- 1Department of Chemistry, Kulliyyah of Science, International Islamic University Malaysia, Kuantan, Malaysia
- 2Department of Pharmaceutical Technology, Kulliyyah of Pharmacy, International Islamic University Malaysia, Kuantan, Malaysia
- 3IKOP Pharma Sdn Bhd, Jalan Sultan Ahmad Shah, Kuantan, Malaysia
- 4Pharmaceutics and Translational Research Group, Kulliyyah of Pharmacy, International Islamic University Malaysia, Kuantan, Malaysia
- 5Department of Pharmacy Practice, Faculty of Pharmacy, Airlangga University, Surabaya, Indonesia
Natural products offer a wide range of bioactivity including antimicrobial properties. There are many reports showing the antimicrobial activities of phytochem icals from plants. However, the bioactivity is limited due to multidrug resistant properties of the microorganism and different composition of cell membrane. The antibacterial activity of the natural products is different toward Gram-negative and Gram-positive bacteria. These phenomena are caused by improper physicochemical conditions of the substance which hinder the phytochemical bioactivity against the broad range of bacteria. One of the strategies to improve the antimicrobial action is by biogenic synthesis via redox balance of the antimicrobial active substance with metal to form nanosized materials or nanoparticles (NPs). Antibiotic resistance is not relevant to NPs because the action of NPs is via direct contact with bacterial cell walls without the need of penetration into microbial cells. The NPs that have shown their effectiveness in preventing or overcoming biofilm formation such as silver-based nanoparticles (AgNPs), gold-based nanoparticles (AuNPs), platinum-based nanoparticles (PtNPs) and Zinc oxide-based nanoparticles (ZnONPs). Due to its considerably simple synthesis procedure has encouraged researchers to explore antimicrobial potency of metallic nanoparticles. Those metallic nanoparticles remarkably express synergistic effects against the microorganisms tested by affecting bacterial redox balance, thus disrupting their homeostasis. In this paper, we discuss the type of metallic nanoparticle which have been used to improve the antimicrobial activity of plant extract/constituents, preparation or synthesis process and characterisation of the plant-based metallic nanoparticles.
Introduction
Antimicrobial resistance occurs when the pathogenic microorganisms survive upon exposure to a drug that would normally kill them and allows them to continue the infection (Oneil, 2016). The cases of antimicrobial resistance increase every year and estimate 10 million deaths every year (Oneil, 2016). Some bacteria are known to develop resistant such as methicillin-resistant Staphylococcus aureus, vancomycin resistant Enterococci, Pseudomonas aeruginosa and Klebsiella pneumonia as well as Clostridium difficile (Khan et al., 2015).
Antimicrobial resistance could be developed at DNA and protein levels (Wang et al., 2017). Antimicrobial resistance happens due to microbe’s interaction with their environment. Naturally, they will be able to develop intrinsic resistance to one or more antimicrobials. Whereas, in clinical setting, the resistance refers to acquired resistance in the microbial population that was previously susceptible to antimicrobial agents (Munita and Arias, 2016).
Antimicrobial from natural products have inspired antibiotic discovery and have been used for microbial control. These include plant extracts, small antimicrobial peptides, essential oils, bacteriocins and various groups of compounds (Stan et al., 2021). There are several reports showing the antimicrobial activity of free compounds isolated from natural sources. Natural products such as tannins are good substances to control microbial growth by interact with bacterial proteins and precipitating them (Puljula et al., 2020). Chalcone derivative from Croton anisodontus Mull. Arg. acts as a competitive inhibitor Mep A efflux pump and potentiates ciprofloxacin’s action against multidrug-resistant Staphylococcus aureus (Xavier 2020). Betulinic acid was reported to have string inhibition against Candida albicans (Lashin et al., 2021). Nimbolide from Azadiracta indica A. Juss possesses significant bactericidal activity against Helicobacter pylori by killing free living bacteria and cells within biofilm (Wylie et al., 2022). The following table (Table 1) presents selected antimicrobial plants which were obtained from database using the keywords of “plant” and “antimicrobial activity”.
Role of NPs in Biofilm Formation
NPs has been recorded as an effective method in combating microbial resistance and multidrug-resistance mutant (Wang et al., 2017) especially on the interaction with microbial biofilm.
Bacterial biofilms are complex surface on the communities of bacteria that consist of microorganism culture, extracellular polymer matrix (EPM), complex biopolysaccharide, secreted protein proteins and extracellular DNA (Muhammad et al., 2020; Shkodenko et al., 2020).
The role of NPs in antimicrobial activity including the disruption of bacterial membranes and interaction with biofilm (Shkodenko et al., 2020). The interaction between NPs and biofilm could occur in following step: transfer of NPs to the biofilm; attachment to the surface and migration into biofilms (Figure 1). The process is affected by physicochemical properties of NPs, the environment and extracellular polymeric matric (Shkodenko et al., 2020).
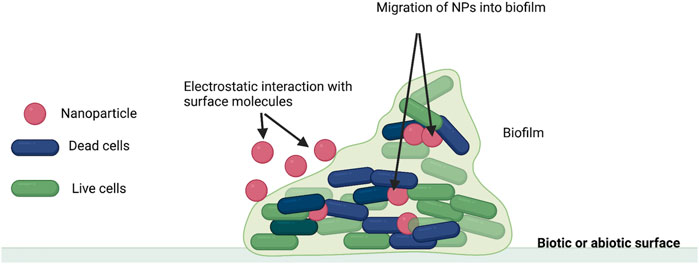
FIGURE 1. Interaction between biofilm and NPs (Adapted from Shkodenko et al., 2020).
Nanoparticle in Drug Delivery
New approach to combat resistant microorganisms is urgently required to reduce the clinical burden in the use of antibiotics. Nanotechnology-based antimicrobials could be one of strategies which is acting synergistically via intrinsically antimicrobials and those as carriers (Makvandi et al., 2020). Nanotechnology is emerging field of science which deals with the synthesis of nanoparticles in improving physicochemical properties of materials for human benefits (Chau et al., 2022). The success research in nanoparticle-based delivery is due to their advantages such as safety assessment, scalable production, and availability for clinical trials (Hagbani et al., 2022).
The nanoparticles fall between 1 and 100 nm size which can be prepared by top-down methods include mechanical milling, laser ablation, etching, sputtering, and electro-explosion and bottom-up approaches such as chemical vapor deposition, solvothermal and hydrothermal methods, sol–gel method, soft and hard templating methods, reverse micelle methods (Baig et al., 2021). Nanoscale delivery has gained interest due to their exceptional activity allowing reaction to be achieved effectively and efficiently (Baig et al., 2021). Metal-based nanomaterials have been continuously investigated for their application due to their potential usage. Metal nanoparticles (MNPs) display potential capacity and higher surface area making them suitable in numerous applications including medicine, drug delivery, cosmetics, food product, sensors, optics, electronics, paints and agriculture (Perveen et al., 2022).
The metal-based nanocarriers have been reported to increase the antimicrobial activity (Makvandi et al., 2020) in addition to their own activity (Yonathan et al., 2022). The use of noble (magnetic) metal nanoparticles (MNPS) especially gold (Au), silver (Ag) and platinum (Pt) have been attracting great attention due to its multiple antibacterial properties and simple synthesis methods (Shafey, 2020). They act selectively attached to a functional molecule and allow transportation to targeted locations under magnetic fields (Ahmad et al., 2022). The noble metal particle can kill bacteria and eradicate biofilms by cell membrane potential stability disruption as well as binding bacterial enzymes or DNA and (Ye et al., 2022). MNPs can also act as free radical damaging bacterial cell membrane (Ye et al., 2022). Meanwhile, the antibacterial agents show similar mechanisms with one or multiple targets on bacterial protein synthesis, bacterial cell wall synthesis, bacterial cell membrane destruction, bacterial DNA replication and repair as well as inhibition of metabolite pathway (Khameneh et al., 2019). When the antimicrobial agent is encapsulated with metal-nanoparticles, they would have a synergistic effect therefore the toxicity level would decrease and the efficacy would increase.
MNPs in Antibiotic Potency Enhancement
Metal nanoparticles are becoming a trend strategy to improve antibiotic activity due to its simple synthesis. Antibacterial activity of the antibiotic is different against bacterial gram stain, due to organization of the structures outside the plasma membrane but below the capsule where in Gram-negative organisms these structures constitute the cell envelope, whereas in Gram-positive organisms they are called a cell wall (Salton and Kim, 1996). The silver nanoparticles alone excellently discompose the polymer sub-units of the cell membrane in micro-organisms. In combination with plant material which is mediated silver nanoparticles consequently rupture the cell membrane and destroy the protein synthesis mechanism in the bacteria (Bukhari et al., 2021).
