- 1Key Laboratory of Drug-Targeting and Drug Delivery System of the Education Ministry and Sichuan Province, Sichuan Engineering Laboratory for Plant-Sourced Drug and Sichuan Research Center for Drug Precision Industrial Technology, West China School of Pharmacy, Sichuan University, Chengdu, China
- 2State Key Laboratory of Southwestern Chinese Medicine Resources, Chengdu University of Traditional Chinese Medicine, Chengdu, China
Tumor angiogenesis is one of the most important processes of cancer deterioration via nurturing an immunosuppressive tumor environment (TME). Targeting tumor angiogenesis has been widely accepted as a cancer intervention approach, which is also synergistically associated with immune therapy. However, drug resistance is the biggest challenge of anti-angiogenesis therapy, which affects the outcomes of anti-angiogeneic agents, and even combined with immunotherapy. Here, emerging targets and representative candidate molecules from ethnopharmacology (including traditional Chinese medicine, TCM) have been focused, and they have been proved to regulate tumor angiogenesis. Further investigations on derivatives and delivery systems of these molecules will provide a comprehensive landscape in preclinical studies. More importantly, the molecule library of ethnopharmacology meets the viability for targeting angiogenesis and TME simultaneously, which is attributed to the pleiotropy of pro-angiogenic factors (such as VEGF) toward cancer cells, endothelial cells, and immune cells. We primarily shed light on the potentiality of ethnopharmacology against tumor angiogenesis, particularly TCM. More research studies concerning the crosstalk between angiogenesis and TME remodeling from the perspective of botanical medicine are awaited.
Introduction
The concept of “angiogenesis switch,” first proposed by Folkman, is traced back to 1971, which depicted that the imbalance between pro-angiogenesis and anti-angiogenesis determines the survival and progression of tumors. The former includes VEGF (vascular endothelial growth factor) family, angiopoietin (Ang), platelet-derived growth factors, fibroblast growth factors (FGFs), neuropilin, transforming growth factor, insulin-like growth factor, chemokines, and semaphorins/plexins/neuropilins, while the latter is composed of endostatin, thrombospondin-1, angiostatin, and interferon-α (Zhou et al., 2017). Beyond the well-known target αvβ3 integrin (Cayrol et al., 2019), VEGF/VEGFR, FGF/FGFR, and PDGF/PDGFR axes were considered the most common signaling and pivotal role in tumor angiogenesis. The expression of numerous angiogenesis-related proteins was found in breast cancer, including VEGF, Ang-1/Tie-2, PDGF, and bFGF(FGF2) (Folkman, 2007).
Advances toward understanding anti-angiogenic therapy that blocks neo-angiogenesis and restricts nutrition and oxygen support have exerted considerable progress against cancer. More than 14 FDA-approved anti-angiogenic drugs have been applied in clinical against several cancers, which are mainly divided into two categories: small molecular tyrosine kinase inhibitors (TKIs) and monoclonal antibodies, with the representative bevacizumab (indication: colorectal, non-small-cell lung, and glioblastoma multiforme) and sorafenib (indication: renal cell and hepatocellular carcinoma), respectively (Rajabi and Mousa, 2017). According to molecular targets, anti-angiogenic drugs were composed of VEGF inhibitor, PDGF inhibitor, Ang inhibitor, and VEGFR inhibitor (Parmar and Apte, 2021). It is noteworthy that some TKIs are multi-targeting and pleiotropic. For example, sorafenib shows multi-kinase-inhibiting efficiency, including VEGFRs and PDGFR. Although anti-angiogenic therapy targeting VEGF/VEGFR prolonged the overall survival of cancer patients, these drugs lead to untoward side effects, including lethal hemoptysis and intestinal perforation (Johnson et al., 2004; Jain et al., 2006). It is reported that the absence of VEGF/VEGFR in normal endothelial cells is responsible for these adverse effects (Rini, 2007). Beyond these, drug resistance and vascular toxicity are still prominent side effects and an insurmountable challenge of anti-angiogenesis therapy (Neves et al., 2020). A cancer combination therapy that partially avoids the progression of drug resistance elevates the risk of hypertension in tumor patients (Guo et al., 2021). The phase II study provides the clinical basis for the combination of bevacizumab and trebananib (median OS 31.4 months): no increase in side effects was observed without chemotherapy (Mooi et al., 2021). After EGFR-TKI resistance, based on real-world data, there was no significant difference between chemoimmunotherapy and chemo-antiangiogenesis in the prognosis of patients with advanced non-small-cell lung cancer (Yu et al., 2021). However, the combination of drug schemes containing anti-angiogenic therapy may cause embryotoxicity, which needs to be paid attention to in pregnant female tumor patients (Al-Asmakh et al., 2021). The single-target mono-therapeutic approaches significantly evoked TKI-resistances in that molecular signaling compensation and recruitment of pro-angiogenic cells were two indispensable causes. Moreover, the phenomenon of the proportion of non-responder patients toward the anti-VEGF approach remained high. Therefore, there is an urgency to introduce new therapy to overcome these shortcomings based on anti-angiogenic therapy. Recently, the combined approach with anti-angiogenesis and immune therapy was considered a promising avenue due to it breaking the mutual support between tumor angiogenesis and immunosuppressive TME (Song et al., 2020). Worldwide traditional medicines exert alternative and supplementary roles in cancer treatment, in all of which traditional Chinese medicine (TCM) has been investigated in abundant literature. The mainstream view is that the application of TCM relieves chemotherapy- and radiotherapy-induced adverse reactions, containing gastrointestinal reactions, cardiotoxicity, and peripheral neuropathy, even acneiform eruptions and diarrhea that are EGFR-TKI related (Zhang et al., 2021a). The traditional decoction and botanical products from native medicine have been extensively observed and explored according to modern pharmacology and molecular sciences in carcinogenesis (Xiang et al., 2019). TCMs have allowed for anti-tumor effects via immune enhancement and perform attributes for anti-angiogenesis, respectively (Zhang et al., 2018a; Wang et al., 2020a). However, few articles have reported the anti-angiogenesis potential of native pharmacology, especially the pleiotropy of candidate molecules toward tumor angiogenesis and tumor microenvironment (TME) concurrently. Importantly, traditional and botanical medicines provide a molecule library for screening candidates against tumor angiogenesis and immunosuppressive tumor microenvironment. Thus, based on PubMed, Web of Science, and Google Scholar, this review selectively introduced dozens of representative candidate anti-angiogenic agents and enumerated several angio-active molecules from ethnopharmacology for the aforementioned combined therapy. More significantly, several candidates with multi-targeting or pleiotropic attributes performed the potential to directly realize the synergistic effects between anti-angiogenic and immune therapy (Figure 1).
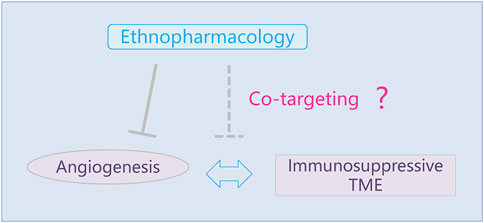
FIGURE 1. Potential and promising candidates were revealed for anti-angiogenic therapy, including traditional Chinese medicine. The attractive perspective that co-regulating angiogenesis and immunosuppressive tumor microenvironment via natural products and its derivatives or delivery system is proposed under the frame of ethnopharmacology.
Potential Targets of Anti-angiogenesis
Emerging targets provide more possibilities and a greater understanding for anti-angiogenesis therapy. It is worth mentioning that tumor angiogenesis is closely associated with tumor cells releasing angiogenic factors and endothelial cells supporting vessel sprouting. As present in Figure 2, several novel targets with anti-angiogenesis nature were selectively reported, including PIN1, KDM2A, EDD, CCN4, and even glycolysis-related PFKFB3. All of these potential targets can be divided into two types: targets in vascular endothelial cells and targets in cancer cells.
Targets in Vascular Endothelial Cells
PIN1 (peptidyl-prolyl cis–trans isomerase NIMA-interacting 1) accelerated tube formation of human umbilical vein endothelial cells (HUVECs) and angiogenesis in chick chorioallantoic membrane (CAM) by stabilizing HIF-2 α and enhancing its transcriptional activity (Choi et al., 2020). Silencing EDD (E3 isolated by differential display gene) induced the weakening of migration and tube formation of HUVECs, which was due to the negative regulation of ACVRL1 (activin receptor-like kinase-1, ALK1) gene and downstream Smad signal by EDD. In addition, transcription factor SP1 was partly responsible for the upregulation of ACVRL1 induced by blocking EDD (Chen et al., 2013a).
In addition, the type 14 family of C-type lectins have been considered promising targets for tumor treatment, including CD93, CLEC14A, and CD248 (Khan et al., 2019). C-type lectin family 14, member A (CLEC14A), overexpressed in tumor endothelial cells, promoted sprouting angiogenesis via VEGF/VEGFR-2/VEGFR-3 pathway (Lee et al., 2017a) and vascular development (Mura et al., 2012; Noy et al., 2016) and mediated cell–cell adhesion via its extracellular C-type lectin-like domain (CTLD) (Rho et al., 2011). HSP70-1A, acting as a molecular chaperone to stabilize the conformation of membrane protein CLEC14A, prompted the CLEC14A–CLEC14A interaction of endothelial cell–cell contact. These interactions triggered ERK phosphorylation and endothelial tube formation (Jang et al., 2017). The antibody deglyco C1 IgG targeting CTLD of CLEC14A was developed to inhibit CLEC14A expression and VEGF dependent angiogenesis (Kim et al., 2018).
The 6-phosphofructo-2-kinase/fructose-2,6-biphosphatase 3 (PFK-2/FBPase 3, PFKFB3), a critical regulator of glycolysis, catalyzes the conversion of fructose-6-phosphate (F6P) to fructose-1,6-bisphosphate (F1,6P2), which serves as an allosteric activator of the rate-limiting enzyme 6-phosphofructo-1-kinase (PFK-1). While PFKFB3 has been proved as an oncogene relating to cell proliferation, survival, and invasion (Shi et al., 2017; Kotowski et al., 2021), it is important to understand its role in the angiogenesis of TME, including tumor cells and vascular endothelial cells (ECs). Knockout of PFKFB3 was conducive to improving chemotherapy response and weakening tumor invasion and metastasis by normalizing tumor blood vessels, particularly converting endothelial barrier dysfunction (Cantelmo et al., 2016). On the other hand, the deficiency of PFKFB3 impaired angiogenesis involved with the modulation of tip cell formation and sprouting, while its overexpression facilitated vessel branching via the suppression of the pro-stalk activity that Notch signaling mediated (De Bock et al., 2013). Moreover, 3-(3-pyridinyl)-1-(4-pyridinyl)-2-propen-1-one (3PO), a molecular blocker of PFKFB3, inhibited vessel sprouting by restraining proliferation and migration of ECs and countered vascular hyper-branching that promoted inhibition of triggered Notch or VEGFR1 (Schoors et al., 2014). Another in vivo study found that the pro-normalization effect toward tumor blood vessels was reversed to vascular fragmentation and decomposition at high dose (70 mg/kg), compared with a low dose at 25 mg/kg. The positive correlation between PFKFB3 and CD163, CD31 suggested that PFKFB3 possibly promoted angiogenesis through modulating the infiltration of CD163 + tumor-associated macrophages (TAMs) in oral squamous cell carcinoma (Li et al., 2019a).
Galectins, cancer-associated and evolutionarily conserved glycoproteins, have been reviewed as potential targets for anti-angiogenic intervention (Varinska et al., 2017; Dings et al., 2018). Globo-H, a hexasaccharide originally found in human breast cancer cell line MCF-7, is strongly expressed in massive malignant tumors and involved in the regulation of the tumor microenvironment (Huang et al., 2020). A large number of evidences support its importance as an antigen in glycan localized tumor vaccines (Smith and Bertozzi, 2021). In the study about the cancer-associated glycans and glycosphingolipids of Globo-H ceramide (GHCer), cancer cells derived GHCer through microvesicles were assimilated by HUVECs, leading to the improvement of the angiogenic attribute, of which molecular mechanism involving the interaction between GHCer and TRAX for consequently inducing the activation of PLCβ1, drivers of early angiogenesis (Cheng et al., 2014). More investigations are expected to focus on the potential role of tumor-specific glycans and their related genes in angiogenesis. Likewise, the potential of glycoproteins in tumor angiogenesis and carcinogenesis is incomprehensively unmasked, for instance, Angiopoietin-like (ANGPTL) protein (Carbone et al., 2018).
Targets in Cancer Cells
KDM2A was reported as a pro-angiogenesis gene in breast cancer by transactivating JAG1 and PDGFA (Chen et al., 2016). WISP-1/CCN4, an extracellular matrix-associated protein, promoted VEGF-A secretion through the integrin αvβ3/FAK/c-Src axis and the EGFR/ERK/HIF1-α signaling pathway that was transactivated subsequently, in oral squamous cell carcinoma (OSCC), and then the VEGF that CCN4 induced mediated the neovascularization of endothelial progenitor cells (EPCs) trigging (Chuang et al., 2015).
Cyclooxygenase-2 (COX-2) is closely related to cancer progression such as cancer stem cell-like activity, apoptosis, proliferation, angiogenesis, inflammation, invasion, and metastasis, which involves enormous signal pathways for which there are varieties of transcription factor-binding sites in its promoter region, including IL-1, IL-6, SP1, AP-2, NF-κB, c-Jun, and CREB (Hashemi Goradel et al., 2019). The COX-2/HIF-1a/VEGF-A axis is one of the contributors to COX-induced angiogenesis. On the other hand, COX-2 mediated arachidonic acid metabolites are conducive to tumor vascular progression. For instance, prostaglandin E2 (PGE2) participates in the production of VEGF and the improvement of sprouting, migration, and tube formation in ECs (Gately and Li, 2004). The expression of COX-2 and VEGF was inactivated by tanshinone II-A, miR-101, and andrographolide in tumor cells, while the andrographolide impaired COX-2 promoter activity and restricted multiple trans-activators to bind the COX-2 promoter, such as CREB-2, c-Fos, and NF-κB (Zhou et al., 2012a; Liu et al., 2018a; Peng et al., 2018). However, mitosis-related centromere protein U (CENPU) suppressed the ubiquitination-dependent degradation of COX-2 to maintain angiogenesis through the activation of the COX-2/p-ERK/HIF-1α/VEGFA signaling axis (Pan et al., 2020).
Not only angiogenesis but some targets also interfere with cancer phenotypes, such as proliferation, apoptosis, and even metabolic reprograming, but in the context the TME regulation is our interest. ILT3, an immune negative regulator in non-solid tumors, potentiated tumor metastasis and angiogenesis in non-small-cell lung cells (NSCLCs). ILT3 recruited SHP2 and SHIP1, followed by phosphorylation of ERK1/2 to induce angiogenesis with increased VEGF-A expression (Li et al., 2021a). CCL5/CCR5 signaling mediates signal transduction cascades related to tumor progression, including PI3K/Akt, JAK/STAT3, MAPK/ERK, and NF-kB, involving in tumor growth, metastasis, cancer stem cell expansion, DNA damage repair, and angiogenesis and metabolic reprograming. It is noteworthy that the axis recruits immune cells and induces immunosuppressive polarization of macrophages to modulate TME reprogramming (Aldinucci et al., 2020). Blocking the CCL5/CCR5 axis induced decreased endothelial cell migration, which was related to decreased activity of the mTOR /Akt pathway, while CCL5 promoted tumor angiogenesis through the PKC δ/c-Src/HIF-1 α/VEGF signaling pathway (Wang et al., 2015a; Sax et al., 2016). PLD1 deficiency triggered a decrease in tumor growth and angiogenesis in the xenograft model and also reduced endothelial cell adhesion by downregulating the phosphorylation of ERK 1/2, p38, and Akt (Chen et al., 2012). In addition to the involvement of apoptosis through the downregulation of pro-apoptotic genes, caspase-3 was found to play an important role in angiogenesis by transactivating pro-angiogenetic genes (VEGFA, ANXA2, and C1GALT1), showing the crosstalk between apoptosis and angiogenesis (Bernard et al., 2019). CDK6, as a component of the transcription complex, induced the expression of P16 and VEGF-A, which bridged the cell cycle and angiogenesis (Kollmann et al., 2013). A C-glycosyl flavone was reported to induce apoptosis, cell cycle arrest, and angiogenesis inhibition via modulating CDK6, which was consistent with CDK6 blocker (Bevara et al., 2018).
The endocannabinoid system (ECS) was associated with declined angiogenesis via downregulating the VEGF/PIGF/Ang-2signaling axis that was mediated by cannabinoid receptors CB1R/CB2R, and it was important that ECS induced TME remodeling for the cannabinoid receptors expressed extensively (Iozzo et al., 2021). The aforementioned effects of proteoglycan agrin remained VEGFR2 dependent (Chakraborty et al., 2020). In addition, perlecan/HSPG2, a heparan sulfate proteoglycan, gathered in the tumor marginal stromal, was considered a molecular switch of angiogenesis in TME (Cruz et al., 2020). The immune checkpoint B7-H3 (CD276) accelerated immunosuppression of TME and exhibited non-immunological attributes for participating in angiogenesis (Feng et al., 2021). In triple-negative breast cancer, targeting B7-H3 led to vessel normalization and consequently improved PD-1 treatment response (Cheng et al., 2021). Moreover, CD276 enhanced the angiogenic function of tumor-associated macrophages, and CD276-blocking antibody raised the therapeutic efficiency of paclitaxel /anti-PD-1 in 4T1 tumor-bearing mice (Cheng et al., 2021). Diversely, the potential role of semaphorin 4D (SEMA4D, CD100), glypican-1, Delta-like 1 (DLL1), and insulin-like growth factor (IGF) in the bi-directional dialog of angiogenesis and immune regulation is also of significance (Lee et al., 2015; Wu et al., 2016; Lund et al., 2020; Zhang et al., 2021b).
The Role of Ethnopharmacology in Tumor Angiogenesis
Natural products or phytochemicals allow for the activity of regulating angiogenesis, which has attracted extensive interest. Some pro-angiogenic molecules have been reported that the notoginsenoside Ft1 induced angiogenesis by activating the VEGF/VEGFR2 signaling, while notoginsenoside R1 activated the Ang2/Tie2 signaling pathway (Shen et al., 2012; Zhong et al., 2020). Xue et al. reported six potential tumor angiogenic inhibitors from Chinese botanical medicine (glycopeptides, flavonoids, artemisinin, arsenic trioxide, ginsenoside, and tanshinone) and their pharmacological mechanisms (Yang and Wu, 2015). As shown in Table 1 and Figure 3, we illuminated the potential of plant-derived components and traditional Chinese medicine for anti-angiogenesis therapy: the former mainly included artemisinin, tanshinone, flavonoids, and saponin, while the latter is mainly composed of decoction of traditional Chinese medicine.
