- 1Department of Pediatrics, Case Western Reserve University, Cleveland, OH, United States
- 2Department of Drug Discovery, Galleon Pharmaceuticals, Inc., Horsham, PA, United States
- 3Pediatric Respiratory Medicine, University of Virginia School of Medicine, Charlottesville, VA, United States
- 4Herman B Wells Center for Pediatric Research, Indiana University School of Medicine, Indianapolis, IN, United States
- 5Department of Physiology, Medical College of Wisconsin, Milwaukee, WI, United States
- 6Department of Anesthesia, University of Iowa Hospitals and Clinics, Iowa City, IA, United States
- 7Basic Sciences, Division of Physiology, School of Medicine, Loma Linda University, Loma Linda, CA, United States
- 8Division of Pulmonary, Critical Care and Sleep Medicine, University Hospitals Case Medical Center, Case Western Reserve University, Cleveland, OH, United States
- 9Department of Pharmacology, Case Western Reserve University, Cleveland, OH, United States
Cell-penetrant thiol esters including the disulfides, D-cystine diethyl ester and D-cystine dimethyl ester, and the monosulfide, L-glutathione ethyl ester, prevent and/or reverse the deleterious effects of opioids, such as morphine and fentanyl, on breathing and gas exchange within the lungs of unanesthetized/unrestrained rats without diminishing the antinociceptive or sedative effects of opioids. We describe here the effects of the monosulfide thiol ester, D-cysteine ethyl ester (D-CYSee), on intravenous morphine-induced changes in ventilatory parameters, arterial blood–gas chemistry, alveolar–arterial (A-a) gradient (i.e., index of gas exchange in the lungs), and sedation and antinociception in freely-moving rats. The bolus injection of morphine (10 mg/kg, IV) elicited deleterious effects on breathing, including depression of tidal volume, minute ventilation, peak inspiratory flow, and inspiratory drive. Subsequent injections of D-CYSee (2 × 500 μmol/kg, IV, given 15 min apart) elicited an immediate and sustained reversal of these effects of morphine. Morphine (10 mg/kg, IV) also A-a gradient, which caused a mismatch in ventilation perfusion within the lungs, and elicited pronounced changes in arterial blood–gas chemistry, including pronounced decreases in arterial blood pH, pO2 and sO2, and equally pronounced increases in pCO2 (all responses indicative of decreased ventilatory drive). These deleterious effects of morphine were immediately reversed by the injection of a single dose of D-CYSee (500 μmol/kg, IV). Importantly, the sedation and antinociception elicited by morphine (10 mg/kg, IV) were minimally affected by D-CYSee (500 μmol/kg, IV). In contrast, none of the effects of morphine were affected by administration of the parent thiol, D-cysteine (1 or 2 doses of 500 μmol/kg, IV). Taken together, these data suggest that D-CYSee may exert its beneficial effects via entry into cells that mediate the deleterious effects of opioids on breathing and gas exchange. Whether D-CYSee acts as a respiratory stimulant or counteracts the inhibitory actions of µ-opioid receptor activation remains to be determined. In conclusion, D-CYSee and related thiol esters may have clinical potential for the reversal of the adverse effects of opioids on breathing and gas exchange, while largely sparing antinociception and sedation.
Introduction
Opioids, such as morphine and fentanyl, are administered to alleviate pain. However, their clinical utilization is complicated by their propensity to adversely affect breathing and ventilatory mechanics (van Dorp et al., 2007; Dahan et al., 2010; Boom et al., 2012; Dahan et al., 2018; Algera et al., 2019). Opioid receptor (OR) antagonists, such as naloxone, cannot only prevent and reverse opioid-induced respiratory depression (OIRD), but also reverse opioid-induced analgesia and sedation. Therefore, their clinical value is limited in many situations, including during surgery and in post-surgical situations where pain relief and sedation are essential to patients (Dahan et al., 2010; Dahan et al., 2018). Accordingly, there is an urgent unmet need to develop drugs that reverse OIRD by mechanisms other than antagonism of ORs. In a recent comprehensive review, Dahan et al. (2018) concluded that no currently available experimental drugs are adequate for the therapeutic reversal of OIRD for a host of reasons, including that they only minimally improve arterial oxygenation, and they all need to be further evaluated for their true efficacy and for potential adverse (i.e., toxicological) effects.
Our recent studies have detailed the ability of reduced (monothiol) and oxidized (disulfide) thiol esters to prevent/reverse the adverse effects of opioids, such as morphine and fentanyl, on ventilatory parameters and arterial blood–gas (ABG) chemistry in rats without compromising the analgesic or sedative actions of the opioids (Mendoza et al., 2013; Gaston et al., 2021; Jenkins et al., 2021). These studies were based on evidence that morphine inhibits L-cysteine uptake into neurons via blockade of excitatory amino acid transporter type 3 (Trivedi et al., 2014; Trivedi and Deth, 2015), and substantial evidence that systemically administered ethyl ester and methyl ester derivatives of reduced and oxidized thiols readily enter cells including cells in the periphery, and neurons in the central nervous system (Servin et al., 1988; Foreman and Benson, 1990; Lailey et al., 1991; Ben-Nun et al., 1992; Hobbs et al., 1993; Lailey and Upshall, 1994; Chu et al., 2004; Rech et al., 2008; Figueiredo et al., 2009; Ito et al., 2012; Mendoza et al., 2013; Sumayao et al., 2013; Gurbuz et al., 2015; Henderson et al., 2016).
We began our investigations with L-cysteine ethyl ester (L-CYSee), and reported that the bolus intravenous injection of L-CYSee produced a prompt and sustained reversal of the adverse effects of morphine on ABG chemistry and alveolar–arterial (A-a) gradient (i.e., an index of alveolar gas exchange) in isoflurane-anesthetized tracheotomized rats, but not in rats without the tracheal tube (Mendoza et al., 2013). The finding that L-cysteine was inactive suggested that the actions of L-CYSee were due to entry into neurons/cells, and the initiation of intracellular signaling events that may involve redox-dependent processes. With respect to therapeutic potential, it was evident that the ability of L-CYSee to overcome the adverse actions of morphine on breathing and A-a gradient is overridden by adverse effects on upper airways, which may include the collapse of the larynx and vocal folds, and/or the loss of muscle activity in the tongue with the flaccid tongue blocking the airway (Mendoza et al., 2013). We are currently examining whether ethyl ester and methyl ester derivatives of D-cysteine and D-cystine can reverse OIRD without the adverse effects of the L-thiol esters with the expectation that (1) D-thiol esters rapidly enter cells/neurons as efficiently as L-thiol esters; (2) the adverse effects of the L-thiol esters involve them entering into metabolic pathways, while D-thiol esters do not; and (3) the specific (as yet undefined) intracellular processes by which thiol esters reverse OIRD are not dependent upon stereospecific processes. Our first report with a D-isomer demonstrated that the intravenous administration of the disulfide esters, D-cystine dimethyl ester or D-cystine diethyl ester, reversed the adverse effects of morphine on ventilatory parameters and ABG chemistry in freely-moving rats without adversely affecting the antinociceptive actions of the opioid (Gaston et al., 2021).
This study extends our understanding of the pharmacological profiles of D-thiol esters by presenting the effects of D-cysteine ethyl ester (D-CYSee) and the parent thiol, D-cysteine, on the pharmacological actions of morphine in unanesthetized/unrestrained adult male Sprague Dawley rats. We report here that the intravenous injection of D-CYSee elicits a rapid and sustained reversal of the adverse effects of morphine on ventilatory parameters, A-a gradient, and ABG chemistry with minimal effects on the analgesic or sedative actions of the opioid, whereas D-cysteine was inactive. This profile of activity of D-CYSee would be advantageous in clinical settings in which opioids are essential for pain relief, yet their adverse effects on ventilation need to be overcome.
Materials and Methods
Permissions, Rats, and Surgical Procedures
All studies were carried out in accordance with the NIH Guide for the Care and Use of Laboratory Animals (NIH Publication No. 80-23) revised in 1996, and in strict compliance with the ARRIVE (Animal Research: Reporting of In Vivo Experiments) guidelines (http://www.nc3rs.org.uk/page.asp?id=1357). All experimental protocols were approved by the Animal Care and Use Committees of Case Western Reserve University, the University of Virginia, and Loma Linda University.
Adult male Sprague Dawley rats were purchased from Harlan Industries (Madison, WI, United States). After four to five days of recovery from transportation, the rats were implanted with femoral artery catheters and/or jugular vein catheters under 2% isoflurane anesthesia as detailed previously (Henderson et al., 2014; Gaston et al., 2021). The rats were given at least 4 days to recover from surgery before use. All femoral arterial catheters were flushed daily with heparin solution (50 units of heparin in 0.1 M, pH 7.4, phosphate-buffered saline). On the day of experimentation, all arterial and venous catheters were flushed with phosphate-buffered saline (0.1 M, pH 7.4) approximately 4 hours before the commencement of experiments. Note that the pH values of all stock solutions of the test drugs (i.e., vehicle, D-cysteine and D-CYSee) were adjusted to approximately 7.2 with 0.25M NaOH. All studies were performed in a quiet laboratory with a relative humidity of 50 ± 2% and room temperature of 21.3 ± 0.2°C. The antinociception and ventilatory/ABG chemistry experiments were performed in separate groups of rats so as to not compromise the respiratory measurements. The plethysmography, antinociception recording sessions, and the arterial blood sampling studies (ABG assays) were conducted by the same investigator who injected the opioid, vehicle, or test drugs (i.e., D-cysteine and D-CYSee). The syringes containing the vehicle or test drugs were made up by another investigator, such that the person running the actual experiment was blinded to the treatment protocol. In every case, the data files resulting from each study were collated and then analyzed by yet another investigator in the group.
Protocols for Whole Body Plethysmography Measurement of Ventilatory Parameters
Ventilatory parameters were recorded continuously in the unrestrained freely-moving rats using a whole body plethysmography system (PLY3223; Data Sciences International, St. Paul, MN) as detailed previously (May et al., 2013a; May et al., 2013b; Young et al., 2013; Getsy et al., 2014, 2020; Henderson et al., 2014; Baby et al., 2018, 2021a,b; Gaston et al., 2020, 2021; Seckler et al., 2021). The directly recorded and calculated (derived) parameters are defined in Supplementary Table S1 (Hamelmann et al., 1997; Lomask, 2006; Tsumuro et al., 2006; Quindry et al., 2016; Gaston et al., 2021). Supplementary Figure S1 shows a diagram of relationships between some of the directly recorded parameters (adapted from Lomask, 2006). On the day of the study, each rat was placed in an individual plethysmography chamber and allowed at least 60 min to acclimatize so that the resting (baseline (pre)) ventilatory parameter values are accurately defined.
Study 1: Two groups of rats received a bolus injection of morphine (10 mg/kg, IV), and after 15 min, one group (n = 5, 77.4 ± 0.4 days of age, and 331 ± 2 g body weight) received a bolus injection of vehicle (saline) and the other group (n = 6, 77.6 ± 0.4 days of age, and 333 ± 2 g body weight) received a bolus injection of D-CYSee (500 μmol/kg, IV). The rats then received a second injection of either vehicle or D-CYSee 15 min later depending on their group. Study 2: Two groups of rats received a bolus injection of morphine (10 mg/kg, IV), and after 15 min, one group (n = 5; 77.9 ± 0.3 days of age; and 329 ± 2 g body weight) received a bolus injection of vehicle (saline) and the other group (n = 6; 77.8 ± 0.4 days of age; and 330 ± 2 g body weight) received a bolus injection of D-cysteine (500 μmol/kg, IV). The rats then received a second injection of either vehicle or D-cysteine 15 min later depending on their group. Ventilatory parameters were monitored for 60 min after the second set of vehicle/D-CYSee/D-cysteine injections in all groups of rats. We gave two injections of D-CYSee to see if the first injection produces an effect, and if not, whether it is needed to prime the effect of the second injection. Study 3: One group of rats (n = 6; 81.6 ± 0.6 days of age; and 345 ± 3 g body weight) received a bolus injection of morphine (10 mg/kg, IV), and after 15 min, they received a bolus injection of D-CYSee (500 μmol/kg, IV), and after another 15 min, they received a bolus injection of vehicle rather than D-CYSee. These rats are referred to as the D-CYSee (1x) group in Figures 5, 6. Whereas another group of rats received two bolus injections of D-CYSee 15 min apart, and they are referred to as the D-CYSee (2x) group. Note that the resting ventilatory parameters of the D-CYSee (1x) rats were similar to those of the D-CYSee (2x) rats (p < 0.05, for all comparisons via an unpaired t-test, data not shown).
The body weights of all the groups of rats were similar to one another, thus corrections of ventilatory parameters (e.g., TV, PIF, and PEF) are shown without correcting for body weight. The FinePointe (DSI) software constantly corrected digitized ventilatory values originating from the actual waveforms for alterations in chamber humidity and temperature. Pressure changes associated with the respiratory waveforms were then converted to volumes (e.g., TV, PIF, PEF, and EF50) by employing the algorithms of Epstein et al. (Epstein and Epstein, 1978; Epstein et al., 1980). Specifically, factoring in chamber temperature and humidity, the cycle analyzers filtered the acquired signals, and FinePointe algorithms generated an array of box flow data that identified a waveform segment as an acceptable breath. From this array, the minimum and maximum box flow values were determined and multiplied by a compensation factor provided by the selected algorithm (Epstein and Epstein, 1978; Epstein et al., 1980), thus producing TV, PIF, and PEF values that were used to determine non-eupneic breathing events expressed as the non-eupneic breathing index (NEBI), reported as the percentage of non-eupneic breathing events per epoch (Getsy et al., 2014). The apneic pause was determined by the formula, (Expiratory Time/Relaxation Time) − 1 (Gaston et al., 2021).
