- 1Department of Pharmacy, Zhejiang Pharmaceutical College, Ningbo, China
- 2The Affiliated Cancer Hospital of Zhengzhou University & Henan Cancer Hospital, Henan Province Engineering Research Center of Artificial Intelligence and Internet of Things Wise Medical, Zhengzhou, China
- 3Institute of Pharmaceutical Sciences, University of Veterinary and Animal Sciences, Lahore, Pakistan
- 4Hospital Laboratory of Nangjing Lishui People’s Hospital, Nangjing, China
- 5Laboratory of Biomedical Engineering for Novel Bio-functional, and Pharmaceutical Nanomaterials, Prince Philip Dental Hospital, Faculty of Dentistry, The University of Hong Kong, Hong Kong, Hong Kong SAR, China
Cancer is a disease that seriously threatens human health. Based on the improvement of traditional treatment methods and the development of new treatment modes, the pattern of cancer treatment is constantly being optimized. Nanomedicine plays an important role in these evolving tumor treatment modalities. In this article, we outline the applications of nanomedicine in three important tumor-related fields: chemotherapy, gene therapy, and immunotherapy. According to the current common problems, such as poor targeting of first-line chemotherapy drugs, easy destruction of nucleic acid drugs, and common immune-related adverse events in immunotherapy, we discuss how nanomedicine can be combined with these treatment modalities, provide typical examples, and summarize the advantages brought by the application of nanomedicine.
Introduction
In recent years, the incidence and mortality of cancer have been increasing year by year under the influence of many factors such as population aging, work pressure, and environmental changes, etc. According to the 2020 Global Cancer Statistics Report, there were approximately 19.3 million new cancer cases and 10 million deaths that year (Sung et al., 2021). The treatment of cancer has now become an important issue to be solved urgently in the medical field. For the treatment of cancers, chemotherapy is a commonly used and effective drug therapy. However, its shortcomings and limitations in clinical application have also been shown, such as application limitations due to poor water solubility, serious adverse events caused by non-specific distribution, etc. In recent years, with the development of technology and the innovation of treatment concepts, new treatment methods such as gene therapy and immunotherapy are changing the pattern of tumor treatment (Libutti, 2019; Tan et al., 2020). However, the in vivo delivery of anti-tumor gene therapy and immunotherapy drugs still faces challenges, such as low tumor cell uptake rate and poor tumor permeability, which seriously affect the therapeutic effect. Nanoparticulate delivery systems (NDSs)is an important way to optimize drug delivery, which can effectively improve the accumulation, penetration and target cell uptake of drugs in tumor tissue and achieve controllable drug release. Replacing traditional drug delivery with NDSs can enhance the efficacy of treatment and reduce the incidence of adverse events, and has shown significant clinical benefits (Collins and Thrasher, 2015; Raza et al., 2019; Riley et al., 2019; Yahya and Alqadhi, 2021; Raza et al., 2022). In this review, we will review the progress of NDSs and the application of nanomedicine in cancer therapy, focusing on the new progress in the application of nanomedicine in chemotherapy, gene therapy and immunotherapy.
Nanoparticulate Delivery Systems
Relying on the vigorous development of nanotechnology, NDSs have attracted more and more attention of scientists, and also showed great application value and broad development prospects. Nanomaterials refer to particles with a size of less than 100 nm, or with a size of less than 1 μm that can exhibit nanoparticle properties, and whose structural units are generally smaller than the cell volume. Compared with traditional drug delivery systems, NDSs can effectively improve pharmacokinetics and pharmacodynamics due to their specificity in size, material, and shape (Kinnear et al., 2017). Due to the heterogeneity and complexity of tumors, nanomaterials used in cancer therapy are often designed as multifunctional nanoplatforms, which are usually composed of loaded drugs, structural frameworks and functional units. Compared with traditional drugs, NDSs has obvious advantages in tumor treatment: 1) NDSs can effectively deliver drugs with different physicochemical properties, such as: solving the difficult problem of hydrophobic drug delivery, effectively delivering charged nucleic acid drugs and protecting them from nuclease degradation. 2) NDSs can deliver multiple types of drugs simultaneously. 3) NDSs can simultaneously realize tumor diagnosis and treatment. 4) NDSs can improve the targeting of drugs, and both passive targeting based on enhanced permeability and retention effects and active targeting under functional element modification have demonstrated distinct advantages. 5) NDSs can achieve controlled release of drugs. Namely engineered stimuli-responsive nanomaterials enable precise drug delivery and controlled release under the influence of endogenous or exogenous stimuli (Zhu G. et al., 2017; Bhushan et al., 2017; Liu et al., 2017; Cao et al., 2020; Zhu et al., 2020; Gote et al., 2021; Li et al., 2021; Yang et al., 2021; Liu et al., 2022).
Chemotherapy Nanomedicine
Chemotherapy is currently one of the most widely used and mature tumor treatment methods in clinical practice. However, there are many problems in the clinical application, such as the lacking target and the large side effects of conventional chemotherapeutic drugs and the poor water solubility of many first-line chemotherapeutic drugs (doxorubicin, paclitaxel, etc.), aggravating the difficulty of clinical treatment. The development and application of nanomaterials optimize the delivery of the above-mentioned chemotherapeutic drugs, and effectively improve the safety and efficacy of chemotherapy (Wei et al., 2021). Nanomaterials currently used for chemotherapeutic drug delivery include organic nanomaterials (Zhang D. Y. et al., 2020), inorganic nanomaterials (Wang C.-S. et al., 2021), composite nanomaterials (Akgöl et al., 2021), and biological nanomaterials (Wang J. et al., 2021). Among them, biological nanomaterials have very high application value in drug delivery because of their high safety, good biocompatibility, easy degradation, and certain targeting properties. Biological nanomaterials include endogenous natural nanomaterials and biomimetic nanomaterials (Navya et al., 2019). Here we take the classic DNA and protein nanomaterials as examples to introduce their application in chemotherapy (Figure 1A).
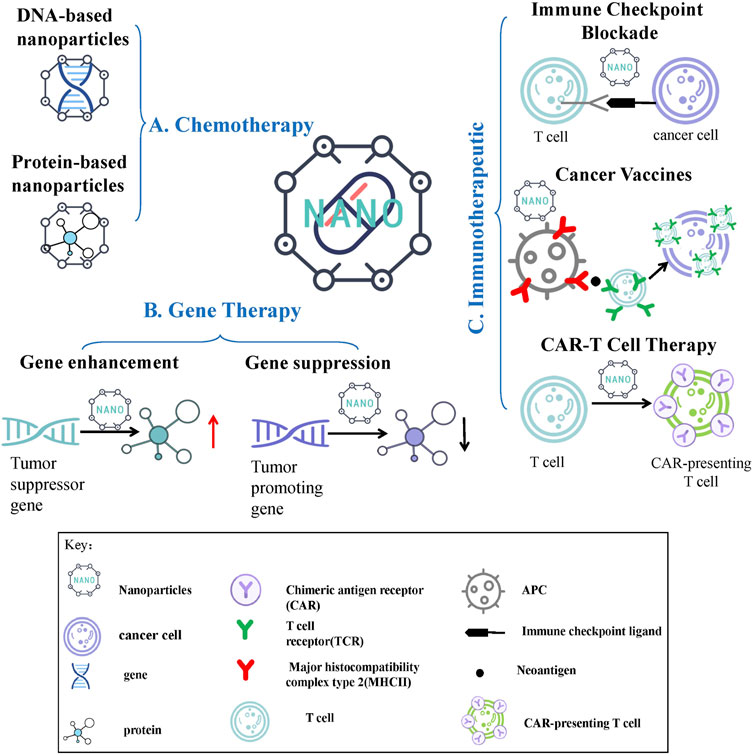
FIGURE 1. Nanomedicine Delivery Strategies for Chemotherapy, Cancer Gene Therapy and Immunotherapy. (A) Chemotherapy nanomedicine: DNA-based nanoparticles and protein-based nanoparticles are shown in this article. (B) Gene therapy nanomedicine: gene enhancement therapy and gene suppression therapy are shown in this article. (C) Immunotherapeutic nanomedicine: immune checkpoint blockade therapy, cancer vaccines and chimeric antigen receptor T cell therapy are shown in this article.
DNA-Based Nanoparticles (NPs)
DNA is the carrier of the genetic information of the organism, plays an important regulatory role in the physiological and pathological processes of the organism, and is an ideal natural drug carrier material. As an emerging drug delivery carrier in recent years, DNA-based nanoparticles (NPs) have significant advantages, with excellent degradability, biocompatibility, and sequence programmability (Xu et al., 2021). DNA-based NPs can effectively load a variety of drugs, and achieve targeted drug delivery to tumor tissues with the assistance of specific functional elements, while improving cellular drug uptake and stimuli-responsive drug release. It is an effective tool to solve common problems of chemotherapy (low efficacy of drugs, large toxic and side effects, etc) and many progress have been made in recent years (Lv et al., 2021). In 1982, Seeman’s group proposed that DNA molecules can construct precise and ordered nanostructures based on the A-T, G-C Watson-Crick base pairing principle, which opened the prelude to DNA nanotechnology. Through rational design, DNA molecules can self-assemble into various kinds of 2D or 3D NPs. The existing DNA self-assembly techniques include DNA Tile self-assembly, rolling circle amplification (RCA), DNA origami, and DNA single-stranded tile self-assembly (Lau and Sleiman, 2016; Mohsen and Kool, 2016; Evans and Winfree, 2017; Ji et al., 2021). In physiological environment, self-assembled DNA NPs can resist degradation to a certain extent and show stronger stability than natural single-stranded or double-stranded DNA, which has high clinical application value (Ahn et al., 2020; Ramezani and Dietz, 2020).
At present, DNA-based NPs have successfully achieved effective delivery of chemotherapeutic drugs such as doxorubicin, daunorubicin, platinum, etc (Halley et al., 2016; Zhang L. et al., 2019; Wu et al., 2019). Doxorubicin, used alone or in combination with other drugs, is one of the most effective chemotherapeutic drugs commonly used clinically, which can treat a variety of tumors including solid tumors and hematological tumors by inhibiting DNA synthesis (Carvalho et al., 2009). We take doxorubicin as an example to illustrate how DNA-based NPs deliver chemotherapeutic drugs. At present, DNA-based NPs of various shapes have been reported for the delivery of doxorubicin by virtue of the drug-embedding properties, covering RCA-based nanostructures (Zhao et al., 2018), DNA tetrahedra (Zhang J. et al., 2021), dendritic DNA nanostructures (Li et al., 2020), and nanostructures based on DNA origami technology (Liu J. et al., 2018), in which tubular and triangular DNA nanocarriers based on DNA origami technology have the characteristics of high drug loading (Guan et al., 2021). Interestingly, DNA-based NPs with specific hydrodynamic dimensions and geometries (triangular DNA origami nanostructures) can passively accumulate in tumor tissues, showing excellent targeting (Zhang et al., 2014; Jiang B. et al., 2019). In order to further improve the targeting of chemotherapeutic drugs and reduce the occurrence of adverse reactions, the latest research pays more attention to the progress of modification. With the help of functional elements such as aptamers, DNA-based NPs can improve the efficacy of chemotherapeutic drugs and reduce adverse reactions through active targeting. For example, Zhang et al. demonstrated that Sgc8 aptamer-modified DNA nanoflowers (NFs) can selectively recognize the cell membrane protein tyrosine kinase 7, and Sgc8-NFs-Ferrocene/Doxorubicin can be selectively protein tyrosine kinase 7 positive cancer cells, significantly improving the tumor-targeting ability of the drug (Zhang Q. et al., 2019). In addition, the significantly different physiological characteristics of tumor tissue and normal tissue have inspired scientists to design many tumor microenvironment-responsive NDSs. Compared with normal tissue, tumor tissue has the following different physiological characteristics, including: 1) high concentration of reducing substances in tumor cells; 2) high concentration of ATP in tumor cells; 3) abnormally expressed enzymes in tumor cells, such as telomerase, matrix metalloproteinase, etc; 4) pH imbalance inside and outside tumor cells (Dai et al., 2017; Jin and Jin, 2020). Given this, the designed DNA-based NPs trigger structural reorganization, exposing the coated drug under the influence of external stimuli such as pH (Zhao et al., 2018), reducing environment (Liu X. et al., 2018), enzymes (Zhang G. et al., 2017), ATP (Lu et al., 2018), etc., which better achieves the precise delivery and release of the drug, thereby effectively increasing the anti-tumor effect of the chemotherapeutic drugs and reducing the damage to normal tissues. For example, Zhao et al. (2018) developed a doxorubicin-loaded DNA NPs with a cancer cell targeting Sgc8 aptamer and a hairpin structure showing pH-responsive doxorubicin loading-releasing capability, which exhibited good biological stability, comparable to doxorubicin loading capacity, specific tumor targeting capacity, and sustained release of pH responsive doxorubicin. The delivery of doxorubicin with the designed DNA-based NPs realized the targeted, controllable and precise release of chemotherapeutic drugs, which enhanced the efficacy of the drug and reduced the occurrence of adverse events. The applications of other DNA nanoparticles in chemotherapy are shown in Table 1.
