- 1Section of Pharmaceutical Technology, Department of Pharmacy, School of Health Sciences, National and Kapodistrian University of Athens, Athens, Greece
- 2Department of Genetic Medicine, Johns Hopkins School of Medicine, Baltimore, MD, United States
- 3The Richman Family Precision Medicine Center of Excellence in Alzheimer’s Disease, Johns Hopkins Medicine, Baltimore, MD, United States
RNA-delivery is a promising tool to develop therapies for difficult to treat diseases such as neurological disorders, by silencing pathological genes or expressing therapeutic proteins. However, in many cases RNA delivery requires a vesicle that could effectively protect the molecule from bio-degradation, bypass barriers i.e., the blood brain barrier, transfer it to a targeted tissue and efficiently release the RNA inside the cells. Many vesicles such as viral vectors, and polymeric nanoparticles have been mentioned in literature. In this review, we focus in the discussion of lipid-based advanced RNA-delivery platforms. Liposomes and lipoplexes, solid lipid nanoparticles and lipid nanoparticles are the main categories of lipidic platforms for RNA-delivery to the central nervous systems (CNS). A variety of surface particles’ modifications and routes of administration have been studied to target CNS providing encouraging results in vivo. It is concluded that lipid-based nanoplatforms will play a key role in the development of RNA neuro-therapies.
Introduction
Approximately 50 years ago, the first conversation of whether gene therapy could be the solution for the treatment of serious diseases such as cancer or genetic disorders began (Friedmann and Roblin, 1972). Since then, the science of gene therapy has flourished. However, currently, only a few products based on in vivo or ex vivo gene therapy have been approved by the US Food and Drug Administration (FDA) and the European Medicines Agency (EMA) as serious limitations have not yet been overcome. Indeed, the first gene therapy clinical trials resulted in serious adverse effects or even deaths that lead the US National Institute of Health (NIH) to suspend the trials in pre-clinical stages (Orkin and Motulsky, 1996).
Although today many of the early shortcomings of this technology such as off-target effects, vector safety, or inefficient administration have been solved, there still remain some unsolved questions. Especially in the case of in vivo gene therapy, nucleic acid should bypass the immune system and bio-degradation processes of the human organism, reach the target tissue, and even cross cell or nucleus membranes. Thus, the accomplishment of the therapeutic genetic modifications requires that the nucleic acid will remain effective till the time of its expression by the target cells. For this purpose, the use of a vector is very common. The vector is responsible for the delivery of the nucleic acid to the target, avoiding its degradation and systematic adverse effects. The majority of vectors are viral vectors such as adenoviruses (Zhu et al., 2020; Voysey et al., 2021; Watanabe et al., 2021), retroviruses (Rastegar et al., 2009), lentiviruses (Maude et al., 2014; Sessa et al., 2016) or adeno-associated viruses (Wuh-Liang et al., 2012; Keiser et al., 2016; Mendell et al., 2017; Wang et al., 2018). Currently, many scientific groups propose generally safe viral vectors as efficient gene delivery platforms (Walther and Stein, 2000; Bulcha et al., 2021; Selvaraj et al., 2021).
Nevertheless, other alternatives to the viral vectors have also been developed and studied over a period of approximately 40 years (Patil et al., 2019; Faneca, 2021). Indeed, the first medicinal product that used a lipid nanoplatform (LNP) for the delivery of a siRNA molecule has been approved by FDA and EMA in 2018 by the trade name Patisiran or Onppatro respectively for the treatment of hereditary transthyretin-mediated amyloidosis (hATTR amyloidosis) in adult patients with stage 1 or stage 2 polyneuropathy (DeWeerdt, 2019). Today, this technology has been used for the development of mRNA COVID-19 vaccines (Tsakiri et al., 2021), while a few other similar platforms are in clinical trials (Damase et al., 2021).
Diseases of the central nervous system (CNS) such as tumors or neurodegenerative pathologies, have high mortality rates as there are no medicinal products to cure them (Liang et al., 2021). For example, approximately 6.2 million American citizens aged ≥65 years were estimated to suffer from Alzheimer’s disease (AD) while 121,499 deaths from the same pathology were reported in 2019 (Wiley, 2021). Centers for disease control and prevention (CDC) mention that by 2050 13.8 million US. adults aged ≥65 years are expected to suffer from AD (Taylor et al., 2017). Notably, these numbers refer to only one degenerative disease. Moreover, in many of these conditions such as AD, the only available treatment options are symptomatic and do not address the underlying cause of the disease (Breijyeh and Karaman, 2020). Thus, gene therapy for the treatment of CNS diseases holds the potential to bring promising results.
Although many approaches to deliver RNA molecules that do not contain viral vectors exist such as chemically modified antisense oligonucleotides (ASO), N-acetylgalactosamine (GalNAc) ligand-modified short interfering RNA (siRNA) conjugates (Kulkarni et al., 2021) or polymeric delivery platforms (Wahane et al., 2020) the role of non-viral, lipid vectors in the development of advanced therapeutic medicinal products for gene therapy is of great importance. In addition to protecting the nucleic acid from degradation, these platforms can be engineered to target the brain and ensure intracellular release through endosomal escape mechanisms in some cases. In this mini-review, we will discuss the approaches that are utilized for the development of lipid gene delivery nanoplatforms that target CNS and the perspectives of this technology in the treatment of brain diseases.
