- 1Instituto de Investigaciones Bioquímicas de Bahía Blanca (INIBIBB) CCT UNS-CONICET, Bahía Blanca, Argentina
- 2Departamento de Biología, Bioquímica y Farmacia, Universidad Nacional Del Sur (UNS), Bahía Blanca, Argentina
- 3Departamento de Química, INQUISUR, Universidad Nacional Del Sur, UNS-CONICET, Bahía Blanca, Argentina
Due to the increase in life expectancy worldwide, age-related disorders such as neurodegenerative diseases (NDs) have become more prevalent. Conventional treatments comprise drugs that only attenuate some of the symptoms, but fail to arrest or delay neuronal proteotoxicity that characterizes these diseases. Due to their diverse biological activities, imidazole rings are intensively explored as powerful scaffolds for the development of new bioactive molecules. By using C. elegans, our work aims to explore novel biological roles for these compounds. To this end, we have tested the in vivo anti-proteotoxic effects of imidazolium salts. Since NDs have been largely linked to impaired antioxidant defense mechanisms, we focused on 1-Mesityl-3-(3-sulfonatopropyl) imidazolium (MSI), one of the imidazolium salts that we identified as capable of improving iron-induced oxidative stress resistance in wild-type animals. By combining mutant and gene expression analysis we have determined that this protective effect depends on the activation of the Heat Shock Transcription Factor (HSF-1), whereas it is independent of other canonical cytoprotective molecules such as abnormal Dauer Formation-16 (DAF-16/FOXO) and Skinhead-1 (SKN-1/Nrf2). To delve deeper into the biological roles of MSI, we analyzed the impact of this compound on previously established C. elegans models of protein aggregation. We found that MSI ameliorates β-amyloid-induced paralysis in worms expressing the pathological protein involved in Alzheimer’s Disease. Moreover, this compound also delays age-related locomotion decline in other proteotoxic C. elegans models, suggesting a broad protective effect. Taken together, our results point to MSI as a promising anti-proteotoxic compound and provide proof of concept of the potential of imidazole derivatives in the development of novel therapies to retard age-related proteotoxic diseases.
1 Introduction
During the last century, scientific advances have accomplished an increase in the life expectancy of the population. This achievement, however, entails new challenges such as a higher prevalence of age-related disorders. Today, neurodegenerative diseases (NDs) such as Alzheimer’s disease (AD), Parkinson’s disease (PD) and Huntington’s disease (HD), have become a major health concern. Despite decades of intensive investigation, these diseases still lack a cure. While each of these disorders has its distinctive features (e.g., the type of neurons affected), they share a common pathological hallmark: the abnormal formation and accumulation of misfolded protein aggregates in specific neurons (Gitler et al., 2017). In AD, the senile plaques mainly consist of regular β-sheet fibrils of β-amyloid peptide (Aβ); in PD, the Lewis bodies are composed of the protein α-synuclein (α-syn); in HD, an abnormally expanded polyglutamine domain of the protein huntingtin induces protein aggregation (Morfini et al., 2005; Huang and Mucke, 2012). Due to the presence of these typical protein aggregates that cause neuronal damage, these diseases are called proteinopathies.
The cellular and molecular mechanisms underlying protein aggregation are not yet understood (Bucciantini et al., 2002; Sweeney et al., 2017). However, protective proteins involved in modulating scavenging mechanisms for free radicals and oxidative metabolites, such as the cytoprotective transcription factors (TFs) Nrf-2 (Nuclear factor erythroid 2-related factor 2), HSF (Heat Shock Transcription Factor) and FOXO (Forkhead box) were found to be actively involved in neuronal proteostasis (Hasanbašić et al., 2018; Nandi et al., 2019). There is strong evidence that saturation of these endogenous antioxidant defenses represents key factors in the progression of NDs (Jenner, 2003; Blesa et al., 2015; Lévy et al., 2019). For instance, it was reported that overexpression of active forms of HSF-1 decreases protein aggregation both in vitro and in vivo models of NDs (Fujimoto et al., 2005; Kumsta et al., 2017). In addition, while downregulation of Nrf-2 activity has been shown to induce α-syn aggregation (He et al., 2013), its expression is neuroprotective in cellular and animal models of PD (Lastres-Becker et al., 2012; Fu et al., 2018). Moreover, FOXO activity delays the onset of protein aggregation in animal models of HD and AD (Jiang et al., 2011; Hesp et al., 2015). Hence, modulating the pathways controlled by these TFs may inhibit or at least retard pathological protein aggregation.
Since the 1970s, the free-living nematode C. elegans has been extensively used as an inexpensive, safe and powerful organism for basic and applied biomedical studies. In the last 20 years, several reports also highlighted the use of this nematode for modeling human pathologies (including NDs) and for the screening of potential therapeutic compounds (Kaletta and Hengartner, 2006; Markaki and Tavernarakis, 2010; Giunti et al., 2021). Its short and genetically tractable lifespan makes C. elegans particularly suitable for exploring age-related diseases (Kenyon et al., 1993; Samuelson et al., 2007; Uno and Nishida, 2016). In addition, the analysis of synaptic connections by serial electron microscopy has led to the reconstruction of the complete neural wiring diagram (White et al., 1986; Cook et al., 2019), which allows the identification of precise neural circuits that control specific behaviors and physiological processes. Moreover, C. elegans transparency permits the visualization of the morphology and activity of specific cells using transgenic reporters and genetically encoded calcium indicators (Chalfie et al., 1994; Kerr and Schafer, 2006; Chung et al., 2013) in freely moving animals. Given the striking conservation in neuronal function throughout the animal kingdom, C. elegans offers the possibility to provide fundamental insights into nervous system (NS) aging. It has been shown that the NS of C. elegans undergoes age-dependent decline with morphological structural alterations comparable to those that take place in the human brain (Burke and Barnes, 2006; Benard and Hobert, 2009; Fjell and Walhovd, 2010; Bénard and Doitsidou, 2016; Hess et al., 2019).
C. elegans has no orthologous gene for Aβ, α-syn, or huntingtin. However, transgenic animals that express the disease-causing protein for AD, PD, and, HD either in body-wall muscle cells, in all neurons, or in a specific subset of neurons have been generated (Link, 1995; Morley et al., 2002; Brignull et al., 2006; Witan et al., 2008; Gidalevitz et al., 2009; Liachko et al., 2010). In most cases, the protein is tagged with a fluorescent reporter, which permits monitoring protein aggregation in whole live animals. In addition, genetic approaches in C. elegans have pioneered the elucidation of highly conserved cellular pathways controlling proteostasis, oxidative stress (OS) resistance, and lifespan (Fontana et al., 2010; Kenyon, 2010). The best-known example is the DAF-2/insulin/insulin-like growth factor signaling (IIS) pathway, which regulates lifespan and proteotoxic stress resistance from worms to flies to mammals (Cohen and Dillin, 2008; Murphy and Hu, 2013; Altintas et al., 2016). The DAF-2/IIS pathway is a crucial regulator of conserved cytoprotective mechanisms such as DAF-16/FOXO, SKN-1/ Nrf-2, and the HSF-1/HSF1. Similar to mammals, activation of these mechanisms reduces the damage induced by protein aggregation in C. elegans models of NDs (Kenyon et al., 1993; Hsu et al., 2003; Cohen et al., 2006; Anckar and Sistonen, 2011; Teixeira-Castro et al., 2011; Zhang et al., 2012; Steinbaugh et al., 2015). Thus, C. elegans has evolved as an excellent whole-animal platform to identify genes, drug targets, and chemical compounds with plausible neuroprotective roles for human NDs (Morley et al., 2002; Lakso et al., 2003; Kang and Avery, 2009; Yerbury et al., 2016; Sanchis et al., 2019; Giunti et al., 2021; Pereira et al., 2022).
To develop new bioactive molecules, the pharmaceutical industry has extensively benefited from the versatility of heterocyclic compounds (Gaba et al., 2014). Due to their capacity to bind to several biological targets, nitrogen-containing heterocycles such as imidazole rings, have been largely used as powerful scaffolds for drug development (Sharma et al., 2016). A wide spectrum of activities, including analgesic, anti-inflammatory, anti-infection, anticonvulsant, and antiparasitic actions have been described for imidazole-containing compounds (Blanco et al., 2018; Shalmali et al., 2018). In addition, the imidazole ring’s biocompatibility together with the feasibility to synthesize new derivatives with different pharmacokinetics and pharmacodynamics properties by chemical modifications further enhance their potential use in drug development (Anderson and Long, 2010).
In this work, we used C. elegans to evaluate the anti-proteotoxic potential of imidazole derivatives. We found that exposure to one of these derivatives, 1-Mesityl-3 (3-sulfonatopropyl) imidazolium (MSI), induces the HSF-1 pathway leading to an increase in OS resistance. Furthermore, this compound delays the onset of proteotoxic-associated phenotypes in transgenic animals expressing ND-related pathological proteins. In addition, we provide a proof-of-concept validation for the screening of imidazole compounds with anti-proteotoxic activity using C. elegans models of protein aggregation.
2 Methods
2.1 Synthesis: General Procedures
All reactions were carried out under a dry nitrogen atmosphere by using Schlenk techniques. Organic solvents were dried and distilled under nitrogen and degassed before use. All imidazolium salts were synthesized according to reported procedures (Blanco et al., 2018) as shown in Scheme 1 (Spectroscopy data, see Supplementary Material electronic). 1H- and 13C-NMR spectra were recorded on a Bruker ARX 300 (300.1 MHz for 1H, 75.5 MHz for 13C) using D2O or DMSO-d6 as solvents. C, H, and N analyses were performed by The Analytical Services of the Universidad Nacional del Sur (Argentina) with an Exeter Analytical Inc. CE-440 microanalyzer.
2.2 Determination of Oxidation-Reduction Potential
The Oxidation-Reduction Potential (ORP) of the complexes was calculated using an Ion-selective pH meter Thermo Scientific Orion 720 A. Electrode Redox/ORP Thermo Scientific Orion 9678BNWP.
2.3 C. elegans Culture and Maintenance
Worms were cultured and maintained on Nematode Growth Medium (NGM) agar plates seeded with Escherichia coli OP50 as a food source (Brenner, 1974; Stiernagle, 2006). Worm culture and all the experiments were carried out at 20°C, unless otherwise noted. The wild-type strain was Bristol N2. The strains were provided by the Caenorhabditis Genetics Center (CGC), which is funded by the NIH Office of Research Infrastructure Programs (P40 OD010440).
Low population density was maintained throughout development and during the assays. For all experiments, animals were age-synchronized. The strains used were: CL2070 dvIs70[Phsp-16.2::gfp + pRF4], CL2166 dvIs19[Pgst−4::gfp::NLS], TJ356 zIs356[Pdaf-16::DAF−16a/b::gfp + pRF4], NL5901 pkIs2386[Punc−54::alphasynuclein::yfp + unc−119(+)], CL2006 dvIs2[pCL12(unc−54/human Aβ peptide 3–42 minigene) + rol−6(su1006)], AM141 rmIs133[Punc−54::Q40::yfp], PS3551 hsf-1(sy441) I, QV225 skn-1(zj15) IV, GR1307 daf−16(mgDf50) I.
2.4 Imidazolium Salts Exposure
The pharmacological assays were performed as described before (Blanco et al., 2018; De Rosa et al., 2019). 10 mM individual imidazole derivatives stocks were prepared with sterile MilliQ water and diluted into NGM agar at 10, 50 or 100 μM. The control condition was prepared with sterile MilliQ without the compound. Plates were stored at 4°C and used within 1 week.
In all experiments, age-synchronized individuals were used. To achieve this synchronization, gravid adults were placed on NGM plates containing the drug, allowed to lay eggs for 8 h, and then removed. Descendants were maintained on these plates until the stage of interest.
2.5 Oxidative Stress Resistance Assay
Iron sulfate (FeSO4) was used as an oxidative stressor as previously reported (De Rosa et al., 2019). Briefly, 20 L4 worms were transferred to 35-mm agar plates (3 plates per condition) containing 10 mM FeSO4. After 60 min, animal survival was immediately scored. Worms were scored as dead if they failed to respond to prodding with a platinum wire pick to the nose.
2.6 Pharyngeal Pumping Rate
Age synchronized animals were grown on NGM plates containing 10 μM of the compound. Young adults (24 h post L4s) were transferred to new NGM plates containing the drug and seeded with fresh bacteria. The number of contractions in the terminal bulb of the pharynx was video-recorded for 15 s using a stereomicroscope coupled to NIKON DS-Qi2 Camera (100x magnification). Recordings were reproduced at a slow speed to manually count the number of pharyngeal pumps. This experiment was independently repeated three times.
2.7 Developmental Rate Evaluation
A short synchronization was performed. Briefly, 4 gravid adults were placed on NGM plates (4 plates per condition), left to lay eggs for 3 h and removed. The number of animals at different developmental stages (L1-L3, L4, and adults) was counted at 24, 48, and 72 h. Results were expressed as the number of animals at a given stage × 100/ Total number of living worms at that time point. This experiment was independently repeated three times.
2.8 Microscopy and Image Analysis
For microscopy analysis, animals were grown on NGM plates containing the compound. At the proper age for each experiment, worms were immobilized in 25 mM Levamisole (in M9) and mounted onto slides with 2% agarose pads. Images were obtained using an epifluorescence microscope (NIKON ECLIPSE TE2000-S) coupled to a monochrome CMOS Nikon DS-Qi2 Camera (NIS-Elements acquisition software) at × 10 and × 20 objective mag. Images were analyzed using Image J FIJI software (ImageJ, National Institutes of Health, Bethesda, MD, United States).