Protocols for Blood–Gas Measurements and Determination of Arterial–Alveolar Gradient
Alterations in key ABG chemistry parameters—pH, pCO2, pO2 and sO2—elicited by the bolus injection of morphine (10 mg/kg, IV) in three different sets of unanesthetized freely-moving rats (n = 9 rats per group) followed 15 min later by a bolus injection of vehicle (saline, IV; 81.3 ± 0.8 days of age; and 343 ± 2 g body weight), D-cysteine (500 μmol/kg, IV; 82.5 ± 0.5 days of age; and 347 ± 3 g body weight), or D-CYSee (500 μmol/kg, IV; 81.4 ± 0.4 days of age; 341 ± 2 g body weight) were determined as detailed previously (Henderson et al., 2014; Baby et al., 2018, Baby et al., 2021 S.; Gaston et al., 2021; Jenkins et al., 2021). Samples of arterial blood (100 μl) were taken 15 min before and 15 min after the injection of morphine (10 mg/kg, IV). The rats then immediately received an injection of vehicle, D-cysteine or D-CYSee, and blood samples were taken at 5-, 15-, 30-, and 45-min time points. The pH, pCO2, pO2 and sO2 were determined with the aid of a radiometer blood–gas analyzer (ABL800 FLEX). The A-a gradient measures the difference between alveolar and arterial blood O2 concentrations (Stein et al., 1995; Story 1996; Henderson et al., 2014). A reduction in PaO2 without a concomitant alteration in A-a gradient is the result of hypoventilation, whereas a reduction in PaO2 with a concomitant elevation in A-a gradient is an indicator of an ongoing mismatch in ventilation–perfusion in the lungs (Stein et al., 1995; Story 1996; Henderson et al., 2014; Gaston et al., 2021). A-a gradient = PAO2 − PaO2, where PAO2 is the partial pressure (p) of alveolar O2, and PaO2 is pO2 in the sampled arterial blood. PAO2 = [(FiO2 x (Patm − PH2O) − (PaCO2/respiratory quotient)], where FiO2 is the fraction of O2 in inspired air, Patm is the atmospheric pressure, PH2O is the partial pressure of H2O in inspired air, PaCO2 is pCO2 in arterial blood, and respiratory quotient (RQ) is the ratio of CO2 eliminated to O2 consumed. We took FiO2 of room air to be 21% = 0.21, Patm to be 760 mmHg, and PH2O to be 47 mmHg (Gaston et al., 2021). We took the RQ value of our adult male rats to be 0.9 (Stengel et al., 2010; Chapman et al., 2012; Gaston et al., 2021; Jenkins et al., 2021).
Antinociception Assessment by Tail-Flick Latency Assay
The antinociceptive effects elicited by an injection of morphine and a subsequent injection of vehicle or D-CYSee were determined by evaluating tail-flick latency (TFL) with the use of a Tail-Flick Analgesia Meter (IITC Life Science Inc., USA) as described previously (Lewis et al., 1991; Meller et al., 1991; Carstens and Wilson, 1993; Henderson et al., 2014; Golder et al., 2015; Gaston et al., 2021; Jenkins et al., 2021). This procedure entailed a minor level of manual restraint during the positioning of the tail to apply a thermal stimulus sufficient to induce a latency of tail withdrawal of about 3.0 s in all rats. Baseline TFL was tested in all rats prior to any drug administration (−20-min time point). One group of rats (n = 6; 78.3 ± 1.4 days of age; and 332 ± 3 g body weight) received an injection of morphine at 5 mg/kg (IV), and after TFL testing at + 20 min, they immediately received a bolus IV injection of vehicle (saline, 100 μl/100 g body weight). A second group of rats (n = 6; 79.5 ± 1.5 days of age; and 335 ± 3 g body weight) received an injection of morphine (5 mg/kg, IV), and after TFL testing at + 20 min, they immediately received a bolus injection of D-CYSee (500 μmol/kg, IV). A third group (n = 6; 77.3 ± 2.2 days of age; and 336 ± 4 g body weight) received an injection of morphine at 10 mg/kg (IV), and after TFL testing at + 20 min, they immediately received a bolus IV injection of vehicle (saline, 100 μl/100 g body weight). A fourth group (n = 6; 78.0 ± 1.8 days of age; and 334 ± 2 g body weight) received an injection of morphine (10 mg/kg, IV), and after TFL testing at + 20 min, they immediately received a bolus injection of D-CYSee (500 μmol/kg, IV). TFL was tested at +20, 40, 60, 90, 120, 180 min time points. The resulting data are presented as actual TFL (sec) and as maximum possible effect (%MPE) determined by the formula, %MPE = [(post-injection TFL − baseline TFL)/(12 − baseline TFL)] x 100 (Lewis et al., 1991; Meller et al., 1991; Carstens and Wilson, 1993; Henderson et al., 2014; Golder et al., 2015; Gaston et al., 2021; Jenkins et al., 2021).
Sedation as Determined by the Modified Righting Reflex Test
Adult male Sprague Dawley rats were used to evaluate the effects of bolus injections of vehicle, D-cysteine (500 μmol/kg, IV) and D-CYSee (500 μmol/kg, IV), on the duration of morphine (10 mg/kg, IV)-induced impairment of the modified righting reflex test. Each rat was placed in an open plastic chamber to allow the duration of the loss of the modified righting reflex to be accurately determined. In essence, the injection of morphine resulted in the rats assuming a variety of distinct postures, including being sprawled out motionless on their stomach on the chamber floor, splayed out with the head up against the chamber wall, and lying motionless on their side. The duration of the sedative effects of morphine was determined by the time interval from the time of injection of morphine to full recovery of the modified righting reflex, taken when the rats assumed and kept a normal posture on all four legs (Ren et al., 2015, 2020; Yu et al., 2018; Jenkins et al., 2021). One set of rats (n = 12; 79.7 ± 0.6 days of age; 330 ± 2 g body weight) received a bolus injection of morphine (10 mg/kg, IV) and after 15 min, an IV injection of vehicle (saline). A second set of rats (n = 12; 80.1 ± 0.6 days of age; and 335 ± 3 g body weight) received a bolus injection of morphine (10 mg/kg, IV) and after 15 min, an injection of D-cysteine (500 μmol/kg, IV). A third set of rats (n = 12; 80.1 ± 0.6 days of age; and 335 ± 3 g body weight) received a bolus injection of morphine (10 mg/kg, IV) and after 15 min, an injection of D-CYSee (500 μmol/kg, IV).
Data Analyses
All data are presented as mean ± SEM and were evaluated using one-way and two-way ANOVAs followed by Bonferroni’s corrections for multiple comparisons between means using the error mean square terms from each ANOVA (Wallenstein et al., 1980; Ludbrook, 1998; McHugh, 2011) as described in detail previously (Getsy et al., 2021). A value of p < 0.05 was taken as the initial level of statistical significance (Wallenstein et al., 1980; Ludbrook, 1998; McHugh, 2011). Statistical analyses were performed using GraphPad Prism software (GraphPad Software, Inc., La Jolla, CA, United States).
Results
Effects of D-CYSee and D-Cysteine on Ventilatory Responses to Morphine
The baseline (pre) ventilatory parameters before injecting vehicle or D-CYSee (500 μmol/kg, IV) are shown in Supplementary Table S2. There were no between group differences for any parameter (p > 0.05, for all comparisons via one-way ANOVA). The responses elicited by morphine in the 15-min period before giving vehicle or D-CYSee are shown in Supplementary Tables S3, S4. Descriptions of individual responses to morphine are given below. Moment-to-moment values of frequency of breathing (Freq), tidal volume (TV), and minute ventilation (MV) before and after injection of morphine (10 mg/kg, IV) and then vehicle or D-CYSee are shown in Figure 1. Morphine elicited a transient increase in Freq followed by a sustained decrease that was present when vehicle or D-CYSee was given (Figure 1A). The vehicle injection did not elicit a response in Freq, which recovered to pre-injection values within 20–25 min. D-CYSee elicited brief decreases in Freq for about 1 min followed by a sustained rise in Freq to pre-morphine values. Morphine elicited a sustained fall in TV that was pronounced at injection of vehicle or D-CYSee (Figure 1B). The injection of vehicle did not immediately change TV, which gradually recovered to pre-injection levels within 90 min. The first injection of D-CYSee elicited a prompt and sustained reversal of the effects of morphine on TV, and the second injection elicited a minor further increase. TV values stayed at or above baseline over the post-D-CYSee injection phases. As a result of the changes in Freq and TV, morphine elicited a sustained decrease in MV in vehicle-treated rats, whereas D-CYSee elicited a prompt and sustained reversal of the adverse effects of morphine on MV (Figure 1C).
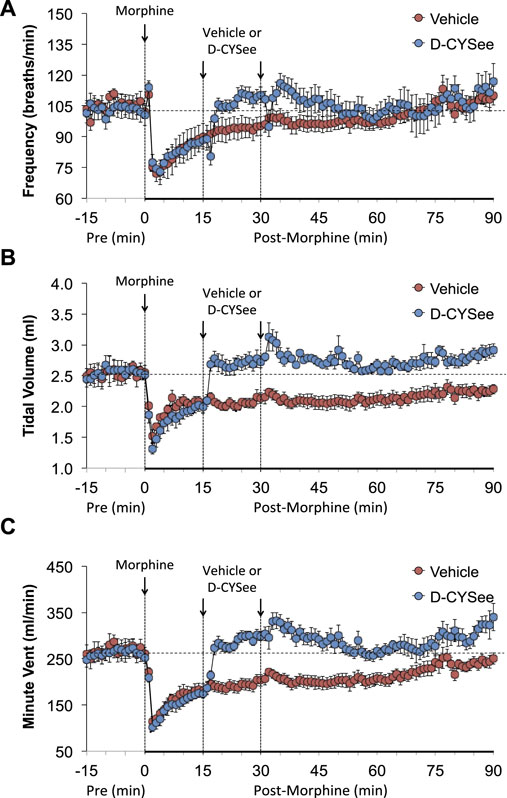
FIGURE 1. Values of frequency of breathing (Panel (A)), tidal volume (Panel (B)), and minute ventilation (Panel (C)) in freely-moving rats prior to (pre) and following injection of morphine (10 mg/kg, IV) and subsequent injections of vehicle (saline, IV) or D-cysteine ethyl ester (D-CYSee, 500 μmol/kg, IV). Data are presented as mean ± SEM. There were 5 rats in the vehicle group and 6 rats in the D-CYSee group.
As shown in Figure 2A, morphine elicited a transient decrease in Ti followed by sustained increases in rats that received vehicle 15 min after injection of morphine. The first and second injections of D-CYSee elicited transient decreases in Ti. The long-lasting rise in Ti elicited by morphine between 45 and 90 min post-injection was essentially similar in vehicle- and D-CYSee–treated rats. As shown in Figure 2B, morphine elicited a transient increase in Te followed by a long-lasting decrease in Te in rats that received vehicle 15 min after injection of morphine. The injections of D-CYSee elicited brief increases in Te, but minimally affected the long-lasting morphine-induced decrease in Te. As shown in Figure 2C, the respiratory quotient (Te/Ti) fell markedly after injection of morphine in vehicle-treated rats and similarly in D-CYSee-treated rats, while it was evident that the injections of D-CYSee elicited brief increases in Te/Ti. As shown in Supplementary Figure S2A, morphine elicited a sustained increase in end inspiratory pause (EIP). The injections of D-CYSee elicited transient decreases in EIP. As seen in Supplementary Figure S2B, morphine elicited robust transient, increases in end expiratory pause (EEP) followed by sustained decreases in EEP. The two injections of D-CYSee elicited minor initial increases in EEP, but did not alter the long-term decrease in EEP.
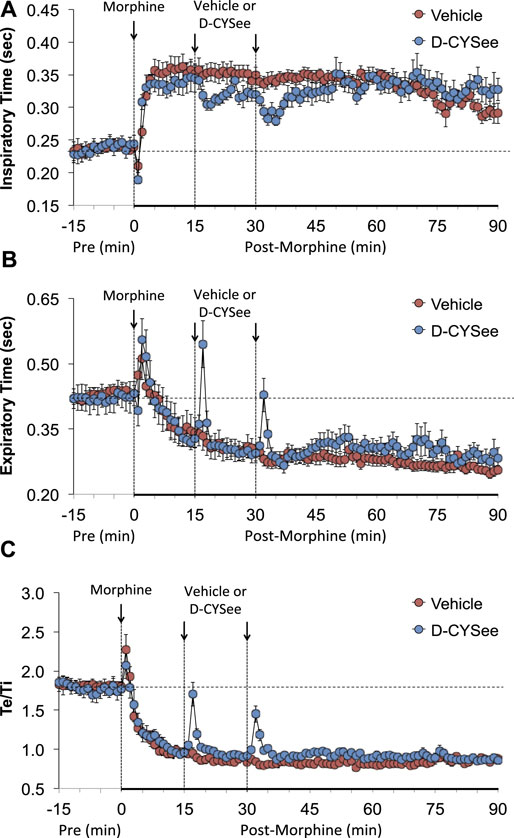
FIGURE 2. Values of inspiratory time (Panel (A)), expiratory time (Panel (B)), and expiratory time/inspiratory time (Te/Ti) (Panel (C)) in freely-moving rats prior to (pre) and following injection of morphine (10 mg/kg, IV) and subsequent injections of vehicle (saline, IV) or D-cysteine ethyl ester (D-CYSee, 500 μmol/kg, IV). Data are presented as mean ± SEM. There were 5 rats in the vehicle group and 6 rats in the D-CYSee group.
