- Department of Cardiology, The Affiliated Hospital of Southwest Medical University, Luzhou, China
Arrhythmias are clinically prevalent with a high mortality rate. They impose a huge economic burden, thereby substantially affecting the quality of life. Sodium-glucose co-transporter 2 inhibitor (SGLT2i) is a new type of hypoglycemic drug, which can regulate blood glucose level safely and effectively. Additionally, it reduces the occurrence and progression of heart failure and cardiovascular events significantly. Recently, studies have found that SGLT2i can alleviate the occurrence and progression of cardiac arrhythmias; however, the exact mechanism remains unclear. In this review, we aimed to discuss and summarize new literature on different modes in which SGLT2i ameliorates the occurrence and development of cardiac arrhythmias.
Introduction
Arrhythmia is a common cardiovascular disease (CVD) generally associated with organic heart diseases. Different types of arrhythmias are known where bradyarrhythmias and tachyarrhythmias are the most common. The development of pacemakers has proven to be a boon for patients with bradyarrhythmias. However, the onset of tachyarrhythmia is usually acute, and some tachyarrhythmias, such as atrial fibrillation (AF), ventricular tachycardia, and ventricular fibrillation (VF), increase the risk of disability and death (Gheini et al., 2020). Although radiofrequency ablation has been shown to improve the health of patients with tachyarrhythmias (Morillo et al., 2014; Guandalini et al., 2019), the overall therapeutic effect is still inadequate, especially the lack of drugs for tachyarrhythmias. This insufficiency is because the currently available ones pose a risk of developing arrhythmias (Tisdale et al., 2020).
Sodium-glucose cotransporter 2 inhibitors (SGLT2is) are a new class of hypoglycemic drug that includes empagliflozin (EMPA), dapagliflozin (DAPA), canagliflozin (CANA), sotagliflozin, and ertugliflozin. Unlike traditional hypoglycemic drugs, this class does not rely on improving insulin secretion or resistance. However, it reduces the renal threshold for glucose and increases its excretion in the urine by inhibiting sodium-glucose cotransporter 2 of the proximal renal tubules from active reabsorption of glucose, thus lowering blood glucose (Zelniker and Braunwald, 2020). This anti-diabetic mechanism is weakened at low blood glucose concentrations, thereby reducing the risk of hypoglycemia. The DECLARE-TIMI 58 trial showed that the incidence of hypoglycemic events was significantly lower in the dapagliflozin group than in the control group (Wiviott et al., 2019). Additionally, SGLT2i also plays a role in lowering blood pressure, lipid regulation, anti-inflammatory response, and lowering of uric acid levels (Chino et al., 2014; Hayashi et al., 2017; Tentolouris et al., 2019; Arow et al., 2020). A large number of studies have confirmed that SGLT2i also plays a significant role in cardioprotection (Zelniker et al., 2019a; Zelniker et al., 2019b; Wiviott et al., 2019). The DECLARE-TIMI 58 trial showed that the cardiorenal secondary composite outcome in patients with type 2 diabetes mellitus (T2DM) was significantly reduced with dapagliflozin compared to placebo (hazard ratio = 0.76, 95% CI = 0.67–0.87; p < 0.0001) (Mosenzon et al., 2019). The DAPA-HF, EMPEROR-Reduced, and EMPA-REG OUTCOME trials have confirmed that SGLT2i can significantly reduce the occurrence and progression of heart failure (HF) and cardiovascular events (Zelniker et al., 2019a; McMurray et al., 2019; Zannad et al., 2020). Recently, SGLT2i has been reported to improve arrhythmias. The DECLARE-TIMI 58 trial showed that DAPA reduced the risk of atrial fibrillation/atrial flutter (AF/AFL) by 19% in patients with type 2 diabetes (Zelniker et al., 2020). In mice with mitral regurgitation, DAPA reduced the induction rate and duration of AF (Lin et al., 2021). EMPA was reported to shorten the Q-T interval of diabetic rats (QTc: 190 ± 4 ms vs. 160 ± 3 ms, p < 0.005) and reduce the duration of action potential (Lee et al., 2019). Additionally, it did not change the heart rate or QTc interval in patients with T2DM, but QTc dispersion could be significantly reduced (Sato et al., 2017). In diabetic mice, EMPA affected the inter-atrial conduction time and a high dose (30 mg/kg) reduced the incidence of AF from 85% to 36.8% (Shao et al., 2019). It also reduced the induction rate of VF post myocardial ischemia (Azam et al., 2021). However, the specific mechanism by which SGLT2i ameliorates arrhythmias remains unclear. Arrhythmias are related to cardiac electrical remodeling, mitochondrial dysfunction, oxidative stress, cardiac structural remodeling, inflammation, and autophagy. Based on the above-mentioned factors, we aimed to review the possible mechanisms of SGLT2i in improving arrhythmia conditions.
Cardiac Electrical Remodeling and Arrhythmias
Arrhythmia is closely related to cardiac electrical remodeling. In 1995, Wijffels et al. found that in the goat model of AF created by implanting a pacemaker, the atrial effective refractory period gradually shortened with the extension of atrial pacing time. This proved that cardiac electrical remodeling is involved in the process of AF and that cardiac electrical remodeling caused AF to persist (AF-induced AF) (Wijffels et al., 1995). Ion channel remodeling is the basis of cardiac electrical remodeling. Notably, intracellular Na+ and Ca2+ overload, caused by the Na+ and Ca2+ channel remodeling, and the disorder of K+ currents, play important roles in the occurrence and maintenance of arrhythmias.
The late sodium current (INa-Late) is formed by a small amount of slow inactivation or reopening of sodium channels during myocardial repolarization, persisting in the repolarization phase of the action potential, especially the plateau phase, and is important for regulating the duration of action potentials (Zaza et al., 2008). Its duration was 10–100 ms, and its amplitude was approximately 1% of the peak sodium current (peak INa). When INa-Late increases, the increased Na+ influx causes Na+ overload in cardiomyocytes (Wu et al., 2009; Sossalla et al., 2010; Poulet et al., 2015; Zhang et al., 2017), which increases the incidence of arrhythmias. Studies have shown that delayed afterdepolarizations (DADs), early afterdepolarizations, and continuous triggering activity occur with the persistence of Anemonia sulcata toxin II. Both DADs and continuous triggering activity decreased after the administration of ranolazine and tetrodotoxin, suggesting that inhibiting INa-Late could ameliorate arrhythmias (Belardinelli et al., 2006; Song et al., 2008). The increased activity of the Na+-H+ exchanger (NHE) also plays an important role in the occurrence and maintenance of arrhythmias (Ayoub et al., 2007; Hui et al., 2008; Altemose et al., 2001). NHE is essential for the regulation of Na+ concentration in cardiomyocytes (Madonna and De Caterina, 2013). In a canine AF model, the NHE-selective inhibitor HOE642 significantly improved the effective refractory period, thereby improving AF. This can be attributed to the reduction in the Na+ concentration in cardiomyocytes (Jayachandran et al., 2000).
Under physiological conditions, L-type Ca2+ channels (ICaL)of cardiomyocyte membrane are activated in the depolarization phase. Thus, a small amount of Ca2+ enters into the cytoplasm and binds to Ca2+-binding sites in the sarcoplasmic reticulum (SR) membrane. This is followed by opening of Ca2+-releasing channels ryanodine receptors 2 (RYR2) in SR leading to generation of the Ca2+ spark. This results in an increase of intracellular Ca2+ to over one hundred times instantly and is referred to as calcium-induced calcium release (CICR) (Fabiato, 1983; Cannell and Kong, 2017). Ca2+ sparks are the basis for excitation-contraction coupling in cardiomyocytes (Cheng et al., 1993). Na+-Ca2+ exchanger (NCX) is a type of membrane protein involved in bidirectional transport (3 Na+ for 1 Ca2+) and is regulated by a transmembrane Na+ gradient. In the case of Na+ overload, NCX reverse transport leads to cytoplasmic Ca2+ increase, eventually leading to Ca2+ overload. Increased Ca2+ levels can induce CICR, enhance the excitability of cardiomyocytes, and increase the possibility of arrhythmias (Greiser and Schotten, 2013; Chu et al., 2019). Ca2+ leakage from SR is also responsible for Ca2+ overload in cardiomyocytes. Hyperphosphorylation of RYR2 induced by the increased activity of Ca2+/calmodulin-dependent protein kinase type-II (CaMKII) is the basis of Ca2+ leakage from the SR in cardiomyocytes of patients with arrhythmias (Sag et al., 2009; Neef et al., 2010; Voigt et al., 2012). Studies have reported that INa-Late influences Ca2+ homeostasis in cardiomyocytes by activating CaMKII (Fischer et al., 2015). In addition, sarcoplasmic/endoplasmic reticulum Ca2+ ATPase 2a (SERCA2a), a calcium pump on SR, plays an important role in maintaining intracellular Ca2+ homeostasis in cardiomyocytes (Zhang et al., 2018). During myocardial diastole, most of the Ca2+ in the cytoplasm of cardiomyocytes is recycled to the SR via SERCA2a. When the quantity and activity of SERCA2a decreases, the amount and rate of Ca2+ clearance in the cytoplasm is affected, leading to Ca2+ overload and therefore arrhythmias (Lyon et al., 2011; Zaman et al., 2016; Samuel et al., 2018).
The K+ currents are the main current in the cardiomyocytes’ repolarization phase, they have been confirmed to be related to arrhythmias, including the transient outward K+ current (Ito), the delayed-rectifier K+ current (IK), the inward rectifier K+ current (IK1), and the ATPsensitive K+ current (KATP) (Tamargo et al., 2004). The Ito plays a major role in the reduced gradient in action potential duration (APD) (Volk et al., 2001). Cardiomyocytes’ APD will be prolonged with the density of Ito decreasing. Yue et al. found that in the AF dog model, the decreasing of the Ito was related to altering cardiac electrophysiology and promoting arrhythmia maintenance (Yue et al., 1999). And the IK is the main current in the repolarization phase 3 of cardiomyocytes in many animals. It is closely related to the duration of cardiomyocytes’ APD and effective refractory period (ERP). The risk of sudden cardiac death may be enhanced by the decreasing of IK (Lengyel et al., 2008). IK1 is the most important current to maintain and stabilize the cardiomyocytes’ resting potential, and modulate the final repolarization phase of the action potential (AP), thus exerting profound effects on cardiac excitability and arrhythmogenesis. Studies reported IK1 is closely with ventricular arrhythmias after myocardial infarction (Zhai et al., 2017; Zhai et al., 2019).
SGLT2i Ameliorates Electrical Remolding
SGLT2i have been shown to improve intracytoplasmic Na+ overload in cardiomyocytes. Philippaert found that EMPA, DAPA, and CANA had no effect on peak INa in the cardiomyocytes of an HF mouse model, but could significantly inhibit INa-Late, which is a potent selective inhibitor of INa-Late. Furthermore, EMPA reduced the incidence of spontaneous calcium transients induced by INa-Late (Philippaert et al., 2021). It also reduced the INa-Late of ventricular myocytes in diabetic rats compared with the control group (Lee et al., 2019). In mouse cardiomyocytes, EMPA, DAPA, and CANA reduced the activity of NHE and concentration of Na+ (Uthman et al., 2018; Uthman et al., 2019). Durak A et al. reported that there was a slight but significant increase in the maximum value of INa measured at -40mV with no change in voltage-dependency, whereas DAPA treatment restored this current in metabolic syndrome rats, significantly. But DAPA didn’t affect the intracellular Na+ (Durak et al., 2018). Another report showed that EMPA could inhibit NHE flux in the rabbit and rat cardiomyocytes under high-glucose environment, reduce Na+ and Ca2+ levels in the cardiomyocytes, and increase the Ca2+ levels in the mitochondria. Under non-high glucose environment, EMPA could also reduce NHE flux and the concentration of Na+ in cardiomyocytes (Baartscheer et al., 2017). It was also reported that DAPA inhibits the upregulation of NHE in mouse cardiofibroblasts exposed to lipopolysaccharides (LPS). This effect may be related to the activation of AMP-activated protein kinase (AMPK) (Ye et al., 2018). Further, similar phenomenon was observed in human cardiomyocytes, and EMPA’s NHE inhibition was comparable to that of the NHE inhibitor, cariporide (Trum et al., 2020). However, the NHE inhibitor cariporide could significantly reduce the activity of NHE in isolated rat ventricular myocytes. Conversely, EMPA did not affect the concentration of Na+ in cardiomyocytes within a wide concentration range. There was no evidence that EMPA could ameliorate the concentration of Na+ in cardiomyocytes by reducing the activity of NHE (Chung et al., 2021).