2.9 hsp-16.2 Expression
hsp-16.2 expression was analyzed in a transgenic strain containing a transcriptional GFP reporter in a wild-type background CL2070 dvIs70[Phsp−16.2::gfp + pRF4].
Briefly, 16 h post-L4 animals (adult day 1) were subjected to heat shock for 2 h at 35°C followed by 4 h of recovery at 20°C. Upon induction by thermal stress, GFP fluorescence was detectable at the head of the worm, including the pharynx (Strayer et al., 2004; Ogawa et al., 2016). Therefore, images were taken in this region, and hsp-16.2::GFP fluorescence intensity was measured in same-sized regions of the pharynx, specifically at the terminal bulb using ImageJ FIJI software. Results were normalized to control conditions (without MSI).
2.10 Worm Paralysis Assay
Paralysis was evaluated in transgenic animals expressing the human amyloid β3-42 (Aβ3−42) in body wall muscle cells (construct [Punc-54::human A-beta 3–42; pRF4 (rol-6(su1006)], see CL2006 in strain list). This strain experiments age-dependent paralysis (Link, 1995), which was assessed under basal conditions (paralysis assay) and after a brief heat-shock of 35°C for 30 min (thermal-induced paralysis assay) (Srivastava et al., 2008; Arya et al., 2009; Regitz et al., 2016).
2.10.1 Paralysis Assay
Animals were synchronized by placing gravid animals in NGM plates, and after 12 h of egg-laying, the adults were removed. After 3.5 days post-hatch, animals were re-synchronized by picking L4 animals. From that moment onwards, worms were tested every day for paralysis by prodding with a platinum wire. Animals were scored as paralyzed if they failed to move their bodies or only moved their head (without rolling). Paralyzed animals were removed from plates for the next day’s reading (Cohen et al., 2006).
2.10.2 Thermal-Induced Paralysis Assay
Heat shock accelerates the paralyzation process (Srivastava et al., 2008; Arya et al., 2009; Regitz et al., 2016). In this case, adult day 1 worms were subject to brief heat stress (35°C for 30 min), and paralyzed animals were subsequently scored as described previously.
2.11 Locomotion Assays
To evaluate mobility, one animal (at the indicated days of adulthood, starting at day 0 (L4 stage)) was transferred to a multi-well plate containing 150 μl of M9 buffer (one worm/well). After 1 min of habituation, thrashing rates were manually counted under a dissecting scope as previously described (Jones et al., 2011; Agotegaray et al., 2020). A single thrash is defined as a change in the direction of bending at the midbody (Buckingham and Sattelle, 2009; Jones et al., 2011). During this assay, the number of times that the concavity of the worm is directed to the same side (e.g., concavity to the left) is counted. This number is then multiplied × 2 (to include the number of times that the body concavity faces the right side) to get the number of thrashes per minute. All assays were carried out by two independent operators and were blinded to the sample identities.
2.12 Alpha-Synuclein Protein Quantification
Alpha-synuclein aggregation was evaluated at increasing ages using the strain NL5901 pkIs2386 [Punc−54::α-synuclein::yfp + unc−119(+)]. These animals express alpha-synuclein fused to YFP in body wall muscle cells (van Ham et al., 2008). After their corresponding incubation with MSI, animals were mounted and the YFP signal in the head region was examined under a fluorescence microscope.
To measure the accumulation of alpha-synuclein, the head area was highlighted with a uniform rectangle, and mean fluorescence intensity was quantified using Image J FIJI software (Jadiya et al., 2011; Jadiya et al., 2016; Tsai et al., 2020). Results were normalized to the control group.
2.13 Polyglutamine (Poly-Q) Aggregation Quantification
AM141 strain [Punc−54::Q40::yfp] was used for the poly-Q aggregation assay (Morley et al., 2002). At the indicated time, at least 30 worms from each condition were immobilized and mounted, as described above. Images were acquired using fluorescence microscopy. The number of aggregates and their size were quantified with the analyze particle function in ImageJ FIJI software.
2.14 In Silico Evaluation of ADME Profile, Bioavailability, and Drug-Likeness
SMILE structure for each compound was generated by using the structure file generator, available at the free online tool SwissADME web server (http://www.swissadme.ch/) (Daina et al., 2017). Considering six physicochemical properties such as solubility, molecular size, polarity, lipophilicity, saturation, and flexibility, this tool predicts and weights the probability of the oral bioavailability of the drug by building a bioavailability radar.
The Abbott bioavailability score was also calculated to predict the probability of a 10% oral bioavailability or Caco-2 diffusion (Martin, 2005; Daina et al., 2017).
Human intestinal absorption (HIA) of the compounds was also simulated (Daina and Zoete, 2016). The drug-likeness analysis was carried out using the validated rules used as high-throughput screens filters in leading pharmaceutical companies, including Lipinski (Pfizer), Ghose (Amgen), Veber (GSK), Egan (Pharmacia), and Muegge (Bayer). All rules were evaluated to predict whether a drug is likely to have useful pharmacokinetic properties.
2.15 Statistics
For most of our experiments, and in accordance with most reports using C. elegans, we used a large number of animals per condition in each assay (more than 40–50 animals). This number of animals is large enough to ensure appropriate statistical power in the test used. All the statistical tests were performed after checking normality. We performed the experiments at least 3 or 4 times to ensure reproducibility. All animals were grown in similar conditions and the experiments were performed on different days, with different animal batches. The results presented in each figure are the mean or median of at least three independent assays, as appropriately indicated. The statistical significance was assessed by performing a Student’s t-test, one-way ANOVA followed by a posthoc test, as appropriate. *p < 0.05, **p < 0.01, ***p < 0.001.
3 Results
3.1 Two Derivatives, 1-(3-sulfonatopropyl) Imidazolium and 1-Mesityl-3-(3-Sulfonatopropyl) Imidazolium Induce Protection Against Oxidative Stress
We have recently synthesized imidazolium-derived compounds to evaluate their biological activity in C. elegans. From an initial set of 11 compounds, we identified 2 with anthelmintic potential (Blanco et al., 2018). All other compounds did not alter nematode survival, even at higher concentrations (Blanco et al., 2018). Different biological effects may result from small differences in the molecular structure of heterocyclic compounds, such as imidazole derivatives (Siwach and Verma, 2021). Therefore, it is likely that these compounds have other biological activities. We decided to evaluate their ability to modulate resistance to oxidative stress (OS). Several molecules or processes that increase resistance to oxidative or thermal stress have been shown to decrease proteotoxicity, including that triggered by proteinopathies (Tsunemi et al., 2012; Chakraborty et al., 2013; Kumar and Devaki, 2015).
To improve the likelihood of success in drug development, it is vital to focus the initial screening on compounds with potentially appropriate pharmacokinetic characteristics (Hay et al., 2014). For this reason, in silico prediction of bioavailability and ADME (absorption, distribution, metabolism, and excretion) parameters have become a common practice during the early stages of pharmaceutical development (Dahlin et al., 2015). Hence, first, we used the SwissADME online tool [Swiss Institute of Bioinformatics (SIB)] (Daina et al., 2017; Bakchi et al., 2022) to estimate the bioavailability of five water-soluble imidazole derivatives. The software analysis showed that all the derivatives have a bioavailability score greater than zero, which means that their pharmacokinetic properties are comparable to most drugs with proven effectiveness in humans (Table 1) (Martin et al., 2005; Khan et al., 2018; Masood and Fatima, 2018; Bojarska et al., 2020). In addition, except for compound number 5, all derivatives show high gastrointestinal absorption (Table 1). We also estimated the oral bioavailability radar for these compounds. This measurement qualitatively assesses six physicochemical properties that are relevant for oral bioavailability (lipophilicity, size, polarity, solubility, flexibility, and saturation) and indicates the probability that a compound could be an oral drug candidate. At first glance, we observed that the bioavailability radar for most compounds falls entirely within the region accepted for oral drug physicochemical properties (pink zone, Figure 1A). The high polarity of compound number five slightly distorts its oral bioavailability radar. Nevertheless, even for this compound the estimated area mostly overlaps with the expected one. Since these in silico estimates allow us to glimpse adequate overall bioavailabilities for each of the compounds, we decided to evaluate their pharmacological action. Specifically, we analyzed their effect on the OS resistance of wild-type worms exposed to the oxidizing agent FeSO4. We found that exposure of wild-type worms to 10 μM of compound 1 (1-(3-Sulfonatopropyl) imidazolium (SI)) and compound 3 (1-Mesityl-3-(3-sulfonatopropyl) imidazolium (MSI)) significantly increased their resistance to OS (Figure 1B). In contrast, no statistically significant changes were detected in animals exposed to the other compounds, even at higher concentrations (100 μM) (Figure 1B; Supplementary Figure S1).

TABLE 1. Drug-likeness parameters of the analyzed compounds. Predictions were obtained after introducing SMILES structures in the SwissADME web server. Bioavailability score, Gastrointestinal (GI) adsorption and the Lipinski’s, Ghose’s, Veber’s, Egan’s and Muegge’s rules define the drug-likeness score for each compound.
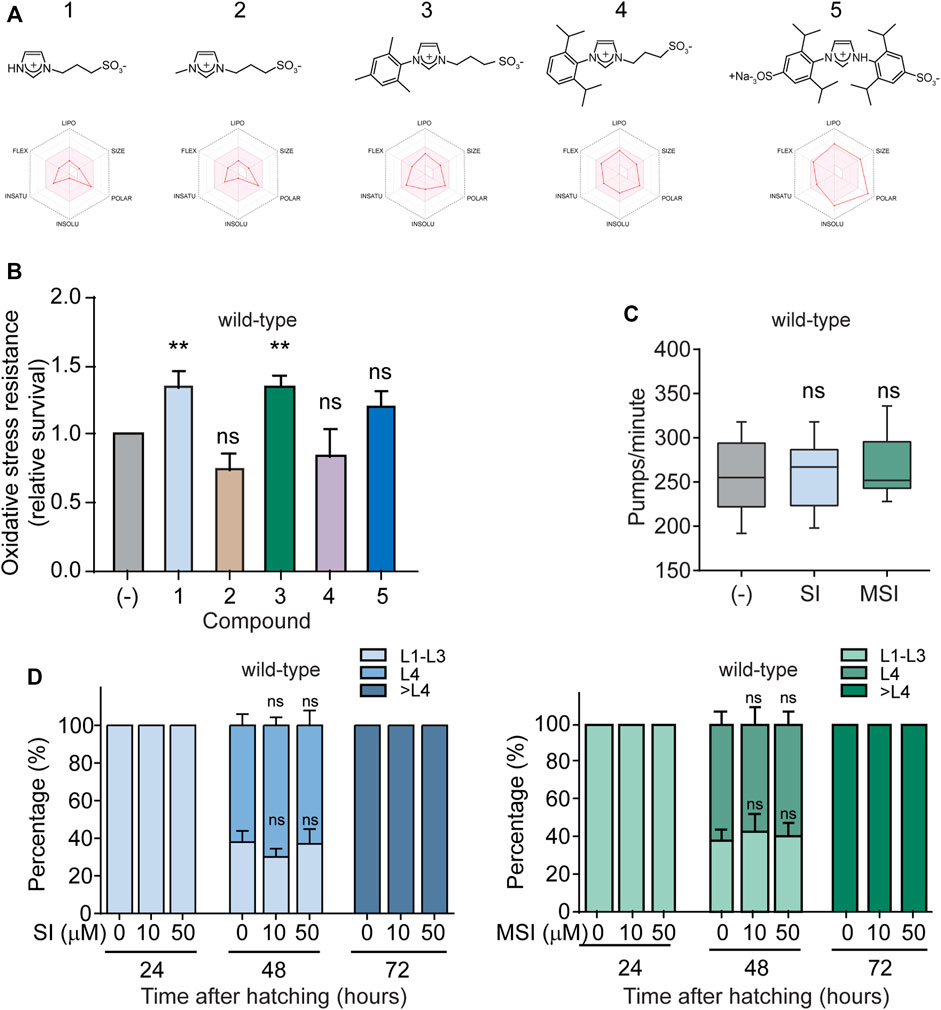
FIGURE 1. Effect of imidazolium salts on oxidative stress resistance in wild-type C. elegans. (A). Chemical structures of imidazolium salts with their corresponding oral bioavailability radar below. The pink area represents the optimal range for each property. Compounds 1: 1-(3-sulfonatopropyl) imidazolium, 2: 1-Methyl-3-(3-sulfonatopropyl) imidazolium, 3: 1-Mesityl-3-(3-sulfonatopropyl) imidazolium, 4: 1-(2,6-Diisopropylphenyl)-3-(3-sulfonatopropyl) imidazolium and 5:1,3-bis(2,6-diisopropyl-4-sodiumsulfonatophenyl) imidazolium. (B). Oxidative stress resistance of wild-type worms in the absence (-) or presence of 10 μM of each imidazolium salt (60–80 animals per condition per experiment, n = 3–8). Oxidative stress was induced by 10 mM FeSO4 for 1 h. Survival was scored immediately after this treatment. Data are normalized to the resistance of wild-type animals in the absence of compound assayed the same day. Data in the bar graph are represented as mean ± SEM. Statistical significance was evaluated by One-way ANOVA followed by Holm–Sidak’s test for multiple comparisons against the control group (-) (**p < 0.01). (C). Pharyngeal pumping rates (pumps per min) of wild-type worms in the absence (-) or presence of 10 μM of SI and MSI (30 animals per condition). Data is represented as a box plot with a line at the median. Statistical analysis of the data was performed with a Kruskal–Wallis one-way ANOVA on ranks. No statistical differences (ns) compared to worms without compound treatment (-). (D). Developmental rate of wild-type worms grown in the absence (-) or presence of 10 and 50 μM of SI (left) and MSI (right). A color code was used to represent each of the following animal stages: L1–L3: early larval stages, L4: last larval stage, > L4: adult stage. Animal classification was evaluated at the indicated time points (24, 48, and 72 h). Data are represented in a stacked bar chart as mean ± SEM. No statistical differences (ns) were found compared to worms without compound treatment (-) (One-way ANOVA for MSI and One-way ANOVA on ranks for SI, n = 4 independent experiments).