As seen in Figure 3, morphine elicited a pronounced and sustained fall in PIF (Figure 3A) but a transient decrease in PEF (Figure 3B) in vehicle-treated rats. The injections of D-CYSee elicited a prompt and sustained reversal of the effects of morphine on PIF and a marked increase in PEF to levels above pre-morphine values. Morphine elicited a prompt and sustained increase in PEF/PIF ratios (Figure 3C) in vehicle-treated rats. D-CYSee elicited robust decreases in PEF/PIF that were not sustained throughout the entire recording period. As shown in Figure 4, morphine elicited a prompt and sustained decrease in inspiratory drive (Figure 4A) and a pronounced transient decreases in expiratory drive (Figure 4B). D-CYSee caused partial recovery of inspiratory drive, and a sustained increase in expiratory drive to above pre-morphine levels. As shown in Supplementary Figure S3A, morphine elicited a sustained increase in EF50 that remained elevated in rats that received vehicle. The first injection of D-CYSee elicited a prompt and sustained increase in EF50 that was unchanged with the second injection. As seen in Supplementary Figure S3B, morphine elicited a prompt and sustained decrease in relaxation time. Vehicle injections elicited minimal responses, whereas D-CYSee elicited transient increases in relaxation time. As seen in Supplementary Figure S3C, morphine elicited a prompt and, after a noticeable decline in peak response, sustained increase in apneic pause. As seen in Supplementary Figure S3D, morphine elicited a prompt and transient increase in expiratory delay. The injections of vehicle elicited minimal changes in both apneic pause and expiratory delay, whereas D-CYSee elicited transient increases in both parameters. As seen in Supplementary Figure S4, morphine elicited robust increases in NEBI (Supplementary Figure S4A) and NEBI/Freq (Supplementary Figure S4B) of about 5 min in duration followed by sustained decreases in these parameters. The injections of vehicle or D-CYSee elicited minor changes in these parameters.
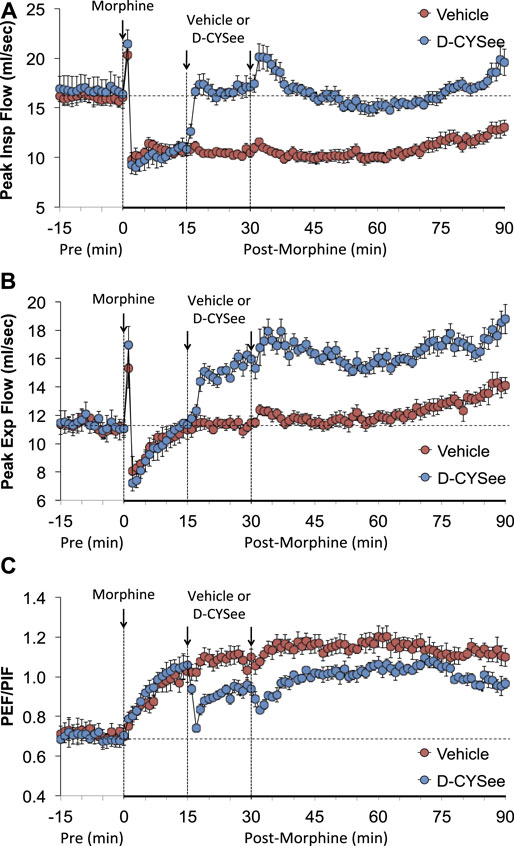
FIGURE 3. Values of peak inspiratory flow (Panel (A)), peak expiratory flow (Panel (B)), and peak expiratory flow/peak inspiratory flow (PEF/PIF) (Panel (C)) in freely-moving rats prior to (pre) and following injection of morphine (10 mg/kg, IV) and subsequent injections of vehicle (saline, IV) or D-cysteine ethyl ester (D-CYSee, 2 × 500 μmol/kg, IV). The data are presented as mean ± SEM. There were 5 rats in the vehicle group and 6 rats in the D-CYSee group.
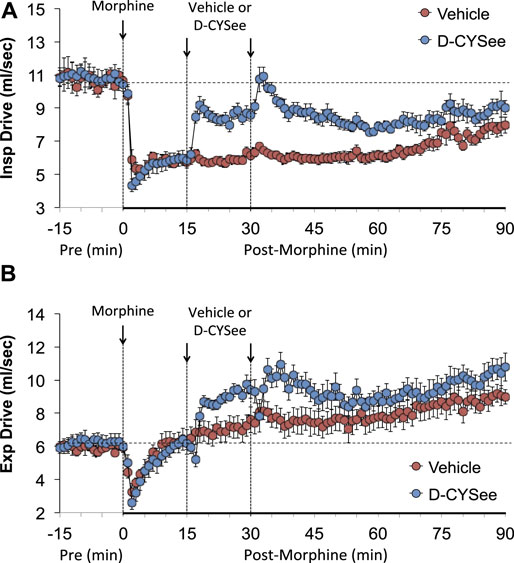
FIGURE 4. Calculated values of inspiratory drive (TV/Ti) (Panel (A)) and expiratory drive (TV/Te) (Panel (B)) in freely moving rats prior to (pre) and following injection of morphine (10 mg/kg, IV) and subsequent injections of vehicle (saline) or D-cysteine ethyl ester (D-CYSee, 500 μmol/kg, IV). The data are presented as mean ± SEM. There were 5 rats in the vehicle group and 6 rats in the D-CYSee group.
The total changes in ventilatory parameters recorded over the 15-min period following the first injection of vehicle or D-CYSee (expressed as % change from pre-values) are summarized in Figure 5. The three groups are those that received two injections of vehicle (VEH group), two injections of D-CYSee (D-CYSee (2x) group), and one injection of D-CYSee (D-CYSee (1x) group). As seen in Figure 5A, the morphine-induced decreases in Freq, TV and MV were significantly diminished in D-CYSee (2x) and D-CYSee (1x) groups, whereas changes in Ti, Te and Te/Ti were only marginally diminished (combined alterations in Ti and Te in D-CYSee (2x) and D-CYSee (1x) groups were significant and consistent with reversal of the decrease in Freq in each group, p < 0.05 for both comparisons via two-way ANOVA). As seen in Figure 5B, the morphine-induced changes in EIP, EEP, PIF, PEF, PEF/PIF and EF50 were all substantially reversed in D-CYSee (2x) and D-CYSee (1x) groups. As seen in Figure 5C, the morphine-induced decreases in inspiratory drive (TV/Ti) were diminished in D-CYSee (2x) and D-CYSee (1x) groups, and morphine-induced increases in expiratory drive (TV/Te) were augmented in D-CYSee (2x) and D-CYSee (1x) rats. In contrast, total changes in relaxation time (RT), apneic pause (ApP), NEBI, and NEBI/Freq were similar in the three groups. The total changes in ventilatory parameters recorded over the 60-min period after the second set of injections (% change from pre-values) are summarized in Figure 6. As seen in Figure 6A, the morphine-induced decreases in TV and MV were diminished in the D-CYSee (2x) and D-CYSee (1x) groups. There were minimal changes in Freq, and the pronounced increases in Ti and decreases in Te were similar in all three groups. As seen in Figure 6B, the decreases in PIF were smaller in D-CYSee (2x) and D-CYSee (1x) rats, whereas the increases in PEF and EF50 were augmented in D-CYSee (2x) and D-CYSee (1x) rats. The morphine-induced changes in EIP, EEP, and PEF/PIF were similar in all three groups. The resting values for Freq, TV, and MV prior to injection of vehicle or D-cysteine (500 umol/kg, IV) are presented in the legend of Supplementary Figure S5. There were no between group differences in any parameter (p > 0.05, for all comparisons via one-way ANOVA). As seen in Supplementary Figure S5, morphine (10 mg/kg, IV) elicited substantial falls in Freq (Supplementary Figure S5A), TV (Supplementary Figure S5B), and MV (Supplementary Figure S5C) that were not affected by the injections of D-cysteine.
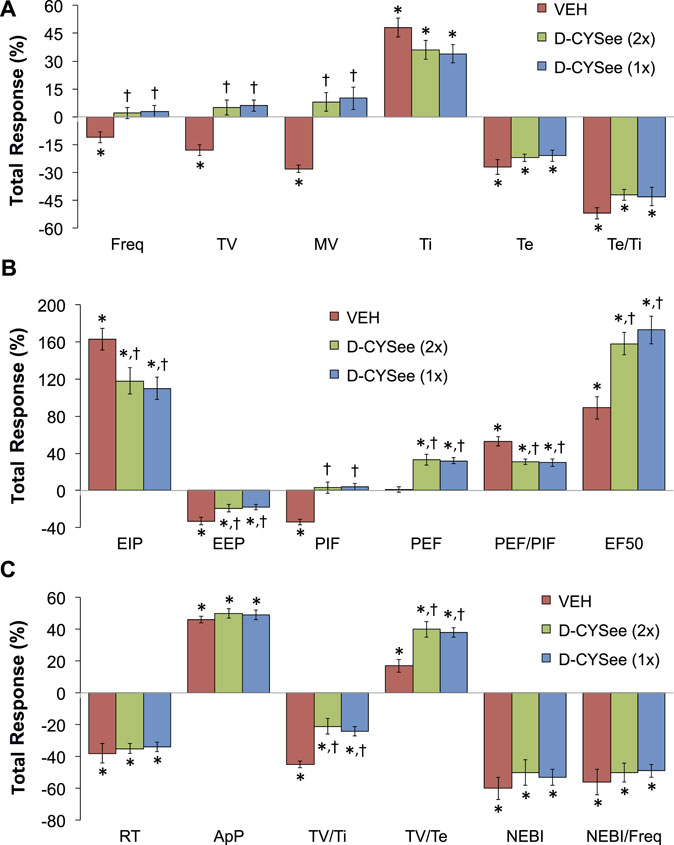
FIGURE 5. Total changes (expressed as % change from pre-values) in Freq, TV, MV, Ti, Te and Te/Ti (A), EIP, EEP, PIF, PEF, PEF/PIF and EF50 (B), and RT, ApP, TV/Ti, TV/Te, NEBI, and NEBI/Freq (C) that occurred over the 15-min period following the first injection of vehicle (VEH, IV) or D-cysteine ethyl ester (D-CYSee, 500 μmol/kg, IV). The vehicle (VEH) group received two injections of vehicle. The D-CYSee (2x) group received two injections of D-CYSee, whereas the D-CYSee (1x) group received one injection of D-CYSee and then an injection of vehicle. There were 5 rats in the vehicle group and 6 rats in the D-CYSee groups. *p < 0.05, significant change from pre. †p < 0.05, D-CYSee versus vehicle.
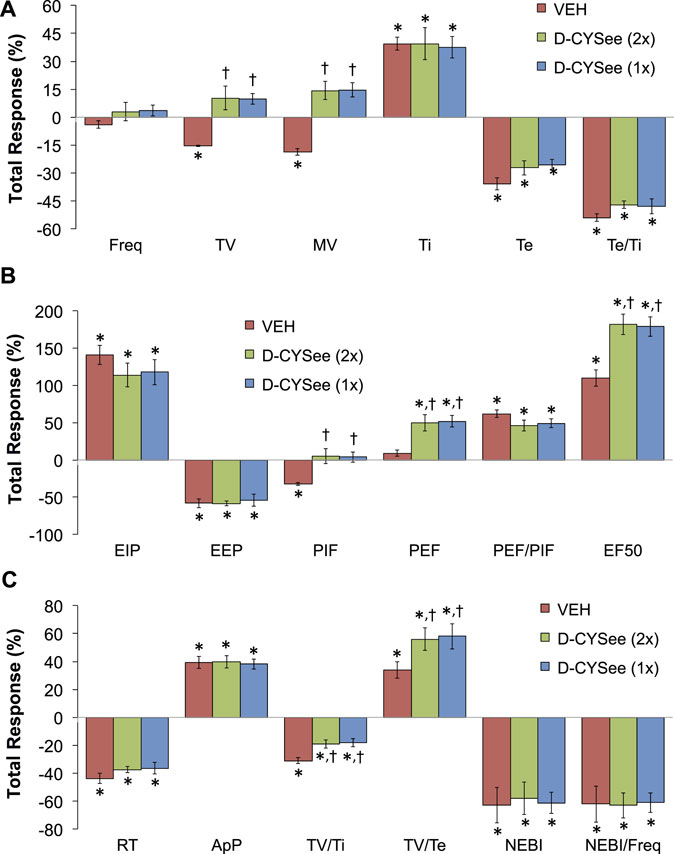
FIGURE 6. Total changes (expressed as % change from pre-values) in ventilatory parameters that occurred over the 60-min period following the second set of injections of either vehicle (VEH) or D-cysteine ethyl ester (D-CYSee, 500 μmol/kg, IV). The vehicle (VEH) group received two injections of vehicle. The D-CYSee (2x) group received two injections of D-CYSee, whereas the D-CYSee (1x) group received one injection of D-CYSee and then an injection of vehicle. There were 5 rats in the vehicle group and 6 rats in the D-CYSee group. *p < 0.05, significant change from pre-values. †p < 0.05, D-CYSee versus vehicle.
Effects of D-CYSee and D-Cysteine on Morphine-Induced Changes in ABG Chemistry and A-a Gradient
ABG values (pH, pCO2, pO2 and sO2) in three groups of freely-moving rats prior to and after injection of morphine (10 mg/kg, IV) and subsequent injection of vehicle (VEH, IV), D-cysteine (500 μmol/kg, IV), or D-CYSee (500 μmol/kg, IV) are summarized in Figure 7. The values denoted M15–M60 are the times post-morphine, whereas values denoted D5–D45 are the times post-vehicle/drug injection. As seen in Figure 7A, morphine elicited a substantial fall in pH in the three groups, and D-CYSee elicited a prompt and sustained reversal of the acidosis. As seen in Figure 7B, morphine elicited a substantial increase in pCO2 in the three groups and D-CYSee elicited an immediate and sustained reversal of the hypercapnia. As seen in Figures 7C,D, morphine elicited sustained decreases in pO2 and sO2, respectively, and D-CYSee elicited a prompt and sustained reversal of this hypoxemia. In contrast, the time-dependent trends toward pre-morphine values for pH, pCO2, pO2, and sO2 were similar in rats that received vehicle or D-cysteine. The A-a gradient values (index of alveolar gas exchange) in three groups of freely-moving rats before and after injection of morphine (10 mg/kg, IV) and then injections of vehicle (VEH, IV), D-cysteine (500 μmol/kg, IV), or D-CYSee (500 μmol/kg, IV) are shown in Figure 8. Morphine caused a sustained increase in A-a gradient, and D-CYSee elicited an immediate and sustained reversal of this effect, whereas vehicle and D-cysteine did not.
