- 1National Clinical Research Center for Mental Disorders, Department of Psychiatry, China National Technology Institute on Mental Disorders, The Second Xiangya Hospital of Central South University, Changsha, China
- 2Department of Ultrasound Diagnostic, The Second Xiangya Hospital of Central South University, Changsha, China
- 3Department of Radiology, The Second Xiangya Hospital of Central South University, Changsha, China
Recent studies have reported that the gut microbiota influences mood and cognitive function through the gut-brain axis, which is involved in the pathophysiology of neurocognitive and mental disorders, including Parkinson’s disease, Alzheimer’s disease, and schizophrenia. These disorders have similar pathophysiology to that of cognitive dysfunction in bipolar disorder (BD), including neuroinflammation and dysregulation of various neurotransmitters (i.e., serotonin and dopamine). There is also emerging evidence of alterations in the gut microbial composition of patients with BD, suggesting that gut microbial dysbiosis contributes to disease progression and cognitive impairment in BD. Therefore, microbiota-centered treatment might be an effective adjuvant therapy for BD-related cognitive impairment. Given that studies focusing on connections between the gut microbiota and BD-related cognitive impairment are lagging behind those on other neurocognitive disorders, this review sought to explore the potential mechanisms of how gut microbial dysbiosis affects cognitive function in BD and identify potential microbiota-centered treatment.
1 Introduction
Bipolar disorder (BD) is a mood disorder characterized by recurring manic or hypomanic episodes alternating with depressive episodes, which presence increases the risk of multisystem complications, including cognitive impairment and metabolic disorders, seriously affecting the quality of life (Goldstein et al., 2011; Carvalho et al., 2020). Moreover, symptomatic remission constantly precedes the recovery of psychosocial function following a mood episode in BD patients, which is a trend mostly attributed to persistent neurocognitive impairment (Gitlin and Miklowitz, 2017). Thus, therapies focusing on cognitive improvement are greatly significant, considering the well-being and quality of life of BD patients (Bonnín et al., 2019).
Cognitive impairment in BD patients is characterized by defects in neurocognitive areas, including executive function, verbal and visual memory, working memory, attention, and reaction time (Cullen et al., 2016). Tatay-Manteiga et al. (2018) observed neurocognitive dysfunction across all stages of BD. Selective cognitive decline even persists during remission in BD patients (Ott et al., 2021). Cross-sectional evidence also suggests that neurocognition may worsen with chronic comorbidity progression in BD patients (Berk et al., 2017). Compared to patients with schizophrenia (Barch and Sheffield, 2014) or neurodegenerative diseases, such as Alzheimer’s disease (AD) (Simjanoski et al., 2021), BD patients exhibit a similar cognitive profile but with a milder degree of impairment, suggesting that there is overlapping pathophysiology underlying the cognitive impairment across these diseases. Currently, potential mechanisms of cognitive impairment in BD patients require further exploration. Neuroimaging studies have identified certain structural abnormalities associated with cognition, including a reduction in the prefrontal lobe (Abe et al., 2015) and hippocampal volume (Hoseth et al., 2016) and cortical thinning. A reduction in brain volume, especially in the hippocampus, is mostly attributed to excessive glucocorticoid exposure induced by oxidative stress (Alfarez et al., 2008) and abnormal neuroplasticity induced by inflammation and reduced brain-derived neurotrophic factor (BDNF) concentrations (Mondelli et al., 2011). Multiple studies have also demonstrated that significant associations exist between cognitive impairment in BD and inflammation, oxidative stress, and metabolic disorders (Hoseth et al., 2016; Cuperfain et al., 2020).
With the emergence of microbiome research, gut microbiota and gut-brain hormones have also been linked to cognitive impairment in severe mental disorders (Bioque et al., 2021; Misiak et al., 2020). Gut microbiota may modulate the function of the central nervous system, thereby altering behavior and cognition (Table 1), while the brain can activate signaling pathways affecting immune and metabolic function and host behavior—influencing the population and composition of the gut microbiota. The 2-way crosstalk between the central nervous system and gut microbiota via various routes including the immune system, enteroendocrine signaling, the vagus nerve and the enteric nervous system, as well as multiple gut microbial metabolites is known as the brain-gut axis (Cryan et al., 2019). The gut microbiota might be involved in postnatal development and have long-term implications for brain function (Sharon et al., 2016). Increased intestinal permeability promotes the release of pro-inflammatory cytokines and microbial-derived metabolites [e.g., lipopolysaccharides (LPS) and lipoproteins] into the circulatory system, causing systemic inflammation and blood-brain barrier impairment (Braniste et al., 2014; Schirmer et al., 2016; Houser and Tansey, 2017; Zhao et al., 2017). Neurochemical signals activated by the gut microbiota can be transmitted from the enteric nervous system to the central nervous system via the vagus nerve (Forsythe et al., 2014; Fung et al., 2017). Gut microbial dysbiosis also alters the expression of 5-hydroxytryptamine (5-HT) receptors, neurotrophic factors (e.g., BDNF), and N-methyl-d-aspartic acid (NMDA) receptor subunits in the hippocampus (Bercik et al., 2011), as well as myelin formation in the prefrontal cortex (Hoban et al., 2016a), leading to impaired social cognition. Such extensive overlapping mechanisms suggest a significant role of the gut microbiota in the development of cognitive impairment in BD.
Studies explicitly linking the gut microbiota to cognitive impairment in BD remain limited in number compared to those focusing on other neurodegenerative and psychiatric disorders. Therefore, this review summarizes preclinical and clinical evidence to explore potential mechanisms by which the gut microbiota affects cognitive function in BD patients and identify potential microbiota-centered treatment. Studies linking the gut microbiota to cognitive impairment in neurodegenerative and mental disorders might have implications for BD patients. Several studies have addressed the involvement of microbial-derived metabolites [e.g., single-chain fatty acids (SCFAs), secondary bile acids (BAs), and LPS], neurotransmitters, and gastrointestinal hormones in cognitive function. Complex interactions with the gut microbiota may also explain some cognitive side effects of certain psychiatric medications (Figure 1). This review concludes by discussing potential cognitive treatment targeting the gut microbiota, which may improve the quality of life of BD patients.
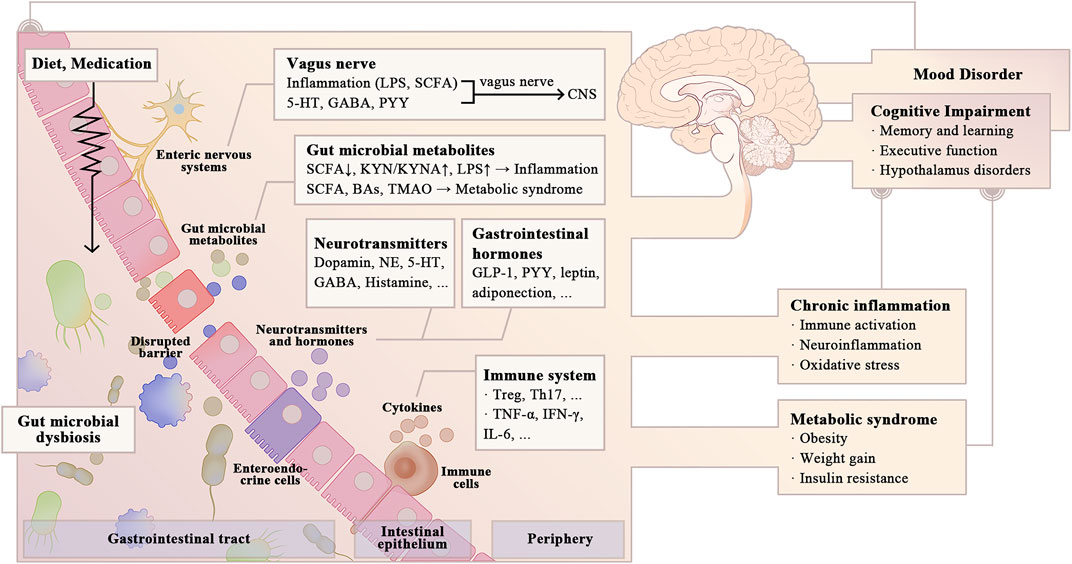
FIGURE 1. Possible pathways for gut microbial dysbiosis to affect cognitive function in BD. Gut microbial dysbiosis is widely viewed in BD patients, which may have a negative effect on cognitive function (e.g., learning and memory, executive function, and cognitive flexibility). Alterations in the gut microbial composition in BD patients increase intestinal permeability, promoting the release of pro-inflammatory cytokines and microbial-derived metabolites into the circulatory system, causing systemic inflammation and metabolic dysbiosis. Gut microbiota also modulates energy metabolism and cognitive function by influencing the synthesis of neurotransmitters and gastrointestinal hormones, as well as the vagal-dependent gut-brain signaling. Psychiatric medications and changing diet patterns in BD patients have complex interactions with gut microbiota, thereby influencing cognitive function. LPS, lipopolysaccharides; SCFA, single-chain fatty acids; GABA, γ-aminobutyric acid; PYY, Peptide YY; KYN, kynurenine; KYNA, kynurenic acid; Ba, bile acid; TMAO, trimethylamine-N-oxide; NE; GLP-1, glucagon-like peptide-1; Treg, regulatory T cell; Th, helper T cell; INF, Interferon; IL, Interleukin.
2 Direct Effects of the Gut Microbiota and its Metabolites on Cognitive Function in BD Patients
Evidence of altered gut microbial composition in BD patients suggests that gut microbial dysbiosis contributes to disease progression and pathophysiology in BD patients (Evans et al., 2017; Lai et al., 2020; McIntyre et al., 2021). Although there are few studies discussing the relationship between gut microbiota and cognitive impairment in BD patients, some have found that specific gut microorganisms are associated with reduced cognitive function (Severance et al., 2016). The relationship between the gut microbiota and specific cognitive domains, including learning, memory, attention, processing speed, and executive function—all of which are also impaired in BD—has been demonstrated in animal and human studies on hepatic encephalopathy (Bajaj et al., 2012), diabetes mellitus (Zheng et al., 2021), and aging (Li et al., 2021). Gut microbial dysbiosis also contributes to cognitive impairment in various neurodegenerative and psychiatric disorders, including Parkinson’s disease (Mulak and Bonaz, 2015; Sampson et al., 2016), AD (Jiang et al., 2017), and schizophrenia (Bioque et al., 2021), suggesting potential overlapping mechanisms between these conditions and BD.
2.1 Gut Microbial Alterations in BD Patients
Disruption of intestinal homeostasis affects the host’s metabolism and immune responses, leading to systemic disorders ranging from metabolic syndrome to chronic inflammation, which is closely related to the development of cognitive impairment (Kesika et al., 2021; Liu et al., 2020; McGrattan et al., 2019; Zeng et al., 2021). Therefore, a quantitative analysis of the gut microbiota in BD patients may help to improve an understanding of the mechanisms behind the development of cognitive impairment in this population. The most dominant gut microbial phyla in healthy adults include Firmicutes, Bacteroidetes, Proteobacteria, Actinobacteria, and Verrucomicrobia, with Firmicutes and Bacteroidetes accounting for nearly 80% of the total amount (Eckburg et al., 2005). In contrast, multiple studies have demonstrated alterations in gut microbial composition in BD patients compared to healthy controls (Figure 2). Lai et al. (2020) found that, in BD patients, counts of Faecalibacterium prausnitzii, Bacteroides, Prevotella, Atopobium cluster, Enterobacter spp., and Clostridium cluster organisms were significantly increased, and the log10 (ratio of Bifidobacteria to Enterobacteriaceae) was decreased. Another two studies have shown an increase in organisms of the Firmicutes and Bacteroides phyla but a decrease in those of the Bacteriodetes phylum in BD patients, with the Firmicutes-to-Bacteriodetes ratio being accordingly increased (Rong et al., 2019; Lai WT. et al., 2021). An increased Firmicutes-to-Bacteriodetes ratio is a recognized indicator of obesity (Crovesy et al., 2020; Magne et al., 2020). However, bacteria of the Ruminococcaceae family and Faecalibacterium genus, which are butyrate-producing bacteria, were reduced in BD patients relative to healthy controls in other studies (Hu et al., 2019; Lai WT. et al., 2021; Sublette et al., 2021). In 4 of 5 studies, lower α-diversity was observed in BD patients compared to healthy controls (Sublette et al., 2021).
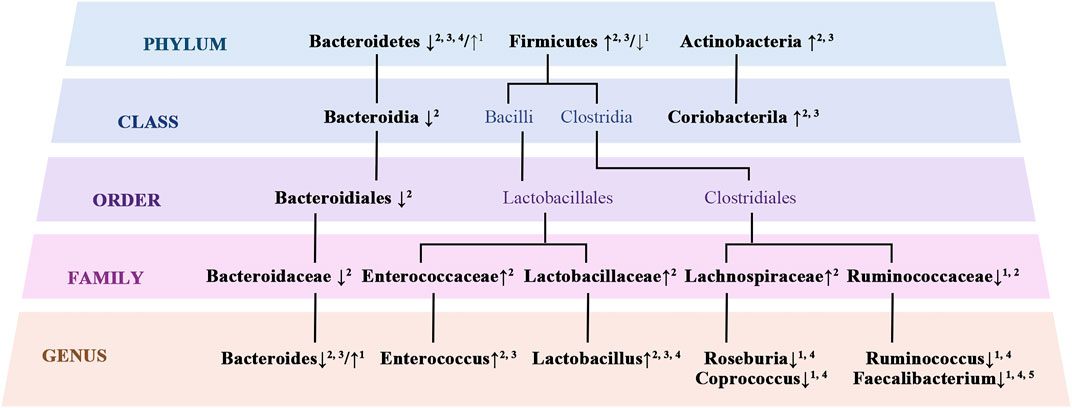
FIGURE 2. Altered gut microbial composition in BD. 1 (Hu et al., 2019); 2 (Lai J. et al., 2021); 3 (Rong et al., 2019); 4 (Zheng et al., 2020); 5 (Evans et al., 2017)
In conclusion, the most convergent taxonomic finding in BD patients is the reduction in butyrate-producing bacteria, gut microbiota known to impact cognitive function by producing SCFAs, including acetate and butyrate (Tanca et al., 2017). A decrease in butyrate-producing bacteria, such as those of the genus Faecalibacterium, was also observed in patients with AD and accompanied by neuroinflammation and impaired cognitive function (Ling et al., 2020; Marizzoni et al., 2020). Associations between cognitive impairment and decreased butyrate-producing bacteria have also been observed in patients with Parkinson’s disease (Nuzum et al., 2020; Tan et al., 2021) and alcohol use disorders (Leclercq et al., 2020) and are likely mediated by butyrate. It has been demonstrated that butyrate levels in the central nervous system can be influenced by gut microbial composition; for example, Clostridium butyricum, as a probiotic bacteria, restored butyrate content in the brain and significantly alleviated cognitive impairment and histopathological changes in a mouse model of vascular dementia (Liu et al., 2015). Clostridium butyricum treatment also attenuated cognitive impairment and prevented microglia-mediated neuroinflammation in a manner mediated by butyrate in an AD mouse model (Sun et al., 2020). Prolonged treatment with sodium butyrate stimulates neurogenesis and improves memory and associative learning (Kim et al., 2009; Govindarajan et al., 2011). On the one hand, butyrate inhibits histone deacetylase, which plays an important role in intestinal barrier regulation and intestinal energy metabolism, thereby affecting cognitive function (Leonel and Alvarez-Leite, 2012; Tanca et al., 2017). Butyrate can also increase hippocampal neurogenesis and the expression of the neurotrophic factor BDNF, improving learning behavior and long-term memory (Levenson et al., 2004; Sleiman et al., 2016). Considering the neuroprotective and cognitive improvement effects of butyrate, inadequate concentrations of butyrate-producing bacteria may be involved in the pathophysiology of cognitive impairment in BD patients.
2.2 Effects of Gut Microbial Metabolites on Cognitive Function in BD Patients
The interaction between butyric acid-producing bacteria and cognitive impairment suggests that microbiota-produced small-molecule metabolites mediate host-microbiome interactions (Donia and Fischbach, 2015). An analysis of brain transcriptomic data from BD patients has also revealed disturbances in gut microbial metabolites, such as tryptophan, SCFAs, and BAs (Moolamalla and Vinod, 2020). These overlapping metabolite profiles suggest potential routes for the involvement of the gut microbiota in cognitive impairment in BD.