Study on antibiotic colistin on anionic gold nanoparticle against Escherichia coli reduces minimum inhibition of 6-folds compared to colistin alone (Fuller et al., 2020). A gold nanoparticle of vancomycin has shown more effective inhibition towards Gram-negative bacteria of E. coli, Klebsiella oxytoca and Pseudomonas aeruginosa with 1.4, 1.6 and 1.8 folds inhibition and towards Gram-positive bacteria, Staphylococcus aureus with 1.6 fold compared to free vancomycin (Hagbani et al., 2022). Combinatorial silver nanopacked Daptomycin inherits synergistic effects of both for their bactericidal activity (Zheng et al., 2016).
Plant Extract Encapsulated With MNPs
Gold Nanoparticle in Antimicrobial Delivery of Natural Products
Gold nanoparticles (AuNPs) is an important class of nanoparticles. They have been widely used in medical and non-medical applications as an ideal material for a variety of purposes. Inertness, biocompatibility, and, most importantly, low toxicity. Au-NPs have been shown to have biological activity and have been studied using a variety of important Au (III) properties that aid in Au-NPs synthesised from a variety of materials. Au-NPs are adaptive, resourceful, and can be designed to execute a wide range of tasks, making them a viable alternative to Au. In comparison to other NPs such as Ag, Au-NPs are more effective at attacking a variety of bacteria and viruses (Aljarba et al., 2022).
While Au is widely used as a nontoxic nanomaterial, the materials used to prepare and modify Au-NPs may be toxic (Guliani et al., 2021). While the presence of Au-NPs in high concentrations may indicate toxicity, there are many studies showing that Au-NPs do appear to possess antibacterial properties. At certain concentrations, it has been demonstrated that these NPs are non-toxic to normal cells (Guliani et al., 2021). Not only do modified Au-NPs have great antimicrobial abilities against standard strains of bacteria, but they also have a unique ability to fight cancer (Aljarba et al., 2022). Au (III) that is attached to different drug systems has been shown to make them more effective at killing bacteria. A lot of different bacteria, like M. luteus, E. coli, S. aureus, and P. aeruginosa can be killed by aminoglycosides that are coated with AuNPs (Bera and Mondal, 2022).
Antimicrobial agents are used to keep moulds, bacteria, and fungi populations under control. To prevent infection, these substances prevent bacteria from multiplying on various surfaces. Au and Ag are two examples of antimicrobial agents that contain active metal ingredients. Numerous studies have established the antimicrobial effect of gold nanoparticles (Hammami et al., 2021). Due to the fact that NPs’ antimicrobial activity is inversely proportional to their size, the smaller the size of Au-NPs, the higher their antimicrobial activity against a variety of microbes such as K. pneumonia, S. aureus, P. vulgaris, E. coli, and B. subtilis. Thangamani and Bhuvaneshwari investigated these synthesised Au-NPs’ antimicrobial activity against the aforementioned microbes (Thangamani and Bhuvaneshwari, 2019). In addition, antibacterial activity of NPs has been investigated against a variety of microbes, including E. coli, E. faecalis, S. typhimurium, P. aeruginosa, Vibrio damsel, Vibrio fluvialis and Candida albicans (Sunderam et al., 2019). The biological activity of Au-NPs synthesised via biogenic synthesis using the endophytic fungus Cladosporium cladosporioides derived from seaweed Sargassum wightii was evaluated against S. aureus, E. coli, P. aeruginosa and B. subtilis. Among the reported microbes, these AuNPs portrayed the lowest activity towards B. subtilis and the greatest effect toward S. aureus. After 3 min, approximately half of the E. coli were died, while 80% were killed after 6 min. After 10 minutes of photoactivation, no bacterial stains were detected (Manjunath et al., 2017).
Formulating NPs from natural extracts containing biologically active pharmacophores is common. Rajathi and colleagues synthesised Au-NPs containing Stoechospermum marginatum and evaluated the antimicrobial activity against P. aeruginosa, Klebsiella oxytoca, Salmonella typhimurium, Enterobacter faecalis, Klebsiella pneumonia and Proteus vulgaris. They discovered that Klebsiella pneumonia was more susceptible to these AuNPs, among other microbes (Rajathi et al., 2012). Dual metallic nanoparticles were synthesised by combining Ag and Au in various ratios using a red alga called Gracilaria sp. These NPs were then tested for antimicrobial activity against a variety of bacteria, including Salmonella typhii, E. coli, S. aureus and K. pneumonia. These NPs have bactericidal effects on S. aureus and K. pneumonia (Ramakritinan et al., 2013). Additionally, Au-NPs from Morus alba L. (mulberry) leaf extract were also synthesised and tested against human pathogens. These Au-NPs successfully inhibited Vibrio cholera and S. aureus (Adavallan and Krishnakumar, 2014). Au-NPs were synthesised from HAuCl4 using a papaya leaf extract via a green synthetic technique. Investigating these NPs for biomedical applications revealed that they are effective against S. aureus and P. putida (Sunkari et al., 2017).
The Au-NPs from Elaeocarpus ganitrus Blume seed extract via a hydrothermal route were synthesised and their antimicrobial activity was observed against Proteus desmolyticum and S. aureus, in which both of the microbes were killed (Vinay et al., 2021). Another group of researchers synthesised Au-NPs from Mentha longifolia (L.) L. extract and demonstrated their efficacy in killing B. subtilis, K. pneumoniae and S. aureus strains (Rauf et al., 2021). Biological Au-NPs containing Jasminum auriculatum Vahl. leaf extract exhibited promising anti-bacterial activity against E. coli, S. aureus, Streptococcus pyogenes, and K. pneumonia) (Balasubramanian et al., 2020). Additionally, Au-NPs were synthesised from a mixture of Catharanthus roseus (L.) G. Don and Carica papaya L. leaf extracts and these NPs possessed antibacterial activity against E. coli, Bacillus subtilis, S. aureus and Proteus vulgaris (Muthukumar et al., 2016).
Antibiotic resistance has emerged as a major global issue, thus reducing the availability of effective antibiotics. As a result, novel antibiotics from various sources especially natural extracts with novel delivery are urgently required (Wang et al., 2017) The Au-NPs produced using H2O2 as a reduction enhancer and starch as a reducing agent exhibited significant bactericidal activity against S. aureus (Emam et al., 2017). Functionalised Au-NPs with arginine and hydroxyapatite portrayed antibacterial activity against three bacteria, S. aureus, P. aeruginosa and E. coli. Besides, it was discovered that the activity of these functionalized Au-NPs was similar to that of Au-NPs, but with a stronger inhibitory effect towards P. aeruginosa strain (Kurtjak et al., 2017).
P. aeruginosa is believed to be the most resistant bacteria due to its mutational capability, demonstrating a high degree of resistance to antibiotics. The Au-NPs were formulated with curcumin extracted from Curcuma pseudomontana J. Graham and hematite (a-Fe2O3). It was reported that these NPs showed antimicrobial action against P. aeruginosa, S. aureus and B. subtilis with a higher degree of antimicrobial effect toward E. coli (Muniyappan et al., 2021). Lists of the Au-NPs containing natural extracts and demonstrates antibacterial activity is presented in Table 2.
Silver and Other Nanoparticles in Antimicrobial Delivery of Natural Products
Silver nanoparticles (AgNPs) showed remarkable biological and physicochemical properties. Silver ions demonstrated antimicrobial activity. The use of AgNPs in encapsulating natural products material will enhance their antimicrobial activity via DNA activity (Ahmad et al., 2022).
Euphorbia serpens Kunth synthetized using green synthesis AgNPs showed significant antibacterial effect against against E. coli with 20 ± 06 mm and S. typhi with 18 ± 0:5 mm zone of inhibition compared to standard antibiotic amoxicillin with 23 ± 0:3 mm and 20 ± 0:4 mm zone of inhibition (Ahmad et al., 2022). Trigonella foenum-graecum-AgNPs was reported to have antimicrobial activity against pathogenic fungi in plant Alternaria alternata and pathogenic bacteria in plant Pseudomonas syringae (Khan et al., 2019).
Biogenic synthesis of Persicaria odorata (Lour.) Sojak silver nanoparticles corroborated improve the bactericidal activity by dose-dependent inhibition against S. epidermis and Methicillin resistant S. aureus (Lubis et al., 2022). A comparison study of antibacterial activity between AgNPS and Ag zeolite A (ZA) showed that AgNPS against E. coli ATCC 11229 and S. aureus ATCC found that the inhibition of AgNPs is higher than AgZA (Asraf et al., 2022). AgNPs of Mentha piperita L. increased the antibacterial activity of the extracts in inhibiting the growth of both S. aureus and P. aeruginosa (Mojally et al., 2022). The synergistic effect of ZnO nanoparticle with plant extract was shown by Agle marmelos (L.) Correa (Am-ZnO) which shown greater antibacterial and antibiofilm effects against Gram-negative bacteria when compared to Gram-positive bacteria (Senthamarai and Malaikozhundan, 2022). Interestingly the Sambucus ebulus L.-AgNPs able to inhibit Gram-negative bacteria as reported by S. aureus with MIC value of 1.5 mg/ml. (Hashemi et al., 2022a). Table 3 displays the silver and other metal nanoparticles which were recently reported.