Plant-Derived Molecules Against Angiogenesis
MicroRNAs played a crucial role in ginsenoside-mediated anti-angiogenesis (Ashrafizadeh et al., 2020). For instance, the ginsenoside Rg1 downregulated miR15-b to induce angiogenesis in an increased VEGFR-2 manner (Chan et al., 2013). Notwithstanding the double-edged sword role of ginsenoside Rg3 in tumor angiogenesis is worth discussing, it was broadly considered an anti-angiogenic agent by modulating pro-angiogenic factors VEGF, FGF, and MMP (Nakhjavani et al., 2020; Liu et al., 2021a). Most recently, Rg3 was reported to induce angiogenesis inhibition in precancerous lesions of gastric cancer through lessening GLUT1 and GLUT4 (Zeng et al., 2022). Interestingly, the optimized combination of Rg3 epimers (50 μM S-Rg3 + 25 μM R-Rg3) more effectively suppressed tube formation, migration, and proliferation of HUVECs than S-Rg3 and R-Rg3, respectively (Nakhjavani et al., 2021). Although several ginsenosides active components of ginseng, including Rb1, Rg3, and Rd served as tumor angiogenesis inhibitors (Li et al., 2021b), it is noteworthy that the angiogenic modulation of the rest of the ginsenosides, containing protopanaxadiols, protopanaxatriols, and oleanane types (Zhou et al., 2022), were incompletely investigated. In addition, neem leaf glycoprotein (NLGP), a natural immune-modulator obtained from the leaves of neem (Azadirachta indica A. juss), balked M2 polarization of TAMs and HIF1α/VEGF signaling with STAT3-dependent manner and induced tumor vessel normalization with CD8+ T cells dependence by downmodulating VEGF and VEGFR2 (Banerjee et al., 2014; Goswami et al., 2014; Saha et al., 2020). As a part of the anti-tumor mechanism of legume lectin proteins, its anti-angiogenesis effect has been reported that Dolichos lablab L. lectin (DLL) protein weakened the expression of pro-angiogenic factors encompassing NF-κB, HIF-1 α, MMP-2 and 9, and VEGF, while the concanavalin A exhibited anti-angiogenic action via targeting IKK-NF-κB-COX-2, SHP-2-MEK-1-ERK, and SHP-2-Ras-ERK cascade (Li et al., 2011; Vigneshwaran et al., 2017). Another study on Lectin from Laetiporus sulphureus (LSL) revealed the LSL effects of anti-angiogenesis in zebrafish and migration inhibition in endothelial cells (Petrović et al., 2020).
Beyond antimalarial drugs, traditional Chinese medicine-derived artemisinin (ART) and its derivatives have attracted emerging concern for the promising potential in cancer therapy (Crespo-Ortiz and Wei, 2012; Li et al., 2020a). Tianshu et al. implied that attributes of artemisinin and its analogs against angiogenesis were associated with PI3K/Akt/mTOR axis, JNK, and p38 MAPK (Wei and Liu, 2017). Dissimilarly, artemisinin facilitated TSP-1 release to inhibit osteosarcoma-induced angiogenesis by activating the phosphorylation of p38 MAPK/CREB (Li et al., 2019b). In HUVECs, dihydroartemisinin (DHA) impaired proliferation and loop formation by inhibiting ERK signaling, p-STAT3 and its downstream fatty acid synthase (FASN) expression, and triggered autophagy via Akt/mTOR pathway (Dong et al., 2015; Liu et al., 2019; Gao et al., 2020). DHA attenuated HUVECs-mediated angiogenesis by modulating IκB-α/NF-κB/VEGFR2 axis (Dong et al., 2014), while DHA promoted VEGFR1 expression via upregulating ETS-1 (Dong et al., 2014; Niu et al., 2018). Unexpectedly, the ethanolic extract of Artemisia sieberi Besser performed stronger antiangiogeneic properties in tube formation and CAM assay in contrast to ART, which was attributed to the discordantly reduced VEGFR-1, VEGFR-2, and CD34 in the transcript (Abdolmaleki et al., 2016).
Tanshinone IIA (Tan IIA) is one of the main active components of Salviae miltiorrhizae radix et rhizome (Salvia miltiorrhiza Bunge) and is famous for its effectiveness in the treatment of cardiovascular diseases (Guo et al., 2020; Zhong et al., 2021). Its broad-spectrum pharmacological activities include but are not limited to anti-tumor, while there are few literatures concerning angiogenesis in tumors. Tan IIA suppressed β-catenin/TCF3/LEF1/VEGF by TGF-β1 at normoxia while by HIF-1α at hypoxia to astrict angiogenesis in colorectal cancer (Sui et al., 2017). Regardless of at hypoxia or at normoxia, tanshinone I subdued angiogenesis in epithelial cells (HMEC-1) and the secretion of VEGF from tumor cells (MCF-7) by the common mechanism: deduction of p-STAT3 and HIF-1α, and also inhibited VEGF against lung carcino-angiogenesis (Tung et al., 2013; Wang et al., 2015b). The angiogenesis EPCs-mediated was diminished by tanshinone IIA in vitro and in vivo by ruling the VEGF/PLC/Akt/JNK signaling axis (Lee et al., 2017b). The VEGF/VEGFR2 pathway and MMP-2/-9 and TIMP-2 expression were downregulated by Tan IIA in HUVECs for countering angiogenesis, while Tan IIA could bind the VEGFR2 kinase domain to inhibit the VEGF/VEGFR axis in lung cancer A549 cells (Tsai et al., 2011; Xie et al., 2015; Xing et al., 2015). Tan IIA also repressed the expression of pro-angiogenic factors (VEGF and bFGF) and HIF-1α in colorectal cancer (CRC) HCT-116 cells and adversely regulated proliferation and tube formation of HUVECs (Zhou et al., 2020a). Moreover, Tan IIA opposed angiogenesis with COX-2 and VEGF dependent in mice xenograft model of CRC and ovarian cancer (Zhou et al., 2012b; Zhou et al., 2020b). The mTOR/p70S6K/4E-BP1 and MAPK signaling pathway involved the anti-angiogenic activity that induced VEGF/HIF-1α suppression of silibinin and imperatorin in cervical and hepatoma cancer cells and colon cancer, respectively (García-Maceira and Mateo, 2009; Mi et al., 2017). Uniformly, Tan IIA weakened VEGF/HIF-1α expression by controlling the mTOR /p70S6K /RPS6 /4E-BP1 axis in breast cancer (Li et al., 2015). It was of importance that Tan IIA caused vascular stability and vascular normalization via downregulating Ang2-Tie2-AKT-MLCK axis in colon cancer (Zou et al., 2021). The anti-angiogenic properties of tanshinone VI were attributed to its downregulation of adhesion molecules ICAM-1 and VCAM-1 in epithelial cells (Nicolin et al., 2013). Moreover, the angiogenesis inhibition of cryptotanshinone (CPT) was implicated in multi-signaling, including Wnt/β-catenin/VEGF axis, VEGFR2 and its downstream Src/FAK, ERK1/2 in HUVECs, while it leads to the downregulation of PI3K/Akt/mTOR signaling and HIF-1α in CT26 colon cancer cells (Chen et al., 2014; Xu et al., 2017; Zhang et al., 2018b).
The silymarin, silibinin (SB), and thalidomide attenuated proliferation in endothelial (EA.hy 926) and colon cancer (LoVo) cell lines and also reduced the LoVo-secreting VEGF (Yang et al., 2003). The two pairs of flavonolignan diastereoisomers (silybin A, silybin B, isosilybin A and isosilybin B) isolated from Silybum marianum (L.) exerted similar effectivity against angiogenesis via downregulating Akt/HIF-1 α/VEGF axis in prostate cancer, and simultaneously modulated VEGF-induced signaling, encompassing VEGFR and its downstream Src, Akt, MAPKs, mTOR and so on in HUVECs (Deep et al., 2012). Hyeon et al. deemed that the anti-angiogenic effect of silibinin in endothelial cells depended on the regulation of NF-κB and apoptosis induction with the Bcl-2 family and Caspases involved (Yoo et al., 2004). Rana et al. found SB-induced cell cycle arrest, apoptosis, and suppression of migration and tube formation to perform anti-angiogenic efficacy in HUVECs, with survivin, Akt, and NF-κB were decreased (Singh et al., 2005). In addition, it reduced iNOS, COX-2, and VEGF expression in colon cancer mice, with the decreased levels of β-catenin, IGF-1Rβ, p-GSK-3β and p-Akt, and enhanced expression of IGFBP-3 (Ravichandran et al., 2010). Research on colon cancer suggested that the downregulation of NOS, COX-2, HIF-1α, VEGF, Ang-2, and Ang-4 was the result of SB treatment (Singh et al., 2008; Sameri et al., 2021). The SB lessened tumor angiogenesis in pancreatic cancer and prostate tumor xenograft (Singh et al., 2003; Nambiar et al., 2013), while SB inhibited tumor angiogenesis via restricting VEGF, VEGFR2, HIF-1α, and iNOS expression in a transgenic mouse of prostate cancer (Raina et al., 2008).
Luteolin’s effect against angiogenesis in vascular endothelial cells was ascribed to multiple mechanisms, such as the inhibition of MAPK and PI3K/Akt pathways that miR-133a-3p/PURB- mediated, repression of the PI3K/Akt/p70 S6K signaling and Gas6/Axl axis (Bagli et al., 2004; Zhu et al., 2013; Li et al., 2017; Pan et al., 2022). In addition, luteolin impaired HIF-1α/VEGF and Notch1-VEGF signaling in melanoma and gastric cancer individually (Zang et al., 2017; Li et al., 2019c). The luteolin showed better attributes in the suppression of blood vessels in CAM assay, cell proliferation and cell migration assay in HT-29 cells than lupeol and lectin (Ambasta et al., 2015). HIF-1 α was considered as a pro-angiogenic factor to facilitate tumor angiogenesis by activating PI3K/MAPK pathway and inducing VEGF release. The combination of asparagus polysaccharide (IC50 ∼ 10 mg/ml) and HIF-1α RNAi significantly inhibited the tube formation in HUVECs under HCC cells (SK-HEP and HEP-3B) induced and tumor angiogenesis in a xenotransplantation mouse model (100 mg/kg by gavage), and reduced the expression of VEGF and HIF-1α by suppressing Akt/Erk axis in vivo and in vitro (Zhu et al., 2021a).
Xanthomicrol, a flavone extracted from Dracocephalum kotschyi Boiss leaf, showed an antiangiogeneic effect in mice melanoma (B16F10) model (50 mg/kg) through negatively regulating the expression of VEGF, HIF-1α, and p-Akt (Ghazizadeh et al., 2020). Curcumin downregulated NF-κB and FAK/P38 MAPK and reduced the expression of VEGF, MMP-2, MMP-9, and COX-2 to exert the anti-tumor angiogenesis attribute in vivo and in vitro (Kumar et al., 2016; Hosseini et al., 2019). Compared with curcumin, bisdemethoxycurcumin is more effective to downregulate angiogenetic makers NF-κB, COX-2, MMP-9, and VEGF in Hep-2 cells (Mohankumar et al., 2021). However, curcumin promoted endothelial progenitor cells (EPCs) to participate in angiogenesis and conduced to neovascularization in animal models in vivo (Wang and Chen, 2019). In addition, VEGF-A and COX2 mRNA was downregulated by umbelliprenin (UMB, a coumarin from Ferula species) in 4T1 tumor mice (2.5 mg/d), with the protein expression of NF-κB and VCAM1 decreasing (Rashidi et al., 2018). The prior review has depicted the anti-angiogenic function of gambogic acid (GA) against tumors depending on the obstruction of HIF-1α/VEGF and prolyl hydroxylase-2 (PHD2)–von Hippel-Lindau gene (VHL)–HIF-1α, along with EGFR2 pathway (Liu et al., 2020). Moreover, GA mediated the inhibition of HIF-1α/VEGF through the downregulation of PI3K/Akt/mTOR in myeloma cells (Wang et al., 2014). After GA treatment, YAP/p-STAT3 and phosphorylation of VEGFR2 (KDR/Flk-1) signaling axis was suppressed in HUVECs (Lu et al., 2007; Wan et al., 2019).
The antiangiogeneic effect of Dioscin, a steroid saponin mainly appearing in Dioscorea opposita Thunb, involved the downregulation of p-Src/p-STAT3/VEGF/MMP-2,9 in melanoma, and attenuated VEGFR2 and Akt/MAPK signaling axis in colon cancer, while led to the constraint of VEGF/VEGFR pathway in ovarian cancer cells (Tong et al., 2014; Guo and Ding, 2018; Liu et al., 2022). Similar in mechanism, moscatilin, a bibenzyl derivative isolated from TCM Orchidaceae Dendrobii Caulis (Dendrobium loddigesii Rolfe), exerted antiangiogeneic attribute in vitro via repressing ERK1/2, Akt, and eNOS axis in HUVECs (Tsai et al., 2010). Anemarrhena saponin AIII, extracted from Anemarrhena asphodeloides Bunge a traditional Chinese medicine, inhibited the formation of internode vessels and subintestinal vessels in zebrafish (0.five to two µM), and significantly decreased the activity (more than 4 uM), migration, invasion and tube formation of HUVEC cells (0.5–4 µM) through attenuating VEGF/PI3K/Akt/MAPK signal transduction (Zhou et al., 2020c). Also, in HUVECs, Farrerol, a natural flavonoid from Rhododendron dauricum L. exerted similar mechanisms against angiogenesis by downregulating Akt/mTOR, ERK and JAK2/STAT3 signal pathway (Dai et al., 2016). Paris saponins I (polyphyllin D), existing in the Chinese herb Paris polyphylla var. yunnanensis, showed excellent anti-angiogenesis on HUVEC cells through downregulation of VEGFR2, JAK2/STAT3 pathways, and VEGFR2 and its downstream PI3K/Akt/MAPK, Src/eNOS, and PLCγ/ERK/MERK (Wang et al., 2020b). The role of Polyphyllin VII and Paris saponin II (formosanin C) against tumor angiogenesis involved NF-κB/VEGF axis on Hepatocellular carcinoma cells and ovarian cancer cells, respectively (Yang et al., 2015; Zhang et al., 2021c).
Traditional Chinese Medicine Against Tumor Angiogenesis
As2O3, a toxic traditional Chinese medicine, inhibited tumor growth and microvessel density by downregulating Notch pathway-related proteins Hes1, Dll4, and Notch1 in the small-cell lung cancer (SCLC) mouse model (2.5 and 5 mg/kg), and As2O3 restrained with the tube-forming ability of endothelial cells through the expression of Notch 1 and Hes1 in HUVECs (Yang et al., 2019a). Indeed, the thymic stromal lymphopoietin (TSLP) protein elicited immune-suppressive TME via interacting with TSLP receptor in CD4+ T cells to promote production of immunosuppressive factors, including IL-10 and IL-13 (Sims et al., 2000). Yu Ping Feng San, a famous decoction in TCM and comprised Astragali Radix (Huangqi, the root of Astragalus membranaceus (Fisch.) Bunge or Astragalus membranaceus (Fisch.) Bunge var. mongholicus (Bunge) P. K. Hsiao), Atractylodis Macrocephalae Rhizoma (Baizhu, the rhizomes of Atractylodes macrocephala Koidz.), and Saposhnikoviae Radix (Fangfeng, the roots of Saposhnikovia divaricata (Turcz.) Schischk.) reduced MVD and VEGF via downregulation of the TSLP /STAT3 pathway in hepatocellular carcinoma and HUVECs (Yuan et al., 2019; Du et al., 2021). The ethanol extract of Amomi Fructus (the fruit of Amomum villosum Lour.) had no influence on the viability of vascular endothelial cells. But it inhibited angiogenesis by restricting the p-STAT3 and NF-κB expression and reducing IL-6 and VEGF secreted by ovarian cancer cells (Chen et al., 2020a). Freshly, Shiquan Yuzhen Decoction, consisting of Ginseng Radix et Rhizoma, Astragalus membranaceus (root of Astragalus membranaceus (Fisch.) Bge. var. mongholicus (Bge.) Hsiao or A. membranaceus (Fisch.) Bge.), Dioscoreae Rhizoma (rhizome of Dioscorea opposita Thunb.), Anemarrhenae Rhizoma (rhizome of Anemarrhena asphodeloides Bge.), radix scrophulariae (root of Scrophularia ningpoensis Hemsl.), Os Draconis, Ostreae Concha, Salviae Miltiorrhizae Radix et Rhizoma (root and rhizome of Salvia miltiorrhiza Bge.), and Curcuma zedoariae (rhizome of Curcuma phaeocaulis Val. or C. kwangsiensis S. G. Lee et C. F. Liang or C. wenyujin Y. H. Chen et C. Ling), triggered the inhibition of tumor angiogenesis via restricting HIF-1α/VEGFA release, recuperated the immunity with the enhancement of CD8+ T and Treg cells, TNF-α level, and the abatement of IL-6 in lung cancer-bearing mice (Sun et al., 2021). The attenuated angiogenesis mediated by Xiaotan Sanjie decoction that comprised eleven herbs (including Pinelliae rhizome, Rhizoma arisaematis, and Poria cocos) in gastric cancer was related to the Notch-1/VEGF and IL-8/VEGF/VEGFR signaling axis (Yan et al., 2014; Shi et al., 2016). Jiedu Recipe, consisting of Pleiones Pseudobulbus (pseudobulb of Cremastra appendiculata (D. Don) Makino or Pleione bulbocodioides (Franch.) Rolfe or P. yunnanensis Rolfe), valvate actinidia (root of Actinidia valvata Dunn.), suppressed hypoxia-induced angiogenesis via restricting IL-8/HIF-1α/PI3K and MAPK/ERK pathways in endothelial EA.hy 926 cells, and inhibited the expression of VEGF, HIF-1α, and IL-8 under hypoxic conditions in HCC Huh-7 cells (Lin et al., 2021).
The Analogs From Medical Chemistry
Based on the reported molecules with vascular regulatory activity, the development of new candidates is still the dominant method, while physiological molecular mimics and receptor blockers also deserve attention, as shown in Figure 4 and Table 2. These candidate molecules primarily consisted of physiological molecular mimics, heterocyclic compounds, flavonoids, anthrone, phenanthraquinones, and polyphenols. After a structure–activity relationship (SAR) investigation, the molecular candidates performed potent anti-cancer effects in tumor angiogenesis and improved pharmacokinetics properties, including but not limited to aqueous solubility and bioavailability.