2.2.1 Effects of Inflammatory Gut Microbial Metabolites
SCFAs (including acetate, propionate and butyrate), which are typical anti-inflammatory molecules produced by the gut microbiota, can exert anti-inflammatory effects by inhibiting interleukin (IL)-6, IL-1β, and tumor necrosis factor-α (TNF-α) expression through the FFAR2 (GPR43) receptor (Pirozzi et al., 2018). Animal studies have provided evidence on the effects of SCFAs on cognitive function through immunological pathways. The effects of butyrate on cognitive function have been described above. Additionally, decreased absolute concentrations of acetate, propionic acid, and butyrate lead to blood-brain barrier dysfunction, microglial activation, and elevated cortical IL-1β, IL-6, and TNF-α expression levels in mice maintained on a high-salt diet, exhibiting a reduced number of organisms in the Bacteroidetes and Proteobacteria phyla and an increased number of those in the Firmicutes phylum, respectively (Hu et al., 2020). Mice with a deficiency of the SCFA receptor FFAR2 showed global defects in microglia similar to those of germ-free mice, suggesting that the gut microbiota regulates microglia maturation and function (Borre et al., 2014; Erny et al., 2015). Microglia cells mediate neuroinflammation as the major innate immune cell population in the brain, playing an important role in the pathophysiology of BD (Maletic and Raison, 2014) and cognitive impairment (Feng et al., 2017; Zhao et al., 2019). However, to our knowledge, studies directly linking SCFAs to cognitive impairment in BD are still scarce. Indirectly, butyrate has been tested as a potential treatment for mood disorders, including BD and major depressive disorder, acting by controlling epigenetic programming associated with cognitive and behavioral regulation as a histone deacetylase inhibitor (Machado-Vieira et al., 2011). Lithium carbonate, one of the most commonly used drugs for treating BD, may activate anti-inflammatory regulatory T-cell responses through an FFAR2-dependent mechanism by altering the SCFA-producing gut microbiota (e.g., through upregulation of the butyric acid-producing bacterium Akkermansia muciniphila) to change SCFA profiles (Huang et al., 2022). With the support of some clinical studies, given the positive effects of lithium carbonate on cognitive functions, including memory and attention (Dias et al., 2012), disruption of the SCFA profile may impair cognitive function in BD patients through systemic inflammation. Marizzoni et al. (2020) found that both cognitive function and endothelial dysfunction in older adults are positively correlated with the pro-inflammatory cytokines acetate and valerate but negatively correlated with the levels of butyric acid and IL-10. The gut microbiota with reduced SCFA production can also trigger an intestinal inflammatory response and progression of Parkinson’s disease (Unger et al., 2016; Vascellari et al., 2020; Aho et al., 2021). Such clinical evidence suggests that SCFAs are involved in the development of cognitive impairment through systemic inflammation caused by endothelial dysfunction.
Gut-derived tryptophan metabolites are also one of the microbiome-dependent signals regulating inflammatory responses in the host. In the gastrointestinal tract, tryptophan metabolism follows three major pathways, including the kynurenine pathway, the aryl hydrocarbon receptor pathway, and the serotonin production pathway (Figure 3). A review by Więdłocha et al., 2021) supposed that metabolites of tryptophan degradation along the kynurenine pathway not only have an adverse effect on several psychiatric disorders, including BD, schizophrenia, depression, dementia, and AD, but also toxicity on cognitive function. An experiment conducted by Leblhuber et al. (2018) revealed that elevation of the kynurenine pathway may be associated with reduced Faecalibacterium prausnitzii and activation of macrophages in AD patients with impaired cognitive function. Notably, BD patients also show reductions in Faecalibacterium populations (Hu et al., 2019). Several studies have determined that gut microbial dysbiosis activates indoleamine 2, 3-dioxygenase 1, the rate-limiting enzyme in the kynurenine pathway (Agus et al., 2018). Depletion of the gut microbiota in mice induces elevation of the kynurenine–kynurenic acid pathway, anxiety-like behavior, and cognitive deficits (Desbonnet et al., 2015). The kynurenine–kynurenic acid pathway is also elevated in germ-free mice transplanted with microbiota from schizophrenic patients, leading to impaired learning and memory functions (Zhu et al., 2020). In contrast, performances in both cognitive function (including object exploration and recognition, passive avoidance, and spatial discrimination, all depending on the integrity of hippocampal function) and synaptic plasticity were improved in a rodent model with reduced kynurenic acid synthesis (Potter et al., 2010). Aside from the kynurenine pathway, tryptophan can also be degraded into aryl hydrocarbon receptor agonists by the gut microbiota, which not only protect against increased gut permeability (Scott et al., 2020) but also reduce neuroinflammation by inducing interferon-I signaling in astrocytes (Rothhammer et al., 2016). Therefore, interventions aimed specifically at reducing kynurenine pathway activation may constitute a promising strategy for cognitive improvement in BD patients.
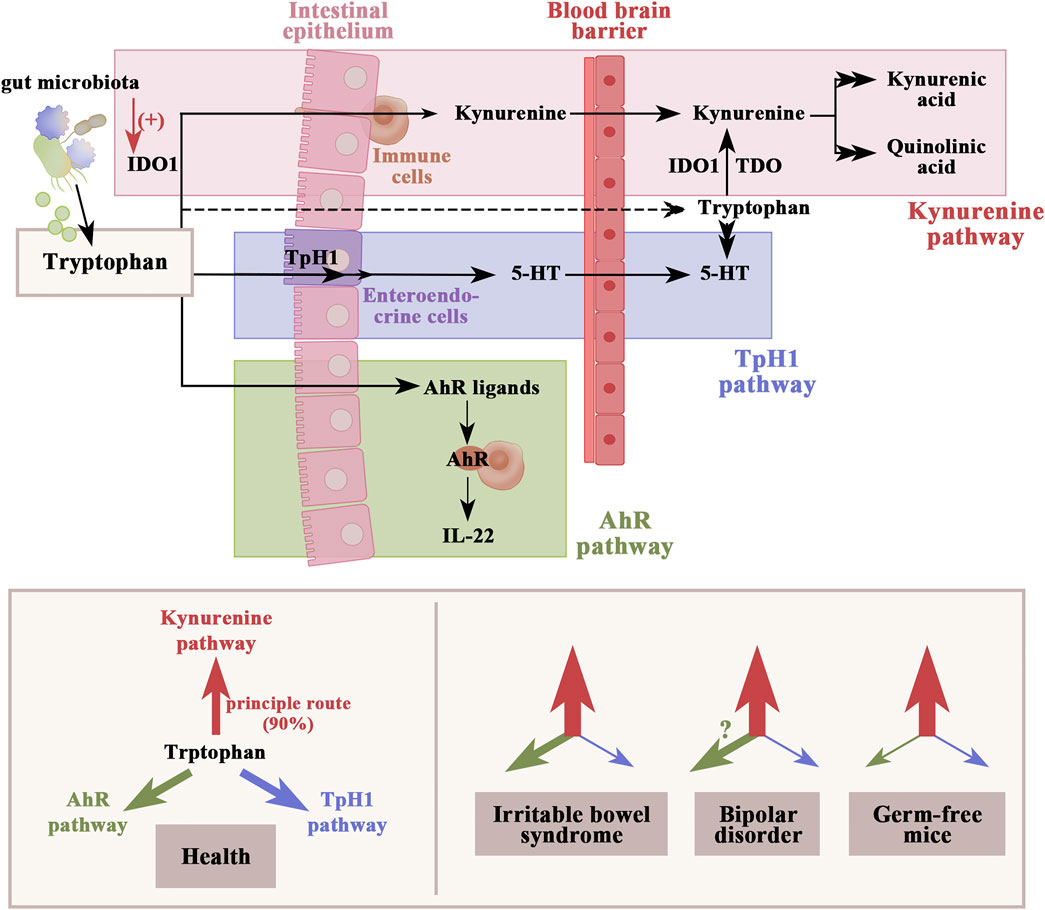
FIGURE 3. Dietary tryptophan metabolism follows three major pathways in the gastrointestinal tract: 1) the kynurenine pathway via IDO1, which can be activated by gut microbial dysbiosis; 2) production of serotonin in enteroendocrine cells and indirect regulation of tryptophan availability under the control of gut microbiota; and 3) the direct conversion to AhR ligands by gut microbiota. The figure shows an altered dynamic of the tryptophan metabolic pathway in IBS, BD, and germ-free mice based on the available clinical data. Weights of arrows indicate the strength of pathway activation. IDO1, indoleamine 2,3-dioxygenase 1; TDO, tryptophan 2,3-dioxygenase; TpH1, tryptophan hydroxylase 1; AhR, aryl hydrocarbon receptor.
Gut microbial dysbiosis also increases the production of LPS, a pro-inflammatory endotoxin, which diffuses into the blood along with increased gut permeability. LPS also contributes to the development of cognitive impairment through systemic inflammation, as evidenced by lower scores on the Boston naming test measuring visual confrontation naming, Verbal Fluency measuring executive function, and Word List Memory test measuring working memory (Chen et al., 2008). Additionally, LPS has adverse effects on both structure and function in the brain, increasing the activity of the amygdala responsible for emotion control and affecting object-exploration behavior by impairing cognitive function (Haba et al., 2012). LPS also decreases BDNF expression in the hippocampus, inducing cognitive impairment (Dinel et al., 2014). Consistently, gut-derived LPS-related immune activation has been observed in BD patients (Rudzki and Szulc, 2018). In mice transplanted with intestinal flora from BD patients, elevated levels of inflammatory cytokines and TRANK1 messenger RNA (TRANK1 is an important risk gene of BD) in the hippocampus and prefrontal cortex may be associated with LPS stimulation of BV-2 microglia (Lai J. et al., 2021). Therefore, LPS leakage due to gut microbial dysbiosis may play an important role in cognitive impairment in BD.
Bidirectional crosstalk between the hypothalamic-pituitary-adrenal (HPA) axis and the gut-brain axis in severe mental illness has been demonstrated in several studies (Misiak et al., 2020; Bioque et al., 2021), in which pro-inflammatory gut microbial metabolites play a key role. SCFAs not only decrease the activity of microglia and limit local inflammatory processes, but also decrease the expression of genes encoding proteins involved in the HPA axis (van de Wouw et al., 2018). LPS can directly stimulate cortisol secretion by human adrenal cells by a cyclooxygenase-dependent mechanism (Vakharia and Hinson, 2005). Reduced SCFA production (Pirozzi et al., 2018) and LPS leakage (Chen et al., 2008) in BD patients can also trigger an intestinal inflammatory response, enhancing release of cytokines, including IL-1β, IL-6 and TNF-α, all of which are potent activators of the HPA axis (Turnbull and Rivier, 1995). Since HPA axis activation and increased basal cortisol are known to be responsible for cognitive impairment including worse performance in visuospatial associative memory, attention and executive function in BD patients (Tournikioti et al., 2018), gut microbial dysbiosis may impair cognitive function in BD patients by activating the HPA axis. A study by Aizawa et al. (2018) provide some insight into the association between gut microbial dysbiosis and the HPA axis dysregulation in BD, as a significant negative correlation between the count of Bifidobacterium and cortisol levels was found. Notably, HPA axis dysregulation in BD may in turn further exacerbate gut microbial dysbiosis and intestinal permeability (Vanuytsel et al., 2014; Yoshikawa et al., 2017), thus creating a vicious cycle leading to further cognitive impairment.
2.2.2 Effects of Gut Microbial Metabolites Associated With Metabolic Syndrome
Studies have shown that gut microbial metabolites (including SCFAs, BAs, and LPS) as signaling molecules regulate physiological processes ranging from appetite and intestinal motility to energy metabolism in the host (Heiss and Olofsson, 2018). Therefore, gut microbial dysbiosis may lead to metabolic disorders and contribute to metabolic comorbidities, including diabetes mellitus and insulin resistance, playing an important role in the pathophysiology of various psychiatric diseases, including BD. BD patients presenting with metabolic syndromes are often at high risk of cognitive impairment.
In addition to SCFAs, food-derived gut microbial metabolites include BAs, trimethylamine-N-oxide (TMAO), and glutamate. BAs are synthesized from cholesterol in the liver and further metabolized to secondary bile acids by the gut microbiota (Schmidt et al., 2010). Gut microbial dysbiosis disrupts the signaling of BAs binding to the nuclear farnesoid X receptor (FXR) and Takeda G protein-coupled membrane receptor 5 (TGR5) (Huang et al., 2016; Chiang and Ferrell, 2020), which is also found in the brain (He et al., 2021). Although the effect of BAs on cognitive function in BD remains unclear, an increased ratio of secondary cytotoxic BAs to primary BAs has been linked to AD and cognitive impairment (MahmoudianDehkordi et al., 2019). INT-777, a TGR5 agonist, ameliorates synaptic dysfunction and reverses Aβ1-42-induced cognitive impairment in the mouse model of acute neurotoxicity by upregulating the expression of postsynaptic and presynaptic proteins (PSD95 and synaptophysin) and inhibiting apoptosis (Wu et al., 2018). BAs, such as tauroursodeoxycholic acid, have also been suggested as a potential treatment for AD (Zangerolamo et al., 2021). Thus, BAs may also be potential mediators between gut microbiota and cognitive impairment in BD patients.
TMAO has also been demonstrated to mediate gut microbiota-induced cognitive impairment. Administration of TMAO increases synaptic impairments by inhibiting the mammalian target of the rapamycin signaling pathway and decreases the expression levels of synaptic plasticity-related proteins, thereby exacerbating cognitive impairment (Li et al., 2018). A choline-induced AD mouse model exhibited increased TMAO synthesis, which is positively correlated with cognitive deterioration (Wang et al., 2020). Therefore, the role of TMAO in BD patients deserves further investigation.
3 The Role of Gastrointestinal Hormones and Neurotransmitters in the Interaction Between the Gut Microbiota and Cognitive Impairment in BD
The brain–gut axis reflects a complex bidirectional communication network between the gut microbiota and the brain, which relies on various neurotransmitters, gastrointestinal hormones, cytokines, and growth factors (Quigley, 2017; Cryan et al., 2019). These neurotransmitters and gastrointestinal hormones are produced by entero-endocrine cells in response to gut microbial inducement (Heiss and Olofsson, 2018). The gut microbiota also regulates the metabolisms of amino acids essential for the synthesis of neurotransmitters and gastrointestinal hormones (Holzer, 2016). Thus, gastrointestinal hormones and neurotransmitters may be important mediators in the interaction between the gut microbiota and cognitive impairment in BD.
3.1 The Role of Gastrointestinal Hormones
Gut microbial dysbiosis increases the intestinal permeability and penetration of pro-inflammatory factors, such as LPS, leading to intestinal inflammation, which, in turn, promotes energy absorption and reduces satiety via gastrointestinal hormones (Gomes et al., 2018; Nagpal et al., 2018). These gastrointestinal hormones have receptors expressed in regions of the brain that regulate not only hunger and energy metabolism but also stress, behaviors, and cognitive function (Chan et al., 2003; Bucinskaite et al., 2009).
Glucagon-like peptide-1 (GLP-1), an insulinotropic hormone secreted by entero-endocrine L-cells, can stimulate glucose-dependent insulin secretion in response to carbohydrate uptake (Holt & Trapp, 2016). In rodent models, butyrate and propionate stimulate GLP-1 secretion from entero-endocrine L-cells via FFAR2 (Tolhurst et al., 2012). Therefore, reduced concentrations of butyrate-producing bacteria in BD patients may downregulate GLP-1 secretion. GLP-1 receptors are expressed in the cerebral cortex, hypothalamus (ventral medial nucleus and arcuate nucleus), and limbic system (amygdala and hippocampus), all of which are regions responsible for the regulation of emotion and cognition (Heppner et al., 2015). GLP-1 and GLP-1 receptor (GLP-1R) signaling have a neuroprotective role in the control of insulin resistance, synaptic plasticity, and neuroinflammation and improve cognitive function in learning, memory, executive function, and attention (Müller et al., 2019; Flintoff et al., 2021). Therefore, reduced GLP-1R signaling caused by reduced butyrate-producing bacteria in BD patients may impair synaptic plasticity and cognitive function, as demonstrated by several studies. Liraglutide, a GLP-1 agonist, reversed manic-like symptoms and impairment in working and recognition memory by improving hippocampal oxidation and BDNF levels in a D-amphetamine-induced BD model (Chaves Filho et al., 2020). Serum GLP-1 levels are significantly lower in BD patients compared to healthy controls and negatively correlate with previous mood episodes (Rosso et al., 2015). In non-diabetic BD patients, liraglutide had beneficial effects on several cognitive domains, including auditory verbal learning, working memory, and attention (Mansur et al., 2017). Therefore, it is likely that gut microbial dysbiosis affects cognitive function in BD patients via GLP-1R signaling.
Peptide YY (PYY) is another intestinal satiety hormone produced by entero-endocrine L-cells, which affects the central nervous system by inhibiting orexigenic neurons expressing neuropeptide Y (Wynne and Bloom, 2006). Although there is limited evidence directly linking PYY to BD, a reduction in peripheral PYY concentrations might underlie the lack of GABA inhibition associated with impaired cognitive function in BD, considering that both neuropeptide Y and γ-aminobutyric acid (GABA) are released by arcuate neuropeptide Y neurons (Acuna-Goycolea et al., 2005; Huber et al., 2018).
Adipokines, represented by adiponectin and leptin, are cytokines or hormones secreted by white adipocytes in response to increased circulating inflammatory factors (Aguilar-Valles et al., 2015). Gut microbial dysbiosis elicits the penetration of microbial components (e.g., LPS), compromising leptin signaling and leading to leptin resistance and sustained high leptin levels (Faggioni et al., 1997). Intracerebroventricular administration of LPS induces an elevation in leptin receptors in the hippocampus, accompanied by impaired learning and memory, suggesting that leptin signaling disturbances in the hippocampus are involved in the regulation of cognitive responses (Da Ré et al., 2020). Studies suggest that leptin levels may affect cognitive function in BD patients. The TNF-α antagonist, infliximab, reduces plasma leptin levels in BD patients by modulating soluble tumor necrosis factor receptor 2, contributing to better performance on non-literal memory tasks and increased overall cortical volume, both of which are negatively correlated with the leptin level (Mansur et al., 2020). Adiponectin is the most abundant plasma adipokine that regulates energy expenditure, and it improves insulin sensitivity and fatty acid oxidation as an anti-inflammatory factor (Berg et al., 2002; Kubota et al., 2007). In current studies, adiponectin has been shown to improve cognitive function mainly by regulating insulin sensitivity and inhibiting inflammation (Rizzo et al., 2020). Additionally, it alleviates isoflurane-induced cognitive impairment in aging models by activating the p38-mitogen-activated protein kinase pathway and promoting the proliferation of hippocampal precursor cells (Zhang et al., 2019). Studies on adiponectin levels in BD patients remain controversial since body mass index seems to be more strongly correlated with adiponectin levels than mood status in BD patients (Platzer et al., 2019). Therefore, it is difficult to identify the role of the gut microbiota in the interaction between adiponectin and cognitive function in BD patients, which may be more directly correlated with comorbidities in BD patients.