Protein-Based Nanoparticles (NPs)
Also as a very important natural biological macromolecules, proteins have been widely constructed as nanocarriers for chemotherapeutic drugs in recent years. Similar to DNA-based NPs, protein-based NPs also have many outstanding advantages, such as: 1) good biocompatibility and degradability; 2) natural biological sources, high availability and low toxicity; 3) unique three-dimensional structure; 4) amphiphilic of hydrophilic and hydrophobic molecules or solvents; 5) amino, carboxyl and hydroxyl groups for chemical bonding (Martínez-López et al., 2020). Proteins often designed as nanocarriers mainly include albumin, transferrin, ferritin, low-density lipoprotein, high-density lipoprotein, etc (Iqbal et al., 2021). The application of protein-based NPs to deliver chemotherapeutic drugs has been widely studied, and has shown good clinical application prospects.
When it comes to protein-based NDSs, the most classic one belongs to albumin-bound paclitaxel. Paclitaxel, a hydrophobic anticancer drug, needs to be dissolved in polyoxyethylene castor oil for clinical use, which may cause severe allergic reactions in some patients. Paclitaxel was loaded into albumin via hydrophobic interaction and then constructed into albumin-bound paclitaxel NPs with a diameter of 130 nm, which is traded as Abraxane (Celgene) (Zhang Y. et al., 2020). As the most abundant serum protein in the human body, albumin does not have the immune response caused by castor oil, which not only solves the problem of allergies, but also improves the efficacy and reduces the toxicity to normal tissues and organs. Currently, Abraxane has become one of the best-selling anticancer drugs and is a milestone in protein-based NPs (Yardley, 2013). In addition to non-covalent interactions such as hydrophobic interactions and electrostatic interactions, albumin has abundant binding sites (amine, thiol and carboxyl groups) and also can be used in combination with chemotherapeutic drugs, showing the huge application prospects of albumin (Hoogenboezem and Duvall, 2018; An and Zhang, 2017). Multiple clinical trials of Albumin NPs are currently being pursued, which we list in Table 2. Such as NCT01673438, NCT01580397, NCT02014844, NCT02049905, NCT00477529, NCT00635284, NCT02009332, NCT02494570 (https://clinicaltrials.gov/). Moreover, with the deepening of the research on protein-based NPs, stimuli-responsive NDSs have emerged to further improve the targeting efficiency, which can specifically respond to stimuli in the tumor microenvironment and precisely deliver the drug to the tumor area, enabling targeted therapy (Mi, 2020). As an example, Yang et al. (2020) designed and developed a doxorubicin-loaded NPs containing pH-sensitive Schiff bovine serum albumin, which not only has a high loading capacity but can also trigger the release of doxorubicin by pH.
Another class of proteins commonly constructed as NDSs are iron homeostasis-related proteins, including transferrin and ferritin, both of which have excellent performance in receptor-mediated active targeted delivery. Transferrin, mainly produced by hepatocytes in the human body, is the main iron-containing hydrophilic transporter in plasma, and is mainly responsible for transporting iron in hepatocytes to cells in other tissues. Transferrin is composed of a single-chain glycoprotein and is divided into two evolutionary lobes, namely C-lobe (343 amino acids) and Nlobe (336 amino acids), which are connected to each other by a short spacer (Elsayed et al., 2016; Gou et al., 2018; Zhang et al., 2018). Transferrin mediates iron uptake by binding to the transferrin receptor (TfR). TfR, a transmembrane glycoprotein including TfR1 and TfR2, is the major protein receptor for iron metabolism in vivo (Fernandes et al., 2021). TfR is expressed in both normal and cancer tissues. However, studies have confirmed that the expression rate of TfR in cancer cells is nearly 100 times higher than that in normal cells. Cancer cells grow rapidly and their demand for iron is greatly increased during DNA synthesis, differentiation and regeneration. Therefore, TfR expression is increased in cancer cells in order to adapt to the increased iron requirement and maintain rapid cell division (Candelaria et al., 2021; Guo et al., 2021).
Based on this active transport capability, transferrin NPs can be used for tumor diagnosis and targeted delivery of therapeutic drugs. Goswami et al. designed Transferrin -templated copper nanoclusters-doxorubicin NPs for bioimaging and targeted drug delivery (Goswami et al., 2018). In addition, transferrin can also be used alone as a ligand to provide active tumor targeting capabilities of drug delivery systems. Research by Wei et al.showed that doxorubicin -loaded Transferrin-binding peptide CGGGHKYLRW significantly enhanced the antitumor efficacy in mice bearing HCT-116 tumors compared to polymersomes without transferrin binding (Wei et al., 2020).
Ferritin, found in most living cells, is the major iron storage protein in organisms. At present, various sources of ferritin have been used in biological nanocarriers, including human ferritin (HFt), horse spleen ferritin (HoSF), Archaeoglobus fulgidus ferritin (AfFtn), Pyrococcus furiosus ferritin (PfFt), and rat heavy-chain ferritin (Zhang P. et al., 2021; Zafar et al., 2021). HFt is a naturally formed spherical hollow nanocages with an outer diameter of 12 nm and an inner diameter of 8 nm, which is composed of 24 subunits of two types: heavy chain ferritin (HFn) and light chain ferritin (LFn). The inner cavity of each ferritin can store up to 4,500 Fe3+ atoms (Chakraborti and Chakrabarti, 2019). At the junction of these subunits, there are 8 hydrophilic channels and 6 hydrophobic channels, wherein the hydrophobic channel has three-fold symmetry and the hydrophilic channel has four-fold symmetry. In particular, hydrophilic channels are flexible enough to allow larger-sized molecules to penetrate into the ferritin cavity (Zhao et al., 2016; Tesarova et al., 2020). More importantly, HFn can selectively bind to tumor cells via TfR1 mediated specific targeting followed by rapid internalization in vitro and in vivo. Therefore, HFn can selectively deliver therapeutic drugs into tumors (Zang et al., 2017; Cheng et al., 2020). The unique nanocage structure and inherent tumor-targeting properties of ferritin make it a highly valuable biological nanocarrier. However, despite the above advantages, the low purification efficiency of natural protein brings difficulties to its practical application, and the recombinant ferritin constructed in various ways makes up for the above shortcomings (Veroniaina et al., 2021). According to the characteristics of ferritin nanocages, metal-based chemotherapeutic drugs such as carboplatin and cisplatin are the first to achieve effective precipitation in the cavity of iron nanocages. Subsequently, ferritin nanocages have also been used to load other non-metallic chemotherapeutic drugs such as doxorubicin (Song et al., 2021). Here we list the applications of ferritin in the delivery of chemotherapeutic drugs in Table 3.
Lipoproteins are mainly responsible for the transport of cholesterol and various lipids in human blood vessels. According to their different densities, human plasma lipoproteins can be divided into chylomicrons, very low density lipoproteins (VLDL), and low density lipoproteins (LDL). and high-density lipoprotein (HDL) (Salter and Brindley, 1988). Lipoproteins are spherical biological macromolecules. Each lipoprotein molecule consists of a non-polar or hydrophobic core composed of cholesterol or triglycerides, and an outer shell of phospholipids and apoproteins, whose structure is a natural NPs (Orlova et al., 1999). Based on structural features and size advantages (less than 25 nm), LDL and HDL are commonly used to deliver chemotherapeutic drug.
LDL is a kind of spherical particles with a diameter of 19–25 nm. Its core is 1,500 cholesteryl esters wrapped by an outer layer of 800 phospholipids and 500 unesterified cholesterol molecules. The hydrophilic head of the phospholipid molecules is exposed outside, making the LDL is soluble in the blood (Hevonoja et al., 2000; Sherman et al., 2003). LDL is a very potential delivery vehicle, which has good biocompatibility as a biological macromolecule. Another very important reason is that there is apolipoprotein B-100 (ApoB-100) with a relative molecular weight of 514 kDa in the outermost layer of LDL, which can be recognized by and bind to LDL receptors with high specificity. Moreover, studies have confirmed that LDL receptors are highly expressed on the surface of tumor cells, which provide the necessary lipid matrix for the synthesis of membrane systems for fast growing tumor cells (Ng et al., 2011; Harisa and Alanazi, 2014). As a delivery carrier, LDL can encapsulate various hydrophobic loads such as photosensitizers, radiolabels, and various chemotherapeutic drugs (Thaxton et al., 2016; Li et al., 2019; Di and Maiseyeu, 2021). Lo et al. (2002) conjugated doxorubicin to the ApoB protein of LDL, and the results showed that it significantly reduced the occurrence of adverse reactions. Meanwhile, LDL can also take advantage of the inherent targeting ability of LDL through the strategy of combining with independent nanocarriers. Such as Zhu et al. reported a new “binary polymer” low-density lipoprotein-N-succinyl chitosan-cystamine-urocanic acid with dual pH/redox sensitivity and targeting effect, which was synthesized for the co-delivery of breast cancer resistance protein small interfering RNA and paclitaxel, showing significant tumor targeting and effectively inhibited tumor growth (Zhu WJ. et al., 2017). In addition, natural LDL is derived from plasma separation, which is difficult to produce on a large scale, and there are more and more studies on recombinant/synthetic LDL (Zhu G. et al., 2017). For example, Li et al. (2019) constructed a pH-sensitive ApoB-100/oleic acid-doxorubicin/nanostructured lipid carrier NPs, similar to LDL, which showed a increased accumulation at tumor site, pH-dependent release of doxorubicin, and potent breast cancer inhibition.
HDL is an endogenous nanocarrier with a structure similar to LDL and a diameter of 8–13 nm (Mulder et al., 2018). Similarly, blood HDL cholesterol levels in cancer patients are also lower than in normal healthy people (Ganjali et al., 2021). Unlike LDL, which recognizes receptors and mediates endocytosis, HDL specifically recognizes scavenger receptor class B type 1 (SR-B1) and the main protein of HDL stays on the surface of the cell membrane, while its core lipophilic cholesteryl ester directly enters the cell cytoplasm (Krieger, 1999). Furthermore, the major apolipoproteins of HDL contain fewer amino acids than those of LDL, effectively avoiding the formation of large irreversible aggregates (Mulder et al., 2018). The above-mentioned points all provide a good basis and favorable conditions for HDL as a delivery vehicle. It is rare to use natural HDL as a delivery vehicle in existing reports, and recombinant HDL (rHDL) NPs can be used to deliver chemotherapeutic drugs. For example, Wang et al. constructed a doxorubicin -loaded rHDL NPs with high affinity for SR-B1 to treat liver cancer. The results confirm that doxorubicin can be efficiently delivered into cells. After SR-B1 was blocked with antibodies, the delivery efficiency was significantly reduced, which well confirmed that the drug delivery efficiency was dependent on the active targeting of SR-B1 expressed in tumors by apolipoprotein A-1 contained in rHDL NPs (Wang et al., 2014). In addition, rHDL can also be used as a co-delivery carrier for the combination therapy of multiple chemotherapeutic drugs, or the combination therapy of chemotherapy and immunotherapy (Iqbal et al., 2021; Mei et al., 2021). Such as Rui et al. (2017) developed a developed a rHDL NPs for paclitaxel and doxorubicin, which were remarkably effective in increasing the ratiometric accumulation of drugs in cancer cells and enhancing antitumor response at synergistic drug ratios. In particular, they exhibited more efficacious anticancer effects in an in vitro cytotoxicity evaluation and in a xenograft tumor model of hepatoma compared with free drug cocktail solutions. Scheetz et al. (2020) co-loaded docetaxel and cholesterol-modified Toll-like receptor 9 agonist CpG oligonucleotides in synthetic HDL to prepare a nanoplatform for combined chemotherapy and immunotherapy. Compared with chemotherapy alone, it can be used for significant Improve survival outcomes.