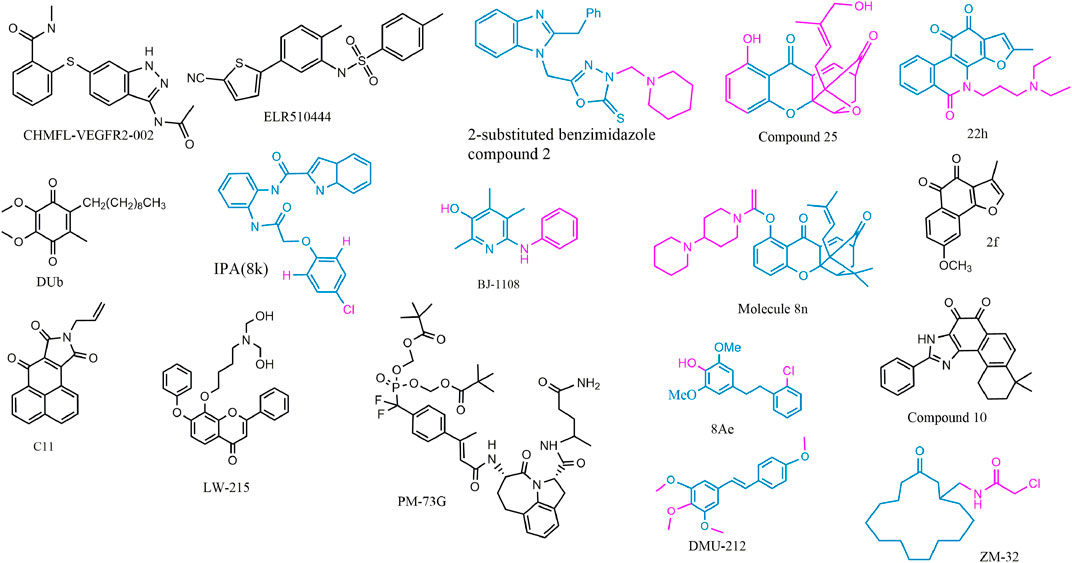
FIGURE 4. 2D structure of potential chemicals with anti-angiogenesis. The structure–activity relationship in coloring molecules was investigated: blue (parent) and red (substituent position).
To avoid the side effects caused by poor selectivity of drugs against VEGFR, a novel inhibitor (CHMFL-VEGFR2-002) with high selectivity toward VEGFR-2 (inhibitory activity of kinase IC50 = 66 nmol/L) has been found to show superb anti-angiogenesis effect in vivo and in vitro with low toxicity (Jiang et al., 2020a). ELR510444, a small molecule blocking HIF and known as a microtubule blocker, inhibited tumor angiogenesis in mice model of renal cell carcinoma, which was contributed to the suppression of HIF-1α and HIF-1β activity (0-100 nM) and the induction of microtubule destabilization (EC50, 27 nM) (Carew et al., 2012). C11, an FGFR1 inhibitor, blocked cell migration and tube formation in HMEC-1 endothelial (1–10 uM) and angiogenesis in CAM assay (0.1 - 10 ng/egg) (Chen et al., 2019a). The JAK/STAT pathway enhances the progression of angiogenesis, which mainly relates that p-STAT3 responds to FGF2 and VEGF stimulated in tumors and ECs (Chen et al., 2008; Zhao et al., 2011).
STAT3 bound the VEGF promoter and transactivated VEGF to touch upon tumor angiogenesis (Niu et al., 2002). PM-73G, phosphopeptide molecular mimic that synthesized to target the SH2 domain of STAT3, reduced MVD and VEGF levels by the suppression of p-STAT3 (Tyr705) in mice bearing MDA-MB-468 tumor (Auzenne et al., 2012).
The 6-amino-2,4,5-trimethylpyridin-3-ol analogs had been investigated as feasible tumor angiogenesis regulators (Kim et al., 2014). BJ-1108, a 6-amino-2,4,5-trimethylpyridin-3-ol derivative, inhibited tumor angiogenesis and 5-HT-induced ROS generation that depended on the PI3K/Akt/NOX signaling pathway (0.1.1 μM) in HUVECs (Banskota et al., 2016). The decylubiquinone (DUb), a coenzyme Q analog, induced tumor angiogenesis inhibition of breast cancer in vivo and ex vivo by the ROS/p53/BAI1 signaling axis (Cao et al., 2020).
The new 2-substituted benzimidazole molecules with heterocyclic were synthesized, in which compound 2 had the best activity of anti-proliferation without genotoxicity in PC-3 and SK-BR-3 cancer cells (IC50 < 20 μg/ml) and anti-angiogenesis in CAM assay (Güner et al., 2019). The IPA(8k), a novel indolephenoxyacetamide analog with anti-proliferative activity against A549 (IC50 ∼5 uM), performed anti-angiogenic activity in vivo and in vitro through inhibiting HIF-1 alpha, VEGF, MMP-2 and -9, and P53 (Al-Ostoot et al., 2021).
DMU-212 (trans-3,4,5,4 ′- tetramethoxystilbene), a resveratrol analog with higher anti-tumor activity and bioavailability than resveratrol, exhibited effective inhibition toward angiogenesis in vitro and in vivo. In mechanism, it suppressed the VEFGR2/Akt/mTOR/p70S6K pathway and c-Src/FAK/Erk 1/2 axis (Chen et al., 2013b). LW-215, derived from flavonoid wogonin, attenuated tube formation by inactivating VEGFR2 and its downstream p-Akt, p-ERK1/2, and p-p38 in endothelial cells (Zhao et al., 2018).
The compound 25, a gambogic acid (GA) analog, inhibited the ATPase activity of Hsp90 with an IC50 value of 3.68 μM compared with GA 21.98 μM. In addition, the compound 25 suppressed migration and angiogenesis by downregulating HIF-1α that was regulated by Hsp90 in HUVEC cells (0.01-0.25 μM) (Xu et al., 2016a). Another investigation about GA derivatives implied molecule 8n that bears a strong resemblance to the aforementioned effects (Xu et al., 2016b).
The compound 22h obtained from tanshinone I elevated water-solubility, bioavailability and anti-tumor potency, while it also suppressed migration and tube formation of HMEC-1 cells (Ding et al., 2018; Tian et al., 2018). The molecule 2f, cleaved ring A of tanshinone IIA and imported a methoxy group at C-8 position, provided feasible physicochemical property and anti-angiogenic activity in HUVECs (0.25, 0.5, 1 μM) and zebrafish model (1,2,4 μM) (Huang et al., 2021). However, the eleven novel tanshinone analogs were obtained from puried tanshinone mixture from Salvia miltiorrhiza by one-pot synthesis modification, in which the molecule 10 exerted potent pro-angiogenesis effect in zebrafish, with at least partly involving VEGF/FGF-Src-MAPK and PI3K-P38 signaling pathways (Zhang et al., 2014).
The moscatilin derivative 8Ae performed more effective angiogenesis inhibition in zebrafish assay (0.62–1.25 μM) than positive drug SU5416 (Guan et al., 2019). Muscone derivative ZM-32 attenuated the stabilizing effect of RNA-binding protein HuR toward Vegf-a and Mmp9 mRNA, thus resulting in downregulation of VEGF-A and MMP-9 expression in HUVECs and breast cancer MDA-MB-231 cells (Yang et al., 2021a).
Pharmaceutical Delivery Systems Against Tumor Angiogenesis
Nano preparations demonstrate the advantages of prolonging drug action time, improving solubility, and active and passive targeting (Hatami et al., 2020). Beyond loading anti-angiogenic drugs, nanoparticles are also combined with other adjuvants to construct multifunctional nano-platforms, including imaging, immunomodulation, photothermal therapy, and photodynamic therapy.
Galbanic acid (GC), delivered by the poly (D, l-lactide)–polyethylene glycol (PLA–PEG) nanosystem, showed lower IC50 than free galbanic acid in colon carcinoma C26 cells with IC50 = 8 μM and 15 μM, respectively, and increased by 15% in anti-angiogenetic activity compared to GC (Afsharzadeh et al., 2019). HA-TQ-Nps, hyaluronic acid-decorated mixed Pluronic® nanoparticles loading thymoquinone, faded tumor angiogenesis via miR-361/VEGF-A in breast cancer MDA-MB-231, MDA-MB-231, and 4T1 cells (IC50 < 9 μg/ml) (Bhattacharya et al., 2020; Peng et al., 2021). The amphiphilic and self-assemble drug (FUDR-PAB nanoparticles) was synthesized by conjugating floxuridine (FUDR) as the hydrophilic moiety and pseudolaric acid B (PAB) as the hydrophobic. The nanoparticle exerted better antiproliferative activity (lower IC50 and smaller tumor volume) in HeLa tumor cells and mice bearing tumors and higher anti-angiogenesis efficiency in HUVECs than PAB, FUDR, and PAB/FUDR mixture (Sun et al., 2019). Likewise, AuNPs-Qu-5, gold nanoparticle-loaded quercetin (50 μM), was observed to perform angiogenic nature against tube and new blood vessel formation ex vivo and in vivo through the VEGE/VEGFR2/PI3K/Akt axis, which was respectively confirmed by tube formation and CEA (Balakrishnan et al., 2016).
Cyclic RGD pentapeptide blocking αvβ3 integrin and tengflavin were linked by low molecular weight heparin to form cRHG nanoparticles that inhibited HIF-1 α, VEGF, CD31, and p-VEGFR2 in U87MG glioblastoma xenograft model (Dahmani et al., 2016). RGD peptide surface-decorated selenium nanoparticles (RGD-NPs) loading with adriamycin significantly promoted the anti-angiogenic activity of SeNPs in vitro and in vivo. RGD-NPs induced apoptosis and S phase cell cycle arrest in HUVECs (2-8 μM) and inhibited neo-angiogenesis in BC MCF-7-bearing tumor mice (2.5–7.5 mg/kg) by the downregulation of VEGF-VEGFR2 (Fu et al., 2016). The spontaneous degradation of pH-degradable poly (vinyl alcohol) (PVA) microgel depended on pH in an acidic tumor microenvironment. The decoration of dopamine (DA) on PVA microgels partially contributed to tumor adhesion and retention, which was the origin of dopamine (DA)-functionalized PVA microgels (DMGs). DMGs@Bev /DTX, PVA microgel encapsulated bevacizumab (Bev) and docetaxel (DTX), facilitated anti-tumor activity in 4T1-Luc cells (48 h treatment IC50 < 10 μg/ml), and anti-angiogenesis in tumor-bearing BALB/c mice (Chen et al., 2020b). The microgels with tumor-targeting and pH-degradable for the combination scheme of Bev and DTX performed better chemotherapy enhancement and anti-angiogenesis than other controls (Chen et al., 2020b). The metal–organic framework nanosystem named as aMMTm, designed on the strategy of photodynamic therapy (PDT) and anti-angiogenesis, was packed with porphyrinic Zr-MOF (photosensitizer) and apatinib (VEGFR2 inhibitor) and coating with MnO2 and cell membrane in the surface (Min et al., 2019). PDT and VEGF/VEGFR double inhibitor (Avastin + Erbitux) lead to an obvious decrease in VEGF and EGFR and considerable tumor elimination in a murine bladder tumor model (Bhuvaneswari et al., 2011). Sunitinib, VEGFR and PDGFR inhibitor, was encapsulated in a polyamide amine (PAMAM) dendrimer cavity, and the nano-scintillator CaF2 and photosensitizer Rose Bengal were distributed on the surface of PAMAM at a suitable distance to construct a nano platform CCT-DPRS. After low dose X-ray irradiation, the doped scintillator transformed the captured energy into green emission, which led to further excitation of Rose Bengal to produce cytotoxic singlet oxygen to eliminate cancer cells. At the same time, the platform released sunitinib and active oxygen to induce apoptosis and inhibit tumor angiogenesis, with increased expression of cleaved PARP and decreased levels of VEGFA, HIF-1α, survivin, and p-STAT3 (Jiang et al., 2021).
Nanoparticles (CA4P-loaded NBP@TiO2) for photothermal therapy (PTT) combined with anti-angiogenesis have been reported, in which Au nanobipyramids (NBPs) were designed as photothermal agents for infrared light excitation at 1064nm and TiO2 shell coating with combretastatin A-4 phosphate (CA4P) for anti-tumor attribute. The inhibition of angiogenesis in HUVECs and reduction of tumor microvessel density in A549 tumor-bearing mice were found in the therapy with synergism between PTT and nano-platform (Chen et al., 2019b). Similar photothermal chemotherapy was applied in cervical cancer for anti-angiogenesis. The delivery nanosystem (cisplatin–AuNRs@SiO2–Avastin@PEI/AE105) carried cisplatin and the anti-angiogenic drug Avastin, with Au nanorods (AuNRs) selected as a photothermal agent. AE105, a polypeptide composed of nine molecular amino acids with a high affinity for uPAR receptor that is highly expressed in cervical cancer tissues, linked by hydrophilic polymers PEI to the nanoparticles for tissue targeting (Hu et al., 2019). RBCs@Se/Av suppressed angiogenesis of HUVEC by triggering apoptosis and decreased vascular density in A375 tumor-bearing mice. The nanosystem was constructed by binding pegylational selenium nanoparticles (SeNPs, Se) and Avastin (VEGF antibody, AV), encapsulated with red blood cells membrane (Liu et al., 2018b). A novel chelating agent, imidazole doped with organic silica (Imi-OSi) nano-materials, performed anti-angiogenesis by copper capture and blocking tumor blood vessels through phosphate and Cu2 + responsive polymerization in breast cancer and colon cancer mice models (Yang et al., 2019b).
Antagonizing cytokines triggering angiogenesis, combined with vascular normalization therapy, reshuffle the balance of pro-vascular factors and anti-vascular factors in TME. The bi-directional nanosystem has been exposed that FLG nanoparticles loaded with VEGF/VEGFR2 pathway inhibitors, low molecular weight heparin (LMWH), and gambogic acid (GA) and modified by F3 peptide targeting tumor vascular endothelial cells, obstructed the abnormal proliferation of vascular endothelial cells, increased pericyte coverage, and improved hypoxia, while the other nanosystem MAR/MPA with CCL5/CCR5 blocker Maraviroc induced the decrease of glycolysis rate, VEGF secretion, and Tregs recruitment as well as the increase of CD8 + T and CD4 + T cell infiltration (Deng et al., 2021).
The ginsenoside Rg3, oridonin, and Ganoderma lucidum polysaccharide (GLP) were introduced into a self-microemulsifying drug delivery system (RGO-SMEDDS) as an anti-angiogenic agent, immune regulator, and apoptosis inducer, respectively. The system evinced a combined strategy against HCC via triggering angiogenesis inhibition, anti-proliferation, and decreasing immunosuppressive cytokines and M2-polarized macrophages, for the suppression of the p-EGFR/AKT/GSK3 axis (He et al., 2021).
Sibusiso et al. have reported the nanosystem doped with artemisinins, including polymeric, metal-based, and lipid nanoparticles, against cancer by enhancing effects recuperating poor solubility and bioavailability and targeting delivery (Alven and Aderibigbe, 2020). Concurrently, Yun et al. depicted a biodegradable poly (ethylene glycol) methyl ether-poly (ε-caprolactone) (MPEG-PCL) loading dihydroartemisinin (DHA) had allowed for a stronger anti-angiogenic effect than free DHA (Lu et al., 2020).
Co-Regulating Tumor Angiogenesis and TME by TCM
The molecular mechanisms through which immunosuppressive microenvironment-caused abnormal tumor vasculature and vascular normalization improved immunotherapy have been reported, respectively (Fukumura et al., 2018; Liu et al., 2021b). In brief, tumor-characteristic metabolism led to an imbalance between pro- and anti-angiogenic factors to induce abnormal vessels: disorganized vessel distribution and dysfunction, which in turn aggravated tissue hypoxia to promote the imbalance. The hypoxia, acidosis, and accumulated pro-angiogenic factors (represented by VEGF and Ang2) cooperatively promoted immunosuppressive TME with multi-mechanisms via recruiting immune-inhibiting cells and repressing the anti-tumor function of dendritic cells and cytotoxic T lymphocyte (Fukumura et al., 2018; Zhu et al., 2021b; Fousek et al., 2021). Also, on the contrary, the adverse TME recruited and activated immunosuppressive cells to facilitate tumor angiogenesis with the VEGF/VEGFR-dependent approach (Yang et al., 2021b).
Advances of angiogenesis dictated the efficiency of immunotherapy, while immune interference induced vascular normalization, which was ascribed to interferon γ secreted by CD4+ /CD8+ T cells, and reshaped TME advantageous to regression of angiogenesis in preclinical observations (Liu et al., 2021b; Yang et al., 2021b). The combined concept of immunocheckpoint blocking (PD-1/PD-L1 antibodies) and anti-VEGF has been generally accepted for the improvement of clinical outcomes and is considered a hopeful and promising treatment approach in therapeutics (Hack et al., 2020; Lee et al., 2020). Instead of directly targeting VEGF, an indirect approach was recommended for materializing dual-modulation of the tumor vascular system and TME through VEGF/HIF-1 mediated by the PI3K/Akt/mTOR cascade and preventing drug resistance (Fokas et al., 2012).
What we are persuasively interested in is the local or ethnic medicine—derived molecules with properties of both anti-angiogenesis and TME remodeling, all of them provide a unique sight on the combined therapy of tumor angiogenesis inhibition and immune modulation. On the other hand, there are abundant evidences implying that natural products and their analogs have a crucial part in regulating TME, such as ginseng and silibinin (Deep and Agarwal, 2013; Li et al., 2021b), while in independent studies the anti-angiogenic attributes in some of them are also reported. The biologically active ingredients with TME regulatory and anti-angiogenic activities confirmed in independent and respective studies are considered second-line or potential evidences. Traditional and empirical medicines, including traditional Chinese medicine, have contributed molecules libraries for cancer treatment and preclinical investigations with multi-potency in the modulating phenotype, such as angiogenesis and TME remodeling (He et al., 2015). The plant-derived flavonoids, alkaloids, glycosides, terpenoids, and coumarin were discussed as dispatchers between anti-VEGF therapy and treatment via immune checkpoint inhibitors (Kumar et al., 2021).
Except for the antiangiogeneic nature, as mentioned earlier, the immunomodulation of Dolichos lablab L. lectin was advantageous to anti-tumor effects mediated by IL-2 (Vigneshwaran et al., 2017). The digitoxin, famous as cardiotonic steroids, rescued HUVEC migration and loop formation that macrophages induced and also arrested SKOV3 cell growth and migration under macrophage conditioned media (Trenti et al., 2018; Whayne, 2018; Bejček et al., 2021). It also weakened HIF-1α protein expression by suppressing the phosphorylation of ribosomal protein S6 kinase (p70S6K) and eIF4E binding protein-1 (4E-BP1) in colon cancer cells (Mi et al., 2022). Significantly, luteolin enabled inhibition of angiogenesis induction of M2-like TAMs, achieved by the downregulation of HIF-1α and STAT3 signaling (Fang et al., 2018). Targeting macrophages in TME, anemoside A3 derived from Pulsatilla saponins induced tumor-suppressive M1-like macrophage by activating TLR4/NF-κB/MAPK signaling and subsequently enhanced expression of IL-12 in macrophages to attenuate angiogenesis of breast cancer in vivo and in vitro (Yin et al., 2021).
