- 1Department of Spine Surgery, The Second Affiliated Hospital of Nantong University, Nantong, Jiangsu, China
- 2Department of Pharmacology, School of Pharmacy, Nantong University, Nantong, Jiangsu, China
Paeoniflorin, a terpenoid glycoside compound extracted from Paeonia lactiflora Pall, shows preventive and therapeutic effects in various types of nervous system disorders. However, to date, no comprehensive knowledge on the pharmacological effects of paeoniflorin on the nervous system is available online. Clarification of this issue may be useful for the development of paeoniflorin as a new drug for the treatment of nervous system disorders. To this end, the authors summarize the pharmacological aspects of paeoniflorin and its possible mechanisms, such as restoration of mitochondrial function; inhibition of neuroinflammation, oxidative stress, and cellular apoptosis; activation of adenosine A1 receptor, cAMP response element-binding protein (CREB) and extracellular signal-regulated kinase 1/2 (ERK1/2); or enhancement of brain-derived neurotrophic factor and serotonin function, in the prevention of disorders such as cerebral ischemia, subarachnoid hemorrhage, vascular dementia, Alzheimer’s disease, Parkinson’s disease, depression, post-traumatic syndrome disorder, and epilepsy, by reviewing the previously published literature.
Introduction
Paeoniflorin (Figure 1; molecular formula: C23H28O11; molecular weight: 482.46; solvent: dimethyl sulfoxide) is a highly water-soluble single terpenoid glycoside compound first extracted from Paeonia lactiflora Pall (Liu et al., 2005a). It is also found in other Paeoniaceae families, such as Paeoniae veitchii and Paeoniae suffrusticosa (Zhang et al., 2019). Functional studies have shown that paeoniflorin administration exhibits various pharmacological activities, such as anti-inflammatory (Zhang et al., 2019; Xin et al., 2019), anti-oxidative (Yuan et al., 2020; Wang et al., 2020), anti-allergic (Wang and Cheng, 2018), anti-proliferative (Xiang et al., 2020), and anti-diabetic (Sun et al., 2021a) activities, making it a promising agent for ameliorating numerous pathological processes, such as allergy (Wang and Cheng, 2018), cancer (Xiang et al., 2020), and diabetes-associated complications (Sun et al., 2021a). In the nervous system, paeoniflorin administration shows a variety of pharmacological activities, such as ameliorating the pathogenesis of cerebral ischemia (Wu et al., 2020a, Guo et al., 2012, Liu et al., 2005b, Xiao et al., 2005), subarachnoid hemorrhage (SAH) (Wang et al., 2020), learning and memory deficits (Sun et al., 2017), vascular dementia (Zhang et al., 2016; Luo et al., 2018), Alzheimer’s disease (Gu et al., 2016; Kong et al., 2020), Parkinson’s disease (Liu et al., 2006; Zheng et al., 2017), depression (Chen et al., 2019; Li et al., 2020), and post-traumatic syndrome disorder (PTSD) (Chen et al., 2020a), pain (Zhou et al., 2019; Liu et al., 2020), and glioblastoma (Wang et al., 2018a; Yu et al., 2019a), suggesting that dietary supplementation with paeoniflorin may have obvious neuroprotective effects on the central nervous system. A comprehensive understanding of the pharmacological effects of paeoniflorin on the central nervous system, which is not available in the literature published online, may advance the development of paeoniflorin as a new drug for the treatment of central nervous system disorders.
Pharmacodynamic studies had shown that paeoniflorin has a low oral bioavailability (about 2.32%) (Yu et al., 2019b), which is due to its metabolic conversion to benzoic acid by carboxylesterase in the intestinal microbiota (Takeda et al., 1995; Yu et al., 2019b). Pharmacological inhibition of carboxylesterase activity can block the conversion of paeoniflorin to benzoic acid in the intestinal microbiota and in the paeoniflorin co-incubation system (Yu et al., 2019b). This property indicates that the paeoniflorin may have difficulty reaching the brain. However, previous studies have also reported that paeoniflorin can be detected in the hippocampus when administered subcutaneously (Xia et al., 2007), and intravenous administration of paeoniflorin to male rats can result in a detectable paeoniflorin in the brain (He et al., 2004). Paeoniflorin has also been detected in cerebral nuclei of the amygdala, hypothalamus, thalamus, and cortex 30 min after intraperitoneal administration (Zhang et al., 2008). Once paeoniflorin enters the brain, it produces neuroprotective effects through a variety of mechanisms, including inhibition of neuroinflammation (Liu et al., 2006; Guo et al., 2012; Liu et al., 2020), oxidative stress (Lan et al., 2013; Wang et al., 2018b; Wang et al., 2020), cellular apoptosis (Gu et al., 2016; Cong et al., 2019; Wang et al., 2020), and mitochondrial dysfunction (Li et al., 2014; Wang et al., 2014; Cong et al., 2019), activation of cyclic adenosine monophosphate-response element binding protein (CREB) (Zhang et al., 2017; Hu et al., 2019) and protein kinase B (Akt) (Wu et al., 2013; Ma et al., 2018), inhibition of c-Jun N-terminal kinase (JNK) (Chu et al., 2017), or enhancement of brain-derived neurotrophic factor (BDNF) (Chen et al., 2019; Mu et al., 2020) and serotonin functions (Qiu et al., 2013; Qiu et al., 2018). Another possible mechanism for the neuroprotective effect of paeoniflorin is its conversion to benzoic acid, an intermittent compound that can be transported to the brain via monocarboxylate transporter 1 (MCT1) (Kido et al., 2000) and produce neuroprotective effects in schizophrenia (Lane et al., 2013), dementia (Lin et al., 2019; Lin et al., 2021), and early-phase Alzheimer’s disease (Lin et al., 2014). The results on the distribution of paeoniflorin in the brain suggest that paeoniflorin may be able to directly cross the blood-brain barrier, and that an important way by which paeoniflorin exerts neuroprotective effects in the central nervous system may be related to its direct action on cells in the brain.
Although paeoniflorin can enter the brain when injected subcutaneously (Xia et al., 2007), intravenously (He et al., 2004), or intraperitoneally (Zhang et al., 2008), researchers still do not know exactly how paeoniflorin crosses the blood-brain barrier. Improving the delivery of paeoniflorin to the brain could improve its therapeutic efficacy in nervous system disorders. To this end, future studies should focus on the physical, chemical, and structural chemistry, biocompatibility, pharmacology, and mechanisms of paeoniflorin to cross the blood-brain barrier. At this stage, several methods could be considered to improve the efficacy of paeoniflorin when delivered to the brain. First, based on the possible promoting effect of the main components of Chuanxiong (a messenger drug that can increase the distribution of drugs in brain tissues) (Wang et al., 2011; Zhen et al., 2012), such as ligustilide, senkyunolide I and senkyunolide A, on the ability of paeoniflorin to cross the blood-brain barrier under in vitro conditions (Hu et al., 2016), researchers could use Chuanxiong or its components to improve the efficacy of paeoniflorin delivery to the brain. Second, researchers could use the other alternative methods to improve the delivery efficacy of paeoniflorin to the brain. Intranasal administration is an effective method to reach the brain directly through the olfactory and trigeminal neural systems, bypassing the blood-brain barrier. In a previous study, researchers found that there is a high concentration of paeoniflorin in the central nervous after intranasal administration of the nanocrystal formulation of paeoniflorin (Wu et al., 2020b), suggesting that intranasal administration may be an alternative method to improve the efficacy of paeoniflorin delivery to the brain. Third, because paeoniflorin can be converted to benzoic acid in advance, researchers could use the strategy of carboxylesterase inhibition to block the conversion of paeoniflorin to benzoic acid (Yu et al., 2019b) and thus increase the concentration of paeoniflorin in the brain. These hypotheses should be tested in future studies.
It is worth noting that although there are few human clinical experiments on the pharmacological effects of paeoniflorin in diseases of the nervous system, in recent years clinical studies have been conducted on the treatment of cerebrovascular diseases including cerebral infarction, cerebral hypoperfusion syndrome and cerebral ischemia, and intracranial arterial stenosis of the brain by Chinese recipes containing Paeonia, such as the Bu Yang Huan Wu, Si Wu, and Huang Chi Wu decoctions. The detailed information on these studies can be found in the following websites: http://www.chinadrugtrials.org.cn and http://www.chictr.org.cn (the Chinese Clinical Trial Registry), http://clinicaltrials.gov (the Global Clinical Trial Registry), and http://clinicaltrialsregister.eu (the EU Clinical Trials Register). Therefore, further elucidation of the pharmacological aspects of paeoniflorin in the nervous system could be of great significance for the development of paeoniflorin as a novel drug for the treatment of central nervous system disorders. However, no comprehensive review on this topic has been presented in public sources so far. In this context, the authors summarize and discuss the pharmacological effects of paeoniflorin and its possible mechanisms in the prevention and/or treatment of nervous system disorders by searching previously published literatures and databases, with the aim of advancing the application of paeoniflorin and paeoniflorin-containing drugs in clinical use and daily life.
Pharmacological effects of paeoniflorin in cerebral ischemia
Cerebral ischemia is a common pathological condition that can cause moderate to severe damage to brain function. It usually occurs in conditions such as hypo-perfusion, stroke, and anemia. Increased levels of oxidative stress and deficits in anti-oxidation are two common aspects of the pathogenesis of cerebral ischemia (Chen et al., 2020b). In a previous study, oral administration of paeoniflorin (60, 120, and 240 mg/kg, 7 days) in a rat model of cerebral ischemia induced by middle cerebral artery occlusion (MCAO) was shown to improve neurological deficits, reduce infarct volume, and decrease neuronal edema by increasing the level of superoxide dismutase (SOD) and decreasing the level of malondialdehyde (MDA) in the cortical area (Wu et al., 2020a) (Figure 2A). This ameliorates the pathological changes associated with cell apoptosis and alleviates the MCAO-induced increase in lactate dehydrogenase, Na+-K+-ATPase activity, and intracellular Ca2+ concentration (Wu et al., 2020a) (Figure 2A). In addition to oxidative stress, microglia-mediated neuroinflammation also plays a key role in the pathogenesis of cerebral ischemia (Jayaraj et al., 2019). Therefore, inhibition of neuroinflammation may be a potential strategy for the treatment of cerebral ischemia (Malone et al., 2019). Treatment with paeoniflorin at a dose of 2.5 or 5 mg/kg (i.p., twice daily) for 7 or 14 days (Guo et al., 2012; Zhang et al., 2015a) or administered intravenously at a dose of 15 or 20 mg/kg before blockade of blood flow (Tang et al., 2010) has been shown to ameliorate neurological deficits and neuronal apoptosis induced by MCAO (with or without reperfusion) by suppressing the production of pro-inflammatory mediators, such as interleukin-1β (IL-1β), tumor necrosis factor-α (TNF-α), and inducible nitric oxide synthase (iNOS), as well as the overactivation of astrocytes (Figure 2A). The pathologically elevated nitric oxide (NO) derived from iNOS, together with reactive oxygen species, causes oxido-nitrosative stress that leads to neurotoxic effects via mechanisms such as down-regulation of neurotrophic factor expression and monoamine levels (Kasala et al., 2014; Yang et al., 2017). Since paeoniflorin administration can inhibit both oxidative stress and neuroinflammation (Jayaraj et al., 2019; Wu et al., 2020a), NO may help to establish a causal relationship between the anti-oxidative stress and the anti-neuroinflammatory effect of paeoniflorin. However, since no direct evidence of the inhibitory effect of paeoniflorin on NO production has been provided in animal models of cerebral ischemia, this hypothesis should be solved in future studies (Figure 2A).
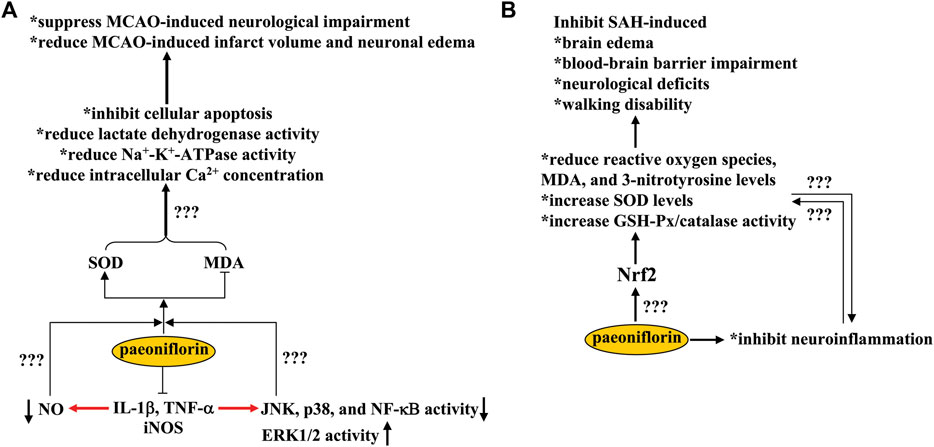
FIGURE 2. Effects and mechanisms of paeoniflorin in cerebral ischemia and subarachnoid hemorrhage. (A) Paeoniflorin administration suppresses MCAO-induced neurological impairment and reduces MCAO-induced infarct volume and neuronal edema through mechanisms dependent on SOD elevation and MDA decrease, including inhibition of cellular apoptosis, lactate dehydrogenase/Na+-K+-ATPase activity, and intracellular Ca2+ concentrations in the brain. Paeoniflorin can also suppress the production of pro-inflammatory mediators in the brain, such as IL-1β, TNF-α, and iNOS, which subsequently leads to a decrease in NO production, a decrease in JNK, p38, and NF-κB activity, and an increase in ERK1/2 activity. However, it remains unclear whether the neuroprotective effects of paeoniflorin could be mediated by these processes. (B) Paeoniflorin administration may suppress SAH-induced brain edema, blood-brain barrier impairments, neurological deficits, and walking disability by reducing reactive oxygen species, MDA, and 3-nitrotyrosine levels and increasing SOD levels and GSH-Px/catalase activities in the brain in a manner that is dependent on Nrf2 activation. However, how paeoniflorin triggers Nrf2 activation remains unclear.