Gene Therapy Nanomedicine
In recent years, with the increasing maturity of gene manipulation technologies such as gene silencing and gene editing, scientists have begun to treat various diseases by site-specific up-regulation or down-regulation of target genes, and have achieved certain progress and widespread attention, especially in cancer therapy. The drugs used in gene therapy are nucleic acid therapeutics with lower cytotoxicity, which show significantly fewer adverse reactions and better therapeutic effects compared with conventional treatments such as chemotherapy (Gutierrez et al., 1992). However, gene therapy also faces certain difficulties. For example, commonly used gene therapy agents are not easily taken up by cells and have poor stability during circulation in vivo; traditional viral vectors (lentivirus, adenovirus, adeno-associated virus, etc.) are limited in application due to safety concerns such as insertional mutagenesis and immunogenicity. NDSs solve the above problems well, showing low toxicity and immunogenicity, high payload capacity, sustained and controlled release characteristics. Commonly used gene therapy strategies include gene enhancement therapy and gene suppression therapy, in which the application value of nanomedicine will be described here (Figure 1B) (Rui et al., 2019).
Gene Enhancement Therapy
Gene enhancement therapy generally refers to “expressing a certain gene” or “expressing a certain protein” by introducing a plasmid or mRNA. Tumor suppressor genes can inhibit cell proliferation when activated or overexpressed. Overexpression of one or more tumor suppressor genes can effectively inhibit the growth and progression of tumors, among which protein 53 (p53)gene and phosphatase and tensin homolog (PTEN)gene are the most classical and the most deeply studied (Lee and Muller, 2010; Álvarez-Garcia et al., 2019; Lacroix et al., 2020). Both mRNA and plasmid can achieve protein expression, but the way via mRNA quickly and without mutation, integration or other adverse events, which is safer and more efficient (Akeno et al., 2015; Que et al., 2018; Sobhani et al., 2021). However, RNA molecules are unstable and impermeable to membranes, requiring rational design of delivery systems. Kong et al. designed a redox-responsive NPs platform for efficient delivery of mRNA encoding p53, which delayed the growth of hepatoma and non-small cell lung cancer cells by inducing cell cycle arrest and apoptosis (Kong et al., 2019). Islam et al. reintroduced PTEN mRNA into PTEN-null prostate cancer cells through polymer-lipid hybrid NPs coated with polyethylene glycol shell, which significantly inhibited tumor growth (Islam et al., 2018). In addition, the suicide gene therapy systems are also commonly used for gene enhancement, such as herpes simplex virus thymidine kinase (HSV-TK), of which TK gene is a drug-susceptibility gene. After tumor cells were transfected with this gene, they were sensitized and killed by the nontoxic prodrugs glycoxyguanosine or acyclovir (Zhao et al., 2014). A study reported that in vivo delivery of the TK-p53- nitroreductase triple therapeutic gene by poly (D,L-lactic-co-glycolic acid)-poly (ethylene glycol)-Polyethylenimine NPs functionalized with SP94 peptide (a peptide that targets hepatocytes) restored p53 function and enhanced cancer cells’ response to the prodrug ganximation glycoxyguanosine and CB 1954 (Sukumar et al., 2020). Due to the negative charge of mRNA, most of the NPs currently used to deliver mRNA drugs contain a cationic gradient, which can form stable complexes with mRNA to achieve high loading rates, such as ionizable lipid NPs (Ding et al., 2021), polymer-lipid hybrids NPs (Islam et al., 2018), and biological nanostructures with higher biocompatibility (Li et al., 2017; Forterre et al., 2020), etc.
Gene Suppression Therapy
Gene suppression can also treat cancer by silencing specific genes that produce abnormal or harmful proteins, such as small interfering RNA (siRNA) therapy. Several in vitro and in vivo studies have confirmed that siRNA-mediated silencing can significantly inhibit abnormal cancer cell proliferation (Shi et al., 2019; Han et al., 2021; Krishn et al., 2022). In addition, siRNA can sensitize drug-resistant cancer cells, showing great promise in enhancing chemotherapy (Shen et al., 2020). An ideal delivery system should protect siRNA from degradation by nucleases, as well as deliver and release it into the cytoplasm of targeted tumor cells without adverse effects. At present, the research on nanocarriers for siRNA delivery has been relatively mature, including lipid nanocarriers, polymer NPs, dendrimers, inorganic NPs, etc (Babu et al., 2017; Subhan and Torchilin, 2019). In addition, the aforementioned biological NPs can also deliver siRNA with high loading and high biocompatibility. Wang et al. constructed a DNA nanodevice using DNA origami technology to co-deliver siRNA and the doxorubicin (Nanodevice-siBcl2-si P-glycoprotein- doxorubicin), which induced potent cytotoxicity and tumor growth inhibition with no observable systemic toxicity (Wang X. et al., 2021).
CRISPR/Cas9 gene editing technology, another cancer gene-suppression therapy, has the potential to permanently destroy tumor survival genes, which overcomes the repeated dosing limitations of traditional cancer therapy and improves the therapeutic effect (Rafii et al., 2022). CRISPR/Cas9 consists of two parts: Cas9, a nuclease that cuts DNA at a target site, and a single guide RNA (sgRNA) that directs Cas9 to cut at a specific or desired site in DNA. Since the CRISPR/Cas9 complex requires manipulation of the nuclear genome, its components need to be translocated into the nucleus (Zhan et al., 2019; Zhang S. et al., 2021). Therefore, it is necessary to overcome the barriers of tissue and cell membranes to effectively deliver CRISPR/Cas9 to the target site, facing considerable challenges. In recent years, nanomaterials have gradually shown unique advantages in gene delivery. Currently developed CRISPR/Cas9 NDSs include cationic liposomes (Yin et al., 2020), lipid NPs (Rosenblum et al., 2020), cationic polymers (Zhang Y. et al., 2019), vesicles (Horodecka and Düchler, 2021), and gold NPs (Tao et al., 2021). In order to better reduce the off-target effects, researchers have developed a stimulus-based intelligent NDSs. Intelligent NPs can be based on endogenous signals (including pH, redox and ATP) and exogenous signals (including radiation, magnetic ultrasound), to control or regulate the delivery of CRISPR/Cas9 to specific cells. For example, Wang et al. designed a multifunctional NPs modified with pH-sensitive epidermal growth factor receptor targeting and nuclear guide peptides to efficiently deliver CRISPR/Cas9 and epirubicin to the human tongue squamous cell carcinoma SAS cells and SAS tumor mice, providing a pH-responsive co-delivery platform for chemotherapy and CRISPR/Cas (Wang Z. et al., 2021). Another study reported near-infrared light-responsive nanocarriers of CRISPR-Cas9 to inhibit tumor cell proliferation in vitro and in vivo through near-infrared light-activated gene editing (Pan et al., 2019). DNA-based NPs can load Cas9/sgRNA complexes by sequence hybridization. Shi et al. designed a miR-21 (overexpressed in tumor cell)-responsive Cas9/sgRNA ribonucleoprotein delivery system based on DNA NFs, which could significantly improve genome editing efficiency and make it possible to control the expression of endogenous genes in a cell-type-specific manner through specific endogenous or exogenous miRNAs (Shi et al., 2020).
Immunotherapeutic Nanomedicine
In recent years, immunotherapy has developed rapidly and gradually matured, and its emergence has revolutionized the treatment standard and treatment concept of cancer (Abdelbaky et al., 2021; Aktar et al., 2022). Radiotherapy and chemotherapy of traditional treatment methods generally use toxic drugs or radiation to directly ablate cancer cells. However, the target of tumor immunotherapy is mainly immune cells, which can activate the body’s anti-tumor immune response to kill tumor cells by inhibiting negative immune regulators and enhancing the ability of immune cells to recognize tumor cell surface antigens (van den Bulk et al., 2018; Zhong et al., 2020). In the early stage of tumor immunotherapy, the main method is to directly attack tumor cells with cytokines produced by immune cells. With the gradual deepening of cancer immunotherapy research, immune checkpoint inhibitors, tumor vaccine immunization and chimeric antigen receptor T (CAR-T) cell therapy have emerged, and they have become the main force in immunotherapy (Sahin and Türeci, 2018; Ma et al., 2019; Galluzzi et al., 2020; Holstein and Lunning, 2020; Raza et al., 2021) With the deepening of research, the value of nanomedicine in tumor immunotherapy is constantly emerging (Figure 1C).
Immune Checkpoint Blockade Therapy
Inhibitory immune checkpoints can suppress the body’s immune response and prevent the occurrence of autoimmunity. Tumor cells can suppress the body’s immune response, thereby evading clearance by the body’s immune system via expressing inhibitory immune checkpoint molecules that interact with T cells (Jhunjhunwala et al., 2021). Currently widely studied immune checkpoints are cytotoxic-T-lymphocyte-associated protein 4 (CTLA-4), program death 1 (PD-1) and program death-ligand 1 (PD-L1), according to which monoclonal antibodies (mAbs) drugs are designed such as ipilimumab (CTLA-4 inhibitor), pembrolizumab (PD-1 inhibitor), atezolizumab (PD-L1 inhibitors) have been approved by the FDA for marketing (Vaddepally et al., 2020). Nanomedicine is being adapted in various ways to improve immune checkpoint inhibitors (ICIs), increasing the effectiveness and surpassing the limitations. MAbs are difficult to penetrate the blood-brain barrier, and NPs -mediated ICIs mAbs are an effective way to solve this problem (Diesendruck and Benhar, 2017). Galstyan et al. covalently attached ICIs (anti-CTLA-4 and/or anti-PD-1) to poly-β-l-malic acid biopolymer scaffolds, and such nanoscale immunoconjugates allow ICIs mAbs to cross the blood-brain barrier to the tumor environment and modulate immune responses. In particular, the use of nanoscale immunoconjugates has shown promising antitumor activity in the treatment of glioblastoma (Galstyan et al., 2019). Moreover, NDSs have also shown their advantages in reducing the dosage of ICIs or controlling immune-related adverse events (irAIEs) Meir et al., reported that αPDL1-conjugated gold NPs effectively prevented tumor growth at a dose reduced to 1/5 the clinical standard of care dose (Meir et al., 2017). Shen et al. coated PD-L1-overexpressing mesenchymal stem cells plasma membranes on polylactic-co-glycolic acid NPs to design immunosuppressive NPs, managing and reducing irAIEs (Shen et al., 2021). Furthermore, nanocarriers can also be designed as smart platforms for controlled drug release in response to different stimuli present in the tumor microenvironment, which is expected to further enhance the therapeutic efficacy of nanoformulations. For example, researchers have developed a class of liposomes that are dual-responsive to pH and matrix metalloproteinases in combination with PD-L1 inhibitor conjugates and low-dose chemotherapy doxorubicin. In an in vivo mouse B16F10 melanoma model, the synergistic effect of chemotherapeutic agents and ICIs enabled dual-responsive liposomes to achieve an optimal tumor suppression efficiency of 78.7% (Liu et al., 2019). Besides, ICIs do not elicit adequate responses in the vast majority of patients with poorly immunogenic tumors due to targeting only major inhibitory axes. Therefore, combining ICIs with nanotechnology-driven immunostimulatory treatments (e.g., nanochemicals, light, and thermal therapy) may help to locally break immune tolerance and enhance systemic antitumor immunity, thereby expanding the availability of proportion of cancer patients benefiting from treatment. Based on the above, the role of nanomaterials in immunotherapy has gone beyond the concept of adjuvant or carrier, which is an effective means to improve the efficacy of ICIs and reduce their toxicity through rational design. At the same time, the dosing cycle and interval time, possible off-target potential and other aspects should be improved to obtain the best solution (Cremolini et al., 2021).