SGLT2i can also improve intracellular Ca2+ overload in cardiomyocytes (Arow et al., 2020; Byrne et al., 2020). SGLT2i ameliorated Ca2+ regulation of cardiomyocytes in diabetic mice by increasing SERCA2a activity, resulting in increased intracellular Ca2+ influx into the SR and decreased intracellular Ca2+ concentration (Hammoudi et al., 2017; Joubert et al., 2017; Goerg et al., 2021). Lee et al. found that EMPA could reduce the decay time of Ca2+ transients in ventricular myocytes of diabetic rats and reduce the incidence and frequency of Ca2+ spark. The duration and width of Ca2+ sparks also could be shortened by EMPA. SERCA2a activity was increased, RYR2 phosphorylation was lower, and intracellular Ca2+ concentration was decreased, but the reverse NCX current could be increased (Lee et al., 2019). EMPA also reduces CaMKII activity, thereby improving intracellular Ca2+ regulation in cardiomyocytes (Mustroph et al., 2018). A study showed that compared with the control group, DAPA didn’t change in both the maximum value and voltage-dependency of ICaL measured at 0mV in metabolic syndrome rats’ cardiomyocytes. Rats’ cardiomyocytes treated with DAPA had larger averaged peak amplitude of Ca2+ transients, shorter time to peak amplitude and the half-time for recovery of Ca2+ transients, and more responses to acute caffeine (10 mM) exposures than in the metabolic syndrome rats’ cardiomyocytes (Durak et al., 2018).
SGLT2i can also ameliorate the disorder of K+ currents. Jhuo SJ et al. found that H9c2 cells were treated with adipocytokines from the pericardial and peripheral fat from the 20 C57BL/6J mice, divided into the control group, the metabolic syndrome group, the EMPA group, and the glibenclamide group, the IK in the EMPA group was significantly higher than that in the metabolic syndrome group and the glibenclamide group in the metabolic syndrome mice model (Jhuo et al., 2020). Durak A et al. found that compared with metabolic syndrome rats, IK in cardiomyocytes from metabolic syndrome rats treated with DAPA were found to be significantly increased at positive voltages such as from 0 to +70mV. Although the IK at negative potentials did not change, DAPA induced a significant increase in IK measured at -120mV. This effect of DAPA can be interpreted as its effect on the membrane potential to keep it at normal levels (Durak et al., 2018). However, there is no more relevant literature confirming that SGLT2is can improve the cardiomyocytes’ K+ currents currently (Figure 1).
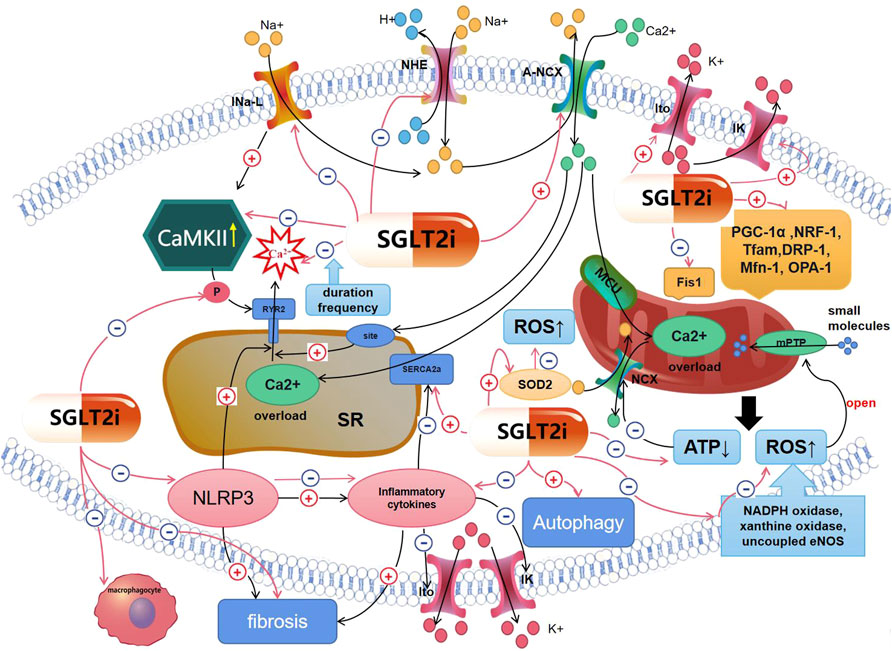
FIGURE 1. Mechanisms of SGLT2is′ effect on arrhythmias. Red lines represent the effect of SGLT2i; black lines mean mechanisms of arrhythmias. (1)SGLT2is can affect cardiomyocytes’ electrical remodeling, including inhibiting INa-Late, NHE,Ca2+ spark, the decreasing of ATP, RYR2 phosphorylation, CaMKII activity, inflammatory cytokines; increasing Ito, IK, SERCA2a activity, so that intracellular Na+ and Ca2+ overload and the disorder of K+ currents could be ameliorated. (2)SGLT2is can ameliorate arrhythmias by inhibiting myocardial inflammation and structural remodeling, including inhibiting NLRP3, inflammatory cytokines and fibrosis, so that K+ currents’ disorder can be improved. (3)SGLT2is can ameliorate arrhythmias by improving mitochondrial dysfunction and oxidative stress, including promoting mitochondrial biogenesis; inhibiting the decreasing of ATP, the increasing of ROS, so that intracellular ions administration can be improved. (4)SGLT2is can ameliorate arrhythmias by ameliorating autophagy.
Inflammation, Cardiac Structural Remodeling, and Arrhythmias
Inflammation is classified as acute and chronic inflammation, which includes three pathological processes: metamorphism, exudation, and hyperplasia. Inflammation is an essential component of the healing process of damaged tissues. However, chronic inflammation occurs when damaged tissue do not heal in a short time, leading to pathological healing and permanent fibrosis at the damaged site (Passino et al., 2015). Cardiac inflammation mainly occurs in CVDs such as myocardial infarction, HF, and myocarditis and is closely related to the occurrence of arrhythmias (Ishii et al., 2005; Peretto et al., 2020). Inflammatory cytokines, such as TNF-α, IL-1, IL-6, IL-8, and chemokines, are indicators of the degree of inflammation. Meanwhile, inflammatory cytokines are related closely to arrhythmias for inducing cellular electrical remodeling. TNF-α and IL-1β are two important cytokines that mediate inflammation and are mainly produced by M1-like macrophages. Saba S et al. found that compared with normal mice, the TNF-α mice (TNF1.6) with the overexpression of TNF-α are prone to spontaneous atrial arrhythmias. Compared with control atrial myocytes, female and male TNF1.6 myocytes displayed a significant reduction in the amplitude, the +dCai 2+/dtmax and -d Cai 2+/dtmax of the Cai 2+ transient, and prolongation of the TD50% (Saba et al., 2005). Fernández-Velasco M et al. found that compared with control, ventricular myocytes from Wistar rats treated with TNF-α showed significantly prolonged APD and reduced Ito, including amplitude and density. This manifestation may be caused by the selective inducible nitric oxide synthase (iNOS) induction and generation of oxidant species. But the ICaL didn’t change (Fernández-Velasco et al., 2007). Monnerat G demonstrated that in the ventricular cardiomyocytes treated with IL-1β, the APD was prolonged, the density of Ito was reduced, the amplitude and frequency of Ca2+ spark increased, but the SR Ca2+ content, the rate constants and the ratio of Ca2+ re-uptake/efflux were not significantly changed. Meanwhile, IL-1β significantly enhanced the number of spontaneous contractile events (NSE) in ventricular myocytes, and the effect of IL-1β on NSE was weakened in cardiomyocytes from mice with genetic CaMKII inhibition by cardiac-specific expression of autocamtide 3 inhibitory peptide (Monnerat et al., 2016). The nucleotide-binding oligomerization domain NOD-like receptor 3 (NLRP3), a molecular switch regulating inflammation, promotes the production of IL-1β and IL-8 in ischemic cardiomyocytes (Zhou et al., 2020a), inducing more serious inflammation. NLRP3 can further cause SR Ca2+ leakage and intracellular Ca2+ overload (Murakami et al., 2012). TNF-α is also closely related to the occurrence of cardiac electrical remodeling (London et al., 2003; Li et al., 2020). In rabbit pulmonary vein cardiomyocytes treated with TNF-α, a significant increase was observed in DADs amplitude, with a longer attenuation of the calcium transient and reduced expression levels of SERCA2a as compared to controls (Lee et al., 2007).
Inflammation can also lead to structural cardiac remodeling. Reportedly, the amount of total left atrial collagen in patients with AF is positively correlated with the level of inflammatory cytokines, including TNF-α, IL-6, MCP-1, and MMP-9 (Abe et al., 2018). Inflammatory cytokines activate immune cells, particularly monocytes, to recruit them from circulation into the myocardium. Once monocytes enter the myocardium, they differentiate into macrophages, which promote inflammation, damage, and fibrosis of the myocardium (Passino et al., 2015; Mack, 2018). In addition, macrophages also play an important role in the conduction of the cardiomyocytes. And connexin 43 (CX43) is crucial for macrophages’ conduction, and its abnormal expression and distribution will lead to the abnormality of the conduction of the cardiomyocytes, decreasing the conduction velocity and changing the anisotropy, producing arrhythmias (Peters et al., 1997; Morel et al., 2012; Zhang et al., 2014). Hulsmans et al. found that elongated cardiac macrophages expressing CX43, interspersing in the distal atrioventricular node, connecting with cardiomyocytes through CX43, facilitate electrical conduction, whereas conditional deletion of CX43 in macrophages and congenital lack of macrophages delay atrioventricular conduction (Hulsmans et al., 2017). Myocardial fibrosis is a sign of cardiac structural remodeling and the basis for the occurrence and persistence of arrhythmias, especially AF (Dzeshka et al., 2015; Moreira et al., 2020). As cardiomyocytes are permanent and non-regenerative, regeneration occurs via cells such as the interstitial cells, macrophages, and fibroblasts, during the hyperplasia phase. Fibroblasts play an important role in myocardial fibrosis development and are non-excitable cells in nature. However, they transmit electricity between cardiomyocytes via connexins, resulting in inhomogeneous conduction, depolarization of resting cardiomyocytes, and induced automatic depolarization of the four phases (Sohns and Marrouche, 2020), thereby increasing the risk of arrhythmias. Transforming growth factor beta-1 (TGF-β1), an effective stimulator of fibroblasts, has a pathogenic effect on valvular heart disease and arrhythmias (Khan and Sheppard, 2006; Salvarani et al., 2017; Liu et al., 2019). In addition, angiotensin II (Ang II) can also lead to myocardial fibrosis and increase the probability of arrhythmia (Purohit et al., 2013; Wang et al., 2019).
SGLT2i can Ameliorate Myocardial Inflammation and Structural Remodeling
SGLT2i have been reported to ameliorate inflammation (Lee et al., 2021; Wang et al., 2021). EMPA is shown to inhibit the increase of TNF-α and IL-6 levels in the cardiac tissue of Zucker diabetic fatty rats and patients with heart failure with preserved ejection fraction (HFpEF), and ameliorate microvascular inflammation (Kolijn et al., 2021). In vitro assays, it decreased the expression levels of TNF-α in LPS-induced mouse atrial myocytes and increased the expression levels of an anti-inflammatory M2 marker protein in LPS-treated macrophages (Koyani et al., 2020). EMPA also inhibits the infiltration of myocardial macrophages exposed to excessive glucocorticoids for a long time (Zhang et al., 2020). Philippaert et al. found that EMPA or tetrodotoxin infusion in isolated mouse hearts prevented the activation of NLRP3 in cardiomyocytes (Philippaert et al., 2021). It also reduced the mRNA levels of IL-6, chemokines, TNF-α, and MCP-1 in cardiomyocytes of diabetic rats significantly (Aragón-Herrera et al., 2019). The expression levels of IL-1β, IL-8, IL-6, and NLRP3 in mouse cardiomyocytes co-incubated with doxorubicin (DOXO)-EMPA were significantly reduced compared with those in mouse cardiomyocytes co-incubated with DOXO (Quagliariello et al., 2021). In addition, DAPA significantly reduced the mRNA levels of IL-1β, IL-6, NLRP3, and TNF-α in cardiomyocytes of T2MD mice (Ye et al., 2017). The anti-inflammatory effects of EMPA have also been observed in the kidneys and liver (Elkazzaz et al., 2021; Nasiri-Ansari et al., 2021).