Lipid-based nanoparticles
Lipid-based nanoparticles are the most well-studied non-viral platforms for the delivery of RNA molecules (Zhao and Huang, 2014; Meng et al., 2017). This broad category includes liposomes, lipoplexes, lipid nanoparticles and solid lipid nanoparticles, as presented in Figure 1. By virtue of their biochemical composition, lipidic nanoparticles provide bio-mimicking and bio-degradable platforms. As a result of their lipophilicity, they can penetrate more easily the BBB while the inclusion of cationic lipids stabilize the negatively charged RNA molecules via electrostatic interactions.
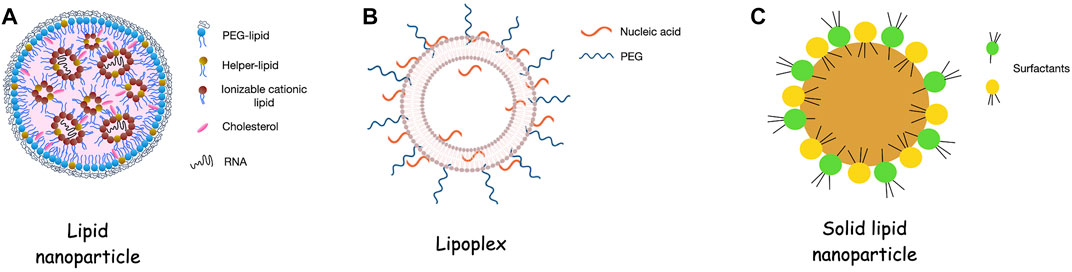
FIGURE 1. Consistency of lipidic nanoparticles for RNA delivery. (A). Lipid nanoparticles (LNPs) contain an ionizable cationic lipid that allows the encapsulation of the RNA molecules into the internal aqueous cavities of the platform. Addition of PEG-lipid, helper lipid and cholesterol contribute in physicochemical and biological stability of the LNPs. (B). Lipoplexes are liposomes that contain cationic lipids and are conjugated with nucleic acids i.e., RNA. IN this case the nucleic acids are mainly in the external surface of the systems leading which indicates that the RNA is more exposed to the environment than in the case of the LNPs. (C). Solid lipid nanoparticles are formed by the lipid core of solid lipids and the amphiphilic surfactants that decorate the surface of the particles. Figure 1B adapted from Pereira et al. (2021).
Liposomes and lipoplexes
Liposomes are self-assembled pseudospherical vesicles in which the lipid bilayers surround the aqueous core. Liposomes have been widely and for a long time used as drug delivery systems for the treatment of a plethora of diseases. Indeed, more than 15 liposomal drugs are currently on the market (Large et al., 2021). However, their application in encapsulating nucleic acids is in the pre-clinical or early clinical phase.
Liposomes can be categorized by their size, charge, key surface molecules or surface modifications to modulate circulation time and avoid detection and elimination by the immune system. The development of liposomes for gene therapy often involves the use of cationic lipids which interact electrostatically with the negatively charged genetic material (Mishra et al., 2022). The complexation of nucleic acids with cationic lipids leads to the formation of the lipoplexes in which nucleic acids are either on the external surface of the structure or in the internal cavity (Pereira et al., 2021). The most commonly used cationic lipids are 2,3-Dioleoyloxy-propyl)-trimethylammonium-chloride (DOTAP) (Lechanteur et al., 2018; Hattori et al., 2019), di-O-octadecenyl-3-trimethylammonium propane (DOTMA) and dimethyldioctadecylammonium bromide DDA (Putzke et al., 2020).
Concerning the central nervous system, certain liposomes/lipoplexes experiments have proved efficacious. Recently, Bender and others developed DOTAP:Cholesterol 1:1 liposomes that were incubated with small interfering RNA (siRNA) that decrease the amount of neuronal cellular prion protein (PrPc) and rabies virus glycoprotein fragment (RVG-9r). As it is presented in the literature, RVG selectively interacts with the nicotinic achetilocholine receptor on the surface of the neuronal cells while the addition of a positively charged arginine residue at the carboxyl terminus of RVG (9r modification) allows the electrostatic interaction of the modified peptide and the siRNA chains (Kumar et al., 2007). Indeed, their formulations can effectively bypass the BBB and prolong the lifetime of prion-infected mice. The targeting to the brain cells was successful due to the RVG-9r protein while the therapeutic effect suggests that the siRNA successfully knockdown the PrPc expression (Zabel, 2013; Bender et al., 2016, 2019). Since many neurodegenerative diseases such as Alzheimer’s, Parkinson’s or Huntington’s pathology, belong to the category of prion diseases, those results could bring great promise for their treatment. In another study, DOTAP: Cholesterol siRNA-liposomes associated with transferrin that were topically administrated in mouse brain proved to have high efficiency in downregulation of pathological genes while having limited toxicity (Cardoso et al., 2008). Finally, Yuan et al. show that siRNA (siGOLPH3) loaded cationic liposomes incorporated with angiopep2GOLPH3 ligand for LRP-1 receptor that is expressed in human BBB and glioma cells, protein provide could result in a potential treatment of glioma (Yuan et al., 2018).