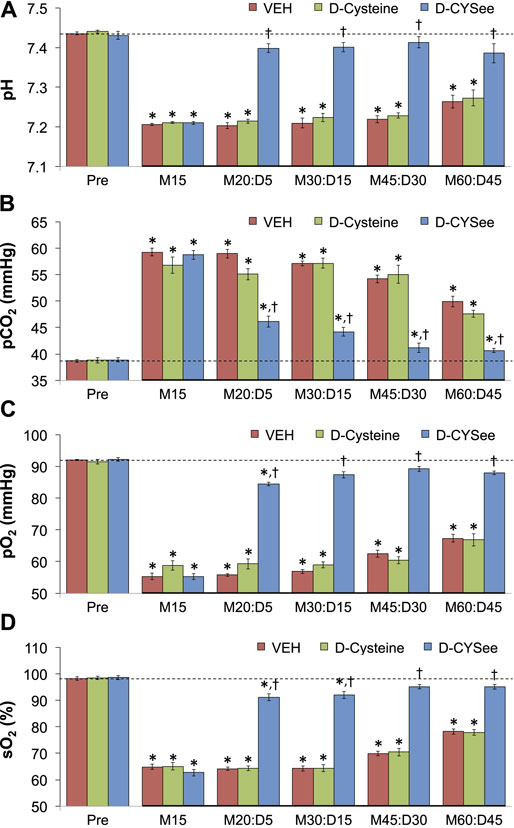
FIGURE 7. Values of pH (Panel (A)), pCO2 (Panel (B)), pO2 (Panel (C)), and sO2 (Panel (D)) before (Pre) and after injection of morphine (10 mg/kg, IV) in three separate groups of freely-moving rats followed by injection of vehicle (VEH (saline), IV), D-cysteine (500 μmol/kg, IV), or D-cysteine ethyl ester (D-CYSee, 500 μmol/kg, IV). The terms M15, M20, M30, M45, and M60 denote 15, 30, 45, and 60 min after injection of morphine. The terms D5, D15, D30, and D45 denote 5, 15, 30, and 45 min after injection of the drug (vehicle, D-cysteine, or D-CYSee). The data are mean ± SEM. There were 8 rats in the vehicle- or D-CYSee–treated groups and 6 rats in the D-cysteine–treated group. *p < 0.05, significant change from pre-values. †p < 0.05, D-CYSee versus vehicle.
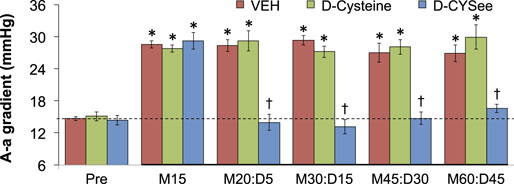
FIGURE 8. Alveolar–arterial (A-a) gradient values before (Pre) and after injection of morphine (10 mg/kg, IV) in three groups of freely-moving rats followed by an injection of vehicle (VEH (saline), IV), D-cysteine (500 μmol/kg, IV), or D-cysteine ethyl ester (D-CYSee, 500 μmol/kg, IV). The terms M15, M20, M30, M45, and M60 denote 15, 30, 45, and 60 min after injection of morphine. The terms D5, D15, D30, and D45 denote 5, 15, 30, and 45 min after injection of drug (vehicle, D-cysteine, or D-CYSee). The data are mean ± SEM. There were 8 rats in the vehicle- or D-CYSee-treated groups and 6 rats in the D-cysteine–treated group. *p < 0.05, significant change from pre-values. †p < 0.05, D-CYSee versus vehicle.
Effects of D-CYSee and D-Cysteine on Antinociceptive Responses to Morphine
The temporal effects of a 5- or 10-mg/kg dose of morphine on TFL and subsequent effects elicited by an injection of vehicle or D-CYSee (500 μmol/kg, IV) are summarized in Figure 9. The 5-mg/kg (Figures 9A,C) and 10-mg/kg (Figures 9B,D) doses of morphine both elicited pronounced antinociception with the effects of the 10-mg/kg dose being more pronounced than the 5-mg/kg dose. As can be seen, the temporal changes in TFL elicited by either dose of morphine were similar in rats that received vehicle or D-CYSee.
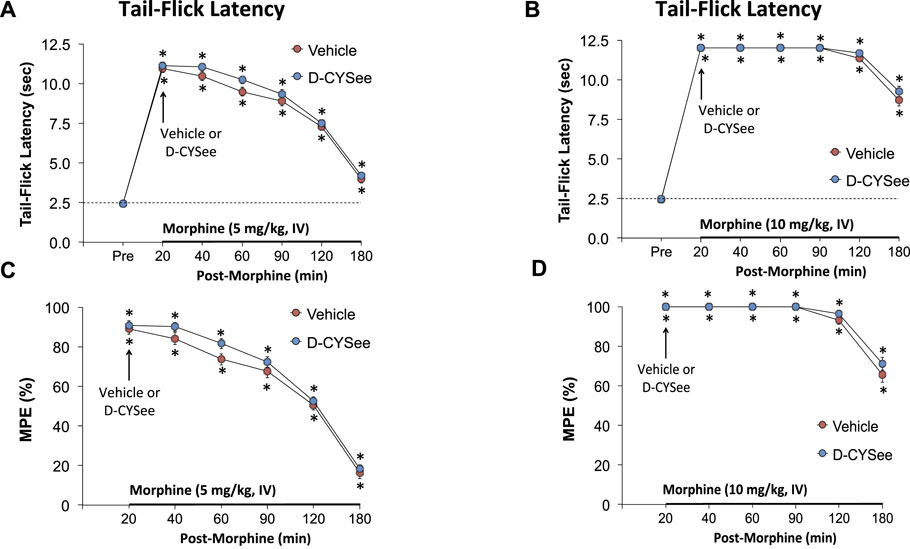
FIGURE 9. Tail-flick latencies elicited by intravenous injection of 5 mg/kg (Panel (A)) or 10 mg/kg (Panel (B)) of morphine in freely-moving rats that received injections of vehicle (VEH (saline), IV) or D-CYSee (500 μmol/kg, IV) 20 min after the injection of morphine. Panels (C,D) display the data as percentage maximum possible effect (%MPE). The data are shown as mean ± SEM. There were 6 rats in each group. *p < 0.05, significant change from pre-values. There were no between group comparisons at any time-point (p > 0.05, for all comparisons).
Effects of D-CYSee and D-Cysteine on the Sedative Effects of Morphine
The general behaviors of the rats that received morphine plus vehicle, D-cysteine or D-CYSee were similar to one another. Morphine caused an immediate (within 2 min) sedative effect in all rats that was manifested as a lack of mobility and unusual body postures. The full return of the righting reflex in vehicle-treated rats (72.3 ± 9.2 min, n = 12), D-cysteine (500 μmol/kg, IV)–treated rats (70.5 ± 10.2 min, n = 12), and D-CYSee (500 μmol/kg, IV)–treated rats (85.6 ± 8.6 min, n = 12) was equal to one another (p > 0.05, for all between-group comparisons via two-way ANOVA).
Discussion
General Observations About D-CYSee and Thiol esters
Our study provides evidence that D-CYSee produces a rapid and long-lasting reversal of the deleterious changes in breathing, ABG chemistry, and alveolar gas exchange (i.e., a reversal of the elevated A-a gradient) elicited by a 10-mg/kg dose of morphine in freely moving (unanesthetized) male Sprague–Dawley rats. However, D-CYSee did not greatly alter the sedative antinociceptive or effects of this dose of morphine (the antinociceptive effects were slightly augmented). Since the above actions of morphine are immediately reversed by systemic injection of OR antagonists (van Dorp et al., 2007; Dahan et al., 2010, 2018; Boom et al., 2012; Algera et al., 2019), it is unlikely that D-CYSee alters the actions of morphine by competitive or non-competitive antagonism of ORs. We have no evidence as to the physiological site(s) of action or molecular processes by which D-CYSee profoundly reverses morphine-induced changes in breathing, ABG chemistry, and A-a gradient, while slightly augmenting the antinociceptive effects of the 10-mg/kg dose of this opioid. There is evidence that morphine-induced inhibition of excitatory amino acid transporter 3 (Trivedi et al., 2014; Trivedi and Deth, 2015) blocks L-cysteine entry into cells, which suggests that the adverse effects of morphine involve (1) reduced intracellular L-cysteine levels and accompanying increase in oxidative status of the cell (Trivedi et al., 2014; Trivedi and Deth, 2015), and/or (2) diminished involvement of L-cysteine in metabolic pathways in cells, such as generation of hydrogen sulfide (Wu, 2009; Yin et al., 2016; Paul et al., 2018). Since the antinociceptive effects of a 5-mg/kg dose of morphine were slightly shorter in duration in D-CYSee-treated rats than in vehicle-treated rats, it suggests that the 10-mg/kg dose of morphine may have masked some adverse effects of D-CYSee with respect to antinociceptive signaling.
Potential Mechanisms of Action of D-CYSee and Other Thiol esters
Although the abilities of D-CYSee and L-CYSee (Mendoza et al., 2013) to reverse the deleterious actions of morphine on breathing and alveolar gas exchange could involve supplying reducing equivalents to target cells, we found that the potent reducing and cell-permeable thiol ester, N-acetyl-L-cysteine methyl ester (L-NACme) (Tsikas et al., 2018), had minimal effects on the ventilatory-depressant effects of morphine (Gaston et al., 2021). The readily conversion of L-NACme to L-cysteine in cells (Lailey and Upshall, 1994) argues that the effects of D-CYSee are not due to conversion to H2S via the D-amino acid oxidase–mercaptopyruvate sulfur transferase system (Shibuya and Kimura, 2013; Kimura, 2014). Since the superoxide anion/free radical scavenger, Tempol, attenuates OIRD elicited by morphine and fentanyl (Baby et al., 2021a,b), it points to the importance of redox mechanisms in the actions of opioids and potentially the ability of thiol esters to reverse OIRD. Aside from direct interactions with functional proteins, potential mechanisms of action of D-CYSee may involve (1) direct binding to myristoylated alanine-rich C-kinase substrate (MARCKS), which is a D-cysteine binding protein (Semenza et al., 2021); (2) modulation of OR-β-arrestin cell signaling events that spare the analgesic G-protein–mediated actions of morphine (Schmid et al., 2017; Grim et al., 2020); and/or (3) conversion of D-CYSee to S-nitroso-D-CYSee by nitric oxide synthase-dependent processes (Perissinotti et al., 2005; Hess and Stamler, 2012; Stomberski et al., 2019; Seckler et al., 2021), which may act similar to the intracellular S-nitrosylating agent, S-nitroso-L-CYSee (Clancy et al., 2001). We determined whether D-CYSee increases NADPH diaphorase expression in tissues, which identifies S-nitrosylated species (both free S-nitrosothiols and S-nitrosylated proteins) in aldehyde-treated tissues (Seckler and Lewis, 2020). S-nitrosothiols, such as S-nitroso-L-cysteine and S-nitroso-L-glutathione, play key roles in ventilatory control processes in brainstem and peripheral structures, such as the carotid bodies (Palmer et al., 2013; Gaston et al., 2014; Palmer et al., 2015; Gaston et al., 2020; Gaston et al., 2021). We reported that the ability of morphine to depress breathing and adversely affect ABG chemistry is reduced in rats undergoing intravenous infusion of S-nitroso-L-cysteine (Getsy et al., 2022), and that whereas S-L-nitrosothiols exert pronounced effects on cardiorespiratory systems, D-isomers exert minimal effects (Davisson et al., 1996; Lewis et al., 1996; Travis et al., 1996; Davisson et al., 1997; Ohta et al., 1997; Travis et al., 1997; Lewis et al., 2005; Lewis et al., 2006; Gaston et al., 2020; Gaston et al., 2021).
Our findings that D-cysteine did not mimic the effects of D-CYSee suggest that (1) D-CYSee activates membrane-bound functional proteins not activated by D-cysteine; (2) D-CYSee acts by entering cells and modulating intracellular signaling pathways as the thiol ester; (3) D-cysteine is unable to enter into cells via uptake systems available to L-cysteine (Tunnicliff, 1994; Shanker and Aschner, 2001; Aoyama et al., 2008; Albrecht and Zielińsk, 2019), including high-affinity sodium-dependent glutamate transporters (e.g., GLT1 and GLAST) (Hargreaves et al., 1988), excitatory amino acid transporters 1–3 (Zerangue and Kavanaugh, 1996; Chen and Swanson, 2003; Himi et al., 2003; Trivedi et al., 2014; Trivedi and Deth, 2015; Albrecht and Zielińsk, 2019), large neutral amino acid transporters, LAT1 and LAT2 (Simmons-Willis et al., 2002; Nemoto et al., 2003; Li and Whorton, 2007; Granillo et al., 2008), and the band 3 protein/anion transport system (Young et al., 1981; Tunnicliff, 1994), with the only direct evidence being that D-cysteine is not transported by LAT1 or LAT2 (Simmons-Willis et al., 2002); and (4) D-cysteine cannot modulate the activity of intracellular proteins modulated by D-CYSee. We are currently exploring whether the formation of D-CYSee: D-glucose and/or D-cysteine: D-glucose, similar to L-cysteine: D-glucose (Gomez et al., 1994; Wróbel et al., 1997; Szwergold, 2006; Li et al., 2015) contributes to the effects of D-CYSee.