SGLT2i can improve cardiac structural remodeling (Chowdhury et al., 2020; Madonna et al., 2020; Sun et al., 2020; Shentu et al., 2021). EMPA was reported to affect the oxidative stress and fibrosis by inhibiting the TGF-β/Smad signaling pathway (Li et al., 2019; Daud et al., 2021) and activate the Nrf2/ARE signaling pathway in cardiomyocytes in the T2MD mouse model (Li et al., 2019). DAPA also inhibits fibroblast activation and myocardial fibrosis (Shih et al., 2021; Tian et al., 2021) by inhibiting the TGF-β/Smad signaling pathway (Tian et al., 2021) in T2MD rats. EMPA could shift myocardial fuel from glucose to ketone bodies, free fatty acids, and branched amino acids to ameliorate the myocardial energetics and left ventricular structural remodeling of the non-diabetic HF pig model. A reduction in myocardial fibrosis was observed in imaging, histological, and cytological assessments (Santos-Gallego et al., 2019; Santos-Gallego et al., 2021). Sabatino et al. also found that, compared with the DOXO group, the degree of myocardial fibrosis in mice was reduced by 50% in the DOXO-EMPA group (Sabatino et al., 2020). DAPA is also shown to reduce the infiltration of myocardial fibroblasts and fibrosis after myocardial infarction in mice (Lee et al., 2017). The levels of collagen-1 and collagen-3 mRNA in the left ventricle of T2MD mice were also decreased by DAPA (Ye et al., 2017). It could inhibit myocardial hypertrophy, myocardial fibrosis, and increase myocardial collagen in SD rats injected with Ang II (Zhang et al., 2021). However, DAPA had a small effect on improving myocardial hypertrophy and fibrosis in the HFpEF model (Withaar et al., 2021). Meanwhile, a study showed compared with the metabolic group, significant attenuation of downregulated connexins (connexin40 and CX43) expression and significantly fewer fibrotic areas in ventricles of metabolic mice treated with EMPA, and the ECG QT interval was significantly shorter. The ERP of the left ventricle was also significantly shorter in the EMPA group than that in the control, metabolic, and glibenclamide groups (Jhuo et al., 2021)(Figure 1).
Mitochondrial Dysfunction, Oxidative Stress, and Arrhythmias
Mitochondria are energy factories that produce adenosine triphosphate (ATP). ATP production is achieved by the mitochondrial membrane potential (ΔΨ). ΔΨ is a criterion for evaluating the function of mitochondria, which can generate the necessary proton propulsion for ATP generation and release the energy required to phosphorylate ADP to ATP (Mitchell, 1961; El Hadi et al., 2019). Mitochondrial dysfunction is closely related to arrhythmias (Lin et al., 2003; Ahmad et al., 2018; Saadeh et al., 2021). When mitochondrial function is impaired, ATP production is hampered, such that the function of Na+ and Ca2+ pumps on the cell membrane is inhibited, concentration of Na+ and Ca2+ in the cardiomyocyte cytoplasm is overloaded, and normal electrical activity of cardiomyocytes is disrupted, resulting in arrhythmias (Gambardella et al., 2017). Overloaded Ca2+ can enter mitochondria through MCU, but due to the decrease in ATP production, NCX dysfunction occurs, resulting in an imbalance of Ca2+ flow in and out of the mitochondria. This imbalance results in the accumulation of Ca2+ in the mitochondria. The increased Ca2+ levels cause mitochondrial swelling, damage to ΔΨ and the respiratory chain, and further reduction of ATP generation, leading to arrhythmias (Boyman et al., 2021; Pool et al., 2021). Mitochondrial division/fusion and biogenesis play important roles in the maintenance of mitochondrial homeostasis. Peroxisome proliferator-activated receptor-γ co-activator-1α (PGC-1α) is a critical regulator of mitochondrial biosynthesis that promotes mitochondrial biogenesis and plays an important role in transcriptional regulation (Gleyzer et al., 2005). PGC-1α can regulate the expression and phosphorylation of mitochondrial fission/fusion proteins mitofusin 2 (Mfn2) and dynamin-related protein 1 (Drp1) to maintain the balance between mitochondrial fission and fusion (Peng et al., 2017), alleviate mitochondrial overdecomposition, and improve mitochondrial fusion levels. The mitochondrial outer membrane fusion is mediated by the membrane-localized GTPase mitofusins Mfn1 and Mfn2, and the intimal fusion is mediated by the membrane-localized GTPase OPA1. When mitochondria are dysfunctional, fission mediated by Drp1 and mitochondrial fission 1 (Fis1) can be promoted (Chen and Chan, 2010).
The mitochondrial respiratory chain is a major component of the reactive oxygen species (ROS) production in the body. NADPH oxidase, xanthine oxidase, and uncoupled endothelial nitric oxide synthase (eNOS) have been identified as the main sources of ROS. Under physiological conditions, ROS is produced in small amounts and can be removed by the antioxidant system over time. Under pathological conditions, ROS production is increased. When a large amount of ROS cannot be removed by the antioxidant system in time, oxidative stress occurs, resulting in the degeneration of proteins, lipids, and nucleic acids, and the normal structure and function of cells is destroyed (Zorov et al., 2014; Senoner and Dichtl, 2019). When mitochondria are dysfunctional, ROS production increases, which can further promote mitochondrial production of a large amount of ROS, a process known as ROS-induced ROS release (Zorov et al., 2000). Increased ROS levels result in loss of ion channel function and ion disorder in cardiomyocytes, leading to an increased probability of arrhythmia (Liu et al., 2010; Sovari and Dudley, 2012). In addition, ROS can further allow the mitochondrial permeability transition pore to open continuously, allowing large numbers of small molecules to enter into the mitochondria at will. Therefore, when ΔΨ is damaged, ATP production is reduced, calcium dyshomeostasis occurs, and the cell dies, stimulating further ROS production and thus promoting the occurrence of arrhythmias (Pérez and Quintanilla, 2017; Gordan et al., 2020). PGC-1α also plays a regulatory role in ROS clearance (Bai et al., 2011).
SGLT2i can Ameliorate Mitochondrial Dysfunction and Oxidative Stress
In recent years, studies have demonstrated that SGLT2i can improve mitochondrial dysfunction. The activation of AMPK mediated by EMPA reduced the consumption of ATP/ADP in cardiomyocytes cultured with LPS in vitro (Koyani et al., 2020). It also ameliorated the decrease of ATP levels in the non-infarct area after myocardial infarction in diabetic mice (Oshima et al., 2019). In atrial myocytes of diabetic mice model, high-dose EMPA (30 mg/kg) significantly improved mitochondrial respiratory function and ΔΨ. This dose led to increase in the expression levels of PGC-1α, NRF-1, Tfam, DRP-1, Mfn-1, and OPA-1. However, low-dose EMPA (10 mg/kg) did not improve DRP-1 significantly (Shao et al., 2019). EMPA also affected the upregulation of Fis1 and oxidative stress and recovered autophagy in the non-infarct area after myocardial infarction in diabetic mice, thus rescuing the mitochondrial numbers and size (Mizuno et al., 2018). In the rat model of cardiac ischemia/reperfusion (I/R), DAPA was more effective in reducing mitochondrial fission as compared to the dipeptidyl peptidase 4-inhibitor vildagliptin (Tanajak et al., 2018).
SGLT2i ameliorates oxidative stress. EMPA has been shown to reduce the oxidative stress by increasing the expression levels of superoxide dismutase 2, the antioxidant enzyme, in the myocardial tissue of diabetic mice (Li et al., 2019). It could also inhibit the activity of NADPH oxidase, significantly reducing the content of NADPH oxidase subtypes NOX1 and NOX2 in aortic cells of type I diabetes mellitus model rats. Further, it was able to reduce the levels of oxidative protein, increase the REDOX-sensitive enzyme aldehyde dehydrogenase 2 levels, significantly reduce the generation of ROS in aortic cells, and dose-dependently reduce the expression levels of eNOS and dihydrofolate reductase (Oelze et al., 2014). In addition, EMPA increased cardiac GTP enzyme cyclohydrolase 1 in cardiomyocytes of diabetic myocardial infarction model rats, thereby reducing ROS production (Asensio Lopez et al., 2020). It also inhibited the gene expression of inducible NO synthase in RAW 264.7 mouse macrophages post induction by LPS (Lee et al., 2021). Durak A et. also found that DAPA could normalize the levels of oxidative stress in cardiomyocytes of rats with metabolic syndrome, thereby inhibiting the prolonged ventricular-repolarization (Durak et al., 2018) (Figure 1).
Autophagy and Arrhythmias
Autophagy, also known as type II programmed cell death, is a lysosome-dependent catabolic pathway, which can be caused by an increase in AMPK activity and decrease in mammalian target of rapamycin (mTOR) activity. It can also be induced by separation of the Beclin protein complex from Bcl-2 (Mizushima et al., 2008; Alers et al., 2012; Jiang et al., 2018; Xu and Qin, 2019). Autophagy plays an important role in maintaining homeostasis (Parzych and Klionsky, 2014). During myocardial ischemia, the fusion of autophagosomes and lysosomes is blocked and autophagosome flow is impaired, leading to the accumulation of autophagosomes. This loss of function of clearing damaged organelles results in oxidative stress injury, ROS accumulation, and increased mitochondrial permeability (Shi et al., 2019), thereby increasing the risk of arrhythmias (Wiersma et al., 2017). In I/R rat model, the autophagy biomarkers Beclin-1 and LC3B-II/LC3B-I were significantly higher in the cardiomyocytes of VF mice than in those of non-VF mice (Meyer et al., 2013). Autophagy is also reduced in patients with AF. Studies have shown that in patients with cardiac valvular disease, the expression levels of autophagy markers, LC3B-II and LC3B-II/LC3B-I, were significantly reduced in patients with AF compared with those in patients with sinus rhythm (Chen et al., 2011; Zhou et al., 2020b).
SGLT2i can Ameliorate Autophagy
SGLT2i can ameliorate autophagy by upregulating mitochondrial autophagy-related protein Bnip3 mRNA levels, thereby increasing Bnip3 protein levels and preventing a decrease in the number of autophagy vacuoles in diabetic myocardial infarction model mice (Mizuno et al., 2018). EMPA and DAPA activated AMPK in the cardiomyocytes (Joubert et al., 2017; Aragón-Herrera et al., 2019) and enhance autophagy in early diabetic rats (Aragón-Herrera et al., 2019). Additionally, the effect of SGLT2i on improving the autophagy response has also been demonstrated in the liver and kidney. EMPA was found to enhance hepatic macrophage autophagy by enhancing the AMPK/mTOR signaling pathway, thereby inhibiting the IL-17/IL-23 inflammatory axis, alleviating inflammation, and significantly improving liver injury in T2DM mice with non-alcoholic fatty liver disease (NAFLD) (Meng et al., 2021). Nasiri-Ansari N et al. reported that EMPA could increase the AMPK/mTOR signaling pathway in NAFLD mice. Moreover, they found that the expression levels of autophagy markers LC3B and Bcl2/Bax was upregulated, leading to increase in autophagy in liver cells (Nasiri-Ansari et al., 2021). EMPA also regulates autophagy in glomerular podocytes. It was found that EMPA could increase the volume density of autophagosomes, autophagolysosomes, and lysosomes. Coupled with an increase in the levels of key regulatory protein Beclin-1 and the autophagy marker LAMP-1, it increased the apoptosis marker Bcl-2 levels in db mouse glomerular podocytes. When EMPA was combined with linagliptin, the expression levels of the apoptotic marker caspase-3 were reduced (Korbut et al., 2020) (Figure 1).
Conclusion
SGLT2i are a new type of hypoglycemic drug with good safety and tolerance. It can regulate blood glucose levels safely and effectively, thereby reducing the occurrence and progression of heart failure and cardiovascular events significantly. Studies have confirmed that SGLT2i ameliorates arrhythmia, but the specific mechanism remains unclear. It may ameliorate the occurrence and development of arrhythmias by improving cardiac electrical remodeling, mitochondrial dysfunction, cardiac structural remodeling, inflammation, oxidative stress, and autophagy. However, there is a lack of relevant studies to directly prove that SGLT2i can improve arrhythmias by improving the above-mentioned factors. A clinical trial to explore the related mechanisms of SGLT2i in ameliorating arrhythmias is required. This can aid in understanding the mechanisms in play and provide effective guidance for the drug treatment of arrhythmias in clinical practice.
Author Contributions
YJ is responsible for the literature review and writing, RY is responsible for the literature review, QY is responsible for sorting out the ideas and revising this article, WC provided advice, read literatures, and revised this article.
Funding
This study was supported by Medical Research project of Sichuan Province (No. S21111)
Conflict of Interest
The authors declare that the research was conducted in the absence of any commercial or financial relationships that could be construed as a potential conflict of interest.
Publisher’s Note
All claims expressed in this article are solely those of the authors and do not necessarily represent those of their affiliated organizations, or those of the publisher, the editors and the reviewers. Any product that may be evaluated in this article, or claim that may be made by its manufacturer, is not guaranteed or endorsed by the publisher.