Furthermore, different routes of administration can improve the brain delivery efficiency of liposomes. Dhaliwal et al. chose to administer their mRNA lipoplexes intranasally. The liposomes were loaded with the mRNA, labeled with GFP-mRNA or luciferase-mRNA to evaluate the transfection efficiency of the formulations (Dhaliwal et al., 2020). The results proved that the expression of the mRNA was higher when the liposomal vesicles were utilized to vehiculate the mRNA than when the mRNA was administered in naked form. In comparison with the administration of the naked mRNA. Interestingly, Hu and others showed that intranasal administration of core-cell lipoplexes could effectively transfer the siRNA (Hu et al., 2022).
Lipid nanoparticles
Disadvantages associated with the use of cationic lipids in liposomes and lipoplexes such as rapid clearance from the reticuloendothelial system (RES) due to opsonization and activation of the complement system and toxicity issues connected with cell apoptotic mechanisms, enhanced reactive oxygen species (ROS) levels, have prompted the development of the ionizable cationic lipids and the lipid nanoparticles (LNPs) (Filion and Phillips, 1997; Knudsen et al., 2015; Cui et al., 2018; Liu et al., 2020; Kulkarni et al., 2021). Cationic lipids that are positively charged at about pH 4, but that are electroneutral at physiological pH are key components of LNPs (Tenchov et al., 2021). Consequently, they provide a safer solution as advanced delivery platforms. Their safety is well-established due to the recent approval and massive administration of Pfizer/BioNTech and Moderna mRNA vaccines (Polack et al., 2020; Baden et al., 2021). However, only a few studies evaluate the safety and efficiency of LNP-RNA platforms intended for therapy in the CNS. To the best of our knowledge, the first research article to address this particular question was published in 2013. In this study, Rugta et al. provide evidence that when their siRNA-LNPs were administrated within mice cortex or via intracerebroventricular injection a good rate of uptake by neuronal cells and reduction of associated protein levels was observed. Furthermore, no toxicity to neurons was observed (Rungta et al., 2013). More recently, two separate research groups from Israel and Denmark have studied the potential role of RNA interference-LNPs in the treatment of glioma. The former, developed Dlin-MC3-DMA, DSPC, Chol, DMG-PEG, and DSPE-PEG LNPs decorated with hyaluronan, which is a CD44 ligand (Cohen et al., 2015). The latter, chose a more complex formulation that externally has a slight negative charge due to a PEGylated cleavable lipopeptide. Metalloproteinases present in the tumor microenvironment are responsible for the cleavage of the PEGylated lipoprotein leading to positively charged LNPs that subsequently endocytosed. Thus, intracellular release of the siRNA is achieved (Bruun et al., 2015). Both groups resulted in good toxicological results and a high knockdown percentage of the targeted mRNA after in vitro experiments and topical administration in mice. Tanaka et al. developed LNPs that contained SS-cleavable proton-activated lipid-like materials as the ionizable elements. These synthetic lipids provide a neutral charge of the platform at physiological pH values while they are protonated at the low endosomal pH. Similarly with the work of Bruun et al. (2015), such a phenomenon leads to the protection of the RNA molecule when administrated in vivo and the intracellular release of this sensitive bio-molecule (Tanaka et al., 2018). Finally, Khare et al., recently showed that LNPs with C12-200 lipidoid, which is a syntetic lipid-like molecule that displays tertiary amines in the headgroups, could provide a safer approach for RNA delivery into the neurons than other platforms that contain lipofectamine [mixture of 2,3-dioleoyloxy-N- [2 (sperminecarboxamido)ethyl]-N,N-dimethyl-1-propaniminium trifluoroacetate (DOSPA) and 1,2-Dioleoyl-sn-glycerophosphoethanolamine (DOPE)]. The platform offers favourable loading capacity, displays efficient cellular uptake and low in vitro cytotoxicity in human cortical cell lines (Khare et al., 2021).
Solid lipid nanoparticles
Solid lipid nanoparticles (SLNs) are another category of lipidic nanoparticles. They from liposomes in that SLNs do not have an aqueous central cavity but a solid lipid:surfactant one (Scioli Montoto et al., 2020). Depending on their composition, size and charge SLNs present unique physicochemical properties (Naseri et al., 2015).
Concerning their role in the protection and delivery of nucleic acids, SLNs have not been widely studied, maybe due to their morphological characteristics. Since nucleic acids are hydrophilic molecules, their interaction with the SLN could take place only on the surface of the delivery platforms electrostatically. However, electrostatic interactions are not particularly stable and could easily break under environmental pressure (Wojnarowska et al., 2018). Nevertheless, an interesting study was performed by Rassu et al. They stabilized BACE-1 siRNA negatively charged molecules, that could have a valuable role in the treatment of Alzheimer’s disease, with RVG-9R, which was positively charged. Afterward, the siRNA-RVG-9R complex solution was mixed with triglycerides, poly (vinyl alcohol) and chitosan for the formation of a double emulsion that contains the SLNs. The chitosan coated SNPs presented higher in vitro mucoadhesiveness and permeability properties than the non-coated SNPs (Rassu et al., 2017). Another study utilized two different siRNA molecules that knockdown the human c-Met, which is found in glioma to deliver them by SLN. The siRNA molecules were first conjugated with polyethylene glycol (PEG) via a disulfide bond. The SLN-siRNA-PEG formulations displayed accumulation in the brain tumor, no systemic toxicity as well as suppression of the tumor growth after intravenous administration in the glioblastoma xenograft tumor model (Jin et al., 2011).