Cancer Vaccines
Cancer vaccines kill tumor cells without damaging healthy cells by activating the body’s immune system, and they can trigger immune memory to provide long-term protection against tumor recurrence. As a potential drug development concept, cancer vaccines are extremely valuable whether they are used alone or in combination with other immunotherapies (Igarashi and Sasada, 2020; Saxena et al., 2021). With the deepening of research, the advantages of applying nanomedicine in cancer vaccines have gradually emerged (Liu J. et al., 2020). Cancer vaccines are typically combinations of immunogenic components (eg, neoantigens and adjuvants) that are delivered to antigen-presenting cells in peripheral lymphoid tissue. First, encapsulating immunogenic components in nanocarriers can prevent antigen degradation and effectively improve antigen stability (Zhang Z. et al., 2019). Second, nanovaccines co-encapsulate and co-deliver antigens and adjuvants, which can effectively enhance the immunogenicity and therapeutic efficacy of vaccines (Zhu WJ. et al., 2017). Heo and Lim et al. developed a poly (lactic-co-glycolic acid) NPs loaded with ovalbumin for the activation of DCs through the toll-like receptor 7, which proved that the nanovaccine loaded with multivalent antigens and adjuvants can effectively reduce tumor volume (Heo and Lim, 2014). Further, nanovaccine can achieve efficient delivery to immune organs (lymph nodes, spleen). Through rational design of physical properties (such as size, colloidal stability, electrostatic interactions, deformability) or chemical properties (such as light, pH, and enzyme responsiveness), nanovaccines are able to deliver more antigens from injection sites or tumors to lymph nodes, or delivered from the blood to the spleen (Evans et al., 2018; Musetti and Huang, 2018; Chen et al., 2020; Gupta et al., 2021). In particular, nanovaccines further modified by targeting ligands can also be actively targeted and delivered to specific subregions of immune cells (Cai et al., 2021). For example, a click chemistry-based active lymphatic accumulation system was developed to enhance the delivery of antigens and adjuvants to the lymphatic subcapsular sinus (Qin et al., 2021). Ultimately, NPs can enhance immune responses through sustained or controlled release capabilities. For example, Chen et al. (2018) showed that a single injection of clay NPs sustained the release of immunogenic agents, which significantly enhanced the immune response in regional lymph nodes for up to 35 days.
CAR-T Cell Therapy
One of the strategies for immune evasion of tumor cells is to reduce the expression of their surface antigens, so that T cells cannot be activated in a human leukocyte antigen-dependent manner, thereby evading the attack of the immune system (Pham et al., 2018). CAR-T works by modifying a patient’s own T cells to more effectively recognize and kill tumor cells (Huang et al., 2020). Initially, the application of nanomedicine to CAR-T therapy was mainly to replace viral vectors for in vitro genetic modification of T cells to reduce costs and improve safety (Olden et al., 2018; Billingsley et al., 2020). However, in vitro CAR-T cell programming is complicated and expensive, and one solution is to program T cells in vivo. Nanomedicines were shown to directly construct chimeric antigen receptors in situ on circulating T cells without ex vivo manipulation in mouse models. Smith et al. designed a polymer NPs carrying a chimeric antigen receptor (CAR)-encoding plasmid and injected leukemia-targeting CAR genes can be efficiently introduced into T cell nuclei, followed by efficient leukemia regression in mice, which is comparable to ex vivo programmed CAR-T cells (Smith et al., 2017). Subsequently, this team reported an injectable nanocarrier that deliverd in vitro transcribed CAR or T cell receptors mRNA for transient reprogramming of circulating T cells to recognize disease-associated antigens (Parayath et al., 2020). Another important advantage of nanomedicine in this field is the safe and effective enhancement of T cell therapy. Tang et al. (2018) designed a T cell receptors signaling-responsive protein nanogel to co-deposit immunostimulatory cytokines, such as interleukin-15 agonists, onto the surface of CAR-T cells, which significantly extended the therapeutic window and improved tumor clearance in CAR-T cell therapy against solid tumors.
Perspectives and Future Directions
In recent years, the concept, method and pattern of tumor treatment are constantly changing, which provides a broad space and prospect for the application of nanomedicine. It is the application of intelligent NDSs for tumor chemotherapy, gene therapy and immunotherapy to solve the problem of drug (chemotherapy, biological drug) delivery, optimize its delivery efficiency, and achieve targeted, precise and controllable delivery to a certain degree. However, how to translate preclinically studied antitumor nanomedicines into clinically feasible therapeutics still faces several key challenges. For example: 1) how to optimize patient population stratification in clinical trials; 2) how to optimize the dosing regimen of nanomedicines in combination therapy; 3) how to ensure high quality and reproducibility for industrialized production of nanomedicines, etc. Expectantly, with the deepening of nanotechnology research, the combination of molecular-level scientific design and precise control of process engineering is expected to overcome the core technology of NDSs research and development, thereby opening a new situation for NDSs.
Author Contributions
SH, ZC, and YL reviewed the literature and drafted the article. MH, ZWC, and FL finalized the paper and provided suggestions to improve it. SH, MMFAB and ZC drew the figure. MMFAB provided suggestions to improve the article. All authors participated in designing the concept of this manuscript. All authors contributed to the article and approved the submitted version.
Funding
This work was supported by the Ningbo Natural Science Foundation (2019A610311); by Scientific Research Fund of Zhejiang Provincial Education Department (Y202045025); by the Zhejiang Province Public Welfare Technology Application Research Project of China (No. LGF18H090015).
Conflict of Interest
The authors declare that the research was conducted in the absence of any commercial or financial relationships that could be construed as a potential conflict of interest.
Publisher’s Note
All claims expressed in this article are solely those of the authors and do not necessarily represent those of their affiliated organizations, or those of the publisher, the editors and the reviewers. Any product that may be evaluated in this article, or claim that may be made by its manufacturer, is not guaranteed or endorsed by the publisher.
References
Abdelbaky, S. B., Ibrahim, M. T., Samy, H., Mohamed, M., Mohamed, H., Mustafa, M., et al. (2021). Cancer Immunotherapy from Biology to Nanomedicine. J. Control. Release 336, 410–432. doi:10.1016/j.jconrel.2021.06.025
Ahn, S. Y., Liu, J., Vellampatti, S., Wu, Y., and Um, S. H. (2020). DNA Transformations for Diagnosis and Therapy. Adv. Funct. Mat. 31, 2008279. doi:10.1002/adfm.202008279
Akeno, N., Miller, A. L., Ma, X., and Wikenheiser-Brokamp, K. A. (2015). p53 Suppresses Carcinoma Progression by Inhibiting mTOR Pathway Activation. Oncogene 34, 589–599. doi:10.1038/onc.2013.589
Akgöl, S., Ulucan‐Karnak, F., Kuru, C. İ., and Kuşat, K. (2021). The Usage of Composite Nanomaterials in Biomedical Engineering Applications. Biotech. Bioeng. 118, 2906–2922. doi:10.1002/bit.27843
Aktar, N., Yueting, C., Abbas, M., Zafar, H., Paiva-Santos, A. C., Zhang, Q., et al. (2022). Understanding of Immune Escape Mechanisms and Advances in Cancer Immunotherapy. J. Oncol. 2022, 8901326. doi:10.1155/2022/8901326
Álvarez-Garcia, V., Tawil, Y., Wise, H. M., and Leslie, N. R. (2019). Mechanisms of PTEN Loss in Cancer: It's All about Diversity. Seminars Cancer Biol. 59, 66–79. doi:10.1016/j.semcancer.2019.02.001
An, F.-F., and Zhang, X.-H. (2017). Strategies for Preparing Albumin-Based Nanoparticles for Multifunctional Bioimaging and Drug Delivery. Theranostics 7, 3667–3689. doi:10.7150/thno.19365
Babu, A., Munshi, A., and Ramesh, R. (2017). Combinatorial Therapeutic Approaches with RNAi and Anticancer Drugs Using Nanodrug Delivery Systems. Drug Dev. Industrial Pharm. 43, 1391–1401. doi:10.1080/03639045.2017.1313861
Bertrand, N., Wu, J., Xu, X., Kamaly, N., and Farokhzad, O. C. (2014). Cancer Nanotechnology: the Impact of Passive and Active Targeting in the Era of Modern Cancer Biology. Adv. Drug Deliv. Rev. 66, 2–25. doi:10.1016/j.addr.2013.11.009
Bhushan, B., Khanadeev, V., Khlebtsov, B., Khlebtsov, N., and Gopinath, P. (2017). Impact of Albumin Based Approaches in Nanomedicine: Imaging, Targeting and Drug Delivery. Adv. Colloid Interface Sci. 246, 13–39. doi:10.1016/j.cis.2017.06.012
Billingsley, M. M., Singh, N., Ravikumar, P., Zhang, R., June, C. H., and Mitchell, M. J. (2020). Ionizable Lipid Nanoparticle-Mediated mRNA Delivery for Human CAR T Cell Engineering. Nano Lett. 20, 1578–1589. doi:10.1021/acs.nanolett.9b04246
Cai, T., Liu, H., Zhang, S., Hu, J., and Zhang, L. (2021). Delivery of Nanovaccine Towards Lymphoid Organs: Recent Strategies in Enhancing Cancer Immunotherapy. J. Nanobiotechnol. 19, 389. doi:10.1186/s12951-021-01146-2
Candelaria, P. V., Leoh, L. S., Penichet, M. L., and Daniels-Wells, T. R. (2021). Antibodies Targeting the Transferrin Receptor 1 (TfR1) as Direct Anti-cancer Agents. Front. Immunol. 12, 607692. doi:10.3389/fimmu.2021.607692
Cao, J., Huang, D., and Peppas, N. A. (2020). Advanced Engineered Nanoparticulate Platforms to Address Key Biological Barriers for Delivering Chemotherapeutic Agents to Target Sites. Adv. Drug Deliv. Rev. 167, 170–188. doi:10.1016/j.addr.2020.06.030
Carvalho, C., Santos, R., Cardoso, S., Correia, S., Oliveira, P., Santos, M., et al. (2009). Doxorubicin: The Good, the Bad and the Ugly Effect. Cmc 16, 3267–3285. doi:10.2174/092986709788803312
Chakraborti, S., and Chakrabarti, P. (2019). Self-Assembly of Ferritin: Structure, Biological Function and Potential Applications in Nanotechnology. 313–329. doi:10.1007/978-981-13-9791-2_10