References
Abe, I., Teshima, Y., Kondo, H., Kaku, H., Kira, S., Ikebe, Y., et al. (2018). Association of Fibrotic Remodeling and Cytokines/chemokines Content in Epicardial Adipose Tissue with Atrial Myocardial Fibrosis in Patients with Atrial Fibrillation. Heart rhythm. 15 (11), 1717–1727. Epub 2018 Jun 13. PMID: 29908372. doi:10.1016/j.hrthm.2018.06.025
Ahmad, S., Valli, H., Chadda, K. R., Cranley, J., Jeevaratnam, K., and Huang, C. L. (2018). Ventricular Pro-arrhythmic Phenotype, Arrhythmic Substrate, Ageing and Mitochondrial Dysfunction in Peroxisome Proliferator Activated Receptor-γ Coactivator-1β Deficient (Pgc-1β-/-) Murine Hearts. Mech. Ageing Dev. 173, 92–103. Epub 2018 May 12. PMID: 29763629; PMCID: PMC6004599. doi:10.1016/j.mad.2018.05.004
Alers, S., Löffler, A. S., Wesselborg, S., and Stork, B. (2012). Role of AMPK-mTOR-Ulk1/2 in the Regulation of Autophagy: Cross Talk, Shortcuts, and Feedbacks. Mol. Cell Biol. 32 (1), 2–11. Epub 2011 Oct 24. PMID: 22025673; PMCID: PMC3255710. doi:10.1128/MCB.06159-11
Altemose, G. T., Zipes, D. P., Weksler, J., Miller, J. M., and Olgin, J. E. (2001). Inhibition of the Na(+)/H(+) Exchanger Delays the Development of Rapid Pacing-Induced Atrial Contractile Dysfunction. Circulation 103 (5), 762–768. PMID: 11156891. doi:10.1161/01.cir.103.5.762
Aragón-Herrera, A., Feijóo-Bandín, S., Otero Santiago, M., Barral, L., Campos-Toimil, M., Gil-Longo, J., et al. (2019). Empagliflozin Reduces the Levels of CD36 and Cardiotoxic Lipids while Improving Autophagy in the Hearts of Zucker Diabetic Fatty Rats. Biochem. Pharmacol. 170, 113677. Epub 2019 Oct 21. PMID: 31647926. doi:10.1016/j.bcp.2019.113677
Arow, M., Waldman, M., Yadin, D., Nudelman, V., Shainberg, A., Abraham, N. G., et al. (2020). Sodium-glucose Cotransporter 2 Inhibitor Dapagliflozin Attenuates Diabetic Cardiomyopathy. Cardiovasc Diabetol. 19 (1), 7. PMID: 31924211; PMCID: PMC6953156. doi:10.1186/s12933-019-0980-4
Asensio Lopez, M. D. C., Lax, A., Hernandez Vicente, A., Saura Guillen, E., Hernandez-Martinez, A., Fernandez Del Palacio, M. J., et al. (2020). Empagliflozin Improves Post-infarction Cardiac Remodeling through GTP Enzyme Cyclohydrolase 1 and Irrespective of Diabetes Status. Sci. Rep. 10 (1), 13553. Erratum in: Sci Rep. 2020 Oct 9; 10(1):17266. PMID: 32782412; PMCID: PMC7419540. doi:10.1038/s41598-020-70454-8
Ayoub, I. M., Kolarova, J. D., Kantola, R. L., Radhakrishnan, J., Wang, S., and Gazmuri, R. J. (2007). Zoniporide Preserves Left Ventricular Compliance during Ventricular Fibrillation and Minimizes Postresuscitation Myocardial Dysfunction through Benefits on Energy Metabolism. Crit. Care Med. 35 (10), 2329–2336. PMID: 17944021. doi:10.1097/01.ccm.0000280569.87413.74
Azam, M. A., Chakraborty, P., Si, D., Du, B., Massé, S., Lai, P. F. H., et al. (2021). Anti-arrhythmic and Inotropic Effects of Empagliflozin Following Myocardial Ischemia. Life Sci. 276, 119440. Epub 2021 Mar 27. PMID: 33781832. doi:10.1016/j.lfs.2021.119440
Baartscheer, A., Schumacher, C. A., Wüst, R. C., Fiolet, J. W., Stienen, G. J., Coronel, R., et al. (2017). Empagliflozin Decreases Myocardial Cytoplasmic Na+ through Inhibition of the Cardiac Na+/H+ Exchanger in Rats and Rabbits. Diabetologia 60 (3), 568–573. Epub 2016 Oct 17. PMID: 27752710; PMCID: PMC6518059. doi:10.1007/s00125-016-4134-x
Bai, P., Cantó, C., Oudart, H., Brunyánszki, A., Cen, Y., Thomas, C., et al. (2011). PARP-1 Inhibition Increases Mitochondrial Metabolism through SIRT1 Activation. Cell Metab. 13 (4), 461–468. PMID: 21459330; PMCID: PMC3086520. doi:10.1016/j.cmet.2011.03.004
Belardinelli, L., Shryock, J. C., and Fraser, H. (2006). Inhibition of the Late Sodium Current as a Potential Cardioprotective Principle: Effects of the Late Sodium Current Inhibitor Ranolazine. Heart 92 (Suppl. 4), iv6–iv14. PMID: 16775092; PMCID: PMC1861317. doi:10.1136/hrt.2005.078790
Boyman, L., Greiser, M., and Lederer, W. J. (2021). Calcium Influx through the Mitochondrial Calcium Uniporter Holocomplex, MCUcx. J. Mol. Cell Cardiol. 151, 145–154. Epub 2020 Nov 2. PMID: 33147447; PMCID: PMC7880866. doi:10.1016/j.yjmcc.2020.10.015
Bridge, J. H., Smolley, J. R., and Spitzer, K. W. (1990). The Relationship between Charge Movements Associated with ICa and INa-Ca in Cardiac Myocytes. Science 248 (4953), 376–378. PMID: 2158147. doi:10.1126/science.2158147
Byrne, N. J., Matsumura, N., Maayah, Z. H., Ferdaoussi, M., Takahara, S., Darwesh, A. M., et al. (2020). Empagliflozin Blunts Worsening Cardiac Dysfunction Associated with Reduced NLRP3 (Nucleotide-Binding Domain-like Receptor Protein 3) Inflammasome Activation in Heart Failure. Circ. Heart Fail 13 (1), e006277. Epub 2020 Jan 20. PMID: 31957470. doi:10.1161/CIRCHEARTFAILURE.119.006277
Cannell, M. B., and Kong, C. H. T. (2017). Quenching the Spark: Termination of CICR in the Submicroscopic Space of the Dyad. J. Gen. Physiol. 149 (9), 837–845. Epub 2017 Aug 10. PMID: 28798280; PMCID: PMC5583711. doi:10.1085/jgp.201711807
Chen, H., and Chan, D. C. (2010). Physiological Functions of Mitochondrial Fusion. Ann. N. Y. Acad. Sci. 1201, 21–25. PMID: 20649534. doi:10.1111/j.1749-6632.2010.05615.x
Chen, M. C., Chang, J. P., Wang, Y. H., Liu, W. H., Ho, W. C., and Chang, H. W. (2011). Autophagy as a Mechanism for Myolysis of Cardiomyocytes in Mitral Regurgitation. Eur. J. Clin. Invest 41 (3), 299–307. Epub 2010 Nov 10. PMID: 21070221. doi:10.1111/j.1365-2362.2010.02410.x
Cheng, H., Lederer, W. J., and Cannell, M. B. (1993). Calcium Sparks: Elementary Events Underlying Excitation-Contraction Coupling in Heart Muscle. Science 262 (5134), 740–744. PMID: 8235594. doi:10.1126/science.8235594
Chino, Y., Samukawa, Y., Sakai, S., Nakai, Y., Yamaguchi, J., Nakanishi, T., et al. (2014). SGLT2 Inhibitor Lowers Serum Uric Acid through Alteration of Uric Acid Transport Activity in Renal Tubule by Increased Glycosuria. Biopharm. Drug Dispos. 35 (7), 391–404. Epub 2014 Aug 6. PMID: 25044127; PMCID: PMC4223977. doi:10.1002/bdd.1909
Chowdhury, B., Luu, A. Z., Luu, V. Z., Kabir, M. G., Pan, Y., Teoh, H., et al. (2020). The SGLT2 Inhibitor Empagliflozin Reduces Mortality and Prevents Progression in Experimental Pulmonary Hypertension. Biochem. Biophys. Res. Commun. 524 (1), 50–56. Epub 2020 Jan 21. PMID: 31980166. doi:10.1016/j.bbrc.2020.01.015
Chu, L., Greenstein, J. L., and Winslow, R. L. (2019). Na+ Microdomains and Sparks: Role in Cardiac Excitation-Contraction Coupling and Arrhythmias in Ankyrin-B Deficiency. J. Mol. Cell Cardiol. 128, 145–157. Epub 2019 Feb 5. PMID: 30731085. doi:10.1016/j.yjmcc.2019.02.001
Chung, Y. J., Park, K. C., Tokar, S., Eykyn, T. R., Fuller, W., Pavlovic, D., et al. (2021). Off-target Effects of Sodium-Glucose Co-transporter 2 Blockers: Empagliflozin Does Not Inhibit Na+/H+ Exchanger-1 or Lower [Na+]i in the Heart. Cardiovasc Res. 117 (14), 2794–2806. PMID: 33135077; PMCID: PMC8683707. doi:10.1093/cvr/cvaa323
Daud, E., Ertracht, O., Bandel, N., Moady, G., Shehadeh, M., Reuveni, T., et al. (2021). The Impact of Empagliflozin on Cardiac Physiology and Fibrosis Early after Myocardial Infarction in Non-diabetic Rats. Cardiovasc Diabetol. 20 (1), 132. PMID: 34215277; PMCID: PMC8254247. doi:10.1186/s12933-021-01322-6
Durak, A., Olgar, Y., Degirmenci, S., Akkus, E., Tuncay, E., and Turan, B. (2018). A SGLT2 Inhibitor Dapagliflozin Suppresses Prolonged Ventricular-Repolarization through Augmentation of Mitochondrial Function in Insulin-Resistant Metabolic Syndrome Rats. Cardiovasc Diabetol. 17 (1), 144. PMID: 30447687; PMCID: PMC6240275. doi:10.1186/s12933-018-0790-0
Dzeshka, M. S., Lip, G. Y., Snezhitskiy, V., and Shantsila, E. (2015). Cardiac Fibrosis in Patients with Atrial Fibrillation: Mechanisms and Clinical Implications. J. Am. Coll. Cardiol. 66 (8), 943–959. PMID: 26293766. doi:10.1016/j.jacc.2015.06.1313
El Hadi, H., Vettor, R., and Rossato, M. (2019). Cardiomyocyte Mitochondrial Dysfunction in Diabetes and its Contribution in Cardiac Arrhythmogenesis. Mitochondrion 46, 6–14. Epub 2019 Mar 21. PMID: 30905865. doi:10.1016/j.mito.2019.03.005
Elkazzaz, S. K., Khodeer, D. M., El Fayoumi, H. M., and Moustafa, Y. M. (2021). Role of Sodium Glucose Cotransporter Type 2 Inhibitors Dapagliflozin on Diabetic Nephropathy in Rats; Inflammation, Angiogenesis and Apoptosis. Life Sci. 280, 119018. Epub 2021 Feb 5. PMID: 33549594. doi:10.1016/j.lfs.2021.119018
Fabiato, A. (1983). Calcium-induced Release of Calcium from the Cardiac Sarcoplasmic Reticulum. Am. J. Physiol. 245 (1), C1–C14. PMID: 6346892. doi:10.1152/ajpcell.1983.245.1.C1
Fernández-Velasco, M., Ruiz-Hurtado, G., Hurtado, O., Moro, M. A., and Delgado, C. (2007). TNF-alpha Downregulates Transient Outward Potassium Current in Rat Ventricular Myocytes through iNOS Overexpression and Oxidant Species Generation. Am. J. Physiol. Heart Circ. Physiol. 293 (1), H238–H245. Epub 2007 Mar 2. PMID: 17337591. doi:10.1152/ajpheart.01122.2006
Fischer, T. H., Herting, J., Mason, F. E., Hartmann, N., Watanabe, S., Nikolaev, V. O., et al. (2015). Late INa Increases Diastolic SR-Ca2+-Leak in Atrial Myocardium by Activating PKA and CaMKII. Cardiovasc Res. 107 (1), 184–196. Epub 2015 May 18. PMID: 25990311; PMCID: PMC4476413. doi:10.1093/cvr/cvv153
Gambardella, J., Sorriento, D., Ciccarelli, M., Del Giudice, C., Fiordelisi, A., Napolitano, L., et al. (2017). Functional Role of Mitochondria in Arrhythmogenesis. Adv. Exp. Med. Biol. 982, 191–202. PMID: 28551788; PMCID: PMC6709870. doi:10.1007/978-3-319-55330-6_10