Cholecystokinin (CCK) is a satiety hormone secreted from I-cells of the proximal small intestines during digestion (Raybould, 2007). Vagal afferent neurons, primary sensory neurons to regulate meal size, exhibit decreased CCK sensitivity when the gastrointestinal tract is colonized with high-fat-type microbiome, leading to increased food intake and body weight (Kim et al., 2020). LPS leakage due to increased inflammatory microbiome including Bacteroides and Prevotella also impairs CCK-induced satiety, and promotes food intake and excessive weight gain in aging mice (Rubio et al., 2021). Therefore, decreased CCK signaling caused by gut microbial dysbiosis may exacerbate emotional and cognitive impairment associated with obesity and metabolic syndrome. Except for binding to local vagally expressed receptors, periphery CCK can also act on CCKB receptors, which is widely expressed in limbic system (including the hippocampus and the prefrontal cortex), and thus directly affect emotion and cognition (Ballaz et al., 2020). Despite multiple studies showing consistent cognitive enhancing effects by CCKB receptor activation (Taghzouti et al., 1999; Hebb et al., 2005; Plagman et al., 2019), its role is virtually unknown in BD. Only one study by Sears et al. (2013) found associations between suicide attempt and 12 SNPs of CCKB receptors in BD patients. Therefore, further investigation into the role of CCK in cognitive function in BD is warranted.
3.2 The Role of Neurotransmitters Regulated by the Gut Microbiota
Gut microbiota can produce most of the neurotransmitters or neuromodulators in the human brain, including dopamine (which can be produced by Bacillus and Serratia), norepinephrine (produced by Escherichia, Bacillus, and Saccharomyces), serotonin (produced by Candida, Streptococcus, Escherichia, and Enterococcus), or GABA (produced by Lactobacillus and Bifidobacterium) (Donia and Fischbach, 2015; Strandwitz, 2018). These neurotransmitters mediate gut-brain signals, communicating with various regions of the brain, including the frontal cortex, limbic system, and autonomic and neuroendocrine centers, regulating not only appetite-related sensations but also emotion, cognition, and behavior (Holzer, 2016).
Dysregulation of the dopaminergic system contributes to both pathophysiology and impaired executive function in BD (Bercik et al., 2011; Kao et al., 2018). Various gut microbiota can produce dopamine, leading to dopaminergic aberrations when gut microbial dysbiosis occurs in BD (Tsavkelova et al., 2000). Mice treated with the dopamine transporter protein inhibitor GBR12909 (15 mg/kg) constitute a validated animal model for BD, whereas germ-free mice are less susceptible to GBR12909 with less mania-like behavior, suggesting that the gut microbiota contributes to the disease progression of BD via the dopaminergic system (de Miranda et al., 2020). Additionally, para-Cresol-treated mice with social-behavioral deficits have a similar gut microbial profile to that of BD patients (e.g., depleted Clostridiales), with a reduction in excitability and number of evoked action potentials of dopamine neurons in the ventral tegmental area (Bermudez-Martin et al., 2021). The increase in Bacteroides and Prevotella, which is also observed in BD patients, is negatively associated with dopamine transporter expression in the brain (Hartstra et al., 2020). The application of probiotics has been demonstrated to prevent cognitive impairment by regulating the gut microbiota, thereby increasing serotonin, dopamine, and GABA levels and restoring neuronal impairment (Song et al., 2021). The evidence presented above strongly supports the interaction between the dopaminergic system and gut microbiota, underlying the cognitive impairment in BD.
Serotonin, also known as 5-HT, is significantly correlated with cognitive function in BD patients (Chou et al., 2012). In clinical studies, tryptophan levels and the kynurenine-dependent tryptophan index were reduced in patients with bipolar mania and were positively correlated with the Young Mania Rating Scale and Brief Psychiatric Rating Scale scores (Myint et al., 2007). Reduced 5-HT levels in the brain lead to impaired cognitive flexibility, which is the characteristic type of cognitive impairment in BD (Evers et al., 2007). Notably, the gut microbiota can divert tryptophan metabolism to the production of kynurenine instead of 5-HT by activating indoleamine 2, 3-dioxygenase and tryptophan 2,3-dioxygenase (Badawy, 2017). A lack of aryl hydrocarbon receptor ligands in the intestinal contents of germ-free mice has been consistently observed (Lamas et al., 2016). In germ-free mice, there are also relatively low plasma kynurenine levels accompanied by increased indoleamine 2,3-dioxygenase activity, and kynurenine levels increase in these animals after recolonization of the gut microbiota (Clarke et al., 2013; Van der Leek et al., 2017). The altered dynamic of the tryptophan metabolic pathway has also been implicated in a study of postmortem anterior cingulate gyrus in BD patients, showing increased tryptophan 2,3-dioxygenase activity and kynurenine levels (Miller et al., 2006). Thus, the activation of the kynurenine pathway by gut microbiota dysbiosis consequently increases tryptophan consumption, which potentially contributes to the reduced 5-HT neurotransmission in BD patients, a statement being supported by studies focusing on comorbid irritable bowel syndrome (IBS) in BD patients (Tseng et al., 2016) (Figure 3). IBS, characterized by abdominal pain, bowel movement disorders, and gut microbial dysbiosis, is considered to be a valid contributor to BD (Castellini et al., 2016; Rosenblat et al., 2020). A follow-up study showed that IBS patients in clinical remission still experience persistent attention impairment, with a continuous increase in plasma IL-6 levels, the kynurenine-to-tryptophan ratio, and inactivation of the cortisol awakening response (Clarke et al., 2020). Acute tryptophan depletion significantly reduces plasma tryptophan and 5-hydroxyindoleacetic acid in IBS patients, inducing a negative shift in affective memory but without significant changes in mood (Kilkens et al., 2004). The evidence above suggests that 5-HT regulation by the gut microbiota may be involved in cognitive impairment in BD patients.
The gut microbiota profoundly affects peripheral GABA levels. Multiple organisms of gut microbiota are also involved in GABA synthesis, including a range of Bifidobacterium, Lactobacillus, and 16 intestinal Bacteroides strains (Barrett et al., 2012; Luck et al., 2021; Otaru et al., 2021). In germ-free animals, there is a significant reduction in GABA levels in the gut, serum, and brain (Matsumoto et al., 2013). Intestinal and peripheral GABA levels may influence the central nervous system through the gastrointestinal vagal nervous system. Bravo et al. (2011) found that treatment with Lactobacillus rhamnosus increases GABA(B1b) messenger RNA expression in cortical (cingulate and prefrontal) regions and decreases expression in the hippocampus and amygdala, consequently reducing corticosterone levels and manic-like behaviors. Moreover, the neurochemical and behavioral effects disappeared in vagotomized mice. Lactobacillus casei also stimulates gastrointestinal afferent vagal activity and inhibits stress-induced activation of cells producing pro-adrenocorticotropic hormone in the hypothalamic paraventricular nucleus in a dose-dependent manner, ameliorating somatic symptoms induced by learning stress (Takada et al., 2016). As reviewed by Wagner-Skacel et al. (2020), Lactobacillus has been significantly associated with the circadian rhythm in BD patients, linking a GABA disorder caused by the gut microbiota to cognitive function in BD. Thus, the gut microbiota may affect the GABA system mostly through the intestinal vagus nerve and modulate stress-related behavioral and cognitive functions in BD patients.
Multiple studies have demonstrated the versatility of glutamate, the agonist of N-methyl-d-aspartate receptor (NMDAR), as the foremost excitatory neurotransmitter in the central nervous system and modulator of gastrointestinal metabolism (Baj et al., 2019). A systematic review conducted by Reddy-Thootkur et al. (2020) shows that hippocampal glutamate levels are increased in BD patients, but no associations between glutamate metabolite levels and memory performance are detected. Considering that many of those included studies suffered from small sample sizes, the relationship between glutamate and cognitive impairment in BD require further exploration (Reddy-Thootkur et al., 2020). A postmortem study in 10 BD patients revealed significant lower protein and mRNA levels of NMDAR, indicating the presence of excitotoxicity induced by abnormal glutamatergic signaling in BD frontal cortex (Rao et al., 2010). Although there is evidence in support of the impact of the glutamatergic system in cognitive decline and disease progression in BD patients, information regarding the possible ways glutamate (either from dietary sources or microbial activities) may influence cognitive function in BD are still scarce. A Pilot study noted that the plasma and fecal glutamate levels, influenced by relative abundance of certain bacterial families, are negatively associated with cognitive function including processing speed, mental flexibility and executive function (Palomo-Buitrago et al., 2019). Dietary or luminal glutamate may also activate vagal afferents which directly or indirectly influence brain areas including the cerebral cortex, limbic system, hypothalamus and basal ganglia (Kondoh et al., 2009). From the foregoing, further study is needed to investigate the impact of a dietary glutamate and gut-endogenous glutamate in cognitive function in BD.
As an important part of monoamine metabolism, the role of D-amino acids in the gut-brain axis is gaining wider attention. Gut microbiota contributes to the host pool of D-amino acids via intrinsic amino acid racemases within certain gram-negative microbiome (Radkov and Moe, 2014). Kawase et al. (2017) found that gut microbiota could modulate the metabolism of D-amino acids in the brain. They noted that D-aspartic acid, D-serine were higher in some brain regions of GF mice than in those of SPF mice, indicating that gut microbiota may regulate the activity of aspartic acid racemase and serine racemase in the host brain (Kawase et al., 2017). D-serine is an endogenous ligand for NMDAR and thus play a key role in synaptic plasticity (Schell, 2004). Long-term potentiation, which underlies learning and memory, depends on calcium dependent release of D-serine from astrocytes in adult rat CA1 pyramidal hippocampus cells (Henneberger et al., 2010). Genetic association studies convince the role of D-serine in the pathology of BD, showing an association between BD with the gene G72, whose product activates the D-serine degrading enzyme (Chen et al., 2004; Müller et al., 2011). Furthermore, ketamine metabolites (rac)-dehydronorketamine and (2S,6S)-hydroxynorketamine decrease intracellular D-serine concentrations in a concentration dependent manner in PC-12 cells (Singh et al., 2016). Previous study has found potential pro-cognitive effects with intravenous subanesthetic ketamine in BD patients (Zhou et al., 2021). Based on these findings, we suggest that gut microbiota may influence synaptic D-serine availability and thus modulate cognitive function in BD patients.
BDNF can also promote the growth and development of neurons as a neurotrophic factor. Alterations in a 5-HT receptor, BDNF, and NMDA receptor subunit expression in the hippocampal region have been demonstrated in germ-free mice (Bercik et al., 2011). However, studies of BDNF levels and specific functional and behavioral alterations in germ-free or antibiotic-treated animal models remain controversial (Holzer, 2016).
4 Interactions Between the Gut Microbiota and Psychiatric Medication
Significantly more attention is being given to the interactions between psychiatric drugs commonly used in BD and the gut microbiota. Cognitive side effects induced using antipsychotics in the treatment of BD include impairments in verbal learning, memory, cognitive control, and spatial working memory (Arts et al., 2013; Flowers et al., 2016). Cognitive side effects have also been previously implicated in chronic inflammation and metabolic syndrome (Fang et al., 2019). Meanwhile, recent studies have considered the interaction between psychiatric medication and gut microbiota as a potential way by which the gut microbiota affects cognitive function (Flowers et al., 2019).
On the one hand, psychiatric medication has an important influence on the gut microbial profile, which, in turn, induces potential adverse events. Valproate significantly reduces fecal microbial richness and induces a gut microbial profile similar to that of patients with autism spectrum disorders (Liu et al., 2018). Flowers et al. (2017) showed that treatment with an atypical antipsychotic (AAP) in BD patients results in reduced gut microbial diversity, especially in women. A subsequent study focusing on patients with BD or schizophrenia also revealed significantly lower gut microbial diversity in AAP users compared to non-AAP users (Flowers et al., 2019). In contrast, Hu et al. (2019) found no significant changes in gut microbial α-diversity but noted an altered gut microbial composition in BD patients treated with AAP monotherapy (quetiapine). After such treatment, organisms of the Klebsiella and Veillonella genera are significantly increased in BD patients. Moreover, almost all AAPs can lead to weight gain, which may be closely related to gut microbial dysbiosis (McEvoy et al., 2005). Bahr et al. (2015b) found that the administration of risperidone inhibited non-aerobic resting metabolism in the gut microbiota, leading to a reduced total resting metabolism rate and increased body weight. The decreased Bacteroidetes-to-Firmicutes ratio induced by risperidone was linked to secondary weight gain in adolescent children (Bahr et al., 2015a). Zeng et al. (2021) also reviewed the potential role of the gut microbiota in cognitive impairment due to the metabolic side effects of AAPs used as a treatment in schizophrenic patients, which included disruption of inflammatory cytokine signaling and neurotransmitter disorders. Therefore, AAPs are likely to increase the risk of metabolic disorders by affecting the gut microbiota, consequently resulting in cognitive side effects.
On the other hand, the gut microbiota may also help to mediate the cognitive improvement effects of psychiatric medications for BD. Oral selective serotonin reuptake inhibitors (SSRIs) increase the excitability of the intestinal vagal nerve system through an intestinal epithelium-dependent mechanism. Critically, blocking the intestinal vagal signal by subdiaphragmatic vagotomy abolishes the antidepressant effect of oral SSRI treatment (McVey Neufeld et al., 2019). Both behavioral and neuroimaging studies have confirmed the positive effects of SSRI treatment on attention, appraisal, and memory before symptomatic remission. Additionally, Harmer and Cowen (2013) suggested that the antidepressant effects of SSRIs are cumulative results of improvements in cognitive functions related to emotion processing. Accumulated evidence highlights the potential role of vagal-dependent gut-brain signaling in cognitive improvement by SSRI treatment. Aripiprazole treatment also significantly increases the richness and diversity of the gut microbiota, especially the relative abundance of organisms of the minor genera Clostridium, Peptoclostridium, Intestinibacter, and Christenellaceae, also accompanied by a rise in acetate, butyrate and isovalerate levels (Cussotto et al., 2019). Increased numbers of butyrate-producing bacteria may underlie the cognitive improvement of aripiprazole (Ning et al., 2021; Peitl et al., 2021). Studies targeting the in vivo interactions of various BD medications with both the gut microbiota and cognitive function will provide new insights into the mechanisms and side effects of these drugs. Microbiota-centered treatment will also be important for optimizing the management of BD patients.
5 Potential Microbiota-Centered Treatments for Cognitive Improvement
Accumulated understanding of the brain-gut axis has led to the development of microbiota-centered treatment in mental illness acting through the gut flora. Both changes in diet habits and psychobiotic supplements can easily modify the gut microbiota. Fecal microbiota transplantation (FMT) facilitates a more stable evolution of gut microbial transplantation.
5.1 Diet
Changing one’s dietary habits is the easiest method to modulate gut microbiota. Gut microbial dysbiosis is a key factor of cognitive impairment in diet-induced obesity (Desbonnet et al., 2014). A dietary survey of 97 BD patients showed that BD patients had greater intake levels of processed meat and sugar, fat, and salt (Davison and Kaplan, 2012). Long-term consumption of a high-fat diet increases the Firmicutes-to-Bacteroides ratio, which is associated with obesity (Shi et al., 2015; Ussar et al., 2015). A high-fat diet also led to insulin resistance and hyperglycemia in diet-induced obese mice who exhibited neurotransmitter disorders, including increased GABA and decreased tryptophan levels (Scott et al., 2020). Further, a high-fat diet can cause a significant decrease in tyrosine phosphorylation of insulin receptors, accompanied by an increase in inflammatory response signals (e.g., nuclear factor kappa-light-chain-enhancer of activated B-cells, c-Jun N-terminal kinase) in whole-brain lysate and a decrease in synaptic plasticity, leading to learning and memory impairment (Kothari et al., 2017).
In contrast, healthy diets have shown therapeutic potential for neurocognitive disorders. The Mediterranean diet, characterized by a high intake of fruits, vegetables, nuts, whole grains, and high-protein foods (i.e., fish), can help to reduce intestinal inflammation, cognitive impairment, and the risk of dementia (Pistollato et al., 2018). People at high risk of cardiovascular diseases also showed higher scores on the mean Mini-Mental State Examination and Cognitive Dysfunction Test after long-term consumption of the Mediterranean diet (Martínez-Lapiscina et al., 2013). In terms of dietary composition, a diet high in protein and saturated fat contributed to a greater abundance of Bacteroides (Wu et al., 2011). Fruits and vegetables are rich in dietary fiber. Microbiota-accessible carbohydrates found in dietary fiber increase the richness and α-diversity of the gut microbiota and inhibit the hippocampal glial activation and neuroinflammation induced by a high-fat diet. In turn, these carbohydrates improved the performance of mice in nest-building and temporal order memory tests (Shi et al., 2020). Perez (2018) found that BD patients have lower compliance with the Mediterranean diet and higher biomarkers of insulin resistance compared to the healthy population. Therefore, dietary management of BD patients is crucial for reducing the risk of metabolic disorders and cognitive impairment in clinical practice.