Discussion
Today, the treatment of brain malignancies such as glioblastoma or neurodegenerative diseases still remains an elusive (Mullard, 2021; Sun and Roy, 2021). Gene therapy is a promising approach to developing new medicinal products for a plethora of different diseases (Sudhakar and Richardson, 2019; Parambi et al., 2022). For instance, the small interfering RNAs can downregulate the expression of pathological proteins that are present in diseases. Such pharmacological action is not possible with other therapeutic approaches (i.e., by small drugs or proteins). Viral vectors have been the gold standard of brain gene delivery in the past years as they can easily pass the BBB, they are taken up by the brain cells and transfer their genes into the cell nucleus (Lundstrom, 2018). Although the viruses that are utilized are either attenuated or non-replicating, concerns about the safety of these vectors exist in terms of immunogenicity and mutagenicity.
The BBB remains one of the main obstacles for the lipidic delivery systems. Three pathways responsible for the lipidic vesicles by-pass through the BBB are mentioned in the literature: 1) adsorptive-mediated transcytosis (AMT), 2) receptor-mediated transcytosis (RMT), and 3) carrier-mediated transcytosis (CMT) (Juhairiyah and de Lange, 2021). Concerning AMT, cationic vesicles interact electrostatically with the endothelial cells of the BBB. However, the cationic charge leads to toxicity effects and fast inactivation of the particles via opsonization processes. The decoration of the lipidic nanoparticles with accessory molecules such as peptides or antibodies is a common procedure. Transferrin (Kong et al., 2020), glucose or mannose and their derivatives (Qu et al., 2014; Arora et al., 2020), vitamin C (Peng et al., 2018) and glutathione (Salem et al., 2015; Trapani et al., 2021) are some of these accessory molecules. Another effective way to avoid the BBB via non-invasive administration is the nose-to-brain pathway. Although the exact mechanism of action of nose-to-brain delivery is not clear, transfer through olfactory nerves or trigeminal nerves seems to be the most common way after intranasal administration. For the former, the nanoparticles paracellularly pass through the gaps of olfactory cells, finally reaching the subarachnoid space (Crowe et al., 2018). Indeed, Godfrey and others found that their intranasally administrated nanoplatforms were located in the olfactory bulb only 5 min after the administration (Godfrey et al., 2018). On the other hand, the transport through the trigeminal nerves seems to be less common but possible both by intra- or extra-cellular routes (Cunha et al., 2017; Bourganis et al., 2018). However, some consideration still exists of whether today, nose-to-brain administration of nanovesicles increase the effectiveness of active molecules (Feng et al., 2018).
The majority of recent and current studies related to the delivery of small molecules or bio-molecules in the CNS with the aid of nanotechnology have focused on liposomal nanovesicles. However, other platforms, such as LNPs may be better choices for the transport of genetic material in the brain due to the better encapsulation of a sensitive RNA cargo. For instance, although many of the studies mentioned above indicate that LNPs containing RNA molecules could play an important role in the treatment of CNS diseases, all of them used a patient unfriendly route of administration and more work is necessary for the development of platforms that are not only safe and efficacious but also not demanding for the patient. Moreover, in vivo toxicity studies after repeated administration is necessary to verify the safety of the platform as in many cases the therapeutic regimen would entail multiple, rather than single, dose protocol. Nevertheless, lipidic nanoparticles that carry RNAs hold great promise as advanced therapeutic medicinal products that could provide effective results in the treatment of neurodegenerative disorders, such as Alzheimer’s disease, or brain tumors like glioblastoma.
Author contributions
Data acquisition (literature review) and conceptualization, MT, CD, and VM; Critical revisions, CZ and VM All authors have read and agreed to the published version of the manuscript.
Funding
The Richman Family Precision Medicine Center of Excellence in Alzheimer’s Disease, Johns Hopkins Medicine and Johns Hopkins Bayview Medical Center, Baltimore, MD, United States.
Conflict of interest
The authors declare that the research was conducted in the absence of any commercial or financial relationships that could be construed as a potential conflict of interest.
Publisher’s note
All claims expressed in this article are solely those of the authors and do not necessarily represent those of their affiliated organizations, or those of the publisher, the editors and the reviewers. Any product that may be evaluated in this article, or claim that may be made by its manufacturer, is not guaranteed or endorsed by the publisher.