Gheini, A., Pourya, A., and Pooria, A. (2020). Atrial Fibrillation and Ventricular Tachyarrhythmias: Advancements for Better Outcomes. Cardiovasc Hematol. Disord. Drug Targets 20 (4), 249–259. PMID: 33001020. doi:10.2174/1871529X20666201001143907
Gleyzer, N., Vercauteren, K., and Scarpulla, R. C. (2005). Control of Mitochondrial Transcription Specificity Factors (TFB1M and TFB2M) by Nuclear Respiratory Factors (NRF-1 and NRF-2) and PGC-1 Family Coactivators. Mol. Cell Biol. 25 (4), 1354–1366. PMID: 15684387; PMCID: PMC548005. doi:10.1128/MCB.25.4.1354-1366.2005
Goerg, J., Sommerfeld, M., Greiner, B., Lauer, D., Seckin, Y., Kulikov, A., et al. (2021). Low-Dose Empagliflozin Improves Systolic Heart Function after Myocardial Infarction in Rats: Regulation of MMP9, NHE1, and SERCA2a. Int. J. Mol. Sci. 22 (11), 5437. PMID: 34063987; PMCID: PMC8196699. doi:10.3390/ijms22115437
Gordan, R., Fefelova, N., Gwathmey, J. K., and Xie, L. H. (2020). Iron Overload, Oxidative Stress and Calcium Mishandling in Cardiomyocytes: Role of the Mitochondrial Permeability Transition Pore. Antioxidants (Basel) 9 (8), 758. PMID: 32824344; PMCID: PMC7465659. doi:10.3390/antiox9080758
Greiser, M., and Schotten, U. (2013). Dynamic Remodeling of Intracellular Ca²⁺ Signaling during Atrial Fibrillation. J. Mol. Cell Cardiol. 58, 134–142. Epub 2013 Jan 5. PMID: 23298712. doi:10.1016/j.yjmcc.2012.12.020
Guandalini, G. S., Liang, J. J., and Marchlinski, F. E. (2019). Ventricular Tachycardia Ablation: Past, Present, and Future Perspectives. JACC Clin. Electrophysiol. 5 (12), 1363–1383. PMID: 31857035. doi:10.1016/j.jacep.2019.09.015
Hammoudi, N., Jeong, D., Singh, R., Farhat, A., Komajda, M., Mayoux, E., et al. (2017). Empagliflozin Improves Left Ventricular Diastolic Dysfunction in a Genetic Model of Type 2 Diabetes. Cardiovasc Drugs Ther. 31 (3), 233–246. PMID: 28643218; PMCID: PMC6681671. doi:10.1007/s10557-017-6734-1
Hayashi, T., Fukui, T., Nakanishi, N., Yamamoto, S., Tomoyasu, M., Osamura, A., et al. (2017). Correction to: Dapagliflozin Decreases Small Dense Low-Density Lipoprotein-Cholesterol and Increases High-Density Lipoprotein 2-cholesterol in Patients with Type 2 Diabetes: Comparison with Sitagliptin. Cardiovasc Diabetol. 16 (1), 149. Erratum for: Cardiovasc Diabetol. 2017 Jan 13; 16(1):8. PMID: 29132354; PMCID: PMC5683452. doi:10.1186/s12933-017-0608-5
Hui, Y., Junzhu, C., and Jianhua, Z. (2008). Gap Junction and Na+-H+ Exchanger Alternations in Fibrillating and Failing Atrium. Int. J. Cardiol. 128 (1), 147–149. Epub 2007 Jul 25. PMID: 17655944. doi:10.1016/j.ijcard.2007.06.070
Hulsmans, M., Clauss, S., Xiao, L., Aguirre, A. D., King, K. R., Hanley, A., et al. (2017). Macrophages Facilitate Electrical Conduction in the Heart. Cell 169 (3), 510–e20. PMID: 28431249; PMCID: PMC5474950. doi:10.1016/j.cell.2017.03.050
Ishii, Y., Schuessler, R. B., Gaynor, S. L., Yamada, K., Fu, A. S., Boineau, J. P., et al. (2005). Inflammation of Atrium after Cardiac Surgery Is Associated with Inhomogeneity of Atrial Conduction and Atrial Fibrillation. Circulation 111 (22), 2881–2888. Epub 2005 May 31. PMID: 15927979. doi:10.1161/CIRCULATIONAHA.104.475194
Jayachandran, J. V., Zipes, D. P., Weksler, J., and Olgin, J. E. (2000). Role of the Na +/H + Exchanger in Short-Term Atrial Electrophysiological Remodeling. Circulation 101 (15), 1861–1866. PMID: 10769289. doi:10.1161/01.cir.101.15.1861
Jhuo, S. J., Liu, I. H., Tasi, W. C., Chou, T. W., Lin, Y. H., Wu, B. N., et al. (2021). Characteristics of Ventricular Electrophysiological Substrates in Metabolic Mice Treated with Empagliflozin. Int. J. Mol. Sci. 22 (11), 6105. PMID: 34198942; PMCID: PMC8200966. doi:10.3390/ijms22116105
Jhuo, S. J., Liu, I. H., Tsai, W. C., Chou, T. W., Lin, Y. H., Wu, B. N., et al. (2020). Effects of Secretome from Fat Tissues on Ion Currents of Cardiomyocyte Modulated by Sodium-Glucose Transporter 2 Inhibitor. Molecules 25 (16), 3606. PMID: 32784369; PMCID: PMC7465695. doi:10.3390/molecules25163606
Jiang, J., Dai, J., and Cui, H. (2018). Vitexin Reverses the Autophagy Dysfunction to Attenuate MCAO-Induced Cerebral Ischemic Stroke via mTOR/Ulk1 Pathway. Biomed. Pharmacother. 99, 583–590. Epub 2018 Feb 20. PMID: 29710456. doi:10.1016/j.biopha.2018.01.067
Joubert, M., Jagu, B., Montaigne, D., Marechal, X., Tesse, A., Ayer, A., et al. (2017). The Sodium-Glucose Cotransporter 2 Inhibitor Dapagliflozin Prevents Cardiomyopathy in a Diabetic Lipodystrophic Mouse Model. Diabetes 66 (4), 1030–1040. Epub 2017 Jan 4. PMID: 28052965. doi:10.2337/db16-0733
Khan, R., and Sheppard, R. (2006). Fibrosis in Heart Disease: Understanding the Role of Transforming Growth Factor-Beta in Cardiomyopathy, Valvular Disease and Arrhythmia. Immunology 118 (1), 10–24. PMID: 16630019; PMCID: PMC1782267. doi:10.1111/j.1365-2567.2006.02336.x
Kolijn, D., Pabel, S., Tian, Y., Lódi, M., Herwig, M., Carrizzo, A., et al. (2021). Empagliflozin Improves Endothelial and Cardiomyocyte Function in Human Heart Failure with Preserved Ejection Fraction via Reduced Pro-inflammatory-oxidative Pathways and Protein Kinase Gα Oxidation. Cardiovasc Res. 117 (2), 495–507. PMID: 32396609. doi:10.1093/cvr/cvaa123
Korbut, A. I., Taskaeva, I. S., Bgatova, N. P., Muraleva, N. A., Orlov, N. B., Dashkin, M. V., et al. (2020). SGLT2 Inhibitor Empagliflozin and DPP4 Inhibitor Linagliptin Reactivate Glomerular Autophagy in Db/db Mice, a Model of Type 2 Diabetes. Int. J. Mol. Sci. 21 (8), 2987. PMID: 32340263; PMCID: PMC7215949. doi:10.3390/ijms21082987
Koyani, C. N., Plastira, I., Sourij, H., Hallström, S., Schmidt, A., Rainer, P. P., et al. (2020). Empagliflozin Protects Heart from Inflammation and Energy Depletion via AMPK Activation. Pharmacol. Res. 158, 104870. Epub 2020 May 17. PMID: 32434052. doi:10.1016/j.phrs.2020.104870
Lee, N., Heo, Y. J., Choi, S. E., Jeon, J. Y., Han, S. J., Kim, D. J., et al. (2021). Anti-inflammatory Effects of Empagliflozin and Gemigliptin on LPS-Stimulated Macrophage via the IKK/NF-κB, MKK7/JNK, and JAK2/STAT1 Signalling Pathways. J. Immunol. Res. 2021, 9944880. PMID: 34124273; PMCID: PMC8192181. doi:10.1155/2021/9944880
Lee, S. H., Chen, Y. C., Chen, Y. J., Chang, S. L., Tai, C. T., Wongcharoen, W., et al. (2007). Tumor Necrosis Factor-Alpha Alters Calcium Handling and Increases Arrhythmogenesis of Pulmonary Vein Cardiomyocytes. Life Sci. 80 (19), 1806–1815. Epub 2007 Mar 1. PMID: 17383682. doi:10.1016/j.lfs.2007.02.029
Lee, T. I., Chen, Y. C., Lin, Y. K., Chung, C. C., Lu, Y. Y., Kao, Y. H., et al. (2019). Empagliflozin Attenuates Myocardial Sodium and Calcium Dysregulation and Reverses Cardiac Remodeling in Streptozotocin-Induced Diabetic Rats. Int. J. Mol. Sci. 20 (7), 1680. PMID: 30987285; PMCID: PMC6479313. doi:10.3390/ijms20071680
Lee, T. M., Chang, N. C., and Lin, S. Z. (2017). Dapagliflozin, a Selective SGLT2 Inhibitor, Attenuated Cardiac Fibrosis by Regulating the Macrophage Polarization via STAT3 Signaling in Infarcted Rat Hearts. Free Radic. Biol. Med. 104, 298–310. Epub 2017 Jan 26. PMID: 28132924. doi:10.1016/j.freeradbiomed.2017.01.035
Lengyel, C., Virág, L., Kovács, P. P., Kristóf, A., Pacher, P., Kocsis, E., et al. (2008). Role of Slow Delayed Rectifier K+-current in QT Prolongation in the Alloxan-Induced Diabetic Rabbit Heart. Acta Physiol. (Oxf) 192 (3), 359–368. Epub 2007 Oct 29. PMID: 17970826. doi:10.1111/j.1748-1716.2007.01753.x
Li, C., Zhang, J., Xue, M., Li, X., Han, F., Liu, X., et al. (2019). SGLT2 Inhibition with Empagliflozin Attenuates Myocardial Oxidative Stress and Fibrosis in Diabetic Mice Heart. Cardiovasc Diabetol. 18 (1), 15. PMID: 30710997; PMCID: PMC6359811. doi:10.1186/s12933-019-0816-2
Li, X., Xue, Y. M., Guo, H. M., Deng, C. Y., Peng, D. W., Yang, H., et al. (2020). High Hydrostatic Pressure Induces Atrial Electrical Remodeling through Upregulation of Inflammatory Cytokines. Life Sci. 242, 117209. Epub 2019 Dec 20. PMID: 31870776. doi:10.1016/j.lfs.2019.117209
Lin, P. H., Lee, S. H., Su, C. P., and Wei, Y. H. (2003). Oxidative Damage to Mitochondrial DNA in Atrial Muscle of Patients with Atrial Fibrillation. Free Radic. Biol. Med. 35 (10), 1310–1318. PMID: 14607530. doi:10.1016/j.freeradbiomed.2003.07.002
Lin, Y. W., Chen, C. Y., Shih, J. Y., Cheng, B. C., Chang, C. P., Lin, M. T., et al. (2021). Dapagliflozin Improves Cardiac Hemodynamics and Mitigates Arrhythmogenesis in Mitral Regurgitation-Induced Myocardial Dysfunction. J. Am. Heart Assoc. 10 (7), e019274. Epub 2021 Mar 20. PMID: 33749310; PMCID: PMC8174384. doi:10.1161/JAHA.120.019274
Liu, J., Zhuang, T., Pi, J., Chen, X., Zhang, Q., Li, Y., et al. (2019). Endothelial Forkhead Box Transcription Factor P1 Regulates Pathological Cardiac Remodeling through Transforming Growth Factor-Β1-Endothelin-1 Signal Pathway. Circulation 140 (8), 665–680. Epub 2019 Jun 10. PMID: 31177814. doi:10.1161/CIRCULATIONAHA.119.039767
Liu, M., Liu, H., and Dudley, S. C. (2010). Reactive Oxygen Species Originating from Mitochondria Regulate the Cardiac Sodium Channel. Circ. Res. 107 (8), 967–974. Epub 2010 Aug 19. PMID: 20724705; PMCID: PMC2955818. doi:10.1161/CIRCRESAHA.110.220673
London, B., Baker, L. C., Lee, J. S., Shusterman, V., Choi, B. R., Kubota, T., et al. (2003). Calcium-dependent Arrhythmias in Transgenic Mice with Heart Failure. Am. J. Physiol. Heart Circ. Physiol. 284 (2), H431–H441. Epub 2002 Oct 17. PMID: 12388316. doi:10.1152/ajpheart.00431.2002