3.2 SI and MSI Protective Mechanisms Involve Specific Signaling Molecules
It has been shown that compounds with significant Redox activities could directly react and reduce oxidative molecules, therefore enhancing the resistance to OS (Devagi et al., 2018). Thus, we performed in vitro measurements of the ORP for these derivatives. The values obtained were 67.0 mV for 1, 70.2 mV for 2, 44 mV for 3, 34,7 mV for 4 and 83.5 mV for 5. These positive values indicate that the reduction potential of these compounds is extremely limited suggesting that the enhanced OS resistance induced by compounds 1 and 3 (hereafter referred to as SI and MSI, respectively) is not likely due to direct antioxidant action.
An alternative mechanism conferring stress resistance could be reduced food intake and the consequent activation of dietary restriction programs. Indeed, C. elegans mutants with diminished pharyngeal function exhibit reduced food intake and increased stress resistance and lifespan (Lakowski and Hekimi, 1998). To assess whether these drugs exert their protective effect by modulating feeding behaviors, we analyzed the pharyngeal pumping rate, which is widely used as a proxy for food intake. We did not observe significant changes in pharyngeal pumping rate in the presence of either SI or MSI compared to controls (Figure 1C). Therefore, the enhanced OS resistance found in SI- and MSI-treated animals are probably not due to reduced caloric intake.
A typical trade-off between stress resistance and development rate has been reported throughout the animal kingdom (Sørensen et al., 2003; Broughton et al., 2005; Zakrzewska et al., 2011). In C. elegans, for example, stress-resistant strains generally have a slower development rate (Kenyon et al., 1993). We examined whether these compounds enhance stress resistance by reducing the animal growth rate. As shown in Figure 1D, no significant changes in C. elegans development rate were observed for both compounds.
Taking together these results suggest that the protective effects of both imidazole derivatives could implicate the modulation of specific stress response pathways rather than redox mechanisms, changes in food intake, or growth rate.
We next performed additional in silico studies for both derivatives. In this case, we run the SwissADME web tool to predict drug-likeness. SwissADME analyzes five different rule-based filters, with diverse ranges of properties inside of which the molecule is defined as drug-like (Daina et al., 2017). We assessed these filters for both SI and MSI. As shown in Table 1, drug-likeness is compatible with Lipinski (Lipinski, 2000), Ghose (Ghose et al., 1999), Veber (Veber et al., 2002), and Egan (Egan et al., 2000) rules. However, the Muegge rules (Muegge et al., 2001) are compatible only for MSI, possibly due to the low molecular weight of SI (Table 1). Given that MSI did not present any violation of these parameters, in this work, we focused on the molecular mechanisms underlying the stress-protective effect of this derivative.
3.3 MSI Regulates the HSF-1 Signaling Pathway to Increase Oxidative Stress Resistance
We explored plausible intracellular mechanisms that could explain MSI-mediated OS protection. To this end, we focused on the cytoprotective activity of transcription factors (TFs) that act downstream of the highly conserved DAF-2/IIS such as DAF-16/FOXO, SKN-1/Nrf-2, and HSF-1/HSF1 (Murphy and Hu, 2013). These TFs activate a genetic program that ultimately confers enhanced stress resistance and longevity (Kenyon et al., 1993; Altintas et al., 2016) (Figure 2A).
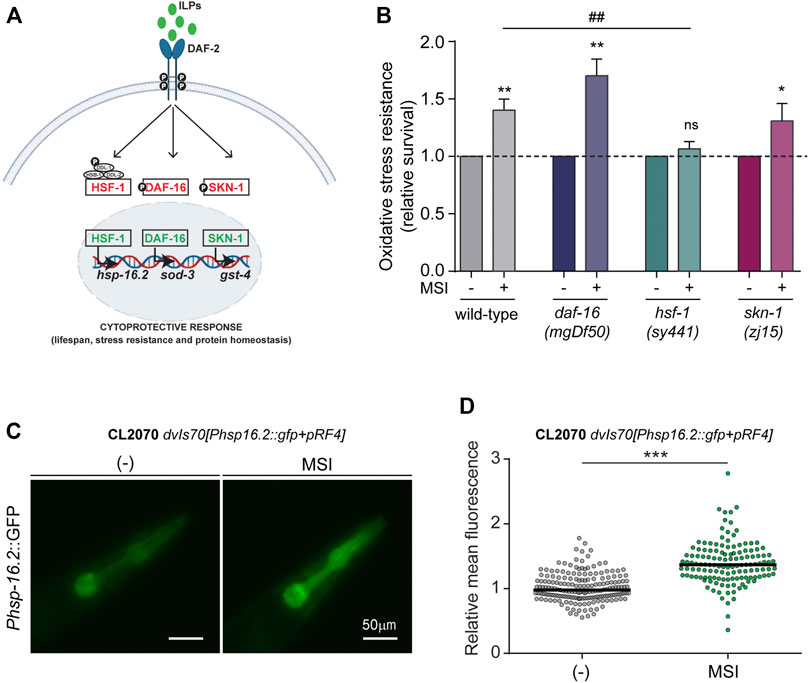
FIGURE 2. Molecular pathways involved in the mechanism of stress resistance of MSI. (A). Schematic diagram showing classical transcription factors involved in the DAF-2/IIS signaling pathway. Activation of the insulin/IGF-1 receptor ortholog, DAF-2 receptor, leads to phosphorylation and cytoplasmatic sequestration of the transcription factors (TFs) DAF-16/FOXO, HSF-1/HSF, and SKN-1/Nrf2. In contrast, downregulation of the DAF-2/IIS signaling permits the nuclearization of these TFs and the triggering of cytoprotective mechanisms. (B). Oxidative stress resistance (10 mM FeSO4, 1 h) of wild-type, GR1307 daf-16(mgDf50), PS3551 hsf-1(sy441), and QV225 skn-1(zj15) mutant animals exposed to MSI. Data are shown as mean ± SEM from at least 4 independent experiments (n = 60–80 animals per experiment per condition). Statistical differences were evaluated by comparison of means (Student’s t-test for daf-16 animals) or medians (Mann-Whitney Rank Sum Test for wild-type and hsf-1 worms). *represents p-value compared with the same strain without MSI and # represents p-value for wild-type and hsf-1 mutant comparison upon MSI exposure (ns, not significant, *p < 0.05, **p < 0.01, ##p < 0.01). (C). Representative fluorescence micrographs depicting the expression of the stress-responsive gene hsp-16.2 in the absence and presence of 50 μM MSI. (D). Quantification of hsp-16.2 expression by measuring fluorescence intensity in the pharynx region. Data are shown in a scatter-dot plot graph, with a horizontal line indicating the median of each dataset, from five independent experiments and 25–40 animals per experiment per condition. Statistical differences were evaluated by Mann-Whitney Rank Sum Test (***p < 0.001).
We analyzed the effect of MSI on the OS resistance of mutant animals with severe deficiencies in these TFs. We first characterized resistance to iron-induced OS in daf-16, hsf-1 and skn-1 mutants in the absence of MSI. Surprisingly, while daf-16 null mutant animals exhibit an expected decline in survival to OS, hypomorphic hsf-1, and skn-1 mutants are not more prone to iron-induced stress (Supplementary Figure S2). Although downregulation of these TFs typically reduces stress resistance, several reports using RNAi silencing or hypomorphic mutants, such as the ones used here, show that resistance to some stress protocols can be unaltered or, moreover, increased (McColl et al., 2010; Baird et al., 2014; Crombie et al., 2016). While these contradictory results could be due to differences in experimental protocols, they suggest that the role of these TFs in stress resistance is much more complex than expected.
As shown in Figure 2B, exposure to 10 μM MSI enhanced OS resistance in daf-16 and skn-1 mutant animals, comparable to the protection observed in wild-type animals. This result suggests that MSI protective effect is independent of these TFs. Accordingly, no induction of basal levels of DAF-16 nuclear translocation (Supplementary Figure S3A) or glutathione S-transferase 4 expression (GST-4, a typical SKN-1 effector often used as a read-out for SKN-1 activity) (Supplementary Figure S3B) were observed even at higher concentrations of MSI (50 μM). It has been reported that certain conditions affecting DAF-16 nuclear translocation or gst-4 expression often require induction of the mechanism by a mild stressor to become apparent (De Rosa et al., 2019). However, we did not observe an increase in the expression of these TFs even under conditions that trigger these processes, such as heat (DAF-16) or juglone exposure (GST-4) (Choe et al., 2009; De Rosa et al., 2019) (Supplementary Figure S3). Moreover, in the latter case, the presence of MSI appears to decrease gst-4 expression (Supplementary Figure S3). These results suggest that DAF-16 and SKN-1 are not implicated in the molecular mechanisms underlying the enhanced stress resistance induced by MSI.
On the other hand, MSI protective effect is abolished in hsf-1 mutants (Figure 2B). To further reinforce these results, we assessed the expression of Heat shock protein-16.2 (HSP-16.2), an HSF-1 downstream effector widely used as a proxy for HSF-1 activation. Through microscopic analysis of transgenic worms containing an hsp-16.2:: Green fluorescent protein (GFP) reporter transgene, we found that MSI exposure increases fluorescence intensity in MSI-treated animals (Figures 2C,D).
Overall, these findings indicate that MSI-induced stress resistance is mediated through the HSF-1 pathway.
3.4 MSI Alleviates Proteotoxic-Associated Phenotypes in Transgenic Animals Expressing Aβ Peptide, α-Synuclein or Poly-Q Repeats
The impaired proteostasis and consequent accumulation of protein aggregates are common features of aging and NDs. It is also reported that both aging and NDs progression are generally associated with high levels of OS biomarkers and low levels of antioxidant defense indicators (Niedzielska et al., 2016; Cenini et al., 2019; Lévy et al., 2019; Volkert and Crowley, 2020). Since OS resistance is largely associated with a major proteostasis capacity, and MSI elicits an HSF-1-dependent cytoprotective mechanism that enhances OS resistance, we next evaluated whether this compound could reduce proteotoxicity in C. elegans models of protein aggregation.
We first investigated whether MSI ameliorates locomotion phenotypes using animals that express the pathological protein involved in Alzheimer Disease (Link, 1995). The C. elegans homolog for the human amyloid precursor protein (APP) gene, apl-1, cannot produce the neurotoxic Aβ peptide (Daigle and Li, 1993). However, Aβ toxicity can be investigated by using a transgenic C. elegans strain expressing human Aβ under the control of a muscle-specific promoter (Link, 1995). As a result of abnormal protein aggregation in the muscle cells, this strain exhibits progressive impairment in mobility and subsequent paralysis (Link, 1995). As shown in Figure 3A, MSI-treated animals exhibit a significant delay in the typical age-dependent paralysis observed in this strain. To further confirm this result, we next performed a thermal-induced paralysis assay, as previously reported (Arya et al., 2009; Regitz et al., 2014). This assay provides an opportunity to measure positive and negative influences in Aβ-induced paralysis. As Figure 3B shows, exposure to MSI (10 and 50 μM) remarkably reduced the number of paralyzed animals upon heat shock. These results demonstrate that MSI can mitigate defects produced by the abnormal aggregation of human Aβ.
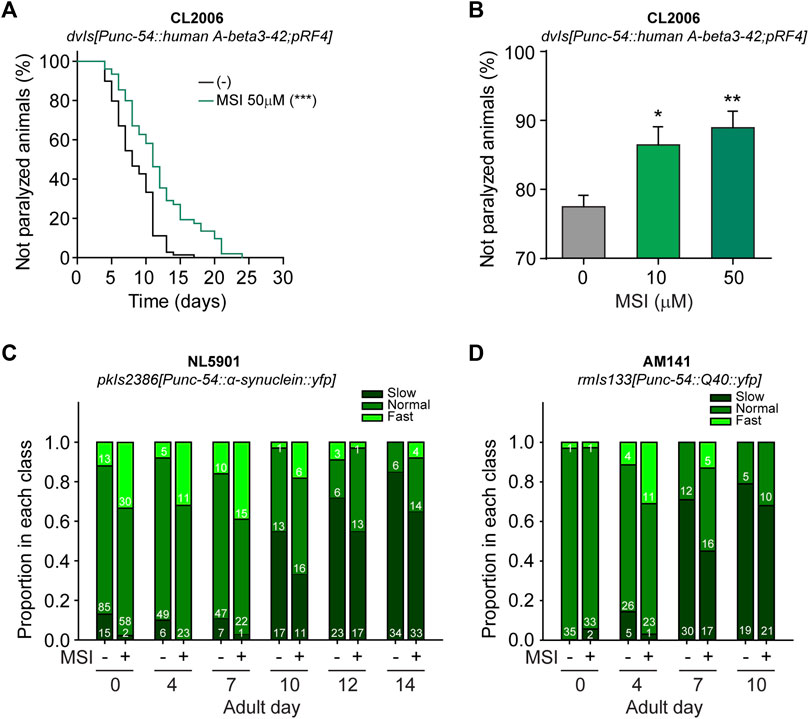
FIGURE 3. Evaluation of MSI impact on proteotoxic-associated phenotypes in C. elegans models of NDs. (A). Influence of MSI on the paralysis of animals expressing human β-amyloid3–42 in muscles throughout its lifetime. Data show a representative Kaplan-Meier curve in the absence and presence of 50 μM MSI. Statistical differences were evaluated by a log-rank test (***p < 0.001). (B). Evaluation of paralysis after a heat shock (35°C for 30 min). Data in the bar graph represent the mean ± SEM from 4 independent experiments with 50–80 animals per condition per experiment. Statistical significance was evaluated by One-way ANOVA followed by Holm–Sidak’s test for multiple comparisons against the control group (0 μM MSI) (*p < 0.05, **p < 0.01). (C,D). Swimming behavior evaluated in C. elegans strains expressing α-synuclein (C) and poly-Q repeats (D) in their body wall muscles. Data were classified into three speed categories based on thrashing rates of young adult animals: normal includes those animals moving at a speed between 25% and 75% of the average thrashing rate at day 0, slow accounts for those animals moving at a speed lower than 25% of average thrashing rate of day 0 adults, and fast comprises those animals moving at a higher speed than 75% of control. Numbers inside bars show the number of animals falling in each category.