D-CYSee Reverses the Deleterious Effects of Morphine on Ventilatory Parameters
As expected (May et al., 2013a,b; Young et al., 2013; Baby et al., 2018, Baby et al., 2021 SM.; Gaston et al., 2021), a 10-mg/kg dose of morphine elicited a relatively transient decrease in Freq, which was not truly indicative of the actual effects of morphine, since morphine elicited sustained increases in Ti (lengthened inspiration), whereas it elicited pronounced decreases in Te (shortened expiration). Morphine also elicited a sustained increase in EIP, but a relatively transient decrease in EEP followed by a sustained decrease. The propensity of morphine to elongate Ti while not greatly affecting Te (Fone and Wilson, 1986; Kasaba et al., 1997; Chevillard et al., 2009) or shortening Te (May et al., 2013a,b; Young et al., 2013; Baby et al., 2018, Baby et al., 2021 SM.; Gaston et al., 2021) is known, as is the ability of opioids to differentially affect EIP and EEP (Henderson et al., 2013, 2014; May et al., 2013a,b; Young et al., 2013; Baby et al., 2018, Baby et al., 2021 SM.; Gaston et al., 2021). The molecular mechanisms and brain sites, such as the nucleus tractus solitarius (Hassan et al., 1982; Li et al., 1996; Iniushkin, 1997; Zhuang et al., 2012), pre-Bötzinger complex (Bachmutsky et al., 2020; Varga et al., 2020), and parabrachial nucleus/Kölliker–Fuse nucleus (Eguchi et al., 1987; Lalley et al., 2014; Bachmutsky et al., 2020; Varga et al., 2020), by which the differential effects of opioids (e.g., morphine and fentanyl) on inspiratory and expiratory timing are accomplished have received attention, and the conclusion is that the qualitative/quantitative effects of opioids on Ti and Te are dose-dependent (Li et al., 1996; Henderson et al., 2013, 2014). In contrast, the sites and mechanisms by which opioids differentially affect EIP and EEP have received limited attention (Henderson et al., 2014; Bachmutsky et al., 2020). Although studies in anesthetized rats and in in vitro preparations suggest that the ability of morphine to decrease Freq involves depression of carotid body chemoreceptor afferent reflexes, the ability of morphine (10 mg/kg, IV) to depress breathing in unanesthetized rats is enhanced in rats, which had undergone prior bilateral carotid sinus nerve transection (Baby et al., 2018), suggesting that morphine promotes rather than inhibits carotid body chemoreflex activity in this circumstance.
The findings that the injections of D-CYSee elicited transient decreases in Freq in morphine-treated rats, and that both responses were followed by sustained reversal of the depressant effects of morphine, points to D-CYSee having multiple mechanisms of action that are not determined as of yet. The brief decreases in Freq were associated primarily with brief increases in Te, relaxation time, apneic pause, and expiratory delay, whereas Ti and EIP fell at these times, and EEP changed minimally. It appears that D-CYSee initially targets mechanisms that regulate some aspects of expiratory timing in morphine-treated rats, and it does not adversely impact inspiratory timing control processes. In contrast, the ability of D-CYSee to reverse the adverse effects of morphine on Freq was almost totally due to the reversal of the enhanced Ti, as D-CYSee elicited relatively minor effects on Te, EIP, EEP, relaxation time, apneic pause or expiratory delay. As such, it would be of great interest to determine whether microinjections of D-CYSee into key brainstem nuclei, such as the nucleus tractus solitarius, pre-Bötzinger complex, Kölliker–Fuse nucleus, and/or parabrachial nucleus, are able to modulate the effects of morphine on respiratory timing and other parameters.
As reported, morphine elicited sustained decreases in TV, MV and PIF; sustained increases in EF50; and transient decreases in PEF (May et al., 2013a,b; Young et al., 2013; Baby et al., 2018, Baby et al., 2021 SM.; Gaston et al., 2021). This pattern of responses speaks to the disparate role of OR signaling mechanisms in the control of breathing. Another key set of findings pertains to the first injection of D-CYSee eliciting a rapid and sustained reversal of the adverse effects of morphine on TV, MV and PIF, and augmentation of PEF and EF50 to values that were above those of morphine alone, with a second injection of D-CYSee generally maintaining the response. We conclude that the second injection of D-CYSee was not necessary for full effect as shown by responses in rats that received a single dose of D-CYSee. Our unpublished data shows that the first injections of a 500-μmol/kg dose of L-cysteine ethyl ester or L-cysteine methyl ester elicit similar responses to D-CYSee, whereas the second injection of the L-thiol esters elicits pronounced ventilatory responses in morphine-treated rats. This suggests that the second dose of L-isomers activates signal/enzymatic pathways that D-CYSee cannot. We also found that morphine elicited a pronounced, but transient increase in NEBI, likely due to greater occurrence of apneas (Henderson et al., 2013, 2014; Gaston et al., 2021) followed by a sustained decrease in this index of respiratory instability. Thus, D-CYSee had no effects on morphine promotion of eupneic breathing.
D-CYSee Reverses the Deleterious Effects of Morphine on ABG Chemistry and A-a Gradient
As expected from our previous studies in rats (Gaston et al., 2021; Getsy et al., 2022), the bolus intravenous injection of morphine elicited pronounced and long-lasting deleterious changes in ABG chemistry (i.e., a decrease in pH, an increase in pCO2, and decreases in pO2 and sO2) that are consistent with hypoventilation, and accompanied by a sustained increase in A-a gradient, which indicates a problem with alveolar gas exchange (Gaston et al., 2021; Getsy et al., 2022). This increase in A-a gradient could be due to alveolar collapse (atelectasis) because of hypoventilation (Gaston et al., 2021; Getsy et al., 2022) or may also involve more complicated effects on surfactant status and/or alveolar fluid clearance, which if reduced would impair gas exchange in alveoli that remain open, despite a decreased TV. As such, the second set of key findings was that D-CYSee elicited an immediate and sustained reversal of the adverse effects of morphine on ABG chemistry and A-a gradient. These findings are important since improved ABG chemistry is the final arbiter of how well a reversal agent combats OIRD. Whether the improved (normalized) status of ABG chemistry and A-a gradient is due to improved ventilation and reversal of atelectasis or whether D-CYSee directly affects alveoli signaling processes controlling surfactant and fluid status is unknown.
D-CYSee Does Not Affect the Antinociceptive Actions of Morphine
The third set of key findings pertains to the lack of effect of D-CYSee on morphine antinociception. As expected, the injections of the 5- or 10-mg/kg doses of morphine elicited robust increases in TFL (antinociceptive effects) of over 180 min in duration (Gaston et al., 2021). D-CYSee (500 μmol/kg, IV) did not alter the temporal changes in TFL elicited by 5- or 10-mg/kg doses of morphine. There is no information about how D-CYSee affects nociceptive signaling although Pathirathna et al. (2006) found that intra-dermal injections of D-cysteine into the ventral side of a hind paw (peripheral receptive field) of rats caused dose-/time-dependent hyperalgesia that was blocked by 3-betaOH, a neuroactive steroid that blocks voltage-dependent T-type Ca2+ channels. These and other data (Todorovic and Jevtovic-Todorovic, 2014) imply that changes in redox status of nociceptors may function as a local intrinsic mechanism in controlling peripheral pain perception. Our data suggest that D-CYSee does not cause (1) dynamic changes in the functional status of ORs (e.g., phosphorylation, desensitization, and internalization events), and (2) modulation of intracellular signaling cascades by which morphine elicits its antinociception effects (Connor and Christie, 1999; Williams et al., 2013).
Study Limitations
Our findings that a 500-μmol/kg dose of D-CYSee reverses the adverse actions of morphine on breathing, ABG chemistry and alveolar gas exchange need to be evaluated at lower doses. The ability of D-CYSee to reverse the adverse effects of higher potency opioids, such as fentanyl and carfentanil, should be determined, especially considering the key role that these synthetic opioids are playing in the current opioid epidemic (Arendt, 2021; Deo et al., 2021). Another important limitation is the lack of evidence as to the efficacy of D-CYSee in reversing OIRD elicited by morphine and fentanyl in female rats knowing that opioids can exert qualitatively/quantitatively different responses in females than males (Dahan et al., 1998; Hosseini et al., 2011). Another limitation pertains to our lack of understanding of molecular mechanisms by which D-CYSee modulates the actions of opioids. We are examining the extent to which systemic D-CYSee generates S-nitrosothiols in brain structures such as, the nucleus tractus solitarius, and peripheral structures such as, the carotid bodies, by NADPH diaphorase histochemistry (Seckler et al., 2020) and sensor technology (Seckler et al., 2017). At present, we do not know whether the ability of D-CYSee to reverse morphine-induced respiratory depression is due to its direct respiratory stimulant effects and/or its ability to counteract the inhibitory actions of µ-opioid receptor activation. We have preliminary data that the injection of D-CYSee (500 μmol/kg, IV) elicits a prompt increase in breathing in freely moving rats that peaks at 2 min (+59.6 ± 7.3%, n = 6 rats, p < 0.05), but subsides before 10 min and at 15 min post-injection, the values were +3.6 ± 7.3% of pre-injection values (p > 0.05). However, despite the recovery to baseline, the injection of morphine at the 15-min post-D-CYSee time point elicited markedly smaller decreases in MV than in vehicle-injected rats. There was a maximal decrease in MV in the vehicle- and D-CYSee-injected rats being −61.5 ± 7.3% (p < 0.05) and −23.8 ± 5.4%, p < 0.05, respectively (p < 0.05, comparing D-CYSee vs. vehicle). Moreover, the return to baseline levels after the injection of morphine occurred within 73.7 ± 9.4 min in vehicle-treated rats, but only 12.3 ± 3.6 min in D-CYSee-treated rats (p < 0.05, comparing D-CYSee vs. vehicle). Clearly, this means that despite the recovery of MV to baseline following the administration of D-CYSee, the D-thiol ester or downstream products, such as S-nitroso-D-CYSee, may be present in sufficient quantities to countermand µ-opioid receptor activation. Ongoing pharmacokinetics studies using LC-MS methods available in our laboratory (Altawallbeh et al., 2019) are determining the temporal distribution of D-CYSee in the blood, peripheral organs and tissues, cerebrospinal fluid, and brain structures. Finally, we need to see whether D-CYSee can diminish the latent deleterious effects of morphine on ventilatory responses to hypoxic (May et al., 2013a) or hypoxic-hypercapnic (May et al., 2013b) gas challenges. Whether any pharmacological actions of D-CYSee involve conversion to the L-isomer is yet to be identified. As always, the most important concern is whether the pharmacological actions of OIRD reversal agents in rats will translate into effective therapies in humans. Many such OIRD reversal agents that have shown efficacy in rats did not show such efficacy in human clinical trials (Dahan et al., 2018; Algera et al., 2019).
Conclusion
The systemic injection of D-CYSee reversed many of the adverse effects of morphine on ventilatory parameters, A-a gradient, and ABG chemistry in unanesthetized rats, whereas it did not markedly influence the antinociceptive or sedative actions of the opioid. Since D-cysteine did not affect the actions of morphine, it appears that as a readily cell-penetrant thiol ester, D-CYSee may modulate the adverse actions of morphine by interfering/reversing the downstream intracellular signaling processes by which morphine acts, rather than by directly blocking ORs. It is tempting to assume that D-CYSee reverses the adverse effects of morphine by entering the brain. However, since peripherally restricted OR antagonists, such as naloxone methiodide, blunt the cardiorespiratory and analgesic actions of opioids (Henderson et al., 2014), we suggest that the ability of D-CYSee to affect the cardiorespiratory actions of morphine involves interactions with peripheral OR signaling pathways such as those in the carotid bodies. These present findings with D-CYSee add to our knowledge about the efficacy of thiol esters (Gaston et al., 2021; Jenkins et al., 2021) and the superoxide anion/free-radical scavenger, Tempol (Baby et al., 2021a,b), against OIRD. Since D-CYSee did not reverse the antinociception or sedation elicited by morphine, it would appear that the ability of D-CYSee to reverse the deleterious effects of morphine on breathing is not due to direct antagonist actions at ORs. However, a key unresolved issue is whether D-CYSee reverses the deleterious effects of morphine on breathing via reversal of OR-mediated cell signaling events or simply by being a respiratory stimulant. We have unpublished data showing that the injection of D-CYSee increases MV in naïve rats, but that the effects subside within 10–15 min. Importantly, the subsequent injection of morphine elicited markedly smaller decreases in MV in these rats compared with naïve rats. Accordingly, it appears that D-CYSee can block morphine-induced respiratory depression independently of it being a respiratory stimulant per se. The mechanisms by which D-CYSee may interfere with OR-signaling events are under investigation.
Data Availability Statement
The raw data supporting the conclusion of this article will be made available by the authors, without undue reservation.
Ethics Statement
The animal study was reviewed and approved by the Animal Care and Use Committees of Case Western Reserve University, the University of Virginia, and Loma Linda University.
Author Contributions
The study was originated and designed by PMG, SMB, BG, JNB, Y-HH, and SJL. The experiments were performed by PMG, WM, AY, JB, SB, CW, TL, and SJL. The data were collated and then statistically analyzed by TL, PMG, WM, AY, CW, and SJL. The figures and tables were prepared by PMG, TL, JB, and SJL. PMG, MH, HF, JB, Y-HH, and SJL contributed to the writing of the original version of the manuscript. All authors contributed to revising the manuscript and in the preparation of the final document that was submitted for publication.
Funding
These studies were supported in part by grants from Galleon Pharmaceuticals, Inc. (to SL), NIH PO1HL101871 (to BG), and NIDA U01DA051373 (to SL).
Conflict of Interest
SB was employed by Galleon Pharmaceuticals, Inc., during the performance of the studies in this manuscript. SL declares that this study was funded in part by Galleon Pharmaceuticals, Inc. The leadership of the company was not directly involved in this study as a commercial entity. Only the principal scientist of the company (SB) was involved in the design of the studies, the collection, analysis, interpretation of data, the writing of the manuscript, and the ultimate decision to submit the manuscript for publication.