References
Arora, S., Sharma, D., and Singh, J. (2020). GLUT-1: an effective target to deliver brain-derived neurotrophic factor gene across the blood brain barrier. ACS Chem. Neurosci. 11, 1620–1633. doi:10.1021/acschemneuro.0c00076
Baden, L. R., El Sahly, H. M., Essink, B., Kotloff, K., Frey, S., Novak, R., et al. (2021). Efficacy and safety of the mRNA-1273 SARS-CoV-2 vaccine. N. Engl. J. Med. 384, 403–416. doi:10.1056/NEJMoa2035389
Bender, H., Noyes, N., Annis, J. L., Hitpas, A., Mollnow, L., Croak, K., et al. (2019). PrPC knockdown by liposome-siRNA-peptide complexes (LSPCs) prolongs survival and normal behavior of prion-infected mice immunotolerant to treatment. PLoS One 14, e0219995. doi:10.1371/journal.pone.0219995
Bender, H. R., Kane, S., and Zabel, M. D. (2016). Delivery of therapeutic siRNA to the CNS using cationic and anionic liposomes. J. Vis. Exp., 54106. doi:10.3791/54106
Bourganis, V., Kammona, O., Alexopoulos, A., and Kiparissides, C. (2018). Recent advances in carrier mediated nose-to-brain delivery of pharmaceutics. Eur. J. Pharm. Biopharm. 128, 337–362. doi:10.1016/j.ejpb.2018.05.009
Breijyeh, Z., and Karaman, R. (2020). Comprehensive review on Alzheimer’s disease: Causes and treatment. Molecules 25, 5789. doi:10.3390/molecules25245789
Bruun, J., Larsen, T. B., Jølck, R. I., Eliasen, R., Holm, R., Gjetting, T., et al. (2015). Investigation of enzyme-sensitive lipid nanoparticles for delivery of siRNA to blood–brain barrier and glioma cells. Int. J. Nanomedicine 10, 5995–6008. doi:10.2147/IJN.S87334
Bulcha, J. T., Wang, Y., Ma, H., Tai, P. W. L., and Gao, G. (2021). Viral vector platforms within the gene therapy landscape. Signal Transduct. Target. Ther. 6, 53. doi:10.1038/s41392-021-00487-6
Cardoso, A. L. C., Simões, S., de Almeida, L. P., Plesnila, N., Pedroso de Lima, M. C., Wagner, E., et al. (2008). Tf-lipoplexes for neuronal siRNA delivery: a promising system to mediate gene silencing in the CNS. J. Control. Release 132, 113–123. doi:10.1016/j.jconrel.2008.08.014
Cohen, Z. R., Ramishetti, S., Peshes-Yaloz, N., Goldsmith, M., Wohl, A., Zibly, Z., et al. (2015). Localized RNAi therapeutics of chemoresistant grade IV glioma using hyaluronan-grafted lipid-based nanoparticles. ACS Nano 9, 1581–1591. doi:10.1021/nn506248s
Crowe, T. P., Greenlee, M. H. W., Kanthasamy, A. G., and Hsu, W. H. (2018). Mechanism of intranasal drug delivery directly to the brain. Life Sci. 195, 44–52. doi:10.1016/j.lfs.2017.12.025
Cui, S., Wang, Y., Gong, Y., Lin, X., Zhao, Y., Zhi, D., et al. (2018). Correlation of the cytotoxic effects of cationic lipids with their headgroups. Toxicol. Res. 7, 473–479. doi:10.1039/C8TX00005K
Cunha, S., Almeida, H., Amaral, M. H., Lobo, J. M. S., and Silva, A. C. (2017). Intranasal lipid nanoparticles for the treatment of neurodegenerative diseases. Curr. Pharm. Des. 23, 6553–6562. doi:10.2174/1381612824666171128105305
Damase, T. R., Sukhovershin, R., Boada, C., Taraballi, F., Pettigrew, R. I., Cooke, J. P., et al. (2021). The limitless future of RNA therapeutics. Front. Bioeng. Biotechnol. 9, 628137. doi:10.3389/fbioe.2021.628137
Dhaliwal, H. K., Fan, Y., Kim, J., and Amiji, M. M. (2020). Intranasal delivery and transfection of mRNA therapeutics in the brain using cationic liposomes. Mol. Pharm. 17, 1996–2005. doi:10.1021/acs.molpharmaceut.0c00170
Faneca, H. (2021). Non-viral gene delivery systems. Pharmaceutics 13, 446. doi:10.3390/pharmaceutics13040446
Feng, Y., He, H., Li, F., Lu, Y., Qi, J., Wu, W., et al. (2018). An update on the role of nanovehicles in nose-to-brain drug delivery. Drug Discov. Today 23, 1079–1088. doi:10.1016/j.drudis.2018.01.005
Filion, M. C., and Phillips, N. C. (1997). Toxicity and immunomodulatory activity of liposomal vectors formulated with cationic lipids toward immune effector cells. Biochim. Biophys. Acta 1329, 345–356. doi:10.1016/s0005-2736(97)00126-0
Friedmann, T., and Roblin, R. (1972). Gene therapy for human genetic disease? Science 175, 949–955. doi:10.1126/science.175.4025.949
Godfrey, L., Iannitelli, A., Garrett, N. L., Moger, J., Imbert, I., King, T., et al. (2018). Nanoparticulate peptide delivery exclusively to the brain produces tolerance free analgesia. J. Control. Release 270, 135–144. doi:10.1016/j.jconrel.2017.11.041
Hattori, Y., Nakamura, M., Takeuchi, N., Tamaki, K., Shimizu, S., Yoshiike, Y., et al. (2019). Effect of cationic lipid in cationic liposomes on siRNA delivery into the lung by intravenous injection of cationic lipoplex. J. Drug Target. 27, 217–227. doi:10.1080/1061186X.2018.1502775