Chang, M., Yang, C.-S., and Huang, D.-M. (2011). Aptamer-Conjugated DNA Icosahedral Nanoparticles as a Carrier of Doxorubicin for Cancer Therapy. ACS Nano 5, 6156–6163. doi:10.1021/nn200693a
Chen, W., Zuo, H., Li, B., Duan, C., Rolfe, B., Zhang, B., et al. (2018). Clay Nanoparticles Elicit Long-Term Immune Responses by Forming Biodegradable Depots for Sustained Antigen Stimulation. Small 14, e1704465. doi:10.1002/smll.201704465
Chen, Y., De Koker, S., and De Geest, B. G. (2020). Engineering Strategies for Lymph Node Targeted Immune Activation. Acc. Chem. Res. 53, 2055–2067. doi:10.1021/acs.accounts.0c00260
Cheng, X., Fan, K., Wang, L., Ying, X., Sanders, A. J., Guo, T., et al. (2020). TfR1 Binding with H-Ferritin Nanocarrier Achieves Prognostic Diagnosis and Enhances the Therapeutic Efficacy in Clinical Gastric Cancer. Cell Death Dis. 11, 92. doi:10.1038/s41419-020-2272-z
Collins, M., and Thrasher, A. (2015). Gene Therapy: Progress and Predictions. Proc. R. Soc. B 282, 20143003. doi:10.1098/rspb.2014.3003
Cremolini, C., Vitale, E., Rastaldo, R., and Giachino, C. (2021). Advanced Nanotechnology for Enhancing Immune Checkpoint Blockade Therapy. Nanomater. (Basel) 11 (3), 661. doi:10.3390/nano11030661
Dai, Z., Leung, H. M., and Lo, P. K. (2017). Stimuli-Responsive Self-Assembled DNA Nanomaterials for Biomedical Applications. Small 13 (7). doi:10.1002/smll.201602881
Di, L., and Maiseyeu, A. (2021). Low-Density Lipoprotein Nanomedicines: Mechanisms of Targeting, Biology, and Theranostic Potential. Drug Deliv. 28, 408–421. doi:10.1080/10717544.2021.1886199
Diesendruck, Y., and Benhar, I. (2017). Novel Immune Check Point Inhibiting Antibodies in Cancer Therapy-Opportunities and Challenges. Drug Resist. Updat. 30, 39–47. doi:10.1016/j.drup.2017.02.001
Ding, F., Zhang, H., Cui, J., Li, Q., and Yang, C. (2021). Boosting Ionizable Lipid Nanoparticle-Mediated In Vivo mRNA Delivery Through Optimization of Lipid Amine-Head Groups. Biomater. Sci. 9, 7534–7546. doi:10.1039/d1bm00866h
Elsayed, M. E., Sharif, M. U., and Stack, A. G. (2016). Transferrin Saturation: A Body Iron Biomarker. Adv. Clin. Chem. 75, 71–97. doi:10.1016/bs.acc.2016.03.002
Evans, C. G., and Winfree, E. (2017). Physical Principles for DNA Tile Self-Assembly. Chem. Soc. Rev. 46, 3808–3829. doi:10.1039/c6cs00745g
Evans, E. R., Bugga, P., Asthana, V., and Drezek, R. (2018). Metallic Nanoparticles for Cancer Immunotherapy. Mater. Today 21, 673–685. doi:10.1016/j.mattod.2017.11.022
Falvo, E., Malagrinò, F., Arcovito, A., Fazi, F., Colotti, G., Tremante, E., et al. (2018). The Presence of Glutamate Residues on the PAS Sequence of the Stimuli-Sensitive Nano-Ferritin Improves In Vivo Biodistribution and Mitoxantrone Encapsulation Homogeneity. J. Control. Release 275, 177–185. doi:10.1016/j.jconrel.2018.02.025
Falvo, E., Tremante, E., Fraioli, R., Leonetti, C., Zamparelli, C., Boffi, A., et al. (2013). Antibody-Drug Conjugates: Targeting Melanoma with Cisplatin Encapsulated in Protein-Cage Nanoparticles Based on Human Ferritin. Nanoscale 5, 12278–12285. doi:10.1039/c3nr04268e
Fernandes, M. A., Hanck-Silva, G., Baveloni, F. G., Oshiro Junior, J. A., de Lima, F. T., Eloy, J. O., et al. (2021). A Review of Properties, Delivery Systems and Analytical Methods for the Characterization of Monomeric Glycoprotein Transferrin. Crit. Rev. Anal. Chem. 51, 399–410.doi:10.1080/10408347.2020.1743639
Forterre, A. V., Wang, J.-H., Delcayre, A., Kim, K., Green, C., Pegram, M. D., et al. (2020). Extracellular Vesicle-Mediated In Vitro Transcribed mRNA Delivery for Treatment of HER2+ Breast Cancer Xenografts in Mice by Prodrug CB1954 Without General Toxicity. Mol. Cancer Ther. 19, 858–867. doi:10.1158/1535-7163.mct-19-0928
Galluzzi, L., Humeau, J., Buqué, A., Zitvogel, L., and Kroemer, G. (2020). Immunostimulation with Chemotherapy in the Era of Immune Checkpoint Inhibitors. Nat. Rev. Clin. Oncol. 17, 725–741. doi:10.1038/s41571-020-0413-z
Galstyan, A., Markman, J. L., Shatalova, E. S., Chiechi, A., Korman, A. J., Patil, R., et al. (2019). Blood-Brain Barrier Permeable Nano Immunoconjugates Induce Local Immune Responses for Glioma Therapy. Nat. Commun. 10, 3850. doi:10.1038/s41467-019-11719-3
Ganjali, S., Banach, M., Pirro, M., Fras, Z., and Sahebkar, A. (2021). HDL and Cancer - Causality Still Needs to Be Confirmed? Update 2020. Seminars Cancer Biol. 73, 169–177. doi:10.1016/j.semcancer.2020.10.007
Goswami, U., Dutta, A., Raza, A., Kandimalla, R., Kalita, S., Ghosh, S. S., et al. (2018). Transferrin-Copper Nanocluster-Doxorubicin Nanoparticles as Targeted Theranostic Cancer Nanodrug. ACS Appl. Mat. Interfaces 10, 3282–3294. doi:10.1021/acsami.7b15165
Gote, V., Nookala, A. R., Bolla, P. K., and Pal, D. (2021). Drug Resistance in Metastatic Breast Cancer: Tumor Targeted Nanomedicine to the Rescue. Int. J. Mol. Sci. 22 (9), 4673. doi:10.3390/ijms22094673
Gou, Y., Miao, D., Zhou, M., Wang, L., Zhou, H., and Su, G. (2018). Bio-Inspired Protein-Based Nanoformulations for Cancer Theranostics. Front. Pharmacol. 9, 421. doi:10.3389/fphar.2018.00421
Gu, C., Zhang, T., Lv, C., Liu, Y., Wang, Y., and Zhao, G. (2020). His-Mediated Reversible Self-Assembly of Ferritin Nanocages Through Two Different Switches for Encapsulation of Cargo Molecules. ACS Nano 12, 17080–17090. doi:10.1021/acsnano.0c06670
Guan, C., Zhu, X., and Feng, C. (2021). DNA Nanodevice-Based Drug Delivery Systems. Biomolecules 11 (12), 1855. doi:10.3390/biom11121855
Guo, Z., Zhang, Y., Fu, M., Zhao, L., Wang, Z., Xu, Z., et al. (2021). The Transferrin Receptor-Directed CAR for the Therapy of Hematologic Malignancies. Front. Immunol. 12, 652924. doi:10.3389/fimmu.2021.652924
Gupta, J., Safdari, H. A., and Hoque, M. (2021). Nanoparticle Mediated Cancer Immunotherapy. Seminars Cancer Biol. 69, 307–324. doi:10.1016/j.semcancer.2020.03.015
Gutierrez, A. A., Lemoine, N. R., and Sikora, K. (1992). Gene Therapy for Cancer. Lancet 339, 715–721. doi:10.1016/0140-6736(92)90606-4
Halley, P. D., Lucas, C. R., Mcwilliams, E. M., Webber, M. J., Patton, R. A., Kural, C., et al. (2016). Daunorubicin-Loaded DNA Origami Nanostructures Circumvent Drug-Resistance Mechanisms in a Leukemia Model. Small 12, 308–320. doi:10.1002/smll.201502118
Han, Q., Xie, Q. R., Li, F., Cheng, Y., Wu, T., Zhang, Y., et al. (2021). Targeted Inhibition of SIRT6 via Engineered Exosomes Impairs Tumorigenesis and Metastasis in Prostate Cancer. Theranostics 11, 6526–6541. doi:10.7150/thno.53886
Harisa, G. I., and Alanazi, F. K. (2014). Low Density Lipoprotein Bionanoparticles: From Cholesterol Transport to Delivery of Anti-Cancer Drugs. Saudi Pharm. J. 22, 504–515. doi:10.1016/j.jsps.2013.12.015
Heo, M. B., and Lim, Y. T. (2014). Programmed Nanoparticles for Combined Immunomodulation, Antigen Presentation and Tracking of Immunotherapeutic Cells. Biomaterials 35 (1), 590–600. doi:10.1016/j.biomaterials.2013.10.009
Hevonoja, T., Pentikäinen, M. O., Hyvönen, M. T., Kovanen, P. T., and Ala-Korpela, M. (2000). Structure of Low Density Lipoprotein (LDL) Particles: Basis for Understanding Molecular Changes in Modified LDL. Biochim. Biophys. Acta 1488, 189–210. doi:10.1016/s1388-1981(00)00123-2
Holstein, S. A., and Lunning, M. A. (2020). CAR T‐Cell Therapy in Hematologic Malignancies: A Voyage in Progress. Clin. Pharmacol. Ther. 107, 112–122. doi:10.1002/cpt.1674
Hoogenboezem, E. N., and Duvall, C. L. (2018). Harnessing Albumin as a Carrier for Cancer Therapies. Adv. Drug Deliv. Rev. 130, 73–89. doi:10.1016/j.addr.2018.07.011
Horodecka, K., and Düchler, M. (2021). CRISPR/Cas9: Principle, Applications, and Delivery Through Extracellular Vesicles. Int. J. Mol. Sci. 22 (11), 6072. doi:10.3390/ijms22116072
Huang, R., Li, X., He, Y., Zhu, W., Gao, L., Liu, Y., et al. (2020). Recent Advances in CAR-T Cell Engineering. J. Hematol. Oncol. 13, 86. doi:10.1186/s13045-020-00910-5
Igarashi, Y., and Sasada, T. (2020). Cancer Vaccines: Toward the Next Breakthrough in Cancer Immunotherapy. J. Immunol. Res. 2020, 5825401. doi:10.1155/2020/5825401
Inoue, I., Chiba, M., Ito, K., Okamatsu, Y., Suga, Y., Kitahara, Y., et al. (2021). One-Step Construction of Ferritin Encapsulation Drugs for Cancer Chemotherapy. Nanoscale 13, 1875–1883. doi:10.1039/d0nr04019c
Iqbal, H., Yang, T., Li, T., Zhang, M., Ke, H., Ding, D., et al. (2021). Serum Protein-Based Nanoparticles for Cancer Diagnosis and Treatment. J. Control. Release 329, 997–1022. doi:10.1016/j.jconrel.2020.10.030
Islam, M. A., Xu, Y., Tao, W., Ubellacker, J. M., Lim, M., Aum, D., et al. (2018). Restoration of Tumour-Growth Suppression In Vivo via Systemic Nanoparticle-Mediated Delivery of PTEN mRNA. Nat. Biomed. Eng. 2, 850–864. doi:10.1038/s41551-018-0284-0
Jhunjhunwala, S., Hammer, C., and Delamarre, L. (2021). Antigen Presentation in Cancer: Insights into Tumour Immunogenicity and Immune Evasion. Nat. Rev. Cancer 21, 298–312. doi:10.1038/s41568-021-00339-z
Ji, J., Karna, D., and Mao, H. (2021). DNA Origami Nano-Mechanics. Chem. Soc. Rev. 50, 11966–11978. doi:10.1039/d1cs00250c
Jiang, B., Zhang, R., Zhang, J., Hou, Y., Chen, X., Zhou, M., et al. (2019a). GRP78-Targeted Ferritin Nanocaged Ultra-High Dose of Doxorubicin for Hepatocellular Carcinoma Therapy. Theranostics 9, 2167–2182. doi:10.7150/thno.30867
Jiang, Q., Zhao, S., Liu, J., Song, L., Wang, Z., and Ding, B. (2019b). Rationally Designed DNA-Based Nanocarriers. Adv. Drug Deliv. Rev. 147, 2–21. doi:10.1016/j.addr.2019.02.003
Jin, M.-Z., and Jin, W.-L. (2020). The Updated Landscape of Tumor Microenvironment and Drug Repurposing. Sig. Transduct. Target Ther. 5, 166. doi:10.1038/s41392-020-00280-x
Kilic, M. A., Ozlu, E., and Calis, S. (2012). A Novel Protein-Based Anticancer Drug Encapsulating Nanosphere: Apoferritin-Doxorubicin Complex. J. Biomed. Nanotechnol. 8, 508–514. doi:10.1166/jbn.2012.1406
Kinnear, C., Moore, T. L., Rodriguez-Lorenzo, L., Rothen-Rutishauser, B., and Petri-Fink, A. (2017). Form Follows Function: Nanoparticle Shape and its Implications for Nanomedicine. Chem. Rev. 117, 11476–11521. doi:10.1021/acs.chemrev.7b00194
Kong, N., Tao, W., Ling, X., Wang, J., Xiao, Y., Shi, S., et al. (2019). Synthetic mRNA Nanoparticle-Mediated Restoration of P53 Tumor Suppressor Sensitizes -deficient Cancers to mTOR Inhibition.