The ketogenic diet is another dietary pattern that has attracted increasing attention in the treatment of neuropsychiatric diseases. Several studies have confirmed the role of the ketogenic diet in improving comorbidities and cognitive function in BD patients. The ketogenic diet reduces hyperinsulinemia in BD patients by alleviating mitochondrial dysfunction mediated by impairment of the phosphatidylinositol-3 kinase/protein kinase B/hypoxia-induced factor-1α signaling pathway (Campbell and Campbell, 2020). The ketogenic diet may affect the function of vesicular glutamate transporters and EAAT, Na+, K + -ATPase, Kir4.1, aquaporin-4, Cx34, and KATP channels by affecting the glutamate-glutamine cycle and glutamate synthase activity in astrocytes, thus reducing mild cognitive impairment in BD patients (Morris et al., 2020). Several studies have confirmed the role of the gut microbiota in the ketogenic diet, although only a few studies have discussed whether the gut microbiota is involved in the cognitive improvement effect of the ketogenic diet in BD patients. The ketogenic diet protects against seizures by regulating gut microbiota colonization, which increases GABA and glutamate levels in the hippocampus (Olson et al., 2018). In AD patients with mild cognitive impairment, a 6-week ketogenic diet increased the abundance of Enterobacteriaceae, Akkermansia, Slackia, Christensenellaceae, and Erysipelotriaceae and the synthesis of butyrate, accompanied by an improvement in cognitive function (Nagpal et al., 2020).
5.2 Psychobiotics
Probiotics are live microorganisms that offer non-specific benefits to the health of the host. Some of them may also modulate functions of the central nervous system, reducing psychiatric symptoms and improving cognitive function in patients, and are therefore known as psychobiotics (Evrensel et al., 2019). Clinical studies have demonstrated the role of probiotics in promoting gut microbial diversity and improving cognitive function in BD. Probiotics, including Lactobacillus rhamnosus strain GG and Bifidobacterium animalis subsp. lactis strain Bb12, reduce the risk of re-hospitalization among BD patients (Dickerson et al., 2018). A cohort study conducted by Reininghaus et al. (2018) showed a significant improvement in attention and psychomotor processing speed on the Digit Symbol Test and executive function on the Trail Making Test B in BD patients after long-term administration of a probiotic supplement, mainly containing organisms of the Lactobacillus genera and Bifidobacterium genera.
Because some prebiotics support the growth of specific gut microbiota with psychophysiological effects, some have been designated as psychobiotics, including fructooligosaccharides, inulin, and galactooligosaccharides (Sarkar et al., 2016). Currently, there are limited studies addressing the effect of prebiotics in BD patients, but studies in animal models confirm their potential for cognitive improvement. Prebiotics might suppress inflammation that affects cognitive function. Chitosan oligosaccharides effectively reduced learning and memory impairment in an AD model by inhibiting oxidative stress and reducing the release of pro-inflammatory factors, such as IL-1 and TNF-α (Jia et al., 2016). Prebiotics also regulate the synthesis of gastrointestinal hormones. Four weeks of supplementation with prebiotics increased the expression of anorexigenic gastrointestinal hormones, such as peptide tyrosine-tyrosine, GLP-1, and leptin, while decreasing levels of ghrelin and other anorexigenic hormones and helping to improve learning and memory function in schizophrenia patients (Kao et al., 2018). Weight gain induced by antipsychotics, including olanzapine and risperidone, can also be reduced by prebiotics. Intake of the prebiotic galactooligosaccharide mixture significantly reduced olanzapine-induced weight gain, possibly in association with an increased and decreased number of organisms belonging to the Bifidobacterium and Firmicutes genera, respectively, together with increased cortical phospho-NMDA receptor 1 levels and decreased plasma TNF-α levels. Therefore, these effects suggest that prebiotics may prevent the metabolic and cognitive side effects of olanzapine.
5.3 Gastrointestinal Hormone Analogs
The possible mechanisms by which the gut microbiota modulates energy metabolism and cognitive function by influencing gastrointestinal hormones have been reviewed above. Gastrointestinal hormone analogs may also improve cognitive function in a mode similar to that of gastrointestinal hormones. Liraglutide, the GLP-1 receptor agonist, improved D-amphetamine-induced mania-like symptoms and working and recognition memory impairment in a BD mouse model (Chaves Filho et al., 2020). In non-diabetic BD patients, liraglutide also showed beneficial effects in several cognitive domains, including auditory verbal learning, working memory, and attention (Mansur et al., 2017). Therefore, the neuroprotective effect of liraglutide illustrates the potential for gastrointestinal hormone analogs to serve as promising adjunctive tools for BD treatment.
5.4 Fecal Microbiota Transplantation
FMT treatment involves the injection of filtrate feces from a healthy donor into a patient. FMT not only increases the microbial diversity but also provides long-term implantation of donor strains compared to the short-term impact on the gut microbiota achieved by changes in the diet or the addition of psychobiotics (Weingarden and Vaughn, 2017). In animal research, FMT treatment reversed cognitive impairment in AD model mice by altering the gut microbial composition and SCFA profile and increasing synaptic plasticity. Currently, few clinical studies have focused on the feasibility and efficacy of FMT in BD patients, although Hinton (2020) claimed that depressive symptoms disappeared with weight loss in a BD patient who had experienced 9 sessions of FMT treatment. Therefore, FMT may have a potential role in improving the cognitive function of BD patients, but further clinical experiments are warranted.
5.5 Vagus Nerve Stimulation
The vagus nerve establishes one of the important connections between emotional and cognitive areas of the brain and gut functions. Vagal afferent fibers express receptors for multiple gastrointestinal hormones (GLP-1, CCK, peptide YY, ghrelin, etc.), neurotransmitters (dopamine, GABA, NE, 5-HT, etc.), and gut microbial metabolites (SCFAs, LPS, etc.), to transfer microbiota signals to the central nervous system (Bonaz et al., 2018; Breit et al., 2018). Brain pathway activated by oral administration of Campylobacter jejuni, which has been proved to influence behavior and brain functions at subclinical doses, has been mapped using c-fos expression as a marker of neuronal activation (Gaykema et al., 2004). In this study, brain activation was observed in the nucleus tractus solitarius, the vagal afferent ending, and the projections of the nucleus tractus solitarius including parabrachial nucleus, paraventricular nucleus of the hypothalamus, amygdala and thalamus, indicating vagally-mediated microbiota effect on mood and cognitive function (Gaykema et al., 2004). Consistently, Bravo et al. (2011) found that treatment with Lactobacillus rhamnosus decreases GABA(B1b) messenger RNA expression in the hippocampus and amygdala, consequently reducing corticosterone levels and manic-like behaviors. Moreover, the neurochemical and behavioral effects disappeared in vagotomized mice, indicating therapeutic potential for treatment targeting vagal tone.
Vagus nerve stimulation, a medical treatment that is routinely used in the treatment of epilepsy and other neurological conditions, works by applying electrical impulses to the vagus nerve (Wheless et al., 2018; González et al., 2019). A 5-year prospective research in patients with treatment-resistant bipolar depression showed that treatment with vagus nerve stimulation was associated with better medication response and significantly greater mean reduction in suicidality compared to treatment-as-usual (McAllister-Williams et al., 2020). Chronic vagus nerve stimulation also produces sustained clinical and cognitive improvements in BD patients in a treatment-resistant depressive episode (McAllister-Williams et al., 2020). Therefore, vagus nerve stimulation seems to be a promising adjunctive therapy for cognitive impairment in BD patients.
6 Conclusion
There is an exciting future potential for research on the connection between gut microbiota and neurocognitive elements in BD patients. Accumulated studies have offered convincing evidence of the participation of microbial-derived metabolites, neurotransmitters, and gastrointestinal hormones in cognitive function. Complex interactions with the gut microbiota may also explain some of the cognitive side effects of certain psychiatric medications. Understanding the potential mechanisms underlying the gut microbiota and cognitive impairment in BD can unlock the door for the application of microbiota-centered treatments in BD management, which may help to prevent adverse events and improve the quality of life in BD patients. However, further investigation is needed before applying these findings in clinical practice despite applauding the recent rise in these strategies.
Author Contributions
HW provided a significant contribution to the conceptualization. WD drafted the manuscript and constructed the figures and tables. JL and HY were responsible for data collection. YQ, SL and ZT reviewed and edited the manuscript. JH, HX, HT, and BW contributed to clinical content and proofreading. All authors contributed to the article and approved the submitted version.
Funding
This study was supported by grants from the National Natural Science Foundation of China (Grant Nos. 81971258, 81270019 and 81501163), Fundamental Research Funds for the Central Universities of Central South University (Nos. 2021zzts390), and Key-Area Research and Development Program of Guangdong Province (Nos. 2018B030334001).
Conflict of Interest
The authors declare that the research was conducted in the absence of any commercial or financial relationships that could be construed as a potential conflict of interest.
Publisher’s Note
All claims expressed in this article are solely those of the authors and do not necessarily represent those of their affiliated organizations, or those of the publisher, the editors and the reviewers. Any product that may be evaluated in this article, or claim that may be made by its manufacturer, is not guaranteed or endorsed by the publisher.
References
Abé, C., Ekman, C. J., Sellgren, C., Petrovic, P., Ingvar, M., and Landén, M. (2015). Manic Episodes Are Related to Changes in Frontal Cortex: a Longitudinal Neuroimaging Study of Bipolar Disorder 1. Brain 138 (Pt 11), 3440–3448. doi:10.1093/brain/awv266
Acuna-Goycolea, C., Tamamaki, N., Yanagawa, Y., Obata, K., and van den Pol, A. N. (2005). Mechanisms of Neuropeptide Y, Peptide YY, and Pancreatic Polypeptide Inhibition of Identified Green Fluorescent Protein-Expressing GABA Neurons in the Hypothalamic Neuroendocrine Arcuate Nucleus. J. Neurosci. 25 (32), 7406–7419. doi:10.1523/jneurosci.1008-05.2005
Aguilar-Valles, A., Inoue, W., Rummel, C., and Luheshi, G. N. (2015). Obesity, Adipokines and Neuroinflammation. Neuropharmacology 96 (Pt A), 124–134. doi:10.1016/j.neuropharm.2014.12.023
Agus, A., Planchais, J., and Sokol, H. (2018). Gut Microbiota Regulation of Tryptophan Metabolism in Health and Disease. Cell Host Microbe 23 (6), 716–724. doi:10.1016/j.chom.2018.05.003
Aho, V. T. E., Houser, M. C., Pereira, P. A. B., Chang, J., Rudi, K., Paulin, L., et al. (2021). Relationships of Gut Microbiota, Short-Chain Fatty Acids, Inflammation, and the Gut Barrier in Parkinson's Disease. Mol. Neurodegener. 16 (1), 6. doi:10.1186/s13024-021-00427-6
Aizawa, E., Tsuji, H., Asahara, T., Takahashi, T., Teraishi, T., Yoshida, S., et al. (2018). Bifidobacterium and Lactobacillus Counts in the Gut Microbiota of Patients with Bipolar Disorder and Healthy Controls. Front. Psychiatry 9, 730. doi:10.3389/fpsyt.2018.00730
Alfarez, D. N., Karst, H., Velzing, E. H., Joëls, M., and Krugers, H. J. (2008). Opposite Effects of Glucocorticoid Receptor Activation on Hippocampal CA1 Dendritic Complexity in Chronically Stressed and Handled Animals. Hippocampus 18 (1), 20–28. doi:10.1002/hipo.20360
Arts, B., Simons, C. J., Drukker, M., and van Os, J. (2013). Antipsychotic Medications and Cognitive Functioning in Bipolar Disorder: Moderating Effects of COMT Val108/158 Met Genotype. BMC Psychiatry 13, 63. doi:10.1186/1471-244x-13-63
Badawy, A. A. (2017). Kynurenine Pathway of Tryptophan Metabolism: Regulatory and Functional Aspects. Int. J. Tryptophan Res. 10, 1178646917691938. doi:10.1177/1178646917691938
Bahr, S. M., Tyler, B. C., Wooldridge, N., Butcher, B. D., Burns, T. L., Teesch, L. M., et al. (2015a). Use of the Second-Generation Antipsychotic, Risperidone, and Secondary Weight Gain Are Associated with an Altered Gut Microbiota in Children. Transl. Psychiatry 5 (10), e652. doi:10.1038/tp.2015.135
Bahr, S. M., Weidemann, B. J., Castro, A. N., Walsh, J. W., deLeon, O., Burnett, C. M., et al. (2015b). Risperidone-induced Weight Gain Is Mediated through Shifts in the Gut Microbiome and Suppression of Energy Expenditure. EBioMedicine 2 (11), 1725–1734. doi:10.1016/j.ebiom.2015.10.018
Baj, A., Moro, E., Bistoletti, M., Orlandi, V., Crema, F., and Giaroni, C. (2019). Glutamatergic Signaling along the Microbiota-Gut-Brain Axis. Int. J. Mol. Sci. 20 (6). doi:10.3390/ijms20061482
Bajaj, J. S., Ridlon, J. M., Hylemon, P. B., Thacker, L. R., Heuman, D. M., Smith, S., et al. (2012). Linkage of Gut Microbiome with Cognition in Hepatic Encephalopathy. Am. J. Physiol. Gastrointest. Liver Physiol. 302 (1), G168–G175. doi:10.1152/ajpgi.00190.2011
Ballaz, S. J., Bourin, M., Akil, H., and Watson, S. J. (2020). Blockade of the Cholecystokinin CCK-2 Receptor Prevents the Normalization of Anxiety Levels in the Rat. Prog. Neuropsychopharmacol. Biol. Psychiatry 96, 109761. doi:10.1016/j.pnpbp.2019.109761