Bufalin, the bioactive C-24 steroids extracted from traditional Chinese medicine toad venom, exerted synergistic effects on angiogenesis with sorafenib via downregulating PI3K/Akt/mTOR/VEGF signaling pathways in HCC and HUVECs (Wang et al., 2016; Wang et al., 2018). Interestingly, bufalin inhibited angiogenesis mediated by TME cells (TAMs, CAFs, and CT26 cells) in the HUVECs model through regulating p-STAT3 and its downstream pro-angiogenic factors, including VEGF, PDGFA, E-selectin, and P-selectin (Fang et al., 2021).
Melittin (MEL), a polypeptide and the capital ingredient of honey bee venom, declined HIF-1α/VEGF levels via the suppression of ERK and mTOR/p70S6K signaling (Shin et al., 2013). It downregulated NF-κB to inhibit the HIF-1α/VEGFA and LDHA expression that caused angiogenesis and descent pH via anaerobic metabolism, in TME, respectively (Mir Hassani et al., 2021). CDDO-Me (Bardoxolone methyl), an analog of the natural triterpenoids oleanolic acid, the methyl-ester of the 2-cyano-3,12-dioxooleana-1,9 (11)-dien-28-oic acid (CDDO), have been abundantly advised for pharmacological applications, including tumor interference (Borella et al., 2019). CDDO-Me was reported to reduce chemokines CXCL12 and CCL2 release and the infiltration of suppressive TAM and inhibit cyclin D1, EGFR, and STAT3 responsible for anti-proliferation in PyMT breast cancer (Tran et al., 2012). Another study in PyMT implied that CDDO-Me decreased IL-10 and VEGF levels while increased TNF expression, concomitantly suppressed TAM tumor infiltration, and CD4 Foxp3 regulatory T cells (Ball et al., 2020). The nanoparticle delivery of CDDO-Me reshaped the immunosuppressive microenvironment with abatement of both Treg cells and MDSCs, and the concurrent rise of cytotoxic T-lymphocyte population, meanwhile, reduced angiogenesis in B16F10 melanoma mice (Zhao et al., 2015). The nanoparticle delivery of anti-tumor agent silibinin and PI3Kγ blocker IPI-549 synergistically remodeled TME in 4T1 breast cancer mice, contributing to decline of TAFs, MDSCs, tumor angiogenesis, and matrix but increased Treg cells (Jiang et al., 2020b).
The nanosystem delivery technology was considered a promising means for reshaping abnormal tumor vasculature to vascular normalization (Liang et al., 2022). On the other hand, vessel normalization was beneficial to the enhancement of drug penetration (Li et al., 2020b). Another strategy for co-targeting angiogenesis and TME is chosen as delivery platforms with functional decorations, in which loaded two or more molecules or drugs achieved the aforementioned goals with a designed enhancement of pharmacokinetics, while nanosystems loading single candidates are also that we are interested in. The normalization of blood vessels based on the scheme of angio-blocker delivery has been reported to reprogram TME for improving immune cell infiltration (Li et al., 2020b). For instance, the nomoplatform based on anti-angiogenic low molecular weight heparin (LMWH) transformed M2 polarized macrophage to M1 type and induced the vessel normalization (Xu et al., 2020). Pleiotropic nanodelivery was vastly commendable to co-modulate suppressive TME and tumor angiogenesis with multiple components, and the evidence entailed poly-lactic-glycolic acid (PLGA) and liposome nanoparticles (Hameed et al., 2018). The abundant multi-potency nanosystems indicated a synergistic strategy by introducing chemotherapy and dynamic therapy (sonodynamic and photothermal dynamics) for anti-angiogenesis (Li et al., 2020b; Zhu et al., 2021b). We expect that a multi-strategy delivery system and single molecules with multi-efficiency emerge to illuminate unlisted angiogenesis blockers for reshaping suppressive TME, while the role of the classic recipe of TCM remains underlying.
Perspectives and Conclusion
Tumor angiogenesis is one of the crucial factors that shape cancer malignancy. The design and development of anti-angiogenic drugs, the identification of potential regulatory networks, and further application of delivery systems are beneficial to provide progressive insights into tumor angiogenesis. Some TCMs or bioactive molecules are typical with the VEGF/VEGFR-dependent approach against tumor angiogenesis, such as Salvia miltiorrhiza, Curcuma longa, ginsenosides, and Scutellaria baicalensis (Zhang et al., 2018a). Several bioactive ingredients or phytomolecules from the ethnopharmacology we mentioned, mainly including artemisinin, tanshinone, flavonoids, and saponin, are a miniature to depict and generalize these molecules’ attributes against tumor angiogenesis and associated regulatory mechanisms. Specifically, whatever natural products or chemical derivatives modulating angiogenesis share cardinal pathways that VEGF mediated, such as VEGFR2 pathway in vascular endothelial cells, HIF-1α/VEGF and its upstream transcriptional signaling in cancer cells, we concern and await the emergence of more potential targets for anti-angiogenesis therapy. Although other natural molecules in TCM also performed anti-angiogenesis property, comprising coumarins (Wu et al., 2020), terpenoids (Kamran et al., 2022), polysaccharides (Li et al., 2021c), and polyphenols (Li et al., 2021c; Marrero et al., 2022), they have no distinct difference in mechanisms in comparison with molecules we reported. Recently, angiopoietin (for instance, ang2) inhibition has been implied to be a strategy for overcoming the resistance of VEGF blockers in clinical, while the potential of natural molecules as angiopoietin inhibitors needs further observation (Parmar and Apte, 2021).
In spite of the fact that great understanding and progress have been achieved in angiogenesis modulation via molecules, such as TKIs in clinical and preclinical candidates, there is a massive dearth of investigation in ethnopharmacology or empirical medicine for comprehensively screening and proving potential angio-inhibitor from the natural molecule library, including TCM. Moreover, thyroid hormone induced angiogenesis through activation of αvβ3 integrin signaling and upregulation of VEGF, which suggests the potential value of endocrine therapy in anti-angiogenesis (Cayrol et al., 2019). What is exciting is that some active ingredients from TCM need to be relocated in angiogenic effects for the shared signaling pathways (such as MAPK and PI3K/Akt/mTOR), implying the viability of co-targeting angiogenesis and other phenotypes, including proliferation, apoptosis, and stem cell like-type. However, there are few studies to demonstrate the role of candidate molecules toward antiangiogenic factors or signalings, such as TNFα, TSP-1, TIMP, and TGF-β/BMP pathway (Ayuso-Íñigo et al., 2021), but IL-8 seems to involve the crosstalk between angiogenesis and TME like VEGF (Fousek et al., 2021). In clinical, these candidate drugs were more considered dietary supplements or adjuvants (Table 3) for the anti-angiogenic strategy, beyond a single drug being used. Thus, it is expected that more botanical or ethnic medicine-related molecules will be pushed into the clinic for anti-tumor and even based on the antagonism of tumor angiogenesis. Given vascular structure distortion and vascular dysfunction are conducive to tumor deterioration and metastasis, tumor vascular normalization and tumor microenvironment reprograming that the candidate molecules triggering may contribute to the progression in clinical and preclinical investigations.

TABLE 3. Clinical trials of molecules based on anti-angiogenic therapy against cancer in ethnopharmacology. Data from ClinicalTrials and WHOICTRP.
Single-cell transcriptome profiling suggests that SQLE and ALDH18A1 may be potential anti-angiogenic targets for modulating epithelial cell metabolism (Rohlenova et al., 2020). Interested in solid tumors based on single-cell transcriptomics, HIF-1α was reported to mediate the functional inhibition of NK cells and regarded as an immune checkpoint of NK cells, which opened novel insights into regulating the crosstalk between angiogenesis and TME via HIF-1α blocking (Ni et al., 2020). Although single-cell RNA sequencing is emerging, it still needs to be further observed for evaluating angiogenesis. However, there is no report concerning tumor angiogenesis in spatial transcriptomics (ST). Interestingly, it can be considered a supplementary method to observe multiple angiogenesis markers on tissue sections for annotation in RNA expression, while the GeoMx Digital Spatial Profiler technology has integrated the transcriptomics and proteome.
While targeting VEGF and /or HIF-1 remains a basic starting point for verifying potential anti-angiogenic candidates and creating a bridge to interact with TME modulation, some novel strategies are imported into ethnopharmacology, composed of vessel normalization for the crosstalk between tumor angiogenesis and TME reconstruction. It should not be ignored that the cardinal role of angiogenic closely related tumor cells and endothelial cells in TME but reshaping tumor vessel via TAMs and macrophages, even fibroblasts, takes more attracted attention. Traditional Chinese medicine and bioactive molecules have been depicted as regulators in the crosstalk between gut microbiota and TME, yet the indirect involvement of TCM toward tumor angiogenesis via modulating the aforementioned crosstalk remains little understood (Wang et al., 2021). There are still challenges in the anti-angiogenic research of bio-active components of herbal or botanical extracts (Heinrich et al., 2020). The limitation of this article is that it just selectively elucidates the potential and representative regulators and delivery systems of tumoral angiogenesis, and there is a lack of view of the interaction of rather new but not fully explored non-coding RNAs and metabolic reprogram toward angiogenesis. Taken together, potential targets in vascular endothelial cells (ECs) and in tumor cells may provide new perspectives on angiogenesis, including CCN4, CCR5, ILT-3, EDD, and PIN. What should be paid attention to was that PFKFB3 played the mediator between glycometabolism and VEGF signaling in ECs. Plant-derived and ethnopharmacology-related molecules contributed to the anti-angiogenesis therapy via modulating VEGFR2 and its downstream PI3K/AKT/mTOR and MAPKs, with NF-κB, STAT3 and β-catenin involved, irrespective of targets in endothelial cells or cancer cells. The molecule library of natural products has not been fully explored in angio-modulation. Two methods were applied for developing anti-angiogenic drugs in medical chemistry: physiological molecular simulant and derivatives of natural products, in which the latter was featured by heterocyclic compounds, flavonoids, anthrone, phenanthraquinones, and polyphenols. Tanshinone’s analog has been extensively investigated as a potential anti-angiogenic agent. Moreover, nano-delivery systems loading functional molecules exerted an approach for anti-angiogenic therapy, even inducing tumor vessel normalization and remodeling TME simultaneously. Candidates with multi-potency, including digitoxin, bufalin, melittin, and CDDO-Me, make co-modulating therapy between tumor angiogenesis and immunosuppressive TME true in ethnopharmacology. We look forward to more clinical trials focusing on the anti-angiogenic and immunomodulatory properties of molecules from ethnopharmacology.
Author Contributions
This manuscript was conceptualized by all the authors; JZ conceived ideas and drafted the original draft preparation; LW edited the manuscript; and FP and CP administrated funding and revised the manuscript. All authors have read and agreed to the published version of the manuscript.
Funding
The study was supported by the National Natural Science Foundation of China (nos.82003879 and U19A2010), the Key Project of Science and Technology Department of Sichuan Province (nos. 2020YFS0053 and 2021YFS0044), the Youth Talent Promotion Project of China Association for Science and Technology (CACM-2020-QNRC1-01), and the Open Research Fund of Chengdu University of Traditional Chinese Medicine Key Laboratory of Systematic Research of Distinctive Chinese Medicine Resources in Southwest China.
Conflict of Interest
The handling editor ZW declared a past co-authorship with the authors CP and FP.
The authors declare that the research was conducted in the absence of any commercial or financial relationships that could be construed as a potential conflict of interest.
Publisher’s Note
All claims expressed in this article are solely those of the authors and do not necessarily represent those of their affiliated organizations, or those of the publisher, the editors, and the reviewers. Any product that may be evaluated in this article, or claim that may be made by its manufacturer, is not guaranteed or endorsed by the publisher.
References
Abdolmaleki, Z., Arab, H.-A., Amanpour, S., and Muhammadnejad, S. (2016). Anti-angiogenic Effects of Ethanolic Extract of Artemisia Sieberi Compared to its Active Substance, Artemisinin. Rev. Bras. Farmacogn. 26, 326–333. doi:10.1016/j.bjp.2015.11.008
Afsharzadeh, M., Abnous, K., Yazdian-Robati, R., Ataranzadeh, A., Ramezani, M., and Hashemi, M. (2019). Formulation and Evaluation of Anticancer and Antiangiogenesis Efficiency of PLA-PEG Nanoparticles Loaded with Galbanic Acid in C26 Colon Carcinoma, In Vitro and In Vivo. J. Cell Physiol. 234, 6099–6107. doi:10.1002/jcp.27346
Al-Asmakh, M., Bawadi, H., Hamdan, M., Gupta, I., Kheraldine, H., Jabeen, A., et al. (2021). Dasatinib and PD-L1 Inhibitors Provoke Toxicity and Inhibit Angiogenesis in the Embryo. Biomed. Pharmacother. 134, 111134. doi:10.1016/j.biopha.2020.111134
Al-Ostoot, F. H., Sherapura, A., V, V., Basappa, G., H K, V., B T, P., et al. (2021). Targeting HIF-1α by Newly Synthesized Indolephenoxyacetamide (IPA) Analogs to Induce Anti-angiogenesis-mediated Solid Tumor Suppression. Pharmacol. Rep. 73, 1328–1343. doi:10.1007/s43440-021-00266-8
Aldinucci, D., Borghese, C., and Casagrande, N. (2020). The CCL5/CCR5 Axis in Cancer Progression. Cancers (Basel) 12. doi:10.3390/cancers12071765
Alven, S., and Aderibigbe, B. A. (2020). Nanoparticles Formulations of Artemisinin and Derivatives as Potential Therapeutics for the Treatment of Cancer, Leishmaniasis and Malaria. Pharmaceutics 12. doi:10.3390/pharmaceutics12080748
Ambasta, R. K., Jha, S. K., Kumar, D., Sharma, R., Jha, N. K., and Kumar, P. (2015). Comparative Study of Anti-angiogenic Activities of Luteolin, Lectin and Lupeol Biomolecules. J. Transl. Med. 13, 307. doi:10.1186/s12967-015-0665-z
Ashrafizadeh, M., Ahmadi, Z., Mohammadinejad, R., Farkhondeh, T., and Samarghandian, S. (2020). MicroRNAs Mediate the Anti-tumor and Protective Effects of Ginsenosides. Nutr. Cancer 72, 1264–1275. doi:10.1080/01635581.2019.1675722
Auzenne, E. J., Klostergaard, J., Mandal, P. K., Liao, W. S., Lu, Z., Gao, F., et al. (2012). A Phosphopeptide Mimetic Prodrug Targeting the SH2 Domain of Stat3 Inhibits Tumor Growth and Angiogenesis. J. Exp. Ther. Oncol. 10, 155–162.