Hu, Y., Jiang, K., Wang, D., Yao, S., Lu, L., Wang, H., et al. (2022). Core-shell lipoplexes inducing active macropinocytosis promote intranasal delivery of c-Myc siRNA for treatment of glioblastoma. Acta Biomater. 138, 478–490. doi:10.1016/j.actbio.2021.10.042
Jin, J., Bae, K. H., Yang, H., Lee, S. J., Kim, H., Kim, Y., et al. (2011). In vivo specific delivery of c-met siRNA to glioblastoma using cationic solid lipid nanoparticles. Bioconjug. Chem. 22, 2568–2572. doi:10.1021/bc200406n
Juhairiyah, F., and de Lange, E. C. M. (2021). Understanding drug delivery to the brain using liposome-based strategies: Studies that provide mechanistic insights are essential. AAPS J. 23, 114. doi:10.1208/s12248-021-00648-z
Keiser, M. S., Monteys, A. M., Corbau, R., Gonzalez-Alegre, P., and Davidson, B. L. (2016). RNAi prevents and reverses phenotypes induced by mutant human ataxin-1. Ann. Neurol. 80, 754–765. doi:10.1002/ana.24789
Khare, P., Dave, K. M., Kamte, Y. S., Manoharan, M. A., O’Donnell, L. A., Manickam, D. S., et al. (2021). Development of lipidoid nanoparticles for siRNA delivery to neural cells. AAPS J. 24, 8. doi:10.1208/s12248-021-00653-2
Knudsen, K. B., Northeved, H., Ek, P. K., Permin, A., Gjetting, T., Andresen, T. L., et al. (2015). In vivo toxicity of cationic micelles and liposomes. Nanomedicine 11, 467–477. doi:10.1016/j.nano.2014.08.004
Kong, L., Li, X., Ni, Y., Xiao, H., Yao, Y., Wang, Y., et al. (2020). Transferrin-modified osthole PEGylated liposomes travel the blood-brain barrier and mitigate alzheimer’s disease-related pathology in APP/PS-1 mice. Int. J. Nanomedicine 15, 2841–2858. doi:10.2147/IJN.S239608
Kulkarni, J. A., Witzigmann, D., Thomson, S. B., Chen, S., Leavitt, B. R., Cullis, P. R., et al. (2021). The current landscape of nucleic acid therapeutics. Nat. Nanotechnol. 16, 630–643. doi:10.1038/s41565-021-00898-0
Kumar, P., Wu, H., McBride, J. L., Jung, K.-E., Hee Kim, M., Davidson, B. L., et al. (2007). Transvascular delivery of small interfering RNA to the central nervous system. Nature 448, 39–43. doi:10.1038/nature05901
Large, D. E., Abdelmessih, R. G., Fink, E. A., and Auguste, D. T. (2021). Liposome composition in drug delivery design, synthesis, characterization, and clinical application. Adv. Drug Deliv. Rev. 176, 113851. doi:10.1016/j.addr.2021.113851
Lechanteur, A., Sanna, V., Duchemin, A., Evrard, B., Mottet, D., Piel, G., et al. (2018). Cationic liposomes carrying siRNA: Impact of lipid composition on physicochemical properties, cytotoxicity and endosomal escape. Nanomaterials 8, 270. doi:10.3390/nano8050270
Liang, C.-S., Li, D.-J., Yang, F.-C., Tseng, P.-T., Carvalho, A. F., Stubbs, B., et al. (2021). Mortality rates in Alzheimer’s disease and non-alzheimer’s dementias: a systematic review and meta-analysis. Lancet Healthy Longev. 2, e479–e488. doi:10.1016/S2666-7568(21)00140-9
Liu, C., Zhang, L., Zhu, W., Guo, R., Sun, H., Chen, X., et al. (2020). Barriers and strategies of cationic liposomes for cancer gene therapy. Mol. Ther. Methods Clin. Dev. 18, 751–764. doi:10.1016/j.omtm.2020.07.015
Maude, S. L., Frey, N., Shaw, P. A., Aplenc, R., Barrett, D. M., Bunin, N. J., et al. (2014). Chimeric antigen receptor T cells for sustained remissions in leukemia. N. Engl. J. Med. 371, 1507–1517. doi:10.1056/NEJMoa1407222
Mendell, J. R., Al-Zaidy, S., Shell, R., Arnold, W. D., Rodino-Klapac, L. R., Prior, T. W., et al. (2017). Single-dose gene-replacement therapy for spinal muscular atrophy. N. Engl. J. Med. 377, 1713–1722. doi:10.1056/NEJMoa1706198
Meng, Z., O’Keeffe-Ahern, J., Lyu, J., Pierucci, L., Zhou, D., Wang, W., et al. (2017). A new developing class of gene delivery: messenger RNA-based therapeutics. Biomater. Sci. 5, 2381–2392. doi:10.1039/c7bm00712d
Mishra, N., Ashique, S., Garg, A., Rai, V. K., Dua, K., Goyal, A., et al. (2022). Role of siRNA-based nanocarriers for the treatment of neurodegenerative diseases. Drug Discov. Today 27, 1431–1440. doi:10.1016/j.drudis.2022.01.003
Mullard, A. (2021). More Alzheimer’s drugs head for FDA review: What scientists are watching. Nature 599, 544–545. doi:10.1038/d41586-021-03410-9
Naseri, N., Valizadeh, H., and Zakeri-Milani, P. (2015). Solid lipid nanoparticles and nanostructured lipid carriers: structure, preparation and application. Adv. Pharm. Bull. 5, 305–313. doi:10.15171/apb.2015.043