In addition to inducing oxido-nitrosative stress, overproduction of pro-inflammatory cytokines can also induce activation of c-Jun N-terminal kinase (JNK) and p38, inhibition of extracellular signal-regulated kinase 1/2 (ERK1/2), degradation of inhibitor kappa B-α (IκB-α), and activation of nuclear factor kappa B (NF-κB) (Neacsu et al., 2015; Biriken and Yazihan, 2021) (Figure 2A). The therapeutic effects of paeoniflorin in cerebral ischemia might be mediated by suppressing JNK/p38 activity, enhancing ERK1/2 activity, and inhibiting IκB-α and NF-κB expression in the hippocampus, cortex, and striatum, thereby reducing the Bax/Bcl-2 ratio in ischemic brain tissue (Tang et al., 2010; Guo et al., 2012; Zhang et al., 2015a; Zhang et al., 2017). JNK-dependent down-regulation of connexin 43 expression may also contribute to the neuroprotective effects of paeoniflorin in cerebral ischemia (Chu et al., 2017). However, whether the regulatory effects of paeoniflorin on JNK, p38, ERK1/2, IκB-α, NF-κB, and connexin 43 are directly related to its anti-oxidative stress effects remains unclear (Figure 2A). It is worth noting that studies in vitro have reported that pretreatment with paeoniflorin at a dose of 100 or 200 μM can protect cultured neurons from N-methyl-D-aspartic acid receptor (NMDA)- or TNF-α-induced cellular apoptosis, by rebalancing the expression of Bcl-2 and Bax and promoting CREB- and Ca2+/calmodulin-dependent protein kinase II (CaMKII) signaling (Guo et al., 2012; Zhang et al., 2017). Since excessive accumulation of glutamate and subsequent activation of NMDA receptors are central mechanisms for the induction of excitotoxicity in ischemic brain tissue (Chamorro et al., 2016; Fern and Matute, 2019), it is necessary to investigate whether the neuroprotective effects of paeoniflorin in cerebral ischemia are mediated by its inhibitory effects on glutamate receptors. Perhaps, researchers should use various molecular techniques to determine whether paeoniflorin can bind directly to glutamate receptors.
Pharmacological effects of paeoniflorin in subarachnoid hemorrhage
SAH is a serious cerebrovascular disease with a high mortality rate. The infiltration of blood into the subarachnoid space of the brain after aneurysm rupture reduces cerebral blood flow and triggers neuronal apoptosis, partly through the induction of oxidative stress and neuroinflammation (Zhang et al., 2021a; Zhan et al., 2021). Therefore, inhibition of oxidative stress and neuroinflammation may be a potential strategy for the treatment of SAH. Treatment with paeoniflorin at a dose of 5 mg/kg after SAH (twice daily, i.p., 3 days) has been reported to inhibit SAH-induced brain edema, blood-brain barrier impairment, neurological deficits, and walking disability in rats by 1) reducing levels of reactive oxygen species, MDA, and 3-nitrotyrosine, 2) increasing the levels of SOD and the activity of glutathione peroxidase (GSH-Px) and catalase in a nuclear factor erythroid-related factor 2 (Nrf2)-dependent manner, and 3) inhibiting the production of pro-inflammatory cytokines in the cortex (Wang et al., 2020) (Figure 2B). These in vivo results were partially supported by the inhibitory effect of paeoniflorin (30 or 100 μM) on hematoma lysate-induced decrease in cell viability in primary cultured cortical neurons (Wang et al., 2020). Overall, these results indicate that paeoniflorin may be a potential drug for the treatment of SAH. However, because the precise molecular and signaling mechanisms underlying the neuroprotective effects of paeoniflorin in animal models of SAH are unclear, further studies should be conducted to elucidate the mechanistic aspect of paeoniflorin in SAH. For example, researchers should clarify whether there is a causal relationship between the anti-oxidative stress and anti-neuroinflammatory effects of paeoniflorin at SAH and how paeoniflorin induces Nrf2 activation: by up-regulating Nrf2 expression, inducing nuclear translocation of Nrf2, or direct binding to Nrf2 (Figure 2B)? Answering these questions may help to find new avenues for paeoniflorin research. Since excessive accumulation of glutamate is an important pathological change for the induction of neuronal damage in SAH (Sun et al., 2021b) and paeoniflorin can protect cultured neurons from glutamate-induced cellular damage through anti-oxidative stress and anti-apoptosis (Mao et al., 2010; Sun et al., 2012), researchers should also investigate whether the neuroprotective effects of paeoniflorin in animal models of SAH are mediated by inhibition of glutamate receptors.
Pharmacological effects of paeoniflorin in pathogenesis associated with cognition, learning, and memory impairment
Learning and memory impairment is a common phenomenon in modern society. They can be observed in various pathological conditions, such as vascular dementia, Alzheimer’s disease, and aging. Improving cognition, learning, and memory is of great importance for the treatment of neurodegenerative diseases. However, no effective drugs have been approved so far. Paeoniflorin could be a potential drug for this purpose. First, daily administration of paeoniflorin (i.p.) at a dose of 40 mg/kg over a 28-day period ameliorated the learning and memory impairment in a common carotid artery clipping model in rats by directing hippocampal microglia toward an anti-inflammatory phenotype and suppressing activation of mammalian target of rapamycin (mTOR)-NF-κB signaling and down-regulation of phosphoinositide 3-kinase (PI3K)-Akt signaling (Luo et al., 2018) (Figure 3). These effects of paeoniflorin may be mediated by cannabinoid receptor 2 (CB2R), as CB2R inhibition can abolish the regulatory effects of paeoniflorin on learning and memory, neuroinflammatory response, and mTOR-NF-κB and PI3K-Akt signaling (Luo et al., 2018) (Figure 3). In another study by Zhang et al. (2016), oral administration of paeoniflorin (20 and 40 mg/kg, daily for 28 days) was shown to inhibit neuronal apoptosis and increase brain BDNF expression in a rat vascular dementia model induced by bilateral common carotid artery occlusion (Zhang et al., 2016) (Figure 3). In future studies, researchers should investigate whether paeoniflorin supplementation improves learning and memory abilities in the carotid artery clipping/occlusion model by acting directly on the CB2R and whether paeoniflorin-mediated activation of CB2R improves learning and memory abilities via the BDNF signaling. Furthermore, as the CB2R is expressed in different cell types such as microglia (de Oliveira et al., 2022), astrocytes (Fernández-Trapero et al., 2017), neurons (Onaivi et al., 2012), and endothelial cells (Nuñez-Lumbreras et al., 2021), researchers should also determine the cellular basis for the ameliorative effects of paeoniflorin on learning and memory. Indeed, some of these hypotheses have been supported by previous studies. For example, paeoniflorin was reported to have the ability to activate the CB2R using a combination of the luc2P/CRE/Hygro reporter gene and the eukaryotic PIRES2-EGFP-CB2R expression plasmid (Luo et al., 2018).
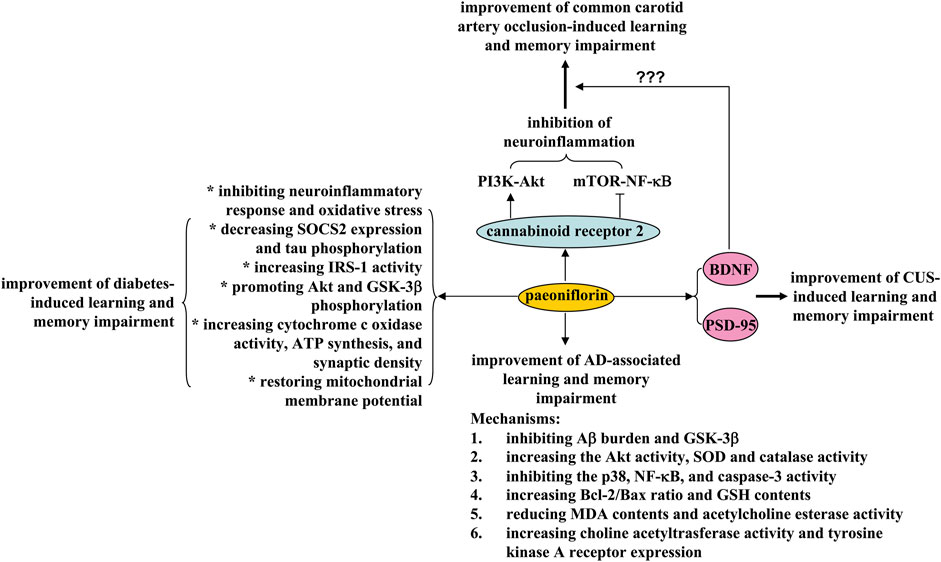
FIGURE 3. Effects and mechanisms of paeoniflorin on learning and memory impairment. Paeoniflorin administration may ameliorate 1) learning and memory impairment induced by common carotid artery occlusion by inhibiting neuroinflammation in a cannabinoid receptor 2-dependent manner; paeoniflorin-triggered activation of cannabinoid receptor 2 induces activation of PI3K-Akt signaling and inhibition of mTOR-NF-κB signaling, thereby improving learning and memory impairment induced by carotid artery occlusion, 2) diabetes-induced learning and memory impairment by inhibiting neuroinflammation and oxidative stress, decreasing SOCS2 expression and tau phosphorylation, increasing IRS-1 activity, promoting Akt and GSK-3β phosphorylation, increasing cytochrome c oxidase activity, ATP synthesis, and synaptic density, and restoration of mitochondrial membrane potential, 3) Alzheimer’s disease-associated learning and memory impairment by inhibiting Aβ burden and GSK-3β, increasing the Akt activity, SOD and catalase activity, inhibiting p38, NF-κB and caspase-3 activity, increasing Bcl-2/Bax ratio and GSH content, reducing MDA content and acetylcholine esterase activity, increasing choline acetyltrasferase activity and tyrosine kinase A receptor expression, and 4) CUS-induced learning and memory impairment by enhancing PSD-95 and BDNF expression in the brain.
Second, chronic treatment with paeoniflorin (5 mg/kg, i.p., 28 days) can reduce the learning and memory ability of transgenic mice with five familial Alzheimer’s disease (5× FAD) mutations via adenosine A1 receptor-dependent inhibition of Aβ burden and neuroinflammatory response (Kong et al., 2020) (Figure 3), and treatment with 2 mg/kg paeoniflorin (1 day, i.p.) can decrease escape distance and latency in mice expressing the human mutant PS2 or in mice with the genetic background C57BL/6×DBA/2 by increasing Akt activity, inhibiting p38, NF-κB, and caspase-3 activity, and increasing the Bcl-2/Bax ratio, which subsequently suppresses neuronal apoptosis and neuroinflammation in the cortex (Gu et al., 2016) (Figure 3). Moreover, chronic paeoniflorin treatment (5 mg/kg, i.p., twice daily, 4 weeks) ameliorates memory deficits in amyloid precursor protein (APP) and presenilin 1 (PS1) double-transgenic (APP/PS1) mice by inhibiting Aβ burden and neuroinflammation in a manner dependent on inhibition of glycogen synthase kinase 3β (GSK-3β) and NF-κB (Zhang et al., 2015b) (Figure 3). Further analysis showed that chronic paeoniflorin treatment (7.5, 15, and 30 mg/kg, once daily, 20 days, i.p.) can reverse Aβ1–42-induced decreases in GSH content and SOD and catalase activity, increases in MDA content and acetylcholine esterase activity, and decreases in choline acetyltrasferase activity and tyrosine kinase A receptor expression in rat hippocampus (Zhong et al., 2009) (Figure 3). These results indicate that anti-neuroinflammation, anti-oxidative stress, and anti-apoptosis may mediate the ameliorative effects of paeoniflorin on learning and memory deficits in animal models of Alzheimer’s disease. A puzzling question is whether and how the adenosine A1 receptor actually mediates the regulatory effects of paeoniflorin on neuroinflammation, oxidative stress, and anti-apoptosis, thereby exerting learning- and memory-enhancing effects. Is there a possibility that paeoniflorin binds directly to the adenosine A1 receptor or requires another accessory molecule to activate the adenosine A1 receptor? This question was clarified to some extent by a previous study demonstrating that paeoniflorin binds specifically with binding sites of the adenosine A1 receptor for 5′-N-ethylcarboxamidoadenosine (NECA) (Liu et al., 2005b). However, it should be noted that this explanation is still a hypothesis. It should be verified by further experiments.
In vitro studies have reported that incubation with paeoniflorin (50, 100, and 200 μM) can ameliorate okadaic acid-induced cell swelling, microtubule de-stabilization, and tau hyper-phosphorylation in cultured SH-SY5Y cells by inhibiting the activity of calpain, GSK-3β, and Akt (Ma et al., 2018). In cultured PC12 cells, paeoniflorin (2, 10, and 50 μM) prevents Aβ25-35-induced cell damage by reducing cytochrome c release and caspase-3/9 activity (Li et al., 2014), and in cultured hippocampal neurons paeoniflorin can inhibit the Aβ1–42-induced increase in intracellular Ca2+, decreases in GSH contents, and increases in nitrite, carbonyl protein, and MDA levels (Zhang et al., 2015b). In addition, pretreatment with paeoniflorin (50 μM, 24 h) can prevent Aβ1-42-induced nuclear translocation of NF-κB and the increase in vascular endothelial growth factor (VEGF) expression levels, which in turn reduces microglial activation and migration (Liu et al., 2015). Whether the findings in cultured cells occur in Alzheimer’s disease in vivo should be determined in future studies.
In addition to its regulatory effects in vascular dementia and Alzheimer’s disease, paeoniflorin intake may also suppress cognition, learning, and memory impairments in other models of CNS disease. For example, administration of 20 mg/kg paeoniflorin (i.p., 30 days) was reported to reverse the decrease in time spent in the target quadrant caused by chronic unpredictable stress (CUS) by increasing the expression of postsynaptic density protein 95 (PSD95) and BDNF (Liu et al., 2019) (Figure 3). Oral administration of paeoniflorin (15 or 30 mg/kg, 7 days) can also decrease latency and increase time spent in the target quadrant in the Morris water maze test in diabetic rats induced by stimuli such as high-fat diet, high sucrose, or low dose streptozotocin, by reducing the production of IL-1β and TNF-α, decreasing the expression of suppressor of cytokine signaling 2 (SOCS2), increasing the activity of insulin receptor substrate-1 (IRS-1), promoting the phosphorylation of Akt and GSK-3β, and inhibiting the hyper-phosphorylation of tau protein in the hippocampus (Sun et al., 2017) (Figure 3). In addition, it was reported that daily treatment with paeoniflorin (10 mg/kg, i.p.) reversed streptozotocin-induced cognitive deficits by increasing cytochrome c oxidase activity, adenosine triphosphate (ATP) synthesis and synaptic density, restoring mitochondrial membrane potential, and inhibiting oxidative stress in the hippocampus and cortex of mice (Wang et al., 2018b), and oral administration of paeoniflorin (1 mg/kg) 90 min before testing attenuates scopolamine-induced deficits in radial maze performance in rats by increasing acetylcholine content or activating the α1-or β1-adrenergic receptor in the striatum (Ohta et al., 1993a; Ohta et al., 1993b; Ohta et al., 1993c) (Figure 3). Investigators should further determine the common molecular basis for the anti-dementia and anti-Alzheimer effects of paeoniflorin, and investigate whether the initial inhibition of Aβ deposition mediates the regulatory effects of paeoniflorin on cognition, learning, and memory in animal models of Alzheimer’s disease?