As proteotoxicity is a common hallmark of many NDs, we expanded our study to worms expressing proteins whose aggregation causes PD and HD in humans. We examined the effect of MSI in animals expressing α-syn (strain NL5901) or poly-Q (strain AM141) in body-wall muscles. Although it is not possible to directly observe neurodegeneration or specific morphological and/or behavioral neuronal defects on these animals, proteotoxicity in muscles is manifested as age-dependent locomotion defects. Therefore, these strains provide a very easily quantifiable readout to evaluate proteotoxicity in vivo. In addition, since muscle cells are larger than neurons, visualization of protein aggregation is also straightforward. Indeed, these strains expressing the pathological protein in muscles have been widely used to evaluate the anti-proteotoxic potential of different compounds (McColl et al., 2012; Sanchis et al., 2019; Chalorak et al., 2021).
As expected, in both strains we observed an age-dependent locomotion impairment probably due to progressive muscular damage induced by protein aggregation (Supplementary Figure S4). For instance, at adult day 4, the locomotion in liquid medium (measured as the number of thrashes/min) of NL5901 and AM141 is 67% and 77% of that of wild-type worms, respectively (Supplementary Figure S4). This locomotion impairment becomes even greater as the age of the animal increases. On day 10 of adulthood, the liquid locomotion of PD and HD animals decreased to 59% and 38% of wild-type worms of the same age (Supplementary Figure S4).
We found that exposure of NL5109 and AM141 animals to MSI reduces the proportion of animals with severely impaired motility at each of the stages tested (Figures 3C,D). Since MSI does not affect locomotion for wild-type animals, we can rule out that the drug induces hyperactivity (Supplementary Figure S5). We can therefore conclude that MSI delays the movement defects produced by the damage associated with protein aggregation of both α-syn and poly-Q repeats (see also Supplementary Figure S6).
It has been shown that clearing the pathological protein or preventing aggregate formation might be an effective strategy to ameliorate proteotoxic diseases (David et al., 2010; Bieschke, 2013; Cooper et al., 2015). Since the NL5901 and the AM141 strains express α-syn and poly-Q fused to YFP (Yellow fluorescent protein), respectively, it is possible to quantify protein aggregation by measuring mean fluorescence intensity and/or counting the number of protein aggregates (Wolozin et al., 2011).
To analyze α-syn deposition, we quantified fluorescence intensity in the head region of NL5901 animals exposed to MSI. As shown in Figure 4, the mean fluorescence of α-syn deposits was significantly reduced in adult worms (day 7) exposed to MSI (Figure 4A). These results could implicate that the improved motility observed in MSI-treated animals may be due to an inhibition of α-syn protein oligomerization and deposition.
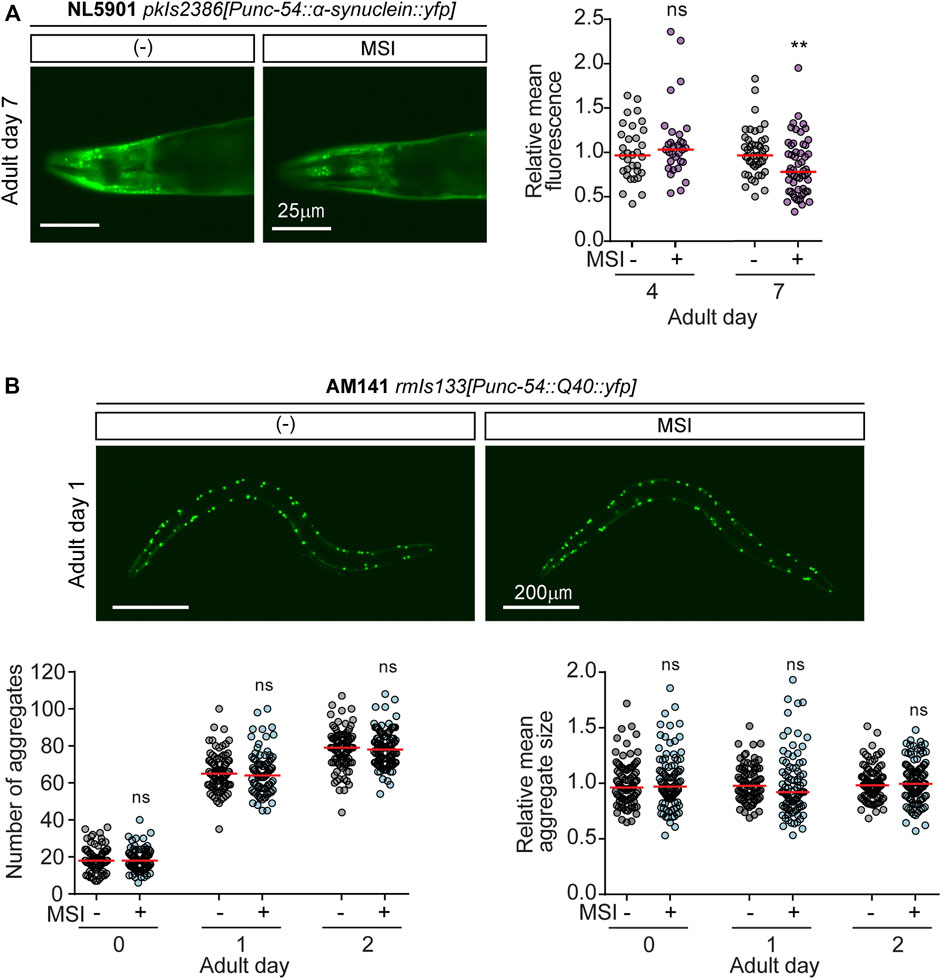
FIGURE 4. MSI effects on protein aggregation in C. elegans models of ND. (A). Quantification of α-synuclein expression levels upon MSI treatment. Left: Representative fluorescence images depicting α-syn::yfp in 7-day adult worms grown in the absence or presence of 50 μM MSI. Right: Relative fluorescence intensity in the head area of the worm at different ages represented in a scatter-dot plot (line at the median). Each dot symbolizes one worm (n = 30–60). Statistical analysis was performed by comparing differences between median values for 0 and 50 μM MSI at each indicated stage (Mann-Whitney Rank Sum Test). Statistical symbols represent **p < 0.01 and ns not significant. (B) Quantification of poly-Q aggregation upon MSI treatment. Top: Representative fluorescence images depicting Q-40::YFP in 1-day adult worms grown in the absence or presence of 50 μM MSI. Bottom: The number of aggregates per animal and their relative mean size at days 0, 1, and 2 of adulthood were quantified using ImageJ FIJI and represented in their corresponding scatter-dot plot (line at the median, n = 60–80). Statistical analysis was performed by comparing differences between the median for 0 vs. 50 μM MSI (Mann-Whitney Rank Sum Test, ns not significant).
The AM141 strain expresses poly-Q::yfp in body muscles (Morley et al., 2002). In these animals, we quantified the number of aggregates at younger stages, since old animals accumulate many aggregates, and their individualization becomes troublesome. Strikingly, MSI does not seem to reduce the number or size of aggregates (Figure 4B). Thus, we can conclude that the improvement in locomotion, is not dependent on an effect on the number or size of poly-Q aggregates.
Taken together, our results indicate that, besides enhancing OS resistance, MSI delays the onset of proteotoxicity-associated phenotypes in worms expressing proteins whose aggregation is pathognomonic of different NDs in humans (Figure 5).
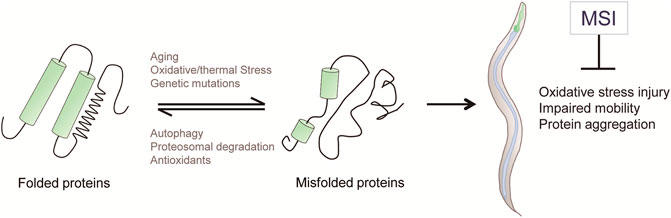
FIGURE 5. Schematic diagram depicting the cytoprotective activities of the imidazolium salt MSI, supporting its role as an antiproteotoxic agent. Cellular protein quality control systems, such as autophagy, proteosome and antioxidants, act to prevent or degrade misfolded proteins. When the capacity of these protective mechanisms is overwhelmed, abnormal proteins can accumulate into toxic protein aggregations that generate cellular damage. MSI reduces oxidative stress injury and pathological phenotypes associated with proteotoxicity accumulation.
4 Discussion
Alzheimer’s disease (AD), Parkinson’s disease (PD), and Huntington’s disease (HD) are considered among the top ten most lethal diseases (G7 Academies’ Joint Statements 2017). Despite exhaustive efforts from the scientific community, there is still no cure and no effective therapeutic strategies to mitigate their deleterious effects. Therefore, there is an urgent need for more innovative approaches to hasten the development of therapies for these disorders.
Invertebrate models, such as C. elegans, have emerged as a convenient strategy, not only to study the molecular basis of certain proteotoxic diseases but also to perform drug screens in a relatively short time frame.
Since the late 1960s, the pharmaceutical industry has used the imidazole nucleus to develop biologically active compounds. Recently, multi-target imidazole derivatives were postulated as candidates for the treatment of NDs (Eliewi et al., 2021). In this work, we found a compound, MSI, that delays the onset of typical locomotor defects in animals over-expressing the proteins that cause AD, HD and PD in humans.
Our results showed that MSI protects wild-type animals against oxidative stress (OS). The increase in stress resistance may be secondary to alterations in general physiological processes. One of the main conserved mechanisms underlying stress resistance and lifespan extension is dietary restriction (Fontana et al., 2010; Swindell, 2012). In C. elegans, feeding depends on a neuromuscular pump, the pharynx, that captures food (bacteria) and pumps it to the intestine. Alterations in pharyngeal pumping lead to reduced food intake, which inhibits the DAF-2/IIS signaling pathway, increasing stress resistance and extending animals’ lifespan (Kenyon et al., 1993). Our results demonstrate that exposure to MSI do not affect pharyngeal pumping. While this suggests that the protective effect is not mediated by reduced food intake, processes acting downstream to pharyngeal pumping may still trigger dietary restriction phenotypes (e.g., a decreased nutrient absorption in the intestine). However, diet restriction has been shown to delay growth and extend the lifespan of a broad range of animals, including C. elegans (Johnson et al., 1984; Vanfleteren and Braeckman, 1999; Greer and Brunet, 2009). The absence of developmental delay in animals exposed to MSI supports the notion that this compound enhances stress resistance without stimulating a typical dietary restriction state.
More than 35 human diseases are associated with protein aggregation and misfolding. Several works reported that cellular and physical stress, such as heat or oxidation, can trigger protein misfolding, unfolding, and aggregation, thus fostering the onset of proteinopathies (Morimoto, 2008; Gidalevitz et al., 2011; Morimoto, 2011; Vasconcellos et al., 2016; Kumsta et al., 2017). In fact, the saturation of endogenous antioxidant defenses was reported as one of the most important factors involved in the pathogenesis and progression of NDs (Jenner, 2003; Blesa et al., 2015; Hasanbašić et al., 2018; Nandi et al., 2019). To get insights into the mechanisms underlying MSI effect, we evaluated the role of three key transcription factors (TF) involved in stress resistance, longevity, and proteostasis from worms to mammals: SKN-1/Nrf-2, DAF-16/FOXO, and HSF-1/HSF1 (Hemdan and Almazan, 2008; Rodriguez et al., 2013; Leja-Szpak et al., 2015). Our results strongly suggest that MSI enhances stress resistance by inducing the HSF-1 pathway. On the other hand, we did not observe evidence of the activation of DAF-16 or SKN-1 upon MSI treatment. Since these 3 TFs are activated when DAF-2 signaling decreases, it is clear that this compound does not produce a general down-regulation of the insulin pathway.
Studies on C. elegans (Servello and Apfeld, 2020) and other organisms such as yeast Saccharomyces cerevisiae (Raitt et al., 2000) and the plant Arabidopsis thaliana (Liu et al., 2011) also highlighted the conserved importance of HSF-1 in resistance to OS. However, it is not clear how MSI induces HSF-1 activity. Since MSI can be considered a mild oxidant, it could be possible that the enhancement of OS resistance depends on an hormetic effect triggered by mild oxidation during animal exposure to the compounds. However, three of the imidazolium compounds exhibit similar ORPs values compared to that of MSI but they do not increase animal stress resistance. Moreover, it has been observed that mild OS induces the activity not only of HSF-1 but also of DAF-16 and SKN-1 (De Rosa et al., 2019). Under this scenario, a specific mechanism for HSF-1 induction is more likely to occur. In this sense, several compounds, such as Geldanamycin, have been proven to indirectly induce HSF-1 by inactivating HSP-90 (Kim et al., 1999). It would be interesting to assess if a similar mechanism underlies the MSI effects.
