemente, J. C., Li, Y., Ruan, C., Cao, J., Danckers, M., et al. (2017a). Anaerobic bacterial fermentation products increase tuberculosis risk in antiretroviral-drug-treated HIV patients. Cell Host Microbe 21, 530. doi:10.1016/j.chom.2017.03.003
Segal, L. N., Clemente, J. C., Wu, B. G., Wikoff, W. R., Gao, Z., Li, Y., et al. (2017b). Randomised, double-blind, placebo-controlled trial with azithromycin selects for anti-inflammatory microbial metabolites in the emphysematous lung. Thorax 72, 13–22. doi:10.1136/thoraxjnl-2016-208599
Segata, N., Izard, J., Waldron, L., Gevers, D., Miropolsky, L., Garrett, W. S., et al. (2011). Metagenomic biomarker discovery and explanation. Genome Biol. 12, R60. doi:10.1186/gb-2011-12-6-r60
Son, S. H., Kang, J., Ahn, M., Nam, S., Jung, Y. W., Lee, K. Y., et al. (2021). Synthesis and biochemical evaluation of baicalein prodrugs. Pharmaceutics 13, 1516. doi:10.3390/pharmaceutics13091516
Tang, Y. S., Zhao, Y. H., Zhong, Y., Li, X. Z., Pu, J. X., Luo, Y. C., et al. (2019). Neferine inhibits LPS-ATP-induced endothelial cell pyroptosis via regulation of ROS/NLRP3/Caspase-1 signaling pathway. Inflamm. Res. 68, 727–738. doi:10.1007/s00011-019-01256-6
Taylor, S. L., Leong, L. E. X., Choo, J. M., Wesselingh, S., Yang, I. A., Upham, J. W., et al. (2018). Inflammatory phenotypes in patients with severe asthma are associated with distinct airway microbiology. J. Allergy Clin. Immunol. 141, 94. doi:10.1016/j.jaci.2017.03.044
Temelkovski, J., Hogan, S. P., Shepherd, D. P., Foster, P. S., and Kumar, R. K. (1998). An improved murine model of asthma: selective airway inflammation, epithelial lesions and increased methacholine responsiveness following chronic exposure to aerosolised allergen. Thorax 53, 849–856. doi:10.1136/thx.53.10.849
Teo, S. M., Tang, H. H. F., Mok, D., Judd, L. M., Watts, S. C., Pham, K., et al. (2018). Airway microbiota dynamics uncover a critical window for interplay of pathogenic bacteria and allergy in childhood respiratory disease. Cell Host Microbe 24, 341. doi:10.1016/j.chom.2018.08.005
Tsang, M. S., Cheng, S. W., Zhu, J., Atli, K., Chan, B. C., Liu, D., et al. (2018). Anti-inflammatory activities of pentaherbs formula and its influence on gut microbiota in allergic asthma. Molecules 23, E2776. doi:10.3390/molecules23112776
Tumes, D. J., Papadopoulos, M., Endo, Y., Onodera, A., Hirahara, K., Nakayama, T., et al. (2017). Epigenetic regulation of T-helper cell differentiation, memory, and plasticity in allergic asthma. Immunol. Rev. 278, 8–19. doi:10.1111/imr.12560
Wenzel, S., Castro, M., Corren, J., Maspero, J., Wang, L., Zhang, B., et al. (2016). Dupilumab efficacy and safety in adults with uncontrolled persistent asthma despite use of medium-to-high-dose inhaled corticosteroids plus a long-acting β2 agonist: a randomised double-blind placebo-controlled pivotal phase 2b dose-ranging trial. Lancet (London, Engl.) 388 (10039), 31–44. doi:10.1016/S0140-6736(16)30307-5
Worth, L., Michel, S., Gaertner, V. D., Kabesch, M., and Schieck, M. (2018). Asthma- and IgE-associated polymorphisms affect expression of TH 17 genes. Allergy 73 (6), 1342–1347. doi:10.1111/all.13422
Yu, L., Wang, J., Zou, Y., Zeng, H., Cheng, W., Jing, X., et al. (2021). Qingfei oral liquid inhibited autophagy to alleviate inflammation via mTOR signaling pathway in RSV-infected asthmatic mice. Biomed. Pharmacother. 138, 111449. doi:10.1016/j.biopha.2021.111449
Zhao, S., Jiang, Y., Yang, X., Guo, D., Wang, Y., Wang, J., et al. (2017). Lipopolysaccharides promote a shift from Th2-derived airway eosinophilic inflammation to Th17-derived neutrophilic inflammation in an ovalbumin-sensitized murine asthma model. J. Asthma 54 (5), 447–455. doi:10.1080/02770903.2016.1223687
Zheng, J., Wu, Q., Zou, Y., Wang, M., He, L., Guo, S., et al. (2021). Respiratory microbiota profiles associated with the progression from airway inflammation to remodeling in mice with OVA-induced asthma. Front. Microbiol. 12, 723152. doi:10.3389/fmicb.2021.723152
Zhou, L. H., Xu, J. Y., Dai, C., Fan, Y. M., and Yuan, B. (2018). Label-free quantitative proteomics reveals fibrinopeptide B and heparin cofactor II as potential serum biomarkers in respiratory syncytial virus-infected mice treated with Qingfei oral liquid formula. Chin. J. Nat. Med. 16 (4), 241–251. doi:10.1016/S1875-5364(18)30054-2
Keywords: airway inflammation, asthma, metagenomic functional prediction, Qingfei oral liquid, respiratory microbiota
Citation: Zheng J, Wu Q, Zhang L, Zou Y, Wang M, He L and Guo S (2022) Anti-inflammatory activities of Qingfei oral liquid and its influence on respiratory microbiota in mice with ovalbumin-induced asthma. Front. Pharmacol. 13:911667. doi: 10.3389/fphar.2022.911667
Received: 03 April 2022; Accepted: 05 July 2022;
Published: 23 August 2022.
Edited by:
Joan Villena García, Universidad de Valparaíso, ChileReviewed by:
Ming-Ling Kuo, Chang Gung University, TaiwanCopyright © 2022 Zheng, Wu, Zhang, Zou, Wang, He and Guo. This is an open-access article distributed under the terms of the Creative Commons Attribution License (CC BY). The use, distribution or reproduction in other forums is permitted, provided the original author(s) and the copyright owner(s) are credited and that the original publication in this journal is cited, in accordance with accepted academic practice. No use, distribution or reproduction is permitted which does not comply with these terms.
*Correspondence: Li He, aGVsQHNoY2hpbGRyZW4uY29tLmNu; Sheng Guo, Z3Vvc2hlbmdAc2hjaGlsZHJlbi5jb20uY24=
†These authors have contributed equally to this work
Disclaimer: All claims expressed in this article are solely those of the authors and do not necessarily represent those of their affiliated organizations, or those of the publisher, the editors and the reviewers. Any product that may be evaluated in this article or claim that may be made by its manufacturer is not guaranteed or endorsed by the publisher.
Research integrity at Frontiers
Learn more about the work of our research integrity team to safeguard the quality of each article we publish.