Green Synthesis MNPs
Several methods have been introduced to synthesise metal NPs including chemical, physical and biological methods using microwave-assisted, radiation-assisted, thermal decomposition, chemical and photo-chemical reaction, and green synthesis methods. Synthesis using bio-organisms or green synthesis compatible with the green chemistry principle. And green synthesis is required to avoid the production of unwanted or harmful by-products through the build-up of reliable, sustainable, and eco-friendly synthesis procedures. To achieve the goal of green synthesis, the natural resources and the ideal solvent systems that are environmentally friendly, non-toxic and safe reagent are essential (Singh et al., 2018).
Green synthesis of MNPs synthesised using biological techniques or green technology have diverse natures, with greater stability and appropriate dimension since they are synthesised using a one-step procedure. Therefore, the green synthesis of MNPs has been adopted to accommodate various biological materials, such as viruses, bacteria, fungi, algae, yeast, plant and plant products. Many researchers prefer to use green synthesis due to some advantages including cost effectiveness because the biological component itself acts as reducing and capping agents, eco-friendly approach as toxic chemicals are not used, and external experimental conditions like high energy and high pressure are not required that lead to energy saving process. Furthermore, the synthesis of a wide range of MNPs has been reported using various plants because it is a simple and easy process to produce MNPs at large scale compared to bacteria and/or fungi mediated synthesis (Song and Kim, 2009; Alarjani et al., 2022; Ye et al., 2022).
Plant mediated synthesis of MNPs is a revolutionary technique that has a wide range of applications in medicine, agriculture and food industries that offer an advantage which increases the life span of NPs. This is due to the physico-chemical properties of the plants based MNPs that overcome the limitation of conventional chemical and physical methods of NPs synthesis (Malik et al., 2014; Kalpana and Devi Rajeswari, 2018). Plant mediated synthesis of MNPs can be achieved by three different methods, intracellularly (inside the plant), extracellularly (using plant extracts), and using individual phytochemicals. Plants can mediate the synthesis of MNPs intracellularly because plants have the capability to accumulate the metals and transform these accumulated metals to NPs intracellularly. Moreover, the presence of several biomolecules such as protein, amino acids, carbohydrates, reducing sugar, vitamins, alkaloids, aldehydes, flavones, ketones, phenolics, saponins, tannins, and terpenoids in the plant plays a key role in the reduction of metals. These biomolecules contain hydroxyl, carbonyl and amine functional groups that react with metal ions and reduce their size into nano size. For example, flavonoids contain several functional groups, and it is believed that the -OH group of flavonoids is responsible for the reduction of metal ions into NPs (Marslin et al., 2018; Dikshit et al., 2021). Flavonoids not only help in bio-reduction of the metal ion to the nano size, but also play an important role in the capping of the NPs which is important for the stability and biocompatibility (Javed et al., 2020). In addition, reducing agents such as phenolics, sterols and alkaloids can reduce the metal ions into NPs in a single reaction (Biswas et al., 2013).
The type and nature of the metal used for MNPs biosynthesis mainly determine the NPs end use industry. Several metal such as silver (Ag), copper (Cu), gold (Au) and many others have been widely used for the biosynthesis of NPs using plant extracts of various plant species (Zhang et al., 2020). Au and Ag were the first metal ion used in plant extract-mediated synthesis of NPs. Acalypha indica, Aloe barbadensis, Datura metel, Nelumbo nucifera, Jatropha curcas, Ocimum leaf, Cassia auriculata, and Rhododedendron dauricam have been used to produce Ag nanoparticles. Gold nanoparticles have also been synthesized using Aloe barbadensis Miller, Medicago sativa, Magnolia kobus, Diopyros kaki, Cinnamomum camphora, and Pelargonium graveolens leaf (Jeevanandam et al., 2016).
Peralta-Videa et al., 2016 reported the synthesis of plant based metallic nanoparticles and their potential application. Metals from their constituents are reduced and stabilised by macromolecules and phytonutrients, such as proteins, reducing sugars, phenolics, flavonoids, ethyl alcohols, terpenes, and phenolic acids present in plant extracts. (Naseer et al., 2020) split the function of biomolecules in the formation of NPs as redoxed intermediaries for metals reductions and capped agents for non-agglomeration and post-surface modification of NPs.
In general, the synthesis of MNPs is initiated by mixing the plant biomass/extract with of noble metal salt precursors with biomaterial at a desired temperature and pH. The presence of various compounds of biomolecules act as reducing and capping agents for the synthesis of NPs from its metal salt precursors. The reduction of metal salt precursors to its successive NPs can be initially confirmed by visualising the colour change of the colloidal solution (Kuppusamy et al., 2016; Sriramulu et al., 2020).
The variation in the size, shape, and properties of accumulated NPs can be observed due to the variation in stabilising and reducing potential of biomolecules present in the plant. For example, the formation of gold NPs inside the living plant, alfalfa was reported by (Gardea-Torresdey et al., 2002) when the plants were grown in AuCl4 rich environment. And in a similar kind of study, (Bali and Harris, 2010) observed the ability of Medicago sativa and Brassica juncea plants to accumulate Au NPs from aqueous solutions of KAuCl4. The NPs were majorly located in the xylem parenchyma cells while some were also accumulated throughout the epidermis, cortex, and vascular tissue. Several studies reported the synthesis of Ag, Au, Cu, Pt, Cd, Pt, Pd, Ru, Rh, etc. using various biological agents in the recent past. The experimental procedure for the synthesis of NPs using plant biomass is depicted in Figure 2.
Unique Antibacterial Mechanism of MNPs
The mechanism of action of MNPs on bacterial cells is affected by the physiochemical properties of NPs such as charge, size, zeta potential, surface morphology and crystal structure. Whereas, the environmental factor, exposure time and bacterial strains are also influence the antibacterial effect of MNPs (Wang et al., 2017). There are three well known antimicrobial effects of NPs includes electrostatic interaction causing mechanical damage of cell walls, generation of reactive oxygen species resulting oxidative stress and disruption of cell structures and protein structure as a result of metal release (Wang et al., 2017).
Despite several approaches that have been made over the years, the precise mechanism of action of MNPs as antimicrobial agents is still not fully understood. Antimicrobial action of MNPs is linked to four main mechanisms, attraction to bacterial surface, destabilisation of bacterial or fungal cell wall and/or membrane with change in its permeability, induction of toxicity and oxidative stress by generation of reactive oxygen species (ROS) and free radical, and modulation of signal transduction pathways (Dakal et al., 2016). The adhesion of MNPs onto the surface of bacteria is the first step of a complex mechanism of bacterial inhibition. The adhesion of MNPs is influenced highly by their size and also zeta potential that depends on the method for their synthesis that might have a positive, negative or neutral surface charge. Varying surface charge of MNPs will cause the fluctuation of the antibacterial activity. Since the bacterial surface shows a slightly negative charge, positively charged MNPs are strongly attracted to the surface of bacteria, resulting in an increasing of antibacterial activity. On the other hand, negative or neutral charged MNPs have a significantly decreased antibacterial effect. However, an increase in the concentration of MNPs allows the attenuation of electrostatic repulsion through a bacterial surface saturation method (Abbaszadegan et al., 2015). After the adhesion onto the bacterial surface, MNPs can interact with the cells via two mechanisms, i.e, smaller MNPs penetrate directly into the cell, while the larger MNPs are retained outside the bacteria. In both situations, MNPs continuously release the metal ions, and these ions bind to the cell membrane structure that will destabilise the membrane potential and cause proton leakage. Cell wall destabilisation highly increases bacterial permeability and allows larger MNPs to enter the cell (Losasso et al., 2014).
There are a number of plant extracts and natural compounds isolated from plants that have antibacterial and antifungal activities. Most natural antimicrobial agents that target bacteria appear to disrupt membrane permeability, leading to membrane rupture and cell lysis. Furthermore, the inhibition of ergosterol biosynthesis, reduction of squalene epoxidase activity and fungal cell apoptosis are the specific mechanisms for antifungal, due to the structural differences between bacterial and fungal. However, not all mechanisms of action have been elucidated, and sometimes the mechanism may be indirect, stimulating the host immune system or inhibiting adhesion to the host cell.
Therefore, due to the unique action of metal nanoparticles that working synergically with botanical drugs may be useful to overcome multi drug resistance bacteria. A proposed site of action MNPs in enhancing antimicrobial activity of natural products is shown in Figure 3.