Pharmacological effects of paeoniflorin in Parkinson’s disease
Parkinson’s disease is a devastating neurodegenerative disease in which there is progressive degeneration of striatal dopaminergic neurons. This disease can lead to severe motor disabilities that can affect the quality of daily life of patients. Due to the lack of effective drugs for the treatment of Parkinson’s disease in the clinic, this disease is in the public focus. In a previous study, oral treatment with paeoniflorin at a dose of 15 or 30 mg/kg was shown to ameliorate MPTP-induced impairments in spontaneous motor performance on the rotarod test, as well as loss of dopaminergic neurons in mice, by increasing the expression of dopaminergic transporters and tyrosine hydroxylase proteins, decreasing monoamine oxidase-B (MAO-B) and caspase-3/9 activity, and increasing Akt activity in striatal and substantia nigra (Zheng et al., 2017) (Figure 4). In another Parkinson’s disease model in rats induced by unilateral striatal 6-hydroxydopamine lesion, subcutaneous administration of paeoniflorin (5 and 10 mg/kg, twice daily for 11 days) has been shown to reduce apomorphine-induced rotation by protecting tyrosine hydroxylase-positive neurons from damage (Liu et al., 2007). In a post-treatment regimen (administered 1 h after the last MPTP dose and followed by 3 days of daily treatment at doses of 2.5 and 5 mg/kg), paeoniflorin was found to attenuate damage of to dopaminergic neurons via adenosine A1 receptor-dependent suppression of the neuroinflammatory response in the striatum (Liu et al., 2006) (Figure 4). Similar to the models of Alzheimer’s disease, further studies should clarify whether and how the adenosine A1 receptor actually mediates the regulatory effects on paeoniflorin dopaminergic cell survival and disease symptoms in animal models of Parkinson’s disease (Figure 4).
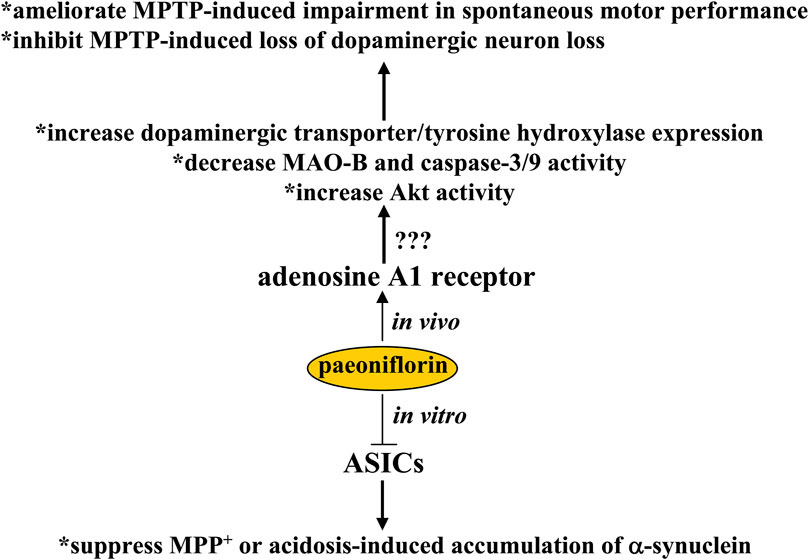
FIGURE 4. Effects and mechanisms of paeoniflorin in Parkinson’s disease. Paeoniflorin administration may ameliorate MPTP-induced impairment of spontaneous motor performance and inhibit MPTP-induced loss of dopaminergic neuron loss by increasing the expression of dopaminergic transporters and tyrosine hydroxylases, decreasing MAO-B and caspase-3/9 activity, or increasing Akt activity via a possible mechanism related to adenosine A1 receptor activation. Under in vitro conditions, paeoniflorin was found to suppress MPP+ or acidosis-induced accumulation of α-synuclein in cultured PC12 cells by inhibiting the activity or expression of ASICs. However, whether this in vitro effect on ASICs could mediate the neuroprotective effect of paeoniflorin in animal models of Parkinson’s disease remains unclear.
In vitro studies have reported that incubation with paeoniflorin (50 μM) can reduce MPP+ or acidosis-induced accumulation of α-synuclein and upregulate the expression of an autophagy-associated protein LC3-II in PC12 cells (Sun et al., 2011) (Figure 4), suggesting that induction of autophagy and inhibition of α-synuclein-associated pathology may mediate the anti-Parkinson’s disease effect of paeoniflorin. Incubation with paeoniflorin (50 μM) can also suppress the activity and expression of acid sensing ion channels (ASICs) in PC12 cells (Sun et al., 2011). Since the ASIC blocker amiloride can protect substantia nigra neurons from MPTP-induced neurodegeneration (Arias et al., 2008) and parkin-mediated enhancement of ASIC activities play a key role in the pathogenesis of neurodegeneration (Joch et al., 2007), the findings on the inhibition of ASICs by paeoniflorin may reveal a new mechanism for the anti-Parkinson’s effect of paeoniflorin (Figure 4). From the above results, the regulation of ASICs by incubation with paeoniflorin may be particularly interesting because ASICs have been widely reported to mediate the pathogenesis of various neuropsychiatric diseases, such as epilepsy (Biagini et al., 2001), Alzheimer’s disease (Gonzales and Sumien, 2017), and pain (Holton et al., 2020). Therefore, future studies need to investigate whether paeoniflorin can bind directly to ASICs, which could drive the development of regulators of ASICs.
Effect of paeoniflorin on epilepsy
Febrile seizure is the most common convulsive disorder in children (Hauser, 1994). There is a lack of effective strategies to treat febrile seizure. One potential strategy to treat febrile seizure is to correct the imbalance between glutamatergic and GABAergic neurotransmission. Paeoniflorin may be a hopeful candidate for this purpose, as oral paeoniflorin supplementation (100 mg/kg, 10 days) can suppress hyperthermia-induced seizures in immature male rats (Hino et al., 2012) (Figure 5). One of the most important mechanisms for the development of febrile seizures is the decrease of GABAnergic signals in the brain (Rating et al., 1983; Audenaert et al., 2006), and the GABA agonist can suppress hyperthermia-induced seizures in developing rats, while inhibition of the GABA receptor produces an opposite effect (Fukuda et al., 1997), suggesting that the anti-epileptic effect of paeoniflorin may be mediated by the changes in the GABAnergic signals. However, the fact is that incubation with paeoniflorin does not affect the production or release of GABA in cultured hippocampal neurons (Hino et al., 2012), which rules out the involvement of GABAnergic signals in the anti-epilepsy effect of paeoniflorin. In addition to GABA, intracellular Ca2+- and Na+-mediated excitotoxicity may also be involved in the anti-epilepsy effect of paeoniflorin, as the alteration of intracellular Ca2+ and Na+ levels may be closely related to the pathogenesis of epilepsy (Missiaen et al., 2000; Das et al., 2010), and incubation with paeoniflorin (50–200 or 300 μM) can block Na+ current (Zhang et al., 2003) and glutamate-induced increase in intracellular Ca2+ levels, membrane depolarization, and neuronal death in primary cultured hippocampal neurons (Hino et al., 2012) (Figure 5). Glutamate mainly acts on membrane receptors in neurons, such as the NMDA receptor, AMPA receptor, and metabolic glutamate receptors. However, the suppression of intracellular Ca2+ increase in cultured hippocampal neurons mediated by paeoniflorin may be mediated only by its inhibitory effects on metabolic glutamate receptor 5 (mGluR5), since incubation with paeoniflorin incubation can suppress the intracellular Ca2+ increase induced by an mGluR5 agonist but not by an NMDA or AMPA receptor agonist (Hino et al., 2012) (Figure 5). This finding suggests that paeoniflorin may be a negative regulator of mGluR5. However, in the absence of evidence to explain the regulatory mode of paeoniflorin on mGluR5, future studies should clarify whether paeoniflorin binds directly to the mGluR5 or requires another accessory molecule to regulate mGluR5. Future studies should also identify how paeoniflorin blocks the Na+ currents in primary cultured neurons. Furthermore, considering that the pathogenesis of epilepsy is related to a variety of pathological mechanisms such as astrogliosis (Seifert et al., 2010), JNK overactivation (Small et al., 2022), and microglial overactivation (Devinsky et al., 2013), researchers should conduct further experiments to investigate whether paeoniflorin exerts an antiepileptic effect via these mechanisms.
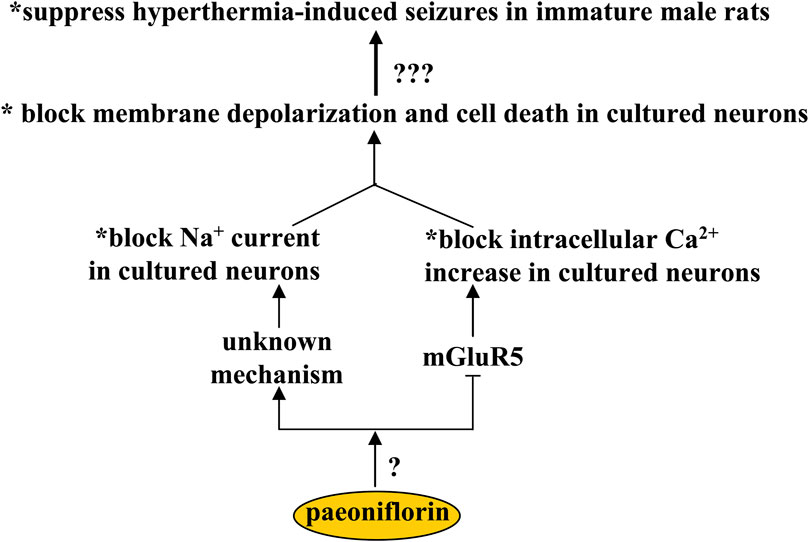
FIGURE 5. Effects and mechanisms of paeoniflorin in epilepsy. Paeoniflorin administration can suppress hyperthermia-induced seizures in immature male rats, probably by blocking membrane depolarization and cell death in primary cultured neurons. This effect of paeoniflorin on membrane depolarization and neuronal survival may be mediated by an unknown mechanism based on blocking Na+ current or mGluR5 inhibition that blocks intracellular Ca2+ increase. How paeoniflorin inhibits mGluR5 remains unclear.
Pharmacological effects of paeoniflorin in depression
Depression is a common disorder of the nervous system caused by various factors such as environmental stress and genetic mutations. Conventional antidepressants such as the selective serotonin reuptake inhibitors were developed based on the monoamine hypothesis. These drugs have a relatively good therapeutic effect in some patients but also elicit adverse effects in others (Kutzer et al., 2020; Ramic et al., 2020). Therefore, the search for new antidepressants is an urgent task for scientists. In a previous study, 30-day treatment with paeoniflorin (20 mg/kg, i.p.) was shown to reverse CUS-induced depression-like behaviors in mice by increasing BDNF and postsynaptic density protein 95 (PSD-95) expression and dendritic spine density (Liu et al., 2019) (Figure 6). Treatment with paeoniflorin at a dose of 30 or 60 mg/kg (i.p., 5 weeks, once daily) or 10 mg/kg (oral administration, 2 weeks) can also reverse CUS-induced depression-like behaviors in rats, by reducing serum corticosterone and adrenocorticotropic hormone levels and 5-HT2A receptor expression levels in the hippocampus and increasing brain noradrenaline and serotonin levels, as well as hippocampal expression levels of ERK1/2, CREB, and the 5-HT1A receptor (Qiu et al., 2013; Huang et al., 2015; Zhong et al., 2019) (Figure 6). The changes in ERK1/2 activities appear to be a key mechanism for the regulation of depression by paeoniflorin, as ERK1/2 inhibition can abolish the antidepressant effect of paeoniflorin (Zhong et al., 2019). The antidepressant-like effect of paeoniflorin might also be mediated by neurogenesis in the hippocampus, as paeoniflorin treatment (60 mg/kg, i.p., 4 weeks) can promote neural stem cell proliferation and increase the number of BrdU+ cells in the hippocampus of CUS rats by activating the BDNF-tyrosine kinase receptor B (TrkB) pathway (Chen et al., 2019) (Figure 6). This speculation may be supported by another in vitro discovery: paeoniflorin pretreatment (200, 400, or 800 μg/ml) can reverse hydrogen peroxide-induced apoptosis through activation of PI3K-Akt signaling in cultured neuronal progenitor cells (Wu et al., 2013).