Krieger, M. (1999). Charting the Fate of the “Good Cholesterol”: Identification and Characterization of the High-Density Lipoprotein Receptor SR-BI. Annu. Rev. Biochem. 68, 523–558. doi:10.1146/annurev.biochem.68.1.523
Krishn, S. R., Garcia, V., Naranjo, N. M., Quaglia, F., Shields, C. D., Harris, M. A., et al. (2022). Small Extracellular Vesicle-Mediated ITGB6 siRNA Delivery Downregulates the αVβ6 Integrin and Inhibits Adhesion and Migration of Recipient Prostate Cancer Cells. Cancer Biol. Ther. 23, 173–185. doi:10.1080/15384047.2022.2030622
Lacroix, M., Riscal, R., Arena, G., Linares, L. K., and Le Cam, L. (2020). Metabolic Functions of the Tumor Suppressor P53: Implications in Normal Physiology, Metabolic Disorders, and Cancer. Mol. Metab. 33, 2–22. doi:10.1016/j.molmet.2019.10.002
Lau, K. L., and Sleiman, H. F. (2016). Minimalist Approach to Complexity: Templating the Assembly of DNA Tile Structures with Sequentially Grown Input Strands. ACS Nano 10, 6542–6551. doi:10.1021/acsnano.6b00134
Lee, E. Y. H. P., and Muller, W. J. (2010). Oncogenes and Tumor Suppressor Genes. Cold Spring Harb. Perspect. Biol. 2, a003236. doi:10.1101/cshperspect.a003236
Li, C., Li, H., and Jie, G. (2020). Click Chemistry Reaction-Triggered DNA Walker Amplification Coupled with Hyperbranched DNA Nanostructure for Versatile Fluorescence Detection and Drug Delivery to Cancer Cells. Microchim. Acta 187, 625. doi:10.1007/s00604-020-04580-5
Li, J., Wang, W., He, Y., Li, Y., Yan, E. Z., Zhang, K., et al. (2017). Structurally Programmed Assembly of Translation Initiation Nanoplex for Superior mRNA Delivery. ACS Nano 11, 2531–2544. doi:10.1021/acsnano.6b08447
Li, W., Fu, J., Ding, Y., Liu, D., Jia, N., Chen, D., et al. (2019). Low Density Lipoprotein-Inspired Nanostructured Lipid Nanoparticles Containing Pro-Doxorubicin to Enhance Tumor-Targeted Therapeutic Efficiency. Acta Biomater. 96, 456–467. doi:10.1016/j.actbio.2019.06.051
Li, Y., Raza, F., Liu, Y., Wei, Y., Rong, R., Zheng, M., et al. (2021). Clinical Progress and Advanced Research of Red Blood Cells Based Drug Delivery System. Biomaterials 279, 121202. doi:10.1016/j.biomaterials.2021.121202
Liang, M., Fan, K., Zhou, M., Duan, D., Zheng, J., Yang, D., et al. (2014). H-Ferritin-Nanocaged Doxorubicin Nanoparticles Specifically Target and Kill Tumors with a Single-Dose Injection. Proc. Natl. Acad. Sci. U.S.A. 111, 14900–14905. doi:10.1073/pnas.1407808111
Libutti, S. K. (2019). Recording 25 Years of Progress in Cancer Gene Therapy. Cancer Gene Ther. 26, 345–346. doi:10.1038/s41417-019-0121-y
Liu, J.-p., Wang, T.-t., Wang, D.-g., Dong, A.-j., Li, Y.-p., and Yu, H.-j. (2017). Smart Nanoparticles Improve Therapy for Drug-Resistant Tumors by Overcoming Pathophysiological Barriers. Acta Pharmacol. Sin. 38, 1–8. doi:10.1038/aps.2016.84
Liu, J., Miao, L., Sui, J., Hao, Y., and Huang, G. (2020a). Nanoparticle Cancer Vaccines: Design Considerations and Recent Advances. Asian J. Pharm. Sci. 15, 576–590. doi:10.1016/j.ajps.2019.10.006
Liu, J., Song, L., Liu, S., Zhao, S., Jiang, Q., and Ding, B. (2018a). A Tailored DNA Nanoplatform for Synergistic RNAi‐/Chemotherapy of Multidrug‐Resistant Tumors. Angew. Chem. Int. Ed. 57, 15486–15490. doi:10.1002/anie.201809452
Liu, M., Zhu, Y., Wu, T., Cheng, J., and Liu, Y. (2020b). Nanobody‐Ferritin Conjugate for Targeted Photodynamic Therapy. Chem. Eur. J. 26, 7442–7450. doi:10.1002/chem.202000075
Liu, W., Lin, Q., Fu, Y., Huang, S., Guo, C., Li, L., et al. (2020c). Target Delivering Paclitaxel by Ferritin Heavy Chain Nanocages for Glioma Treatment. J. Control. Release 323, 191–202. doi:10.1016/j.jconrel.2019.12.010
Liu, X., Wu, L., Wang, L., and Jiang, W. (2018b). A Dual-Targeting DNA Tetrahedron Nanocarrier for Breast Cancer Cell Imaging and Drug Delivery. Talanta 179, 356–363. doi:10.1016/j.talanta.2017.11.034
Liu, Y., Chen, X.-G., Yang, P.-P., Qiao, Z.-Y., and Wang, H. (2019). Tumor Microenvironmental pH and Enzyme Dual Responsive Polymer-Liposomes for Synergistic Treatment of Cancer Immuno-Chemotherapy. Biomacromolecules 20, 882–892. doi:10.1021/acs.biomac.8b01510
Liu, Y., Ran, Y., Ge, Y., Raza, F., Li, S., Zafar, H., et al. (2022). pH-Sensitive Peptide Hydrogels as a Combination Drug Delivery System for Cancer Treatment. Pharmaceutics 14, 652. doi:10.3390/pharmaceutics14030652
Lo, E. H. K., Ooi, V. E. L., and Fung, K. P. (2002). Circumvention of Multidrug Resistance and Reduction of Cardiotoxicity of Doxorubicin In Vivo by Coupling it with Low Density Lipoprotein. Life Sci. 72, 677–687. doi:10.1016/s0024-3205(02)02180-x
Lu, S., Zhao, F., Zhang, Q., and Chen, P. (2018). Therapeutic Peptide Amphiphile as a Drug Carrier with ATP-Triggered Release for Synergistic Effect, Improved Therapeutic Index, and Penetration of 3D Cancer Cell Spheroids. Int. J. Mol. Sci. 19, 2773. doi:10.3390/ijms19092773
Lv, Y., Hu, R., Zhu, G., Zhang, X., Mei, L., Liu, Q., et al. (2015). Preparation and Biomedical Applications of Programmable and Multifunctional DNA Nanoflowers. Nat. Protoc. 10, 1508–1524. doi:10.1038/nprot.2015.078
Lv, Z., Zhu, Y., and Li, F. (2021). DNA Functional Nanomaterials for Controlled Delivery of Nucleic Acid-Based Drugs. Front. Bioeng. Biotechnol. 9, 720291. doi:10.3389/fbioe.2021.720291
Ma, S., Li, X., Wang, X., Cheng, L., Li, Z., Zhang, C., et al. (2019). Current Progress in CAR-T Cell Therapy for Solid Tumors. Int. J. Biol. Sci. 15, 2548–2560. doi:10.7150/ijbs.34213
Ma, Y., Wang, Z., Ma, Y., Han, Z., Zhang, M., Chen, H., et al. (2018). A Telomerase-Responsive DNA Icosahedron for Precise Delivery of Platinum Nanodrugs to Cisplatin-Resistant Cancer. Angew. Chem. Int. Ed. 57, 5389–5393. doi:10.1002/anie.201801195
Martínez-López, A. L., Pangua, C., Reboredo, C., Campión, R., Morales-Gracia, J., and Irache, J. M. (2020). Protein-Based Nanoparticles for Drug Delivery Purposes. Int. J. Pharm. 581, 119289. doi:10.1016/j.ijpharm.2020.119289
Mei, Y., Tang, L., Xiao, Q., Zhang, Z., Zhang, Z., Zang, J., et al. (2021). Reconstituted High Density Lipoprotein (rHDL), a Versatile Drug Delivery Nanoplatform for Tumor Targeted Therapy. J. Mat. Chem. B 9, 612–633. doi:10.1039/d0tb02139c
Meir, R., Shamalov, K., Sadan, T., Motiei, M., Yaari, G., Cohen, C. J., et al. (2017). Fast Image-Guided Stratification Using Anti-Programmed Death Ligand 1 Gold Nanoparticles for Cancer Immunotherapy. ACS Nano 11, 11127–11134. doi:10.1021/acsnano.7b05299
Mi, P. (2020). Stimuli-Responsive Nanocarriers for Drug Delivery, Tumor Imaging, Therapy and Theranostics. Theranostics 10, 4557–4588. doi:10.7150/thno.38069
Mohsen, M. G., and Kool, E. T. (2016). The Discovery of Rolling Circle Amplification and Rolling Circle Transcription. Acc. Chem. Res. 49, 2540–2550. doi:10.1021/acs.accounts.6b00417
Monti, D. M., Ferraro, G., and Merlino, A. (2019). Ferritin-Based Anticancer Metallodrug Delivery: Crystallographic, Analytical and Cytotoxicity Studies. Nanomedicine 20. 101997. doi:10.1016/j.nano.2019.04.001
Mulder, W. J. M., van Leent, M. M. T., Lameijer, M., Fisher, E. A., Fayad, Z. A., and Pérez-Medina, C. (2018). High-Density Lipoprotein Nanobiologics for Precision Medicine. Acc. Chem. Res. 51, 127–137. doi:10.1021/acs.accounts.7b00339
Musetti, S., and Huang, L. (2018). Nanoparticle-Mediated Remodeling of the Tumor Microenvironment to Enhance Immunotherapy. ACS Nano 12, 11740–11755. doi:10.1021/acsnano.8b05893
Navya, P. N., Kaphle, A., Srinivas, S. P., Bhargava, S. K., Rotello, V. M., and Daima, H. K. (2019). Current Trends and Challenges in Cancer Management and Therapy Using Designer Nanomaterials. Nano Converg. 6, 23. doi:10.1186/s40580-019-0193-2
Ng, K. K., Lovell, J. F., and Zheng, G. (2011). Lipoprotein-Inspired Nanoparticles for Cancer Theranostics. Acc. Chem. Res. 44, 1105–1113. doi:10.1021/ar200017e
Olden, B. R., Cheng, Y., Yu, J. L., and Pun, S. H. (2018). Cationic Polymers for Non-viral Gene Delivery to Human T Cells. J. Control. Release 282, 140–147. doi:10.1016/j.jconrel.2018.02.043
Orlova, E. V., Sherman, M. B., Chiu, W., Mowri, H., Smith, L. C., and Gotto, A. M. (1999). Three-Dimensional Structure of Low Density Lipoproteins by Electron Cryomicroscopy. Proc. Natl. Acad. Sci. U.S.A. 96, 8420–8425. doi:10.1073/pnas.96.15.8420
Pan, Y., Yang, J., Luan, X., Liu, X., Li, X., Yang, J., et al. (2019). Near-Infrared Upconversion-Activated CRISPR-Cas9 System: A Remote-Controlled Gene Editing Platform. Sci. Adv. 5, eaav7199. doi:10.1126/sciadv.aav7199
Parayath, N. N., Stephan, S. B., Koehne, A. L., Nelson, P. S., and Stephan, M. T. (2020). In Vitro-Transcribed Antigen Receptor mRNA Nanocarriers for Transient Expression in Circulating T Cells In Vivo. Nat. Commun. 11, 6080. doi:10.1038/s41467-020-19486-2
Pham, T., Roth, S., Kong, J., Guerra, G., Narasimhan, V., Pereira, L., et al. (2018). An Update on Immunotherapy for Solid Tumors: A Review. Ann. Surg. Oncol. 25, 3404–3412. doi:10.1245/s10434-018-6658-4
Qin, H., Zhao, R., Qin, Y., Zhu, J., Chen, L., Di, C., et al. (2021). Development of a Cancer Vaccine Using In Vivo Click-Chemistry-Mediated Active Lymph Node Accumulation for Improved Immunotherapy. Adv. Mater. 33. e2006007. doi:10.1002/adma.202006007
Que, W.-c., Qiu, H.-q., Cheng, Y., Liu, M.-b., and Wu, C.-y. (2018). PTEN in Kidney Cancer: A Review and Meta-Analysis. Clin. Chim. Acta 480, 92–98. doi:10.1016/j.cca.2018.01.031
