- 1Jiangsu Collaborative Innovation Center of Regional Modern Agriculture and Environmental Protection, School of Life Sciences, Huaiyin Normal University, Huaian, China
- 2School of Pharmaceutical Sciences, Institute for Chinese Materia Medica, Tsinghua University, Beijing, China
- 3Beijing Increasepharm Safety and Efficacy Co., Ltd, Beijing, China
- 4Institute of Applied Chemistry, Academy of Sciences, Nanchang, China
Ginsenoside Rh2 (G-Rh2), a rare protopanaxadiol (PPD)-type triterpene saponin, from Panax ginseng has anti-proliferation, anti-invasion, and anti-metastatic activity. However, the mechanisms by which G-Rh2 induces apoptosis of lung cancer cells are unclear. In the present work, a G-Rh2 target-lung cancer network was constructed and analyzed by the network pharmacology approach. A total of 91 compound-targets of G-Rh2 was obtained based on the compound-target network analysis, and 217 targets were identified for G-Rh2 against lung cancer by PPI network analysis. The 217 targets were significantly enriched in 103 GO terms with FDR <0.05 as threshold in the GO enrichment analysis. In KEGG pathway enrichment analysis, all the candidate targets were significantly enriched in 143 pathways, among of which PI3K-Akt signaling pathway was identified as one of the top enriched pathway. Besides, G-Rh2 induced apoptosis in human lung epithelial (A549) cells was verified in this work. G-Rh2 significantly inhibited the proliferation of A549 cells in a dose-dependent manner, and the apoptosis rate significantly increased from 4.4% to 78.7% using flow cytometry. Western blot analysis revealed that the phosphorylation levels of p85, PDK1, Akt and IκBα were significantly suppressed by G-Rh2. All the experimental findings were consistent with the network pharmacology results. Research findings in this work will provide potential therapeutic value for further mechanism investigations.
Introduction
Lung cancer is one of the most diagnosed cancers and a leading cause of cancer deaths. Smoking is the main cause (∼80% of cases) of lung cancer (Huang et al., 2021). Other causes are exposure to radon, secondhand smoke, and air pollution. In 2020, lung cancer was the second most common human cancer globally, there were 2,206,771 new cases (11.4% of all cases). The number of new lung cancer deaths was 17,966,144, accounting for 18% of all cancer deaths (Wild et al., 2020). Lung cancer can be divided into non-small cell lung cancer (NSCLC) and small cell lung cancer (SCLC) (Hanna and Onaitis, 2013). Treatment for lung cancer differs according to subtype and stage (Hayashi et al., 2011). Chemotherapy and radiotherapy have side effects (Albano et al., 2021), and even targeted immunotherapeutic have a considerable symptom burden (Lim et al., 2020). Therefore, research on new drugs and combined therapies is needed.
Ginseng, a traditional Chinese herb, has been used as medicine for thousands of years. It is used in cancer treatment and prevention based on its multi-target activity and low toxicity. Ginsenosides are the main active constituents in ginseng. G-Rh2, a 20 (S)-protopanaxadiol saponin extracted from the root of Panax ginseng (Wong et al., 2015), has been reported to show cytotoxic activity and decreased cancer cells viability via JAK2/STAT3 pathway in human colorectal cancer cells (Han et al., 2016), stimulates ROS production in human HeLa cervical cancer cell lines (Liu et al., 2021). G-Rh2 was also reported with antitumor effects in liver, lung, prostate, and colorectal cancer (Ge et al., 2017; Shi et al., 2017; Wu et al., 2018; Zhang et al., 2021). G-Rh2 inhibits proliferation, metastasis, and apoptosis by activating the mitochondrial or membrane death receptor (Wang et al., 2017). However, the molecular targets and signaling pathways underlying the effect of G-Rh2 on lung cancer are unclear. Cancer is typically caused by multiple genes and risk factors, so identification of multiple targets is important for understanding the mechanisms underlying the effect of G-Rh2 on lung cancer.