Lyon, A. R., Bannister, M. L., Collins, T., Pearce, E., Sepehripour, A. H., Dubb, S. S., et al. (2011). SERCA2a Gene Transfer Decreases Sarcoplasmic Reticulum Calcium Leak and Reduces Ventricular Arrhythmias in a Model of Chronic Heart Failure. Circ. Arrhythm. Electrophysiol. 4 (3), 362–372. Epub 2011 Mar 15. PMID: 21406682; PMCID: PMC3119354. doi:10.1161/CIRCEP.110.961615
Mack, M. (2018). Inflammation and Fibrosis. Matrix Biol. 68-69, 106–121. Epub 2017 Nov 28. PMID: 29196207. doi:10.1016/j.matbio.2017.11.010
Madonna, R., and De Caterina, R. (2013). Sodium-hydrogen Exchangers (NHE) in Human Cardiovascular Diseases: Interfering Strategies and Their Therapeutic Applications. Vasc. Pharmacol. 59 (5-6), 127–130. Epub 2013 Oct 15. PMID: 24140414. doi:10.1016/j.vph.2013.10.001
Madonna, R., Doria, V., Minnucci, I., Pucci, A., Pierdomenico, D. S., and De Caterina, R. (2020). Empagliflozin Reduces the Senescence of Cardiac Stromal Cells and Improves Cardiac Function in a Murine Model of Diabetes. J. Cell Mol. Med. 24 (21), 12331–12340. Epub 2020 Sep 17. PMID: 32940423; PMCID: PMC7687009. doi:10.1111/jcmm.15699
McMurray, J. J. V., Solomon, S. D., Inzucchi, S. E., Køber, L., Kosiborod, M. N., Martinez, F. A., et al. (2019). Dapagliflozin in Patients with Heart Failure and Reduced Ejection Fraction. N. Engl. J. Med. 381 (21), 1995–2008. Epub 2019 Sep 19. PMID: 31535829. doi:10.1056/NEJMoa1911303
Meng, Z., Liu, X., Li, T., Fang, T., Cheng, Y., Han, L., et al. (2021). The SGLT2 Inhibitor Empagliflozin Negatively Regulates IL-17/IL-23 Axis-Mediated Inflammatory Responses in T2DM with NAFLD via the AMPK/mTOR/autophagy Pathway. Int. Immunopharmacol. 94, 107492. Epub 2021 Feb 26. PMID: 33647823. doi:10.1016/j.intimp.2021.107492
Meyer, G., Czompa, A., Reboul, C., Csepanyi, E., Czegledi, A., Bak, I., et al. (2013). The Cellular Autophagy Markers Beclin-1 and LC3B-II Are Increased during Reperfusion in Fibrillated Mouse Hearts. Curr. Pharm. Des. 19 (39), 6912–6918. PMID: 23590156. doi:10.2174/138161281939131127122510
Mitchell, P. (1961). Coupling of Phosphorylation to Electron and Hydrogen Transfer by a Chemi-Osmotic Type of Mechanism. Nature 191, 144–148. PMID: 13771349. doi:10.1038/191144a0
Mizuno, M., Kuno, A., Yano, T., Miki, T., Oshima, H., Sato, T., et al. (2018). Empagliflozin Normalizes the Size and Number of Mitochondria and Prevents Reduction in Mitochondrial Size after Myocardial Infarction in Diabetic Hearts. Physiol. Rep. 6 (12), e13741. PMID: 29932506; PMCID: PMC6014462. doi:10.14814/phy2.13741
Mizushima, N., Levine, B., Cuervo, A. M., and Klionsky, D. J. (2008). Autophagy Fights Disease through Cellular Self-Digestion. Nature 451 (7182), 1069–1075. PMID: 18305538; PMCID: PMC2670399. doi:10.1038/nature06639
Monnerat, G., Alarcón, M. L., Vasconcellos, L. R., Hochman-Mendez, C., Brasil, G., Bassani, R. A., et al. (2016). Macrophage-dependent IL-1β Production Induces Cardiac Arrhythmias in Diabetic Mice. Nat. Commun. 7, 13344. Erratum in: Nat Commun. 2021 Dec 8;12(1):7261. PMID: 27882934; PMCID: PMC5123037. doi:10.1038/ncomms13344
Moreira, L. M., Takawale, A., Hulsurkar, M., Menassa, D. A., Antanaviciute, A., Lahiri, S. K., et al. (2020). Paracrine Signalling by Cardiac Calcitonin Controls Atrial Fibrogenesis and Arrhythmia. Nature 587 (7834), 460–465. Epub 2020 Nov 4. PMID: 33149301. doi:10.1038/s41586-020-2890-8
Morel, S., Frias, M. A., Rosker, C., James, R. W., Rohr, S., and Kwak, B. R. (2012). The Natural Cardioprotective Particle HDL Modulates Connexin43 Gap Junction Channels. Cardiovasc Res. 93 (1), 41–49. Epub 2011 Sep 29. PMID: 21960685. doi:10.1093/cvr/cvr257
Morillo, C. A., Verma, A., Connolly, S. J., Kuck, K. H., Nair, G. M., Champagne, J., et al. (2014). Radiofrequency Ablation vs Antiarrhythmic Drugs as First-Line Treatment of Paroxysmal Atrial Fibrillation (RAAFT-2): a Randomized Trial. JAMA 311 (7), 692–700. Erratum in: JAMA. 2014 Jun 11; 311(22):2337. Erratum in: JAMA. 2021 Jul 27; 326(4):360. PMID: 24549549. doi:10.1001/jama.2014.467
Mosenzon, O., Wiviott, S. D., Cahn, A., Rozenberg, A., Yanuv, I., Goodrich, E. L., et al. (2019). Effects of Dapagliflozin on Development and Progression of Kidney Disease in Patients with Type 2 Diabetes: an Analysis from the DECLARE-TIMI 58 Randomised Trial. Lancet Diabetes Endocrinol. 7 (8), 606–617. Epub 2019 Jun 10. Erratum in: Lancet Diabetes Endocrinol. 2019 Aug; 7(8):e20. PMID: 31196815. doi:10.1016/S2213-8587(19)30180-9
Murakami, T., Ockinger, J., Yu, J., Byles, V., McColl, A., Hofer, A. M., et al. (2012). Critical Role for Calcium Mobilization in Activation of the NLRP3 Inflammasome. Proc. Natl. Acad. Sci. U. S. A. 109 (28), 11282–11287. Epub 2012 Jun 25. PMID: 22733741; PMCID: PMC3396518. doi:10.1073/pnas.1117765109
Mustroph, J., Wagemann, O., Lücht, C. M., Trum, M., Hammer, K. P., Sag, C. M., et al. (2018). Empagliflozin Reduces Ca/calmodulin-dependent Kinase II Activity in Isolated Ventricular Cardiomyocytes. Esc. Heart Fail 5 (4), 642–648. PMCID: PMC6073019PMID: 30117720. doi:10.1002/ehf2.12336
Nasiri-Ansari, N., Nikolopoulou, C., Papoutsi, K., Kyrou, I., Mantzoros, C. S., Kyriakopoulos, G., et al. (2021). Empagliflozin Attenuates Non-alcoholic Fatty Liver Disease (NAFLD) in High Fat Diet Fed ApoE(-/-) Mice by Activating Autophagy and Reducing ER Stress and Apoptosis. Ijms 22 (2), 818. PMID: 33467546; PMCID: PMC7829901. doi:10.3390/ijms22020818
Neef, S., Dybkova, N., Sossalla, S., Ort, K. R., Fluschnik, N., Neumann, K., et al. (2010). CaMKII-dependent Diastolic SR Ca2+ Leak and Elevated Diastolic Ca2+ Levels in Right Atrial Myocardium of Patients with Atrial Fibrillation. Circ. Res. 106 (6), 1134–1144. Epub 2010 Jan 7. PMID: 20056922. doi:10.1161/CIRCRESAHA.109.203836
Oelze, M., Kröller-Schön, S., Welschof, P., Jansen, T., Hausding, M., Mikhed, Y., et al. (2014). The Sodium-Glucose Co-transporter 2 Inhibitor Empagliflozin Improves Diabetes-Induced Vascular Dysfunction in the Streptozotocin Diabetes Rat Model by Interfering with Oxidative Stress and Glucotoxicity. PLoS One 9 (11), e112394. PMID: 25402275; PMCID: PMC4234367. doi:10.1371/journal.pone.0112394
Oshima, H., Miki, T., Kuno, A., Mizuno, M., Sato, T., Tanno, M., et al. (2019). Empagliflozin, an SGLT2 Inhibitor, Reduced the Mortality Rate after Acute Myocardial Infarction with Modification of Cardiac Metabolomes and Antioxidants in Diabetic Rats. J. Pharmacol. Exp. Ther. 368 (3), 524–534. Epub 2018 Dec 14. PMID: 30552292. doi:10.1124/jpet.118.253666
Parzych, K. R., and Klionsky, D. J. (2014). An Overview of Autophagy: Morphology, Mechanism, and Regulation. Antioxid. Redox Signal 20 (3), 460–473. Epub 2013 Aug 2. PMID: 23725295; PMCID: PMC3894687. doi:10.1089/ars.2013.5371
Passino, C., Barison, A., Vergaro, G., Gabutti, A., Borrelli, C., Emdin, M., et al. (2015). Markers of Fibrosis, Inflammation, and Remodeling Pathways in Heart Failure. Clin. Chim. Acta 443, 29–38. Epub 2014 Sep 10. PMID: 25218738. doi:10.1016/j.cca.2014.09.006
Peng, K., Yang, L., Wang, J., Ye, F., Dan, G., Zhao, Y., et al. (2017). The Interaction of Mitochondrial Biogenesis and Fission/Fusion Mediated by PGC-1α Regulates Rotenone-Induced Dopaminergic Neurotoxicity. Mol. Neurobiol. 54 (5), 3783–3797. Epub 2016 Jun 7. PMID: 27271125. doi:10.1007/s12035-016-9944-9
Peretto, G., Sala, S., Rizzo, S., Palmisano, A., Esposito, A., De Cobelli, F., et al. (2020). Ventricular Arrhythmias in Myocarditis: Characterization and Relationships with Myocardial Inflammation. J. Am. Coll. Cardiol. 75 (9), 1046–1057. PMID: 32138965. doi:10.1016/j.jacc.2020.01.036
Pérez, M. J., and Quintanilla, R. A. (2017). Development or Disease: Duality of the Mitochondrial Permeability Transition Pore. Dev. Biol. 426 (1), 1–7. Epub 2017 Apr 28. PMID: 28457864. doi:10.1016/j.ydbio.2017.04.018
Peters, N. S., Coromilas, J., Severs, N. J., and Wit, A. L. (1997). Disturbed Connexin43 Gap Junction Distribution Correlates with the Location of Reentrant Circuits in the Epicardial Border Zone of Healing Canine Infarcts that Cause Ventricular Tachycardia. Circulation 95 (4), 988–996. PMID: 9054762. doi:10.1161/01.cir.95.4.988
Philippaert, K., Kalyaanamoorthy, S., Fatehi, M., Long, W., Soni, S., Byrne, N. J., et al. (2021). Cardiac Late Sodium Channel Current Is a Molecular Target for the Sodium/Glucose Cotransporter 2 Inhibitor Empagliflozin. Circulation 143 (22), 2188–2204. Epub 2021 Apr 9. PMID: 33832341; PMCID: PMC8154177. doi:10.1161/CIRCULATIONAHA.121.053350
Pool, L., Wijdeveld, L. F. J. M., de Groot, N. M. S., and Brundel, B. J. J. M. (2021). The Role of Mitochondrial Dysfunction in Atrial Fibrillation: Translation to Druggable Target and Biomarker Discovery. Int. J. Mol. Sci. 22 (16), 8463. PMID: 34445167; PMCID: PMC8395135. doi:10.3390/ijms22168463
Poulet, C., Wettwer, E., Grunnet, M., Jespersen, T., Fabritz, L., Matschke, K., et al. (2015). Late Sodium Current in Human Atrial Cardiomyocytes from Patients in Sinus Rhythm and Atrial Fibrillation. PLoS One 10 (6), e0131432. PMID: 26121051; PMCID: PMC4485891. doi:10.1371/journal.pone.0131432
Purohit, A., Rokita, A. G., Guan, X., Chen, B., Koval, O. M., Voigt, N., et al. (2013). Oxidized Ca(2+)/calmodulin-dependent Protein Kinase II Triggers Atrial Fibrillation. Circulation 128 (16), 1748–1757. Epub 2013 Sep 12. PMID: 24030498; PMCID: PMC3876034. doi:10.1161/CIRCULATIONAHA.113.003313