We also found that MSI delays the onset of locomotion phenotypes in C. elegans expressing α-syn, Aβ peptide or poly-Q in their muscle cells. Moreover, MSI induces the HSF-1 dependent chaperone HSP-16.2, suggesting activation of the heat shock response (HSR). It has been shown in Drosophila models of poly-Q diseases that HSF-1-activating compounds can reduce poly-Q-triggered toxicity through the induction of multiple molecular chaperones (Fujikake et al., 2008). Moreover, several studies in different models have also demonstrated that upregulation of chaperones, either by pharmacological induction or overexpression, decreases protein aggregation toxicity (Muchowski et al., 2000; Sittler et al., 2001; Auluck et al., 2002; Hoshino et al., 2011; Chafekar et al., 2012; Holmes et al., 2014; Nagy et al., 2016). Similarly, it is possible that MSI up-regulates HSF-1-dependent chaperones involved in the HSR, leading to decreased proteotoxicity and delayed onset of locomotion phenotypes in C. elegans models of protein aggregation. Supporting this idea, high levels of HSP-16.2 promote the sequestration, degradation, or refolding of abnormal oligomers, thus suppressing in vivo toxicity of the Aβ peptide in worms (Fonte et al., 2008). Since C. elegans does not naturally produce the amyloid peptide, it is possible that this chaperone could affect the multimerization/aggregation pathways of many proteins that can potentially form toxic oligomers (Fonte et al., 2008). This general function of HSR chaperones could explain the general anti-proteotoxic effect of MSI regardless of the protein causing the proteotoxicity.
Beyond chaperones induction, activation of HSF-1 can increase stress resistance by stimulation of non-classical effectors such as Aspartyl protease-4 (ASP-4)/Cathepsin and Death-associated protein kinase-1 (DAPK-1)/DAPK (Servello and Apfeld, 2020). ASP-4 is an aspartyl protease related to cathepsins D and E that have been implicated in AD (Syntichaki et al., 2002). Likewise, RNAi knockdown of asp-4 in C. elegans enhanced the paralysis rate and caused higher Aβ accumulation (Florez-McClure et al., 2007). Therefore, MSI effects could also involve pathways not related to classical chaperone induction.
As expected, PD animals treated with MSI show a significant decrease in α-syn aggregation. Strikingly, in animals expressing poly-Q repeats in their muscles, neither the number of aggregates nor their size is significantly altered by MSI treatment, suggesting that locomotion improvement is not dependent on poly-Q aggregate number/size. This paradox can be due to methodological limitations since many aggregation intermediates cannot be resolved (Cooper et al., 2018). However, it has been shown that the structure and solubility of the aggregates could be more critical for proteotoxicity than their number or size (Xi et al., 2016; Chiti and Dobson, 2017; De et al., 2019). For example, some oligomers could expose hydrophobic residues and unpaired structures making them highly interactive with other proteins, with critical components of the proteasome, and even with membranes (Kayed et al., 2003; Olzscha et al., 2011; Winner et al., 2011; Kim et al., 2016; Woerner et al., 2016). Moreover, large fibrillar aggregate deposits do not always correlate with disease onset or severity (Kayed et al., 2003; Leitman et al., 2013; Chiti and Dobson, 2017). In many cases, large amyloid aggregates exert a protective effect by sequestering the more-toxic oligomeric species (Saudou et al., 1998; Arrasate et al., 2004; Douglas et al., 2009; Kim et al., 2016). Thus, it is possible that while aggregation still occurs in MSI-treated animals (or changes cannot be experimentally detected), these aggregates are less proteotoxic, leading to the delayed onset of locomotion defects.
In conclusion, our work demonstrated that a water-soluble imidazole derivative, MSI, protects wild-type worms against OS and reduces proteotoxicity in C. elegans protein aggregation models. Despite the differences in the proteins that form aggregates among ND diseases, MSI evokes a general protective mechanism that prevents the formation or helps the degradation of more harmful aggregates. This mechanism involves the activation of the HSF-1 pathway.
Taking advantage of the biological versatility of imidazole compounds and the power of C. elegans to model human diseases, we have identified MSI as a potential therapeutic agent that may delay proteotoxicity in vivo. It is now necessary to test whether this protective effect observed in C. elegans could be extended into vertebrate models of proteinopathies.
Data Availability Statement
The original contributions presented in the study are included in the article/Supplementary Material, further inquiries can be directed to the corresponding authors.
Author Contributions
NA, DR, and MJDR designed and conceived the study. NA, TV, MB, and GS acquired the data. NA, TV, MB, and GS analyzed and interpreted the data. NA, TV, and MB performed the statistical analysis. NA, DR, and MJDR drafted the manuscript. DR and MJDR supervised the study and obtained funding.
Funding
This work was supported by Grants from: 1) Universidad Nacional Del Sur to DR (PGI: 24/B291), MJDR (PGI: 24/B261) and GS (PGI: 24/Q108); 2) Agencia Nacional de Promoción de la Ciencia y la Tecnología ANPCYT Argentina to DR (PICT 2019-0480), MJDR (PICT-2017-0566 and PICT-2020-1734), TV (PICT-2018-03164) and NA (PICT-2020-01826) and Consejo Nacional de Investigaciones Científicas y Técnicas, Argentina to DR, MJDR, TV, and NA (PIPNo. 11220200101606CO). The funders had no role in the study design, data collection, and analysis, decision to publish, or preparation of the manuscript.
Conflict of Interest
The authors declare that the research was conducted in the absence of any commercial or financial relationships that could be construed as a potential conflict of interest.
Publisher’s Note
All claims expressed in this article are solely those of the authors and do not necessarily represent those of their affiliated organizations, or those of the publisher, the editors and the reviewers. Any product that may be evaluated in this article, or claim that may be made by its manufacturer, is not guaranteed or endorsed by the publisher.
Acknowledgments
Some strains were provided by the CGC, which is funded by the NIH Office of Research Infrastructure Programs (P40 OD010440).
Supplementary Material
The Supplementary Material for this article can be found online at: https://www.frontiersin.org/articles/10.3389/fphar.2022.908696/full#supplementary-material
Abbreviations
ND, Neurodegenerative diseases; AD, Alzheimer’s disease; PD, Parkinson’s disease; HD, Huntington’s disease; MSI, 1-Mesityl-3-(3-sulfonatopropyl) imidazolium; SI, 1-(3-Sulfonatopropyl) imidazolium; HSF-1, heat shock transcription factor; DAF-16/FOXO, DAF-16/ ortholog of human Forkhead box protein O; SKN-1/Nrf2, transcription factor skinhead-1/ ortholog of human NF-E2-related factor 2 Nrf2; DAF-2/IIS, DAF-2/ insulin/insulin-like growth factor 1 signaling; TFs, transcription factors; Aβ, Amyloid-beta; α-syn, Alpha-synuclein; OS, oxidative stress; GST-4, Glutathione S-transferase 4; HSP-16.2, Heat shock protein hsp-16.2; poly-Q, Polyglutamine; ORP, oxidation-reduction potential.
References
Agotegaray, M., Blanco, M. G., Campelo, A., García, E., Zysler, R., Massheimer, V., et al. (2020). β-Cyclodextrin Coating: Improving Biocompatibility of Magnetic Nanocomposites for Biomedical Applications. J. Mater Sci. Mater Med. 31 (2), 22. doi:10.1007/s10856-020-6361-4
Altintas, O., Park, S., and Lee, S. J. (2016). The Role of insulin/IGF-1 Signaling in the Longevity of Model Invertebrates, C. elegans and D. melanogaster. BMB Rep. 49 (2), 81–92. doi:10.5483/bmbrep.2016.49.2.261
Anckar, J., and Sistonen, L. (2011). Regulation of HSF1 Function in the Heat Stress Response: Implications in Aging and Disease. Annu. Rev. Biochem. 80, 1089–1115. doi:10.1146/annurev-biochem-060809-095203
Anderson, E. B., and Long, T. E. (2010). Imidazole- and Imidazolium-Containing Polymers for Biology and Material Science Applications. Polymer 51, 2447–2454. doi:10.1016/j.polymer.2010.02.006
Arrasate, M., Mitra, S., Schweitzer, E. S., Segal, M. R., and Finkbeiner, S. (2004). Inclusion Body Formation Reduces Levels of Mutant Huntingtin and the Risk of Neuronal Death. Nature 431 (7010), 805–810. doi:10.1038/nature02998
Arya, U., Dwivedi, H., and Subramaniam, J. R. (2009). Reserpine Ameliorates Abeta Toxicity in the Alzheimer's Disease Model in Caenorhabditis elegans. Exp. Gerontol. 44, 462–466. doi:10.1016/j.exger.2009.02.010
Auluck, P. K., Chan, H. Y., Trojanowski, J. Q., Lee, V. M., and Bonini, N. M. (2002). Chaperone Suppression of Alpha-Synuclein Toxicity in a Drosophila Model for Parkinson's Disease. Science 295 (5556), 865–868. doi:10.1126/science.1067389
Baird, N. A., Douglas, P. M., Simic, M. S., Grant, A. R., Moresco, J. J., Wolff, S. C., et al. (2014). HSF-1-mediated Cytoskeletal Integrity Determines Thermotolerance and Life Span. Science 346 (6207), 360–363. doi:10.1126/science.1253168
Bakchi, B., Krishna, A. D., Sreecharan, E., Ganesh, V. B. J., Niharika, M., Maharshi, S., et al. (2022). An Overview on Applications of SwissADME Web Tool in the Design and Development of Anticancer, Antitubercular and Antimicrobial Agents: A Medicinal Chemist's Perspective. J. Mol. Struct. 1259, 132712. doi:10.1016/j.molstruc.2022.132712
Bénard, C., and Doitsidou, M. (2016). Nervous System Ageing. Springer, 163–189. doi:10.1007/978-3-319-44703-2_8
Bénard, C., and Hobert, O. (2009). Looking beyond Development: Maintaining Nervous System Architecture. Curr. Top. Dev. Biol. 87, 175–194. doi:10.1016/s0070-2153(09)01206-x
Bieschke, J. (2013). Natural Compounds May Open New Routes to Treatment of Amyloid Diseases. Neurotherapeutics 10 (3), 429–439. doi:10.1007/s13311-013-0192-7
Blanco, M. G., Vela Gurovic, M. S., Silbestri, G. F., Garelli, A., Giunti, S., Rayes, D., et al. (2018). Diisopropylphenyl-imidazole (DII): A New Compound that Exerts Anthelmintic Activity through Novel Molecular Mechanisms. PLoS Negl. Trop. Dis. 12 (12), e0007021. doi:10.1371/journal.pntd.0007021
Blesa, J., Trigo-Damas, I., Quiroga-Varela, A., and Jackson-Lewis, V. R. (2015). Oxidative Stress and Parkinson's Disease. Front. Neuroanat. 9, 91. doi:10.3389/fnana.2015.00091
Bojarska, J., Remko, M., Breza, M., Madura, I. D., Kaczmarek, K., Zabrocki, J., et al. (2020). A Supramolecular Approach to Structure-Based Design with A Focus on Synthons Hierarchy in Ornithine-Derived Ligands: Review, Synthesis, Experimental and In Silico Studies. Molecules 25 (5). doi:10.3390/molecules25051135
Brenner, S. (1974). The Genetics of Caenorhabditis elegans. Genetics 77 (1), 71–94. doi:10.1093/genetics/77.1.71