Network pharmacology is a systematic approach that integrates pharmacologic, computational, and experimental methods to illuminate the molecular mechanisms of drugs (Zhao and He, 2018; Song et al., 2019; Zhou et al., 2020). It can describe the complex pharmacological mechanisms of traditional Chinese medicines from a network perspective by multitarget, multichannel, and multilink analysis (Li et al., 2015; Park et al., 2018). In this work, we used the network pharmacology approach to evaluate the molecular mechanisms underlying the effect of G-Rh2 in lung cancer.
Materials and Methods
Materials and Reagents
G-Rh2 was purchased from Chengdu Phytoelite Bio-Technology Co., Ltd. (Chengdu, Sichuan, China) and the purity (>98%) was determined by high-performance liquid chromatography (Figure 1). 3-(4,5-Dimethylthiazol-2-yl)-2,5-diphenyltetrazolium bromide (MTT) and Annexin V-FITC apoptosis detection kit were purchased from Sigma-Alorich (St. Louis, MO, United States). The fetal bovine serum (FBS) was purchased from Corning (Medford, MA, United States). Antibiotics (100× penicillin/streptomycin) and 0.25% Trypsin-EDTA were purchased from Gibco (California, United States). Minimum essential medium (MEM) was purchased from Hyclone (Logan, UT, United States). RIPA Lysis Buffer and eECL Western Blot kit were purchased from CWBio (Taizhou, Jiangsu, China). The primary antibodies against p-AKT, AKT, p-PDK1, PDK1, p-p85, p85, p-IκBα, IκBα, and anti-rabbit IgG HRP (#7074) were from Cell Signaling Technology (Danvers, MA, United States).
Potential Targets Screening
The chemical structure of G-Rh2 was imported into PharmMapper server (http://www.lilab-ecust.cn/pharmmapper/, version 2017) (Xia et al., 2017), STITCH database http://stitch.embl.de/, version 5.0) (Szklarczyk et al., 2016), SwissTargetPrediction (http://www.swisstargetprediction.ch/) (David et al., 2014), and Similarity ensemble approach (http://sea.bkslab.org/) (Keiser et al., 2007) to obtained the related targets of G-Rh2.
Lung Cancer-Related Targets Screening
The potential targets of Lung cancer were identified by the Genetic Association Database (https://geneticassociationdb.nih.gov/), which is a database of genetic association data from complex diseases and disorders. Lung cancer was imported as a keyword and the disease targets associated with it were provided in the database.
Network Construction
In order to explore the relationship between G-Rh2 related targets and lung cancer disease related targets, protein-protein interaction (PPI) were analysed by the Database of Interacting Proteins (DIPTM), Biological General Repository for Interaction Datasets (BioGRID), Human Protein Reference Database (HPRD), IntAct Molecular Interaction Database (IntAct), Molecular INTeraction database (MINT), and biomolecular interaction network database (BIND) using the plug-in Bisogenet (Martin et al., 2010) of Cytoscape 3.7.1 software. The PPI networks of G-Rh2 putative targets and lung cancer-related targets were established and visualized by the plug-in Bisogenet of Cytoscape 3.7.1 software.
Bioinformatic Analysis
GO analysis with the biological process, cellular component, and molecular function was carried out using the Database for Annotation, Visualization and Integrated Discovery (DAVID, https://david.ncifcrf.gov/, v6.8) (Huang et al., 2009). Functional categories were enriched within genes (FDR <0.005) and the top 10 GO functional categories were selected. KOBAS 3.0 (http://kobas.cbi.pku.edu.cn/) that assigned Kyoto Encyclopedia of Genes and Genomes (KEGG) database was used for pathway analysis (Xie et al., 2011). The significantly changed pathways which corrected p value <0.005 were selected and genes regulated these pathways were enriched by gene-pathway network analysis. The gene-pathway network was constructed to screen the key target genes for G-Rh2 against lung cancer.