The remaining authors declare that the research was conducted in the absence of any commercial or financial relationships that could be construed as a potential conflict of interest.
Publisher’s Note
All claims expressed in this article are solely those of the authors and do not necessarily represent those of their affiliated organizations, or those of the publisher, the editors, and the reviewers. Any product that may be evaluated in this article, or claim that may be made by its manufacturer, is not guaranteed or endorsed by the publisher.
Acknowledgments
The authors wish to sincerely thank the staff at the Animal Care Facilities at Case Western Reserve University, the University of Virginia, and Galleon Pharmaceuticals, Inc., for their expert assistance with the care of the rats. The authors also wish to acknowledge David Kalergis (CEO, Atelerix Life Sciences) for his assistance in detailing the clinical significance of our findings.
Supplementary Material
The Supplementary Material for this article can be found online at: https://www.frontiersin.org/articles/10.3389/fphar.2022.883329/full#supplementary-material
Abbreviations
A-a gradient, alveolar–arterial gradient; ABG, arterial blood–gas chemistry; D-CYSee, D-cysteine ethyl ester; EEP, end-expiratory pause; EF50, expiratory flow at 50% expired tidal volume; EIP, end-inspiratory pause; FiO2, fraction of O2 in inspired air; Freq, frequency of breathing; HPL, hind-paw withdrawal latency; L-NACme, N-acetyl-L-cysteine methyl ester; MPE, maximal positive effect; MV, minute ventilation; NEBI, non-eupneic breathing index; OIRD, opioid-induced respiratory depression; Patm, atmospheric pressure; PEF, peak expiratory flow; PH2O, partial pressure of H2O in inspired air; PIF, peak inspiratory flow; RQ, respiratory quotient; RT, relaxation time; Te, expiratory time; TFL, tail-flick latency; Ti, inspiratory time; TV, tidal volume; and VEH, vehicle.
References
Albrecht, J., and Zielińska, M. (2019). Exchange-mode Glutamine Transport across CNS Cell Membranes. Neuropharmacology 161, 107560. doi:10.1016/j.neuropharm.2019.03.003
Algera, M. H., Kamp, J., van der Schrier, R., van Velzen, M., Niesters, M., Aarts, L., et al. (2019). Opioid-induced Respiratory Depression in Humans: a Review of Pharmacokinetic-Pharmacodynamic Modelling of Reversal. Br. J. Anaesth. 122, e168–e179. doi:10.1016/j.bja.2018.12.023
Altawallbeh, G., Smith, L., Lewis, S. J., Authier, S., Bujold, K., Gaston, B., et al. (2019). Pharmacokinetic Study of Sudaxine in Dog Plasma Using Novel LC-MS/MS Method. Drug Test. Anal. 11, 403–410. doi:10.1002/dta.2507
Aoyama, K., Watabe, M., and Nakaki, T. (2008). Regulation of Neuronal Glutathione Synthesis. J. Pharmacol. Sci. 108, 227–238. doi:10.1254/jphs.08r01cr
Arendt, F. (2021). The Opioid-Overdose Crisis and Fentanyl: The Role of Online Information Seeking via Internet Search Engines. Health Commun. 36, 1148–1154. doi:10.1080/10410236.2020.1748820
Baby, S., Gruber, R., Discala, J., Puskovic, V., Jose, N., Cheng, F., et al. (2021a). Systemic Administration of Tempol Attenuates the Cardiorespiratory Depressant Effects of Fentanyl. Front. Pharmacol. 12, 690407. doi:10.3389/fphar.2021.690407
Baby, S. M., Discala, J. F., Gruber, R., Getsy, P. M., Cheng, F., Damron, D. S., et al. (2021b). Tempol Reverses the Negative Effects of Morphine on Arterial Blood-Gas Chemistry and Tissue Oxygen Saturation in Freely-Moving Rats. Front. Pharmacol. 12, 749084. doi:10.3389/fphar.2021.749084
Baby, S. M., Gruber, R. B., Young, A. P., MacFarlane, P. M., Teppema, L. J., and Lewis, S. J. (2018). Bilateral Carotid Sinus Nerve Transection Exacerbates Morphine-Induced Respiratory Depression. Eur. J. Pharmacol. 834, 17–29. doi:10.1016/j.ejphar.2018.07.018
Bachmutsky, I., Wei, X. P., Kish, E., and Yackle, K. (2020). Opioids Depress Breathing through Two Small Brainstem Sites. Elife 9, e52694. doi:10.7554/eLife.52694
Ben-Nun, A., Bashan, N., Potashnik, R., Cohen-Luria, R., and Moran, A. (1992). Cystine Dimethyl Ester Reduces the Forces Driving Sodium-dependent Transport in LLC-PK1 Cells. Am. J. Physiol. 263, C516–C520. doi:10.1152/ajpcell.1992.263.2.C516
Boom, M., Niesters, M., Sarton, E., Aarts, L., Smith, T. W., and Dahan, A. (2012). Non-analgesic Effects of Opioids: Opioid-Induced Respiratory Depression. Curr. Pharm. Des. 18, 5994–6004. doi:10.2174/138161212803582469
Carstens, E., and Wilson, C. (1993). Rat Tail Flick Reflex: Magnitude Measurement of Stimulus-Response Function, Suppression by Morphine and Habituation. J. Neurophysiol. 70, 630–639. doi:10.1152/jn.1993.70.2.630
Chapman, C. D., Dono, L. M., French, M. C., Weinberg, Z. Y., Schuette, L. M., and Currie, P. J. (2012). Paraventricular Nucleus Anandamide Signaling Alters Eating and Substrate Oxidation. Neuroreport 23, 425–429. doi:10.1097/WNR.0b013e32835271d1
Chen, Y., and Swanson, R. A. (2003). The Glutamate Transporters EAAT2 and EAAT3 Mediate Cysteine Uptake in Cortical Neuron Cultures. J. Neurochem. 84, 1332–1339. doi:10.1046/j.1471-4159.2003.01630.x
Chevillard, L., Mégarbane, B., Risède, P., and Baud, F. J. (2009). Characteristics and Comparative Severity of Respiratory Response to Toxic Doses of Fentanyl, Methadone, Morphine, and Buprenorphine in Rats. Toxicol. Lett. 191, 327–340. doi:10.1016/j.toxlet.2009.09.017
Chu, F., Chen, L. H., and O'Brian, C. A. (2004). Cellular Protein Kinase C Isozyme Regulation by Exogenously Delivered Physiological Disulfides-Iimplications of Oxidative Protein Kinase C Regulation to Cancer Prevention. Carcinogenesis 25, 585–596. doi:10.1093/carcin/bgh041
Clancy, R., Cederbaum, A. I., and Stoyanovsky, D. A. (2001). Preparation and Properties of S-Nitroso-L-Cysteine Ethyl Ester, an Intracellular Nitrosating Agent. J. Med. Chem. 44, 2035–2038. doi:10.1021/jm000463f
Connor, M., and Christie, M. D. (1999). Opioid Receptor Signalling Mechanisms. Clin. Exp. Pharmacol. Physiol. 26, 493–499. doi:10.1046/j.1440-1681.1999.03049.x
Dahan, A., Aarts, L., and Smith, T. W. (2010). Incidence, Reversal, and Prevention of Opioid-Induced Respiratory Depression. Anesthesiology 112, 226–238. doi:10.1097/ALN.0b013e3181c38c25
Dahan, A., Sarton, E., Teppema, L., and Olievier, C. (1998). Sex-related Differences in the Influence of Morphine on Ventilatory Control in Humans. Anesthesiology 88, 903–913. doi:10.1097/00000542-199804000-00009
Dahan, A., van der Schrier, R., Smith, T., Aarts, L., van Velzen, M., and Niesters, M. (2018). Averting Opioid-Induced Respiratory Depression without Affecting Analgesia. Anesthesiology 128, 1027–1037. doi:10.1097/ALN.0000000000002184
Davisson, R. L., Travis, M. D., Bates, J. N., Johnson, A. K., and Lewis, S. J. (1997). Stereoselective Actions of S-Nitrosocysteine in Central Nervous System of Conscious Rats. Am. J. Physiol. 272, H2361–H2368. doi:10.1152/ajpheart.1997.272.5.H2361
Davisson, R. L., Travis, M. D., Bates, J. N., and Lewis, S. J. (1996). Hemodynamic Effects of L- and D-S-Nitrosocysteine in the Rat. Stereoselective S-Nitrosothiol Recognition Sites. Circ. Res. 79, 256–262. doi:10.1161/01.res.79.2.256
Deo, V. S., Gilson, T. P., Kaspar, C., and Singer, M. E. (2021). The Fentanyl Phase of the Opioid Epidemic in Cuyahoga County, Ohio, United States. J. Forensic. Sci. 66, 926–933. doi:10.1111/1556-4029.14665
Eguchi, K., Tadaki, E., Simbulan, D., and Kumazawa, T. (1987). Respiratory Depression Caused by Either Morphine Microinjection or Repetitive Electrical Stimulation in the Region of the Nucleus Parabrachialis of Cats. Pflugers Arch. 409, 367–373. doi:10.1007/BF00583790
Epstein, M. A., and Epstein, R. A. (1978). A Theoretical Analysis of the Barometric Method for Measurement of Tidal Volume. Respir. Physiol. 32, 105–120. doi:10.1016/0034-5687(78)90103-2
Epstein, R. A., Epstein, M. A., Haddad, G. G., and Mellins, R. B. (1980). Practical Implementation of the Barometric Method for Measurement of Tidal Volume. J. Appl. Physiol. Respir. Environ. Exerc Physiol. 49, 1107–1115. doi:10.1152/jappl.1980.49.6.1107
Figueiredo, V. C., Feksa, L. R., and Wannmacher, C. M. (2009). Cysteamine Prevents Inhibition of Adenylate Kinase Caused by Cystine in Rat Brain Cortex. Metab. Brain Dis. 24, 373–381. doi:10.1007/s11011-009-9141-x10.1007/s11011-009-9142-9
Fone, K. C., and Wilson, H. (1986). The Effects of Alfentanil and Selected Narcotic Analgesics on the Rate of Action Potential Discharge of Medullary Respiratory Neurones in Anaesthetized Rats. Br. J. Pharmacol. 89, 67–76. doi:10.1111/j.1476-5381.1986.tb11121.x
Foreman, J. W., and Benson, L. (1990). Effect of Cystine Loading and Cystine Dimethylester on Renal Brushborder Membrane Transport. Biosci. Rep. 10, 455–459. doi:10.1007/BF01152292
Gaston, B., Baby, S. M., May, W. J., Young, A. P., Grossfield, A., Bates, J. N., et al. (2021). D-Cystine di(m)ethyl ester reverses the deleterious effects of morphine on ventilation and arterial blood gas chemistry while promoting antinociception. Sci. Rep. 11, 10038. doi:10.1038/s41598-021-89455-2
Gaston, B., May, W. J., Sullivan, S., Yemen, S., Marozkina, N. V., Palmer, L. A., et al. (2014). Essential Role of Hemoglobin Beta-93-Cysteine in Posthypoxia Facilitation of Breathing in Conscious Mice. J. Appl. Physiol. (1985) 116, 1290–1299. doi:10.1152/japplphysiol.01050.2013
Gaston, B., Smith, L., Bosch, J., Seckler, J., Kunze, D., Kiselar, J., et al. (2020). Voltage-gated Potassium Channel Proteins and Stereoselective S-Nitroso-L-Cysteine Signaling. JCI Insight 5, e134174. doi:10.1172/jci.insight.134174
Getsy, P. M., Coffee, G. A., and Lewis, S. J. (2020). The Role of Carotid Sinus Nerve Input in the Hypoxic-Hypercapnic Ventilatory Response in Juvenile Rats. Front. Physiol. 11, 613786. doi:10.3389/fphys.2020.613786
Getsy, P. M., Davis, J., Coffee, G. A., May, W. J., Palmer, L. A., Strohl, K. P., et al. (2014). Enhanced Non-eupneic Breathing Following Hypoxic, Hypercapnic or Hypoxic-Hypercapnic Gas Challenges in Conscious Mice. Respir. Physiol. Neurobiol. 204, 147–159. doi:10.1016/j.resp.2014.09.006
Getsy, P. M., Sundararajan, S., May, W. J., von Schill, G. C., McLaughlin, D. K., Palmer, L. A., et al. (2021). Short-term Facilitation of Breathing upon Cessation of Hypoxic Challenge Is Impaired in Male but Not Female Endothelial NOS Knock-Out Mice. Sci. Rep. 11, 18346. doi:10.1038/s41598-021-97322-3
Getsy, P. M., Young, A. P., Gaston, B., Bates, J. N., Baby, S. M., Seckler, J. M., et al. (2022). S-Nitroso-L-Cysteine Stereoselectively Blunts the Negative Effects of Morphine on Breathing and Arterial Blood Gas Chemistry while Promoting Analgesia. Front. Pharmacol. in press.