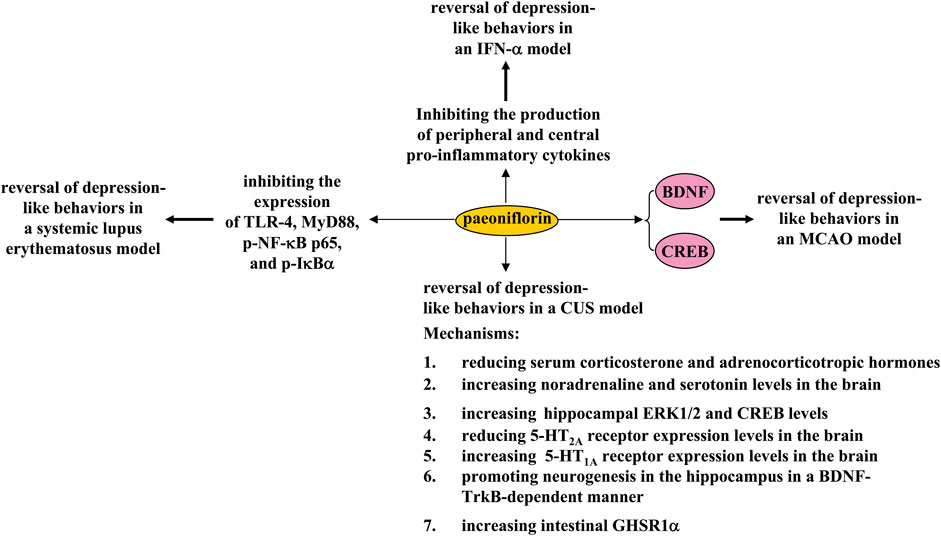
FIGURE 6. Effects and mechanisms of paeoniflorin in depression. Paeoniflorin administration can reverse 1) CUS-induced depression-like behaviors by reducing serum corticosterone and adrenocorticotropic hormone levels, increasing brain noradrenaline and serotonin levels, increasing hippocampal ERK1/2 and CREB levels, reducing 5-HT2A receptor expression levels in the brain, increasing brain 5-HT1A receptor expression levels, promoting hippocampal neurogenesis in a BDNF-TrkB-dependent manner, or increasing intestinal GHSR1a; 2) MCAO-induced depression-like behaviors by enhancing CREB-BDNF signaling; 3) systemic lupus erythematosus-induced depression-like behaviors by inhibiting the expression of TLR-4, MyD88, p-NF-κB p65, and p-IκBα; and 4) IFN-α-induced depression-like behaviors by inhibiting the production of peripheral and central pro-inflammatory cytokines.
The authors also found that paeoniflorin treatment (1 mg/kg, i.p.) produced rapid and sustained antidepressant-like effects in several models of depression in mice, including the forced swimming test and exposure to CUS, which appear to be dependent on increased expression of the gut growth hormone secretagogue receptor 1α (GHSR1α) (Zhang et al., 2021b). A major focus of this finding is the rapid and sustained antidepressant-like effect of paeoniflorin. It is well known that ketamine is an antidepressant with rapid and sustained antidepressant effects (Zanos and Gould, 2018). Although it has been reported that ketamine exerts rapid and sustained antidepressant effects via various mechanisms such as promoting neurotransmission and enhancing BDNF-TrkB signaling (Nguyen et al., 2016; Abdallah et al., 2018), there are still some puzzling questions about the antidepressant effects of ketamine (Price and Mathew, 2015). The intestinal GHSR1α-dependent rapid and sustained antidepressant effects provide further opportunities to investigate the antidepressant mechanisms of ketamine in detail. Given the possible similarities in the rapid and sustained antidepressant effects of paeoniflorin and ketamine, it is also necessary to investigate whether the rapid and sustained antidepressant effects of paeoniflorin are indeed mediated by BDNF-TrkB signaling.
In addition to its role in CUS models, paeoniflorin treatment may also produce antidepressant effects in some other models associated with depression. For example, paeoniflorin administration (5 mg/kg, 3 weeks, i.p.) was reported to reverse MCAO-induced depression-like behaviors in rats by enhancing CREB-BDNF signaling in the hippocampus (Hu et al., 2019) (Figure 6), suggesting that paeoniflorin administration may be a novel strategy for the treatment of post-stroke depression. In a mouse model of systemic lupus erythematosus associated with depressive symptoms, intravenous infusion of paeoniflorin (20 mg/kg, 6 h) can inhibit despair-like behaviors by reducing the expression of toll-like receptor-4 (TLR-4), myeloid differentiation primary response 88 (MyD88), phospho-NF-κB p65, and phospho-IκBα, and reducing the production of pro-inflammatory cytokines (Wang et al., 2018c) (Figure 6), suggesting that paeoniflorin could be developed as a novel drug to improve depressive symptoms in patients with systemic lupus erythematosus. Moreover, 4 weeks of pretreatment with paeoniflorin (20 or 40 mg/kg) was shown to reverse IFN-α-induced depression-like behaviors in mice by down-regulating the production of pro-inflammatory cytokines in the serum, medial prefrontal cortex, hippocampus, and amygdala (Li et al., 2017) (Figure 6).
A common signaling pathway that could be regulated by paeoniflorin is that mediated by BDNF. Regardless of the type of stress to which animals are exposed, decreased BDNF may affect many functions in the brain, such as neurogenesis, neuron survival, and neurogenesis, promoting the occurrence of depression-like behaviors in stressed animals. At this stage, it is unclear how paeoniflorin restores impaired BDNF expression and signaling under stress conditions. One possibility is that paeoniflorin enhances BDNF expression or function by activating intermittent kinases, such as ERK1/2 and CREB. Indeed, this hypothesis has been supported by numerous previously published studies. Future studies should determine how paeoniflorin triggers the activation of various intracellular kinases, such as ERK1/2 and CREB (Zhong et al., 2019). Another possibility is that paeoniflorin promotes the release of already synthesized BDNF in cell different types in the brain into their environment, and the increased extracellular BDNF acts on the TrkB receptor to mediate the antidepressant effects of paeoniflorin. These hypotheses should be investigated in future studies.
Pharmacological effects of paeoniflorin in post traumatic stress disorder
Post traumatic stress disorder (PTSD) is a stressor- and trauma-associated disorder with a group of psychological symptoms that include re-experiencing natural disasters, anxiety, military combat, and avoidance of trauma-associated stimuli (Morland et al., 2020). Selective serotonin reuptake inhibitors are the most commonly used medications for the treatment of PTSD (Ehret, 2019). However, these medications have limited efficacy in PTSD patients. The search for new drugs is of great importance for improving the therapeutic condition of PTSD. It has been shown that pre-treatment with paeoniflorin (10 and 20 mg/kg, i.p.) can prevent the increase in freezing time in contextual fear paradigm induced by single prolonged stress (SPS) (Chen et al., 2020a) (Figure 7). Since SPS is commonly used to induce PTSD-like symptoms in rodents, these results indicate that the paeoniflorin may be a novel drug for the treatment of PTSD. Further analysis showed that pretreatment with paeoniflorin reversed the SPS-induced increases in serum corticosterone, corticotropin-releasing hormone, and adrenocorticotropic hormone, as well as the SPS-induced decrease in 5-HT levels in the prefrontal cortex and hippocampus (Chen et al., 2020a) (Figure 7), suggesting that the anti-PTSD effect of paeoniflorin may be related to its regulatory action on the HPA axis and the 5-HT system. However, more experiments should be performed in future studies before this conclusion can be drawn. In addition, because the high levels of neuroinflammatory response (Zass et al., 2017) and impairment of CREB-BDNF signaling (Ji et al., 2017) and hippocampal neurogenesis (Besnard and Sahay, 2016) have been shown to be closely related to the pathogenesis of PTSD, researchers should clarify whether the anti-PTSD effect of paeoniflorin could be mediated by its inhibitory effect on neuroinflammation and its ameliorative effect on CREB-BDNF signaling and hippocampal neurogenesis.
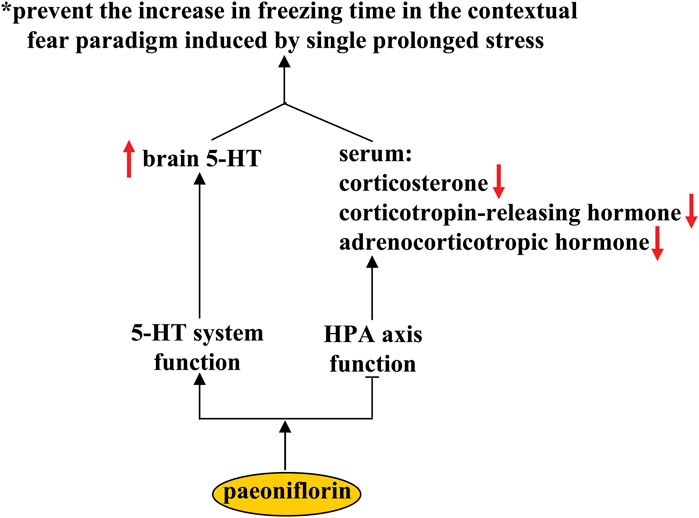
FIGURE 7. Effects and mechanisms of paeoniflorin in post-traumatic syndrome disorder. Paeoniflorin administration can prevent the increase in freezing time in the contextual fear paradigm induced by single prolonged stress by increasing brain concentrations of 5-HT and decreasing serum concentrations of corticosterone, corticotropin-releasing hormone, and adrenocorticotropic hormone. However, it is still unclear how paeoniflorin improve the function of the 5-HT system and how paeoniflorin suppresses the functions of the HPA axis.
Pharmacological effects of paeoniflorin in pain
Pain is a common pathological process in various disorders such as cancer (Wu et al., 2021). The search for new mechanisms-based drugs for the treatment of pain is an old but still new focus. It has been previously reported that paeoniflorin exerts analgesic effects through a variety of mechanisms. The first mechanism is activation of the adenosine A1 receptor. Pretreatment with paeoniflorin (50 or 100 mg/kg, i.p.; 0.1 and 1% ethanol solution applied topically on plantar surfaces twice daily, beginning 24 h after model construction; 180 mg/kg, i.p.) has been shown to prevent visceral pain induced by colorectal distention in rats undergoing neonatal maternal separation (Zhang et al., 2009) and to increase mechanical threshold in mice induced by partial sciatic nerve ligation (Yin et al., 2016) or paclitaxel, an anti-microtubule agent used to treat solid neoplasms (Wu et al., 2021). Antagonization of the adenosine A1 receptor or knockout of the adenosine A1 receptor gene may abolish the analgesic effect of paeoniflorin (Zhang et al., 2009; Yin et al., 2016; Andoh et al., 2017) (Figure 8).
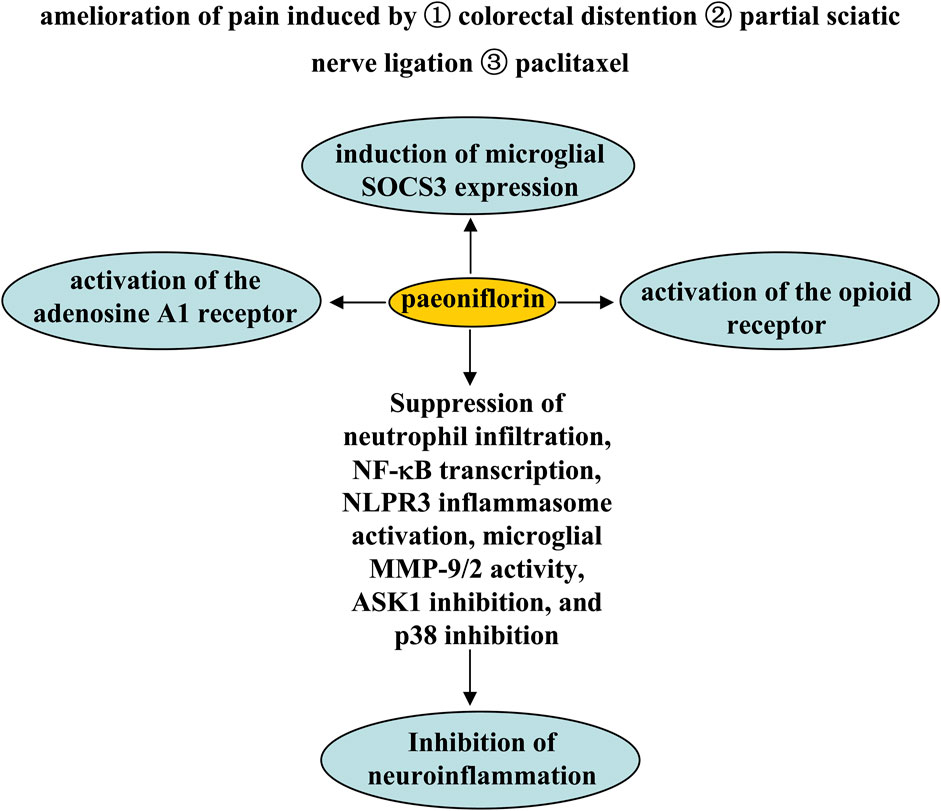
FIGURE 8. Effects and mechanisms of paeoniflorin on pain. Administration of paeoniflorin may relieve pain induced by colorectal distention partial sciatic nerve ligation, or paclitaxel. Mechanisms such as adenosine A1 and opioid receptor activation, induction of microglial SOCS3 expression, and inhibition of neutrophil infiltration, NF-κB transcription, NLPR3 inflammasome activation, microglial MMP-9/2 activity, ASK1 inhibition, and p38 inhibition may mediate the analgesic effect of paeoniflorin.
The second mechanism may be dependent on suppressor of cytokine signaling 3 (SOCS3). Studies by Fan et al. (2018a) showed that consecutive paeoniflorin treatment (30, 60, 90 mg/kg, i.p., 5 days) can attenuate plantar incision-induced mechanical allodynia in mice in a SOCS3-dependent manner in the spinal cord (Figure 8). In vitro studies have shown that incubation with paeoniflorin (0.1–10 µM) can induce SOCS3 expression in BV-2 microglia (Fan et al., 2018a), suggesting that the analgesic effect of paeoniflorin may be related to microglial SOCS3. However, since this result was obtained in microglial cell lines and there is no evidence to support the influence of microglial SOCS3 on the analgesic effect of paeoniflorin, further studies should be conducted to investigate the relationships between microglial SOCS3 and the analgesic effect of paeoniflorin. For example, researchers can use the technique of conditional SOCS3 gene knockout in microglia to confirm the influence of microglial SOCS3 on the analgesic effect of paeoniflorin.
Third, the endogenous opioid receptor may be involved in the analgesic effect of paeoniflorin, because inhibition of the opioid receptor has been shown to prevent the amelioration effect of systemic paeoniflorin administration before (5, 50, 100 mg/kg, i.p., 10 min before) or after (50 mg/kg, i.p., 5 min or 3 h after) bee venom-induced persistent spontaneous noiception, heat hypersensitivity, and paw edema and swelling in conscious rats (Yu et al., 2007) (Figure 8). It has been reported that activation of the adenosine A2a receptor can upregulate the expression of SOCS3 in lung tissue in a rat model of pulmonary hypertension (Fan et al., 2016). Future studies should investigate whether the above three mechanisms are linked in the analgesic effect of paeoniflorin.