Cell Line and Cell Culture
The A549 lung cancer cell line was purchased from the China Center for Type Culture Collection (Wuhan, Hubei, China) and cultured in MEM supplemented with 10% FBS, 100 U/mL penicillin and 100 μg/ml streptomycin at 37°C in a humidified atmosphere with 5% CO2.
Cell Viability Assay
The viability of A549 cells was measured by MTT assay. Briefly, cells were seeded into 96-well plates at 2 × 104 cells/well. After adhered to the plates for overnight, cells were treated with different concentrations of G-Rh2 for 24 and 48 h. The medium was then removed and supplemented with 100 µL MTT solution for 4 h, following with 100 µL stopping buffer. The absorbance was determined at 550 nm using a microplate reader (Tecan Infinite M200 Pro, Männedorf, Switzerland).
Cell Apoptosis Assays
The apoptosis ratio of cells was analyzed by Annexin V-FITC Apoptosis Detection Kit according to the instruction of the manufacturer. In brief, the cells treated with different concentration of G-Rh2 for 24 h were harvested and centrifuged at 1,500 rpm for 3 min to remove the medium. The precipitation was then resuspended in 100 µL 1 × binding buffer. Subsequently, the cells were stained with Annexin-FITC and PI for 15 min in the dark. After added 400 µL 1 × binding buffer, the samples were evaluated by a Accuri C6 Plus flow cytometer (Becton, Dickinson and Company, CA, United States).
Western Blot Analysis
A total of 1 × 106 A549 cells were seeded into 40 mm Petri dish and grown for overnight. Then the cells were treated with G-Rh2 for different time points. After collection, the cells were lysed by RIPA lysis buffer and the protein content was determined by Bradford reagent, using bovine serum albumin (BSA) as a standard. Total protein were separated by 20% SDS-polyacrylamide gels for 1 h at 100 V, transferred to PVDF membranes, and blocked with 5% BSA in Tris-buffered saline containing Tween 20 (1 × TTBS) for 2 h. After washed with 1 × TTBS, the PVDF membranes were then incubated with primary antibodies (1:500–1:2000) in 5% BSA at 4°C for overnight, followed by washing and incubated for 1 h with HRP-conjugated secondary anti-IgG (1:500). The bands were then visualized by the eECL Kit and photographed using Tanon 5200 Multi imaging system (Tanon, China).
Statistical Analysis
The results have been represented as the mean ± SD. Variances among two groups were analyzed by Student’s t-test. Data analysis was completed using SPSS 20.0 (SPSS Inc., Chicago, IL, United States). p < 0.05 indicated significant differences.
Results and Discussion
Compound-Target Network Analysis
We evaluated the ability of G-Rh2 to inhibit the viability and induce apoptosis of A549 cells using a network pharmacology approach. The compound-target network was created by one approach. The network of G-Rh2 and its targets from PharmMapper server, SwissTargetPrediction, a similarity ensemble approach, and the STITCH database was constructed as shown in Figure 2. Ninety-one targets were obtained, among of which, 70 targets were obtained from PharmMapper server, 14 targets from SwissTargetPrediction, 5 from the similarity ensemble approach, and 2 from the STITCH database. After removing the duplicates, A total of 91 compound-targets was obtained.