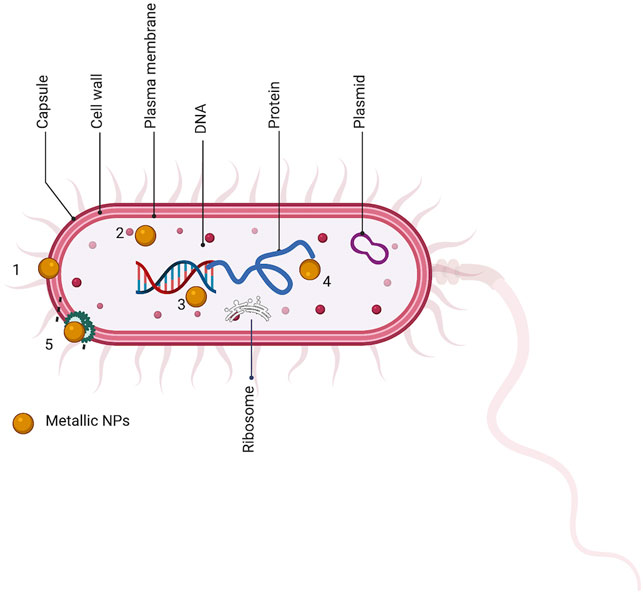
FIGURE 3. Sites of action of metal nanoparticle synthesized with natural product (MNps) on bacteria. Both components give synergically activity via similar mechanism of action bacterial cells. 1. bacterial efflux pump. 2. bacterial DNA. 3. bacterial ribosome. 4. bacterial protein. 5. bacterial cell walls integrity.
Once inside the cell, MNPs and their metal ions interact with numerous structures and biomolecules such as proteins, lipids and DNA, resulting in cell dysfunction. MNPs are well known by their high capacity to produce ROS and free radicals such as hydrogen peroxide (H2O2), superoxide anion (O−), and hydroxyl radical (OH•). Even though ROS occur naturally in bacteria as a result of cellular respiration, under normal circumstances bacteria have defense mechanism such as glutathione (GSH), superoxide dismutase, and catalase that act as antioxidant enzymes and eliminate these toxic species. High concentrations of metal ions released by MNPs produce extreme levels of oxidative stress. However, antioxidant enzymes will remove some of the released metal ions and these are not enough to neutralise ROS and free radical by the MNPs amount (Qing et al., 2018). These species interact with respiratory chain proteins on the membrane and inactivate the enzyme due to their high affinity to phosphates, thiol and carboxyl groups (Gordon et al., 2010). Their linkage to phosphate groups of the enzyme will inhibit the phosphorylation of proteins which is important for enzymatic activation, and this process cause the inhibition of bacterial growth. Furthermore, dephosphorylation of tyrosine residues of protein was also been implicated in disruption of biosynthesis and transport of exopolysaccharide and capsular polysaccharide to the membrane that will cause the disruption of cell cycle (Dakal et al., 2016). In addition, metal ions can intercalate DNA strands forming complexes with nucleic acids between the purine and pyrimidine base pairs that cause the disruption of hydrogen between them (Qing et al., 2018) and cause the DNA damage.
Characterisation MNPs
NPs’ physicochemical qualities influence their behaviour, biodistribution, safety, and efficacy. Thus, characterisation of metal NPs is critical for assessing the functional properties of the produced particles. Dynamic light scattering (DLS), zeta potential analyser, scanning electron microscopy (SEM), transmission electron microscopy (TEM), atomic force microscopy (AFM), X-ray diffractometry (XRD), Fourier transform infrared spectroscopy (FTIR) and UV-vis spectroscopy are some of the examples of technique used for NPs’ characterization. Combining these spectroscopic techniques offers information that could not be obtained with a single approach and helps in determine the quality of the synthesised nanoparticles.
The information such as size, size distribution, shape and surface chemistry are essential since these characteristics affect the absorption of NPs at the targeted site. Size of synthesised metal NPs is usually in the range of 5–100 nm and proven to enhance the antibacterial activity of plant extract loaded in NPs (Anbumani et al., 2022; Deivanathan and Prakash, 2022). The spectroscopy techniques such as DLS usually used to measure NPs size, size distribution and degree of aggregation.
Additionally, zeta potential analyser is used for determining the NPs surface charge. The surface charge above +30 mV and below −30 mV is generally regarded as targeted value to portrays lower degree of NPs aggregation and nanoparticle stability (Samimi et al., 2019). Shape is another NPs characteristic which can be measured using microscopy techniques such as SEM and TEM. TEM for instance, is a common technique to analyse NPs size, size distribution and shape. Other microscopy technique such as AFM can also being used to analyse NPs size, 3D-shape and elemental composition (Mourdikoudis et al., 2018) Usually after NPs synthesis, it will be characterised in terms of their crystal structure and lattice dimension using XRD. This technique was developed primarily to determine the 3D architectures and crystallinity of NPs.
FTIR spectroscopy is a method for determining NP structure and content. This method measures the absorption of electromagnetic radiation in the mid-infrared region (4,000–400 cm−1). FTIR analysis can be performed on both solid and liquid samples, and it has also been used to describe bacteria exposed to nanoparticles. The characteristics of functional groups and metabolites present on the surface of nanoparticles may be easily recognised using FTIR, which can occasionally aid in the reduction and stabilisation of nanoparticles (Nizamov et al., 2022).
UV-visible spectroscopy can be used to examine the scattering and absorption of light travelling through the material. This technique aids in the identification and characterisation of nanomaterials, as well as the determination of the stability of the nanoparticles generated. Other than that, HPLC provides more selective and specific measurement on the encapsulation efficiency, loading capacity and stability of plant extracts in NPs. Figure 4 summarizes the NPs characteristics and techniques used.
Limitations and Prospects
The antimicrobial activity of plant extracts loaded in NPs is still unclear, like some are emphasized on bacterial oxidative stress, whereas other NPs such as MgO-NPs may not be associated with bacterial metabolism (Wang et al., 2017). The lack of evidence of mechanism of action of plant extracts itself become a major issue in this review. Another limitation is the complex structure of microorganisms not permitted to be stimulated from in vitro study to in vivo condition. The environmental issue especially to fish and plankton population should be considered another limitation of NPs, due the release of NPs to the environment. The NPs will be ingested by aquatic organisms and be accumulated in animals and further in the food chain (Maharramov et al., 2019).
The combination of two metal or bimetallic nanoparticles in formulation plant extract may increase the antimicrobial activity. The successful bimetallic nanoparticle of Ag-Fe (AgNPs and FeNPs) has been produced using redox reactions of aqueous Gardenia jasminoides. It showed a synergistic effect as bactericidal and fungicidal effects against Gram-positive, Gram-negative and yeast (Padilla-Cruz et al., 2021). On the other hand, the hybrid silver-iron nanoparticle containing aminolevulinic acid showed significant enhancement in their cytotoxic activity on MCF-7 cell lines (de Oliveira Gonçalves et al., 2020). In the case of multidrug resistance, NPs seems to be an alternative strategy to deliver antimicrobial agents to fight numerous antibiotic resistance bacteria.
Conclusion
Metal nanoparticles alone have great potency as antimicrobial agents by showing different mechanisms of action. With the current development of drug delivery, the combination or green synthesis of natural products and in metal nanoparticles provide the advantage to produce synergistic activity of antimicrobial agents. Interestingly, there are not many purified compounds which possess antimicrobial agents that have been formulated with metal nanoparticles. It will give room to the researcher to do more research in this area. But this research would produce negative effects on the environment if not fully controlled. The use of uncontrolled metal nanoparticle can increase AMR incidents due to the effectiveness of metal nanoparticle in transformation of extracellular antibiotic resistant genes by 11-folds compared to the effects of antibiotics alone (Zhang et al., 2022).
Author Contributions
The research was planned and designed by MT and DS. MT, DS, and MH conducted the research, write the draft of the manuscript. JK critically reviewed and improved the manuscript. MT, DS, and MH equally contributed to the manuscript. All authors played their own role in the manuscript preparation, reviewing and funding.
Funding
This research was funded by the Ministry of Education, Culture, Research and Technology of the Republic of Indonesia via Research Grant PDUPT for 2021-2022.
Conflict of Interest
MH was employed by IKOP Pharma Sdn Bhd.
The remaining authors declare that the research was conducted in the absence of any commercial or financial relationships that could be construed as a potential conflict of interest.
Publisher’s Note
All claims expressed in this article are solely those of the authors and do not necessarily represent those of their affiliated organizations, or those of the publisher, the editors and the reviewers. Any product that may be evaluated in this article, or claim that may be made by its manufacturer, is not guaranteed or endorsed by the publisher.