Brignull, H. R., Moore, F. E., Tang, S. J., and Morimoto, R. I. (2006). Polyglutamine Proteins at the Pathogenic Threshold Display Neuron-specific Aggregation in a Pan-Neuronal Caenorhabditis elegans Model. J. Neurosci. 26 (29), 7597–7606. doi:10.1523/jneurosci.0990-06.2006
Broughton, S. J., Piper, M. D., Ikeya, T., Bass, T. M., Jacobson, J., Driege, Y., et al. (2005). Longer Lifespan, Altered Metabolism, and Stress Resistance in Drosophila from Ablation of Cells Making Insulin-like Ligands. Proc. Natl. Acad. Sci. U. S. A. 102(8), 3105–3110. doi:10.1073/pnas.0405775102
Bucciantini, M., Giannoni, E., Chiti, F., Baroni, F., Formigli, L., Zurdo, J., et al. (2002). Inherent Toxicity of Aggregates Implies a Common Mechanism for Protein Misfolding Diseases. Nature 416 (6880), 507–511. doi:10.1038/416507a
Buckingham, S. D., and Sattelle, D. B. (2009). Fast, Automated Measurement of Nematode Swimming (Thrashing) without Morphometry. BMC Neurosci. 10, 84. doi:10.1186/1471-2202-10-84
Burke, S. N., and Barnes, C. A. (2006). Neural Plasticity in the Ageing Brain. Nat. Rev. Neurosci. 7 (1), 30–40. doi:10.1038/nrn1809
Cenini, G., Lloret, A., and Cascella, R. (2019). Oxidative Stress in Neurodegenerative Diseases: From a Mitochondrial Point of View. Oxid. Med. Cell Longev. 2019, 2105607. doi:10.1155/2019/2105607
Chafekar, S. M., Wisén, S., Thompson, A. D., Echeverria, A., Walter, G. M., Evans, C. G., et al. (2012). Pharmacological Tuning of Heat Shock Protein 70 Modulates Polyglutamine Toxicity and Aggregation. ACS Chem. Biol. 7 (9), 1556–1564. doi:10.1021/cb300166p
Chakraborty, S., Bornhorst, J., Nguyen, T. T., and Aschner, M. (2013). Oxidative Stress Mechanisms Underlying Parkinson's Disease-Associated Neurodegeneration in C. elegans. Int. J. Mol. Sci. 14 (11), 23103–23128. doi:10.3390/ijms141123103
Chalfie, M., Tu, Y., Euskirchen, G., Ward, W. W., and Prasher, D. C. (1994). Green Fluorescent Protein as a Marker for Gene Expression. Science 263 (5148), 802–805. doi:10.1126/science.8303295
Chalorak, P., Sanguanphun, T., Limboonreung, T., and Meemon, K. (2021). Neurorescue Effects of Frondoside A and Ginsenoside Rg3 in C. elegans Model of Parkinson's Disease. Molecules 26 (16), 4843. doi:10.3390/molecules26164843
Chiti, F., and Dobson, C. M. (2017). Protein Misfolding, Amyloid Formation, and Human Disease: A Summary of Progress over the Last Decade. Annu. Rev. Biochem. 86, 27–68. doi:10.1146/annurev-biochem-061516-045115
Choe, K. P., Przybysz, A. J., and Strange, K. (2009). The WD40 Repeat Protein WDR-23 Functions with the CUL4/DDB1 Ubiquitin Ligase to Regulate Nuclear Abundance and Activity of SKN-1 in Caenorhabditis elegans. Mol. Cell Biol. 29 (10), 2704–2715. MCB.01811-08 [pii];. doi:10.1128/MCB.01811-08
Chung, S. H., Sun, L., and Gabel, C. V. (2013). In Vivo neuronal Calcium Imaging in C. elegans. J. Vis. Exp. 74. doi:10.3791/50357
Cohen, E., Bieschke, J., Perciavalle, R. M., Kelly, J. W., and Dillin, A. (2006). Opposing Activities Protect against Age-Onset Proteotoxicity. Science 313 (5793), 1604–1610. 1124646 [pii]. doi:10.1126/science.1124646
Cohen, E., and Dillin, A. (2008). The Insulin Paradox: Aging, Proteotoxicity and Neurodegeneration. Nat. Rev. Neurosci. 9 (10), 759–767. doi:10.1038/nrn2474
Cook, S. J., Jarrell, T. A., Brittin, C. A., Wang, Y., Bloniarz, A. E., Yakovlev, M. A., et al. (2019). Whole-animal Connectomes of Both Caenorhabditis elegans Sexes. Nature 571 (7763), 63–71. doi:10.1038/s41586-019-1352-7
Cooper, J. F., Dues, D. J., Spielbauer, K. K., Machiela, E., Senchuk, M. M., and Van Raamsdonk, J. M. (2015). Delaying Aging Is Neuroprotective in Parkinson's Disease: a Genetic Analysis in C. elegans Models. NPJ Park. Dis. 1, 15022. doi:10.1038/npjparkd.2015.22
Cooper, J. F., Spielbauer, K. K., Senchuk, M. M., Nadarajan, S., Colaiácovo, M. P., and Van Raamsdonk, J. M. (2018). α-Synuclein Expression from a Single Copy Transgene Increases Sensitivity to Stress and Accelerates Neuronal Loss in Genetic Models of Parkinson's Disease. Exp. Neurol. 310, 58–69. doi:10.1016/j.expneurol.2018.09.001
Crombie, T. A., Tang, L., Choe, K. P., and Julian, D. (2016). Inhibition of the Oxidative Stress Response by Heat Stress in Caenorhabditis elegans. J. Exp. Biol. 219 (Pt 14), 2201–2211. doi:10.1242/jeb.135327
Dahlin, J. L., Inglese, J., and Walters, M. A. (2015). Mitigating Risk in Academic Preclinical Drug Discovery. Nat. Rev. Drug Discov. 14 (4), 279–294. doi:10.1038/nrd4578
Daigle, I., and Li, C. (1993). apl-1, a Caenorhabditis elegans Gene Encoding a Protein Related to the Human Beta-Amyloid Protein Precursor. Proc. Natl. Acad. Sci. U. S. A. 90 (24), 12045–12049. doi:10.1073/pnas.90.24.12045
Daina, A., Michielin, O., and Zoete, V. (2017). SwissADME: a Free Web Tool to Evaluate Pharmacokinetics, Drug-Likeness and Medicinal Chemistry Friendliness of Small Molecules. Sci. Rep. 7, 42717. doi:10.1038/srep42717
Daina, A., and Zoete, V. (2016). A BOILED-Egg to Predict Gastrointestinal Absorption and Brain Penetration of Small Molecules. ChemMedChem 11 (11), 1117–1121. doi:10.1002/cmdc.201600182
David, D. C., Ollikainen, N., Trinidad, J. C., Cary, M. P., Burlingame, A. L., and Kenyon, C. (2010). Widespread Protein Aggregation as an Inherent Part of Aging in C. elegans. PLoS Biol. 8 (8), e1000450. doi:10.1371/journal.pbio.1000450
De Rosa, M. J., Veuthey, T., Florman, J., Grant, J., Blanco, M. G., Andersen, N., et al. (2019). The Flight Response Impairs Cytoprotective Mechanisms by Activating the Insulin Pathway. Nature 573 (7772), 135–138. doi:10.1038/s41586-019-1524-5
De, S., Wirthensohn, D. C., Flagmeier, P., Hughes, C., Aprile, F. A., Ruggeri, F. S., et al. (2019). Different Soluble Aggregates of Aβ42 Can Give Rise to Cellular Toxicity through Different Mechanisms. Nat. Commun. 10 (1), 1541. doi:10.1038/s41467-019-09477-3
Devagi, G., Mohankumar, A., Shanmugam, G., Nivitha, S., Dallemer, F., Kalaivani, P., et al. (2018). Organoruthenium(II) Complexes Ameliorates Oxidative Stress and Impedes the Age Associated Deterioration in Caenorhabditis elegans through JNK-1/DAF-16 Signalling. Sci. Rep. 8 (1), 7688. doi:10.1038/s41598-018-25984-7
Douglas, P. M., Summers, D. W., Ren, H. Y., and Cyr, D. M. (2009). Reciprocal Efficiency of RNQ1 and Polyglutamine Detoxification in the Cytosol and Nucleus. Mol. Biol. Cell 20 (19), 4162–4173. doi:10.1091/mbc.e09-02-0170
Egan, W. J., Merz, K. M., and Baldwin, J. J. (2000). Prediction of Drug Absorption Using Multivariate Statistics. J. Med. Chem. 43 (21), 3867–3877. doi:10.1021/jm000292e
Eliewi, A. G., Al-Garawi, Z. S., Al-Kazzaz, F. F., and Atia, A. J. K. (2021). Multi Target-Directed Imidazole Derivatives for Neurodegenerative Diseases. J. Phys. Conf. Ser. 1853, 012066. doi:10.1088/1742-6596/1853/1/012066
Fjell, A. M., and Walhovd, K. B. (2010). Structural Brain Changes in Aging: Courses, Causes and Cognitive Consequences. Rev. Neurosci. 21 (3), 187–221. doi:10.1515/revneuro.2010.21.3.187
Florez-McClure, M. .L., Hohsfield, L. A., Fonte, G., Bealor, M. T., and Link, C. D. (2007). Decreased Insulin-Receptor Signaling Promotes the Autophagic Degradation of Beta-Amyloid Peptide in C. elegans. Autophagy 3 (6), 569–580. doi:10.4161/auto.4776
Fontana, L., Partridge, L., and Longo, V. D. (2010). Extending Healthy Life Span-Ffrom Yeast to Humans. Science 328 (5976), 321–326. doi:10.1126/science.1172539
Fonte, V., Kipp, D. R., Yerg, J., Merin, D., Forrestal, M., Wagner, E., et al. (2008). Suppression of In Vivo Beta-Amyloid Peptide Toxicity by Overexpression of the HSP-16.2 Small Chaperone Protein. J. Biol. Chem. 283 (2), 784–791. doi:10.1074/jbc.M703339200
Fu, M. H., Wu, C. W., Lee, Y. C., Hung, C. Y., Chen, I. C., and Wu, K. L. H. (2018). Nrf2 Activation Attenuates the Early Suppression of Mitochondrial Respiration Due to the α-synuclein Overexpression. Biomed. J. 41 (3), 169–183. doi:10.1016/j.bj.2018.02.005
Fujikake, N., Nagai, Y., Popiel, H. A., Okamoto, Y., Yamaguchi, M., and Toda, T. (2008). Heat Shock Transcription Factor 1-activating Compounds Suppress Polyglutamine-Induced Neurodegeneration through Induction of Multiple Molecular Chaperones. J. Biol. Chem. 283 (38), 26188–26197. doi:10.1074/jbc.M710521200
Fujimoto, M., Takaki, E., Hayashi, T., Kitaura, Y., Tanaka, Y., Inouye, S., et al. (2005). Active HSF1 Significantly Suppresses Polyglutamine Aggregate Formation in Cellular and Mouse Models. J. Biol. Chem. 280 (41), 34908–34916. doi:10.1074/jbc.M506288200
Gaba, M., Singh, S., and Mohan, C. (2014). Benzimidazole: an Emerging Scaffold for Analgesic and Anti-inflammatory Agents. Eur. J. Med. Chem. 76, 494–505. doi:10.1016/j.ejmech.2014.01.030
Ghose, A. K., Viswanadhan, V. N., and Wendoloski, J. J. (1999). A Knowledge-Based Approach in Designing Combinatorial or Medicinal Chemistry Libraries for Drug Discovery. 1. A Qualitative and Quantitative Characterization of Known Drug Databases. J. Comb. Chem. 1 (1), 55–68. doi:10.1021/cc9800071
Gidalevitz, T., Krupinski, T., Garcia, S., and Morimoto, R. I. (2009). Destabilizing Protein Polymorphisms in the Genetic Background Direct Phenotypic Expression of Mutant SOD1 Toxicity. PLoS Genet. 5 (3), e1000399. doi:10.1371/journal.pgen.1000399
Gidalevitz, T., Prahlad, V., and Morimoto, R. I. (2011), The Stress of Protein Misfolding: from Single Cells to Multicellular Organisms, Cold Spring Harb. Perspect. Biol., 3, 6. doi:10.1101/cshperspect.a009704
Gitler, A. D., Dhillon, P., and Shorter, J. (2017). Neurodegenerative Disease: Models, Mechanisms, and a New Hope. Dis. Model Mech. 10 (5), 499–502. doi:10.1242/dmm.030205
Giunti, S., Andersen, N., Rayes, D., and De Rosa, M. J. (2021). Drug Discovery: Insights from the Invertebrate Caenorhabditis elegans. Pharmacol. Res. Perspect. 9 (2), e00721. doi:10.1002/prp2.721
Greer, E. L., and Brunet, A. (2009). Different Dietary Restriction Regimens Extend Lifespan by Both Independent and Overlapping Genetic Pathways in C. elegans. Aging Cell 8 (2), 113–127. doi:10.1111/j.1474-9726.2009.00459.x
Hasanbašić, S., Jahić, A., Berbić, S., Žnidarič, M. T., and Žerovnik, E. (2018). Inhibition of Protein Aggregation by Several Antioxidants. Oxid. Med. Cell Longev. 2018, 8613209. doi:10.1155/2018/8613209
Hay, M., Thomas, D. W., Craighead, J. L., Economides, C., and Rosenthal, J. (2014). Clinical Development Success Rates for Investigational Drugs. Nat. Biotechnol. 32 (1), 40–51. doi:10.1038/nbt.2786
He, Q., Song, N., Jia, F., Xu, H., Yu, X., Xie, J., et al. (2013). Role of α-synuclein Aggregation and the Nuclear Factor E2-Related Factor 2/heme Oxygenase-1 Pathway in Iron-Induced Neurotoxicity. Int. J. Biochem. Cell Biol. 45 (6), 1019–1030. doi:10.1016/j.biocel.2013.02.012
Hemdan, S., and Almazan, G. (2008). Dopamine-induced Toxicity Is Synergistically Potentiated by Simultaneous HSP-90 and Akt Inhibition in Oligodendrocyte Progenitors. J. Neurochem. 105 (4), 1223–1234. doi:10.1111/j.1471-4159.2008.05227.x