Fourth, the anti-neuroinflammatory effect could mediate the analgesic effect of paeoniflorin. Researchers have found that treatment with paeoniflorin (50 and 100 mg/kg, i.p., 7 or 11 days) can attenuate the pain caused by chronic constriction injury by inhibiting the activation of NOD-like receptor protein 3 (NLRP3) infammasome in the spinal cord, partly by inhibiting NF-κB in the spinal cord (Liu et al., 2020). Pretreatment with paeoniflorin (5 or 25 mg/kg, i.p., 7 days; 1 or 2 μg administered intrathecally, daily, 8 days) can also prevent the inflammatory pain and paw edema induced by LPS or Freund’s complete adjuvant in mice by inhibiting neutrophil infiltration, NF-κB transcription, activation of nucleotide-binding oligomerization domain receptor protein 3 (NLPR3) inflammasome, and production of pro-inflammatory cytokines in footpad tissues (Hu et al., 2018; Yin et al., 2020) (Figure 8). In addition, administration of paeoniflorin (30, 60, and 90 mg/kg, i.p., 7 days; 40 mg/kg, i.p., 21 days; 50 mg/kg, i.p., once daily, 15 days) ameliorated plantar incision-induced mechanical allodynia in mice by inhibiting matrix metalloproteinases-9/2 (MMP-9/2) activity in microglia (Fan et al., 2018b), or mechanical and thermal thresholds in rats, stimulated by chronic constrictive injury through apoptosis signal-regulating kinase 1 (ASK1) inhibition (Zhou et al., 2019) or p38 inhibition (Zhou et al., 2016)-dependent suppression of pro-inflammatory cytokine production in the spinal cord (Figure 8). In future studies, researchers should investigate the correlations between the anti-neuroinflammatory effects of paeoniflorin and the adenosine A2a receptor or the opioid receptor in the spinal cord. In addition, researchers should investigate whether paeoniflorin affects the adenosine A2a receptor or the opioid receptor in a manner mediated by direct binding of paeoniflorin to these receptors? Regarding the regulation of the adenosine A2a receptor by paeoniflorin, researchers might consult the study on the activation of the adenosine A1 receptor by paeoniflorin (Liu et al., 2005b).
Pharmacological effect of paeoniflorin on glioblastoma
Glioblastoma (GMB) is a common brain tumor in the nervous system. In the clinic, methods available for the treatment of GMB include chemotherapy, radiotherapy, and surgery (Janjua et al., 2021). However, although these methods are useful for ameliorating GMB symptoms, the median survival time of patients receiving these therapies is usually less than 18 months. Therefore, there is an urgent need to search for new drugs for GMB treatment. C-Met-mediated ras homolog family member A (RhoA)/Rho-associated protein kinase (ROCK) signaling (Xiong et al., 2016) and epithelial-to-mesenchymal transition (EMT), characterized by loss of cell polarity, play important roles in tumor invasion and metastasis (Coelho et al., 2020). Their inhibition is a promising strategy for GMB treatment. Previous studies have shown that treatment with paeoniflorin (10, 15, 20, or 40 μM) suppresses hepatocyte growth factor-induced migration and invasion and actin cytoskeleton rearrangement in glioblastoma cells, by inhibiting c-Met-mediated RhoA/ROCK signaling and EMT in U87, U251, and T98G cells or in the U87 xenograft mouse model, thereby reducing tumor size (Figure 9) (Wang et al., 2018d; Yu et al., 2019a). Another study by Nie et al. (2015) had shown that paeoniflorin treatment at a dose of 10, 15, or 20 mM can increase cellular apoptosis and inhibit cellular proliferation in U87 and U251 cells by promoting the degradation and de-phosphorylation of Signal Transducer and Activator of Transcription 3 (STAT3) (Figure 9) (Nie et al., 2015). Since over-expression of STAT3 in glioma cells can induce resistance to the effects of paeoniflorin on cellular apoptosis and proliferation (Nie et al., 2015), these results indicate that STAT3 may be a key protein in the regulation of glioma cells by paeoniflorin. In future studies, researchers should investigate how exactly paeoniflorin promotes the degradation of STAT3 in glioma cells.
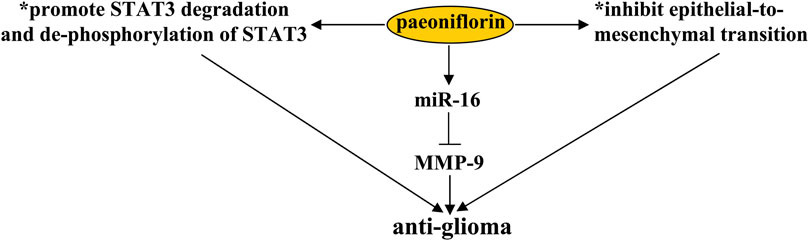
FIGURE 9. Effects and mechanisms of paeoniflorin in glioma. Paeoniflorin administration can suppress glioma growth by promoting STAT3 degradation and de-phosphorylation of STAT3 and inhibiting epithelial-to-mesenchymal transition. Increasing the expression of miR-16 is another mechanism for the anti-glioma effect of paeoniflorin. The increased miR-16 upon paeoniflorin treatment induces glioma cell apoptosis by down-regulating MMP-9 expression.
Studies by Li et al. (2015) had reported that the anti-glioma effect of paeoniflorin might also be related to miR-16, a unique miRNA whose level was reduced in glioma cell lines (Yang et al., 2014), because treatment with paeoniflorin (10 and 20 μM) can increase the levels of miR-16 in U87 cells and the pro-apoptotic effect of paeoniflorin can be abrogated by the anti-miR-16 antibody in cultured U87 cells (Figure 9) (Li et al., 2015). Further analysis suggested that the pro-apoptotic effect of paeoniflorin may be due to miR-16-mediated down-regulation of the expression of matrix metalloproteinase-9 (MMP-9), an indicator of malignant human brain glioma (Figure 9) (Nakano et al., 1995), as exogenous miR-16 up-regulation can reduce the expression levels of MMP-9 protein in U87 cells (Li et al., 2015). Considering the importance of miR-16 in glioma development (Li et al., 2013; Yang et al., 2014), future studies should investigate the exact mechanisms mediating the down-regulatory effect of miR-16 on MMP-9 expression.
In glioma studies, various glioblastoma cells with different gene mutation backgrounds have been established to test the anti-glioma effects of different compounds. In clinical practice, the glioblastomas of different patients also have different gene mutation background, so the currently used anticancer drugs are not as effective against glioma cells (Venkatesan et al., 2016). The search for new agents that play a comprehensive role in suppressing glioblastoma is of great importance in improving the current therapeutic situation in glioma. It is known that the lack of comprehensive therapeutic effects of anti-glioma drugs is mediated in part by O6-methylguanine-DNA methyltransferase (MGMT)-mediated drug resistance (Lang et al., 2021). The absence of MGMT protein may predict sensitivity to temozolomide (TMZ) (van Nifterik et al., 2010). Interestingly, one of the aforementioned glioblastoma cell lines T98G was reported to have high MGMT levels (van Nifterik et al., 2010; Szeliga et al., 2012), which may indicate high resistance to TMZ. Since the addition of paeoniflorin can strongly suppress the growth of T98G cells (Wang et al., 2018d), compare with TMZ, paeoniflorin may have advantages in the treatment of glioma. However, more studies should be conducted in future investigations before definitive conclusions can be drawn. Researcher should also determine whether paeoniflorin affect the sensitivity of glioma cells to TMZ by altering the expression of MGMT.
Conclusion
In this review, the authors outline the pharmacological effects of paeoniflorin on the nervous system. Paeoniflorin may exert preventive and/or therapeutic effects on nervous system disorders through two common mechanisms: anti-oxidative stress and anti-neuroinflammation (Liu et al., 2006; Guo et al., 2012; Cong et al., 2019; Liu et al., 2020). The changes in signaling molecules such as CREB (Zhang et al., 2017; Hu et al., 2019), BDNF (Chen et al., 2019), ERK1/2 (Zhong et al., 2019), CaMKII (Zhang et al., 2017), Akt (Ma et al., 2018), GSK-3β (Ma et al., 2018), IκB-α and NF-κB (Neacsu et al., 2015; Biriken and Yazihan, 2021), JNK (Chu et al., 2017), p38 (Gu et al., 2016), and STAT3 (Nie et al., 2015) may mediate the pharmacological effects of paeoniflorin in the nervous system. In glioblastoma cells, paeoniflorin treatment can suppress tumor cell migration and invasion and actin cytoskeleton rearrangement by inhibiting c-Met-mediated RhoA/ROCK signaling and EMT (Wang et al., 2018d; Yu et al., 2019a), deactivating STAT3 signaling (Nie et al., 2015), or increasing miR-16 expression (Li et al., 2015). Activation of the adenosine A1 receptor (Liu et al., 2006; Kong et al., 2020) and opioid receptor (Yu et al., 2007), enhancement of SOCS3 signaling (Fan et al., 2018a), and inhibition of microglial activation (Liu et al., 2020) play critical roles in the analgesic effects of paeoniflorin. In general, paeoniflorin intake may be beneficial in the prevention and/or treatment of nervous system disorders, including cerebral ischemia (Guo et al., 2012; Zhang et al., 2015a; Hu et al., 2019; Wu et al., 2020a), SAH (Wang et al., 2020), vascular dementia (Zhang et al., 2016; Luo et al., 2018), Alzheimer’s disease (Gu et al., 2016; Kong et al., 2020), Parkinson’s disease (Liu et al., 2006; Liu et al., 2007; Zheng et al., 2017), depression (Qiu et al., 2013; Huang et al., 2015; Zhang et al., 2021b), PTSD (Chen et al., 2020a), pain (Yu et al., 2007; Zhang et al., 2009; Yin et al., 2016; Andoh et al., 2017; Fan et al., 2018a), epilepsy (Hino et al., 2012), and glioblastoma (Nie et al., 2015; Wang et al., 2018d; Yu et al., 2019a). Further exploration of its therapeutic basis in nervous system disorders may promote the future application and development of paeoniflorin.
Of particular note, although paeoniflorin can be administered by various routes, oral administration may result in low concentrations of paeoniflorin in the brain, which may be due to its poor oral bioavailability (Yu et al., 2019b) and may limit its use in the treatment of disease. However, it should also be noted that once paeoniflorin enters into the body, it can be converted into another molecule that crosses the blood-brain barrier, benzoic acid (Yu et al., 2019b). Benzoic acid supplementation can improve cognitive function in patients with schizophrenia (Lane et al., 2013), dementia (Lin et al., 2019; Lin et al., 2021), and early-phase Alzheimer’s disease (Lin et al., 2014), and benzoic acid derivatives have been reported to exert numerous pharmacological effects in the nervous system, such as suppression of glaucoma-associated neurodegeneration (Luo et al., 2020), inhibition of microglial activation and pathological processes of amyotrophic lateral sclerosis (Ibarburu et al., 2021), and inhibition of proliferation of human glioma cells (Yue et al., 2016), researchers should clarify whether the pharmacological effects of paeoniflorin in the central nervous system are mediated by benzoic acid.
Author contributions
HH wrote the main text. JC and JZ retrieved and organized documents. XL revised the primary manuscript. CC and CW made the pictures. ZC and CH conceived the idea and provided important information for the completion.
Funding
This work was supported by the Science and Technology Project of Nantong City (JC2020013 and JC2020020), Postgraduate Research and Practice Innovation Program of Jiangsu Province (KYCX21_3107), and the Natural Science Foundation of the Higher Education Institutions of Jiangsu Province (20KJB310025).
Conflict of interest
The authors declare that the research was conducted in the absence of any commercial or financial relationships that could be construed as a potential conflict of interest.
Publisher’s note
All claims expressed in this article are solely those of the authors and do not necessarily represent those of their affiliated organizations, or those of the publisher, the editors and the reviewers. Any product that may be evaluated in this article, or claim that may be made by its manufacturer, is not guaranteed or endorsed by the publisher.