Rafii, S., Tashkandi, E., Bukhari, N., and Al-Shamsi, H. O. (2022). Current Status of CRISPR/Cas9 Application in Clinical Cancer Research: Opportunities and Challenges. Cancers (Basel) 14, 947. doi:10.3390/cancers14040947
Ramezani, H., and Dietz, H. (2020). Building Machines with DNA Molecules. Nat. Rev. Genet. 21, 5–26. doi:10.1038/s41576-019-0175-6
Raniolo, S., Vindigni, G., Ottaviani, A., Unida, V., Iacovelli, F., Manetto, A., et al. (2018). Selective Targeting and Degradation of Doxorubicin-Loaded Folate-Functionalized DNA Nanocages. Nanomedicine Nanotechnol. Biol. Med. 14, 1181–1190. doi:10.1016/j.nano.2018.02.002
Raza, F., Siyu, L., Zafar, H., Kamal, Z., Zheng, B., Su, J., et al. (2022). Recent Advances in Gelatin-Based Nanomedicine for Targeted Delivery of Anti-Cancer Drugs. Cpd 28, 380–394. doi:10.2174/1381612827666211102100118
Raza, F., Zafar, H., You, X., Khan, A., Wu, J., and Ge, L. (2019). Cancer Nanomedicine: Focus on Recent Developments and Self-Assembled Peptide Nanocarriers. J. Mat. Chem. B 7, 7639–7655. doi:10.1039/c9tb01842e
Raza, F., Zafar, H., Zhang, S., Kamal, Z., Su, J., Yuan, W., et al. (2021). Recent Advances in Cell Membrane-Derived Biomimetic Nanotechnology for Cancer Immunotherapy. Adv. Healthc. Mater. 10, e2002081. doi:10.1002/adhm.202002081
Riley, R. S., June, C. H., Langer, R., and Mitchell, M. J. (2019). Delivery Technologies for Cancer Immunotherapy. Nat. Rev. Drug Discov. 18, 175–196. doi:10.1038/s41573-018-0006-z
Rosenblum, D., Gutkin, A., Kedmi, R., Ramishetti, S., Veiga, N., Jacobi, A. M., et al. (2020). CRISPR-Cas9 Genome Editing Using Targeted Lipid Nanoparticles for Cancer Therapy. Sci. Adv. 6, eabc9450. doi:10.1126/sciadv.abc9450
Rui, M., Xin, Y., Li, R., Ge, Y., Feng, C., and Xu, X. (2017). Targeted Biomimetic Nanoparticles for Synergistic Combination Chemotherapy of Paclitaxel and Doxorubicin. Mol. Pharm. 14, 107–123. doi:10.1021/acs.molpharmaceut.6b00732
Rui, Y., Wilson, D. R., and Green, J. J. (2019). Non-Viral Delivery to Enable Genome Editing. Trends Biotechnol. 37, 281–293. doi:10.1016/j.tibtech.2018.08.010
Sahin, U., and Türeci, Ö. (2018). Personalized Vaccines for Cancer Immunotherapy. Science 359, 1355–1360. doi:10.1126/science.aar7112
Salter, A. M., and Brindley, D. N. (1988). The Biochemistry of Lipoproteins. J. Inherit. Metab. Dis. 11, 4–17. doi:10.1007/BF01800566
Saxena, M., van der Burg, S. H., Melief, C. J. M., and Bhardwaj, N. (2021). Therapeutic Cancer Vaccines. Nat. Rev. Cancer 21, 360–378. doi:10.1038/s41568-021-00346-0
Scheetz, L. M., Yu, M., Li, D., Castro, M. G., Moon, J. J., and Schwendeman, A. (2020). Synthetic HDL Nanoparticles Delivering Docetaxel and CpG for Chemoimmunotherapy of Colon Adenocarcinoma. Int. J. Mol. Sci. 21, 1777. doi:10.3390/ijms21051777
Shen, S., Dai, H., Fei, Z., Chai, Y., Hao, Y., Fan, Q., et al. (2021). Immunosuppressive Nanoparticles for Management of Immune-Related Adverse Events in Liver. ACS Nano 15, 9111–9125. doi:10.1021/acsnano.1c02391
Shen, Z., Zhou, L., Zhang, C., and Xu, J. (2020). Reduction of Circular RNA Foxo3 Promotes Prostate Cancer Progression and Chemoresistance to Docetaxel. Cancer Lett. 468, 88–101. doi:10.1016/j.canlet.2019.10.006
Sherman, M. B., Orlova, E. V., Decker, G. L., Chiu, W., and Pownall, H. J. (2003). Structure of Triglyceride-Rich Human Low-Density Lipoproteins According to Cryoelectron Microscopy. Biochemistry 42, 14988–14993. doi:10.1021/bi0354738
Shi, J., Yang, X., Li, Y., Wang, D., Liu, W., Zhang, Z., et al. (2020). MicroRNA-Responsive Release of Cas9/sgRNA from DNA Nanoflower for Cytosolic Protein Delivery and Enhanced Genome Editing. Biomaterials 256, 120221. doi:10.1016/j.biomaterials.2020.120221
Shi, S.-J., Wang, L.-J., Han, D.-H., Wu, J.-H., Jiao, D., Zhang, K.-L., et al. (2019). Therapeutic Effects of Human Monoclonal PSMA Antibody-Mediated TRIM24 siRNA Delivery in PSMA-Positive Castration-Resistant Prostate Cancer. Theranostics 9, 1247–1263. doi:10.7150/thno.29884
Smith, T. T., Stephan, S. B., Moffett, H. F., Mcknight, L. E., Ji, W., Reiman, D., et al. (2017). In Situ Programming of Leukaemia-Specific T Cells Using Synthetic DNA Nanocarriers. Nat. Nanotech. 12, 813–820. doi:10.1038/nnano.2017.57
Sobhani, N., Roviello, G., D'Angelo, A., Roudi, R., Neeli, P. K., and Generali, D. (2021). p53 Antibodies as a Diagnostic Marker for Cancer: A Meta-Analysis. Molecules 26, 6215. doi:10.3390/molecules26206215
Song, N., Zhang, J., Zhai, J., Hong, J., Yuan, C., and Liang, M. (2021). Ferritin: A Multifunctional Nanoplatform for Biological Detection, Imaging Diagnosis, and Drug Delivery. Acc. Chem. Res. 54, 3313–3325. doi:10.1021/acs.accounts.1c00267
Subhan, M. A., and Torchilin, V. P. (2019). Efficient Nanocarriers of siRNA Therapeutics for Cancer Treatment. Transl. Res. 214, 62–91. doi:10.1016/j.trsl.2019.07.006
Sukumar, U. K., Rajendran, J. C. B., Gambhir, S. S., Massoud, T. F., and Paulmurugan, R. (2020). SP94-Targeted Triblock Copolymer Nanoparticle Delivers Thymidine Kinase-P53-Nitroreductase Triple Therapeutic Gene and Restores Anticancer Function Against Hepatocellular Carcinoma In Vivo. ACS Appl. Mat. Interfaces 12, 11307–11319. doi:10.1021/acsami.9b20071
Sung, H., Ferlay, J., Siegel, R. L., Laversanne, M., Soerjomataram, I., Jemal, A., et al. (2021). Global Cancer Statistics 2020: GLOBOCAN Estimates of Incidence and Mortality Worldwide for 36 Cancers in 185 Countries. CA A Cancer J. Clin. 71, 209–249. doi:10.3322/caac.21660
Tan, S., Li, D., and Zhu, X. (2020). Cancer Immunotherapy: Pros, Cons and Beyond. Biomed. Pharmacother. 124, 109821. doi:10.1016/j.biopha.2020.109821
Tan, T., Wang, H., Cao, H., Zeng, L., Wang, Y., Wang, Z., et al. (2018). Deep Tumor-Penetrated Nanocages Improve Accessibility to Cancer Stem Cells for Photothermal-Chemotherapy of Breast Cancer Metastasis. Adv. Sci. 5, 1801012. doi:10.1002/advs.201801012
Tang, L., Zheng, Y., Melo, M. B., Mabardi, L., Castaño, A. P., Xie, Y.-Q., et al. (2018). Enhancing T Cell Therapy Through TCR-Signaling-Responsive Nanoparticle Drug Delivery. Nat. Biotechnol. 36, 707–716. doi:10.1038/nbt.4181
Tao, Y., Yi, K., Hu, H., Shao, D., and Li, M. (2021). Coassembly of Nucleus-Targeting Gold Nanoclusters with CRISPR/Cas9 for Simultaneous Bioimaging and Therapeutic Genome Editing. J. Mater Chem. B 9, 94–100. doi:10.1039/d0tb01925a
Tesarova, B., Musilek, K., Rex, S., and Heger, Z. (2020). Taking Advantage of Cellular Uptake of Ferritin Nanocages for Targeted Drug Delivery. J. Control. Release 325, 176–190. doi:10.1016/j.jconrel.2020.06.026
Thaxton, C. S., Rink, J. S., Naha, P. C., and Cormode, D. P. (2016). Lipoproteins and Lipoprotein Mimetics for Imaging and Drug Delivery. Adv. Drug Deliv. Rev. 106, 116–131. doi:10.1016/j.addr.2016.04.020
Vaddepally, R. K., Kharel, P., Pandey, R., Garje, R., and Chandra, A. B. (2020). Review of Indications of FDA-Approved Immune Checkpoint Inhibitors Per NCCN Guidelines with the Level of Evidence. Cancers (Basel) 12, 738. doi:10.3390/cancers12030738
van den Bulk, J., Verdegaal, E. M., and de Miranda, N. F. (2018). Cancer Immunotherapy: Broadening the Scope of Targetable Tumours. Open Biol. 8, 180037. doi:10.1098/rsob.180037
Veroniaina, H., Wu, Z., and Qi, X. (2021). Innate Tumor-Targeted Nanozyme Overcoming Tumor Hypoxia for Cancer Theranostic Use. J. Adv. Res. 33, 201–213. doi:10.1016/j.jare.2021.02.004
Wang, B., Yuan, Y., Han, L., Ye, L., Shi, X., and Feng, M. (2014). Recombinant Lipoproteins Reinforce Cytotoxicity of Doxorubicin to Hepatocellular Carcinoma. J. Drug Target. 22, 76–85. doi:10.3109/1061186x.2013.839687
Wang, C.-S., Chang, C.-H., Tzeng, T.-Y., Lin, A. M.-Y., and Lo, Y.-L. (2021a). Gene-Editing by CRISPR-Cas9 in Combination with Anthracycline Therapy via Tumor Microenvironment-Switchable, EGFR-Targeted, and Nucleus-Directed Nanoparticles for Head and Neck Cancer Suppression. Nanoscale Horiz. 6, 729–743. doi:10.1039/d1nh00254f
Wang, J., Li, Y., and Nie, G. (2021b). Multifunctional Biomolecule Nanostructures for Cancer Therapy. Nat. Rev. Mater. 6, 766–783. doi:10.1038/s41578-021-00315-x
Wang, X., Zhong, X., Li, J., Liu, Z., and Cheng, L. (2021c). Inorganic Nanomaterials with Rapid Clearance for Biomedical Applications. Chem. Soc. Rev. 50, 8669–8742. doi:10.1039/d0cs00461h
Wang, Z., Song, L., Liu, Q., Tian, R., Shang, Y., Liu, F., et al. (2021d). A Tubular DNA Nanodevice as a siRNA/Chemo‐Drug Co‐Delivery Vehicle for Combined Cancer Therapy. Angew. Chem. Int. Ed. 60, 2594–2598. doi:10.1002/anie.202009842
Wang, Z., Zhao, Y., Zhang, S., Chen, X., Sun, G., Zhang, B., et al. (2022). Re-Engineering the Inner Surface of Ferritin Nanocage Enables Dual Drug Payloads for Synergistic Tumor Therapy. Theranostics 12, 1800–1815. doi:10.7150/thno.68459
Wei, G., Wang, Y., Yang, G., Wang, Y., and Ju, R. (2021). Recent Progress in Nanomedicine for Enhanced Cancer Chemotherapy. Theranostics 11, 6370–6392. doi:10.7150/thno.57828
Wei, Y., Gu, X., Sun, Y., Meng, F., Storm, G., and Zhong, Z. (2020). Transferrin-Binding Peptide Functionalized Polymersomes Mediate Targeted Doxorubicin Delivery to Colorectal Cancer In Vivo. J. Control. Release 319, 407–415. doi:10.1016/j.jconrel.2020.01.012
Wu, T., Liu, J., Liu, M., Liu, S., Zhao, S., Tian, R., et al. (2019). A Nanobody‐Conjugated DNA Nanoplatform for Targeted Platinum‐Drug Delivery. Angew. Chem. Int. Ed. 58, 14224–14228. doi:10.1002/anie.201909345