Golder, F. J., Dax, S., Baby, S. M., Gruber, R., Hoshi, T., Ideo, C., et al. (2015). Identification and Characterization of GAL-021 as a Novel Breathing Control Modulator. Anesthesiology 123, 1093–1104. doi:10.1097/ALN.0000000000000844
Gomez, M. R., Benzick, A. E., Rogers, L. K., Heird, W. C., and Smith, C. V. (1994). Attenuation of Acetaminophen Hepatotoxicity in Mice as Evidence for the Bioavailability of the Cysteine in D-Glucose-L-Cysteine In Vivo. Toxicol. Lett. 70, 101–108. doi:10.1016/0378-4274(94)90149-x
Granillo, O. M., Brahmajothi, M. V., Li, S., Whorton, A. R., Mason, S. N., McMahon, T. J., et al. (2008). Pulmonary Alveolar Epithelial Uptake of S-Nitrosothiols Is Regulated by L-type Amino Acid Transporter. Am. J. Physiol. Lung Cell Mol. Physiol. 295, L38–L43. doi:10.1152/ajplung.00280.2007
Grim, T. W., Acevedo-Canabal, A., and Bohn, L. M. (2020). Toward Directing Opioid Receptor Signaling to Refine Opioid Therapeutics. Biol. Psychiatry 87, 15–21. doi:10.1016/j.biopsych.2019.10.020
Gurbuz, N., Park, M. A., Dent, P., Abdel Mageed, A. B., Sikka, S. C., and Baykal, A. (2015). Cystine Dimethyl Ester Induces Apoptosis through Regulation of PKC-δ and PKC-ε in Prostate Cancer Cells. Anticancer Agents Med. Chem. 15, 217–227. doi:10.2174/1871520614666141120121901
Hamelmann, E., Schwarze, J., Takeda, K., Oshiba, A., Larsen, G. L., Irvin, C. G., et al. (1997). Noninvasive Measurement of Airway Responsiveness in Allergic Mice Using Barometric Plethysmography. Am. J. Respir. Crit. Care Med. 156, 766–775. doi:10.1164/ajrccm.156.3.9606031
Hargreaves, K., Dubner, R., Brown, F., Flores, C., and Joris, J. (1988). A New and Sensitive Method for Measuring Thermal Nociception in Cutaneous Hyperalgesia. Pain 32, 77–88. doi:10.1016/0304-3959(88)90026-7
Hassen, A. H., Feuerstein, G., Pfeiffer, A., and Faden, A. I. (1982). Delta versus Mu Receptors: Cardiovascular and Respiratory Effects of Opiate Agonists Microinjected into Nucleus Tractus Solitarius of Cats. Regul. Pept. 4, 299–309. doi:10.1016/0167-0115(82)90140-9
Hatfield, M. J., Umans, R. A., Hyatt, J. L., Edwards, C. C., Wierdl, M., Tsurkan, L., et al. (2016). Carboxylesterases: General Detoxifying Enzymes. Chem. Biol. Interact. 259, 327–331. doi:10.1016/j.cbi.2016.02.011
Henderson, F., May, W. J., Gruber, R. B., Discala, J. F., Puskovic, V., Young, A. P., et al. (2014). Role of Central and Peripheral Opiate Receptors in the Effects of Fentanyl on Analgesia, Ventilation and Arterial Blood-Gas Chemistry in Conscious Rats. Respir. Physiol. Neurobiol. 191, 95–105. doi:10.1016/j.resp.2013.11.005
Henderson, F., May, W. J., Gruber, R. B., Young, A. P., Palmer, L. A., Gaston, B., et al. (2013). Low-dose Morphine Elicits Ventilatory Excitant and Depressant Responses in Conscious Rats: Role of Peripheral μ-opioid Receptors. Open J. Mol. Integr. Physiol. 3, 111–124. doi:10.4236/ojmip.2013.33017
Henderson, M., Rice, B., Sebastian, A., Sullivan, P. G., King, C., Robinson, R. A., et al. (2016). Neuroproteomic Study of Nitrated Proteins in Moderate Traumatic Brain Injured Rats Treated with Gamma Glutamyl Cysteine Ethyl Ester Administration Post Injury: Insight into the Role of Glutathione Elevation in Nitrosative Stress. Proteomics Clin. Appl. 10, 1218–1224. doi:10.1002/prca.201600004
Hess, D. T., and Stamler, J. S. (2012). Regulation by S-Nitrosylation of Protein Post-translational Modification. J. Biol. Chem. 287, 4411–4418. doi:10.1074/jbc.R111.285742
Himi, T., Ikeda, M., Yasuhara, T., Nishida, M., and Morita, I. (2003). Role of Neuronal Glutamate Transporter in the Cysteine Uptake and Intracellular Glutathione Levels in Cultured Cortical Neurons. J. Neural Transm. (Vienna) 110, 1337–1348. doi:10.1007/s00702-003-0049-z
Hobbs, M. J., Butterworth, M., Cohen, G. M., and Upshall, D. G. (1993). Structure-activity Relationships of Cysteine Esters and Their Effects on Thiol Levels in Rat Lung In Vitro. Biochem. Pharmacol. 45, 1605–1612. doi:10.1016/0006-2952(93)90301-c
Hosseini, M., Taiarani, Z., Hadjzadeh, M. A., Salehabadi, S., Tehranipour, M., and Alaei, H. A. (2011). Different Responses of Nitric Oxide Synthase Inhibition on Morphine-Induced Antinociception in Male and Female Rats. Pathophysiology 18, 143–149. doi:10.1016/j.pathophys.2010.05.004
Iniushkin, A. N. (1997). Respiratory and Hemodynamic Responses to Microinjections of Opioids into the Solitary Tract Nucleus. Ross. Fiziol. Zh. Im. I. M.Sechenova. 83, 112–121.
Ito, L., Okumura, M., Tao, K., Kasai, Y., Tomita, S., Oosuka, A., et al. (2012). Glutathione Ethylester, a Novel Protein Refolding Reagent, Enhances Both the Efficiency of Refolding and Correct Disulfide Formation. Protein J. 31, 499–503. doi:10.1007/s10930-012-9427-4
Jenkins, M. W., Khalid, F., Baby, S. M., May, W. J., Young, A. P., Bates, J. N., et al. (2021). Glutathione Ethyl Ester Reverses the Deleterious Effects of Fentanyl on Ventilation and Arterial Blood-Gas Chemistry while Prolonging Fentanyl-Induced Analgesia. Sci. Rep. 11, 6985. doi:10.1038/s41598-021-86458-x
Kasaba, T., Takeshita, M., and Takasaki, M. (1997). The Effects of Caffeine on the Respiratory Depression by Morphine. Masui 46, 1570–1574.
Kimura, H. (2014). The Physiological Role of Hydrogen Sulfide and beyond. Nitric Oxide 41, 4–10. doi:10.1016/j.niox.2014.01.002
Lailey, A. F., Hill, L., Lawston, I. W., Stanton, D., and Upshall, D. G. (1991). Protection by Cysteine Esters against Chemically Induced Pulmonary Oedema. Biochem. Pharmacol. 42 (Suppl. l), S47–S54. doi:10.1016/0006-2952(91)90391-h
Lailey, A. F., and Upshall, D. G. (1994). Thiol Levels in Rat Bronchio-Alveolar Lavage Fluid after Administration of Cysteine Esters. Hum. Exp. Toxicol. 13, 776–780. doi:10.1177/096032719401301106
Lalley, P. M., Pilowsky, P. M., Forster, H. V., and Zuperku, E. J. (2014). CrossTalk Opposing View: the Pre-botzinger Complex Is Not Essential for Respiratory Depression Following Systemic Administration of Opioid Analgesics. J. Physiol. 592, 1163–1166. doi:10.1113/jphysiol.2013.258830
Lewis, S. J., Hoque, A., and Bates, J. N. (2005). Differentiation of L- and D-S-Nitrosothiol Recognition Sites In Vivo. J. Cardiovasc. Pharmacol. 46, 660–671. doi:10.1097/01.fjc.0000181714.94827.5d
Lewis, S. J., Owen, J. R., and Bates, J. N. (2006). S-nitrosocysteine Elicits Hemodynamic Responses Similar to Those of the Bezold-Jarisch Reflex via Activation of Stereoselective Recognition Sites. Eur. J. Pharmacol. 531, 254–258. doi:10.1016/j.ejphar.2005.11.027
Lewis, S. J., Travis, M. D., and Bates, J. N. (1996). Stereoselective S-Nitrosocysteine Recognition Sites in Rat Brain. Eur. J. Pharmacol. 312, R3–R5. doi:10.1016/0014-2999(96)00607-3
Lewis, S. J., Meller, S. T., Brody, M. J., and Gebhart, G. F. (1991). Reduced Nociceptive Effects of Intravenous Serotonin (5-HT) in the Spontaneously Hypertensive Rat. Clin. Exp. Hypertens. Part A Theory Pract. 13, 849–857. doi:10.3109/10641969109042089
Li, S., and Whorton, A. R. (2007). Functional Characterization of Two S-Nitroso-L-Cysteine Transporters, Which Mediate Movement of NO Equivalents into Vascular Cells. Am. J. Physiol. Cell Physiol. 292, C1263–C1271. doi:10.1152/ajpcell.00382.2006
Li, W. M., Sato, A., Sato, Y., and Schmidt, R. F. (1996). Morphine Microinjected into the Nucleus Tractus Solitarius and Rostral Ventrolateral Medullary Nucleus Enhances Somatosympathetic A- and C- Reflexes in Anesthetized Rats. Neurosci. Lett. 221, 53–56. doi:10.1016/s0304-3940(96)13286-9
Li, Y., Su, L., Li, F., Wang, C., Yuan, D., Chen, J., et al. (20152015). Acute and Sub-chronic Toxicity of Glucose-Cysteine Maillard Reaction Products in Sprague-Dawley Rats. Food Chem. Toxicol. 80, 271–276. doi:10.1016/j.fct.2015.03.021
Lipton, A. J., Johnson, M. A., Macdonald, T., Lieberman, M. W., Gozal, D., and Gaston, B. (2001). S-nitrosothiols Signal the Ventilatory Response to Hypoxia. Nature 413, 171–174. doi:10.1038/35093117
Lomask, M. (2006). Further Exploration of the Penh Parameter. Exp. Toxicol. Pathol. 5 (2), 13–20. doi:10.1016/j.etp.2006.02.014
Ludbrook, J. (1998). Multiple Comparison Procedures Updated. Clin. Exp. Pharmacol. Physiol. 25, 1032–1037. doi:10.1111/j.1440-1681.1998.tb02179.x
May, W. J., Gruber, R. B., Discala, J. F., Puskovic, V., Henderson, F., Palmer, L. A., et al. (2013b). Morphine Has Latent Deleterious Effects on the Ventilatory Responses to a Hypoxic Challenge. Open J. Mol. Integr. Physiol. 3, 166–180. doi:10.4236/ojmip.2013.34022
May, W. J., Henderson, F., Gruber, R. B., Discala, J. F., Young, A. P., Bates, J. N., et al. (2013a). Morphine Has Latent Deleterious Effects on the Ventilatory Responses to a Hypoxic-Hypercapnic Challenge. Open J. Mol. Integr. Physiol. 3, 134–145. doi:10.4236/ojmip.2013.33019
McHugh, M. L. (2011). Multiple Comparison Analysis Testing in ANOVA. Biochem. Med. Zagreb. 21, 203–209. doi:10.11613/bm.2011.029
Meller, S. T., Lewis, S. J., Brody, M. J., and Gebhart, G. F. (1991). The Peripheral Nociceptive Actions of Intravenously Administered 5-HT in the Rat Requires Dual Activation of Both 5-HT2 and 5-HT3 Receptor Subtypes. Brain Res. 561, 61–68. doi:10.1016/0006-8993(91)90749-l
Mendoza, J., Passafaro, R., Baby, S., Young, A. P., Bates, J. N., Gaston, B., et al. (2013). L-cysteine Ethyl Ester Reverses the Deleterious Effects of Morphine on, Arterial Blood-Gas Chemistry in Tracheotomized Rats. Respir. Physiol. Neurobiol. 189, 136–143. doi:10.1016/j.resp.2013.07.007
Nemoto, T., Shimma, N., Horie, S., Saito, T., Okuma, Y., Nomura, Y., et al. (2003). Involvement of the System L Amino Acid Transporter on Uptake of S-Nitroso-L-Cysteine, an Endogenous S-Nitrosothiol, in PC12 Cells. Eur. J. Pharmacol. 458, 17–24. doi:10.1016/s0014-2999(02)02699-7
Ohta, H., Bates, J. N., Lewis, S. J., and Talman, W. T. (1997). Actions of S-Nitrosocysteine in the Nucleus Tractus Solitarii Are Unrelated to Release of Nitric Oxide. Brain Res. 746, 98–104. doi:10.1016/s0006-8993(96)01188-2
Palmer, L. A., Kimberly deRonde, K., Brown-Steinke, K., Gunter, S., Jyothikumar, V., Forbes, M. S., et al. (2015). Hypoxia-induced Changes in Protein S-Nitrosylation in Female Mouse Brainstem. Am. J. Respir. Cell. Mol. Biol. 52, 37–45. doi:10.1165/rcmb.2013-0359OC
Palmer, L. A., May, W. J., deRonde, K., Brown-Steinke, K., Bates, J. N., Gaston, B., et al. (2013). Ventilatory Responses during and Following Exposure to a Hypoxic Challenge in Conscious Mice Deficient or Null in S-Nitrosoglutathione Reductase. Respir. Physiol. Neurobiol. 185, 571–581. doi:10.1016/j.resp.2012.11.009
Pathirathna, S., Covey, D. F., Todorovic, S. M., and Jevtovic-Todorovic, V. (2006). Differential Effects of Endogenous Cysteine Analogs on Peripheral Thermal Nociception in Intact Rats. Pain 125, 53–64. doi:10.1016/j.pain.2006.04.024
Paul, B. D., Sbodio, J. I., and Snyder, S. H. (2018). Cysteine Metabolism in Neuronal Redox Homeostasis. Trends Pharmacol. Sci. 39, 513–524. doi:10.1016/j.tips.2018.02.007
Perissinotti, L. L., Turjanski, A. G., Estrin, D. A., and Doctorovich, F. (20052005). Transnitrosation of Nitrosothiols: Characterization of an Elusive Intermediate. J. Am. Chem. Soc. 127 (2), 486–487. doi:10.1021/ja044056v