Hesp, K., Smant, G., and Kammenga, J. E. (2015). Caenorhabditis elegans DAF-16/FOXO Transcription Factor and its Mammalian Homologs Associate with Age-Related Disease. Exp. Gerontol. 72, 1–7. doi:10.1016/j.exger.2015.09.006
Hess, M., Gomariz, A., Goksel, O., and Ewald, C. Y. (2019). In-Vivo Quantitative Image Analysis of Age-Related Morphological Changes of C. elegans Neurons Reveals a Correlation between Neurite Bending and Novel Neurite Outgrowths. eNeuro 6 (4). doi:10.1523/eneuro.0014-19.2019
Holmes, B. B., Furman, J. L., Mahan, T. E., Yamasaki, T. R., Mirbaha, H., Eades, W. C., et al. (2014). Proteopathic Tau Seeding Predicts Tauopathy In Vivo. Proc. Natl. Acad. Sci. U. S. A. 111 (41), E4376–E4385. doi:10.1073/pnas.1411649111
Hoshino, T., Murao, N., Namba, T., Takehara, M., Adachi, H., Katsuno, M., et al. (2011). Suppression of Alzheimer's Disease-Related Phenotypes by Expression of Heat Shock Protein 70 in Mice. J. Neurosci. 31 (14), 5225–5234. doi:10.1523/jneurosci.5478-10.2011
Hsu, A. L., Murphy, C. T., and Kenyon, C. (2003). Regulation of Aging and Age-Related Disease by DAF-16 and Heat-Shock Factor. Science 300 (5622), 1142–1145. 300/5622/1142 [pii]. doi:10.1126/science.1083701
Huang, Y., and Mucke, L. (2012). Alzheimer Mechanisms and Therapeutic Strategies. Cell 148 (6), 1204–1222. doi:10.1016/j.cell.2012.02.040
Jadiya, P., Fatima, S., Baghel, T., Mir, S. S., and Nazir, A. (2016). A Systematic RNAi Screen of Neuroprotective Genes Identifies Novel Modulators of Alpha-Synuclein-Associated Effects in Transgenic Caenorhabditis elegans. Mol. Neurobiol. 53 (9), 6288–6300. pii. doi:10.1007/s12035-015-9517-3
Jadiya, P., Khan, A., Sammi, S. R., Kaur, S., Mir, S. S., and Nazir, A. (2011). Anti-Parkinsonian Effects of Bacopa Monnieri: Insights from Transgenic and Pharmacological Caenorhabditis elegans Models of Parkinson's Disease. Biochem. Biophys. Res. Commun. 413 (4), 605–610. doi:10.1016/j.bbrc.2011.09.010
Jenner, P. (2003). Oxidative Stress in Parkinson's Disease. Ann. Neurol. 53 (Suppl. 3), S26–S28. doi:10.1002/ana.10483
Jiang, M., Wang, J., Fu, J., Du, L., Jeong, H., West, T., et al. (2011). Neuroprotective Role of Sirt1 in Mammalian Models of Huntington's Disease through Activation of Multiple Sirt1 Targets. Nat. Med. 18 (1), 153–158. doi:10.1038/nm.2558
Johnson, T. E., Mitchell, D. H., Kline, S., Kemal, R., and Foy, J. (1984). Arresting Development Arrests Aging in the Nematode Caenorhabditis elegans. Mech. Ageing Dev. 28 (1), 23–40. doi:10.1016/0047-6374(84)90150-7
Jones, A. K., Rayes, D., Al-Diwani, A., Maynard, T. P., Jones, R., Hernando, G., et al. (2011). A Cys-Loop Mutation in the Caenorhabditis elegans Nicotinic Receptor Subunit UNC-63 Impairs but Does Not Abolish Channel Function. J. Biol. Chem. 286 (4), 2550–2558. doi:10.1074/jbc.M110.177238
Kaletta, T., and Hengartner, M. O. (2006). Finding Function in Novel Targets: C. elegans as a Model Organism. Nat. Rev. Drug Discov. 5 (5), 387–398. doi:10.1038/nrd2031
Kang, C., and Avery, L. (2009). Systemic Regulation of Starvation Response in Caenorhabditis elegans. Genes Dev. 23 (1), 12–17. doi:10.1101/gad.1723409
Kayed, R., Head, E., Thompson, J. L., McIntire, T. M., Milton, S. C., Cotman, C. W., et al. (2003). Common Structure of Soluble Amyloid Oligomers Implies Common Mechanism of Pathogenesis. Science 300 (5618), 486–489. doi:10.1126/science.1079469
Kenyon, C., Chang, J., Gensch, E., Rudner, A., and Tabtiang, R. (1993). A C. elegans Mutant that Lives Twice as Long as Wild Type. Nature 366 (6454), 461–464. doi:10.1038/366461a0
Kerr, R. A., and Schafer, W. R. (2006). Intracellular Ca2+ Imaging in C. elegans. Methods Mol. Biol. 351, 253–264. doi:10.1385/1-59745-151-7:253
Khan, T., Ahmad, R., Azad, I., Raza, S., Joshi, S., and Khan, A. R. (2018). Computer-aided Drug Design and Virtual Screening of Targeted Combinatorial Libraries of Mixed-Ligand Transition Metal Complexes of 2-butanone Thiosemicarbazone. Comput. Biol. Chem. 75, 178–195. doi:10.1016/j.compbiolchem.2018.05.008
Kim, H. R., Kang, H. S., and Kim, H. D. (1999). Geldanamycin Induces Heat Shock Protein Expression through Activation of HSF1 in K562 Erythroleukemic Cells. IUBMB Life 48 (4), 429–433. doi:10.1080/713803536
Kim, Y., Park, J. H., Lee, H., and Nam, J. M. (2016). How Do the Size, Charge and Shape of Nanoparticles Affect Amyloid β Aggregation on Brain Lipid Bilayer? Sci. Rep. 6 (1), 19548. doi:10.1038/srep19548
Kumar, M., and Devaki, T. (2015). Geraniol, a Component of Plant Essential Oils-A Review of its Pharmacological Activities. Int. J. Pharm. Pharm. Sci. 7, 67–70.
Kumsta, C., Chang, J. T., Schmalz, J., and Hansen, M. (2017). Hormetic Heat Stress and HSF-1 Induce Autophagy to Improve Survival and Proteostasis in C. elegans. Nat. Commun. 8, 14337. doi:10.1038/ncomms14337
Lakowski, B., and Hekimi, S. (1998). The Genetics of Caloric Restriction in Caenorhabditis elegans. Proc. Natl. Acad. Sci. U. S. A. 95 (22), 13091–13096. doi:10.1073/pnas.95.22.13091
Lakso, M., Vartiainen, S., Moilanen, A. M., Sirviö, J., Thomas, J. H., Nass, R., et al. (2003). Dopaminergic Neuronal Loss and Motor Deficits in Caenorhabditis elegans Overexpressing Human Alpha-Synuclein. J. Neurochem. 86 (1), 165–172. doi:10.1046/j.1471-4159.2003.01809.x
Lastres-Becker, I., Ulusoy, A., Innamorato, N. G., Sahin, G., Rábano, A., Kirik, D., et al. (2012). α-Synuclein Expression and Nrf2 Deficiency Cooperate to Aggravate Protein Aggregation, Neuronal Death and Inflammation in Early-Stage Parkinson's Disease. Hum. Mol. Genet. 21 (14), 3173–3192. doi:10.1093/hmg/dds143
Leitman, J., Ulrich Hartl, F., and Lederkremer, G. Z. (2013). Soluble Forms of polyQ-Expanded Huntingtin rather Than Large Aggregates Cause Endoplasmic Reticulum Stress. Nat. Commun. 4 (1), 2753. doi:10.1038/ncomms3753
Leja-Szpak, A., Pierzchalski, P., Goralska, M., Nawrot-Porabka, K., Bonior, J., Link-Lenczowski, P., et al. (2015). Kynuramines Induce Overexpression of Heat Shock Proteins in Pancreatic Cancer Cells via 5-hydroxytryptamine and MT1/MT2 Receptors. J. Physiol. Pharmacol. 66 (5), 711–718.
Lévy, E., El Banna, N., Baïlle, D., Heneman-Masurel, A., Truchet, S., Rezaei, H., et al. (2019). Causative Links between Protein Aggregation and Oxidative Stress: A Review. Ijms 20 (16), 3896. doi:10.3390/ijms20163896
Liachko, N. F., Guthrie, C. R., and Kraemer, B. C. (2010). Phosphorylation Promotes Neurotoxicity in a Caenorhabditis elegans Model of TDP-43 Proteinopathy. J. Neurosci. 30 (48), 16208–16219. doi:10.1523/jneurosci.2911-10.2010
Link, C. D. (1995). Expression of Human Beta-Amyloid Peptide in Transgenic Caenorhabditis elegans. Proc. Natl. Acad. Sci. U. S. A. 92 (20), 9368–9372. doi:10.1073/pnas.92.20.9368
Lipinski, C. A. (2000). Drug-like Properties and the Causes of Poor Solubility and Poor Permeability. J. Pharmacol. Toxicol. Methods 44 (1), 235–249. doi:10.1016/s1056-8719(00)00107-6
Liu, H. C., Liao, H. T., and Charng, Y. Y. (2011). The Role of Class A1 Heat Shock Factors (HSFA1s) in Response to Heat and Other Stresses in Arabidopsis. Plant Cell Environ. 34 (5), 738–751. doi:10.1111/j.1365-3040.2011.02278.x
Markaki, M., and Tavernarakis, N. (2010). Modeling Human Diseases in Caenorhabditis elegans. Biotechnol. J. 5 (12), 1261–1276. doi:10.1002/biot.201000183
Martin, R. J., Verma, S., Levandoski, M., Clark, C. L., Qian, H., Stewart, M., et al. (2005). Drug Resistance and Neurotransmitter Receptors of Nematodes: Recent Studies on the Mode of Action of Levamisole. Parasitology 131 (Suppl. l), S71–S84. doi:10.1017/S0031182005008668
Martin, Y. C. (2005). A Bioavailability Score. J. Med. Chem. 48 (9), 3164–3170. doi:10.1021/jm0492002
Masood, F. N. K., and Fatima, S. T. (2018). A Computational Approach for Mutational Analysis of KRAS Snps and Toxicity Prediction of Screened Compounds of Lethal G12R KRAS SNP. J. Appl. Bioinforma. Comput. Biol. 7 (4). doi:10.4172/2329-9533.1000158
McColl, G., Roberts, B. R., Pukala, T. L., Kenche, V. B., Roberts, C. M., Link, C. D., et al. (2012). Utility of an Improved Model of Amyloid-Beta (Aβ1-42) Toxicity in Caenorhabditis elegans for Drug Screening for Alzheimer's Disease. Mol. Neurodegener. 7, 57. doi:10.1186/1750-1326-7-57
McColl, G., Rogers, A. N., Alavez, S., Hubbard, A. E., Melov, S., Link, C. D., et al. (2010). Insulin-like Signaling Determines Survival during Stress via Posttranscriptional Mechanisms in C. elegans. Cell Metab. 12 (3), 260–272. doi:10.1016/j.cmet.2010.08.004
Morfini, G., Pigino, G., and Brady, S. T. (2005). Polyglutamine Expansion Diseases: Failing to Deliver. Trends Mol. Med. 11 (2), 64–70. doi:10.1016/j.molmed.2004.12.002
Morimoto, R. I. (2008). Proteotoxic Stress and Inducible Chaperone Networks in Neurodegenerative Disease and Aging. Genes Dev. 22 (11), 1427–1438. 22/11/1427 [pii];10.1101/gad.1657108. doi:10.1101/gad.1657108
Morimoto, R. I. (2011). The Heat Shock Response: Systems Biology of Proteotoxic Stress in Aging and Disease. Cold Spring Harb. Symp. Quant. Biol. 76, 91–99. sqb.2012.76.010637 [pii]. doi:10.1101/sqb.2012.76.010637
Morley, J. F., Brignull, H. R., Weyers, J. J., and Morimoto, R. I. (2002). The Threshold for Polyglutamine-Expansion Protein Aggregation and Cellular Toxicity Is Dynamic and Influenced by Aging in Caenorhabditis elegans. Proc. Natl. Acad. Sci. U. S. A. 99 (16), 10417–10422. 152161099 [pii]. doi:10.1073/pnas.152161099
Muchowski, P. J., Schaffar, G., Sittler, A., Wanker, E. E., Hayer-Hartl, M. K., and Hartl, F. U. (2000). Hsp70 and Hsp40 Chaperones Can Inhibit Self-Assembly of Polyglutamine Proteins into Amyloid-like Fibrils. Proc. Natl. Acad. Sci. U. S. A. 97 (14), 7841–7846. doi:10.1073/pnas.140202897
Muegge, I., Heald, S. L., and Brittelli, D. (2001). Simple Selection Criteria for Drug-like Chemical Matter. J. Med. Chem. 44 (12), 1841–1846. doi:10.1021/jm015507e
Murphy, C. T., and Hu, P. J. (2013). Insulin/Insulin-Like Growth Factor Signaling in C. elegans. WormBook, 1–43. doi:10.1895/wormbook.1.164.1
Nagy, M., Fenton, W. A., Li, D., Furtak, K., and Horwich, A. L. (2016). Extended Survival of Misfolded G85R SOD1-Linked ALS Mice by Transgenic Expression of Chaperone Hsp110. Proc. Natl. Acad. Sci. U. S. A. 113 (19), 5424–5428. doi:10.1073/pnas.1604885113
Nandi, A., Yan, L. J., Jana, C. K., and Das, N. (2019). Role of Catalase in Oxidative Stress- and Age-Associated Degenerative Diseases. Oxid. Med. Cell Longev. 2019, 9613090. doi:10.1155/2019/9613090
Niedzielska, E., Smaga, I., Gawlik, M., Moniczewski, A., Stankowicz, P., Pera, J., et al. (2016). Oxidative Stress in Neurodegenerative Diseases. Mol. Neurobiol. 53 (6), 4094–4125. doi:10.1007/s12035-015-9337-5