Quagliariello, V., De Laurentiis, M., Rea, D., Barbieri, A., Monti, M. G., Carbone, A., et al. (2021). The SGLT-2 Inhibitor Empagliflozin Improves Myocardial Strain, Reduces Cardiac Fibrosis and Pro-inflammatory Cytokines in Non-diabetic Mice Treated with Doxorubicin. Cardiovasc Diabetol. 20 (1), 150. PMID: 34301253; PMCID: PMC8305868. doi:10.1186/s12933-021-01346-y
Saadeh, K., Chadda, K. R., Ahmad, S., Valli, H., Nanthakumar, N., Fazmin, I. T., et al. (2021). Molecular Basis of Ventricular Arrhythmogenicity in a Pgc-1α Deficient Murine Model. Mol. Genet. Metabolism Rep. 27, 100753. PMID: 33898262; PMCID: PMC8059080. doi:10.1016/j.ymgmr.2021.100753
Saba, S., Janczewski, A. M., Baker, L. C., Shusterman, V., Gursoy, E. C., Feldman, A. M., et al. (2005). Atrial Contractile Dysfunction, Fibrosis, and Arrhythmias in a Mouse Model of Cardiomyopathy Secondary to Cardiac-specific Overexpression of Tumor Necrosis Factor-{alpha}. Am. J. Physiol. Heart Circ. Physiol. 289 (4), H1456–H1467. Epub 2005 May 27. PMID: 15923312. doi:10.1152/ajpheart.00733.2004
Sabatino, J., De Rosa, S., Tammè, L., Iaconetti, C., Sorrentino, S., Polimeni, A., et al. (2020). Empagliflozin Prevents Doxorubicin-Induced Myocardial Dysfunction. Cardiovasc Diabetol. 19 (1), 66. PMID: 32414364; PMCID: PMC7229599. doi:10.1186/s12933-020-01040-5
Sag, C. M., Wadsack, D. P., Khabbazzadeh, S., Abesser, M., Grefe, C., Neumann, K., et al. (2009). Calcium/calmodulin-dependent Protein Kinase II Contributes to Cardiac Arrhythmogenesis in Heart Failure. Circ. Heart Fail 2 (6), 664–675. Epub 2009 Jul 31. PMID: 19919992; PMCID: PMC2835502. doi:10.1161/CIRCHEARTFAILURE.109.865279
Salvarani, N., Maguy, A., De Simone, S. A., Miragoli, M., Jousset, F., and Rohr, S. (2017). TGF-β1 (Transforming Growth Factor-Β1) Plays a Pivotal Role in Cardiac Myofibroblast Arrhythmogenicity. Circ. Arrhythm. Electrophysiol. 10 (5), e004567. PMID: 28500173. doi:10.1161/CIRCEP.116.004567
Samuel, T. J., Rosenberry, R. P., Lee, S., and Pan, Z. (2018). Correcting Calcium Dysregulation in Chronic Heart Failure Using SERCA2a Gene Therapy. Int. J. Mol. Sci. 19 (4), 1086. PMID: 29621141; PMCID: PMC5979534. doi:10.3390/ijms19041086
Santos-Gallego, C. G., Requena-Ibanez, J. A., San Antonio, R., Garcia-Ropero, A., Ishikawa, K., Watanabe, S., et al. (2021). Empagliflozin Ameliorates Diastolic Dysfunction and Left Ventricular Fibrosis/Stiffness in Nondiabetic Heart Failure: A Multimodality Study. JACC Cardiovasc Imaging 14 (2), 393–407. Epub 2020 Oct 29. PMID: 33129742. doi:10.1016/j.jcmg.2020.07.042
Santos-Gallego, C. G., Requena-Ibanez, J. A., San Antonio, R., Ishikawa, K., Watanabe, S., Picatoste, B., et al. (2019). Empagliflozin Ameliorates Adverse Left Ventricular Remodeling in Nondiabetic Heart Failure by Enhancing Myocardial Energetics. J. Am. Coll. Cardiol. 73 (15), 1931–1944. PMID: 30999996. doi:10.1016/j.jacc.2019.01.056
Sato, T., Miki, T., Ohnishi, H., Yamashita, T., Takada, A., Yano, T., et al. (2017). Effect of Sodium-Glucose Co-transporter-2 Inhibitors on Impaired Ventricular Repolarization in People with Type 2 Diabetes. Diabet. Med. 34 (10), 1367–1371. Epub 2017 Aug 3. PMID: 28703863. doi:10.1111/dme.13424
Senoner, T., and Dichtl, W. (2019). Oxidative Stress in Cardiovascular Diseases: Still a Therapeutic Target? Nutrients 11 (9), 2090. PMID: 31487802; PMCID: PMC6769522. doi:10.3390/nu11092090
Shao, Q., Meng, L., Lee, S., Tse, G., Gong, M., Zhang, Z., et al. (2019). Empagliflozin, a Sodium Glucose Co-transporter-2 Inhibitor, Alleviates Atrial Remodeling and Improves Mitochondrial Function in High-Fat Diet/streptozotocin-Induced Diabetic Rats. Cardiovasc Diabetol. 18 (1), 165. PMID: 31779619; PMCID: PMC6882319. doi:10.1186/s12933-019-0964-4
Shentu, Y., Li, Y., Xie, S., Jiang, H., Sun, S., Lin, R., et al. (2021). Empagliflozin, a Sodium Glucose Cotransporter-2 Inhibitor, Ameliorates Peritoneal Fibrosis via Suppressing TGF-β/Smad Signaling. Int. Immunopharmacol. 93, 107374. Epub 2021 Jan 29. PMID: 33517222. doi:10.1016/j.intimp.2021.107374
Shi, B., Ma, M., Zheng, Y., Pan, Y., and Lin, X. (2019). mTOR and Beclin1: Two Key Autophagy-Related Molecules and Their Roles in Myocardial Ischemia/reperfusion Injury. J. Cell Physiol. 234 (8), 12562–12568. Epub 2019 Jan 7. PMID: 30618070. doi:10.1002/jcp.28125
Shih, J. Y., Lin, Y. W., Fisch, S., Cheng, J. T., Kang, N. W., Hong, C. S., et al. (2021). Dapagliflozin Suppresses ER Stress and Improves Subclinical Myocardial Function in Diabetes: From Bedside to Bench. Diabetes 70 (1), 262–267. Epub 2020 Oct 28. PMID: 33115828. doi:10.2337/db20-0840
Sohns, C., and Marrouche, N. F. (2020). Atrial Fibrillation and Cardiac Fibrosis. Eur. Heart J. 41 (10), 1123–1131. PMID: 31713590. doi:10.1093/eurheartj/ehz786
Song, Y., Shryock, J. C., and Belardinelli, L. (2008). An Increase of Late Sodium Current Induces Delayed Afterdepolarizations and Sustained Triggered Activity in Atrial Myocytes. Am. J. Physiol. Heart Circ. Physiol. 294 (5), H2031–H2039. Epub 2008 Feb 29. PMID: 18310511. doi:10.1152/ajpheart.01357.2007
Sossalla, S., Kallmeyer, B., Wagner, S., Mazur, M., Maurer, U., Toischer, K., et al. (2010). Altered Na(+) Currents in Atrial Fibrillation Effects of Ranolazine on Arrhythmias and Contractility in Human Atrial Myocardium. J. Am. Coll. Cardiol. 55 (21), 2330–2342. PMID: 20488304. doi:10.1016/j.jacc.2009.12.055
Sovari, A. A., and Dudley, S. C. (2012). Reactive Oxygen Species-Targeted Therapeutic Interventions for Atrial Fibrillation. Front. Physiol. 3, 311. PMID: 22934062; PMCID: PMC3429082. doi:10.3389/fphys.2012.00311
Sun, X., Han, F., Lu, Q., Li, X., Ren, D., Zhang, J., et al. (2020). Empagliflozin Ameliorates Obesity-Related Cardiac Dysfunction by Regulating Sestrin2-Mediated AMPK-mTOR Signaling and Redox Homeostasis in High-Fat Diet-Induced Obese Mice. Diabetes 69 (6), 1292–1305. Epub 2020 Mar 31. PMID: 32234722. doi:10.2337/db19-0991
Tamargo, J., Caballero, R., Gómez, R., Valenzuela, C., and Delpón, E. (2004). Pharmacology of Cardiac Potassium Channels. Cardiovasc Res. 62 (1), 9–33. PMID: 15023549. doi:10.1016/j.cardiores.2003.12.026
Tanajak, P., Sa-Nguanmoo, P., Sivasinprasasn, S., Thummasorn, S., Siri-Angkul, N., Chattipakorn, S. C., et al. (2018). Cardioprotection of Dapagliflozin and Vildagliptin in Rats with Cardiac Ischemia-Reperfusion Injury. J. Endocrinol. 236 (2), 69–84. Epub 2017 Nov 15. PMID: 29142025. doi:10.1530/JOE-17-0457
Tentolouris, A., Vlachakis, P., Tzeravini, E., Eleftheriadou, I., and Tentolouris, N. (2019). SGLT2 Inhibitors: A Review of Their Antidiabetic and Cardioprotective Effects. Int. J. Environ. Res. Public Health 16 (16), 2965. PMID: 31426529; PMCID: PMC6720282. doi:10.3390/ijerph16162965
Tian, J., Zhang, M., Suo, M., Liu, D., Wang, X., Liu, M., et al. (2021). Dapagliflozin Alleviates Cardiac Fibrosis through Suppressing EndMT and Fibroblast Activation via AMPKα/TGF-β/Smad Signalling in Type 2 Diabetic Rats. J. Cell Mol. Med. 25 (16), 7642–7659. Epub 2021 Jun 25. PMID: 34169635; PMCID: PMC8358881. doi:10.1111/jcmm.16601
Tisdale, J. E., Chung, M. K., Campbell, K. B., Hammadah, M., Joglar, J. A., Leclerc, J., et al. (2020). Drug-Induced Arrhythmias: A Scientific Statement from the American Heart Association. Circulation 142 (15), e214–e233. Epub 2020 Sep 15. PMID: 32929996. doi:10.1161/CIR.0000000000000905
Trum, M., Riechel, J., Lebek, S., Pabel, S., Sossalla, S. T., Hirt, S., et al. (2020). Empagliflozin Inhibits Na +/H + Exchanger Activity in Human Atrial Cardiomyocytes. Esc. Heart Fail. 7 (6), 4429–4437. Epub ahead of print. PMID: 32946200; PMCID: PMC7755005. doi:10.1002/ehf2.13024
Uthman, L., Baartscheer, A., Bleijlevens, B., Schumacher, C. A., Fiolet, J. W. T., Koeman, A., et al. (2018). Class Effects of SGLT2 Inhibitors in Mouse Cardiomyocytes and Hearts: Inhibition of Na+/H+ Exchanger, Lowering of Cytosolic Na+ and Vasodilation. Diabetologia 61 (3), 722–726. Epub 2017 Dec 2. PMID: 29197997; PMCID: PMC6448958. doi:10.1007/s00125-017-4509-7
Uthman, L., Nederlof, R., Eerbeek, O., Baartscheer, A., Schumacher, C., Buchholtz, N., et al. (2019). Delayed Ischaemic Contracture Onset by Empagliflozin Associates with NHE1 Inhibition and Is Dependent on Insulin in Isolated Mouse Hearts. Cardiovasc Res. 115 (10), 1533–1545. PMID: 30649212. doi:10.1093/cvr/cvz004
Voigt, N., Li, N., Wang, Q., Wang, W., Trafford, A. W., Abu-Taha, I., et al. (2012). Enhanced Sarcoplasmic Reticulum Ca 2+ Leak and Increased Na + -Ca 2+ Exchanger Function Underlie Delayed Afterdepolarizations in Patients with Chronic Atrial Fibrillation. Circulation 125 (17), 2059–2070. Epub 2012 Mar 28. PMID: 22456474; PMCID: PMC4663993. doi:10.1161/CIRCULATIONAHA.111.067306
Volk, T., Nguyen, T. H., Schultz, J. H., Faulhaber, J., and Ehmke, H. (2001). Regional Alterations of Repolarizing K+ Currents Among the Left Ventricular Free Wall of Rats with Ascending Aortic Stenosis. J. Physiol. 530 (Pt 3), 443–455. PMID: 11158275; PMCID: PMC2278429. doi:10.1111/j.1469-7793.2001.0443k.x
Wang, K., Li, Z., Sun, Y., Liu, X., Ma, W., Ding, Y., et al. (2021). Dapagliflozin Improves Cardiac Function, Remodeling, Myocardial Apoptosis, and Inflammatory Cytokines in Mice with Myocardial Infarction. J. Cardiovasc. Trans. Res. Epub ahead of print. PMID: 34855147. doi:10.1007/s12265-021-10192-y