Ayuso-Íñigo, B., Méndez-García, L., Pericacho, M., and Muñoz-Félix, J. M. (2021). The Dual Effect of the BMP9-ALK1 Pathway in Blood Vessels: An Opportunity for Cancer Therapy Improvement? Cancers 13, 5412. doi:10.3390/cancers13215412
Bagli, E., Stefaniotou, M., Morbidelli, L., Ziche, M., Psillas, K., Murphy, C., et al. (2004). Luteolin Inhibits Vascular Endothelial Growth Factor-Induced Angiogenesis; Inhibition of Endothelial Cell Survival and Proliferation by Targeting Phosphatidylinositol 3'-kinase Activity. Cancer Res. 64, 7936–7946. doi:10.1158/0008-5472.Can-03-3104
Balakrishnan, S., Bhat, F. A., Raja Singh, P., Mukherjee, S., Elumalai, P., Das, S., et al. (2016). Gold Nanoparticle-Conjugated Quercetin Inhibits Epithelial-Mesenchymal Transition, Angiogenesis and Invasiveness via EGFR/VEGFR-2-mediated Pathway in Breast Cancer. Cell Prolif. 49, 678–697. doi:10.1111/cpr.12296
Ball, M. S., Bhandari, R., Torres, G. M., Martyanov, V., ElTanbouly, M. A., Archambault, K., et al. (2020). CDDO-me Alters the Tumor Microenvironment in Estrogen Receptor Negative Breast Cancer. Sci. Rep. 10, 6560. doi:10.1038/s41598-020-63482-x
Banerjee, S., Ghosh, T., Barik, S., Das, A., Ghosh, S., Bhuniya, A., et al. (2014). Neem Leaf Glycoprotein Prophylaxis Transduces Immune Dependent Stop Signal for Tumor Angiogenic Switch within Tumor Microenvironment. PLoS One 9, e110040. doi:10.1371/journal.pone.0110040
Banskota, S., Gautam, J., Regmi, S. C., Gurung, P., Park, M. H., Kim, S. J., et al. (2016). BJ-1108, a 6-Amino-2,4,5-Trimethylpyridin-3-Ol Analog, Inhibits Serotonin-Induced Angiogenesis and Tumor Growth through PI3K/NOX Pathway. Plos One 11, e0148133. doi:10.1371/journal.pone.0148133
Bejček, J., Spiwok, V., Kmoníčková, E., and Rimpelová, S. (2021). Na+/K+-ATPase Revisited: On its Mechanism of Action, Role in Cancer, and Activity Modulation. Molecules 26, 1905. doi:10.3390/molecules26071905
Bernard, A., Chevrier, S., Beltjens, F., Dosset, M., Viltard, E., Lagrange, A., et al. (2019). Cleaved Caspase-3 Transcriptionally Regulates Angiogenesis-Promoting Chemotherapy Resistance. Cancer Res. 79, 5958–5970. doi:10.1158/0008-5472.Can-19-0840
Bevara, G. B., Naveen Kumar, A. D., Koteshwaramma, K. L., Badana, A., Kumari, S., and Malla, R. R. (2018). C-glycosyl Flavone from Urginea Indica Inhibits Proliferation & Angiogenesis & Induces Apoptosis via Cyclin-dependent Kinase 6 in Human Breast, Hepatic & Colon Cancer Cell Lines. Indian J. Med. Res. 147, 158–168. doi:10.4103/ijmr.IJMR_51_16
Bhattacharya, S., Ghosh, A., Maiti, S., Ahir, M., Debnath, G. H., Gupta, P., et al. (2020). Delivery of Thymoquinone through Hyaluronic Acid-Decorated Mixed Pluronic® Nanoparticles to Attenuate Angiogenesis and Metastasis of Triple-Negative Breast Cancer. J. Control Release 322, 357–374. doi:10.1016/j.jconrel.2020.03.033
Bhuvaneswari, R., Yuen, G. Y., Chee, S. K., and Olivo, M. (2011). Antiangiogenesis Agents Avastin and Erbitux Enhance the Efficacy of Photodynamic Therapy in a Murine Bladder Tumor Model. Lasers Surg. Med. 43, 651–662. doi:10.1002/lsm.21109
Borella, R., Forti, L., Gibellini, L., De Gaetano, A., De Biasi, S., Nasi, M., et al. (2019). Synthesis and Anticancer Activity of CDDO and CDDO-Me, Two Derivatives of Natural Triterpenoids. Molecules 24. doi:10.3390/molecules24224097
Cantelmo, A. R., Conradi, L. C., Brajic, A., Goveia, J., Kalucka, J., Pircher, A., et al. (2016). Inhibition of the Glycolytic Activator PFKFB3 in Endothelium Induces Tumor Vessel Normalization, Impairs Metastasis, and Improves Chemotherapy. Cancer Cell 30, 968–985. doi:10.1016/j.ccell.2016.10.006
Cao, J., Liu, X., Yang, Y., Wei, B., Li, Q., Mao, G., et al. (2020). Decylubiquinone Suppresses Breast Cancer Growth and Metastasis by Inhibiting Angiogenesis via the ROS/p53/Bai1 Signaling Pathway. Angiogenesis 23, 325–338. doi:10.1007/s10456-020-09707-z
Carbone, C., Piro, G., Merz, V., Simionato, F., Santoro, R., Zecchetto, C., et al. (2018). Angiopoietin-Like Proteins in Angiogenesis, Inflammation and Cancer. Int. J. Mol. Sci. 19. doi:10.3390/ijms19020431
Carew, J. S., Esquivel, J. A., Espitia, C. M., Schultes, C. M., Mülbaier, M., Lewis, J. D., et al. II (2012). ELR510444 Inhibits Tumor Growth and Angiogenesis by Abrogating HIF Activity and Disrupting Microtubules in Renal Cell Carcinoma. Plos One 7, e31120. doi:10.1371/journal.pone.0031120
Cayrol, F., Sterle, H. A., Díaz Flaqué, M. C., Barreiro Arcos, M. L., and Cremaschi, G. A. (2019). Non-genomic Actions of Thyroid Hormones Regulate the Growth and Angiogenesis of T Cell Lymphomas. Front. Endocrinol. (Lausanne) 10, 63. doi:10.3389/fendo.2019.00063
Chakraborty, S., Njah, K., and Hong, W. (2020). Agrin Mediates Angiogenesis in the Tumor Microenvironment. Trends Cancer 6, 81–85. doi:10.1016/j.trecan.2019.12.002
Chan, L. S., Yue, P. Y., Wong, Y. Y., and Wong, R. N. (2013). MicroRNA-15b Contributes to Ginsenoside-Rg1-Induced Angiogenesis through Increased Expression of VEGFR-2. Biochem. Pharmacol. 86, 392–400. doi:10.1016/j.bcp.2013.05.006
Chen, C., You, F., Wu, F., Luo, Y., zheng, G., Xu, H., et al. (2020). Antiangiogenesis Efficacy of Ethanol Extract from Amomum Tsaoko in Ovarian Cancer through Inducing ER Stress to Suppress p-STAT3/NF-kB/IL-6 and VEGF Loop. Evid. Based Complement. Altern. Med. 2020, 2390125. doi:10.1155/2020/2390125
Chen, H. W., Yang, C. C., Hsieh, C. L., Liu, H., Lee, S. C., and Tan, B. C. (2013). A Functional Genomic Approach Reveals the Transcriptional Role of EDD in the Expression and Function of Angiogenesis Regulator ACVRL1. Biochim. Biophys. Acta 1829, 1309–1319. doi:10.1016/j.bbagrm.2013.10.006
Chen, J. L., Zhang, H., Huang, X. Q., Wan, H. Y., Li, J., Fan, X. X., et al. (2019). Antiangiogenesis-Combined Photothermal Therapy in the Second Near-Infrared Window at Laser Powers below the Skin Tolerance Threshold. Nanomicro Lett. 11, 93. doi:10.1007/s40820-019-0327-4
Chen, J. Y., Li, C. F., Chu, P. Y., Lai, Y. S., Chen, C. H., Jiang, S. S., et al. (2016). Lysine Demethylase 2A Promotes Stemness and Angiogenesis of Breast Cancer by Upregulating Jagged1. Oncotarget 7, 27689–27710. doi:10.18632/oncotarget.8381
Chen, L. K., Qiang, P. F., Xu, Q. P., Zhao, Y. H., Dai, F., and Zhang, L. (2013). Trans-3,4,5,4'-tetramethoxystilbene, a Resveratrol Analog, Potently Inhibits Angiogenesis In Vitro and In Vivo. Acta Pharmacol. Sin. 34, 1174–1182. doi:10.1038/aps.2013.60
Chen, Q., Hongu, T., Sato, T., Zhang, Y., Ali, W., Cavallo, J. A., et al. (2012). Key Roles for the Lipid Signaling Enzyme Phospholipase D1 in the Tumor Microenvironment during Tumor Angiogenesis and Metastasis. Sci. Signal 5, ra79. doi:10.1126/scisignal.2003257
Chen, Q., Zhuang, Q., Mao, W., Xu, X. M., Wang, L. H., and Wang, H. B. (2014). Inhibitory Effect of Cryptotanshinone on Angiogenesis and Wnt/β-Catenin Signaling Pathway in Human Umbilical Vein Endothelial Cells. Chin. J. Integr. Med. 20, 743–750. doi:10.1007/s11655-014-1810-x
Chen, S. H., Murphy, D. A., Lassoued, W., Thurston, G., Feldman, M. D., and Lee, W. M. (2008). Activated STAT3 Is a Mediator and Biomarker of VEGF Endothelial Activation. Cancer Biol. Ther. 7, 1994–2003. doi:10.4161/cbt.7.12.6967
Chen, X., Qian, H., Qiao, H., Dong, B., Chen, E., Huang, D., et al. (2020). Tumor-Adhesive and pH-Degradable Microgels by Microfluidics and Photo-Cross-Linking for Efficient Antiangiogenesis and Enhanced Cancer Chemotherapy. Biomacromolecules 21, 1285–1294. doi:10.1021/acs.biomac.0c00049
Chen, Z., Tong, L. J., Tang, B. Y., Liu, H. Y., Wang, X., Zhang, T., et al. (2019). C11, a Novel Fibroblast Growth Factor Receptor 1 (FGFR1) Inhibitor, Suppresses Breast Cancer Metastasis and Angiogenesis. Acta Pharmacol. Sin. 40, 823–832. doi:10.1038/s41401-018-0191-7
Cheng, J. Y., Wang, S. H., Lin, J., Tsai, Y. C., Yu, J., Wu, J. C., et al. (2014). Globo-H Ceramide Shed from Cancer Cells Triggers Translin-Associated Factor X-dependent Angiogenesis. Cancer Res. 74, 6856–6866. doi:10.1158/0008-5472.CAN-14-1651
Cheng, N., Bei, Y., Song, Y., Zhang, W., Xu, L., Zhang, W., et al. (2021). B7-H3 Augments the Pro-angiogenic Function of Tumor-Associated Macrophages and Acts as a Novel Adjuvant Target for Triple-Negative Breast Cancer Therapy. Biochem. Pharmacol. 183, 114298. doi:10.1016/j.bcp.2020.114298
Choi, M. A., Saeidi, S., Han, H. J., Kim, S. J., Kwon, N., Kim, D. H., et al. (2020). The Peptidyl Prolyl Isomerase, PIN1 Induces Angiogenesis through Direct Interaction with HIF-2α. Biochem. Biophys. Res. Commun. 533, 995–1003. doi:10.1016/j.bbrc.2020.08.015
Chuang, J. Y., Chen, P. C., Tsao, C. W., Chang, A. C., Lein, M. Y., Lin, C. C., et al. (2015). WISP-1 a Novel Angiogenic Regulator of the CCN Family Promotes Oral Squamous Cell Carcinoma Angiogenesis through VEGF-A Expression. Oncotarget 6, 4239–4252. doi:10.18632/oncotarget.2978
Crespo-Ortiz, M. P., and Wei, M. Q. (2012). Antitumor Activity of Artemisinin and its Derivatives: from a Well-Known Antimalarial Agent to a Potential Anticancer Drug. J. Biomed. Biotechnol. 2012, 247597. doi:10.1155/2012/247597
Cruz, L. A., Tellman, T. V., and Farach-Carson, M. C. (2020). Flipping the Molecular Switch: Influence of Perlecan and its Modifiers in the Tumor Microenvironment. Adv. Exp. Med. Biol. 1245, 133–146. doi:10.1007/978-3-030-40146-7_6
Dahmani, F. Z., Xiao, Y., Zhang, J., Yu, Y., Zhou, J., and Yao, J. (2016). Multifunctional Polymeric Nanosystems for Dual-Targeted Combinatorial Chemo/Angiogenesis Therapy of Tumors. Adv. Healthc. Mater 5, 1447–1461. doi:10.1002/adhm.201600169
Dai, F., Gao, L., Zhao, Y., Wang, C., and Xie, S. (2016). Farrerol Inhibited Angiogenesis through Akt/mTOR, Erk and Jak2/Stat3 Signal Pathway. Phytomedicine 23, 686–693. doi:10.1016/j.phymed.2016.03.008
De Bock, K., Georgiadou, M., Schoors, S., Kuchnio, A., Wong, B. W., Cantelmo, A. R., et al. (2013). Role of PFKFB3-Driven Glycolysis in Vessel Sprouting. Cell 154, 651–663. doi:10.1016/j.cell.2013.06.037
Deep, G., and Agarwal, R. (2013). Targeting Tumor Microenvironment with Silibinin: Promise and Potential for a Translational Cancer Chemopreventive Strategy. Curr. Cancer Drug Targets 13, 486–499. doi:10.2174/15680096113139990041
Deep, G., Gangar, S. C., Rajamanickam, S., Raina, K., Gu, M., Agarwal, C., et al. (2012). Angiopreventive Efficacy of Pure Flavonolignans from Milk Thistle Extract against Prostate Cancer: Targeting VEGF-VEGFR Signaling. PloS one 7, e34630. doi:10.1371/journal.pone.0034630
Deng, Y., Jiang, Z., Jin, Y., Qiao, J., Yang, S., Xiong, H., et al. (2021). Reinforcing Vascular Normalization Therapy with a Bi-directional Nano-System to Achieve Therapeutic-Friendly Tumor Microenvironment. J. Control Release 340, 87–101. doi:10.1016/j.jconrel.2021.10.016
Ding, C., Tian, Q., Li, J., Jiao, M., Song, S., Wang, Y., et al. (2018). Structural Modification of Natural Product Tanshinone I Leading to Discovery of Novel Nitrogen-Enriched Derivatives with Enhanced Anticancer Profile and Improved Drug-like Properties. J. Med. Chem. 61, 760–776. doi:10.1021/acs.jmedchem.7b01259
Dings, R. P. M., Miller, M. C., Griffin, R. J., and Mayo, K. H. (2018). Galectins as Molecular Targets for Therapeutic Intervention. Int. J. Mol. Sci. 19. doi:10.3390/ijms19030905
Dong, F., Tian, H., Yan, S., Li, L., Dong, X., Wang, F., et al. (2015). Dihydroartemisinin Inhibits Endothelial Cell Proliferation through the Suppression of the ERK Signaling Pathway. Int. J. Mol. Med. 35, 1381–1387. doi:10.3892/ijmm.2015.2140
Dong, F., Zhou, X., Li, C., Yan, S., Deng, X., Cao, Z., et al. (2014). Dihydroartemisinin Targets VEGFR2 via the NF-Κb Pathway in Endothelial Cells to Inhibit Angiogenesis. Cancer Biol. Ther. 15, 1479–1488. doi:10.4161/15384047.2014.955728
Du, Y., Zheng, Y., Yu, C. X., Zhong, L., Li, Y., Wu, B., et al. (2021). The Mechanisms of Yu Ping Feng San in Tracking the Cisplatin-Resistance by Regulating ATP-Binding Cassette Transporter and Glutathione S-Transferase in Lung Cancer Cells. Front. Pharmacol. 12. doi:10.3389/fphar.2021.678126
Fang, B., Chen, X., Wu, M., Kong, H., Chu, G., Zhou, Z., et al. (2018). Luteolin Inhibits Angiogenesis of the M2-like TAMs via the D-ownregulation of H-ypoxia I-nducible F-actor-1α and the STAT3 S-ignalling P-athway under H-ypoxia. Mol. Med. Rep. 18, 2914–2922. doi:10.3892/mmr.2018.9250
Fang, K., Zhan, Y., Zhu, R., Wang, Y., Wu, C., Sun, M., et al. (2021). Bufalin Suppresses Tumour Microenvironment-Mediated Angiogenesis by Inhibiting the STAT3 Signalling Pathway. J. Transl. Med. 19, 383. doi:10.1186/s12967-021-03058-z
Feng, R., Chen, Y., Liu, Y., Zhou, Q., and Zhang, W. (2021). The Role of B7-H3 in Tumors and its Potential in Clinical Application. Int. Immunopharmacol. 101, 108153. doi:10.1016/j.intimp.2021.108153
Fokas, E., McKenna, W. G., and Muschel, R. J. (2012). The Impact of Tumor Microenvironment on Cancer Treatment and its Modulation by Direct and Indirect Antivascular Strategies. Cancer Metastasis Rev. 31, 823–842. doi:10.1007/s10555-012-9394-4
Folkman, J. (2007). Angiogenesis: an Organizing Principle for Drug Discovery? Nat. Rev. Drug Discov. 6, 273–286. doi:10.1038/nrd2115
Fousek, K., Horn, L. A., and Palena, C. (2021). Interleukin-8: A Chemokine at the Intersection of Cancer Plasticity, Angiogenesis, and Immune Suppression. Pharmacol. Ther. 219, 107692. doi:10.1016/j.pharmthera.2020.107692
Fu, X., Yang, Y., Li, X., Lai, H., Huang, Y., He, L., et al. (2016). RGD Peptide-Conjugated Selenium Nanoparticles: Antiangiogenesis by Suppressing VEGF-VEGFR2-ERK/AKT Pathway. Nanomedicine 12, 1627–1639. doi:10.1016/j.nano.2016.01.012