References
Abdallah, C. G., Sanacora, G., Duman, R. S., and Krystal, J. H. (2018). The neurobiology of depression, ketamine and rapid-acting antidepressants: Is it glutamate inhibition or activation? Pharmacol. Ther. 190, 148–158. doi:10.1016/j.pharmthera.2018.05.010
Andoh, T., Kobayashi, N., Uta, D., and Kuraishi, Y. (2017). Prophylactic topical paeoniflorin prevents mechanical allodynia caused by paclitaxel in mice through adenosine A1 receptors. Phytomedicine. 25, 1–7. doi:10.1016/j.phymed.2016.12.010
Arias, R. L., Sung, M. L., Vasylyev, D., Zhang, M. Y., Albinson, K., Kubek, K., et al. (2008). Amiloride is neuroprotective in an MPTP model of Parkinson's disease. Neurobiol. Dis. 31, 334–341. doi:10.1016/j.nbd.2008.05.008
Audenaert, D., Schwartz, E., Claeys, K. G., Claes, L., Deprez, L., Suls, A., et al. (2006). A novel GABRG2 mutation associated with febrile seizures. Neurology 67, 687–690. doi:10.1212/01.wnl.0000230145.73496.a2
Besnard, A., and Sahay, A. (2016). Adult hippocampal neurogenesis, fear generalization, and stress. Neuropsychopharmacology 41, 24–44. doi:10.1038/npp.2015.167
Biagini, G., Babinski, K., Avoli, M., Marcinkiewicz, M., and Séguéla, P. (2001). Regional and subunit-specific downregulation of acid-sensing ion channels in the pilocarpine model of epilepsy. Neurobiol. Dis. 8, 45–58. doi:10.1006/nbdi.2000.0331
Biriken, D., and Yazihan, N. (2021). Modulation of proliferation, apoptosis and inflammation of Caco-2 epithelial cells and THP-1 macrophage-like monocytes in LPS stimulated co-culture model. Bratisl. Lek. Listy 122, 138–144. doi:10.4149/BLL_2021_021
Chamorro, Á., Dirnagl, U., Urra, X., and Planas, A. M. (2016). Neuroprotection in acute stroke: Targeting excitotoxicity, oxidative and nitrosative stress, and inflammation. Lancet. Neurol. 15, 869–881. doi:10.1016/S1474-4422(16)00114-9
Chen, H., He, Y., Chen, S., Qi, S., and Shen, J. (2020). Therapeutic targets of oxidative/nitrosative stress and neuroinflammation in ischemic stroke: Applications for natural product efficacy with omics and systemic biology. Pharmacol. Res. 158, 104877. doi:10.1016/j.phrs.2020.104877
Chen, J., Ye, W., Li, L., Su, J., Huang, Y., Liu, L., et al. (2020). Paeoniflorin regulates the hypothalamic-pituitary-adrenal axis negative feedback in a rat model of post-traumatic stress disorder. Iran. J. Basic Med. Sci. 23, 439–448. doi:10.22038/ijbms.2020.41214.9738
Chen, L. B., Qiu, F. M., Zhong, X. M., Hong, C., and Huang, Z. (2019). Promoting neurogenesis in hippocampal dentate gyrus of chronic unpredictable stress-induced depressive-like rats with paeoniflorin. J. Integr. Neurosci. 18, 43–49. doi:10.31083/j.jin.2019.01.116
Chu, H., Huang, C., Gao, Z., Dong, J., Tang, Y., and Dong, Q. (2017). Reduction of ischemic brain edema by combined use of paeoniflorin and astragaloside IV via down-regulating connexin 43. Phytother. Res. 31, 1410–1418. doi:10.1002/ptr.5868
Coelho, B. P., Fernandes, C. F. L., Boccacino, J. M., Souza, M. C. D. S., Melo-Escobar, M. I., Alves, R. N., et al. (2020). Multifaceted WNT signaling at the crossroads between epithelial-mesenchymal transition and autophagy in glioblastoma. Front. Oncol. 10, 597743. doi:10.3389/fonc.2020.597743
Cong, C., Kluwe, L., Li, S., Liu, X., Liu, Y., Liu, H., et al. (2019). Paeoniflorin inhibits tributyltin chloride-induced apoptosis in hypothalamic neurons via inhibition of MKK4-JNK signaling pathway. J. Ethnopharmacol. 237, 1–8. doi:10.1016/j.jep.2019.03.030
Das, A., McDowell, M., O'Dell, C. M., Busch, M. E., Smith, J. A., Ray, S. K., et al. (2010). Post-treatment with voltage-gated Na(+) channel blocker attenuates kainic acid-induced apoptosis in rat primary hippocampal neurons. Neurochem. Res. 35, 2175–2183. doi:10.1007/s11064-010-0321-1
de Oliveira, A. C. P., Moreira, F. A., and Fiebich, B. L. (2022). CB2 and toll-like receptors crosstalk in microglia. Trends Neurosci. 45, 1–2. doi:10.1016/j.tins.2021.10.012
Devinsky, O., Vezzani, A., Najjar, S., De Lanerolle, N. C., and Rogawski, M. A. (2013). Glia and epilepsy: Excitability and inflammation. Trends Neurosci. 36, 174–184. doi:10.1016/j.tins.2012.11.008
Ehret, M. (2019). Treatment of posttraumatic stress disorder: Focus on pharmacotherapy. Ment. Health Clin. 9, 373–382. doi:10.9740/mhc.2019.11.373
Fan, R., Huang, X. Y., Du, K. Y., Fan, Y. F., and Wang, L. X. (2016). The regulative effcets of A2a adenosine receptor on expression of SOCS-3 in rats of hypoxic pulmonary hypertension. Zhonghua Jie He He Hu Xi Za Zhi 39, 469–473. doi:10.3760/cma.j.issn.1001-0939.2016.06.014
Fan, Y. X., Hu, L., Zhu, S. H., Han, Y., Liu, W. T., Yang, Y. J., et al. (2018). Paeoniflorin attenuates postoperative pain by suppressing Matrix Metalloproteinase-9/2 in mice. Eur. J. Pain 22, 272–281. doi:10.1002/ejp.1116
Fan, Y. X., Qian, C., Liu, B., Wang, C., Liu, H., Pan, X., et al. (2018). Induction of suppressor of cytokine signaling 3 via HSF-1-HSP70-TLR4 axis attenuates neuroinflammation and ameliorates postoperative pain. Brain Behav. Immun. 68, 111–122. doi:10.1016/j.bbi.2017.10.006
Fern, R., and Matute, C. (2019). Glutamate receptors and white matter stroke. Neurosci. Lett. 694, 86–92. doi:10.1016/j.neulet.2018.11.031
Fernández-Trapero, M., Espejo-Porras, F., Rodríguez-Cueto, C., Coates, J. R., Pérez-Díaz, C., de Lago, E., et al. (2017). Upregulation of CB2 receptors in reactive astrocytes in canine degenerative myelopathy, a disease model of amyotrophic lateral sclerosis. Dis. Model. Mech. 10, 551–558. doi:10.1242/dmm.028373
Fukuda, M., Morimoto, T., Nagao, H., and Kida, K. (1997). The effect of GABAergic system activity on hyperthermia-induced seizures in rats. Brain Res. Dev. Brain Res. 104, 197–199. doi:10.1016/s0165-3806(97)00157-0
Gonzales, E. B., and Sumien, N. (2017). Acidity and acid-sensing ion channels in the normal and alzheimer's disease brain. J. Alzheimers Dis. 57, 1137–1144. doi:10.3233/JAD-161131
Gu, X., Cai, Z., Cai, M., Liu, K., Liu, D., Zhang, Q., et al. (2016). Protective effect of paeoniflorin on inflammation and apoptosis in the cerebral cortex of a transgenic mouse model of Alzheimer’s disease. Mol. Med. Rep. 13, 2247–2252. doi:10.3892/mmr.2016.4805
Guo, R. B., Wang, G. F., Zhao, A. P., Gu, J., Sun, X. L., and Hu, G. (2012). Paeoniflorin protects against ischemia-induced brain damages in rats via inhibiting MAPKs/NF-κB-mediated inflammatory responses. PLoS One 7, e49701. doi:10.1371/journal.pone.0049701
Hauser, W. A. (1994). The prevalence and incidence of convulsive disorders in children. Epilepsia 35, S1–S6. doi:10.1111/j.1528-1157.1994.tb05932.x
He, X., Xing, D., Ding, Y., Li, Y., Xiang, L., Wang, W., et al. (2004). Determination of paeoniflorin in rat hippocampus by high-performance liquid chromatography after intravenous administration of Paeoniae Radix extract. J. Chromatogr. B Anal. Technol. Biomed. Life Sci. 802, 277–281. doi:10.1016/j.jchromb.2003.11.040
Hino, H., Takahashi, H., Suzuki, Y., Tanaka, J., Ishii, E., and Fukuda, M. (2012). Anticonvulsive effect of paeoniflorin on experimental febrile seizures in immature rats: Possible application for febrile seizures in children. PLoS One 7, e42920. doi:10.1371/journal.pone.0042920
Holton, C. M., Strother, L. C., Dripps, I., Pradhan, A. A., Goadsby, P. J., and Holland, P. R. (2020). Acid-sensing ion channel 3 blockade inhibits durovascular and nitric oxide-mediated trigeminal pain. Br. J. Pharmacol. 177, 2478–2486. doi:10.1111/bph.14990
Hu, B., Xu, G., Zhang, X., Xu, L., Zhou, H., Ma, Z., et al. (2018). Paeoniflorin attenuates inflammatory pain by inhibiting microglial activation and akt-NF-κb signaling in the central nervous system. Cell. Physiol. biochem. 47, 842–850. doi:10.1159/000490076
Hu, M. Z., Wang, A. R., Zhao, Z. Y., Chen, X. Y., Li, Y. B., and Liu, B. (2019). Antidepressant-like effects of paeoniflorin on post-stroke depression in a rat model. Neurol. Res. 41, 446–455. doi:10.1080/01616412.2019.1576361
Hu, P. Y., Liu, D., Zheng, Q., Wu, Q., Tang, Y., and Yang, M. (2016). Elucidation of transport mechanism of paeoniflorin and the influence of ligustilide, senkyunolide I and senkyunolide A on paeoniflorin transport through mdck-mdr1 cells as blood-brain barrier in vitro model. Molecules 21, 300. doi:10.3390/molecules21030300
Huang, H., Zhao, J., Jiang, L., Xie, Y., Xia, Y., LvDong, R. L., et al. (2015). Paeoniflorin improves menopause depression in ovariectomized rats under chronic unpredictable mild stress. Int. J. Clin. Exp. Med. 8, 5103–5111.
Ibarburu, S., Kovacs, M., Varela, V., Rodríguez-Duarte, J., Ingold, M., Invernizzi, P., et al. (2021). A nitroalkene benzoic acid derivative targets reactive microglia and prolongs survival in an inherited model of ALS via NF-κB inhibition. Neurotherapeutics 18, 309–325. doi:10.1007/s13311-020-00953-z
Janjua, T. I., Rewatkar, P., Ahmed-Cox, A., Saeed, I., Mansfeld, F. M., Kulshreshtha, R., et al. (2021). Frontiers in the treatment of glioblastoma: Past, present and emerging. Adv. Drug Deliv. Rev. 21 (21), 10800022–10801383. doi:10.1016/j.addr.2021.01.012
Jayaraj, R. L., Azimullah, S., Beiram, R., Jalal, F. Y., and Rosenberg, G. A. (2019). Neuroinflammation: Friend and foe for ischemic stroke. J. Neuroinflammation 16, 142. doi:10.1186/s12974-019-1516-2
Ji, L. L., Peng, J. B., Fu, C. H., Tong, L., and Wang, Z. Y. (2017). Sigma-1 receptor activation ameliorates anxiety-like behavior through NR2A-CREB-BDNF signaling pathway in a rat model submitted to single-prolonged stress. Mol. Med. Rep. 16, 4987–4993. doi:10.3892/mmr.2017.7185
Joch, M., Ase, A. R., Chen, C. X., MacDonald, P. A., Kontogiannea, M., Corera, A. T., et al. (2007). Parkin-mediated monoubiquitination of the PDZ protein PICK1 regulates the activity of acid-sensing ion channels. Mol. Biol. Cell 18, 3105–3118. doi:10.1091/mbc.e05-11-1027
Kasala, E. R., Bodduluru, L. N., Maneti, Y., and Thipparaboina, R. (2014). Effect of meditation on neurophysiological changes in stress mediated depression. Complement. Ther. Clin. Pract. 20, 74–80. doi:10.1016/j.ctcp.2013.10.001
Kido, Y., Tamai, I., Okamoto, M., Suzuki, F., and Tsuji, A. (2000). Functional clarification of MCT1-mediated transport of monocarboxylic acids at the blood-brain barrier using in vitro cultured cells and in vivo BUI studies. Pharm. Res. 17, 55–62. doi:10.1023/a:1007518525161
Kong, Y., Peng, Q., Lv, N., Yuan, J., Deng, Z., Liang, X., et al. (2020). Paeoniflorin exerts neuroprotective effects in a transgenic mouse model of Alzheimer's disease via activation of adenosine A1 receptor. Neurosci. Lett. 730, 135016. doi:10.1016/j.neulet.2020.135016
Kutzer, T., Dick, M., Scudamore, T., Wiener, M., and Schwartz, T. (2020). Antidepressant efficacy and side effect burden: An updated guide for clinicians. Drugs Context 9, 1–112. doi:10.7573/dic.2020-2-2
Lan, Z., Chen, L., Fu, Q., Ji, W., Wang, S., Liang, Z., et al. (2013). Paeoniflorin attenuates amyloid-beta peptide-induced neurotoxicity by ameliorating oxidative stress and regulating the NGF-mediated signaling in rats. Brain Res. 1498, 9–19. doi:10.1016/j.brainres.2012.12.040
Lane, H. Y., Lin, C. H., Green, M. F., Hellemann, G., Huang, C. C., Chen, P. W., et al. (2013). Add-on treatment of benzoate for schizophrenia: A randomized, double-blind, placebo-controlled trial of D-amino acid oxidase inhibitor. JAMA Psychiatry 70, 1267–1275. doi:10.1001/jamapsychiatry.2013.2159
Lang, F., Liu, Y., Chou, F. J., and Yang, C. (2021). Genotoxic therapy and resistance mechanism in gliomas. Pharmacol. Ther. 228, 107922. doi:10.1016/j.pharmthera.2021.107922
Li, J., Huang, S., Huang, W., Wang, W., Wen, G., Gao, L., et al. (2017). Paeoniflorin ameliorates interferon-alpha-induced neuroinflammation and depressive-like behaviors in mice. Oncotarget 8, 8264–8282. doi:10.18632/oncotarget.14160
Li, J., Ji, X., Zhang, J., Shi, G., Zhu, X., and Wang, K. (2014). Original article Paeoniflorin attenuates Aβ 25-35 -induced neurotoxicity in PC12 cells by preventing mitochondrial dysfunction. Folia Neuropathol. 52, 285–290. doi:10.5114/fn.2014.45569
Li, W., Qi, Z., Wei, Z., Liu, S., Wang, P., Chen, Y., et al. (2015). Paeoniflorin inhibits proliferation and induces apoptosis of human glioma cells via microRNA-16 upregulation and matrix metalloproteinase-9 downregulation. Mol. Med. Rep. 12, 2735–2740. doi:10.3892/mmr.2015.3718
Li, X., Ling, N., Bai, Y., Dong, W., Hui, G. Z., Liu, D., et al. (2013). MiR-16-1 plays a role in reducing migration and invasion of glioma cells. Anat. Rec. Hob. 296, 427–432. doi:10.1002/ar.22626