Xing, R., Wang, X., Zhang, C., Zhang, Y., Wang, Q., Yang, Z., et al. (2009). Characterization and Cellular Uptake of Platinum Anticancer Drugs Encapsulated in Apoferritin. J. Inorg. Biochem. 103, 1039–1044. doi:10.1016/j.jinorgbio.2009.05.001
Xu, W., He, W., Du, Z., Zhu, L., Huang, K., Lu, Y., et al. (2021). Functional Nucleic Acid Nanomaterials: Development, Properties, and Applications. Angew. Chem. Int. Ed. 60, 6890–6918. doi:10.1002/anie.201909927
Yahya, E. B., and Alqadhi, A. M. (2021). Recent Trends in Cancer Therapy: A Review on the Current State of Gene Delivery. Life Sci. 269, 119087. doi:10.1016/j.lfs.2021.119087
Yan, J., Chen, J., Zhang, N., Yang, Y., Zhu, W., Li, L., et al. (2020). Mitochondria-Targeted Tetrahedral DNA Nanostructures for Doxorubicin Delivery and Enhancement of Apoptosis. J. Mater. Chem. B 8, 492–503. doi:10.1039/c9tb02266j
Yang, M., Li, J., Gu, P., and Fan, X. (2021). The Application of Nanoparticles in Cancer Immunotherapy: Targeting Tumor Microenvironment. Bioact. Mater. 6, 1973–1987. doi:10.1016/j.bioactmat.2020.12.010
Yang, Z., Zhang, N., Ma, T., Liu, L., Zhao, L., and Xie, H. (2020). Engineered Bovine Serum Albumin-Based Nanoparticles with pH-Sensitivity for Doxorubicin Delivery and Controlled Release. Drug Deliv. 27, 1156–1164. doi:10.1080/10717544.2020.1797243
Yardley, D. A. (2013). Nab-Paclitaxel Mechanisms of Action and Delivery. J. Control. Release 170, 365–372. doi:10.1016/j.jconrel.2013.05.041
Yin, H., Yuan, X., Luo, L., Lu, Y., Qin, B., Zhang, J., et al. (2020). Appropriate Delivery of the CRISPR/Cas9 System Through the Nonlysosomal Route: Application for Therapeutic Gene Editing. Adv. Sci. 7, 1903381. doi:10.1002/advs.201903381
Zafar, H., Raza, F., Ma, S., Wei, Y., Zhang, J., and Shen, Q. (2021). Recent Progress on Nanomedicine-Induced Ferroptosis for Cancer Therapy. Biomater. Sci. 9, 5092–5115. doi:10.1039/d1bm00721a
Zang, J., Chen, H., Zhao, G., Wang, F., and Ren, F. (2017). Ferritin Cage for Encapsulation and Delivery of Bioactive Nutrients: From Structure, Property to Applications. Crit. Rev. Food Sci. Nutr. 57, 3673–3683. doi:10.1080/10408398.2016.1149690
Zhan, T., Rindtorff, N., Betge, J., Ebert, M. P., and Boutros, M. (2019). CRISPR/Cas9 for Cancer Research and Therapy. Seminars Cancer Biol. 55, 106–119. doi:10.1016/j.semcancer.2018.04.001
Zhang, D. Y., Dmello, C., Chen, L., Arrieta, V. A., Gonzalez-Buendia, E., Kane, J. R., et al. (2020a). Ultrasound-Mediated Delivery of Paclitaxel for Glioma: A Comparative Study of Distribution, Toxicity, and Efficacy of Albumin-Bound versus Cremophor Formulations. Clin. Cancer Res. 26, 477–486. doi:10.1158/1078-0432.ccr-19-2182
Zhang, G., Zhang, Z., and Yang, J. (2017a). DNA Tetrahedron Delivery Enhances Doxorubicin-Induced Apoptosis of HT-29 Colon Cancer Cells. Nanoscale Res. Lett. 12, 495. doi:10.1186/s11671-017-2272-9
Zhang, J., Cheng, D., He, J., Hong, J., Yuan, C., and Liang, M. (2021a). Cargo Loading within Ferritin Nanocages in Preparation for Tumor-Targeted Delivery. Nat. Protoc. 16, 4878–4896. doi:10.1038/s41596-021-00602-5
Zhang, L., Abdullah, R., Hu, X., Bai, H., Fan, H., He, L., et al. (2019a). Engineering of Bioinspired, Size-Controllable, Self-Degradable Cancer-Targeting DNA Nanoflowers via the Incorporation of an Artificial Sandwich Base. J. Am. Chem. Soc. 141, 4282–4290. doi:10.1021/jacs.8b10795
Zhang, P., Ouyang, Y., Sohn, Y. S., Nechushtai, R., Pikarsky, E., Fan, C., et al. (2021b). pH- and miRNA-Responsive DNA-Tetrahedra/Metal-Organic Framework Conjugates: Functional Sense-And-Treat Carriers. ACS Nano 15, 6645–6657. doi:10.1021/acsnano.0c09996
Zhang, Q., Chen, J., Shen, J., Chen, S., Liang, K., Wang, H., et al. (2019b). Inlaying Radiosensitizer onto the Polypeptide Shell of Drug-Loaded Ferritin for Imaging and Combinational Chemo-Radiotherapy. Theranostics 9, 2779–2790. doi:10.7150/thno.33472
Zhang, Q., Jiang, Q., Li, N., Dai, L., Liu, Q., Song, L., et al. (2014). DNA Origami as an In Vivo Drug Delivery Vehicle for Cancer Therapy. ACS Nano 8, 6633–6643. doi:10.1021/nn502058j
Zhang, S., Shen, J., Li, D., and Cheng, Y. (2021c). Strategies in the Delivery of Cas9 Ribonucleoprotein for CRISPR/Cas9 Genome Editing. Theranostics 11, 614–648. doi:10.7150/thno.47007
Zhang, Y., Fang, F., Li, L., and Zhang, J. (2020b). Self-Assembled Organic Nanomaterials for Drug Delivery, Bioimaging, and Cancer Therapy. ACS Biomater. Sci. Eng. 6, 4816–4833. doi:10.1021/acsbiomaterials.0c00883
Zhang, Y., Jiang, S., Zhang, D., Bai, X., Hecht, S. M., and Chen, S. (2017b). DNA-Affibody Nanoparticles for Inhibiting Breast Cancer Cells Overexpressing HER2. Chem. Commun. 53, 573–576. doi:10.1039/c6cc08495h
Zhang, Y., Lin, S., Wang, X., and Zhu, G. (2019c). Nanovaccines for Cancer Immunotherapy. Wiley Interdiscip. Rev. Nanomed. Nanobiotechnol. 11. e1559. doi:10.1002/wnan.1559
Zhang, Y., Sun, T., and Jiang, C. (2018). Biomacromolecules as Carriers in Drug Delivery and Tissue Engineering. Acta Pharm. Sin. B 8, 34–50. doi:10.1016/j.apsb.2017.11.005
Zhang, Z., Jiao, Y., Zhu, M., and Zhang, S. (2017c). Nuclear-Shell Biopolymers Initiated by Telomere Elongation for Individual Cancer Cell Imaging and Drug Delivery. Anal. Chem. 89, 4320–4327. doi:10.1021/acs.analchem.7b00591
Zhang, Z., Wan, T., Chen, Y., Chen, Y., Sun, H., Cao, T., et al. (2019d). Cationic Polymer-Mediated CRISPR/Cas9 Plasmid Delivery for Genome Editing. Macromol. Rapid Commun. 40, e1800068. doi:10.1002/marc.201800068
Zhao, F., Tian, J., An, L., and Yang, K. (2014). Prognostic Utility of Gene Therapy with Herpes Simplex Virus Thymidine Kinase for Patients with High-Grade Malignant Gliomas: A Systematic Review and Meta Analysis. J. Neurooncol. 118, 239–246. doi:10.1007/s11060-014-1444-z
Zhao, H., Yuan, X., Yu, J., Huang, Y., Shao, C., Xiao, F., et al. (2018). Magnesium-Stabilized Multifunctional DNA Nanoparticles for Tumor-Targeted and pH-Responsive Drug Delivery. ACS Appl. Mat. Interfaces 10, 15418–15427. doi:10.1021/acsami.8b01932
Zhao, Y., Liang, M., Li, X., Fan, K., Xiao, J., Li, Y., et al. (2016). Bioengineered Magnetoferritin Nanoprobes for Single-Dose Nuclear-Magnetic Resonance Tumor Imaging. ACS Nano 10, 4184–4191. doi:10.1021/acsnano.5b07408
Zhen, Z., Tang, W., Chen, H., Lin, X., Todd, T., Wang, G., et al. (2013). RGD-Modified Apoferritin Nanoparticles for Efficient Drug Delivery to Tumors. ACS Nano 7, 4830–4837. doi:10.1021/nn305791q
Zhong, X., Zhang, H., Zhu, Y., Liang, Y., Yuan, Z., Li, J., et al. (2020). Circulating Tumor Cells in Cancer Patients: Developments and Clinical Applications for Immunotherapy. Mol. Cancer 19, 15. doi:10.1186/s12943-020-1141-9
Zhu, G., Zhang, F., Ni, Q., Niu, G., and Chen, X. (2017a). Efficient Nanovaccine Delivery in Cancer Immunotherapy. ACS Nano 11, 2387–2392. doi:10.1021/acsnano.7b00978
Zhu, W. J., Yang, S. D., Qu, C. X., Zhu, Q. L., Chen, W. L., Li, F., et al. (2017b). Low-Density Lipoprotein-Coupled Micelles with Reduction and pH Dual Sensitivity for Intelligent Co-Delivery of Paclitaxel and siRNA to Breast Tumor. Int. J. Nanomedicine 12, 3375–3393. doi:10.2147/IJN.S126310
Zhu, Y., Wang, L., Li, Y., Huang, Z., Luo, S., He, Y., et al. (2020). Injectable pH and Redox Dual Responsive Hydrogels Based on Self-Assembled Peptides for Anti-Tumor Drug Delivery. Biomater. Sci. 8, 5415–5426. doi:10.1039/d0bm01004a
Glossary
NDSs, nanoparticulate delivery systems
NPs, nanoparticles
NFs, nanoflowers
RCA, rolling circle amplification
TfR, transferrin receptor
HFt, human ferritin
HoSF, horse spleen ferritin
AfFtn, Archaeoglobus fulgidus ferritin
PfFt, Pyrococcus furiosus ferritin
HFn, heavy chain ferritin
LFn, light chain ferritin
VLDL, very low density lipoproteins
LDL, low density lipoproteins
HDL, high-density lipoprotein
rHDL, recombinant HDL apolipoprotein B-100
SR-B1, scavenger receptor class B type 1
p53, protein 53
PTEN, phosphatase and tensin homolog
HSV-TK, herpes simplex virus thymidine kinase
mRNA, messenger RNA
siRNA, small interfering RNA
sgRNA, single guide RNA
CAR-T, chimeric antigen receptor T
CTLA-4, cytotoxic-T-lymphocyte-associated protein 4
PD-1, program death 1
PD-L1, program death-ligand 1
CRISPR, clustered regularly interspaced short palindromic repeat
mAbs, monoclonal antibodies
ICIs, immune checkpoint inhibitors
irAIEs, immune-related adverse events
Keywords: nanomedicine, chemotherapy, gene therapy, immunotherapy, cancer
Citation: Hou S, Hasnat M, Chen Z, Liu Y, Faran Ashraf Baig MM, Liu F and Chen Z (2022) Application Perspectives of Nanomedicine in Cancer Treatment. Front. Pharmacol. 13:909526. doi: 10.3389/fphar.2022.909526
Received: 31 March 2022; Accepted: 16 May 2022;
Published: 01 July 2022.
Edited by:
Faisal Raza, Shanghai Jiao Tong University, ChinaReviewed by:
Ruoning Wang, Nanjing University of Chinese Medicine, ChinaChunming Tang, Nanjing Medical University, China
Copyright © 2022 Hou, Hasnat, Chen, Liu, Faran Ashraf Baig, Liu and Chen. This is an open-access article distributed under the terms of the Creative Commons Attribution License (CC BY). The use, distribution or reproduction in other forums is permitted, provided the original author(s) and the copyright owner(s) are credited and that the original publication in this journal is cited, in accordance with accepted academic practice. No use, distribution or reproduction is permitted which does not comply with these terms.
*Correspondence: Zelong Chen, emx5eWN6bDQyNjZAenp1LmVkdS5jbg==; Fuhe Liu, ODk5OTQyMjZAcXEuY29t
†These authors have contributed equally to this work