Fukumura, D., Kloepper, J., Amoozgar, Z., Duda, D. G., and Jain, R. K. (2018). Enhancing Cancer Immunotherapy Using Antiangiogenics: Opportunities and Challenges. Nat. Rev. Clin. Oncol. 15, 325–340. doi:10.1038/nrclinonc.2018.29
Gao, P., Wang, L. L., Liu, J., Dong, F., Song, W., Liao, L., et al. (2020). Dihydroartemisinin Inhibits Endothelial Cell Tube Formation by Suppression of the STAT3 Signaling Pathway. Life Sci. 242, 117221. doi:10.1016/j.lfs.2019.117221
García-Maceira, P., and Mateo, J. (2009). Silibinin Inhibits Hypoxia-Inducible Factor-1α and mTOR/p70S6K/4E-BP1 Signalling Pathway in Human Cervical and Hepatoma Cancer Cells: Implications for Anticancer Therapy. Oncogene 28, 313–324. doi:10.1038/onc.2008.398
Gately, S., and Li, W. W. (2004). Multiple Roles of COX-2 in Tumor Angiogenesis: a Target for Antiangiogenic Therapy. Semin. Oncol. 31, 2–11. doi:10.1053/j.seminoncol.2004.03.040
Ghazizadeh, F., Shafiei, M., Falak, R., Panahi, M., Rakhshani, N., Ebrahimi, S. A., et al. (2020). Xanthomicrol Exerts Antiangiogenic and Antitumor Effects in a Mouse Melanoma (B16F10) Allograft Model. Evid. Based Complement. Altern. Med. 2020, 8543872. doi:10.1155/2020/8543872
Goswami, K. K., Barik, S., Sarkar, M., Bhowmick, A., Biswas, J., Bose, A., et al. (2014). Targeting STAT3 Phosphorylation by Neem Leaf Glycoprotein Prevents Immune Evasion Exerted by Supraglottic Laryngeal Tumor Induced M2 Macrophages. Mol. Immunol. 59, 119–127. doi:10.1016/j.molimm.2014.01.015
Guan, L., Zhou, J., Lin, Q., Zhu, H., Liu, W., Liu, B., et al. (2019). Design, Synthesis and Antitumour and Anti-angiogenesis Evaluation of 22 Moscatilin Derivatives. Bioorg Med. Chem. 27, 2657–2665. doi:10.1016/j.bmc.2019.04.027
Güner, A., Polatli, E., Akkan, T., Bektaş, H., and Albay, C. (2019). Anticancer and Antiangiogenesis Activities of Novel Synthesized 2-substitutedbenzimidazoles Molecules. Turk J. Chem. 43, 1270–1289. doi:10.3906/kim-1904-46
Guo, R., Li, L., Su, J., Li, S., Duncan, S. E., Liu, Z., et al. (2020). Pharmacological Activity and Mechanism of Tanshinone IIA in Related Diseases. Drug Des. Devel Ther. 14, 4735–4748. doi:10.2147/DDDT.S266911
Guo, X., and Ding, X. (2018). Dioscin Suppresses the Viability of Ovarian Cancer Cells by Regulating the VEGFR2 and PI3K/AKT/MAPK Signaling Pathways. Oncol. Lett. 15, 9537–9542. doi:10.3892/ol.2018.8454
Guo, X., Qian, X., Jin, Y., Kong, X., Qi, Z., Cai, T., et al. (2021). Hypertension Induced by Combination Therapy of Cancer: A Systematic Review and Meta-Analysis of Global Clinical Trials. Front. Pharmacol. 12, 12. doi:10.3389/fphar.2021.712995
Hack, S. P., Zhu, A. X., and Wang, Y. (2020). Augmenting Anticancer Immunity through Combined Targeting of Angiogenic and PD-1/pd-L1 Pathways: Challenges and Opportunities. Front. Immunol. 11, 598877. doi:10.3389/fimmu.2020.598877
Hameed, S., Bhattarai, P., and Dai, Z. (2018). Nanotherapeutic Approaches Targeting Angiogenesis and Immune Dysfunction in Tumor Microenvironment. Sci. China Life Sci. 61, 380–391. doi:10.1007/s11427-017-9256-1
Hashemi Goradel, N., Najafi, M., Salehi, E., Farhood, B., and Mortezaee, K. (2019). Cyclooxygenase-2 in Cancer: A Review. J. Cell Physiol. 234, 5683–5699. doi:10.1002/jcp.27411
Hatami, E., Jaggi, M., Chauhan, S. C., and Yallapu, M. M. (2020). Gambogic Acid: A Shining Natural Compound to Nanomedicine for Cancer Therapeutics. Biochim. Biophys. Acta Rev. Cancer 1874, 188381. doi:10.1016/j.bbcan.2020.188381
He, S., Tian, S., He, X., Le, X., Ning, Y., Chen, J., et al. (2021). Multiple Targeted Self-Emulsifying Compound RGO Reveals Obvious Anti-tumor Potential in Hepatocellular Carcinoma. Mol. Ther. Oncolytics 22, 604–616. doi:10.1016/j.omto.2021.08.008
He, X. R., Han, S. Y., and Li, P. P. (2015). Recent Highlights of Experimental Research for Inhibiting Tumor Growth by Using Chinese Medicine. Chin. J. Integr. Med. 21, 727–732. doi:10.1007/s11655-015-2318-8
Heinrich, M., Appendino, G., Efferth, T., Fürst, R., Izzo, A. A., Kayser, O., et al. (2020). Best Practice in Research - Overcoming Common Challenges in Phytopharmacological Research. J. Ethnopharmacol. 246, 112230. doi:10.1016/j.jep.2019.112230
Hosseini, A., Rasmi, Y., Rahbarghazi, R., Aramwit, P., Daeihassani, B., and Saboory, E. (2019). Curcumin Modulates the Angiogenic Potential of Human Endothelial Cells via FAK/P-38 MAPK Signaling Pathway. Gene 688, 7–12. doi:10.1016/j.gene.2018.11.062
Hu, X., Mandika, C., He, L., You, Y., Chang, Y., Wang, J., et al. (2019). Construction of Urokinase-type Plasminogen Activator Receptor-Targeted Heterostructures for Efficient Photothermal Chemotherapy against Cervical Cancer to Achieve Simultaneous Anticancer and Antiangiogenesis. ACS Appl. Mater Interfaces 11, 39688–39705. doi:10.1021/acsami.9b15751
Huang, C. S., Yu, A. L., Tseng, L. M., Chow, L. W. C., Hou, M. F., Hurvitz, S. A., et al. (2020). Globo H-KLH Vaccine Adagloxad Simolenin (OBI-822)/OBI-821 in Patients with Metastatic Breast Cancer: Phase II Randomized, Placebo-Controlled Study. J. Immunother. Cancer 8, 8. doi:10.1136/jitc-2019-000342
Huang, H., Yao, Y., Hou, G., Zhao, C., Qin, J., Zhang, Y., et al. (2021). Design, Synthesis and Biological Evaluation of Tanshinone IIA-Based Analogues: Potent Inhibitors of Microtubule Formation and Angiogenesis. Eur. J. Med. Chem. 224, 113708. doi:10.1016/j.ejmech.2021.113708
Iozzo, M., Sgrignani, G., Comito, G., Chiarugi, P., and Giannoni, E. (2021). Endocannabinoid System and Tumour Microenvironment: New Intertwined Connections for Anticancer Approaches. Cells 10. doi:10.3390/cells10123396
Jain, R. K., Duda, D. G., Clark, J. W., and Loeffler, J. S. (2006). Lessons from Phase III Clinical Trials on Anti-VEGF Therapy for Cancer. Nat. Clin. Pract. Oncol. 3, 24–40. doi:10.1038/ncponc0403
Jang, J., Kim, M. R., Kim, T. K., Lee, W. R., Kim, J. H., Heo, K., et al. (2017). CLEC14a-HSP70-1A Interaction Regulates HSP70-1a-Induced Angiogenesis. Sci. Rep. 7, 10666. doi:10.1038/s41598-017-11118-y
Jiang, M., He, K., Qiu, T., Sun, J., Liu, Q., Zhang, X., et al. (2020). Tumor-targeted Delivery of Silibinin and IPI-549 Synergistically Inhibit Breast Cancer by Remodeling the Microenvironment. Int. J. Pharm. 581, 119239. doi:10.1016/j.ijpharm.2020.119239
Jiang, Z., Wang, L., Liu, X., Chen, C., Wang, B., Wang, W., et al. (2020). Discovery of a Highly Selective VEGFR2 Kinase Inhibitor CHMFL-VEGFR2-002 as a Novel Anti-angiogenesis Agent. Acta Pharm. Sin. B 10, 488–497. doi:10.1016/j.apsb.2019.10.004
Jiang, Z., He, L., Yu, X., Yang, Z., Wu, W., Wang, X., et al. (2021). Antiangiogenesis Combined with Inhibition of the Hypoxia Pathway Facilitates Low-Dose, X-Ray-Induced Photodynamic Therapy. ACS Nano 15, 11112–11125. doi:10.1021/acsnano.1c01063
Johnson, D. H., Fehrenbacher, L., Novotny, W. F., Herbst, R. S., Nemunaitis, J. J., Jablons, D. M., et al. (2004). Randomized Phase II Trial Comparing Bevacizumab Plus Carboplatin and Paclitaxel with Carboplatin and Paclitaxel Alone in Previously Untreated Locally Advanced or Metastatic Non-small-cell Lung Cancer. J. Clin. Oncol. 22, 2184–2191. doi:10.1200/jco.2004.11.022
Kamran, S., Sinniah, A., Abdulghani, M. A. M., and Alshawsh, M. A. (2022). Therapeutic Potential of Certain Terpenoids as Anticancer Agents: A Scoping Review. Cancers (Basel) 14. doi:10.3390/cancers14051100
Khan, K. A., McMurray, J. L., Mohammed, F., and Bicknell, R. (2019). C-type Lectin Domain Group 14 Proteins in Vascular Biology, Cancer and Inflammation. FEBS J. 286, 3299–3332. doi:10.1111/febs.14985
Kim, D. G., Kang, Y., Lee, H., Lee, E. K., Nam, T. G., Kim, J. A., et al. (2014). 6-Amino-2,4,5-trimethylpyridin-3-ols: A New General Synthetic Route and Antiangiogenic Activity. Eur. J. Med. Chem. 78, 126–139. doi:10.1016/j.ejmech.2014.03.045
Kim, T. K., Park, C. S., Jang, J., Kim, M. R., Na, H. J., Lee, K., et al. (2018). Inhibition of VEGF-dependent Angiogenesis and Tumor Angiogenesis by an Optimized Antibody Targeting CLEC14a. Mol. Oncol. 12, 356–372. doi:10.1002/1878-0261.12169
Kollmann, K., Heller, G., Schneckenleithner, C., Warsch, W., Scheicher, R., Ott, R. G., et al. (2013). A Kinase-independent Function of CDK6 Links the Cell Cycle to Tumor Angiogenesis. Cancer Cell 24, 167–181. doi:10.1016/j.ccr.2013.07.012
Kotowski, K., Rosik, J., Machaj, F., Supplitt, S., Wiczew, D., Jabłońska, K., et al. (2021). Role of PFKFB3 and PFKFB4 in Cancer: Genetic Basis, Impact on Disease Development/Progression, and Potential as Therapeutic Targets. Cancers (Basel) 13. doi:10.3390/cancers13040909
Kumar, A. R., Devan, A. R., Nair, B., and Nath, L. R. (2021). Anti-VEGF Mediated Immunomodulatory Role of Phytochemicals: Scientific Exposition for Plausible HCC Treatment. Curr. Drug Targets 22, 1288–1316. doi:10.2174/1389450122666210203194036
Kumar, M., Dhatwalia, S. K., and Dhawan, D. K. (2016). Role of Angiogenic Factors of Herbal Origin in Regulation of Molecular Pathways that Control Tumor Angiogenesis. Tumour Biol. 37, 14341–14354. doi:10.1007/s13277-016-5330-5
Lee, H. P., Liu, Y. C., Chen, P. C., Tai, H. C., Li, T. M., Fong, Y. C., et al. (2017). Tanshinone IIA Inhibits Angiogenesis in Human Endothelial Progenitor Cells In Vitro and In Vivo. Oncotarget 8, 109217–109227. doi:10.18632/oncotarget.22649
Lee, J. S., Kang, J. H., Boo, H. J., Hwang, S. J., Hong, S., Lee, S. C., et al. (2015). STAT3-mediated IGF-2 Secretion in the Tumour Microenvironment Elicits Innate Resistance to Anti-IGF-1r Antibody. Nat. Commun. 6, 8499. doi:10.1038/ncomms9499
Lee, S., Rho, S. S., Park, H., Park, J. A., Kim, J., Lee, I. K., et al. (2017). Carbohydrate-binding Protein CLEC14A Regulates VEGFR-2- and VEGFR-3-dependent Signals during Angiogenesis and Lymphangiogenesis. J. Clin. Invest. 127, 457–471. doi:10.1172/jci85145
Lee, W. S., Yang, H., Chon, H. J., and Kim, C. (2020). Combination of Anti-angiogenic Therapy and Immune Checkpoint Blockade Normalizes Vascular-Immune Crosstalk to Potentiate Cancer Immunity. Exp. Mol. Med. 52, 1475–1485. doi:10.1038/s12276-020-00500-y
Li, C., Wang, Q., Shen, S., Wei, X., and Li, G. (2019). HIF-1α/VEGF Signaling-Mediated Epithelial-Mesenchymal Transition and Angiogenesis Is Critically Involved in Anti-metastasis Effect of Luteolin in Melanoma Cells. Phytother. Res. 33, 798–807. doi:10.1002/ptr.6273
Li, G., Shan, C., Liu, L., Zhou, T., Zhou, J., Hu, X., et al. (2015). Tanshinone IIA Inhibits HIF-1α and VEGF Expression in Breast Cancer Cells via mTOR/p70S6K/RPS6/4E-BP1 Signaling Pathway. PLoS One 10, e0117440. doi:10.1371/journal.pone.0117440
Li, J., Gao, A., Zhang, F., Wang, S., Wang, J., Wang, J., et al. (2021). ILT3 Promotes Tumor Cell Motility and Angiogenesis in Non-small Cell Lung Cancer. Cancer Lett. 501, 263–276. doi:10.1016/j.canlet.2020.10.048
Li, J. J., Mao, X. H., Tian, T., Wang, W. M., Su, T., Jiang, C. H., et al. (2019). Role of PFKFB3 and CD163 in Oral Squamous Cell Carcinoma Angiogenesis. Curr. Med. Sci. 39, 410–414. doi:10.1007/s11596-019-2051-1
Li, M., Wang, X., Wang, Y., Bao, S., Chang, Q., Liu, L., et al. (2021). Strategies for Remodeling the Tumor Microenvironment Using Active Ingredients of Ginseng-A Promising Approach for Cancer Therapy. Front. Pharmacol. 12, 12. doi:10.3389/fphar.2021.797634
Li, R., Song, X., Guo, Y., Song, P., Duan, D., and Chen, Z.-S. (2021). Natural Products: A Promising Therapeutics for Targeting Tumor Angiogenesis. Front. Oncol. 11, 11. doi:10.3389/fonc.2021.772915
Li, W. W., Yu, J. Y., Xu, H. L., and BaoConcanavalin, J. K. A. (2011). Concanavalin A: a Potential Anti-neoplastic Agent Targeting Apoptosis, Autophagy and Anti-angiogenesis for Cancer Therapeutics. Biochem. Biophys. Res. Commun. 414, 282–286. doi:10.1016/j.bbrc.2011.09.072
Li, X., Chen, M., Lei, X., Huang, M., Ye, W., Zhang, R., et al. (2017). Luteolin Inhibits Angiogenesis by Blocking Gas6/Axl Signaling Pathway. Int. J. Oncol. 51, 677–685. doi:10.3892/ijo.2017.4041
Li, Y., Liu, Y., Du, B., and Cheng, G. (2020). Reshaping Tumor Blood Vessels to Enhance Drug Penetration with a Multistrategy Synergistic Nanosystem. Mol. Pharm. 17, 3151–3164. doi:10.1021/acs.molpharmaceut.0c00077
Li, Y., Zhou, X., Liu, J., Yuan, X., and He, Q. (2020). Therapeutic Potentials and Mechanisms of Artemisinin and its Derivatives for Tumorigenesis and Metastasis. Anticancer Agents Med. Chem. 20, 520–535. doi:10.2174/1871520620666200120100252
Li, Z., Ding, X., Wu, H., and Liu, C. (2019). Artemisinin Inhibits Angiogenesis by Regulating P38 MAPK/CREB/TSP-1 Signaling Pathway in Osteosarcoma. J. Cell Biochem. 120, 11462–11470. doi:10.1002/jcb.28424
Liang, Q., Zhou, L., Li, Y., Liu, J., and Liu, Y. (2022). Nano Drug Delivery System Reconstruct Tumour Vasculature for the Tumour Vascular Normalisation. J. Drug Target 30, 119–130. doi:10.1080/1061186x.2021.1927056
Lin, W., Wang, H., Zhong, M., Yu, S., Zhao, S., Liang, S., et al. (2021). Effect and Molecular Mechanisms of Jiedu Recipe on Hypoxia-Induced Angiogenesis after Transcatheter Arterial Chemoembolization in Hepatocellular Carcinoma. Evid. Based Complement. Altern. Med. 2021, 6529376. doi:10.1155/2021/6529376
Liu, J., Ren, Y., Hou, Y., Zhang, C., Wang, B., Li, X., et al. (2019). Dihydroartemisinin Induces Endothelial Cell Autophagy through Suppression of the Akt/mTOR Pathway. J. Cancer 10, 6057–6064. doi:10.7150/jca.33704
Liu, T., Shi, C., Duan, L., Zhang, Z., Luo, L., Goel, S., et al. (2018). A Highly Hemocompatible Erythrocyte Membrane-Coated Ultrasmall Selenium Nanosystem for Simultaneous Cancer Radiosensitization and Precise Antiangiogenesis. J. Mater Chem. B 6, 4756–4764. doi:10.1039/c8tb01398e