Li, Y. C., Zheng, X. X., Xia, S. Z., Li, Y., Deng, H. H., Wang, X., et al. (2020). Paeoniflorin ameliorates depressive-like behavior in prenatally stressed offspring by restoring the HPA axis- and glucocorticoid receptor-associated dysfunction. J. Affect. Disord. 274, 471–481. doi:10.1016/j.jad.2020.05.078
Lin, C. H., Chen, P. K., Chang, Y. C., Chuo, L. J., Chen, Y. S., Tsai, G. E., et al. (2014). Benzoate, a D-amino acid oxidase inhibitor, for the treatment of early-phase Alzheimer disease: A randomized, double-blind, placebo-controlled trial. Biol. Psychiatry 75, 678–685. doi:10.1016/j.biopsych.2013.08.010
Lin, C. H., Chen, P. K., Wang, S. H., and Lane, H. Y. (2021). Effect of sodium benzoate on cognitive function among patients with behavioral and psychological symptoms of dementia: Secondary analysis of a randomized clinical trial. JAMA Netw. Open 4, e216156. doi:10.1001/jamanetworkopen.2021.6156
Lin, C. H., Chen, P. K., Wang, S. H., and Lane, H. Y. (2019). Sodium benzoate for the treatment of behavioral and psychological symptoms of dementia (bpsd): A randomized, double-blind, placebo-controlled, 6-week trial. J. Psychopharmacol. 33, 1030–1033. doi:10.1177/0269881119849815
Liu, D. Z., Xie, K. Q., Ji, X. Q., Ye, Y., Jiang, C. L., and Zhu, X. Z. (2005). Neuroprotective effect of paeoniflorin on cerebral ischemic rat by activating adenosine A1 receptor in a manner different from its classical agonists. Br. J. Pharmacol. 146, 604–611. doi:10.1038/sj.bjp.0706335
Liu, D. Z., Zhu, J., Jin, D. Z., Zhang, L. M., Ji, X. Q., Ye, Y., et al. (2007). Behavioral recovery following sub-chronic paeoniflorin administration in the striatal 6-OHDA lesion rodent model of Parkinson's disease. J. Ethnopharmacol. 112, 327–332. doi:10.1016/j.jep.2007.03.022
Liu, H., Wang, J., Wang, J., Wang, P., and Xue, Y. (2015). Paeoniflorin attenuates Aβ1-42-induced inflammation and chemotaxis of microglia in vitro and inhibits NF-κB- and VEGF/Flt-1 signaling pathways. Brain Res. 1618, 149–158. doi:10.1016/j.brainres.2015.05.035
Liu, H. Q., Zhang, W. Y., Luo, X. T., Ye, Y., and Zhu, X. Z. (2006). Paeoniflorin attenuates neuroinflammation and dopaminergic neurodegeneration in the MPTP model of Parkinson's disease by activation of adenosine A1 receptor. Br. J. Pharmacol. 148, 314–325. doi:10.1038/sj.bjp.0706732
Liu, P., Cheng, J., Ma, S., and Zhou, J. (2020). Paeoniflorin attenuates chronic constriction injury-induced neuropathic pain by suppressing spinal NLRP3 inflammasome activation. Inflammopharmacology 28, 1495–1508. doi:10.1007/s10787-020-00737-z
Liu, S. C., Hu, W. Y., Zhang, W. Y., Yang, L., Li, Y., Xiao, Z. C., et al. (2019). Paeoniflorin attenuates impairment of spatial learning and hippocampal long-term potentiation in mice subjected to chronic unpredictable mild stress. Psychopharmacol. Berl. 236, 2823–2834. doi:10.1007/s00213-019-05257-5
Liu, Z. Q., Zhou, H., Liu, L., Jiang, Z. H., Wong, Y. F., Xie, Y., et al. (2005). Influence of co-administrated sinomenine on pharmacokinetic fate of paeoniflorin in unrestrained conscious rats. J. Ethnopharmacol. 99, 61–67. doi:10.1016/j.jep.2005.01.052
Luo, L. J., Nguyen, D. D., and Lai, J. Y. (2020). Benzoic acid derivative-modified chitosan-g-poly(N-isopropylacrylamide): Methoxylation effects and pharmacological treatments of Glaucoma-related neurodegeneration. J. Control. Release 317, 246–258. doi:10.1016/j.jconrel.2019.11.038
Luo, X. Q., Li, A., Yang, X., Xiao, X., Hu, R., Wang, T. W., et al. (2018). Paeoniflorin exerts neuroprotective effects by modulating the M1/M2 subset polarization of microglia/macrophages in the hippocampal CA1 region of vascular dementia rats via cannabinoid receptor 2. Chin. Med. 13, 14. doi:10.1186/s13020-018-0173-1
Ma, X. H., Duan, W. J., Mo, Y. S., Chen, J. L., Li, S., Zhao, W., et al. (2018). Neuroprotective effect of paeoniflorin on okadaic acid-induced tau hyperphosphorylation via calpain/Akt/GSK-3β pathway in SH-SY5Y cells. Brain Res. 1690, 1–11. doi:10.1016/j.brainres.2018.03.022
Malone, K., Amu, S., Moore, A. C., and Waeber, C. (2019). The immune system and stroke: From current targets to future therapy. Immunol. Cell Biol. 97, 5–16. doi:10.1111/imcb.12191
Mao, Q. Q., Zhong, X. M., Feng, C. R., Pan, A. J., Li, Z. Y., and Huang, Z. (2010). Protective effects of paeoniflorin against glutamate-induced neurotoxicity in PC12 cells via antioxidant mechanisms and Ca(2+) antagonism. Cell. Mol. Neurobiol. 30, 1059–1066. doi:10.1007/s10571-010-9537-5
Missiaen, L., Robberecht, W., van den Bosch, L., Callewaert, G., Parys, J. B., Wuytack, F., et al. (2000). Abnormal intracellular ca(2+)homeostasis and disease. Cell Calcium 28, 1–21. doi:10.1054/ceca.2000.0131
Morland, L. A., Wells, S. Y., Glassman, L. H., Greene, C. J., Hoffman, J. E., and Rosen, C. S. (2020). Advances in PTSD treatment delivery: Review of findings and clinical considerations for the use of telehealth interventions for PTSD. Curr. Treat. Options Psychiatry 30, 221–241. doi:10.1007/s40501-020-00215-x
Mu, D. Z., Xue, M., Xu, J. J., Hu, Y., Chen, Y., Ren, P., et al. (2020). Antidepression and prokinetic effects of paeoniflorin on rats in the forced swimming test via polypharmacology. Evid. Based. Complement. Altern. Med. 2020, 2153571. doi:10.1155/2020/2153571
Nakano, A., Tani, E., Miyazaki, K., Yamamoto, Y., and Furuyama, J. (1995). Matrix metalloproteinases and tissue inhibitors of metalloproteinases in human gliomas. J. Neurosurg. 83, 298–307. doi:10.3171/jns.1995.83.2.0298
Neacsu, P., Mazare, A., Schmuki, P., and Cimpean, A. (2015). Attenuation of the macrophage inflammatory activity by TiO₂ nanotubes via inhibition of MAPK and NF-κB pathways. Int. J. Nanomedicine 10, 6455–6467. doi:10.2147/IJN.S92019
Nguyen, L., Lucke-Wold, B. P., Logsdon, A. F., Scandinaro, A. L., Huber, J. D., and Matsumoto, R. R. (2016). Behavioral and biochemical effects of ketamine and dextromethorphan relative to its antidepressant-like effects in Swiss Webster mice. Neuroreport 27, 1004–1011. doi:10.1097/WNR.0000000000000646
Nie, X. H., Ou-yang, J., Xing, Y., Li, D. Y., Dong, X. Y., Liu, R. E., et al. (2015). Paeoniflorin inhibits human glioma cells via STAT3 degradation by the ubiquitin-proteasome pathway. Drug Des. devel. Ther. 9, 5611–5622. doi:10.2147/DDDT.S93912
Nuñez-Lumbreras, M. L. Á., Castañeda-Cabral, J. L., Valle-Dorado, M. G., Sánchez-Valle, V., Orozco-Suárez, S., Guevara-Guzmán, R., et al. (2021). Drug-resistant temporal lobe epilepsy alters the expression and functional coupling to gαi/o proteins of CB1 and CB2 receptors in the microvasculature of the human brain. Front. Behav. Neurosci. 14, 611780. doi:10.3389/fnbeh.2020.611780
Ohta, H., Matsumoto, K., Watanabe, H., and Shimizu, M. (1993). Involvement of alpha 1- but not alpha 2-adrenergic systems in the antagonizing effect of paeoniflorin on scopolamine-induced deficit in radial maze performance in rats. Jpn. J. Pharmacol. 62, 199–202. doi:10.1254/jjp.62.199
Ohta, H., Matsumoto, K., Watanabe, H., and Shimizu, M. (1993). Involvement of beta-adrenergic systems in the antagonizing effect of paeoniflorin on the scopolamine-induced deficit in radial maze performance in rats. Jpn. J. Pharmacol. 62, 345–349. doi:10.1254/jjp.62.345
Ohta, H., Ni, J. W., Matsumoto, K., Watanabe, H., and Shimizu, M. (1993). Peony and its major constituent, paeoniflorin, improve radial maze performance impaired by scopolamine in rats. Pharmacol. Biochem. Behav. 45, 719–723. doi:10.1016/0091-3057(93)90530-7
Onaivi, E. S., Ishiguro, H., Gu, S., and Liu, Q. R. (2012). CNS effects of CB2 cannabinoid receptors: Beyond neuro-immuno-cannabinoid activity. J. Psychopharmacol. 26, 92–103. doi:10.1177/0269881111400652
Price, R. B., and Mathew, S. J. (2015). Does ketamine have anti-suicidal properties? Current status and future directions. CNS Drugs 29, 181–188. doi:10.1007/s40263-015-0232-4
Qiu, F. M., Zhong, X. M., Mao, Q. Q., and Huang, Z. (2013). Antidepressant-like effects of paeoniflorin on the behavioural, biochemical, and neurochemical patterns of rats exposed to chronic unpredictable stress. Neurosci. Lett. 541, 209–213. doi:10.1016/j.neulet.2013.02.029
Qiu, Z. K., He, J. L., Liu, X., Zeng, J., Xiao, W., Fan, Q. H., et al. (2018). Anxiolytic-like effects of paeoniflorin in an animal model of post traumatic stress disorder. Metab. Brain Dis. 33, 1175–1185. doi:10.1007/s11011-018-0216-4
Ramic, E., Prasko, S., Gavran, L., and Spahic, E. (2020). Assessment of the antidepressant side effects occurrence in patients treated in primary care. Mat. Sociomed. 32, 131–134. doi:10.5455/msm.2020.32.131-134
Rating, D., Siemes, H., and Löscher, W. (1983). Low CSF GABA concentration in children with febrile convulsions, untreated epilepsy, and meningitis. J. Neurol. 230, 217–225. doi:10.1007/BF00313697
Seifert, G., Carmignoto, G., and Steinhäuser, C. (2010). Astrocyte dysfunction in epilepsy. Brain Res. Rev. 63, 212–221. doi:10.1016/j.brainresrev.2009.10.004
Small, C., Dagra, A., Martinez, M., Williams, E., and Lucke-Wold, B. (2022). Examining the role of astrogliosis and JNK signaling in post-traumatic epilepsy. Egypt. J. Neurosurg. 37, 1. doi:10.1186/s41984-021-00141-x
Sun, J. Y., Zhao, S. J., Wang, H. B., Hou, Y. J., Mi, Q. J., Yang, M. F., et al. (2021). Ifenprodil improves long-term neurologic deficits through antagonizing glutamate-induced excitotoxicity after experimental subarachnoid hemorrhage. Transl. Stroke Res. 12, 1067–1080. doi:10.1007/s12975-021-00906-4
Sun, R., Wang, K., Wu, D., Li, X., and Ou, Y. (2012). Protective effect of paeoniflorin against glutamate-induced neurotoxicity in PC12 cells via Bcl-2/Bax signal pathway. Folia Neuropathol. 50, 270–276. doi:10.5114/fn.2012.30527
Sun, X., Cao, Y. B., Hu, L. F., Yang, Y. P., Li, J., Wang, F., et al. (2011). ASICs mediate the modulatory effect by paeoniflorin on α-synuclein autophagic degradation. Brain Res. 1396, 77–87. doi:10.1016/j.brainres.2011.04.011
Sun, X., Li, S., Xu, L., Wang, H., Ma, Z., Fu, Q., et al. (2017). Paeoniflorin ameliorates cognitive dysfunction via regulating SOCS2/IRS-1 pathway in diabetic rats. Physiol. Behav. 174, 162–169. doi:10.1016/j.physbeh.2017.03.020
Sun, X., WangZhao, X. Z., Chen, J., Li, C., and Zhao, G. (2021). Paeoniflorin inhibited nod-like receptor protein-3 inflammasome and NF-κB-mediated inflammatory reactions in diabetic foot ulcer by inhibiting the chemokine receptor CXCR2. Drug Dev. Res. 82, 404–411. doi:10.1002/ddr.21763
Szeliga, M., Zgrzywa, A., Obara-Michlewska, M., and Albrecht, J. (2012). Transfection of a human glioblastoma cell line with liver-type glutaminase (LGA) down-regulates the expression of DNA-repair gene MGMT and sensitizes the cells to alkylating agents. J. Neurochem. 123, 428–436. doi:10.1111/j.1471-4159.2012.07917.x
Takeda, S., Isono, T., Wakui, Y., Matsuzaki, Y., Sasaki, H., Amagaya, S., et al. (1995). Absorption and excretion of paeoniflorin in rats. J. Pharm. Pharmacol. 47, 1036–1040. doi:10.1111/j.2042-7158.1995.tb03293.x
Tang, N. Y., Liu, C. H., Hsieh, C. T., and Hsieh, C. L. (2010). The anti-inflammatory effect of paeoniflorin on cerebral infarction induced by ischemia-reperfusion injury in Sprague-Dawley rats. Am. J. Chin. Med. 38, 51–64. doi:10.1142/S0192415X10007786
van Nifterik, K. A., van den Berg, J., van der Meide, W. F., Ameziane, N., Wedekind, L. E., Steenbergen, R. D., et al. (2010). Absence of the MGMT protein as well as methylation of the MGMT promoter predict the sensitivity for temozolomide. Br. J. Cancer 103, 29–35. doi:10.1038/sj.bjc.6605712
Venkatesan, S., Lamfers, M. L., Dirven, C. M., and Leenstra, S. (2016). Genetic biomarkers of drug response for small-molecule therapeutics targeting the RTK/Ras/PI3K, p53 or Rb pathway in glioblastoma. CNS Oncol. 5, 77–90. doi:10.2217/cns-2015-0005
Wang, D., Liu, L., Li, S., and Wang, C. (2018). Effects of paeoniflorin on neurobehavior, oxidative stress, brain insulin signaling, and synaptic alterations in intracerebroventricular streptozotocin-induced cognitive impairment in mice. Physiol. Behav. 191, 12–20. doi:10.1016/j.physbeh.2018.03.016
Wang, G., and Cheng, N. (2018). Paeoniflorin inhibits mast cell-mediated allergic inflammation in allergic rhinitis. J. Cell. Biochem. 119, 8636–8642. doi:10.1002/jcb.27135
Wang, K., Zhu, L., Zhu, X., Zhang, K., Huang, B., Zhang, J., et al. (2014). Protective effect of paeoniflorin on Aβ25-35-induced SH-SY5Y cell injury by preventing mitochondrial dysfunction. Cell. Mol. Neurobiol. 34, 227–234. doi:10.1007/s10571-013-0006-9
Wang, P., Wang, C., and Lou, Y. C. (2011). Borneol and Rhizome of Chuanxiong on facilitating permeation for compound shuyu jiangnao decoction through blood brain barrier. J. Hubei Univ. Chin. Med. 13, 21–23.