References
Abbaszadegan, A., Ghahramani, Y., Gholami, A., Hemmateenejad, B., Dorostkar, S., Nabavizadeh, M., et al. (2015). The Effect of Charge at the Surface of Silver Nanoparticles on Antimicrobial Activity against Gram-Positive and Gram-Negative Bacteria: A Preliminary Study. J. Nanomater. 2015, 1–8. doi:10.1155/2015/720654
Abd El-Moaty, H. I., Soliman, N. A., Hamad, R. S., Ismail, E. H., Sabry, D. Y., and Khalil, M. M. H. (2021). Comparative Therapeutic Effects of Pituranthos Tortuosus Aqueous Extract and Phyto-Synthesized Gold Nanoparticles on Helicobacter pylori, Diabetic and Cancer Proliferation. South Afr. J. Bot. 139, 167–174. doi:10.1016/j.sajb.2021.02.009
Adavallan, K., and Krishnakumar, N. (2014). Mulberry Leaf Extract Mediated Synthesis of Gold Nanoparticles and its Anti-bacterial Activity against Human Pathogens. Adv. Nat. Sci. Nanosci. Nanotechnol. 5, 025018. doi:10.1088/2043-6262/5/2/025018
Ahmad, N., FoziaJabeen, M., Jabeen, M., Haq, Z. U., Ahmad, I., Wahab, A., et al. (2022). Green Fabrication of Silver Nanoparticles Using Euphorbia Serpens Kunth Aqueous Extract, Their Characterization, and Investigation of its In Vitro Antioxidative, Antimicrobial, Insecticidal, and Cytotoxic Activities. BioMed Res. Int. 2022, 1–11. doi:10.1155/2022/5562849
Al-Radadi, N. S. (2021). Facile One-step Green Synthesis of Gold Nanoparticles (AuNp) Using Licorice Root Extract: Antimicrobial and Anticancer Study against HepG2 Cell Line. Arabian J. Chem. 14, 102956. doi:10.1016/j.arabjc.2020.102956
Alarjani, K. M., Huessien, D., Rasheed, R. A., and Kalaiyarasi, M. (2022). Green Synthesis of Silver Nanoparticles by Pisum Sativum L. (Pea) Pod against Multidrug Resistant Foodborne Pathogens. J. King Saud Univ. - Sci. 34, 101897. doi:10.1016/j.jksus.2022.101897
Aljarba, N. H., Imtiaz, S., Anwar, N., Alanazi, I. S., and Alkahtani, S. (2022). Anticancer and Microbial Activities of Gold Nanoparticles: A Mechanistic Review. J. King Saud Univ. - Sci. 34, 101907. doi:10.1016/j.jksus.2022.101907
Allafchian, A., Vahabi, M. R., Jalali, S. A. H., Saeid Mahdavi, S., Sepahvand, S., and Farhang, H. R. (2022). Design of Green Silver Nanoparticles Mediated by Ferula Ovina Boiss. Extract with Enhanced Antibacterial Effect. Chem. Phys. Lett. 791, 139392. doi:10.1016/j.cplett.2022.139392
Anbumani, D., Dhandapani, K. v., Manoharan, J., Babujanarthanam, R., Bashir, A. K. H., Muthusamy, K., et al. (2022). Green Synthesis and Antimicrobial Efficacy of Titanium Dioxide Nanoparticles Using Luffa acutangula Leaf Extract. J. King Saud Univ. - Sci. 34, 101896. doi:10.1016/j.jksus.2022.101896
Arockiya Aarthi Rajathi, F., Parthiban, C., Ganesh Kumar, V., and Anantharaman, P. (2012). Biosynthesis of Antibacterial Gold Nanoparticles Using Brown Alga, Stoechospermum Marginatum (Kützing). Spectrochim. Acta A Mol. Biomol. Spectrosc. 99, 166–173. doi:10.1016/j.saa.2012.08.081
Asraf, M. H., Sani, N. S., Williams, C. D., Jemon, K., and Malek, N. A. N. N. (2022). In Situ biosynthesized Silver Nanoparticle-Incorporated Synthesized Zeolite A Using Orthosiphon Aristatus Extract for In Vitro Antibacterial Wound Healing. Particuology 67, 27–34. doi:10.1016/j.partic.2021.09.007
Baig, N., Kammakakam, I., and Falath, W. (2021). Nanomaterials: a Review of Synthesis Methods, Properties, Recent Progress, and Challenges. Mat. Adv. 2, 1821–1871. doi:10.1039/D0MA00807A
Balachandar, R., Navaneethan, R., Biruntha, M., Ashok Kumar, K. K., Govarthanan, M., and Karmegam, N. (2022). Antibacterial Activity of Silver Nanoparticles Phytosynthesized from Glochidion Candolleanum Leaves. Mater. Lett. 311, 131572. doi:10.1016/j.matlet.2021.131572
Balasubramanian, S., Kala, S. M. J., and Pushparaj, T. L. (2020). Biogenic Synthesis of Gold Nanoparticles Using Jasminum Auriculatum Leaf Extract and Their Catalytic, Antimicrobial and Anticancer Activities. J. Drug Deliv. Sci. Technol. 57, 101620. doi:10.1016/j.jddst.2020.101620
Bali, R., and Harris, A. T. (2010). Biogenic Synthesis of Au Nanoparticles Using Vascular Plants. Ind. Eng. Chem. Res. 49, 12762–12772. doi:10.1021/ie101600m
Bera, S., and Mondal, D. (2022). Antibacterial Efficacies of Nanostructured Aminoglycosides. ACS Omega 7, 4724–4734. doi:10.1021/acsomega.1c04399
Biswas, B., Rogers, K., McLaughlin, F., Daniels, D., and Yadav, A. (2013). Antimicrobial Activities of Leaf Extracts of Guava (Psidium Guajava L.) on Two Gram-Negative and Gram-Positive Bacteria. Int. J. Microbiol. 2013, 746165–746167. doi:10.1155/2013/746165
Bukhari, A., Ijaz, I., Gilani, E., Nazir, A., Zain, H., Saeed, R., et al. (2021). Green Synthesis of Metal and Metal Oxide Nanoparticles Using Different Plants' Parts for Antimicrobial Activity and Anticancer Activity: A Review Article. Coatings 11, 1374. doi:10.3390/coatings11111374
Chau, T. P., Veeraragavan, G. R., Narayanan, M., Chinnathambi, A., Alharbi, S. A., Subramani, B., et al. (2022). Green Synthesis of Zirconium Nanoparticles Using Punica Granatum (Pomegranate) Peel Extract and Their Antimicrobial and Antioxidant Potency. Environ. Res. 209, 112771. doi:10.1016/j.envres.2022.112771
Ciriminna, R., Fidalgo, A., Meneguzzo, F., Presentato, A., Scurria, A., Nuzzo, D., et al. (2020). Pectin: A Long-Neglected Broad-Spectrum Antibacterial. ChemMedChem 15, 2228–2235. doi:10.1002/cmdc.202000518
Da, X., Nishiyama, Y., Tie, D., Hein, K. Z., Yamamoto, O., and Morita, E. (2019). Antifungal Activity and Mechanism of Action of Ou-Gon (Scutellaria Root Extract) Components against Pathogenic Fungi. Sci. Rep. 9, 1683. doi:10.1038/s41598-019-38916-w
Dakal, T. C., Kumar, A., Majumdar, R. S., and Yadav, V. (2016). Mechanistic Basis of Antimicrobial Actions of Silver Nanoparticles. Front. Microbiol. 7, 1831. doi:10.3389/fmicb.2016.01831
de Oliveira Gonçalves, K., Vieira, D. P., Levy, D., Bydlowski, S. P., and Courrol, L. C. (2020). Uptake of Silver, Gold, and Hybrids Silver-Iron, Gold-Iron and Silver-Gold Aminolevulinic Acid Nanoparticles by MCF-7 Breast Cancer Cells. Photodiagnosis Photodyn. Ther. 32, 102080. doi:10.1016/j.pdpdt.2020.102080
Deivanathan, S. K., and Prakash, J. T. J. (2022). Green Synthesis of Silver Nanoparticles Using Aqueous Leaf Extract of Guettarda Speciosa and its Antimicrobial and Anti-oxidative Properties. Chem. Data Collect. 38, 100831. doi:10.1016/j.cdc.2022.100831
Dikshit, P., Kumar, J., Das, A., Sadhu, S., Sharma, S., Singh, S., et al. (2021). Green Synthesis of Metallic Nanoparticles: Applications and Limitations. Catalysts 11, 902. doi:10.3390/catal11080902
Dorosti, N., and Jamshidi, F. (2016). Plant-mediated Gold Nanoparticles by Dracocephalum Kotschyi as Anticholinesterase Agent: Synthesis, Characterization, and Evaluation of Anticancer and Antibacterial Activity. Jab 14, 235–245. doi:10.1016/j.jab.2016.03.001
Dridi, R., Essghaier, B., Hannachi, H., Khedher, G. B., Chaffei, C., and Zid, M. F. (2022). Biosynthesized Silver Nanoparticles Using Anagallis Monelli: Evaluation of Antioxidant Activity, Antibacterial and Antifungal Effects. J. Mol. Struct. 1251, 132076. doi:10.1016/j.molstruc.2021.132076