Barch, D. M., and Sheffield, J. M. (2014). Cognitive Impairments in Psychotic Disorders: Common Mechanisms and Measurement. World Psychiatry 13 (3), 224–232. doi:10.1002/wps.20145
Barrett, E., Ross, R. P., O'Toole, P. W., Fitzgerald, G. F., and Stanton, C. (2012). γ-Aminobutyric Acid Production by Culturable Bacteria from the Human Intestine. J. Appl. Microbiol. 113 (2), 411–417. doi:10.1111/j.1365-2672.2012.05344.x
Bercik, P., Denou, E., Collins, J., Jackson, W., Lu, J., Jury, J., et al. (2011). The Intestinal Microbiota Affect Central Levels of Brain-Derived Neurotropic Factor and Behavior in Mice. Gastroenterology 141 (2), 599–609, 609 e1-3. doi:10.1053/j.gastro.2011.04.052
Berg, A. H., Combs, T. P., and Scherer, P. E. (2002). ACRP30/adiponectin: an Adipokine Regulating Glucose and Lipid Metabolism. Trends Endocrinol. Metab. 13 (2), 84–89. doi:10.1016/s1043-2760(01)00524-0
Berk, M., Post, R., Ratheesh, A., Gliddon, E., Singh, A., Vieta, E., et al. (2017). Staging in Bipolar Disorder: from Theoretical Framework to Clinical Utility. World Psychiatry 16 (3), 236–244. doi:10.1002/wps.20441
Bermudez-Martin, P., Becker, J. A. J., Caramello, N., Fernandez, S. P., Costa-Campos, R., Canaguier, J., et al. (2021). The Microbial Metabolite P-Cresol Induces Autistic-like Behaviors in Mice by Remodeling the Gut Microbiota. Microbiome 9 (1), 157. doi:10.1186/s40168-021-01103-z
Bioque, M., González-Rodríguez, A., Garcia-Rizo, C., Cobo, J., Monreal, J. A., Usall, J., et al. (2021). Targeting the Microbiome-Gut-Brain axis for Improving Cognition in Schizophrenia and Major Mood Disorders: A Narrative Review. Prog. Neuropsychopharmacol. Biol. Psychiatry 105, 110130. doi:10.1016/j.pnpbp.2020.110130
Bonaz, B., Bazin, T., and Pellissier, S. (2018). The Vagus Nerve at the Interface of the Microbiota-Gut-Brain Axis. Front. Neurosci. 12, 49. doi:10.3389/fnins.2018.00049
Bonnín, C. D. M., Reinares, M., Martínez-Arán, A., Jiménez, E., Sánchez-Moreno, J., Solé, B., et al. (2019). Improving Functioning, Quality of Life, and Well-Being in Patients with Bipolar Disorder. Int. J. Neuropsychopharmacol. 22 (8), 467–477. doi:10.1093/ijnp/pyz018
Borre, Y. E., O'Keeffe, G. W., Clarke, G., Stanton, C., Dinan, T. G., and Cryan, J. F. (2014). Microbiota and Neurodevelopmental Windows: Implications for Brain Disorders. Trends Mol. Med. 20 (9), 509–518. doi:10.1016/j.molmed.2014.05.002
Braniste, V., Al-Asmakh, M., Kowal, C., Anuar, F., Abbaspour, A., Tóth, M., et al. (2014). The Gut Microbiota Influences Blood-Brain Barrier Permeability in Mice. Sci. Transl. Med. 6 (263), 263ra158. doi:10.1126/scitranslmed.3009759
Bravo, J. A., Forsythe, P., Chew, M. V., Escaravage, E., Savignac, H. M., Dinan, T. G., et al. (2011). Ingestion of Lactobacillus Strain Regulates Emotional Behavior and Central GABA Receptor Expression in a Mouse via the Vagus Nerve. Proc. Natl. Acad. Sci. U. S. A. 108 (38), 16050–16055. doi:10.1073/pnas.1102999108
Breit, S., Kupferberg, A., Rogler, G., and Hasler, G. (2018). Vagus Nerve as Modulator of the Brain-Gut Axis in Psychiatric and Inflammatory Disorders. Front. Psychiatry 9, 44. doi:10.3389/fpsyt.2018.00044
Bucinskaite, V., Tolessa, T., Pedersen, J., Rydqvist, B., Zerihun, L., Holst, J. J., et al. (2009). Receptor-mediated Activation of Gastric Vagal Afferents by Glucagon-like Peptide-1 in the Rat. Neurogastroenterol. Motil. 21 (9), 978–e78. doi:10.1111/j.1365-2982.2009.01317.x
Campbell, I., and Campbell, H. (2020). Mechanisms of Insulin Resistance, Mitochondrial Dysfunction and the Action of the Ketogenic Diet in Bipolar Disorder. Focus on the PI3K/AKT/HIF1-a Pathway. Med. Hypotheses 145, 110299. doi:10.1016/j.mehy.2020.110299
Carvalho, A. F., Firth, J., and Vieta, E. (2020). Bipolar Disorder. Reply. N. Engl. J. Med. 383 (1), 58–66. doi:10.1056/NEJMc2026462
Castellini, G., Pecchioli, S., Cricelli, I., Mazzoleni, F., Cricelli, C., Ricca, V., et al. (2016). How to Early Recognize Mood Disorders in Primary Care: A Nationwide, Population-Based, Cohort Study. Eur. Psychiatry 37, 63–69. doi:10.1016/j.eurpsy.2016.04.003
Ceylani, T., Jakubowska-Doğru, E., Gurbanov, R., Teker, H. T., and Gozen, A. G. (2018). The Effects of Repeated Antibiotic Administration to Juvenile BALB/c Mice on the Microbiota Status and Animal Behavior at the Adult Age. Heliyon 4 (6), e00644. doi:10.1016/j.heliyon.2018.e00644
Chan, J. L., Heist, K., DePaoli, A. M., Veldhuis, J. D., and Mantzoros, C. S. (2003). The Role of Falling Leptin Levels in the Neuroendocrine and Metabolic Adaptation to Short-Term Starvation in Healthy Men. J. Clin. Invest 111 (9), 1409–1421. doi:10.1172/JCI17490
Chaves Filho, A. J. M., Cunha, N. L., de Souza, A. G., Soares, M. V., Jucá, P. M., de Queiroz, T., et al. (2020). The GLP-1 Receptor Agonist Liraglutide Reverses Mania-like Alterations and Memory Deficits Induced by D-Amphetamine and Augments Lithium Effects in Mice: Relevance for Bipolar Disorder. Prog. Neuropsychopharmacol. Biol. Psychiatry 99, 109872. doi:10.1016/j.pnpbp.2020.109872
Chen, J., Buchanan, J. B., Sparkman, N. L., Godbout, J. P., Freund, G. G., and Johnson, R. W. (2008). Neuroinflammation and Disruption in Working Memory in Aged Mice after Acute Stimulation of the Peripheral Innate Immune System. Brain Behav. Immun. 22 (3), 301–311. doi:10.1016/j.bbi.2007.08.014
Chen, Y. S., Akula, N., Detera-Wadleigh, S. D., Schulze, T. G., Thomas, J., Potash, J. B., et al. (2004). Findings in an Independent Sample Support an Association between Bipolar Affective Disorder and the G72/G30 Locus on Chromosome 13q33. Mol. Psychiatry 9 (1), 87–92. doi:10.1038/sj.mp.4001453
Chiang, J. Y. L., and Ferrell, J. M. (2020). Bile Acid Receptors FXR and TGR5 Signaling in Fatty Liver Diseases and Therapy. Am. J. Physiol. Gastrointest. Liver Physiol. 318 (3), G554–g573. doi:10.1152/ajpgi.00223.2019
Chou, Y. H., Wang, S. J., Lirng, J. F., Lin, C. L., Yang, K. C., Chen, C. K., et al. (2012). Impaired Cognition in Bipolar I Disorder: the Roles of the Serotonin Transporter and Brain-Derived Neurotrophic Factor. J. Affect Disord. 143 (1-3), 131–137. doi:10.1016/j.jad.2012.05.043
Clarke, G., Grenham, S., Scully, P., Fitzgerald, P., Moloney, R. D., Shanahan, F., et al. (2013). The Microbiome-Gut-Brain axis during Early Life Regulates the Hippocampal Serotonergic System in a Sex-dependent Manner. Mol. Psychiatry 18 (6), 666–673. doi:10.1038/mp.2012.77
Clarke, G., Kennedy, P. J., Groeger, J. A., Quigley, E. M., Shanahan, F., Cryan, J. F., et al. (2020). Impaired Cognitive Function in Crohn's Disease: Relationship to Disease Activity. Brain Behav. Immun. Health 5, 100093. doi:10.1016/j.bbih.2020.100093
Crovesy, L., Masterson, D., and Rosado, E. L. (2020). Profile of the Gut Microbiota of Adults with Obesity: a Systematic Review. Eur. J. Clin. Nutr. 74 (9), 1251–1262. doi:10.1038/s41430-020-0607-6
Crumeyrolle-Arias, M., Jaglin, M., Bruneau, A., Vancassel, S., Cardona, A., Daugé, V., et al. (2014). Absence of the Gut Microbiota Enhances Anxiety-like Behavior and Neuroendocrine Response to Acute Stress in Rats. Psychoneuroendocrinology 42, 207–217. doi:10.1016/j.psyneuen.2014.01.014
Cryan, J. F., O'Riordan, K. J., Cowan, C. S. M., Sandhu, K. V., Bastiaanssen, T. F. S., Boehme, M., et al. (2019). The Microbiota-Gut-Brain Axis. Physiol. Rev. 99 (4), 1877–2013. doi:10.1152/physrev.00018.2018
Cullen, B., Ward, J., Graham, N. A., Deary, I. J., Pell, J. P., Smith, D. J., et al. (2016). Prevalence and Correlates of Cognitive Impairment in Euthymic Adults with Bipolar Disorder: A Systematic Review. J. Affect Disord. 205, 165–181. doi:10.1016/j.jad.2016.06.063
Cuperfain, A. B., Kennedy, J. L., and Gonçalves, V. F. (2020). Overlapping Mechanisms Linking Insulin Resistance with Cognition and Neuroprogression in Bipolar Disorder. Neurosci. Biobehav Rev. 111, 125–134. doi:10.1016/j.neubiorev.2020.01.022
Cussotto, S., Strain, C. R., Fouhy, F., Strain, R. G., Peterson, V. L., Clarke, G., et al. (2019). Differential Effects of Psychotropic Drugs on Microbiome Composition and Gastrointestinal Function. Psychopharmacol. Berl. 236 (5), 1671–1685. doi:10.1007/s00213-018-5006-5
Da Ré, C., Souza, J. M., Fróes, F., Taday, J., Dos Santos, J. P., Rodrigues, L., et al. (2020). Neuroinflammation Induced by Lipopolysaccharide Leads to Memory Impairment and Alterations in Hippocampal Leptin Signaling. Behav. Brain Res. 379, 112360. doi:10.1016/j.bbr.2019.112360
Davison, K. M., and Kaplan, B. J. (2012). Food Intake and Blood Cholesterol Levels of Community-Based Adults with Mood Disorders. BMC Psychiatry 12, 10. doi:10.1186/1471-244x-12-10
de Miranda, A. S., Vieira, É. L. M., Dos Reis Bastos, J., Ferreira, R. N., Nicoli, J. R., Teixeira, M. M., et al. (2020). Role of Gut Microbiota in the GBR12909 Model of Mania-like Behavior in Mice. J. Neuroimmunol. 346, 577292. doi:10.1016/j.jneuroim.2020.577292
Desbonnet, L., Clarke, G., Shanahan, F., Dinan, T. G., and Cryan, J. F. (2014). Microbiota Is Essential for Social Development in the Mouse. Mol. Psychiatry 19 (2), 146–148. doi:10.1038/mp.2013.65
Desbonnet, L., Clarke, G., Traplin, A., O'Sullivan, O., Crispie, F., Moloney, R. D., et al. (2015). Gut Microbiota Depletion from Early Adolescence in Mice: Implications for Brain and Behaviour. Brain Behav. Immun. 48, 165–173. doi:10.1016/j.bbi.2015.04.004
Dias, V. V., Balanzá-Martinez, V., Soeiro-de-Souza, M. G., Moreno, R. A., Figueira, M. L., Machado-Vieira, R., et al. (2012). Pharmacological Approaches in Bipolar Disorders and the Impact on Cognition: a Critical Overview. Acta Psychiatr. Scand. 126 (5), 315–331. doi:10.1111/j.1600-0447.2012.01910.x
Dickerson, F., Adamos, M., Katsafanas, E., Khushalani, S., Origoni, A., Savage, C., et al. (2018). Adjunctive Probiotic Microorganisms to Prevent Rehospitalization in Patients with Acute Mania: A Randomized Controlled Trial. Bipolar Disord. 20 (7), 614–621. doi:10.1111/bdi.12652
Dinel, A. L., André, C., Aubert, A., Ferreira, G., Layé, S., and Castanon, N. (2014). Lipopolysaccharide-induced Brain Activation of the Indoleamine 2,3-dioxygenase and Depressive-like Behavior Are Impaired in a Mouse Model of Metabolic Syndrome. Psychoneuroendocrinology 40, 48–59. doi:10.1016/j.psyneuen.2013.10.014
Donia, M. S., and Fischbach, M. A. (2015). HUMAN MICROBIOTA. Small Molecules from the Human Microbiota. Science 349 (6246), 1254766. doi:10.1126/science.1254766
Eckburg, P. B., Bik, E. M., Bernstein, C. N., Purdom, E., Dethlefsen, L., Sargent, M., et al. (2005). Diversity of the Human Intestinal Microbial Flora. Science 308 (5728), 1635–1638. doi:10.1126/science.1110591
Erny, D., Hrabě de Angelis, A. L., Jaitin, D., Wieghofer, P., Staszewski, O., David, E., et al. (2015). Host Microbiota Constantly Control Maturation and Function of Microglia in the CNS. Nat. Neurosci. 18 (7), 965–977. doi:10.1038/nn.4030
Evans, S. J., Bassis, C. M., Hein, R., Assari, S., Flowers, S. A., Kelly, M. B., et al. (2017). The Gut Microbiome Composition Associates with Bipolar Disorder and Illness Severity. J. Psychiatr. Res. 87, 23–29. doi:10.1016/j.jpsychires.2016.12.007
Evers, E. A., van der Veen, F. M., Fekkes, D., and Jolles, J. (2007). Serotonin and Cognitive Flexibility: Neuroimaging Studies into the Effect of Acute Tryptophan Depletion in Healthy Volunteers. Curr. Med. Chem. 14 (28), 2989–2995. doi:10.2174/092986707782794032
Evrensel, A., Ünsalver, B. Ö., and Ceylan, M. E. (2019). Psychobiotics. Adv. Exp. Med. Biol. 1192, 565–581. doi:10.1007/978-981-32-9721-0_28
Faggioni, R., Fuller, J., Moser, A., Feingold, K. R., and Grunfeld, C. (1997). LPS-induced Anorexia in Leptin-Deficient (Ob/ob) and Leptin Receptor-Deficient (Db/db) Mice. Am. J. Physiol. 273 (1 Pt 2), R181–R186. doi:10.1152/ajpregu.1997.273.1.R181
Fang, X., Wang, Y., Chen, Y., Ren, J., and Zhang, C. (2019). Association between IL-6 and Metabolic Syndrome in Schizophrenia Patients Treated with Second-Generation Antipsychotics. Neuropsychiatr. Dis. Treat. 15, 2161–2170. doi:10.2147/ndt.S202159
Feng, X., Valdearcos, M., Uchida, Y., Lutrin, D., Maze, M., and Koliwad, S. K. (2017). Microglia Mediate Postoperative Hippocampal Inflammation and Cognitive Decline in Mice. JCI Insight 2 (7), e91229. doi:10.1172/jci.insight.91229
Flintoff, J., Kesby, J. P., Siskind, D., and Burne, T. H. (2021). Treating Cognitive Impairment in Schizophrenia with GLP-1RAs: an Overview of Their Therapeutic Potential. Expert Opin. Investig. Drugs 30 (8), 877–891. doi:10.1080/13543784.2021.1951702
Flowers, S. A., Baxter, N. T., Ward, K. M., Kraal, A. Z., McInnis, M. G., Schmidt, T. M., et al. (2019). Effects of Atypical Antipsychotic Treatment and Resistant Starch Supplementation on Gut Microbiome Composition in a Cohort of Patients with Bipolar Disorder or Schizophrenia. Pharmacotherapy 39 (2), 161–170. doi:10.1002/phar.2214
Flowers, S. A., Evans, S. J., Ward, K. M., McInnis, M. G., and Ellingrod, V. L. (2017). Interaction between Atypical Antipsychotics and the Gut Microbiome in a Bipolar Disease Cohort. Pharmacotherapy 37 (3), 261–267. doi:10.1002/phar.1890
Flowers, S. A., Ryan, K. A., Lai, Z., McInnis, M. G., and Ellingrod, V. L. (2016). Interaction between COMT Rs5993883 and Second Generation Antipsychotics Is Linked to Decreases in Verbal Cognition and Cognitive Control in Bipolar Disorder. BMC Psychol. 4, 14. doi:10.1186/s40359-016-0118-3
Forsythe, P., Bienenstock, J., and Kunze, W. A. (2014). Vagal Pathways for Microbiome-Brain-Gut axis Communication. Adv. Exp. Med. Biol. 817, 115–133. doi:10.1007/978-1-4939-0897-4_5
Fröhlich, E. E., Farzi, A., Mayerhofer, R., Reichmann, F., Jačan, A., Wagner, B., et al. (2016). Cognitive Impairment by Antibiotic-Induced Gut Dysbiosis: Analysis of Gut Microbiota-Brain Communication. Brain Behav. Immun. 56, 140–155. doi:10.1016/j.bbi.2016.02.020
Fung, T. C., Olson, C. A., and Hsiao, E. Y. (2017). Interactions between the Microbiota, Immune and Nervous Systems in Health and Disease. Nat. Neurosci. 20 (2), 145–155. doi:10.1038/nn.4476