Orkin, S. H., and Motulsky, A. G. (1996). Report and recommendations of the panel to assess the NIH investment in research on gene therapy. Bull. Med. Ethics 116, 10
Parambi, D. G. T., Alharbi, K. S., Kumar, R., Harilal, S., Batiha, G. E.-S., Cruz-Martins, N., et al. (2022). Gene therapy approach with an emphasis on growth factors: theoretical and clinical outcomes in neurodegenerative diseases. Mol. Neurobiol. 59, 191–233. doi:10.1007/s12035-021-02555-y
Patil, S., Gao, Y.-G., Lin, X., Li, Y., Dang, K., Tian, Y., et al. (2019). The development of functional non-viral vectors for gene delivery. Int. J. Mol. Sci. 20, 5491. doi:10.3390/ijms20215491
Peng, Y., Zhao, Y., Chen, Y., Yang, Z., Zhang, L., Xiao, W., et al. (2018). Dual-targeting for brain-specific liposomes drug delivery system: synthesis and preliminary evaluation. Bioorg. Med. Chem. 26, 4677–4686. doi:10.1016/j.bmc.2018.08.006
Pereira, S., Santos, R. S., Moreira, L., Guimarães, N., Gomes, M., Zhang, H., et al. (2021). Lipoplexes to deliver oligonucleotides in gram-positive and gram-negative bacteria: towards treatment of blood infections. Pharmaceutics 13, 989. doi:10.3390/pharmaceutics13070989
Polack, F. P., Thomas, S. J., Kitchin, N., Absalon, J., Gurtman, A., Lockhart, S., et al. (2020). Safety and efficacy of the BNT162b2 mRNA covid-19 vaccine. N. Engl. J. Med. 383, 2603–2615. doi:10.1056/NEJMoa2034577
Putzke, S., Feldhues, E., Heep, I., Ilg, T., and Lamprecht, A. (2020). Cationic lipid/pDNA complex formation as potential generic method to generate specific IRF pathway stimulators. Eur. J. Pharm. Biopharm. 155, 112–121. doi:10.1016/j.ejpb.2020.08.010
Qu, B., Li, X., Guan, M., Li, X., Hai, L., Wu, Y., et al. (2014). Design, synthesis and biological evaluation of multivalent glucosides with high affinity as ligands for brain targeting liposomes. Eur. J. Med. Chem. 72, 110–118. doi:10.1016/j.ejmech.2013.10.007
Rastegar, M., Hotta, A., Pasceri, P., Makarem, M., Cheung, A. Y. L., Elliott, S., et al. (2009). MECP2 isoform-specific vectors with regulated expression for rett syndrome gene therapy. PLoS One 4, e6810. doi:10.1371/journal.pone.0006810
Rassu, G., Soddu, E., Posadino, A. M., Pintus, G., Sarmento, B., Giunchedi, P., et al. (2017). Nose-to-brain delivery of BACE1 siRNA loaded in solid lipid nanoparticles for Alzheimer’s therapy. Colloids Surf. B Biointerfaces 152, 296–301. doi:10.1016/j.colsurfb.2017.01.031
Rungta, R. L., Choi, H. B., Lin, P. J., Ko, R. W., Ashby, D., Nair, J., et al. (2013). Lipid nanoparticle delivery of siRNA to silence neuronal gene expression in the brain. Mol. Ther. Nucleic Acids 2, e136. doi:10.1038/mtna.2013.65
Salem, H. F., Ahmed, S. M., Hassaballah, A. E., and Omar, M. M. (2015). Targeting brain cells with glutathione-modulated nanoliposomes: In vitro and in vivo study. Drug Des. devel. Ther. 9, 3705–3727. doi:10.2147/DDDT.S85302
Scioli Montoto, S., Muraca, G., and Ruiz, M. E. (2020). Solid lipid nanoparticles for drug delivery: pharmacological and biopharmaceutical aspects. Front. Mol. Biosci. 7, 587997. doi:10.3389/fmolb.2020.587997
Selvaraj, N., Wang, C.-K., Bowser, B., Broadt, T., Shaban, S., Burns, J., et al. (2021). Detailed protocol for the novel and scalable viral vector upstream process for AAV gene therapy manufacturing. Hum. Gene Ther. 32, 850–861. doi:10.1089/hum.2020.054
Sessa, M., Lorioli, L., Fumagalli, F., Acquati, S., Redaelli, D., Baldoli, C., et al. (2016). Lentiviral haemopoietic stem-cell gene therapy in early-onset metachromatic leukodystrophy: an ad-hoc analysis of a non-randomised, open-label, phase 1/2 trial. Lancet 388, 476–487. doi:10.1016/S0140-6736(16)30374-9
Sudhakar, V., and Richardson, R. M. (2019). Gene therapy for neurodegenerative diseases. Neurotherapeutics 16, 166–175. doi:10.1007/s13311-018-00694-0
Sun, J., and Roy, S. (2021). Gene-based therapies for neurodegenerative diseases. Nat. Neurosci. 24, 297–311. doi:10.1038/s41593-020-00778-1
Tanaka, H., Nakatani, T., Furihata, T., Tange, K., Nakai, Y., Yoshioka, H., et al. (2018). In vivo introduction of mRNA encapsulated in lipid nanoparticles to brain neuronal cells and astrocytes via intracerebroventricular administration. Mol. Pharm. 15, 2060–2067. doi:10.1021/acs.molpharmaceut.7b01084
Taylor, C. A., Greenlund, S. F., McGuire, L. C., Lu, H., and Croft, J. B. (2017). Deaths from Alzheimer’s disease—United States, 1999–2014. MMWR. Morb. Mortal. Wkly. Rep. 66, 521–526. doi:10.15585/mmwr.mm6620a1