Elisha, I. L., Botha, F. S., McGaw, L. J., and Eloff, J. N. (2017). The Antibacterial Activity of Extracts of Nine Plant Species with Good Activity against Escherichia coli against Five Other Bacteria and Cytotoxicity of Extracts. BMC Complement. Altern. Med. 17, 133. doi:10.1186/s12906-017-1645-z
Emam, H. E., Zahran, M. K., and Ahmed, H. B. (2017). Generation of Biocompatible Nanogold Using H 2 O 2 -starch and Their Catalytic/antimicrobial Activities. Eur. Polym. J. 90, 354–367. doi:10.1016/j.eurpolymj.2017.03.034
Fuller, M., Whiley, H., and Köper, I. (2020). Antibiotic Delivery Using Gold Nanoparticles. SN Appl. Sci. 2, 1022. doi:10.1007/s42452-020-2835-8
Gardea-Torresdey, J. L., Parsons, J. G., Gomez, E., Peralta-Videa, J., Troiani, H. E., Santiago, P., et al. (2002). Formation and Growth of Au Nanoparticles inside Live Alfalfa Plants. Nano Lett. 2, 397–401. doi:10.1021/nl015673+
Gordon, O., Vig Slenters, T., Brunetto, P. S., Villaruz, A. E., Sturdevant, D. E., Otto, M., et al. (2010). Silver Coordination Polymers for Prevention of Implant Infection: Thiol Interaction, Impact on Respiratory Chain Enzymes, and Hydroxyl Radical Induction. Antimicrob. Agents Chemother. 54, 4208–4218. doi:10.1128/AAC.01830-09
Govindaraju, K., Vasantharaja, R., Uma Suganya, K. S., Anbarasu, S., Revathy, K., Pugazhendhi, A., et al. (2020). Unveiling the Anticancer and Antimycobacterial Potentials of Bioengineered Gold Nanoparticles. Process Biochem. 96, 213–219. doi:10.1016/j.procbio.2020.06.016
Guliani, A., Kumari, A., and Acharya, A. (2021). Green Synthesis of Gold Nanoparticles Using Aqueous Leaf Extract of Populus Alba: Characterization, Antibacterial and Dye Degradation Activity. Int. J. Environ. Sci. Technol. 18, 4007–4018. doi:10.1007/s13762-020-03065-5
Hagbani, T. A., Yadav, H., Moin, A., Lila, A. S. A., Mehmood, K., Alshammari, F., et al. (2022). Enhancement of Vancomycin Potential against Pathogenic Bacterial Strains via Gold Nano-Formulations: A Nano-Antibiotic Approach. Mater. (Basel) 15, 1108. doi:10.3390/ma15031108
Hammami, I., Alabdallah, N. M., jomaa, A. A., and kamoun, M. (2021). Gold Nanoparticles: Synthesis Properties and Applications. J. King Saud Univ. - Sci. 33, 101560. doi:10.1016/j.jksus.2021.101560
Hashemi, Z., Mizwari, Z. M., Mohammadi-Aghdam, S., Mortazavi-Derazkola, S., and Ali Ebrahimzadeh, M. (2022a). Sustainable Green Synthesis of Silver Nanoparticles Using Sambucus Ebulus Phenolic Extract (AgNPs@SEE): Optimization and Assessment of Photocatalytic Degradation of Methyl Orange and Their In Vitro Antibacterial and Anticancer Activity. Arabian J. Chem. 15, 103525. doi:10.1016/j.arabjc.2021.103525
Hashemi, Z., Shirzadi-Ahodashti, M., Mortazavi-Derazkola, S., and Ebrahimzadeh, M. A. (2022b). Sustainable Biosynthesis of Metallic Silver Nanoparticles Using Barberry Phenolic Extract: Optimization and Evaluation of Photocatalytic, In Vitro Cytotoxicity, and Antibacterial Activities against Multidrug-Resistant Bacteria. Inorg. Chem. Commun. 139, 109320. doi:10.1016/j.inoche.2022.109320
He, Z., Huang, Z., Jiang, W., and Zhou, W. (2019). Antimicrobial Activity of Cinnamaldehyde on Streptococcus Mutans Biofilms. Front. Microbiol. 10, 2241. doi:10.3389/fmicb.2019.02241
Hu, X., Wu, L., Du, M., and Wang, L. (2022). Eco-friendly Synthesis of Size-Controlled Silver Nanoparticles by Using Areca Catechu Nut Aqueous Extract and Investigation of Their Potent Antioxidant and Anti-bacterial Activities. Arabian J. Chem. 15, 103763. doi:10.1016/j.arabjc.2022.103763
Javed, R., Zia, M., Naz, S., Aisida, S. O., Ain, N. U., and Ao, Q. (2020). Role of Capping Agents in the Application of Nanoparticles in Biomedicine and Environmental Remediation: Recent Trends and Future Prospects. J. Nanobiotechnology 18, 172. doi:10.1186/s12951-020-00704-4
Jeevanandam, J., Chan, Y. S., and Danquah, M. K. (2016). Biosynthesis of Metal and Metal Oxide Nanoparticles. ChemBioEng Rev. 3, 55–67. doi:10.1002/cben.201500018
Kalpana, V. N., and Devi Rajeswari, V. (2018). A Review on Green Synthesis, Biomedical Applications, and Toxicity Studies of ZnO NPs. Bioinorg. Chem. Appl. 2018, 3569758. doi:10.1155/2018/3569758
Kaplan, Ö., Gökşen Tosun, N., İmamoğlu, R., Türkekul, İ., Gökçe, İ., and Özgür, A. (2022). Biosynthesis and Characterization of Silver Nanoparticles from Tricholoma Ustale and Agaricus Arvensis Extracts and Investigation of Their Antimicrobial, Cytotoxic, and Apoptotic Potentials. J. Drug Deliv. Sci. Technol. 69, 103178. doi:10.1016/j.jddst.2022.103178
Khameneh, B., Iranshahy, M., Soheili, V., and Fazly Bazzaz, B. S. (2019). Review on Plant Antimicrobials: a Mechanistic Viewpoint. Antimicrob. Resist Infect. Control 8, 118. doi:10.1186/s13756-019-0559-6
Khan, A. U., Khan, M., and Khan, M. M. (2019). Antifungal and Antibacterial Assay by Silver Nanoparticles Synthesized from Aqueous Leaf Extract of Trigonella Foenum-Graecum. BioNanoSci. 9, 597–602. doi:10.1007/s12668-019-00643-x
Khan, H. A., Ahmad, A., and Mehboob, R. (2015). Nosocomial Infections and Their Control Strategies. Asian Pac. J. Trop. Biomed. 5, 509–514. doi:10.1016/j.apjtb.2015.05.001
Kim, J., Ha Quang Bao, T., Shin, Y. K., and Kim, K. Y. (2018). Antifungal Activity of Magnoflorine against Candida Strains. World J. Microbiol. Biotechnol. 34, 167. doi:10.1007/s11274-018-2549-x
Kuete, V., Fozing, D. C., Kapche, W. F., Mbaveng, A. T., Kuiate, J. R., Ngadjui, B. T., et al. (2009). Antimicrobial Activity of the Methanolic Extract and Compounds from Morus Mesozygia Stem Bark. J. Ethnopharmacol. 124, 551–555. doi:10.1016/j.jep.2009.05.004
Kuppusamy, P., Yusoff, M. M., Maniam, G. P., and Govindan, N. (2016). Biosynthesis of Metallic Nanoparticles Using Plant Derivatives and Their New Avenues in Pharmacological Applications - an Updated Report. Saudi Pharm. J. 24, 473–484. doi:10.1016/j.jsps.2014.11.013
Kurtjak, M., Vukomanović, M., and Suvorov, D. (2017). Antibacterial Nanocomposite of Functionalized Nanogold and Gallium-Doped Hydroxyapatite. Mater. Lett. 193, 126–129. doi:10.1016/j.matlet.2017.01.092
Lashin, I. I., Aref, M. S., Abd El-Kader, E. M., Heussin, E. A., and Ewais, E. E.-D. A. (2021). Antimicrobial and In-Vitro Cytotoxic Efficacy of Betulinic Acid from the Callus, Regeneration, and Mother Plant of Dillenia Indica L. Egypt. J. Bot. 62, 213–226. doi:10.21608/ejbo.2021.81121.170610.21608/ejbo.2021.81121.1706
Lee, J., and Lee, D. G. (2015). Novel Antifungal Mechanism of Resveratrol: Apoptosis Inducer in Candida Albicans. Curr. Microbiol. 70, 383–389. doi:10.1007/s00284-014-0734-1
Li, Z. J., Liu, M., Dawuti, G., Dou, Q., Ma, Y., Liu, H. G., et al. (2017). Antifungal Activity of Gallic Acid In Vitro and In Vivo. Phytother. Res. 31, 1039–1045. doi:10.1002/ptr.5823
Loganathan, S., Selvam, K., Padmavathi, G., Shivakumar, M. S., Senthil-Nathan, S., Sumathi, A. G., et al. (2022). Biological Synthesis and Characterization of Passiflora Subpeltata Ortega Aqueous Leaf Extract in Silver Nanoparticles and Their Evaluation of Antibacterial, Antioxidant, Anti-cancer and Larvicidal Activities. J. King Saud Univ. - Sci. 34, 101846. doi:10.1016/j.jksus.2022.101846