Wang, S., Zhao, X., Qiao, Z., Jia, X., and Qi, Y. (2018). Paeoniflorin attenuates depressive behaviors in systemic lupus erythematosus mice. Biomed. Pharmacother. 103, 248–252. doi:10.1016/j.biopha.2018.03.149
Wang, T., Xu, L., Gao, L., Zhao, L., Liu, X. H., Chang, Y. Y., et al. (2020). Paeoniflorin attenuates early brain injury through reducing oxidative stress and neuronal apoptosis after subarachnoid hemorrhage in rats. Metab. Brain Dis. 35, 959–970. doi:10.1007/s11011-020-00571-w
Wang, Z., Liu, Z., Yu, G., Nie, X., Jia, W., Liu, R. E., et al. (2018). Paeoniflorin inhibits migration and invasion of human glioblastoma cells via suppression transforming growth factor β-induced epithelial-mesenchymal transition. Neurochem. Res. 43, 760–774. doi:10.1007/s11064-018-2478-y
Wang, Z., Yu, G., Liu, Z., Zhu, J., Chen, C., Liu, R. E., et al. (2018). Paeoniflorin inhibits glioblastoma growth in vivo and in vitro: A role for the Triad3A-dependent ubiquitin proteasome pathway in TLR4 degradation. Cancer Manag. Res. 10, 887–897. doi:10.2147/CMAR.S160292
Wu, C., Li, B., Zhang, Y., Chen, T., Chen, C., Jiang, W., et al. (2020). Intranasal delivery of paeoniflorin nanocrystals for brain targeting. Asian J. Pharm. Sci. 15, 326–335. doi:10.1016/j.ajps.2019.11.002
Wu, C. C., Wang, Y. Z., Hu, H. Y., and Wang, X. Q. (2021). Bibliometric analysis of research on the comorbidity of cancer and pain. J. Pain Res. 14, 213–228. doi:10.2147/JPR.S291741
Wu, W., Qiu, C., Feng, X., Tao, X., Zhu, Q., Chen, Z., et al. (2020). Protective effect of paeoniflorin on acute cerebral infarction in rats. Curr. Pharm. Biotechnol. 21, 702–709. doi:10.2174/1389201021666191224151634
Wu, Y. M., Jin, R., Yang, L., Zhang, J., Yang, Q., Guo, Y. Y., et al. (2013). Phosphatidylinositol 3 kinase/protein kinase B is responsible for the protection of paeoniflorin upon H₂O₂-induced neural progenitor cell injury. Neuroscience 240, 54–62. doi:10.1016/j.neuroscience.2013.02.037
Xia, S. M., Shen, R., Sun, X. Y., Shen, L. L., Yang, Y. M., Ke, Y., et al. (2007). Development and validation of a sensitive liquid chromatography-tandem mass spectrometry method for the determination of paeoniflorin in rat brain and its application to pharmacokinetic study. J. Chromatogr. B Anal. Technol. Biomed. Life Sci. 857, 32–39. doi:10.1016/j.jchromb.2007.06.022
Xiang, Y., Zhang, Q., Wei, S., Huang, C., Li, Z., and GaoPaeoniflorin, Y. (2020). Paeoniflorin: A monoterpene glycoside from plants of Paeoniaceae family with diverse anticancer activities. J. Pharm. Pharmacol. 72, 483–495. doi:10.1111/jphp.13204
Xiao, L., Wang, Y. Z., Liu, J., Luo, X. T., Ye, Y., and Zhu, X. Z. (2005). Effects of paeoniflorin on the cerebral infarction, behavioral and cognitive impairments at the chronic stage of transient middle cerebral artery occlusion in rats. Life Sci. 78, 413–420. doi:10.1016/j.lfs.2005.04.069
Xin, Q., Yuan, R., Shi, W., Zhu, Z., Wang, Y., and Cong, W. (2019). A review for the anti-inflammatory effects of paeoniflorin in inflammatory disorders. Life Sci. 237, 116925. doi:10.1016/j.lfs.2019.116925
Xiong, W., Yin, A., Mao, X., Zhang, W., Huang, H., and Zhang, X. (2016). Resveratrol suppresses human glioblastoma cell migration and invasion via activation of RhoA/ROCK signaling pathway. Oncol. Lett. 11, 484–490. doi:10.3892/ol.2015.3888
Yang, R., Wang, P., Chen, Z., Hu, W., Gong, Y., Zhang, W., et al. (2017). WY-14643, a selective agonist of peroxisome proliferator-activated receptor-α, ameliorates lipopolysaccharide-induced depressive-like behaviors by preventing neuroinflammation and oxido-nitrosative stress in mice. Pharmacol. Biochem. Behav. 153, 97–104. doi:10.1016/j.pbb.2016.12.010
Yang, T. Q., Lu, X. J., Wu, T. F., Ding, D. D., Zhao, Z. H., Chen, G. L., et al. (2014). MicroRNA-16 inhibits glioma cell growth and invasion through suppression of BCL2 and the nuclear factor-κB1/MMP9 signaling pathway. Cancer Sci. 105, 265–271. doi:10.1111/cas.12351
Yin, D., Liu, Y. Y., Wang, T. X., Hu, Z. Z., Qu, W. M., Chen, J. F., et al. (2016). Paeoniflorin exerts analgesic and hypnotic effects via adenosine A1 receptors in a mouse neuropathic pain model. Psychopharmacol. Berl. 233, 281–293. doi:10.1007/s00213-015-4108-6
Yin, N., Gao, Q., Tao, W., Chen, J., Bi, J., Ding, F., et al. (2020). Paeoniflorin relieves LPS-induced inflammatory pain in mice by inhibiting NLRP3 inflammasome activation via transient receptor potential vanilloid 1. J. Leukoc. Biol. 108, 229–241. doi:10.1002/JLB.3MA0220-355R
Yu, G., Wang, Z., Zeng, S., Liu, S., Zhu, C., Xu, R., et al. (2019). Paeoniflorin inhibits hepatocyte growth factor- (HGF-) induced migration and invasion and actin rearrangement via suppression of c-met-mediated RhoA/ROCK signaling in glioblastoma. Biomed. Res. Int. 2019, 9053295. doi:10.1155/2019/9053295
Yu, H. Y., Liu, M. G., Liu, D. N., Shang, G. W., Wang, Y., Qi, C., et al. (2007). Antinociceptive effects of systemic paeoniflorin on bee venom-induced various 'phenotypes' of nociception and hypersensitivity. Pharmacol. Biochem. Behav. 88, 131–140. doi:10.1016/j.pbb.2007.07.013
Yu, J. B., Zhao, Z. X., Peng, R., Pan, L. B., Fu, J., Ma, S. R., et al. (2019). Gut microbiota-based pharmacokinetics and the antidepressant mechanism of paeoniflorin. Front. Pharmacol. 10, 268. doi:10.3389/fphar.2019.00268
Yuan, J., Lu, Y., Wang, H., Feng, Y., Jiang, S., Gao, X. H., et al. (2020). Paeoniflorin resists H2O2-induced oxidative stress in melanocytes by JNK/Nrf2/HO-1 pathway. Front. Pharmacol. 11, 536. doi:10.3389/fphar.2020.00536
Yue, P., Lopez-Tapia, F., Paladino, D., Li, Y., Chen, C. H., Namanja, A. T., et al. (2016). Hydroxamic acid and benzoic acid-based STAT3 inhibitors suppress human glioma and breast cancer phenotypes in vitro and in vivo. Cancer Res. 76, 652–663. doi:10.1158/0008-5472.CAN-14-3558
Zanos, P., and Gould, T. D. (2018). Mechanisms of ketamine action as an antidepressant. Mol. Psychiatry 23, 801–811. doi:10.1038/mp.2017.255
Zass, L. J., Hart, S. A., Seedat, S., Hemmings, S. M., and Malan-Müller, S. (2017). Neuroinflammatory genes associated with post-traumatic stress disorder: Implications for comorbidity. Psychiatr. Genet. 27, 1–16. doi:10.1097/YPG.0000000000000143
Zhan, C. P., Zhuge, C. J., Yan, X. J., Dai, W. M., and Yu, G. F. (2021). Measuring serum melatonin concentrations to predict clinical outcome after aneurysmal subarachnoid hemorrhage. Clin. Chim. Acta. 513, 1–5. doi:10.1016/j.cca.2020.12.006
Zhang, G. Q., Hao, X. M., Chen, S. Z., Zhou, P. A., Cheng, H. P., and Wu, C. H. (2003). Blockade of paeoniflorin on sodium current in mouse hippocampal CA1 neurons. Acta Pharmacol. Sin. 24, 1248–1252.
Zhang, H. R., Peng, J. H., Cheng, X. B., Shi, B. Z., Zhang, M. Y., and Xu, R. X. (2015). Paeoniflorin atttenuates amyloidogenesis and the inflammatory responses in a transgenic mouse model of alzheimer's disease. Neurochem. Res. 40, 1583–1592. doi:10.1007/s11064-015-1632-z
Zhang, X., Zhai, Y., Yuan, J., and Hu, Y. (2019). New insights into Paeoniaceae used as medicinal plants in China. Sci. Rep. 9, 18469. doi:10.1038/s41598-019-54863-y
Zhang, X. J., Chen, H. L., Li, Z., Zhang, H. Q., Xu, H. X., Sung, J. J., et al. (2009). Analgesic effect of paeoniflorin in rats with neonatal maternal separation-induced visceral hyperalgesia is mediated through adenosine A(1) receptor by inhibiting the extracellular signal-regulated protein kinase (ERK) pathway. Pharmacol. Biochem. Behav. 94, 88–97. doi:10.1016/j.pbb.2009.07.013
Zhang, X. J., Li, Z., Leung, W. M., Liu, L., and XuBian, X. Z. X. (2008). The analgesic effect of paeoniflorin on neonatal maternal separation-induced visceral hyperalgesia in rats. J. Pain 9, 497–505. doi:10.1016/j.jpain.2007.12.009
Zhang, Y., Li, H., Huang, M., Huang, M., Chu, K., Xu, W., et al. (2015). Paeoniflorin, a monoterpene glycoside, protects the brain from cerebral ischemic injury via inhibition of apoptosis. Am. J. Chin. Med. 43, 543–557. doi:10.1142/S0192415X15500342
Zhang, Y., Qiao, L., Xu, W., Wang, X., Li, H., Xu, W., et al. (2017). Paeoniflorin attenuates cerebral ischemia-induced injury by regulating Ca2+/CaMKII/CREB signaling pathway. Molecules 22, 359. doi:10.3390/molecules22030359
Zhang, Y., Wang, L. L., Wu, Y., Wang, N., Wang, S. M., Zhang, B., et al. (2016). Paeoniflorin attenuates hippocampal damage in a rat model of vascular dementia. Exp. Ther. Med. 12, 3729–3734. doi:10.3892/etm.2016.3849
Zhang, Y., Zhu, M. Z., Qin, X. H., Zeng, Y. N., and Zhu, X. H. (2021). The ghrelin/growth hormone secretagogue receptor system is involved in the rapid and sustained antidepressant-like effect of paeoniflorin. Front. Neurosci. 15, 631424. doi:10.3389/fnins.2021.631424
Zhang, Z. Y., Fang, Y. J., Lenahan, C., and Chen, S. (2021). The role of immune inflammation in aneurysmal subarachnoid hemorrhage. Exp. Neurol. 336, 113535. doi:10.1016/j.expneurol.2020.113535
Zhen, Q., Liu, J. Y., Fu, S. L., Liu, Y. J., Qian, J., and Yang, M. (2012). Effects of chuanxiong rhizoma on brain pharmacokinetics of gastrodigenin in rats. Chin. J. New Drugs Clin. Rem. 31, 263–266.
Zheng, M., Liu, C., Fan, Y., Yan, P., Shi, D., and Zhang, Y. (2017). Neuroprotection by Paeoniflorin in the MPTP mouse model of Parkinson's disease. Neuropharmacology 116, 412–420. doi:10.1016/j.neuropharm.2017.01.009
Zhong, S. Z., Ge, Q. H., Li, Q., Qu, R., and Ma, S. P. (2009). Peoniflorin attentuates Abeta((1-42))-mediated neurotoxicity by regulating calcium homeostasis and ameliorating oxidative stress in hippocampus of rats. J. Neurol. Sci. 280, 71–78. doi:10.1016/j.jns.2009.01.027
Zhong, X., Li, G., Qiu, F., and Huang, Z. (2019). Paeoniflorin ameliorates chronic stress-induced depression-like behaviors and neuronal damages in rats via activation of the ERK-CREB pathway. Front. Psychiatry 9, 772. doi:10.3389/fpsyt.2018.00772
Zhou, D., Zhang, S., Hu, L., Gu, Y. F., Cai, Y., Wu, D., et al. (2019). Inhibition of apoptosis signal-regulating kinase by paeoniflorin attenuates neuroinflammation and ameliorates neuropathic pain. J. Neuroinflammation 16, 83. doi:10.1186/s12974-019-1476-6
Keywords: paeoniflorin, brain, neuroinflammation, oxidative stress, neuroprotection
Citation: Hong H, Lu X, Wu C, Chen J, Chen C, Zhang J, Huang C and Cui Z (2022) A review for the pharmacological effects of paeoniflorin in the nervous system. Front. Pharmacol. 13:898955. doi: 10.3389/fphar.2022.898955
Received: 18 March 2022; Accepted: 22 July 2022;
Published: 15 August 2022.
Edited by:
Brandon Peter Lucke-Wold, University of Florida, United StatesReviewed by:
Yanling Zhao, Fifth Medical Center of the PLA General Hospital, ChinaFaheem Ullah, University of Science Malaysia, Malaysia
Copyright © 2022 Hong, Lu, Wu, Chen, Chen, Zhang, Huang and Cui. This is an open-access article distributed under the terms of the Creative Commons Attribution License (CC BY). The use, distribution or reproduction in other forums is permitted, provided the original author(s) and the copyright owner(s) are credited and that the original publication in this journal is cited, in accordance with accepted academic practice. No use, distribution or reproduction is permitted which does not comply with these terms.
*Correspondence: Zhiming Cui, Y3ptbnR5eUAxNjMuY29t