Wang, Q., Yu, X., Dou, L., Huang, X., Zhu, K., Guo, J., et al. (2019). miR-154-5p Functions as an Important Regulator of Angiotensin II-Mediated Heart Remodeling. Oxid. Med. Cell Longev. 2019, 8768164. PMID: 31612078; PMCID: PMC6757276. doi:10.1155/2019/8768164
Wiersma, M., Meijering, R. A. M., Qi, X. Y., Zhang, D., Liu, T., Hoogstra-Berends, F., et al. (2017). Endoplasmic Reticulum Stress Is Associated with Autophagy and Cardiomyocyte Remodeling in Experimental and Human Atrial Fibrillation. J. Am. Heart Assoc. 6 (10), e006458. PMID: 29066441; PMCID: PMC5721854. doi:10.1161/JAHA.117.006458
Wijffels, M. C., Kirchhof, C. J., Dorland, R., and Allessie, M. A. (1995). Atrial Fibrillation Begets Atrial Fibrillation. A Study in Awake Chronically Instrumented Goats. Circulation 92 (7), 1954–1968. PMID: 7671380. doi:10.1161/01.cir.92.7.1954
Withaar, C., Meems, L. M. G., Markousis-Mavrogenis, G., Boogerd, C. J., Silljé, H. H. W., Schouten, E. M., et al. (2021). The Effects of Liraglutide and Dapagliflozin on Cardiac Function and Structure in a Multi-Hit Mouse Model of Heart Failure with Preserved Ejection Fraction. Cardiovasc Res. 117 (9), 2108–2124. PMID: 32871009; PMCID: PMC8318109. doi:10.1093/cvr/cvaa256
Wiviott, S. D., Raz, I., Bonaca, M. P., Mosenzon, O., Kato, E. T., Cahn, A., et al. (2019). Dapagliflozin and Cardiovascular Outcomes in Type 2 Diabetes. N. Engl. J. Med. 380 (4), 347–357. Epub 2018 Nov 10. PMID: 30415602. doi:10.1056/NEJMoa1812389
Wu, L., Rajamani, S., Li, H., January, C. T., Shryock, J. C., and Belardinelli, L. (2009). Reduction of Repolarization Reserve Unmasks the Proarrhythmic Role of Endogenous Late Na(+) Current in the Heart. Am. J. Physiol. Heart Circ. Physiol. 297 (3), H1048–H1057. Epub 2009 Jul 10. PMID: 19592609. doi:10.1152/ajpheart.00467.2009
Xu, H. D., and Qin, Z. H. (2019). Beclin 1, Bcl-2 and Autophagy. Adv. Exp. Med. Biol. 1206, 109–126. PMID: 31776982. doi:10.1007/978-981-15-0602-4_5
Ye, Y., Bajaj, M., Yang, H. C., Perez-Polo, J. R., and Birnbaum, Y. (2017). SGLT-2 Inhibition with Dapagliflozin Reduces the Activation of the Nlrp3/ASC Inflammasome and Attenuates the Development of Diabetic Cardiomyopathy in Mice with Type 2 Diabetes. Further Augmentation of the Effects with Saxagliptin, a DPP4 Inhibitor. Cardiovasc Drugs Ther. 31 (2), 119–132. PMID: 28447181. doi:10.1007/s10557-017-6725-2
Ye, Y., Jia, X., Bajaj, M., and Birnbaum, Y. (2018). Dapagliflozin Attenuates Na+/H+ Exchanger-1 in Cardiofibroblasts via AMPK Activation. Cardiovasc Drugs Ther. 32 (6), 553–558. PMID: 30367338. doi:10.1007/s10557-018-6837-3
Yue, L., Melnyk, P., Gaspo, R., Wang, Z., and Nattel, S. (1999). Molecular Mechanisms Underlying Ionic Remodeling in a Dog Model of Atrial Fibrillation. Circ. Res. 84 (7), 776–784. PMID: 10205145. doi:10.1161/01.res.84.7.776
Zaman, J. A., Harling, L., Ashrafian, H., Darzi, A., Gooderham, N., Athanasiou, T., et al. (2016). Post-operative Atrial Fibrillation Is Associated with a Pre-existing Structural and Electrical Substrate in Human Right Atrial Myocardium. Int. J. Cardiol. 220, 580–588. Epub 2016 Jun 28. PMID: 27390994; PMCID: PMC4994770. doi:10.1016/j.ijcard.2016.06.249
Zannad, F., Ferreira, J. P., Pocock, S. J., Anker, S. D., Butler, J., Filippatos, G., et al. (2020). SGLT2 Inhibitors in Patients with Heart Failure with Reduced Ejection Fraction: a Meta-Analysis of the EMPEROR-Reduced and DAPA-HF Trials. Lancet 396 (10254), 819–829. Epub 2020 Aug 30. PMID: 32877652. doi:10.1016/S0140-6736(20)31824-9
Zaza, A., Belardinelli, L., and Shryock, J. C. (2008). Pathophysiology and Pharmacology of the Cardiac "late Sodium current.". Pharmacol. Ther. 119 (3), 326–339. Epub 2008 Jul 1. PMID: 18662720. doi:10.1016/j.pharmthera.2008.06.001
Zelniker, T. A., Bonaca, M. P., Furtado, R. H. M., Mosenzon, O., Kuder, J. F., Murphy, S. A., et al. (2020). Effect of Dapagliflozin on Atrial Fibrillation in Patients with Type 2 Diabetes Mellitus: Insights from the DECLARE-TIMI 58 Trial. Circulation 141 (15), 1227–1234. Epub 2020 Jan 27. PMID: 31983236. doi:10.1161/CIRCULATIONAHA.119.044183
Zelniker, T. A., and Braunwald, E. (2020). Mechanisms of Cardiorenal Effects of Sodium-Glucose Cotransporter 2 Inhibitors: JACC State-Of-The-Art Review. J. Am. Coll. Cardiol. 75 (4), 422–434. Erratum in: J Am Coll Cardiol. 2020 Sep 22; 76(12):1505. PMID: 32000955. doi:10.1016/j.jacc.2019.11.031
Zelniker, T. A., Wiviott, S. D., Raz, I., Im, K., Goodrich, E. L., Bonaca, M. P., et al. (2019). SGLT2 Inhibitors for Primary and Secondary Prevention of Cardiovascular and Renal Outcomes in Type 2 Diabetes: a Systematic Review and Meta-Analysis of Cardiovascular Outcome Trials. Lancet 393 (10166), 31–39. Epub 2018 Nov 10. Erratum in: Lancet. 2019 Jan 5; 393(10166):30. PMID: 30424892. doi:10.1016/S0140-6736(18)32590-X
Zelniker, T. A., Wiviott, S. D., Raz, I., Im, K., Goodrich, E. L., Furtado, R. H. M., et al. (2019). Comparison of the Effects of Glucagon-like Peptide Receptor Agonists and Sodium-Glucose Cotransporter 2 Inhibitors for Prevention of Major Adverse Cardiovascular and Renal Outcomes in Type 2 Diabetes Mellitus. Circulation 139 (17), 2022–2031. PMID: 30786725. doi:10.1161/CIRCULATIONAHA.118.038868
Zhai, X., Qiao, X., Zhang, L., Wang, D., Zhang, L., Feng, Q., et al. (2019). Ik1 Channel Agonist Zacopride Suppresses Ventricular Arrhythmias in Conscious Rats with Healing Myocardial Infarction. Life Sci. 239, 117075. Epub 2019 Nov 18. PMID: 31751587. doi:10.1016/j.lfs.2019.117075
Zhai, X. W., Zhang, L., Guo, Y. F., Yang, Y., Wang, D. M., Zhang, Y., et al. (2017). The Ik1/Kir2.1 Channel Agonist Zacopride Prevents and Cures Acute Ischemic Arrhythmias in the Rat. PLoS One 12 (5), e0177600. PMID: 28542320; PMCID: PMC5436763. doi:10.1371/journal.pone.0177600
Zhang, J., Wang, L. L., Du, W., Yu, Y. C., Ju, W. Z., Man, Y. L., et al. (2014). Hepatocyte Growth Factor Modification Enhances the Anti-arrhythmic Properties of Human Bone Marrow-Derived Mesenchymal Stem Cells. PLoS One 9 (10), e111246. PMID: 25360679; PMCID: PMC4216066. doi:10.1371/journal.pone.0111246
Zhang, Q. Q., Li, G. Q., Zhong, Y., Wang, J., Wang, A. N., Zhou, X., et al. (2020). Empagliflozin Improves Chronic Hypercortisolism-Induced Abnormal Myocardial Structure and Cardiac Function in Mice. Ther. Adv. Chronic Dis. 11, 2040622320974833. PMID: 33294147; PMCID: PMC7705186. doi:10.1177/2040622320974833
Zhang, Y., Jiao, L., Sun, L., Li, Y., Gao, Y., Xu, C., et al. (2018). LncRNA ZFAS1 as a SERCA2a Inhibitor to Cause Intracellular Ca2+ Overload and Contractile Dysfunction in a Mouse Model of Myocardial Infarction. Circ. Res. 122 (10), 1354–1368. Epub 2018 Feb 23. PMID: 29475982; PMCID: PMC5959220. doi:10.1161/CIRCRESAHA.117.312117
Zhang, Y., Lin, X., Chu, Y., Chen, X., Du, H., Zhang, H., et al. (2021). Dapagliflozin: a Sodium-Glucose Cotransporter 2 Inhibitor, Attenuates Angiotensin II-Induced Cardiac Fibrotic Remodeling by Regulating TGFβ1/Smad Signaling. Cardiovasc Diabetol. 20 (1), 121. PMID: 34116674; PMCID: PMC8196449. doi:10.1186/s12933-021-01312-8
Zhang, Y., Wang, H. M., Wang, Y. Z., Zhang, Y. Y., Jin, X. X., Zhao, Y., et al. (2017). Increment of Late Sodium Currents in the Left Atrial Myocytes and its Potential Contribution to Increased Susceptibility of Atrial Fibrillation in Castrated Male Mice. Heart rhythm. 14 (7), 1073–1080. Epub 2017 Feb 6. PMID: 28185917. doi:10.1016/j.hrthm.2017.01.046
Zhao, C., and Zhao, W. (2020). NLRP3 Inflammasome-A Key Player in Antiviral Responses. Front. Immunol. 11, 211. PMID: 32133002; PMCID: PMC7040071. doi:10.3389/fimmu.2020.00211
Zhou, S., Dai, W., Zhong, G., and Jiang, Z. (2020). Impaired Mitophagy: A New Potential Mechanism of Human Chronic Atrial Fibrillation. Cardiol. Res. Pract. 2020, 6757350. PMCID: PMC7547333PMID: 33062320. doi:10.1155/2020/6757350
Zhou, Y., Tong, Z., Jiang, S., Zheng, W., Zhao, J., and Zhou, X. (2020). The Roles of Endoplasmic Reticulum in NLRP3 Inflammasome Activation. Cells 9 (5), 1219. PMID: 32423023; PMCID: PMC7291288. doi:10.3390/cells9051219
Zorov, D. B., Filburn, C. R., Klotz, L. O., Zweier, J. L., and Sollott, S. J. (2000). Reactive Oxygen Species (ROS)-induced ROS Release: a New Phenomenon Accompanying Induction of the Mitochondrial Permeability Transition in Cardiac Myocytes. J. Exp. Med. 192 (7), 1001–1014. PMID: 11015441; PMCID: PMC2193314. doi:10.1084/jem.192.7.1001
Keywords: arrhythmia, SGLT2I, cardiac electrical remodeling, mitochondrial dysfunction, oxidative stress, cardiac structural remodeling, inflammation
Citation: Jing Y, Yang R, Chen W and Ye Q (2022) Anti-Arrhythmic Effects of Sodium-Glucose Co-Transporter 2 Inhibitors. Front. Pharmacol. 13:898718. doi: 10.3389/fphar.2022.898718
Received: 17 March 2022; Accepted: 04 May 2022;
Published: 24 June 2022.
Edited by:
Annalisa Capuano, University of Campania Luigi Vanvitelli, ItalyReviewed by:
Elisabetta Cerbai, University of Florence, ItalyOscar Casis, Universidad del País Vasco UPV/EHU, Spain
Copyright © 2022 Jing, Yang, Chen and Ye. This is an open-access article distributed under the terms of the Creative Commons Attribution License (CC BY). The use, distribution or reproduction in other forums is permitted, provided the original author(s) and the copyright owner(s) are credited and that the original publication in this journal is cited, in accordance with accepted academic practice. No use, distribution or reproduction is permitted which does not comply with these terms.
*Correspondence: Qiang Ye, YXJ0MDA2MDIzQHllYWgubmV0