Losasso, C., Belluco, S., Cibin, V., Zavagnin, P., Mičetić, I., Gallocchio, F., et al. (2014). Antibacterial Activity of Silver Nanoparticles: Sensitivity of Different Salmonella Serovars. Front. Microbiol. 5, 227. doi:10.3389/fmicb.2014.00227
Lubis, F. A., Malek, N. A. N. N., Sani, N. S., and Jemon, K. (2022). Biogenic Synthesis of Silver Nanoparticles Using Persicaria Odorata Leaf Extract: Antibacterial, Cytocompatibility, and In Vitro Wound Healing Evaluation. Particuology 70, 10–19. doi:10.1016/j.partic.2022.01.001
Maharramov, A. M., Hasanova, U. A., Suleymanova, I. A., Osmanova, G. E., and Hajiyeva, N. E. (2019). The Engineered Nanoparticles in Food Chain: Potential Toxicity and Effects. SN Appl. Sci. 1, 1362. doi:10.1007/s42452-019-1412-5
Makvandi, P., Wang, C. y., Zare, E. N., Borzacchiello, A., Niu, L. n., and Tay, F. R. (2020). Metal‐Based Nanomaterials in Biomedical Applications: Antimicrobial Activity and Cytotoxicity Aspects. Adv. Funct. Mat. 30, 1910021. doi:10.1002/adfm.201910021
Malik, P., Shankar, R., Malik, V., Sharma, N., and Mukherjee, T. K. (2014). Green Chemistry Based Benign Routes for Nanoparticle Synthesis. J. Nanoparticles 2014, 1–14. doi:10.1155/2014/302429
Manjunath, H. M., Joshi, C. G., Danagoudar, A., Poyya, J., Kudva, A. K., and Dhanajaya, B. L. (2017). Biogenic Synthesis of Gold Nanoparticles by Marine Endophytic Fungus-Cladosporium Cladosporioides Isolated from Seaweed and Evaluation of Their Antioxidant and Antimicrobial Properties. Process Biochem. 63, 137–144. doi:10.1016/j.procbio.2017.09.008
Marslin, G., Siram, K., Maqbool, Q., Selvakesavan, R., Kruszka, D., Kachlicki, P., et al. (2018). Secondary Metabolites in the Green Synthesis of Metallic Nanoparticles. Materials 11, 940. doi:10.3390/ma11060940
Mojally, M., Sharmin, E., Obaid, N. A., Alhindi, Y., and Abdalla, A. N. (2022). Polyvinyl Alcohol/corn Starch/castor Oil Hydrogel Films, Loaded with Silver Nanoparticles Biosynthesized in Mentha Piperita Leaves' Extract. J. King Saud Univ. - Sci. 34, 101879. doi:10.1016/j.jksus.2022.101879
Mondal, A., Chowdhury, S., Mondal, N. K., Shaikh, W. A., Debnath, P., and Chakraborty, S. (2022). Insecticidal and Fungicidal Performance of Bio-Fabricated Silver and Gold Nanoparticles. Int. J. Environ. Sci. Technol. 19, 1573–1592. doi:10.1007/s13762-021-03181-w
Mourdikoudis, S., Pallares, R. M., and Thanh, N. T. K. (2018). Characterization Techniques for Nanoparticles: Comparison and Complementarity upon Studying Nanoparticle Properties. Nanoscale 10, 12871–12934. doi:10.1039/C8NR02278J
Muhammad, M. H., Idris, A. L., Fan, X., Guo, Y., Yu, Y., Jin, X., et al. (2020). Beyond Risk: Bacterial Biofilms and Their Regulating Approaches. Front. Microbiol. 11, 928. doi:10.3389/fmicb.2020.00928
Munita, J. M., and Arias, C. A. (2016). Mechanisms of Antibiotic Resistance. Microbiol. Spectr. 4, 10.1128/microbiolspec.VMBF-0016-2015. doi:10.1128/microbiolspec.VMBF-0016-2015
Muniyappan, N., Pandeeswaran, M., and Amalraj, A. (2021). Green Synthesis of Gold Nanoparticles Using Curcuma Pseudomontana Isolated Curcumin: Its Characterization, Antimicrobial, Antioxidant and Anti- Inflammatory Activities. Environ. Chem. Ecotoxicol. 3, 117–124. doi:10.1016/j.enceco.2021.01.002
Muthukumar, T., SudhakumariSambandam, B., Sambandam, B., Aravinthan, A., Sastry, T. P., and Kim, J.-H. (2016). Green Synthesis of Gold Nanoparticles and Their Enhanced Synergistic Antitumor Activity Using HepG2 and MCF7 Cells and its Antibacterial Effects. Process Biochem. 51, 384–391. doi:10.1016/j.procbio.2015.12.017
Naseer, M., Aslam, U., Khalid, B., and Chen, B. (2020). Green Route to Synthesize Zinc Oxide Nanoparticles Using Leaf Extracts of Cassia Fistula and Melia Azadarach and Their Antibacterial Potential. Sci. Rep. 10, 9055. doi:10.1038/s41598-020-65949-3
Nizamov, S., Sazdovska, S. D., and Mirsky, V. M. (2022). A Review of Optical Methods for Ultrasensitive Detection and Characterization of Nanoparticles in Liquid Media with a Focus on the Wide Field Surface Plasmon Microscopy. Anal. Chim. Acta 1204, 339633. doi:10.1016/j.aca.2022.339633
Okon, E., Kukula-Koch, W., Halasa, M., Jarzab, A., Baran, M., Dmoszynska-Graniczka, M., et al. (2020). Magnoflorine-Isolation and the Anticancer Potential against NCI-H1299 Lung, MDA-MB-468 Breast, T98G Glioma, and TE671 Rhabdomyosarcoma Cancer Cells. Biomolecules 10, 1532. doi:10.3390/biom10111532
Padilla-Cruz, A. L., Garza-Cervantes, J. A., Vasto-Anzaldo, X. G., García-Rivas, G., León-Buitimea, A., and Morones-Ramírez, J. R. (2021). Synthesis and Design of Ag-Fe Bimetallic Nanoparticles as Antimicrobial Synergistic Combination Therapies against Clinically Relevant Pathogens. Sci. Rep. 11, 5351. doi:10.1038/s41598-021-84768-8
Peixoto, L. R., Rosalen, P. L., Ferreira, G. L., Freires, I. A., de Carvalho, F. G., Castellano, L. R., et al. (2017). Antifungal Activity, Mode of Action and Anti-biofilm Effects of Laurus Nobilis Linnaeus Essential Oil against Candida Spp. Arch. Oral Biol. 73, 179–185. doi:10.1016/j.archoralbio.2016.10.013
Peralta-Videa, J. R., Huang, Y., Parsons, J. G., Zhao, L., Lopez-Moreno, L., Hernandez-Viezcas, J. A., et al. (2016). Plant-based Green Synthesis of Metallic Nanoparticles: Scientific Curiosity or a Realistic Alternative to Chemical Synthesis? Nanotechnol. Environ. Eng. 1, 4. doi:10.1007/s41204-016-0004-5
Perveen, S., Nadeem, R., Rehman, S. u., Afzal, N., Anjum, S., Noreen, S., et al. (2022). Green Synthesis of Iron (Fe) Nanoparticles Using Plumeria Obtusa Extract as a Reducing and Stabilizing Agent: Antimicrobial, Antioxidant and Biocompatibility Studies. Arabian J. Chem. 15, 103764. doi:10.1016/j.arabjc.2022.103764
Puljula, E., Walton, G., Woodward, M. J., and Karonen, M. (2020). Antimicrobial Activities of Ellagitannins against Clostridiales Perfringens, Escherichia coli, Lactobacillus Plantarum and Staphylococcus aureus. Molecules 25, 3714. doi:10.3390/molecules25163714
Qing, Y., Cheng, L., Li, R., Liu, G., Zhang, Y., Tang, X., et al. (2018). Potential Antibacterial Mechanism of Silver Nanoparticles and the Optimization of Orthopedic Implants by Advanced Modification Technologies. Int. J. Nanomedicine 13, 3311–3327. doi:10.2147/IJN.S165125
Ramakritinan, C. M., Kaarunya, E., Shankar, S., and Kumaraguru, A. K. (2013). Antibacterial Effects of Ag, Au and Bimetallic (Ag-Au) Nanoparticles Synthesized from Red Algae. SSP 201, 211–230. doi:10.4028/www.scientific.net/SSP.201.211
Rauf, A., Ahmad, T., Khan, A., MaryamUddin, G., Uddin, G., Ahmad, B., et al. (2021). Green Synthesis and Biomedicinal Applications of Silver and Gold Nanoparticles Functionalized with Methanolic Extract of Mentha Longifolia. Artif. Cells, Nanomedicine, Biotechnol. 49, 194–203. doi:10.1080/21691401.2021.1890099
Salton, M. R. J., and Kim, K.-S. (1996). “Structure,” in Medical Microbiology. Editors S. Baron. (Galveston (TX): University of Texas Medical Branch). Available at: http://www.ncbi.nlm.nih.gov/books/NBK8477/ (Accessed March 12, 2022).