Gareau, M. G., Wine, E., Rodrigues, D. M., Cho, J. H., Whary, M. T., Philpott, D. J., et al. (2011). Bacterial Infection Causes Stress-Induced Memory Dysfunction in Mice. Gut 60 (3), 307–317. doi:10.1136/gut.2009.202515
Gaykema, R. P., Goehler, L. E., and Lyte, M. (2004). Brain Response to Cecal Infection with Campylobacter Jejuni: Analysis with Fos Immunohistochemistry. Brain Behav. Immun. 18 (3), 238–245. doi:10.1016/j.bbi.2003.08.002
Gitlin, M. J., and Miklowitz, D. J. (2017). The Difficult Lives of Individuals with Bipolar Disorder: A Review of Functional Outcomes and Their Implications for Treatment. J. Affect Disord. 209, 147–154. doi:10.1016/j.jad.2016.11.021
Goldstein, B. I., Liu, S. M., Zivkovic, N., Schaffer, A., Chien, L. C., and Blanco, C. (2011). The Burden of Obesity Among Adults with Bipolar Disorder in the United States. Bipolar Disord. 13 (4), 387–395. doi:10.1111/j.1399-5618.2011.00932.x
Gomes, A. C., Hoffmann, C., and Mota, J. F. (2018). The Human Gut Microbiota: Metabolism and Perspective in Obesity. Gut Microbes 9 (4), 308–325. doi:10.1080/19490976.2018.1465157
González, H. F. J., Yengo-Kahn, A., and Englot, D. J. (2019). Vagus Nerve Stimulation for the Treatment of Epilepsy. Neurosurg. Clin. N. Am. 30 (2), 219–230. doi:10.1016/j.nec.2018.12.005
Govindarajan, N., Agis-Balboa, R. C., Walter, J., Sananbenesi, F., and Fischer, A. (2011). Sodium Butyrate Improves Memory Function in an Alzheimer's Disease Mouse Model when Administered at an Advanced Stage of Disease Progression. J. Alzheimers Dis. 26 (1), 187–197. doi:10.3233/jad-2011-110080
Haba, R., Shintani, N., Onaka, Y., Wang, H., Takenaga, R., Hayata, A., et al. (2012). Lipopolysaccharide Affects Exploratory Behaviors toward Novel Objects by Impairing Cognition And/or Motivation in Mice: Possible Role of Activation of the Central Amygdala. Behav. Brain Res. 228 (2), 423–431. doi:10.1016/j.bbr.2011.12.027
Harmer, C. J., and Cowen, P. J. (2013). 'It's the Way that You Look at It'-Aa Cognitive Neuropsychological Account of SSRI Action in Depression. Philos. Trans. R. Soc. Lond B Biol. Sci. 368 (1615), 20120407. doi:10.1098/rstb.2012.0407
Hartstra, A. V., Schüppel, V., Imangaliyev, S., Schrantee, A., Prodan, A., Collard, D., et al. (2020). Infusion of Donor Feces Affects the Gut-Brain axis in Humans with Metabolic Syndrome. Mol. Metab. 42, 101076. doi:10.1016/j.molmet.2020.101076
He, H., Chen, Z., Chen, D., Lu, X., Huang, C., and Chen, J. (2021). Identification of the Expression of Farnesoid X Receptor in Astrocytes. Neuroreport 32 (14), 1216–1222. doi:10.1097/wnr.0000000000001717
Hebb, A. L., Poulin, J. F., Roach, S. P., Zacharko, R. M., and Drolet, G. (2005). Cholecystokinin and Endogenous Opioid Peptides: Interactive Influence on Pain, Cognition, and Emotion. Prog. Neuropsychopharmacol. Biol. Psychiatry 29 (8), 1225–1238. doi:10.1016/j.pnpbp.2005.08.008
Heiss, C. N., and Olofsson, L. E. (2018). Gut Microbiota-dependent Modulation of Energy Metabolism. J. Innate Immun. 10 (3), 163–171. doi:10.1159/000481519
Henneberger, C., Papouin, T., Oliet, S. H., and Rusakov, D. A. (2010). Long-term Potentiation Depends on Release of D-Serine from Astrocytes. Nature 463 (7278), 232–236. doi:10.1038/nature08673
Heppner, K. M., Kirigiti, M., Secher, A., Paulsen, S. J., Buckingham, R., Pyke, C., et al. (2015). Expression and Distribution of Glucagon-like Peptide-1 Receptor mRNA, Protein and Binding in the Male Nonhuman Primate (Macaca mulatta) Brain. Endocrinology 156 (1), 255–267. doi:10.1210/en.2014-1675
Hinton, R. (2020). A Case Report Looking at the Effects of Faecal Microbiota Transplantation in a Patient with Bipolar Disorder. Aust. N. Z. J. Psychiatry 54 (6), 649–650. doi:10.1177/0004867420912834
Hoban, A. E., Moloney, R. D., Golubeva, A. V., McVey Neufeld, K. A., O'Sullivan, O., Patterson, E., et al. (2016a). Behavioural and Neurochemical Consequences of Chronic Gut Microbiota Depletion during Adulthood in the Rat. Neuroscience 339, 463–477. doi:10.1016/j.neuroscience.2016.10.003
Hoban, A. E., Stilling, R. M., Ryan, F. J., Shanahan, F., Dinan, T. G., Claesson, M. J., et al. (2016b). Regulation of Prefrontal Cortex Myelination by the Microbiota. Transl. Psychiatry 6, e774. doi:10.1038/tp.2016.42
Holt, M. K., and Trapp, S. (2016). The Physiological Role of the Brain GLP-1 System in Stress. Cogent Biol. 2 (1), 1229086. doi:10.1080/23312025.2016.1229086
Holzer, P. (2016). Neuropeptides, Microbiota, and Behavior. Int. Rev. Neurobiol. 131, 67–89. doi:10.1016/bs.irn.2016.08.005
Hoseth, E. Z., Westlye, L. T., Hope, S., Dieset, I., Aukrust, P., Melle, I., et al. (2016). Association between Cytokine Levels, Verbal Memory and hippocampus Volume in Psychotic Disorders and Healthy Controls. Acta Psychiatr. Scand. 133 (1), 53–62. doi:10.1111/acps.12467
Houser, M. C., and Tansey, M. G. (2017). The Gut-Brain axis: Is Intestinal Inflammation a Silent Driver of Parkinson's Disease Pathogenesis? NPJ Park. Dis. 3, 3. doi:10.1038/s41531-016-0002-0
Hu, L., Zhu, S., Peng, X., Li, K., Peng, W., Zhong, Y., et al. (2020). High Salt Elicits Brain Inflammation and Cognitive Dysfunction, Accompanied by Alternations in the Gut Microbiota and Decreased SCFA Production. J. Alzheimers Dis. 77 (2), 629–640. doi:10.3233/jad-200035
Hu, S., Li, A., Huang, T., Lai, J., Li, J., Sublette, M. E., et al. (2019). Gut Microbiota Changes in Patients with Bipolar Depression. Adv. Sci. (Weinh) 6 (14), 1900752. doi:10.1002/advs.201900752
Hua, D., Li, S., Li, S., Wang, X., Wang, Y., Xie, Z., et al. (2021). Gut Microbiome and Plasma Metabolome Signatures in Middle-Aged Mice with Cognitive Dysfunction Induced by Chronic Neuropathic Pain. Front. Mol. Neurosci. 14, 806700. doi:10.3389/fnmol.2021.806700
Huang, C., Wang, J., Hu, W., Wang, C., Lu, X., Tong, L., et al. (2016). Identification of Functional Farnesoid X Receptors in Brain Neurons. FEBS Lett. 590 (18), 3233–3242. doi:10.1002/1873-3468.12373
Huang, S., Hu, S., Liu, S., Tang, B., Liu, Y., Tang, L., et al. (2022). Lithium Carbonate Alleviates Colon Inflammation through Modulating Gut Microbiota and Treg Cells in a GPR43-dependent Manner. Pharmacol. Res. 175, 105992. doi:10.1016/j.phrs.2021.105992
Huber, R. S., Kondo, D. G., Shi, X. F., Prescot, A. P., Clark, E., Renshaw, P. F., et al. (2018). Relationship of Executive Functioning Deficits to N-Acetyl Aspartate (NAA) and Gamma-Aminobutyric Acid (GABA) in Youth with Bipolar Disorder. J. Affect Disord. 225, 71–78. doi:10.1016/j.jad.2017.07.052
Jia, S., Lu, Z., Gao, Z., An, J., Wu, X., Li, X., et al. (2016). Chitosan Oligosaccharides Alleviate Cognitive Deficits in an Amyloid-Β1-42-Induced Rat Model of Alzheimer's Disease. Int. J. Biol. Macromol. 83, 416–425. doi:10.1016/j.ijbiomac.2015.11.011
Jiang, C., Li, G., Huang, P., Liu, Z., and Zhao, B. (2017). The Gut Microbiota and Alzheimer's Disease. J. Alzheimers Dis. 58 (1), 1–15. doi:10.3233/jad-161141
Kao, A. C., Burnet, P. W. J., and Lennox, B. R. (2018). Can Prebiotics Assist in the Management of Cognition and Weight Gain in Schizophrenia? Psychoneuroendocrinology 95, 179–185. doi:10.1016/j.psyneuen.2018.05.027
Kawase, T., Nagasawa, M., Ikeda, H., Yasuo, S., Koga, Y., and Furuse, M. (2017). Gut Microbiota of Mice Putatively Modifies Amino Acid Metabolism in the Host Brain. Br. J. Nutr. 117 (6), 775–783. doi:10.1017/s0007114517000678
Kesika, P., Suganthy, N., Sivamaruthi, B. S., and Chaiyasut, C. (2021). Role of Gut-Brain axis, Gut Microbial Composition, and Probiotic Intervention in Alzheimer's Disease. Life Sci. 264, 118627. doi:10.1016/j.lfs.2020.118627
Kilkens, T. O., Honig, A., van Nieuwenhoven, M. A., Riedel, W. J., and Brummer, R. J. (2004). Acute Tryptophan Depletion Affects Brain-Gut Responses in Irritable Bowel Syndrome Patients and Controls. Gut 53 (12), 1794–1800. doi:10.1136/gut.2004.041657
Kim, H. J., Leeds, P., and Chuang, D. M. (2009). The HDAC Inhibitor, Sodium Butyrate, Stimulates Neurogenesis in the Ischemic Brain. J. Neurochem. 110 (4), 1226–1240. doi:10.1111/j.1471-4159.2009.06212.x
Kim, J. S., Kirkland, R. A., Lee, S. H., Cawthon, C. R., Rzepka, K. W., Minaya, D. M., et al. (2020). Gut Microbiota Composition Modulates Inflammation and Structure of the Vagal Afferent Pathway. Physiol. Behav. 225, 113082. doi:10.1016/j.physbeh.2020.113082
Kondoh, T., Mallick, H. N., and Torii, K. (2009). Activation of the Gut-Brain axis by Dietary Glutamate and Physiologic Significance in Energy Homeostasis. Am. J. Clin. Nutr. 90 (3), 832s–837s. doi:10.3945/ajcn.2009.27462V
Kothari, V., Luo, Y., Tornabene, T., O'Neill, A. M., Greene, M. W., Geetha, T., et al. (2017). High Fat Diet Induces Brain Insulin Resistance and Cognitive Impairment in Mice. Biochim. Biophys. Acta Mol. Basis Dis. 1863 (2), 499–508. doi:10.1016/j.bbadis.2016.10.006
Kubota, N., Yano, W., Kubota, T., Yamauchi, T., Itoh, S., Kumagai, H., et al. (2007). Adiponectin Stimulates AMP-Activated Protein Kinase in the Hypothalamus and Increases Food Intake. Cell Metab. 6 (1), 55–68. doi:10.1016/j.cmet.2007.06.003
Lai, J., Jiang, J., Zhang, P., Xi, C., Wu, L., Gao, X., et al. (2020). Gut Microbial Clues to Bipolar Disorder: State-Of-The-Art Review of Current Findings and Future Directions. Clin. Transl. Med. 10 (4), e146. doi:10.1002/ctm2.146
Lai, J., Zhang, P., Jiang, J., Mou, T., Li, Y., Xi, C., et al. (2021a). New Evidence of Gut Microbiota Involvement in the Neuropathogenesis of Bipolar Depression by TRANK1 Modulation: Joint Clinical and Animal Data. Front. Immunol. 12, 789647. doi:10.3389/fimmu.2021.789647
Lai, W. T., Zhao, J., Xu, S. X., Deng, W. F., Xu, D., Wang, M. B., et al. (2021b). Shotgun Metagenomics Reveals Both Taxonomic and Tryptophan Pathway Differences of Gut Microbiota in Bipolar Disorder with Current Major Depressive Episode Patients. J. Affect Disord. 278, 311–319. doi:10.1016/j.jad.2020.09.010
Lamas, B., Richard, M. L., Leducq, V., Pham, H. P., Michel, M. L., Da Costa, G., et al. (2016). CARD9 Impacts Colitis by Altering Gut Microbiota Metabolism of Tryptophan into Aryl Hydrocarbon Receptor Ligands. Nat. Med. 22 (6), 598–605. doi:10.1038/nm.4102
Leblhuber, F., Steiner, K., Schuetz, B., Fuchs, D., and Gostner, J. M. (2018). Probiotic Supplementation in Patients with Alzheimer's Dementia - an Explorative Intervention Study. Curr. Alzheimer Res. 15 (12), 1106–1113. doi:10.2174/1389200219666180813144834
Leclercq, S., Le Roy, T., Furgiuele, S., Coste, V., Bindels, L. B., Leyrolle, Q., et al. (2020). Gut Microbiota-Induced Changes in β-Hydroxybutyrate Metabolism Are Linked to Altered Sociability and Depression in Alcohol Use Disorder. Cell Rep. 33 (2), 108238. doi:10.1016/j.celrep.2020.108238
Lee, J., Venna, V. R., Durgan, D. J., Shi, H., Hudobenko, J., Putluri, N., et al. (2020a). Young versus Aged Microbiota Transplants to Germ-free Mice: Increased Short-Chain Fatty Acids and Improved Cognitive Performance. Gut Microbes 12 (1), 1–14. doi:10.1080/19490976.2020.1814107
Lee, K. E., Kim, J. K., Han, S. K., Lee, D. Y., Lee, H. J., Yim, S. V., et al. (2020b). The Extracellular Vesicle of Gut Microbial Paenalcaligenes Hominis Is a Risk Factor for Vagus Nerve-Mediated Cognitive Impairment. Microbiome 8 (1), 107. doi:10.1186/s40168-020-00881-2
Leonel, A. J., and Alvarez-Leite, J. I. (2012). Butyrate: Implications for Intestinal Function. Curr. Opin. Clin. Nutr. Metab. Care 15 (5), 474–479. doi:10.1097/MCO.0b013e32835665fa
Levenson, J. M., O'Riordan, K. J., Brown, K. D., Trinh, M. A., Molfese, D. L., and Sweatt, J. D. (2004). Regulation of Histone Acetylation during Memory Formation in the hippocampus. J. Biol. Chem. 279 (39), 40545–40559. doi:10.1074/jbc.M402229200
Li, D., Ke, Y., Zhan, R., Liu, C., Zhao, M., Zeng, A., et al. (2018). Trimethylamine-N-oxide Promotes Brain Aging and Cognitive Impairment in Mice. Aging Cell 17 (4), e12768. doi:10.1111/acel.12768
Li, H., Ni, J., and Qing, H. (2021). Gut Microbiota: Critical Controller and Intervention Target in Brain Aging and Cognitive Impairment. Front. Aging Neurosci. 13 (279), 671142. doi:10.3389/fnagi.2021.671142
Ling, Z., Zhu, M., Yan, X., Cheng, Y., Shao, L., Liu, X., et al. (2020). Structural and Functional Dysbiosis of Fecal Microbiota in Chinese Patients with Alzheimer's Disease. Front. Cell Dev. Biol. 8, 634069. doi:10.3389/fcell.2020.634069
Liu, F., Horton-Sparks, K., Hull, V., Li, R. W., and Martínez-Cerdeño, V. (2018). The Valproic Acid Rat Model of Autism Presents with Gut Bacterial Dysbiosis Similar to that in Human Autism. Mol. Autism 9, 61. doi:10.1186/s13229-018-0251-3
Liu, J., Sun, J., Wang, F., Yu, X., Ling, Z., Li, H., et al. (2015). Neuroprotective Effects of Clostridium Butyricum against Vascular Dementia in Mice via Metabolic Butyrate. Biomed. Res. Int. 2015, 412946. doi:10.1155/2015/412946
Liu, Z., Dai, X., Zhang, H., Shi, R., Hui, Y., Jin, X., et al. (2020). Gut Microbiota Mediates Intermittent-Fasting Alleviation of Diabetes-Induced Cognitive Impairment. Nat. Commun. 11 (1), 855. doi:10.1038/s41467-020-14676-4
Lu, J., Synowiec, S., Lu, L., Yu, Y., Bretherick, T., Takada, S., et al. (2018). Microbiota Influence the Development of the Brain and Behaviors in C57BL/6J Mice. PLoS One 13 (8), e0201829. doi:10.1371/journal.pone.0201829
Luck, B., Horvath, T. D., Engevik, K. A., Ruan, W., Haidacher, S. J., Hoch, K. M., et al. (2021). Neurotransmitter Profiles Are Altered in the Gut and Brain of Mice Mono-Associated with Bifidobacterium Dentium. Biomolecules 11 (8), 1091. doi:10.3390/biom11081091
Luczynski, P., Whelan, S. O., O'Sullivan, C., Clarke, G., Shanahan, F., Dinan, T. G., et al. (2016). Adult Microbiota-Deficient Mice Have Distinct Dendritic Morphological Changes: Differential Effects in the Amygdala and hippocampus. Eur. J. Neurosci. 44 (9), 2654–2666. doi:10.1111/ejn.13291
Machado-Vieira, R., Ibrahim, L., and Zarate, C. A. (2011). Histone Deacetylases and Mood Disorders: Epigenetic Programming in Gene-Environment Interactions. CNS Neurosci. Ther. 17 (6), 699–704. doi:10.1111/j.1755-5949.2010.00203.x
Magne, F., Gotteland, M., Gauthier, L., Zazueta, A., Pesoa, S., Navarrete, P., et al. (2020). The Firmicutes/Bacteroidetes Ratio: A Relevant Marker of Gut Dysbiosis in Obese Patients? Nutrients 12 (5). doi:10.3390/nu12051474