Ogawa, T., Kodera, Y., Hirata, D., Blackwell, T. K., and Mizunuma, M. (2016). Natural Thioallyl Compounds Increase Oxidative Stress Resistance and Lifespan in Caenorhabditis elegans by Modulating SKN-1/Nrf. Sci. Rep. 6 (1), 21611. doi:10.1038/srep21611
Olzscha, H., Schermann, S. M., Woerner, A. C., Pinkert, S., Hecht, M. H., Tartaglia, G. G., et al. (2011). Amyloid-like Aggregates Sequester Numerous Metastable Proteins with Essential Cellular Functions. Cell 144 (1), 67–78. doi:10.1016/j.cell.2010.11.050
Pereira, F. S. O., Barbosa, F. A. R., Canto, R. F. S., Lucchese, C., Pinton, S., Braga, A. L., et al. (2022). Dihydropyrimidinone-derived Selenoesters Efficacy and Safety in an In Vivo Model of Aβ Aggregation. Neurotoxicology 88, 14–24. doi:10.1016/j.neuro.2021.10.012
Raitt, D. C., Johnson, A. L., Erkine, A. M., Makino, K., Morgan, B., Gross, D. S., et al. (2000). The Skn7 Response Regulator of Saccharomyces cerevisiae Interacts with Hsf1 In Vivo and Is Required for the Induction of Heat Shock Genes by Oxidative Stress. Mol. Biol. Cell 11 (7), 2335–2347. doi:10.1091/mbc.11.7.2335
Regitz, C., Dußling, L. M., and Wenzel, U. (2014). Amyloid-beta (Aβ1-42)-Induced Paralysis in Caenorhabditis elegans Is Inhibited by the Polyphenol Quercetin through Activation of Protein Degradation Pathways. Mol. Nutr. Food Res. 58 (10), 1931–1940. doi:10.1002/mnfr.201400014
Regitz, C., Fitzenberger, E., Mahn, F. L., Dußling, L. M., and Wenzel, U. (2016). Resveratrol Reduces Amyloid-Beta (Aβ1-42)-Induced Paralysis through Targeting Proteostasis in an Alzheimer Model of Caenorhabditis elegans. Eur. J. Nutr. 55 (2), 741–747. doi:10.1007/s00394-015-0894-1
Rodriguez, M., Snoek, L. B., De Bono, M., and Kammenga, J. E. (2013). Worms under Stress: C. elegans Stress Response and its Relevance to Complex Human Disease and Aging. Trends Genet. 29 (6), 367–374. doi:10.1016/j.tig.2013.01.010
Samuelson, A. V., Klimczak, R. R., Thompson, D. B., Carr, C. E., and Ruvkun, G. (2007). Identification of Caenorhabditis elegans Genes Regulating Longevity Using Enhanced RNAi-Sensitive Strains. Cold Spring Harb. Symp. Quant. Biol. 72, 489–497. doi:10.1101/sqb.2007.72.068
Sanchis, A., García-Gimeno, M. A., Cañada-Martínez, A. J., Sequedo, M. D., Millán, J. M., Sanz, P., et al. (2019). Metformin Treatment Reduces Motor and Neuropsychiatric Phenotypes in the zQ175 Mouse Model of Huntington Disease. Exp. Mol. Med. 51 (6), 1–16. doi:10.1038/s12276-019-0264-9
Saudou, F., Finkbeiner, S., Devys, D., and Greenberg, M. E. (1998). Huntingtin Acts in the Nucleus to Induce Apoptosis but Death Does Not Correlate with the Formation of Intranuclear Inclusions. Cell 95 (1), 55–66. doi:10.1016/s0092-8674(00)81782-1
Servello, F. A., and Apfeld, J. (2020). The Heat Shock Transcription Factor HSF-1 Protects Caenorhabditis elegans from Peroxide Stress. Translat. Med. Aging 4, 88–92. doi:10.1016/j.tma.2020.07.002
Shalmali, N., Ali, M. R., and Bawa, S. (2018). Imidazole: An Essential Edifice for the Identification of New Lead Compounds and Drug Development. Mini Rev. Med. Chem. 18 (2), 142–163. doi:10.2174/1389557517666170228113656
Sharma, A., Kumar, V., Kharb, R., Kumar, S., Sharma, P. C., and Pathak, D. P. (2016). Imidazole Derivatives as Potential Therapeutic Agents. Curr. Pharm. Des. 22 (21), 3265–3301. doi:10.2174/1381612822666160226144333
Sittler, A., Lurz, R., Lueder, G., Priller, J., Lehrach, H., Hayer-Hartl, M. K., et al. (2001). Geldanamycin Activates a Heat Shock Response and Inhibits Huntingtin Aggregation in a Cell Culture Model of Huntington's Disease. Hum. Mol. Genet. 10 (12), 1307–1315. doi:10.1093/hmg/10.12.1307
Siwach, A., and Verma, P. K. (2021). Synthesis and Therapeutic Potential of Imidazole Containing Compounds. BMC Chem. 15 (1), 12. doi:10.1186/s13065-020-00730-1
Sørensen, J. G., Kristensen, T. N., and Loeschcke, V. (2003). The Evolutionary and Ecological Role of Heat Shock Proteins. Ecol. Lett. 6, 1025–1037. doi:10.1046/j.1461-0248.2003.00528.x
Srivastava, D., Arya, U., SoundaraRajan, T., Dwivedi, H., Kumar, S., and Subramaniam, J. R. (2008). Reserpine Can Confer Stress Tolerance and Lifespan Extension in the Nematode C. elegans. Biogerontology 9 (5), 309–316. doi:10.1007/s10522-008-9139-5
Steinbaugh, M. J., Narasimhan, S. D., Robida-Stubbs, S., Moronetti Mazzeo, L. E., Dreyfuss, J. M., Hourihan, J. M., et al. (2015). Lipid-mediated Regulation of SKN-1/Nrf in Response to Germ Cell Absence. Elife 4. doi:10.7554/eLife.07836
Strayer, A., Wu, Z., Christen, Y., Link, C. D., and Luo, Y. (2004). Expression of the Small Heat-Shock Protein Hsp16-2 in Caenorhabditis elegans Is Suppressed by Ginkgo Biloba Extract EGb 761. FASEB J. 17, 2305–2307. doi:10.1096/fj.03-0376fje
Sweeney, P., Park, H., Baumann, M., Dunlop, J., Frydman, J., Kopito, R., et al. (2017). Protein Misfolding in Neurodegenerative Diseases: Implications and Strategies. Transl. Neurodegener. 6, 6. doi:10.1186/s40035-017-0077-5
Swindell, W. R. (2012). Dietary Restriction in Rats and Mice: a Meta-Analysis and Review of the Evidence for Genotype-dependent Effects on Lifespan. Ageing Res. Rev. 11 (2), 254–270. doi:10.1016/j.arr.2011.12.006
Syntichaki, P., Xu, K., Driscoll, M., and Tavernarakis, N. (2002). Specific Aspartyl and Calpain Proteases Are Required for Neurodegeneration in C. elegans. Nature 419 (6910), 939–944. doi:10.1038/nature01108
Teixeira-Castro, A., Ailion, M., Jalles, A., Brignull, H. R., Vilaça, J. L., Dias, N., et al. (2011). Neuron-specific Proteotoxicity of Mutant Ataxin-3 in C. elegans: Rescue by the DAF-16 and HSF-1 Pathways. Hum. Mol. Genet. 20 (15), 2996–3009. ddr203 [pii]. doi:10.1093/hmg/ddr203
Tsai, R. T., Tsai, C. W., Liu, S. P., Gao, J. X., Kuo, Y. H., Chao, P. M., et al. (2020). Maackiain Ameliorates 6-Hydroxydopamine and SNCA Pathologies by Modulating the PINK1/Parkin Pathway in Models of Parkinson's Disease in Caenorhabditis elegans and the SH-Sy5y Cell Line. Int. J. Mol. Sci. 21 (12). doi:10.3390/ijms21124455
Tsunemi, T., Ashe, T. D., Morrison, B. E., Soriano, K. R., Au, J., Roque, R. A., et al. (2012). PGC-1α Rescues Huntington's Disease Proteotoxicity by Preventing Oxidative Stress and Promoting TFEB Function. Sci. Transl. Med. 4 (142), 142ra97. doi:10.1126/scitranslmed.3003799
Uno, M., and Nishida, E. (2016). Lifespan-regulating Genes in C. elegans. NPJ Aging Mech. Dis. 2, 16010. doi:10.1038/npjamd.2016.10
van Ham, T. J., Thijssen, K. L., Breitling, R., Hofstra, R. M., Plasterk, R. H., and Nollen, E. A. (2008). C. elegans Model Identifies Genetic Modifiers of Alpha-Synuclein Inclusion Formation during Aging. PLoS Genet. 4 (3), e1000027. doi:10.1371/journal.pgen.1000027
Vanfleteren, J. R., and Braeckman, B. P. (1999). Mechanisms of Life Span Determination in Caenorhabditis elegans. Neurobiol. Aging 20 (5), 487–502. doi:10.1016/s0197-4580(99)00087-1
Vasconcellos, L. R., Dutra, F. F., Siqueira, M. S., Paula-Neto, H. A., Dahan, J., Kiarely, E., et al. (2016). Protein Aggregation as a Cellular Response to Oxidative Stress Induced by Heme and Iron. Proc. Natl. Acad. Sci. U. S. A. 113 (47), E7474–e7482. doi:10.1073/pnas.1608928113
Veber, D. F., Johnson, S. R., Cheng, H. Y., Smith, B. R., Ward, K. W., and Kopple, K. D. (2002). Molecular Properties that Influence the Oral Bioavailability of Drug Candidates. J. Med. Chem. 45 (12), 2615–2623. doi:10.1021/jm020017n
Volkert, M. R., and Crowley, D. J. (2020). Preventing Neurodegeneration by Controlling Oxidative Stress: The Role of OXR1. Front. Neurosci. 14, 611904. doi:10.3389/fnins.2020.611904
White, J. G., Southgate, E., Thomson, J. N., and Brenner, S. (1986). The Structure of the Nervous System of the Nematode Caenorhabditis elegans. Philos. Trans. R. Soc. Lond B Biol. Sci. 314 (1165), 1–340. doi:10.1098/rstb.1986.0056
Winner, B., Jappelli, R., Maji, S. K., Desplats, P. A., Boyer, L., Aigner, S., et al. (2011). In Vivo demonstration that Alpha-Synuclein Oligomers Are Toxic. Proc. Natl. Acad. Sci. U. S. A. 108 (10), 4194–4199. doi:10.1073/pnas.1100976108
Witan, H., Kern, A., Koziollek-Drechsler, I., Wade, R., Behl, C., and Clement, A. M. (2008). Heterodimer Formation of Wild-type and Amyotrophic Lateral Sclerosis-Causing Mutant Cu/Zn-Superoxide Dismutase Induces Toxicity Independent of Protein Aggregation. Hum. Mol. Genet. 17 (10), 1373–1385. doi:10.1093/hmg/ddn025
Woerner, A. C., Frottin, F., Hornburg, D., Feng, L. R., Meissner, F., Patra, M., et al. (2016). Cytoplasmic Protein Aggregates Interfere with Nucleocytoplasmic Transport of Protein and RNA. Science 351 (6269), 173–176. doi:10.1126/science.aad2033
Wolozin, B., Gabel, C., Ferree, A., Guillily, M., and Ebata, A. (2011). Watching Worms Whither: Modeling Neurodegeneration in C. elegans. Prog. Mol. Biol. Transl. Sci. 100, 499–514. doi:10.1016/b978-0-12-384878-9.00015-7
Xi, W., Wang, X., Laue, T. M., and Denis, C. L. (2016). Multiple Discrete Soluble Aggregates Influence Polyglutamine Toxicity in a Huntington's Disease Model System. Sci. Rep. 6 (1), 34916. doi:10.1038/srep34916
Yerbury, J. J., Ooi, L., Dillin, A., Saunders, D. N., Hatters, D. M., Beart, P. M., et al. (2016). Walking the Tightrope: Proteostasis and Neurodegenerative Disease. J. Neurochem. 137 (4), 489–505. doi:10.1111/jnc.13575
Zakrzewska, A., van Eikenhorst, G., Burggraaff, J. E., Vis, D. J., Hoefsloot, H., Delneri, D., et al. (2011). Genome-wide Analysis of Yeast Stress Survival and Tolerance Acquisition to Analyze the Central Trade-Off between Growth Rate and Cellular Robustness. Mol. Biol. Cell 22 (22), 4435–4446. doi:10.1091/mbc.E10-08-0721
Keywords: Caenorhabditis elegans, imidazolium salts, neurodegenerative disease, proteotoxicity, oxidative stress
Citation: Andersen N, Veuthey T, Blanco MG, Silbestri GF, Rayes D and De Rosa MJ (2022) 1-Mesityl-3-(3-Sulfonatopropyl) Imidazolium Protects Against Oxidative Stress and Delays Proteotoxicity in C. elegans. Front. Pharmacol. 13:908696. doi: 10.3389/fphar.2022.908696
Received: 30 March 2022; Accepted: 28 April 2022;
Published: 24 May 2022.
Edited by:
Jeremy Turnbull, University of Liverpool, United KingdomReviewed by:
Matthias Truttmann, University of Michigan, United StatesPooja Jadiya, Wake Forest University, United States
Alex Parker, Centre de Recherche du Centre Hospitalier de l’Université de Montreal, Canada
Copyright © 2022 Andersen, Veuthey, Blanco, Silbestri, Rayes and De Rosa. This is an open-access article distributed under the terms of the Creative Commons Attribution License (CC BY). The use, distribution or reproduction in other forums is permitted, provided the original author(s) and the copyright owner(s) are credited and that the original publication in this journal is cited, in accordance with accepted academic practice. No use, distribution or reproduction is permitted which does not comply with these terms.
*Correspondence: Diego Rayes, ZHJheWVzQGNyaWJhLmVkdS5hcg==; María José De Rosa, bWpkZXJvc2FAY3JpYmEuZWR1LmFy