Quindry, J. C., Ballmann, C. G., Epstein, E. E., and Selsby, J. T. (2016). Plethysmography Measurements of Respiratory Function in Conscious Unrestrained Mice. J. Physiol. Sci. 66, 157–164. doi:10.1007/s12576-015-0408-1
Rech, V. C., Feksa, L. R., Fleck, R. M., Athaydes, G. A., Dornelles, P. K., Rodrigues-Junior, V., et al. (2008). Cysteamine Prevents Inhibition of Thiol-Containing Enzymes Caused by Cystine or Cystine Dimethylester Loading in Rat Brain Cortex. Metab. Brain Dis. 23, 133–145. doi:10.1007/s11011-008-9081-x
Ren, J., Ding, X., and Greer, J. J. (20152015). 5-HT1A Receptor Agonist Befiradol Reduces Fentanyl-Induced Respiratory Depression, Analgesia, and Sedation in Rats. Anesthesiology 122, 424–434. doi:10.1097/ALN.0000000000000490
Ren, J., Ding, X., and Greer, J. J. (2020). Countering Opioid-Induced Respiratory Depression in Male Rats with Nicotinic Acetylcholine Receptor Partial Agonists Varenicline and ABT 594. Anesthesiology 132, 1197–1211. doi:10.1097/ALN.0000000000003128
Satoh, T., and Hosokawa, M. (2006). Structure, Function and Regulation of Carboxylesterases. Chem. Biol. Interact. 162, 195–211. doi:10.1016/j.cbi.2006.07.001
Satoh, T., and Hosokawa, M. (1998). The Mammalian Carboxylesterases: from Molecules to Functions. Annu. Rev. Pharmacol. Toxicol. 38, 257–288. doi:10.1146/annurev.pharmtox.38.1.257
Schmid, C. L., Kennedy, N. M., Ross, N. C., Lovell, K. M., Yue, Z., Morgenweck, J., et al. (2017). Bias Factor and Therapeutic Window Correlate to Predict Safer Opioid Analgesics. Cell 171, 1165–e13. e13. doi:10.1016/j.cell.2017.10.035
Seckler, J. M., Grossfield, A., May, W. J., Getsy, P. M., and Lewis, S. J. (2022). Nitrosyl Factors Play a Vital Role in the Ventilatory Depressant Effects of Fentanyl in Unanesthetized Rats. Biomed. Pharmacother. 146, 112571. doi:10.1016/j.biopha.2021.112571
Seckler, J. M., and Lewis, S. J. (2020). Advances in D-Amino Acids in Neurological Research. Int. J. Mol. Sci. 21, 7325. doi:10.3390/ijms21197325
Seckler, J. M., Meyer, N. M., Burton, S. T., Bates, J. N., Gaston, B., and Lewis, S. J. (2017). Detection of Trace Concentrations of S-Nitrosothiols by Means of a Capacitive Sensor. PLoS One 12, e0187149. doi:10.1371/journal.pone.0187149
Seckler, J. M., Shen, J., Lewis, T. H. J., Abdulameer, M. A., Zaman, K., Palmer, L. A., et al. (2020). NADPH Diaphorase Detects S-Nitrosylated Proteins in Aldehyde-Treated Biological Tissues. Sci. Rep. 10, 21088. doi:10.1038/s41598-020-78107-6
Semenza, E. R., Harraz, M. M., Abramson, E., Malla, A. P., Vasavda, C., Gadalla, M. M., et al. (2021). D-cysteine Is an Endogenous Regulator of Neural Progenitor Cell Dynamics in the Mammalian Brain. Proc. Natl. Acad. Sci. U. S. A. 118, e2110610118. doi:10.1073/pnas.2110610118
Servin, A. L., Goulinet, S., and Renault, H. (1988). Pharmacokinetics of Cysteine Ethyl Ester in Rat. Xenobiotica 18, 839–847. doi:10.3109/00498258809041722
Shanker, G., and Aschner, M. (2001). Identification and Characterization of Uptake Systems for Cystine and Cysteine in Cultured Astrocytes and Neurons: Evidence for Methylmercury-Targeted Disruption of Astrocyte Transport. J. Neurosci. Res. 66, 998–1002. doi:10.1002/jnr.10066
Shibuya, N., and Kimura, H. (2013). Production of Hydrogen Sulfide from D-Cysteine and its Therapeutic Potential. Front. Endocrinol. (Lausanne) 4, 87. doi:10.3389/fendo.2013.00087
Simmons-Willis, T. A., Koh, A. S., Clarkson, T. W., and Ballatori, N. (2002). Transport of a Neurotoxicant by Molecular Mimicry: the Methylmercury-L-Cysteine Complex Is a Substrate for Human L-type Large Neutral Amino Acid Transporter (LAT) 1 and LAT2. Biochem. J. 367, 239–246. doi:10.1042/BJ20020841
Stein, P. D., Goldhaber, S. Z., and Henry, J. W. (1995). Alveolar-arterial Oxygen Gradient in the Assessment of Acute Pulmonary Embolism. Chest 107, 139–143. doi:10.1378/chest.107.1.139
Stengel, A., Coskun, T., Goebel, M., Wang, L., Craft, L., Alsina-Fernandez, J., et al. (2010). Central Injection of the Stable Somatostatin Analog ODT8-SST Induces a Somatostatin2 Receptor-Mediated Orexigenic Effect: Role of Neuropeptide Y and Opioid Signaling Pathways in Rats. Endocrinology 151, 4224–4235. doi:10.1210/en.2010-0195
Stomberski, C. T., Hess, D. T., and Stamler, J. S. (2019). Protein S-Nitrosylation: Determinants of Specificity and Enzymatic Regulation of S-Nitrosothiol-Based Signaling. Antioxid. Redox Signal. 30, 1331–1351. doi:10.1089/ars.2017.7403
Story, D. A. (1996). Alveolar Oxygen Partial Pressure, Alveolar Carbon Dioxide Partial Pressure, and the Alveolar Gas Equation. Anesthesiology 84, 1011. doi:10.1097/00000542-199604000-00036
Sumayao, R., McEvoy, B., Martin-Martin, N., McMorrow, T., and Newsholme, P. (2013). Cystine Dimethylester Loading Promotes Oxidative Stress and a Reduction in ATP Independent of Lysosomal Cystine Accumulation in a Human Proximal Tubular Epithelial Cell Line. Exp. Physiol. 98, 1505–1517. doi:10.1113/expphysiol.2013.073809
Szwergold, B. S. (2006). Alpha-thiolamines Such as Cysteine and Cysteamine Act as Effective Transglycating Agents Due to Formation of Irreversible Thiazolidine Derivatives. Med. Hypotheses 66, 698–707. doi:10.1016/j.mehy.2005.10.029
Todorovic, S. M., and Jevtovic-Todorovic, V. (2014). Redox Regulation of Neuronal Voltage-Gated Calcium Channels. Antioxid. Redox Signal. 21, 880–891. doi:10.1089/ars.2013.5610
Travis, M. D., Davisson, R. L., Bates, J. N., and Lewis, S. J. (1997). Hemodynamic Effects of L- and D-S-Nitroso-Beta,beta-Dimethylcysteine in Rats. Am. J. Physiol. 273, H1493–H1501. doi:10.1152/ajpheart.1997.273.3.H1493
Travis, M. D., Stoll, L. L., Bates, J. N., and Lewis, S. J. (1996). L- and D-S-Nitroso-Beta,beta-Dimethylcysteine Differentially Increase cGMP in Cultured Vascular Smooth Muscle Cells. Eur. J. Pharmacol. 318, 47–53. doi:10.1016/s0014-2999(96)00719-4
Trivedi, M., Shah, J., Hodgson, N., Byun, H. M., and Deth, R. (2014). Morphine Induces Redox-Based Changes in Global DNA Methylation and Retrotransposon Transcription by Inhibition of Excitatory Amino Acid Transporter Type 3-mediated Cysteine Uptake. Mol. Pharmacol. 85, 747–757. doi:10.1124/mol.114.091728
Trivedi, M. S., and Deth, R. (2015). Redox-based Epigenetic Status in Drug Addiction: a Potential Contributor to Gene Priming and a Mechanistic Rationale for Metabolic Intervention. Front. Neurosci. 8, 444. doi:10.3389/fnins.2014.00444
Tsikas, D., Schwedhelm, K. S., Surdacki, A., Giustarini, D., Rossi, R., Kukoc-Modun, L., et al. (2018). S-Nitroso-N-acetyl-L-cysteine Ethyl Ester (SNACET) and N-Acetyl-L-Cysteine Ethyl Ester (NACET)-Cysteine-based Drug Candidates with Unique Pharmacological Profiles for Oral Use as NO, H2S and GSH Suppliers and as Antioxidants: Results and Overview. J. Pharm. Anal. 8, 1–9. doi:10.1016/j.jpha.2017.12.003
Tsumuro, T., Alejandra Hossen, M., Kishi, Y., Fujii, Y., and Kamei, C. (2006). Nasal Congestion Model in Brown Norway Rats and the Effects of Some H1-Antagonists. Int. Immunopharmacol. 6, 759–763. doi:10.1016/j.intimp.2005.11.009
Tunnicliff, G. (1994). Amino Acid Transport by Human Erythrocyte Membranes. Comp. Biochem. Physiol. Comp. Physiol. 108, 471–478. doi:10.1016/0300-9629(94)90329-8
van Dorp, E., Yassen, A., and Dahan, A. (2007). Naloxone Treatment in Opioid Addiction: the Risks and Benefits. Expert Opin. Drug Saf. 6, 125–132. doi:10.1517/14740338.6.2.125
Varga, A. G., Reid, B. T., Kieffer, B. L., and Levitt, E. S. (2020). Differential Impact of Two Critical Respiratory Centres in Opioid-Induced Respiratory Depression in Awake Mice. J. Physiol. 598, 189–205. doi:10.1113/JP278612
Wallenstein, S., Zucker, C. L., and Fleiss, J. L. (1980). Some Statistical Methods Useful in Circulation Research. Circ. Res. 47, 1–9. doi:10.1161/01.res.47.1.1
Williams, F. M. (1985). Clinical Significance of Esterases in Man. Clin. Pharmacokinet. 10, 392–403. doi:10.2165/00003088-198510050-00002
Williams, J. T., Ingram, S. L., Henderson, G., Chavkin, C., von Zastrow, M., Schulz, S., et al. (2013). Regulation of μ-opioid Receptors: Desensitization, Phosphorylation, Internalization, and Tolerance. Pharmacol. Rev. 65, 223–254. doi:10.1124/pr.112.00594243
Wróbel, M., Ubuka, T., Yao, W. B., and Abe, T. (1997). Effect of Glucose-Cysteine Adduct on Cysteine Desulfuration in guinea Pig Tissues. Physiol. Chem. Phys. Med. NMR 29, 11–14.
Wu, G. (2009). Amino Acids: Metabolism, Functions, and Nutrition. Amino Acids 37, 1–17. doi:10.1007/s00726-009-0269-0
Yin, J., Ren, W., Yang, G., Duan, J., Huang, X., Fang, R., et al. (2016). L-cysteine Metabolism and its Nutritional Implications. Mol. Nutr. Food Res. 60, 134–146. doi:10.1002/mnfr.201500031
Young, A. P., Gruber, R. B., Discala, J. F., May, W. J., Palmer, L. A., and Lewis, S. J. (2013). Co-activation of μ- and δ-opioid Receptors Elicits Tolerance to Morphine-Induced Ventilatory Depression via Generation of Peroxynitrite. Resp. Physiol. Neurobiol. 186, 255–264. doi:10.1016/j.resp.2013.02.028
Young, J. D., Jones, S. E., and Ellory, J. C. (1981). Amino Acid Transport via the Red Cell Anion Transport System. Biochim. Biophys. Acta 645, 157–160. doi:10.1016/0005-2736(81)90524-1
Yu, C., Yuan, M., Yang, H., Zhuang, X., and Li, H. (2018). P-glycoprotein on Blood-Brain Barrier Plays a Vital Role in Fentanyl Brain Exposure and Respiratory Toxicity in Rats. Toxicol. Sci. 164, 353–362. doi:10.1093/toxsci/kfy093
Zerangue, N., and Kavanaugh, M. P. (1996). Interaction of L-Cysteine with a Human Excitatory Amino Acid Transporter. J. Physiol. 493 ( Pt 2), 419–423. doi:10.1113/jphysiol.1996.sp021393
Keywords: D-cysteine ethyl ester, thiol esters, morphine, respiratory depression, gas exchange, antinociception, sedation, Sprague Dawley rats
Citation: Getsy PM, Baby SM, May WJ, Young AP, Gaston B, Hodges MR, Forster HV, Bates JN, Wilson CG, Lewis THJ, Hsieh Y-H and Lewis SJ (2022) D-Cysteine Ethyl Ester Reverses the Deleterious Effects of Morphine on Breathing and Arterial Blood–Gas Chemistry in Freely-Moving Rats. Front. Pharmacol. 13:883329. doi: 10.3389/fphar.2022.883329
Received: 24 February 2022; Accepted: 09 May 2022;
Published: 23 June 2022.
Edited by:
Gregory D. Funk, University of Alberta, CanadaReviewed by:
Donatella Mutolo, University of Florence, ItalyKevin Yackle, University of California, San Francisco, United States
Copyright © 2022 Getsy, Baby, May, Young, Gaston, Hodges, Forster, Bates, Wilson, Lewis, Hsieh and Lewis. This is an open-access article distributed under the terms of the Creative Commons Attribution License (CC BY). The use, distribution or reproduction in other forums is permitted, provided the original author(s) and the copyright owner(s) are credited and that the original publication in this journal is cited, in accordance with accepted academic practice. No use, distribution or reproduction is permitted which does not comply with these terms.
*Correspondence: Paulina M. Getsy, cHhnNTVAY2FzZS5lZHU=
†Present address: Santhosh M. Baby, Translational Sciences Treatment Discovery, Galvani Bioelectronics, Inc., Collegeville, PA, United States