MahmoudianDehkordi, S., Arnold, M., Nho, K., Ahmad, S., Jia, W., Xie, G., et al. (2019). Altered Bile Acid Profile Associates with Cognitive Impairment in Alzheimer's Disease-An Emerging Role for Gut Microbiome. Alzheimers Dement. 15 (1), 76–92. doi:10.1016/j.jalz.2018.07.217
Maletic, V., and Raison, C. (2014). Integrated Neurobiology of Bipolar Disorder. Front. Psychiatry 5, 98. doi:10.3389/fpsyt.2014.00098
Mansur, R. B., Ahmed, J., Cha, D. S., Woldeyohannes, H. O., Subramaniapillai, M., Lovshin, J., et al. (2017). Liraglutide Promotes Improvements in Objective Measures of Cognitive Dysfunction in Individuals with Mood Disorders: A Pilot, Open-Label Study. J. Affect Disord. 207, 114–120. doi:10.1016/j.jad.2016.09.056
Mansur, R. B., Subramaniapillai, M., Lee, Y., Pan, Z., Carmona, N. E., Shekotikhina, M., et al. (2020). Leptin Mediates Improvements in Cognitive Function Following Treatment with Infliximab in Adults with Bipolar Depression. Psychoneuroendocrinology 120, 104779. doi:10.1016/j.psyneuen.2020.104779
Marizzoni, M., Cattaneo, A., Mirabelli, P., Festari, C., Lopizzo, N., Nicolosi, V., et al. (2020). Short-Chain Fatty Acids and Lipopolysaccharide as Mediators between Gut Dysbiosis and Amyloid Pathology in Alzheimer's Disease. J. Alzheimers Dis. 78 (2), 683–697. doi:10.3233/jad-200306
Martínez-Lapiscina, E. H., Clavero, P., Toledo, E., Estruch, R., Salas-Salvadó, J., San Julián, B., et al. (2013). Mediterranean Diet Improves Cognition: the PREDIMED-NAVARRA Randomised Trial. J. Neurol. Neurosurg. Psychiatry 84 (12), 1318–1325. doi:10.1136/jnnp-2012-304792
Matsumoto, M., Kibe, R., Ooga, T., Aiba, Y., Sawaki, E., Koga, Y., et al. (2013). Cerebral Low-Molecular Metabolites Influenced by Intestinal Microbiota: a Pilot Study. Front. Syst. Neurosci. 7, 9. doi:10.3389/fnsys.2013.00009
McAllister-Williams, R. H., Sousa, S., Kumar, A., Greco, T., Bunker, M. T., Aaronson, S. T., et al. (2020). The Effects of Vagus Nerve Stimulation on the Course and Outcomes of Patients with Bipolar Disorder in a Treatment-Resistant Depressive Episode: a 5-year Prospective Registry. Int. J. Bipolar Disord. 8 (1), 13. doi:10.1186/s40345-020-0178-4
McEvoy, J. P., Meyer, J. M., Goff, D. C., Nasrallah, H. A., Davis, S. M., Sullivan, L., et al. (2005). Prevalence of the Metabolic Syndrome in Patients with Schizophrenia: Baseline Results from the Clinical Antipsychotic Trials of Intervention Effectiveness (CATIE) Schizophrenia Trial and Comparison with National Estimates from NHANES III. Schizophr. Res. 80 (1), 19–32. doi:10.1016/j.schres.2005.07.014
McGrattan, A. M., McGuinness, B., McKinley, M. C., Kee, F., Passmore, P., Woodside, J. V., et al. (2019). Diet and Inflammation in Cognitive Ageing and Alzheimer's Disease. Curr. Nutr. Rep. 8 (2), 53–65. doi:10.1007/s13668-019-0271-4
McIntyre, R. S., Subramaniapillai, M., Shekotikhina, M., Carmona, N. E., Lee, Y., Mansur, R. B., et al. (2021). Characterizing the Gut Microbiota in Adults with Bipolar Disorder: a Pilot Study. Nutr. Neurosci. 24 (3), 173–180. doi:10.1080/1028415x.2019.1612555
McVey Neufeld, K. A., Bienenstock, J., Bharwani, A., Champagne-Jorgensen, K., Mao, Y., West, C., et al. (2019). Oral Selective Serotonin Reuptake Inhibitors Activate Vagus Nerve Dependent Gut-Brain Signalling. Sci. Rep. 9 (1), 14290. doi:10.1038/s41598-019-50807-8
Miller, C. L., Llenos, I. C., Dulay, J. R., and Weis, S. (2006). Upregulation of the Initiating Step of the Kynurenine Pathway in Postmortem Anterior Cingulate Cortex from Individuals with Schizophrenia and Bipolar Disorder. Brain Res. 1073-1074, 25–37. doi:10.1016/j.brainres.2005.12.056
Misiak, B., Łoniewski, I., Marlicz, W., Frydecka, D., Szulc, A., Rudzki, L., et al. (2020). The HPA axis Dysregulation in Severe Mental Illness: Can We Shift the Blame to Gut Microbiota? Prog. Neuropsychopharmacol. Biol. Psychiatry 102, 109951. doi:10.1016/j.pnpbp.2020.109951
Möhle, L., Mattei, D., Heimesaat, M. M., Bereswill, S., Fischer, A., Alutis, M., et al. (2016). Ly6C(hi) Monocytes Provide a Link between Antibiotic-Induced Changes in Gut Microbiota and Adult Hippocampal Neurogenesis. Cell Rep. 15 (9), 1945–1956. doi:10.1016/j.celrep.2016.04.074
Mondelli, V., Cattaneo, A., Murri, M. B., Di Forti, M., Handley, R., Hepgul, N., et al. (2011). Stress and Inflammation Reduce Brain-Derived Neurotrophic Factor Expression in First-Episode Psychosis: a Pathway to Smaller Hippocampal Volume. J. Clin. Psychiatry 72 (12), 1677–1684. doi:10.4088/JCP.10m06745
Moolamalla, S. T. R., and Vinod, P. K. (2020). Genome-scale Metabolic Modelling Predicts Biomarkers and Therapeutic Targets for Neuropsychiatric Disorders. Comput. Biol. Med. 125, 103994. doi:10.1016/j.compbiomed.2020.103994
Morris, G., Maes, M., Berk, M., Carvalho, A. F., and Puri, B. K. (2020). Nutritional Ketosis as an Intervention to Relieve Astrogliosis: Possible Therapeutic Applications in the Treatment of Neurodegenerative and Neuroprogressive Disorders. Eur. Psychiatry 63 (1), e8. doi:10.1192/j.eurpsy.2019.13
Mulak, A., and Bonaz, B. (2015). Brain-gut-microbiota axis in Parkinson's Disease. World J. Gastroenterol. 21 (37), 10609–10620. doi:10.3748/wjg.v21.i37.10609
Müller, D. J., Zai, C. C., Shinkai, T., Strauss, J., and Kennedy, J. L. (2011). Association between the DAOA/G72 Gene and Bipolar Disorder and Meta-Analyses in Bipolar Disorder and Schizophrenia. Bipolar Disord. 13 (2), 198–207. doi:10.1111/j.1399-5618.2011.00905.x
Müller, T. D., Finan, B., Bloom, S. R., D'Alessio, D., Drucker, D. J., Flatt, P. R., et al. (2019). Glucagon-like Peptide 1 (GLP-1). Mol. Metab. 30, 72–130. doi:10.1016/j.molmet.2019.09.010
Myint, A. M., Kim, Y. K., Verkerk, R., Park, S. H., Scharpé, S., Steinbusch, H. W., et al. (2007). Tryptophan Breakdown Pathway in Bipolar Mania. J. Affect Disord. 102 (1-3), 65–72. doi:10.1016/j.jad.2006.12.008
Nagpal, R., Neth, B. J., Wang, S., Mishra, S. P., Craft, S., and Yadav, H. (2020). Gut Mycobiome and its Interaction with Diet, Gut Bacteria and Alzheimer's Disease Markers in Subjects with Mild Cognitive Impairment: A Pilot Study. EBioMedicine 59, 102950. doi:10.1016/j.ebiom.2020.102950
Nagpal, R., Newman, T. M., Wang, S., Jain, S., Lovato, J. F., and Yadav, H. (20182018). Obesity-Linked Gut Microbiome Dysbiosis Associated with Derangements in Gut Permeability and Intestinal Cellular Homeostasis Independent of Diet. J. Diabetes Res. 2018, 3462092. doi:10.1155/2018/3462092
Ning, X., Xia, L., Shi, Y., Hao, R., Geng, F., Zhang, K., et al. (2021). Cognitive Improvement Function of Aripiprazole in Adolescents with First-Episode and Repeated-Episode Schizophrenia. Asian J. Psychiatr. 58, 102598. doi:10.1016/j.ajp.2021.102598
Nuzum, N. D., Loughman, A., Szymlek-Gay, E. A., Hendy, A., Teo, W. P., and Macpherson, H. (2020). Gut Microbiota Differences between Healthy Older Adults and Individuals with Parkinson's Disease: A Systematic Review. Neurosci. Biobehav Rev. 112, 227–241. doi:10.1016/j.neubiorev.2020.02.003
Olson, C. A., Vuong, H. E., Yano, J. M., Liang, Q. Y., Nusbaum, D. J., and Hsiao, E. Y. (2018). The Gut Microbiota Mediates the Anti-Seizure Effects of the Ketogenic Diet. Cell 173 (7), 1728–1741. doi:10.1016/j.cell.2018.04.027
Otaru, N., Ye, K., Mujezinovic, D., Berchtold, L., Constancias, F., Cornejo, F. A., et al. (2021). GABA Production by Human Intestinal Bacteroides spp.: Prevalence, Regulation, and Role in Acid Stress Tolerance. Front. Microbiol. 12, 656895. doi:10.3389/fmicb.2021.656895
Ott, C. V., Knorr, U., Jespersen, A., Obenhausen, K., Røen, I., Purdon, S. E., et al. (2021). Norms for the Screen for Cognitive Impairment in Psychiatry and Cognitive Trajectories in Bipolar Disorder. J. Affect Disord. 281, 33–40. doi:10.1016/j.jad.2020.11.119
Palomo-Buitrago, M. E., Sabater-Masdeu, M., Moreno-Navarrete, J. M., Caballano-Infantes, E., Arnoriaga-Rodríguez, M., Coll, C., et al. (2019). Glutamate Interactions with Obesity, Insulin Resistance, Cognition and Gut Microbiota Composition. Acta Diabetol. 56 (5), 569–579. doi:10.1007/s00592-019-01313-w
Pearson-Leary, J., Zhao, C., Bittinger, K., Eacret, D., Luz, S., Vigderman, A. S., et al. (2020). The Gut Microbiome Regulates the Increases in Depressive-type Behaviors and in Inflammatory Processes in the Ventral hippocampus of Stress Vulnerable Rats. Mol. Psychiatry 25 (5), 1068–1079. doi:10.1038/s41380-019-0380-x
Peitl, V., Štefanović, M., Orlović, I., Culej, J., Rendulić, A., Matešić, K., et al. (2021). Long Acting Aripiprazole Influences Cognitive Functions in Recent Onset Schizophrenia. Psychopharmacol. Berl. 238 (6), 1563–1573. doi:10.1007/s00213-021-05788-w
Perez, L. (2018). The Role of Dietary Patterns in Mood Disorders: Prospective Research in Youth Populations. Am. J. Lifestyle Med. 12 (4), 286–290. doi:10.1177/1559827618765937
Pirozzi, C., Francisco, V., Guida, F. D., Gómez, R., Lago, F., Pino, J., et al. (2018). Butyrate Modulates Inflammation in Chondrocytes via GPR43 Receptor. Cell Physiol. Biochem. 51 (1), 228–243. doi:10.1159/000495203
Pistollato, F., Iglesias, R. C., Ruiz, R., Aparicio, S., Crespo, J., Lopez, L. D., et al. (2018). Nutritional Patterns Associated with the Maintenance of Neurocognitive Functions and the Risk of Dementia and Alzheimer's Disease: A Focus on Human Studies. Pharmacol. Res. 131, 32–43. doi:10.1016/j.phrs.2018.03.012
Plagman, A., Hoscheidt, S., McLimans, K. E., Klinedinst, B., Pappas, C., Anantharam, V., et al. (2019). Cholecystokinin and Alzheimer's Disease: a Biomarker of Metabolic Function, Neural Integrity, and Cognitive Performance. Neurobiol. Aging 76, 201–207. doi:10.1016/j.neurobiolaging.2019.01.002
Platzer, M., Fellendorf, F. T., Bengesser, S. A., Birner, A., Dalkner, N., Hamm, C., et al. (2019). Adiponectin Is Decreased in Bipolar Depression. World J. Biol. Psychiatry 20 (10), 813–820. doi:10.1080/15622975.2018.1500033
Potter, M. C., Elmer, G. I., Bergeron, R., Albuquerque, E. X., Guidetti, P., Wu, H. Q., et al. (2010). Reduction of Endogenous Kynurenic Acid Formation Enhances Extracellular Glutamate, Hippocampal Plasticity, and Cognitive Behavior. Neuropsychopharmacology 35 (8), 1734–1742. doi:10.1038/npp.2010.39
Quigley, E. M. M. (2017). Microbiota-Brain-Gut Axis and Neurodegenerative Diseases. Curr. Neurol. Neurosci. Rep. 17 (12), 94. doi:10.1007/s11910-017-0802-6
Radkov, A. D., and Moe, L. A. (2014). Bacterial Synthesis of D-Amino Acids. Appl. Microbiol. Biotechnol. 98 (12), 5363–5374. doi:10.1007/s00253-014-5726-3
Rao, J. S., Harry, G. J., Rapoport, S. I., and Kim, H. W. (2010). Increased Excitotoxicity and Neuroinflammatory Markers in Postmortem Frontal Cortex from Bipolar Disorder Patients. Mol. Psychiatry 15 (4), 384–392. doi:10.1038/mp.2009.47
Raybould, H. E. (2007). Mechanisms of CCK Signaling from Gut to Brain. Curr. Opin. Pharmacol. 7 (6), 570–574. doi:10.1016/j.coph.2007.09.006
Reddy-Thootkur, M., Kraguljac, N. V., and Lahti, A. C. (2020). The Role of Glutamate and GABA in Cognitive Dysfunction in Schizophrenia and Mood Disorders - A Systematic Review of Magnetic Resonance Spectroscopy Studies. Schizophrenia Res., S0920-9964(20)30077-3. doi:10.1016/j.schres.2020.02.001
Reininghaus, E. Z., Wetzlmair, L. C., Fellendorf, F. T., Platzer, M., Queissner, R., Birner, A., et al. (2018). The Impact of Probiotic Supplements on Cognitive Parameters in Euthymic Individuals with Bipolar Disorder: A Pilot Study. Neuropsychobiology, 1–8. doi:10.1159/000492537
Rizzo, M. R., Fasano, R., and Paolisso, G. (2020). Adiponectin and Cognitive Decline. Int. J. Mol. Sci. 21 (6). doi:10.3390/ijms21062010
Rong, H., Xie, X. H., Zhao, J., Lai, W. T., Wang, M. B., Xu, D., et al. (2019). Similarly in Depression, Nuances of Gut Microbiota: Evidences from a Shotgun Metagenomics Sequencing Study on Major Depressive Disorder versus Bipolar Disorder with Current Major Depressive Episode Patients. J. Psychiatr. Res. 113, 90–99. doi:10.1016/j.jpsychires.2019.03.017
Rosenblat, J. D., Mansur, R. B., Brietzke, E., Kennedy, S. H., Carvalho, A. F., Lee, Y., et al. (2020). Association of History of Adverse Childhood Experiences with Irritable Bowel Syndrome (IBS) in Individuals with Mood Disorders. Psychiatry Res. 288, 112967. doi:10.1016/j.psychres.2020.112967
Rosso, G., Cattaneo, A., Zanardini, R., Gennarelli, M., Maina, G., and Bocchio-Chiavetto, L. (2015). Glucose Metabolism Alterations in Patients with Bipolar Disorder. J. Affect Disord. 184, 293–298. doi:10.1016/j.jad.2015.06.006
Rothhammer, V., Mascanfroni, I. D., Bunse, L., Takenaka, M. C., Kenison, J. E., Mayo, L., et al. (2016). Type I Interferons and Microbial Metabolites of Tryptophan Modulate Astrocyte Activity and Central Nervous System Inflammation via the Aryl Hydrocarbon Receptor. Nat. Med. 22 (6), 586–597. doi:10.1038/nm.4106
Rubio, C., Lizárraga, E., Álvarez-Cilleros, D., Pérez-Pardo, P., Sanmartín-Salinas, P., Toledo-Lobo, M. V., et al. (2021). Aging in Male Wistar Rats Associates with Changes in Intestinal Microbiota, Gut Structure, and Cholecystokinin-Mediated Gut-Brain Axis Function. J. Gerontol. A Biol. Sci. Med. Sci. 76 (11), 1915–1921. doi:10.1093/gerona/glaa313
Rudzki, L., and Szulc, A. (2018). "Immune Gate" of Psychopathology-The Role of Gut Derived Immune Activation in Major Psychiatric Disorders. Front. Psychiatry 9, 205. doi:10.3389/fpsyt.2018.00205
Sampson, T. R., Debelius, J. W., Thron, T., Janssen, S., Shastri, G. G., Ilhan, Z. E., et al. (2016). Gut Microbiota Regulate Motor Deficits and Neuroinflammation in a Model of Parkinson's Disease. Cell 167 (6), 1469–e12. e1412. doi:10.1016/j.cell.2016.11.018
Sarkar, A., Lehto, S. M., Harty, S., Dinan, T. G., Cryan, J. F., and Burnet, P. W. J. (2016). Psychobiotics and the Manipulation of Bacteria-Gut-Brain Signals. Trends Neurosci. 39 (11), 763–781. doi:10.1016/j.tins.2016.09.002
Schell, M. J. (2004). The N-Methyl D-Aspartate Receptor glycine Site and D-Serine Metabolism: an Evolutionary Perspective. Philos. Trans. R. Soc. Lond B Biol. Sci. 359 (1446), 943–964. doi:10.1098/rstb.2003.1399