Liu, Y., Chen, Y., Lin, L., and Li, H. (2020). Gambogic Acid as a Candidate for Cancer Therapy: A Review. Int. J. Nanomedicine 15, 10385–10399. doi:10.2147/ijn.S277645
Liu, Y., Li, H., Zhao, C., and Jia, H. (2018). MicroRNA-101 Inhibits Angiogenesis via COX-2 in Endometrial Carcinoma. Mol. Cell Biochem. 448, 61–69. doi:10.1007/s11010-018-3313-0
Liu, Y.-X., Xu, B.-W., Niu, X.-D., Chen, Y.-J., Fu, X.-Q., Wang, X.-Q., et al. (2022). Inhibition of Src/STAT3 Signaling-Mediated Angiogenesis Is Involved in the Anti-melanoma Effects of Dioscin. Pharmacol. Res. 175, 105983. doi:10.1016/j.phrs.2021.105983
Liu, Z., Liu, T., Li, W., Li, J., Wang, C., and Zhang, K. (2021). Insights into the Antitumor Mechanism of Ginsenosides Rg3. Mol. Biol. Rep. 48, 2639–2652. doi:10.1007/s11033-021-06187-2
Liu, Z., Zhao, Q., Zheng, Z., Liu, S., Meng, L., Dong, L., et al. (2021). Vascular Normalization in Immunotherapy: A Promising Mechanisms Combined with Radiotherapy. Biomed. Pharmacother. 139, 111607. doi:10.1016/j.biopha.2021.111607
Lu, N., Yang, Y., You, Q. D., Ling, Y., Gao, Y., Gu, H. Y., et al. (2007). Gambogic Acid Inhibits Angiogenesis through Suppressing Vascular Endothelial Growth Factor-Induced Tyrosine Phosphorylation of KDR/Flk-1. Cancer Lett. 258, 80–89. doi:10.1016/j.canlet.2007.08.015
Lu, Y., Wen, Q., Luo, J., Xiong, K., Wu, Z., Wang, B., et al. (2020). Self-assembled Dihydroartemisinin Nanoparticles as a Platform for Cervical Cancer Chemotherapy. Drug Deliv. 27, 876–887. doi:10.1080/10717544.2020.1775725
Lund, M. E., Campbell, D. H., and Walsh, B. J. (2020). The Role of Glypican-1 in the Tumour Microenvironment. Adv. Exp. Med. Biol. 1245, 163–176. doi:10.1007/978-3-030-40146-7_8
Marrero, A. D., Quesada, A. R., Martínez-Poveda, B., and Medina, M. Á. (2022). Antiangiogenic Phytochemicals Constituent of Diet as Promising Candidates for Chemoprevention of Cancer. Antioxidants 11, 302. doi:10.3390/antiox11020302
Mi, C., Cao, X., Ma, K., Wei, M., Xu, W., Lin, Y., et al. (2022). Digitoxin Promotes Apoptosis and Inhibits Proliferation and Migration by Reducing HIF-1α and STAT3 in KRAS Mutant Human Colon Cancer Cells. Chem. Biol. Interact. 351, 109729. doi:10.1016/j.cbi.2021.109729
Mi, C., Ma, J., Wang, K. S., Zuo, H. X., Wang, Z., Li, M. Y., et al. (2017). Imperatorin Suppresses Proliferation and Angiogenesis of Human Colon Cancer Cell by Targeting HIF-1α via the mTOR/p70S6K/4E-BP1 and MAPK Pathways. J. Ethnopharmacol. 203, 27–38. doi:10.1016/j.jep.2017.03.033
Min, H., Wang, J., Qi, Y., Zhang, Y., Han, X., Xu, Y., et al. (2019). Biomimetic Metal-Organic Framework Nanoparticles for Cooperative Combination of Antiangiogenesis and Photodynamic Therapy for Enhanced Efficacy. Adv. Mater 31, e1808200. doi:10.1002/adma.201808200
Mir Hassani, Z., Nabiuni, M., Parivar, K., Abdirad, S., and Karimzadeh, L. (2021). Melittin Inhibits the Expression of Key Genes Involved in Tumor Microenvironment Formation by Suppressing HIF-1α Signaling in Breast Cancer Cells. Med. Oncol. 38, 77. doi:10.1007/s12032-021-01526-6
Mohankumar, K., Francis, A. P., Pajaniradje, S., and Rajagopalan, R. (2021). Synthetic Curcumin Analog: Inhibiting the Invasion, Angiogenesis, and Metastasis in Human Laryngeal Carcinoma Cells via NF-kB Pathway. Mol. Biol. Rep. 48, 6065–6074. doi:10.1007/s11033-021-06610-8
Mooi, J., Chionh, F., Savas, P., Da Gama Duarte, J., Chong, G., Brown, S., et al. (2021). Dual Antiangiogenesis Agents Bevacizumab Plus Trebananib, without Chemotherapy, in First-Line Treatment of Metastatic Colorectal Cancer: Results of a Phase II Study. Clin. Cancer Res. 27, 2159–2167. doi:10.1158/1078-0432.CCR-20-2714
Mura, M., Swain, R. K., Zhuang, X., Vorschmitt, H., Reynolds, G., Durant, S., et al. (2012). Identification and Angiogenic Role of the Novel Tumor Endothelial Marker CLEC14A. Oncogene 31, 293–305. doi:10.1038/onc.2011.233
Nakhjavani, M., Smith, E., Townsend, A. R., Price, T. J., and Hardingham, J. E. (2020). Anti-Angiogenic Properties of Ginsenoside Rg3. Molecules 25. doi:10.3390/molecules25214905
Nakhjavani, M., Smith, E., Yeo, K., Palethorpe, H. M., Tomita, Y., Price, T. J., et al. (2021). Anti-Angiogenic Properties of Ginsenoside Rg3 Epimers: In Vitro Assessment of Single and Combination Treatments. Cancers (Basel) 13. doi:10.3390/cancers13092223
Nambiar, D., Prajapati, V., Agarwal, R., and Singh, R. P. (2013). In Vitro and In Vivo Anticancer Efficacy of Silibinin against Human Pancreatic Cancer BxPC-3 and PANC-1 Cells. Cancer Lett. 334, 109–117. doi:10.1016/j.canlet.2012.09.004
Neves, K. B., Montezano, A. C., Lang, N. N., and Touyz, R. M. (2020). Vascular Toxicity Associated with Anti-angiogenic Drugs. Clin. Sci. (Lond) 134, 2503–2520. doi:10.1042/cs20200308
Ni, J., Wang, X., Stojanovic, A., Zhang, Q., Wincher, M., Bühler, L., et al. (2020). Single-Cell RNA Sequencing of Tumor-Infiltrating NK Cells Reveals that Inhibition of Transcription Factor HIF-1α Unleashes NK Cell Activity. Immunity 52, 1075–e8. e1078. doi:10.1016/j.immuni.2020.05.001
Nicolin, V., Bossi, F., Viggiano, A., Valentini, R., and Nori, S. L. (2013). Tanshinone VI Inhibits the Expression of Intercellular Adhesion Molecule-1 and Vascular Cell Adhesion Molecule-1. Int. J. Immunopathol. Pharmacol. 26, 977–982. doi:10.1177/039463201302600418
Niu, G., Wright, K. L., Huang, M., Song, L., Haura, E., Turkson, J., et al. (2002). Constitutive Stat3 Activity Up-Regulates VEGF Expression and Tumor Angiogenesis. Oncogene 21, 2000–2008. doi:10.1038/sj.onc.1205260
Niu, N., Yu, C., Li, L., Liu, Q., Zhang, W., Liang, K., et al. (2018). Dihydroartemisinin Enhances VEGFR1 Expression through Up-Regulation of ETS-1 Transcription Factor. J. Cancer 9, 3366–3372. doi:10.7150/jca.25082
Noy, P. J., Swain, R. K., Khan, K., Lodhia, P., and Bicknell, R. (2016). Sprouting Angiogenesis Is Regulated by Shedding of the C-type Lectin Family 14, Member A (CLEC14A) Ectodomain, Catalyzed by Rhomboid-like 2 Protein (RHBDL2). FASEB J. 30, 2311–2323. doi:10.1096/fj.201500122R
Pan, J., Cai, X., Zheng, X., Zhu, X., Feng, J., and Wang, X. (2022). Luteolin Inhibits Viability, Migration, Angiogenesis and Invasion of Non-small Cell Lung Cancer Vascular Endothelial Cells via miR-133a-3p/purine Rich Element Binding Protein B-Mediated MAPK and PI3K/Akt Signaling Pathways. Tissue Cell 75, 101740. doi:10.1016/j.tice.2022.101740
Pan, T., Zhou, D., Shi, Z., Qiu, Y., Zhou, G., Liu, J., et al. (2020). Centromere Protein U (CENPU) Enhances Angiogenesis in Triple-Negative Breast Cancer by Inhibiting Ubiquitin-Proteasomal Degradation of COX-2. Cancer Lett. 482, 102–111. doi:10.1016/j.canlet.2019.11.003
Parmar, D., and Apte, M. (2021). Angiopoietin Inhibitors: A Review on Targeting Tumor Angiogenesis. Eur. J. Pharmacol. 899, 174021. doi:10.1016/j.ejphar.2021.174021
Peng, F., Fan, H., Li, S., Peng, C., and Pan, X. (2021). MicroRNAs in Epithelial-Mesenchymal Transition Process of Cancer: Potential Targets for Chemotherapy. Ijms 22, 7526. doi:10.3390/ijms22147526
Peng, Y., Wang, Y., Tang, N., Sun, D., Lan, Y., Yu, Z., et al. (2018). Andrographolide Inhibits Breast Cancer through Suppressing COX-2 Expression and Angiogenesis via Inactivation of P300 Signaling and VEGF Pathway. J. Exp. Clin. Cancer Res. 37, 248. doi:10.1186/s13046-018-0926-9
Petrović, J., Glamočlija, J., Ilić-Tomić, T., Soković, M., Robajac, D., Nedić, O., et al. (2020). Lectin from Laetiporus Sulphureus Effectively Inhibits Angiogenesis and Tumor Development in the Zebrafish Xenograft Models of Colorectal Carcinoma and Melanoma. Int. J. Biol. Macromol. 148, 129–139. doi:10.1016/j.ijbiomac.2020.01.033
Raina, K., Rajamanickam, S., Singh, R. P., Deep, G., Chittezhath, M., and Agarwal, R. (2008). Stage-specific Inhibitory Effects and Associated Mechanisms of Silibinin on Tumor Progression and Metastasis in Transgenic Adenocarcinoma of the Mouse Prostate Model. Cancer Res. 68, 6822–6830. doi:10.1158/0008-5472.Can-08-1332
Rajabi, M., and Mousa, S. A. (2017). The Role of Angiogenesis in Cancer Treatment. Biomedicines 5. doi:10.3390/biomedicines5020034
Rashidi, M., Khalilnezhad, A., Amani, D., Jamshidi, H., Muhammadnejad, A., Bazi, A., et al. (2018). Umbelliprenin Shows Antitumor, Antiangiogenesis, Antimetastatic, Anti-inflammatory, and Immunostimulatory Activities in 4T1 Tumor-Bearing Balb/c Mice. J. Cell Physiol. 233, 8908–8918. doi:10.1002/jcp.26814
Ravichandran, K., Velmurugan, B., Gu, M., Singh, R. P., and Agarwal, R. (2010). Inhibitory Effect of Silibinin against Azoxymethane-Induced Colon Tumorigenesis in A/J Mice. Clin. Cancer Res. 16, 4595–4606. doi:10.1158/1078-0432.Ccr-10-1213
Rho, S. S., Choi, H. J., Min, J. K., Lee, H. W., Park, H., Park, H., et al. (2011). Clec14a Is Specifically Expressed in Endothelial Cells and Mediates Cell to Cell Adhesion. Biochem. Biophys. Res. Commun. 404, 103–108. doi:10.1016/j.bbrc.2010.11.075
Rini, B. I. (2007). Vascular Endothelial Growth Factor-Targeted Therapy in Renal Cell Carcinoma: Current Status and Future Directions. Clin. Cancer Res. 13, 1098–1106. doi:10.1158/1078-0432.Ccr-06-1989
Rohlenova, K., Goveia, J., García-Caballero, M., Subramanian, A., Kalucka, J., Treps, L., et al. (2020). Single-Cell RNA Sequencing Maps Endothelial Metabolic Plasticity in Pathological Angiogenesis. Cell Metab. 31, 862–e14. e814. doi:10.1016/j.cmet.2020.03.009
Saha, A., Nandi, P., Dasgupta, S., Bhuniya, A., Ganguly, N., Ghosh, T., et al. (2020). Neem Leaf Glycoprotein Restrains VEGF Production by Direct Modulation of HIF1α-Linked Upstream and Downstream Cascades. Front. Oncol. 10, 260. doi:10.3389/fonc.2020.00260
Sameri, S., Mohammadi, C., Mehrabani, M., and Najafi, R. (2021). Targeting the Hallmarks of Cancer: the Effects of Silibinin on Proliferation, Cell Death, Angiogenesis, and Migration in Colorectal Cancer. BMC Complement. Med. Ther. 21, 160. doi:10.1186/s12906-021-03330-1
Sax, M. J., Gasch, C., Athota, V. R., Freeman, R., Rasighaemi, P., Westcott, D. E., et al. (2016). Cancer Cell CCL5 Mediates Bone Marrow Independent Angiogenesis in Breast Cancer. Oncotarget 7, 85437–85449. doi:10.18632/oncotarget.13387
Schoors, S., De Bock, K., Cantelmo, A. R., Georgiadou, M., Ghesquière, B., Cauwenberghs, S., et al. (2014). Partial and Transient Reduction of Glycolysis by PFKFB3 Blockade Reduces Pathological Angiogenesis. Cell Metab. 19, 37–48. doi:10.1016/j.cmet.2013.11.008
Shen, K., Ji, L., Gong, C., Ma, Y., Yang, L., Fan, Y., et al. (2012). Notoginsenoside Ft1 Promotes Angiogenesis via HIF-1α Mediated VEGF Secretion and the Regulation of PI3K/AKT and Raf/MEK/ERK Signaling Pathways. Biochem. Pharmacol. 84, 784–792. doi:10.1016/j.bcp.2012.05.024
Shi, J., Lu, Y., and Wei, P. (2016). Xiaotan Sanjie Decoction Inhibits Angiogenesis in Gastric Cancer through Interleukin-8-Linked Regulation of the Vascular Endothelial Growth Factor Pathway. J. Ethnopharmacol. 189, 230–237. doi:10.1016/j.jep.2016.05.043
Shi, L., Pan, H., Liu, Z., Xie, J., and Han, W. (2017). Roles of PFKFB3 in Cancer. Signal Transduct. Target Ther. 2, 17044. doi:10.1038/sigtrans.2017.44
Shin, J. M., Jeong, Y. J., Cho, H. J., Park, K. K., Chung, I. K., Lee, I. K., et al. (2013). Melittin Suppresses HIF-1α/VEGF Expression through Inhibition of ERK and mTOR/p70S6K Pathway in Human Cervical Carcinoma Cells. PLoS One 8, e69380. doi:10.1371/journal.pone.0069380
Sims, J. E., Williams, D. E., Morrissey, P. J., Garka, K., Foxworthe, D., Price, V., et al. (2000). Molecular Cloning and Biological Characterization of a Novel Murine Lymphoid Growth Factor. J. Exp. Med. 192, 671–680. doi:10.1084/jem.192.5.671
Singh, R. P., Dhanalakshmi, S., Agarwal, C., and Agarwal, R. (2005). Silibinin Strongly Inhibits Growth and Survival of Human Endothelial Cells via Cell Cycle Arrest and Downregulation of Survivin, Akt and NF-kappaB: Implications for Angioprevention and Antiangiogenic Therapy. Oncogene 24, 1188–1202. doi:10.1038/sj.onc.1208276
Singh, R. P., Gu, M., and Agarwal, R. (2008). Silibinin Inhibits Colorectal Cancer Growth by Inhibiting Tumor Cell Proliferation and Angiogenesis. Cancer Res. 68, 2043–2050. doi:10.1158/0008-5472.Can-07-6247
Singh, R. P., Sharma, G., Dhanalakshmi, S., Agarwal, C., and Agarwal, R. (2003). Suppression of Advanced Human Prostate Tumor Growth in Athymic Mice by Silibinin Feeding Is Associated with Reduced Cell Proliferation, Increased Apoptosis, and Inhibition of Angiogenesis. Cancer Epidemiol. Biomarkers Prev. 12, 933–939.
Smith, B. A. H., and Bertozzi, C. R. (2021). The Clinical Impact of Glycobiology: Targeting Selectins, Siglecs and Mammalian Glycans. Nat. Rev. Drug Discov. 20, 217–243. doi:10.1038/s41573-020-00093-1
Song, Y., Fu, Y., Xie, Q., Zhu, B., Wang, J., and Zhang, B. (2020). Anti-angiogenic Agents in Combination with Immune Checkpoint Inhibitors: A Promising Strategy for Cancer Treatment. Front. Immunol. 11, 1956. doi:10.3389/fimmu.2020.01956
Sui, H., Zhao, J., Zhou, L., Wen, H., Deng, W., Li, C., et al. (2017). Tanshinone IIA Inhibits β-catenin/VEGF-mediated Angiogenesis by Targeting TGF-Β1 in Normoxic and HIF-1α in Hypoxic Microenvironments in Human Colorectal Cancer. Cancer Lett. 403, 86–97. doi:10.1016/j.canlet.2017.05.013
Sun, M., Qian, Q., Shi, L., Xu, L., Liu, Q., Zhou, L., et al. (2019). Amphiphilic Drug-Drug Conjugate for Cancer Therapy with Combination of Chemotherapeutic and Antiangiogenesis Drugs. Sci. China Chem. 63, 35–41. doi:10.1007/s11426-019-9602-4
Sun, Z., Shen, K., Xie, Y., Hu, B., He, P., Lu, Y., et al. (2021). Shiquan Yuzhen Decoction Inhibits Angiogenesis and Tumor Apoptosis Caused by Non-small Cell Lung Cancer and Promotes Immune Response. Am. J. Transl. Res. 13, 7492–7507.