Samimi, S., Maghsoudnia, N., Eftekhari, R. B., and Dorkoosh, F. (2019). “Lipid-Based Nanoparticles for Drug Delivery Systems,” in Characterization and Biology of Nanomaterials for Drug Delivery (Amsterdam: Elsevier), 47–76. doi:10.1016/B978-0-12-814031-4.00003-9
Senthamarai, M. D., and Malaikozhundan, B. (2022). Synergistic Action of Zinc Oxide Nanoparticle Using the Unripe Fruit Extract of Aegle Marmelos (L.) - Antibacterial, Antibiofilm, Radical Scavenging and Ecotoxicological Effects. Mater. Today Commun. 30, 103228. doi:10.1016/j.mtcomm.2022.103228
Shaban, S., Patel, M., and Ahmad, A. (2020). Improved Efficacy of Antifungal Drugs in Combination with Monoterpene Phenols against Candida Auris. Sci. Rep. 10, 1162. doi:10.1038/s41598-020-58203-3
Shafey, A. M. E. (2020). Green Synthesis of Metal and Metal Oxide Nanoparticles from Plant Leaf Extracts and Their Applications: A Review. Green Process. Synthesis 9, 304–339. doi:10.1515/gps-2020-0031
Shang, Z. C., Han, C., Xu, J. L., Liu, R. H., Yin, Y., Wang, X. B., et al. (2019). Twelve Formyl Phloroglucinol Meroterpenoids from the Leaves of Eucalyptus Robusta. Phytochemistry 163, 111–117. doi:10.1016/j.phytochem.2019.04.008
Shkodenko, L., Kassirov, I., and Koshel, E. (2020). Metal Oxide Nanoparticles against Bacterial Biofilms: Perspectives and Limitations. Microorganisms 8, 1545. doi:10.3390/microorganisms8101545
Singh, J., Dutta, T., Kim, K. H., Rawat, M., Samddar, P., and Kumar, P. (2018). 'Green' Synthesis of Metals and Their Oxide Nanoparticles: Applications for Environmental Remediation. J. Nanobiotechnology 16, 84. doi:10.1186/s12951-018-0408-4
Singh, S., Fatima, Z., and Hameed, S. (2016). Citronellal-induced Disruption of Membrane Homeostasis in Candida Albicans and Attenuation of its Virulence Attributes. Rev. Soc. Bras. Med. Trop. 49, 465–472. doi:10.1590/0037-8682-0190-2016
Song, J. Y., and Kim, B. S. (2009). Rapid Biological Synthesis of Silver Nanoparticles Using Plant Leaf Extracts. Bioprocess Biosyst. Eng. 32, 79–84. doi:10.1007/s00449-008-0224-6
Sriramulu, M., Shanmugam, S., and Ponnusamy, V. K. (2020). Agaricus Bisporus Mediated Biosynthesis of Copper Nanoparticles and its Biological Effects: An In-Vitro Study. Colloid Interface Sci. Commun. 35, 100254. doi:10.1016/j.colcom.2020.100254
Stan, D., Enciu, A. M., Mateescu, A. L., Ion, A. C., Brezeanu, A. C., Stan, D., et al. (2021). Natural Compounds with Antimicrobial and Antiviral Effect and Nanocarriers Used for Their Transportation. Front. Pharmacol. 12, 723233. doi:10.3389/fphar.2021.723233
Sunderam, V., Thiyagarajan, D., Lawrence, A. V., Mohammed, S. S. S., and Selvaraj, A. (2019). In-vitro Antimicrobial and Anticancer Properties of Green Synthesized Gold Nanoparticles Using Anacardium Occidentale Leaves Extract. Saudi J. Biol. Sci. 26, 455–459. doi:10.1016/j.sjbs.2018.12.001
Sunkari, S., Gangapuram, B. R., Dadigala, R., Bandi, R., Alle, M., and Guttena, V. (2017). Microwave-irradiated Green Synthesis of Gold Nanoparticles for Catalytic and Anti-bacterial Activity. J. Anal. Sci. Technol. 8, 13. doi:10.1186/s40543-017-0121-1
Thangamani, N., and Bhuvaneshwari, N. (2019). Green Synthesis of Gold Nanoparticles Using Simarouba Glauca Leaf Extract and Their Biological Activity of Micro-organism. Chem. Phys. Lett. 732, 136587. doi:10.1016/j.cplett.2019.07.015
Vinay, S. P., UdayabhanuSumedha, H. N., Sumedha, H. N., Shashank, M., Nagaraju, G., and Chandrasekhar, N. (2021). In-vitro Antibacterial, Antioxidant and Cytotoxic Potential of Gold Nanoparticles Synthesized Using Novel Elaeocarpus Ganitrus Seeds Extract. J. Sci. Adv. Mater. Devices 6, 127–133. doi:10.1016/j.jsamd.2020.09.008
Wang, D. G., Liu, W. Y., and Chen, G. T. (2013). A Simple Method for the Isolation and Purification of Resveratrol from Polygonum Cuspidatum. J. Pharm. Anal. 3, 241–247. doi:10.1016/j.jpha.2012.12.001
Wang, L., Hu, C., and Shao, L. (2017). The Antimicrobial Activity of Nanoparticles: Present Situation and Prospects for the Future. Int. J. Nanomedicine 12, 1227–1249. doi:10.2147/IJN.S121956
Wylie, M. R., Windham, I. H., Blum, F. C., Wu, H., and Merrell, D. S. (2022). In Vitro antibacterial Activity of Nimbolide against Helicobacter pylori. J. Ethnopharmacol. 285, 114828. doi:10.1016/j.jep.2021.114828
Ye, L., Cao, Z., Liu, X., Cui, Z., Li, Z., Liang, Y., et al. (2022). Noble Metal-Based Nanomaterials as Antibacterial Agents. J. Alloys Compd. 904, 164091. doi:10.1016/j.jallcom.2022.164091
Yonathan, K., Mann, R., Mahbub, K. R., and Gunawan, C. (2022). The Impact of Silver Nanoparticles on Microbial Communities and Antibiotic Resistance Determinants in the Environment. Environ. Pollut. 293, 118506. doi:10.1016/j.envpol.2021.118506
Zayed, M. F., Mahfoze, R. A., El-kousy, S. M., and Al-Ashkar, E. A. (2020). In-vitro Antioxidant and Antimicrobial Activities of Metal Nanoparticles Biosynthesized Using Optimized Pimpinella Anisum Extract. Colloids Surfaces A Physicochem. Eng. Aspects 585, 124167. doi:10.1016/j.colsurfa.2019.124167
Zhang, D., Ma, X. L., Gu, Y., Huang, H., and Zhang, G. W. (2020). Green Synthesis of Metallic Nanoparticles and Their Potential Applications to Treat Cancer. Front. Chem. 8, 799. doi:10.3389/fchem.2020.00799
Zhang, S., Lu, J., Wang, Y., Verstraete, W., Yuan, Z., and Guo, J. (2022). Insights of Metallic Nanoparticles and Ions in Accelerating the Bacterial Uptake of Antibiotic Resistance Genes. J. Hazard Mater 421, 126728. doi:10.1016/j.jhazmat.2021.126728
Keywords: antimicrobial, nanoparticles, gold nanoparticle, silver nanoparticle, natural products, green synthesis
Citation: Susanti D, Haris MS, Taher M and Khotib J (2022) Natural Products-Based Metallic Nanoparticles as Antimicrobial Agents. Front. Pharmacol. 13:895616. doi: 10.3389/fphar.2022.895616
Received: 14 March 2022; Accepted: 04 May 2022;
Published: 02 June 2022.
Edited by:
Mukhlesur Rahman, University of East London, United KingdomReviewed by:
Hamed Barabadi, Shahid Beheshti University of Medical Sciences, IranMaria De Lourdes Pereira, University of Aveiro, Portugal
Copyright © 2022 Susanti, Haris, Taher and Khotib. This is an open-access article distributed under the terms of the Creative Commons Attribution License (CC BY). The use, distribution or reproduction in other forums is permitted, provided the original author(s) and the copyright owner(s) are credited and that the original publication in this journal is cited, in accordance with accepted academic practice. No use, distribution or reproduction is permitted which does not comply with these terms.
*Correspondence: Deny Susanti, deny@iium.edu.my; Muhammad Salahuddin Haris, solah@iium.edu.my; Muhammad Taher, mtaher@iium.edu.my; Junaidi Khotib, junaidi-k@ff.unair.ac.id