Tenchov, R., Bird, R., Curtze, A. E., and Zhou, Q. (2021). Lipid Nanoparticles─From liposomes to mRNA vaccine delivery, a landscape of research diversity and advancement. ACS Nano 15, 16982–17015. doi:10.1021/acsnano.1c04996
Trapani, A., De Giglio, E., Cometa, S., Bonifacio, M. A., Dazzi, L., Di Gioia, S., et al. (2021). Dopamine-loaded lipid based nanocarriers for intranasal administration of the neurotransmitter: a comparative study. Eur. J. Pharm. Biopharm. 167, 189–200. doi:10.1016/j.ejpb.2021.07.015
Tsakiri, M., Naziris, N., and Demetzos, C. (2021). Innovative vaccine platforms against infectious diseases: under the scope of the COVID-19 pandemic. Int. J. Pharm. 610, 121212. doi:10.1016/j.ijpharm.2021.121212
Voysey, M., Clemens, S. A. C., Madhi, S. A., Weckx, L. Y., Folegatti, P. M., Aley, P. K., et al. (2021). Safety and efficacy of the ChAdOx1 nCoV-19 vaccine (AZD1222) against SARS-CoV-2: an interim analysis of four randomised controlled trials in Brazil, South Africa, and the UK. Lancet 397, 99–111. doi:10.1016/S0140-6736(20)32661-1
Wahane, A., Waghmode, A., Kapphahn, A., Dhuri, K., Gupta, A., Bahal, R., et al. (2020). Role of lipid-based and polymer-based non-viral vectors in nucleic acid delivery for next-generation gene therapy. Molecules 25, 2866. doi:10.3390/molecules25122866
Walther, W., and Stein, U. (2000). Viral vectors for gene transfer: a review of their use in the treatment of human diseases. Drugs 60, 249–271. doi:10.2165/00003495-200060020-00002
Wang, L., Chen, S.-R., Ma, H., Chen, H., Hittelman, W. N., Pan, H.-L., et al. (2018). Regulating nociceptive transmission by VGluT2-expressing spinal dorsal horn neurons. J. Neurochem. 147, 526–540. doi:10.1111/jnc.14588
Watanabe, M., Nishikawaji, Y., Kawakami, H., and Kosai, K.-I. (2021). Adenovirus biology, recombinant adenovirus, and adenovirus usage in gene therapy. Viruses 13, 2502. doi:10.3390/v13122502
Wiley, J. (2021). Alzheimer’s disease facts and figures. Alzheimers Dement. 17, 327–406. doi:10.1002/alz.12328
Wojnarowska, Z., Smolka, W., Zotova, J., Knapik-Kowalczuk, J., Sherif, A., Tajber, L., et al. (2018). The effect of electrostatic interactions on the formation of pharmaceutical eutectics. Phys. Chem. Chem. Phys. 20, 27361–27367. doi:10.1039/C8CP05905E
Wuh-Liang, H., Shin-ichi, M., Sheng-Hong, T., Kai-Yuan, T., Ni-Chung, L., Yin-Hsiu, C., et al. (2012). Gene therapy for aromatic l-amino acid decarboxylase deficiency. Sci. Transl. Med. 4, 134ra61. doi:10.1126/scitranslmed.3003640
Yuan, Z., Zhao, L., Zhang, Y., Li, S., Pan, B., Hua, L., et al. (2018). Inhibition of glioma growth by a GOLPH3 siRNA-loaded cationic liposomes. J. Neurooncol. 140, 249–260. doi:10.1007/s11060-018-2966-6
Zabel, M. D. (2013). Lipopeptide delivery of siRNA to the central nervous system. Methods Mol. Biol. 948, 251–262. doi:10.1007/978-1-62703-140-0_17
Zhao, Y., and Huang, L. (2014). “Chapter two - lipid nanoparticles for gene delivery,” in Nonviral vectors for gene therapy. Editors L. Huang, D. Liu, and G. Wagner (Academic Press), 13–36. doi:10.1016/B978-0-12-800148-6.00002-X
Zhu, F.-C., Guan, X.-H., Li, Y.-H., Huang, J.-Y., Jiang, T., Hou, L.-H., et al. (2020). Immunogenicity and safety of a recombinant adenovirus type-5-vectored COVID-19 vaccine in healthy adults aged 18 years or older: a randomised, double-blind, placebo-controlled, phase 2 trial. Lancet 396, 479–488. doi:10.1016/S0140-6736(20)31605-6
Keywords: lipid nanoparticles, liposomes, lipoplexes, solid lipid nanoparticles, gene delivery, RNA, central nervous system
Citation: Tsakiri M, Zivko C, Demetzos C and Mahairaki V (2022) Lipid-based nanoparticles and RNA as innovative neuro-therapeutics. Front. Pharmacol. 13:900610. doi: 10.3389/fphar.2022.900610
Received: 20 March 2022; Accepted: 13 July 2022;
Published: 09 August 2022.
Edited by:
Nejat Duzgunes, University of the Pacific, United StatesReviewed by:
Mario Ariatti, University of KwaZulu-Natal, South AfricaCopyright © 2022 Tsakiri, Zivko, Demetzos and Mahairaki. This is an open-access article distributed under the terms of the Creative Commons Attribution License (CC BY). The use, distribution or reproduction in other forums is permitted, provided the original author(s) and the copyright owner(s) are credited and that the original publication in this journal is cited, in accordance with accepted academic practice. No use, distribution or reproduction is permitted which does not comply with these terms.
*Correspondence: Vasiliki Mahairaki, dm1hY2hhaTFAamhtaS5lZHU=
†These authors share last authorship