Tian, Q. T., Ding, C. Y., Song, S. S., Wang, Y. Q., Zhang, A., and Miao, Z. H. (2018). New Tanshinone I Derivatives S222 and S439 Similarly Inhibit Topoisomerase I/II but Reveal Different P53-Dependency in Inducing G2/M Arrest and Apoptosis. Biochem. Pharmacol. 154, 255–264. doi:10.1016/j.bcp.2018.05.006
Tong, Q., Qing, Y., Wu, Y., Hu, X., Jiang, L., and Wu, X. (2014). Dioscin Inhibits Colon Tumor Growth and Tumor Angiogenesis through Regulating VEGFR2 and AKT/MAPK Signaling Pathways. Toxicol. Appl. Pharmacol. 281, 166–173. doi:10.1016/j.taap.2014.07.026
Tran, K., Risingsong, R., Royce, D., Williams, C. R., Sporn, M. B., and Liby, K. (2012). The Synthetic Triterpenoid CDDO-Methyl Ester Delays Estrogen Receptor-Negative Mammary Carcinogenesis in Polyoma Middle T Mice. Cancer Prev. Res. (Phila) 5, 726–734. doi:10.1158/1940-6207.Capr-11-0404
Trenti, A., Boscaro, C., Tedesco, S., Cignarella, A., Trevisi, L., and Bolego, C. (2018). Effects of Digitoxin on Cell Migration in Ovarian Cancer Inflammatory Microenvironment. Biochem. Pharmacol. 154, 414–423. doi:10.1016/j.bcp.2018.06.008
Tsai, A. C., Pan, S. L., Liao, C. H., Guh, J. H., Wang, S. W., Sun, H. L., et al. (2010). Moscatilin, a Bibenzyl Derivative from the India Orchid Dendrobrium Loddigesii, Suppresses Tumor Angiogenesis and Growth In Vitro and In Vivo. Cancer Lett. 292, 163–170. doi:10.1016/j.canlet.2009.11.020
Tsai, M. Y., Yang, R. C., Wu, H. T., Pang, J. H., and Huang, S. T. (2011). Anti-angiogenic Effect of Tanshinone IIA Involves Inhibition of Matrix Invasion and Modification of MMP-2/TIMP-2 Secretion in Vascular Endothelial Cells. Cancer Lett. 310, 198–206. doi:10.1016/j.canlet.2011.06.031
Tsui, K. H., Wu, M. Y., Lin, L. T., Wen, Z. H., Li, Y. H., Chu, P. Y., et al. (2019). Disruption of Mitochondrial Homeostasis with Artemisinin Unravels Anti-angiogenesis Effects via Auto-Paracrine Mechanisms. Theranostics 9, 6631–6645. doi:10.7150/thno.33353
Tung, Y. T., Chen, H. L., Lee, C. Y., Chou, Y. C., Lee, P. Y., Tsai, H. C., et al. (2013). Active Component of Danshen (Salvia Miltiorrhiza Bunge), Tanshinone I, Attenuates Lung Tumorigenesis via Inhibitions of VEGF, Cyclin A, and Cyclin B Expressions. Evid. Based Complement. Altern. Med. 2013, 319247. doi:10.1155/2013/319247
Varinska, L., Kubatka, P., Mojzis, J., Zulli, A., Gazdikova, K., Zubor, P., et al. (2017). Angiomodulators in Cancer Therapy: New Perspectives. Biomed. Pharmacother. 89, 578–590. doi:10.1016/j.biopha.2017.02.071
Vigneshwaran, V., Thirusangu, P., Vijay Avin, B. R., Krishna, V., Pramod, S. N., and Prabhakar, B. T. (2017). Immunomodulatory Glc/man-Directed Dolichos Lablab Lectin (DLL) Evokes Anti-tumour Response In Vivo by Counteracting Angiogenic Gene Expressions. Clin. Exp. Immunol. 189, 21–35. doi:10.1111/cei.12959
Wan, L., Zhang, Q., Wang, S., Gao, Y., Chen, X., Zhao, Y., et al. (2019). Gambogic Acid Impairs Tumor Angiogenesis by Targeting YAP/STAT3 Signaling axis. Phytother. Res. 33, 1579–1591. doi:10.1002/ptr.6350
Wang, F., Zhang, W., Guo, L., Bao, W., Jin, N., Liu, R., et al. (2014). Gambogic Acid Suppresses Hypoxia-Induced Hypoxia-Inducible Factor-1α/vascular Endothelial Growth Factor Expression via Inhibiting Phosphatidylinositol 3-kinase/Akt/mammalian Target Protein of Rapamycin Pathway in Multiple Myeloma Cells. Cancer Sci. 105, 1063–1070. doi:10.1111/cas.12458
Wang, H., Zhang, C., Chi, H., and Meng, Z. (2018). Synergistic Anti-hepatoma Effect of Bufalin Combined with Sorafenib via Mediating the Tumor Vascular Microenvironment by Targeting mTOR/VEGF Signaling. Int. J. Oncol. 52, 2051–2060. doi:10.3892/ijo.2018.4351
Wang, H., Zhang, C., Ning, Z., Xu, L., Zhu, X., and Meng, Z. (2016). Bufalin Enhances Anti-angiogenic Effect of Sorafenib via AKT/VEGF Signaling. Int. J. Oncol. 48, 1229–1241. doi:10.3892/ijo.2016.3326
Wang, L., Peng, F., Peng, C., and Du, J. R. (2021). Gut Microbiota in Tumor Microenvironment: A Critical Regulator in Cancer Initiation and Development as Potential Targets for Chinese Medicine. Am. J. Chin. Med. 49, 609–626. doi:10.1142/s0192415x21500270
Wang, S. W., Liu, S. C., Sun, H. L., Huang, T. Y., Chan, C. H., Yang, C. Y., et al. (2015). CCL5/CCR5 axis Induces Vascular Endothelial Growth Factor-Mediated Tumor Angiogenesis in Human Osteosarcoma Microenvironment. Carcinogenesis 36, 104–114. doi:10.1093/carcin/bgu218
Wang, T. Y., and Chen, J. X. (2019). Effects of Curcumin on Vessel Formation Insight into the Pro- and Antiangiogenesis of Curcumin. Evid. Based Complement. Altern. Med. 2019, 1390795. doi:10.1155/2019/1390795
Wang, W., Liu, Y., You, L., Sun, M., Qu, C., Dong, X., et al. (2020). Inhibitory Effects of Paris Saponin I, II, Ⅵ and Ⅶ on HUVEC Cells through Regulation of VEGFR2, PI3K/AKT/mTOR, Src/eNOS, PLCγ/ERK/MERK, and JAK2-STAT3 Pathways. Biomed. Pharmacother. 131, 110750. doi:10.1016/j.biopha.2020.110750
Wang, Y., Li, J. X., Wang, Y. Q., and Miao, Z. H. (2015). Tanshinone I Inhibits Tumor Angiogenesis by Reducing Stat3 Phosphorylation at Tyr705 and Hypoxia-Induced HIF-1α Accumulation in Both Endothelial and Tumor Cells. Oncotarget 6, 16031–16042. doi:10.18632/oncotarget.3648
Wang, Y., Zhang, Q., Chen, Y., Liang, C. L., Liu, H., Qiu, F., et al. (2020). Antitumor Effects of Immunity-Enhancing Traditional Chinese Medicine. Biomed. Pharmacother. 121, 109570. doi:10.1016/j.biopha.2019.109570
Wei, T., and Liu, J. (2017). Anti-angiogenic Properties of Artemisinin Derivatives (Review). Int. J. Mol. Med. 40, 972–978. doi:10.3892/ijmm.2017.3085
Whayne, T. F. (2018). Clinical Use of Digitalis: A State of the Art Review. Am. J. Cardiovasc Drugs 18, 427–440. doi:10.1007/s40256-018-0292-1
Wu, M., Li, J., Gao, Q., and Ye, F. (2016). The Role of Sema4D/CD100 as a Therapeutic Target for Tumor Microenvironments and for Autoimmune, Neuroimmune and Bone Diseases. Expert Opin. Ther. Targets 20, 885–901. doi:10.1517/14728222.2016.1139083
Wu, Y., Xu, J., Liu, Y., Zeng, Y., and Wu, G. (2020). A Review on Anti-tumor Mechanisms of Coumarins. Front. Oncol. 10, 592853. doi:10.3389/fonc.2020.592853
Xiang, Y., Guo, Z., Zhu, P., Chen, J., and Huang, Y. (2019). Traditional Chinese Medicine as a Cancer Treatment: Modern Perspectives of Ancient but Advanced Science. Cancer Med. 8, 1958–1975. doi:10.1002/cam4.2108
Xie, J., Liu, J., Liu, H., Liang, S., Lin, M., Gu, Y., et al. (2015). The Antitumor Effect of Tanshinone IIA on Anti-proliferation and Decreasing VEGF/VEGFR2 Expression on the Human Non-small Cell Lung Cancer A549 Cell Line. Acta Pharm. Sin. B 5, 554–563. doi:10.1016/j.apsb.2015.07.008
Xing, Y., Tu, J., Zheng, L., Guo, L., and Xi, T. (2015). Anti-angiogenic Effect of Tanshinone IIA Involves Inhibition of the VEGF/VEGFR2 Pathway in Vascular Endothelial Cells. Oncol. Rep. 33, 163–170. doi:10.3892/or.2014.3592
Xu, C., Yang, S., Jiang, Z., Zhou, J., and Yao, J. (2020). Self-Propelled Gemini-like LMWH-Scaffold Nanodrugs for Overall Tumor Microenvironment Manipulation via Macrophage Reprogramming and Vessel Normalization. Nano Lett. 20, 372–383. doi:10.1021/acs.nanolett.9b04024
Xu, X., Wu, L., Zhou, X., Zhou, N., Zhuang, Q., Yang, J., et al. (2017). Cryptotanshinone Inhibits VEGF-Induced Angiogenesis by Targeting the VEGFR2 Signaling Pathway. Microvasc. Res. 111, 25–31. doi:10.1016/j.mvr.2016.12.011
Xu, X., Wu, Y., Hu, M., Li, X., Bao, Q., Bian, J., et al. (2016). Novel Natural Product-like Caged Xanthones Bearing a Carbamate Moiety Exhibit Antitumor Potency and Anti-angiogenesis Activity In Vivo. Sci. Rep. 6, 35771. doi:10.1038/srep35771
Xu, X., Wu, Y., Hu, M., Li, X., Gu, C., You, Q., et al. (2016). Structure-activity Relationship of Garcinia Xanthones Analogues: Potent Hsp90 Inhibitors with Cytotoxicity and Antiangiogenesis Activity. Bioorg Med. Chem. 24, 4626–4635. doi:10.1016/j.bmc.2016.07.067
Yan, B., Liu, L., Zhao, Y., Xiu, L. J., Sun, D. Z., Liu, X., et al. (2014). Xiaotan Sanjie Decoction Attenuates Tumor Angiogenesis by Manipulating Notch-1-Regulated Proliferation of Gastric Cancer Stem-like Cells. World J. Gastroenterol. 20, 13105–13118. doi:10.3748/wjg.v20.i36.13105
Yang, L.-q., Yu, S.-p., Yang, Y.-t., Zhao, Y.-s., Wang, F.-y., Chen, Y., et al. (2021). Muscone Derivative ZM-32 Inhibits Breast Tumor Angiogenesis by Suppressing HuR-Mediated VEGF and MMP9 Expression. Biomed. Pharmacother. 136, 111265. doi:10.1016/j.biopha.2021.111265
Yang, M., Zou, J., Zhu, H., Liu, S., Wang, H., Bai, P., et al. (2015). Paris Saponin II Inhibits Human Ovarian Cancer Cell-Induced Angiogenesis by Modulating NF-Κb Signaling. Oncol. Rep. 33, 2190–2198. doi:10.3892/or.2015.3836
Yang, M. H., Chang, K. J., Li, B., and Chen, W. S. (2019). Arsenic Trioxide Suppresses Tumor Growth through Antiangiogenesis via Notch Signaling Blockade in Small-Cell Lung Cancer. Biomed. Res. Int. 2019, 4647252. doi:10.1155/2019/4647252
Yang, S. H., Lin, J. K., Chen, W. S., and Chiu, J. H. (2003). Anti-angiogenic Effect of Silymarin on Colon Cancer LoVo Cell Line. J. Surg. Res. 113, 133–138. doi:10.1016/s0022-4804(03)00229-4
Yang, T., Xiao, H., Liu, X., Wang, Z., Zhang, Q., Wei, N., et al. (2021). Vascular Normalization: A New Window Opened for Cancer Therapies. Front. Oncol. 11, 719836. doi:10.3389/fonc.2021.719836
Yang, X., and Wu, X. Z. (2015). Main Anti-tumor Angiogenesis Agents Isolated from Chinese Herbal Medicines. Mini Rev. Med. Chem. 15, 1011–1023. doi:10.2174/138955751512150731113242
Yang, Y., Tang, J., Zhang, M., Gu, Z., Song, H., Yang, Y., et al. (2019). Responsively Aggregatable Sub-6 Nm Nanochelators Induce Simultaneous Antiangiogenesis and Vascular Obstruction for Enhanced Tumor Vasculature Targeted Therapy. Nano Lett. 19, 7750–7759. doi:10.1021/acs.nanolett.9b02691
Yin, L., Fan, Z., Liu, P., Chen, L., Guan, Z., Liu, Y., et al. (2021). Anemoside A3 Activates TLR4-dependent M1-Phenotype Macrophage Polarization to Represses Breast Tumor Growth and Angiogenesis. Toxicol. Appl. Pharmacol. 432, 115755. doi:10.1016/j.taap.2021.115755
Yoo, H. G., Jung, S. N., Hwang, Y. S., Park, J. S., Kim, M. H., Jeong, M., et al. (2004). Involvement of NF-kappaB and Caspases in Silibinin-Induced Apoptosis of Endothelial Cells. Int. J. Mol. Med. 13, 81–86. doi:10.3892/ijmm.13.1.81
Yu, X., Li, J., Ye, L., Zhao, J., Xie, M., Zhou, J., et al. (2021). Real-world Outcomes of Chemo-Antiangiogenesis versus Chemo-Immunotherapy Combinations in EGFR-Mutant Advanced Non-small Cell Lung Cancer Patients after Failure of EGFR-TKI Therapy. Transl. Lung Cancer Res. 10, 3782–3792. doi:10.21037/tlcr-21-681
Yuan, Q., Yao, F., Zhou, L., Liang, G., Song, X., Jiang, G., et al. (2019). Yu Ping Feng San Exert Anti-angiogenesis Effects through the Inhibition of TSLP-STAT3 Signaling Pathways in Hepatocellular Carcinoma. Evidence-Based Complementary Altern. Med. 2019, 1–16. doi:10.1155/2019/1947156
Zang, M., Hu, L., Zhang, B., Zhu, Z., Li, J., Zhu, Z., et al. (2017). Luteolin Suppresses Angiogenesis and Vasculogenic Mimicry Formation through Inhibiting Notch1-VEGF Signaling in Gastric Cancer. Biochem. Biophys. Res. Commun. 490, 913–919. doi:10.1016/j.bbrc.2017.06.140
Zeng, Z., Nian, Q., Chen, N., Zhao, M., Zheng, Q., Zhang, G., et al. (2022). Ginsenoside Rg3 Inhibits Angiogenesis in Gastric Precancerous Lesions through Downregulation of Glut1 and Glut4. Biomed. Pharmacother. 145, 112086. doi:10.1016/j.biopha.2021.112086
Zhang, C., Li, Q., Qin, G., Zhang, Y., Li, C., Han, L., et al. (2021). Anti-angiogenesis and Anti-metastasis Effects of Polyphyllin VII on Hepatocellular Carcinoma Cells In Vitro and In Vivo. Chin. Med. 16, 41. doi:10.1186/s13020-021-00447-w
Zhang, C., Wang, N., Tan, H. Y., Guo, W., Li, S., and Feng, Y. (2018). Targeting VEGF/VEGFRs Pathway in the Antiangiogenic Treatment of Human Cancers by Traditional Chinese Medicine. Integr. Cancer Ther. 17, 582–601. doi:10.1177/1534735418775828
Zhang, L., Chen, C., Duanmu, J., Wu, Y., Tao, J., Yang, A., et al. (2018). Cryptotanshinone Inhibits the Growth and Invasion of Colon Cancer by Suppressing Inflammation and Tumor Angiogenesis through Modulating MMP/TIMP System, PI3K/Akt/mTOR Signaling and HIF-1α Nuclear Translocation. Int. Immunopharmacol. 65, 429–437. doi:10.1016/j.intimp.2018.10.035
Zhang, N., Yin, R., Zhou, P., Liu, X., Fan, P., Qian, L., et al. (2021). DLL1 Orchestrates CD8 + T Cells to Induce Long-Term Vascular Normalization and Tumor Regression. Proc. Natl. Acad. Sci. U.S.A. 118, 118. doi:10.1073/pnas.2020057118
Zhang, X., Qiu, H., Li, C., Cai, P., and Qi, F. (2021). The Positive Role of Traditional Chinese Medicine as an Adjunctive Therapy for Cancer. Biosci. Trends 15, 283–298. doi:10.5582/bst.2021.01318
Zhang, Z. R., Li, J. H., Li, S., Liu, A. L., Hoi, P. M., Tian, H. Y., et al. (2014). In Vivo angiogenesis Screening and Mechanism of Action of Novel Tanshinone Derivatives Produced by One-Pot Combinatorial Modification of Natural Tanshinone Mixture from Salvia Miltiorrhiza. PLoS One 9, e100416. doi:10.1371/journal.pone.0100416
Zhao, K., Yuan, Y., Lin, B., Miao, Z., Li, Z., Guo, Q., et al. (2018). LW-215, a Newly Synthesized Flavonoid, Exhibits Potent Anti-angiogenic Activity In Vitro and In Vivo. Gene 642, 533–541. doi:10.1016/j.gene.2017.11.065
Zhao, M., Gao, F. H., Wang, J. Y., Liu, F., Yuan, H. H., Zhang, W. Y., et al. (2011). JAK2/STAT3 Signaling Pathway Activation Mediates Tumor Angiogenesis by Upregulation of VEGF and bFGF in Non-small-cell Lung Cancer. Lung Cancer 73, 366–374. doi:10.1016/j.lungcan.2011.01.002
Zhao, Y., Huo, M., Xu, Z., Wang, Y., and Huang, L. (2015). Nanoparticle Delivery of CDDO-Me Remodels the Tumor Microenvironment and Enhances Vaccine Therapy for Melanoma. Biomaterials 68, 54–66. doi:10.1016/j.biomaterials.2015.07.053
Zhong, C., Lin, Z., Ke, L., Shi, P., Li, S., Huang, L., et al. (2021). Recent Research Progress (2015-2021) and Perspectives on the Pharmacological Effects and Mechanisms of Tanshinone IIA. Front. Pharmacol. 12, 778847. doi:10.3389/fphar.2021.778847
Zhong, J., Lu, W., Zhang, J., Huang, M., Lyu, W., Ye, G., et al. (2020). Notoginsenoside R1 Activates the Ang2/Tie2 Pathway to Promote Angiogenesis. Phytomedicine 78, 153302. doi:10.1016/j.phymed.2020.153302
Zhou, J., Jiang, Y. Y., Wang, X. X., Wang, H. P., Chen, H., Wu, Y. C., et al. (2020). Tanshinone IIA Suppresses Ovarian Cancer Growth through Inhibiting Malignant Properties and Angiogenesis. Ann. Transl. Med. 8, 1295. doi:10.21037/atm-20-5741
Zhou, L., Sui, H., Wang, T., Jia, R., Zhang, Z., Fu, J., et al. (2020). Tanshinone IIA Reduces Secretion of Pro-angiogenic F-actors and I-nhibits A-ngiogenesis in H-uman C-olorectal C-ancer. Oncol. Rep. 43, 1159–1168. doi:10.3892/or.2020.7498
Zhou, L. H., Hu, Q., Sui, H., Ci, S. J., Wang, Y., Liu, X., et al. (2012). Tanshinone II-A Inhibits Angiogenesis through Down Regulation of COX-2 in Human Colorectal Cancer. Asian Pac. J. Cancer Prev. 13, 4453–4458. doi:10.7314/apjcp.2012.13.9.4453
Zhou, L. H., Hu, Q., Sui, H., Ci, S. J., Wang, Y., Liu, X., et al. (2012). Tanshinone II-Aa Inhibits Angiogenesis through Down Regulation of COX-2 in Human Colorectal Cancer. Asian Pac J. Cancer Prev. 13, 4453–4458. doi:10.7314/apjcp.2012.13.9.4453
Zhou, L., Li, Z. K., Li, C. Y., Liang, Y. Q., and Yang, F. (2022). Anticancer Properties and Pharmaceutical Applications of Ginsenoside Compound K: A Review. Chem. Biol. Drug Des. 99, 286–300. doi:10.1111/cbdd.13983
Zhou, Z. Y., Zhao, W. R., Xiao, Y., Zhou, X. M., Huang, C., Shi, W. T., et al. (2020). Antiangiogenesis Effect of Timosaponin AIII on HUVECs In Vitro and Zebrafish Embryos In Vivo. Acta Pharmacol. Sin. 41, 260–269. doi:10.1038/s41401-019-0291-z
Zhou, Z., Yao, H., and Hu, H. (2017). “Disrupting Tumor Angiogenesis and “The Hunger Games” for Breast Cancer,” in Translational Research in Breast Cancer: Biomarker Diagnosis, Targeted Therapies and Approaches to Precision Medicine. Editors E. Song, and H. Hu (Singapore: Springer), 171–195. doi:10.1007/978-981-10-6020-5_8
Zhu, D., Li, Y., Zhang, Z., Xue, Z., Hua, Z., Luo, X., et al. (2021). Recent Advances of Nanotechnology-Based Tumor Vessel-Targeting Strategies. J. Nanobiotechnol 19, 435. doi:10.1186/s12951-021-01190-y
Zhu, M., Chen, D., Li, D., Ding, H., Zhang, T., Xu, T., et al. (2013). Luteolin Inhibits Angiotensin II-Induced Human Umbilical Vein Endothelial Cell Proliferation and Migration through Downregulation of Src and Akt Phosphorylation. Circ. J. 77, 772–779. doi:10.1253/circj.cj-12-0310
Zhu, T., Cheng, Z., Peng, X., Xing, D., and Zhang, M. (2021). HIF-1α RNAi Combined with Asparagus Polysaccharide Exerts an Antiangiogenesis Effect on Hepatocellular Carcinoma In Vitro and In Vivo. Evid. Based Complement. Altern. Med. 2021, 9987383. doi:10.1155/2021/9987383
Keywords: tumor angiogenesis, targets, delivery system, immunosuppressive microenvironment, molecular intervention
Citation: Zhou J, Wang L, Peng C and Peng F (2022) Co-Targeting Tumor Angiogenesis and Immunosuppressive Tumor Microenvironment: A Perspective in Ethnopharmacology. Front. Pharmacol. 13:886198. doi: 10.3389/fphar.2022.886198
Received: 28 February 2022; Accepted: 04 May 2022;
Published: 15 June 2022.
Edited by:
Zhiyu Wang, Guangzhou University of Chinese Medicine, ChinaReviewed by:
Vijay Avin Balaji Ragunathrao, University of Illinois at Chicago, United StatesVaishali Aggarwal, University of Pittsburgh, United States
Copyright © 2022 Zhou, Wang, Peng and Peng. This is an open-access article distributed under the terms of the Creative Commons Attribution License (CC BY). The use, distribution or reproduction in other forums is permitted, provided the original author(s) and the copyright owner(s) are credited and that the original publication in this journal is cited, in accordance with accepted academic practice. No use, distribution or reproduction is permitted which does not comply with these terms.
*Correspondence: Fu Peng, ZnVqaW5nMTI2QHllYWgubmV0; Cheng Peng, cGVuZ2NoZW5nY2hlbmdkdUAxMjYuY29t