Schirmer, M., Smeekens, S. P., Vlamakis, H., Jaeger, M., Oosting, M., Franzosa, E. A., et al. (2016). Linking the Human Gut Microbiome to Inflammatory Cytokine Production Capacity. Cell 167 (4), 1125–e8. e1128. doi:10.1016/j.cell.2016.10.020
Schmidt, D. R., Holmstrom, S. R., Fon Tacer, K., Bookout, A. L., Kliewer, S. A., and Mangelsdorf, D. J. (2010). Regulation of Bile Acid Synthesis by Fat-Soluble Vitamins A and D. J. Biol. Chem. 285 (19), 14486–14494. doi:10.1074/jbc.M110.116004
Scott, S. A., Fu, J., and Chang, P. V. (2020). Microbial Tryptophan Metabolites Regulate Gut Barrier Function via the Aryl Hydrocarbon Receptor. Proc. Natl. Acad. Sci. U. S. A. 117 (32), 19376–19387. doi:10.1073/pnas.2000047117
Sears, C., Wilson, J., and Fitches, A. (2013). Investigating the Role of BDNF and CCK System Genes in Suicidality in a Familial Bipolar Cohort. J. Affect Disord. 151 (2), 611–617. doi:10.1016/j.jad.2013.07.006
Severance, E. G., Gressitt, K. L., Stallings, C. R., Katsafanas, E., Schweinfurth, L. A., Savage, C. L., et al. (2016). Candida Albicans Exposures, Sex Specificity and Cognitive Deficits in Schizophrenia and Bipolar Disorder. NPJ Schizophr. 2, 16018. doi:10.1038/npjschz.2016.18
Sharon, G., Sampson, T. R., Geschwind, D. H., and Mazmanian, S. K. (2016). The Central Nervous System and the Gut Microbiome. Cell 167 (4), 915–932. doi:10.1016/j.cell.2016.10.027
Shi, H., Wang, Q., Zheng, M., Hao, S., Lum, J. S., Chen, X., et al. (2020). Supplement of Microbiota-Accessible Carbohydrates Prevents Neuroinflammation and Cognitive Decline by Improving the Gut Microbiota-Brain axis in Diet-Induced Obese Mice. J. Neuroinflammation 17 (1), 77. doi:10.1186/s12974-020-01760-1
Shi, L. L., Li, Y., Wang, Y., and Feng, Y. (2015). MDG-1, an Ophiopogon Polysaccharide, Regulate Gut Microbiota in High-Fat Diet-Induced Obese C57BL/6 Mice. Int. J. Biol. Macromol. 81, 576–583. doi:10.1016/j.ijbiomac.2015.08.057
Simjanoski, M., Azevedo, T. d., McIntyre, A., and Kapczinski, F. (2021). Cognitive Impairment in Bipolar Disorder in Comparison to Mild Cognitive Impairment and Dementia: a Systematic Review. Trends Psychiatry Psychother. doi:10.47626/2237-6089-2021-0300
Singh, N. S., Rutkowska, E., Plazinska, A., Khadeer, M., Moaddel, R., Jozwiak, K., et al. (2016). Ketamine Metabolites Enantioselectively Decrease Intracellular D-Serine Concentrations in PC-12 Cells. PLoS One 11 (4), e0149499. doi:10.1371/journal.pone.0149499
Sleiman, S. F., Henry, J., Al-Haddad, R., El Hayek, L., Abou Haidar, E., Stringer, T., et al. (2016). Exercise Promotes the Expression of Brain Derived Neurotrophic Factor (BDNF) through the Action of the Ketone Body β-hydroxybutyrate. Elife 5. doi:10.7554/eLife.15092
Song, X., Zhao, Z., Zhao, Y., Wang, Z., Wang, C., Yang, G., et al. (2021). Lactobacillus Plantarum DP189 Prevents Cognitive Dysfunction in D-galactose/AlCl3 Induced Mouse Model of Alzheimer's Disease via Modulating Gut Microbiota and PI3K/Akt/GSK-3β Signaling Pathway. Nutr. Neurosci., 1–13. doi:10.1080/1028415x.2021.1991556
Strandwitz, P. (2018). Neurotransmitter Modulation by the Gut Microbiota. Brain Res. 1693 (Pt B), 128–133. doi:10.1016/j.brainres.2018.03.015
Sublette, M. E., Cheung, S., Lieberman, E., Hu, S., Mann, J. J., Uhlemann, A. C., et al. (2021). Bipolar Disorder and the Gut Microbiome: A Systematic Review. Bipolar Disord. 23 (6), 544–564. doi:10.1111/bdi.13049
Sun, J., Xu, J., Yang, B., Chen, K., Kong, Y., Fang, N., et al. (2020). Effect of Clostridium Butyricum against Microglia-Mediated Neuroinflammation in Alzheimer's Disease via Regulating Gut Microbiota and Metabolites Butyrate. Mol. Nutr. Food Res. 64 (2), e1900636. doi:10.1002/mnfr.201900636
Taghzouti, K., Léna, I., Dellu, F., Roques, B. P., Daugé, V., and Simon, H. (1999). Cognitive Enhancing Effects in Young and Old Rats of pBC264, a Selective CCK(B) Receptor Agonist. Psychopharmacol. Berl. 143 (2), 141–149. doi:10.1007/s002130050929
Takada, M., Nishida, K., Kataoka-Kato, A., Gondo, Y., Ishikawa, H., Suda, K., et al. (2016). Probiotic Lactobacillus Casei Strain Shirota Relieves Stress-Associated Symptoms by Modulating the Gut-Brain Interaction in Human and Animal Models. Neurogastroenterol. Motil. 28 (7), 1027–1036. doi:10.1111/nmo.12804
Tan, A. H., Chong, C. W., Lim, S. Y., Yap, I. K. S., Teh, C. S. J., Loke, M. F., et al. (2021). Gut Microbial Ecosystem in Parkinson Disease: New Clinicobiological Insights from Multi-Omics. Ann. Neurol. 89 (3), 546–559. doi:10.1002/ana.25982
Tanca, A., Abbondio, M., Palomba, A., Fraumene, C., Manghina, V., Cucca, F., et al. (2017). Potential and Active Functions in the Gut Microbiota of a Healthy Human Cohort. Microbiome 5 (1), 79. doi:10.1186/s40168-017-0293-3
Tatay-Manteiga, A., Correa-Ghisays, P., Cauli, O., Kapczinski, F. P., Tabarés-Seisdedos, R., and Balanzá-Martínez, V. (2018). Staging, Neurocognition and Social Functioning in Bipolar Disorder. Front. Psychiatry 9, 709. doi:10.3389/fpsyt.2018.00709
Tolhurst, G., Heffron, H., Lam, Y. S., Parker, H. E., Habib, A. M., Diakogiannaki, E., et al. (2012). Short-chain Fatty Acids Stimulate Glucagon-like Peptide-1 Secretion via the G-Protein-Coupled Receptor FFAR2. Diabetes 61 (2), 364–371. doi:10.2337/db11-1019
Tournikioti, K., Dikeos, D., Alevizaki, M., Michopoulos, I., Ferentinos, P., Porichi, E., et al. (2018). Hypothalamus-pituitary-adrenal (HPA) axis Parameters and Neurocognitive Evaluation in Patients with Bipolar Disorder. Psychiatriki 29 (3), 199–208. doi:10.22365/jpsych.2018.293.199
Tsavkelova, E. A., Botvinko, I. V., Kudrin, V. S., and Oleskin, A. V. (2000). Detection of Neurotransmitter Amines in Microorganisms with the Use of High-Performance Liquid Chromatography. Dokl. Biochem. 372 (1-6), 115–117.
Tseng, P. T., Zeng, B. S., Chen, Y. W., Wu, M. K., Wu, C. K., and Lin, P. Y. (2016). A Meta-Analysis and Systematic Review of the Comorbidity between Irritable Bowel Syndrome and Bipolar Disorder. Med. Baltim. 95 (33), e4617. doi:10.1097/MD.0000000000004617
Turnbull, A. V., and Rivier, C. (1995). Regulation of the HPA axis by Cytokines. Brain Behav. Immun. 9 (4), 253–275. doi:10.1006/brbi.1995.1026
Unger, M. M., Spiegel, J., Dillmann, K. U., Grundmann, D., Philippeit, H., Bürmann, J., et al. (2016). Short Chain Fatty Acids and Gut Microbiota Differ between Patients with Parkinson's Disease and Age-Matched Controls. Park. Relat. Disord. 32, 66–72. doi:10.1016/j.parkreldis.2016.08.019
Ussar, S., Griffin, N. W., Bezy, O., Fujisaka, S., Vienberg, S., Softic, S., et al. (2015). Interactions between Gut Microbiota, Host Genetics and Diet Modulate the Predisposition to Obesity and Metabolic Syndrome. Cell Metab. 22 (3), 516–530. doi:10.1016/j.cmet.2015.07.007
Vakharia, K., and Hinson, J. P. (2005). Lipopolysaccharide Directly Stimulates Cortisol Secretion by Human Adrenal Cells by a Cyclooxygenase-dependent Mechanism. Endocrinology 146 (3), 1398–1402. doi:10.1210/en.2004-0882
van de Wouw, M., Boehme, M., Lyte, J. M., Wiley, N., Strain, C., O'Sullivan, O., et al. (2018). Short-chain Fatty Acids: Microbial Metabolites that Alleviate Stress-Induced Brain-Gut axis Alterations. J. Physiol. 596 (20), 4923–4944. doi:10.1113/jp276431
Van der Leek, A. P., Yanishevsky, Y., and Kozyrskyj, A. L. (2017). The Kynurenine Pathway as a Novel Link between Allergy and the Gut Microbiome. Front. Immunol. 8, 1374. doi:10.3389/fimmu.2017.01374
Vascellari, S., Palmas, V., Melis, M., Pisanu, S., Cusano, R., Uva, P., et al. (2020). Gut Microbiota and Metabolome Alterations Associated with Parkinson's Disease. mSystems 5 (5). doi:10.1128/mSystems.00561-20
Vanuytsel, T., van Wanrooy, S., Vanheel, H., Vanormelingen, C., Verschueren, S., Houben, E., et al. (2014). Psychological Stress and Corticotropin-Releasing Hormone Increase Intestinal Permeability in Humans by a Mast Cell-Dependent Mechanism. Gut 63 (8), 1293–1299. doi:10.1136/gutjnl-2013-305690
Wagner-Skacel, J., Dalkner, N., Moerkl, S., Kreuzer, K., Farzi, A., Lackner, S., et al. (2020). Sleep and Microbiome in Psychiatric Diseases. Nutrients 12 (8). doi:10.3390/nu12082198
Wang, Q. J., Shen, Y. E., Wang, X., Fu, S., Zhang, X., Zhang, Y. N., et al. (2020). Concomitant Memantine and Lactobacillus Plantarum Treatment Attenuates Cognitive Impairments in APP/PS1 Mice. Aging (Albany NY) 12 (1), 628–649. doi:10.18632/aging.102645
Wang, Z., Chen, W. H., Li, S. X., He, Z. M., Zhu, W. L., Ji, Y. B., et al. (2021). Gut Microbiota Modulates the Inflammatory Response and Cognitive Impairment Induced by Sleep Deprivation. Mol. Psychiatry 26 (11), 6277–6292. doi:10.1038/s41380-021-01113-1
Weingarden, A. R., and Vaughn, B. P. (2017). Intestinal Microbiota, Fecal Microbiota Transplantation, and Inflammatory Bowel Disease. Gut Microbes 8 (3), 238–252. doi:10.1080/19490976.2017.1290757
Wheless, J. W., Gienapp, A. J., and Ryvlin, P. (2018). Vagus Nerve Stimulation (VNS) Therapy Update. Epilepsy Behav. 88s, 2–10. doi:10.1016/j.yebeh.2018.06.032
Więdłocha, M., Marcinowicz, P., Janoska-Jaździk, M., and Szulc, A. (2021). Gut Microbiota, Kynurenine Pathway and Mental Disorders - Review. Prog. Neuropsychopharmacol. Biol. Psychiatry 106, 110145. doi:10.1016/j.pnpbp.2020.110145
Wu, G. D., Chen, J., Hoffmann, C., Bittinger, K., Chen, Y. Y., Keilbaugh, S. A., et al. (2011). Linking Long-Term Dietary Patterns with Gut Microbial Enterotypes. Science 334 (6052), 105–108. doi:10.1126/science.1208344
Wu, X., Lv, Y. G., Du, Y. F., Chen, F., Reed, M. N., Hu, M., et al. (2018). Neuroprotective Effects of INT-777 against Aβ1-42-Induced Cognitive Impairment, Neuroinflammation, Apoptosis, and Synaptic Dysfunction in Mice. Brain Behav. Immun. 73, 533–545. doi:10.1016/j.bbi.2018.06.018
Wynne, K., and Bloom, S. R. (2006). The Role of Oxyntomodulin and Peptide Tyrosine-Tyrosine (PYY) in Appetite Control. Nat. Clin. Pract. Endocrinol. Metab. 2 (11), 612–620. doi:10.1038/ncpendmet0318
Xie, B., Zhang, Y., Qi, H., Yao, H., Shang, Y., Yuan, S., et al. (2020). Red Light Exaggerated Sepsis-Induced Learning Impairments and Anxiety-like Behaviors. Aging (Albany NY) 12 (23), 23739–23760. doi:10.18632/aging.103940
Yoshikawa, K., Kurihara, C., Furuhashi, H., Takajo, T., Maruta, K., Yasutake, Y., et al. (2017). Psychological Stress Exacerbates NSAID-Induced Small Bowel Injury by Inducing Changes in Intestinal Microbiota and Permeability via Glucocorticoid Receptor Signaling. J. Gastroenterol. 52 (1), 61–71. doi:10.1007/s00535-016-1205-1
Zangerolamo, L., Vettorazzi, J. F., Solon, C., Bronczek, G. A., Engel, D. F., Kurauti, M. A., et al. (2021). The Bile Acid TUDCA Improves Glucose Metabolism in Streptozotocin-Induced Alzheimer's Disease Mice Model. Mol. Cell Endocrinol. 521, 111116. doi:10.1016/j.mce.2020.111116
Zeng, C., Yang, P., Cao, T., Gu, Y., Li, N., Zhang, B., et al. (2021). Gut Microbiota: An Intermediary between Metabolic Syndrome and Cognitive Deficits in Schizophrenia. Prog. Neuropsychopharmacol. Biol. Psychiatry 106, 110097. doi:10.1016/j.pnpbp.2020.110097
Zhan, G., Yang, N., Li, S., Huang, N., Fang, X., Zhang, J., et al. (2018). Abnormal Gut Microbiota Composition Contributes to Cognitive Dysfunction in SAMP8 Mice. Aging (Albany NY) 10 (6), 1257–1267. doi:10.18632/aging.101464
Zhang, J. C., Zhang, W. J., Zhao, Q., Chen, B. N., Wang, X., Luo, Y. P., et al. (2019). Adiponectin Improves Isoflurane-Induced Cognitive Dysfunction in Elderly Rats via Inhibiting P38-MAPK Signal Pathway in hippocampus. Eur. Rev. Med. Pharmacol. Sci. 23 (3 Suppl. l), 171–176. doi:10.26355/eurrev_201908_18644
Zhao, J., Bi, W., Xiao, S., Lan, X., Cheng, X., Zhang, J., et al. (2019). Neuroinflammation Induced by Lipopolysaccharide Causes Cognitive Impairment in Mice. Sci. Rep. 9 (1), 5790. doi:10.1038/s41598-019-42286-8
Zhao, Y., Cong, L., Jaber, V., and Lukiw, W. J. (2017). Microbiome-Derived Lipopolysaccharide Enriched in the Perinuclear Region of Alzheimer's Disease Brain. Front. Immunol. 8, 1064. doi:10.3389/fimmu.2017.01064
Zheng, H., Xu, P., Jiang, Q., Xu, Q., Zheng, Y., Yan, J., et al. (2021). Depletion of Acetate-Producing Bacteria from the Gut Microbiota Facilitates Cognitive Impairment through the Gut-Brain Neural Mechanism in Diabetic Mice. Microbiome 9 (1), 145. doi:10.1186/s40168-021-01088-9
Zheng, P., Yang, J., Li, Y., Wu, J., Liang, W., Yin, B., et al. (2020). Gut Microbial Signatures Can Discriminate Unipolar from Bipolar Depression. Adv. Sci. (Weinh) 7 (7), 1902862. doi:10.1002/advs.201902862
Zhou, Y., Wang, C., Lan, X., Zheng, W., Li, H., Chao, Z., et al. (2021). The Potential Pro-cognitive Effects with Intravenous Subanesthetic Ketamine in Adults with Treatment-Resistant Major Depressive or Bipolar Disorders and Suicidality. J. Psychiatr. Res. 144, 312–319. doi:10.1016/j.jpsychires.2021.10.037
Zhu, F., Guo, R., Wang, W., Ju, Y., Wang, Q., Ma, Q., et al. (2020). Transplantation of Microbiota from Drug-free Patients with Schizophrenia Causes Schizophrenia-like Abnormal Behaviors and Dysregulated Kynurenine Metabolism in Mice. Mol. Psychiatry 25 (11), 2905–2918. doi:10.1038/s41380-019-0475-4
Keywords: bipolar disorder, gut microbiota, cognitive function, gut-brain axis, psychobiotic
Citation: Dai W, Liu J, Qiu Y, Teng Z, Li S, Yuan H, Huang J, Xiang H, Tang H, Wang B, Chen J and Wu H (2022) Gut Microbial Dysbiosis and Cognitive Impairment in Bipolar Disorder: Current Evidence. Front. Pharmacol. 13:893567. doi: 10.3389/fphar.2022.893567
Received: 10 March 2022; Accepted: 20 April 2022;
Published: 23 May 2022.
Edited by:
Aurelijus Burokas, Vilnius University, LithuaniaCopyright © 2022 Dai, Liu, Qiu, Teng, Li, Yuan, Huang, Xiang, Tang, Wang, Chen and Wu. This is an open-access article distributed under the terms of the Creative Commons Attribution License (CC BY). The use, distribution or reproduction in other forums is permitted, provided the original author(s) and the copyright owner(s) are credited and that the original publication in this journal is cited, in accordance with accepted academic practice. No use, distribution or reproduction is permitted which does not comply with these terms.
*Correspondence: Jindong Chen, Y2hlbmppbmRvbmdAY3N1LmVkdS5jbg==; Haishan Wu, d3VoYWlzaGFuQGNzdS5lZHUuY24=