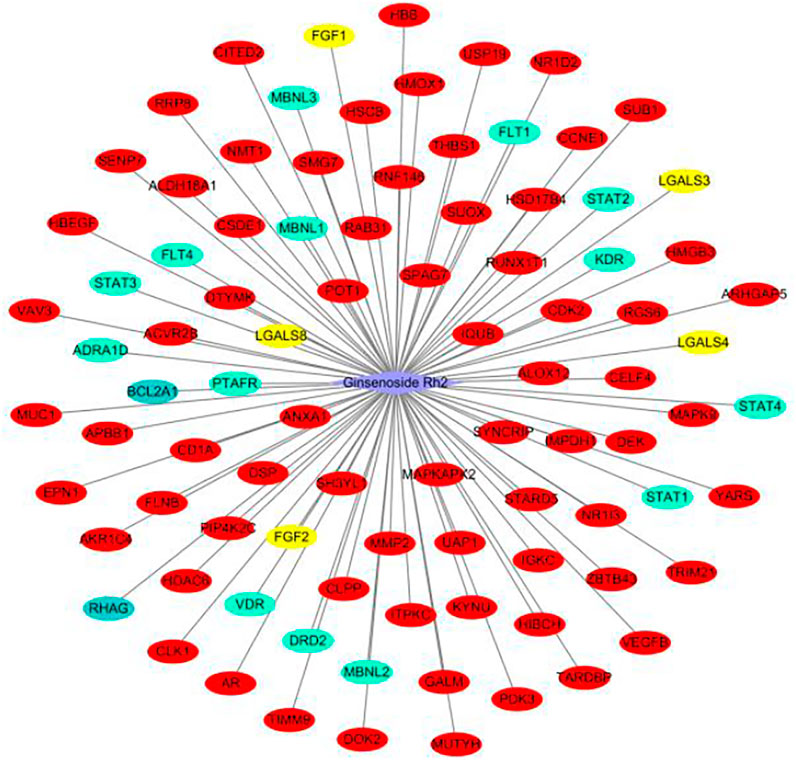
FIGURE 2. Target network of G-Rh2. Red, yellow, green, and blue ovals, are targets obtained from PharmMapper Server, Similarity ensemble approach, SwissTargetPrediction, and the STITCH database.
Identification of Targets for G-Rh2
Biological networked systems include functional units or protein complexes in PPI networks and disease- or drug-target genes can be analyzed according to complex network theory (Peng et al., 2018). PPIs are important in the regulation of biological systems and are the targets of an increasing number of drugs (Scott et al., 2016). PPI networks of G-Rh2 putative targets and lung cancer-related targets were structured with PPI data. The PPI network of G-Rh2 putative targets contained 3444 nodes and 86990 edges, which represented 3444 interacting proteins and 86990 interactions (Figure 3A). The PPI network of lung cancer-related targets contained 7493 interacting proteins and 179815 interactions (Figure 3B).
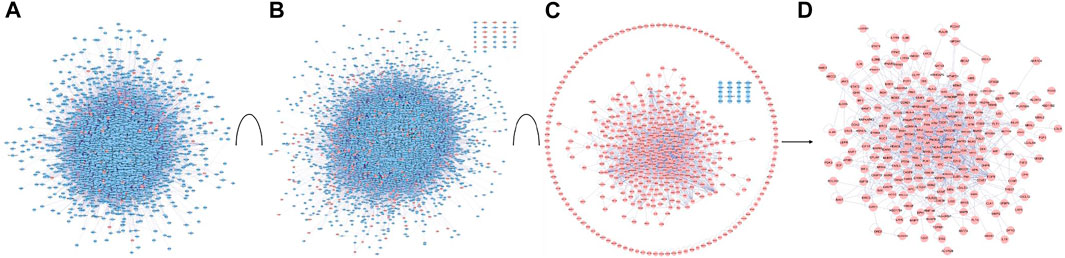
FIGURE 3. PPI network construction and analysis for targets of G-Rh2 in lung cancer. (A) PPI network of G-Rh2 putative targets; (B) PPI network of lung cancer-related targets; (C) Interaction network of G-Rh2 targets and lung cancer-related targets; (D) Merged PPI network.
The interaction network of G-Rh2 comprised 91 putative targets of G-Rh2 and 431 lung cancer-related targets. The interaction network encompassed 512 interacting proteins and 1,589 interactions (Figure 3C). The structured interaction network was merged with the PPI networks of G-Rh2 putative targets and lung cancer-related targets to identify targets for G-Rh2 in lung cancer. The new network had 217 nodes and 1,028 edges (Figure 3D); thus, 217 targets for G-Rh2 in lung cancer were identified.
GO and KEGG Pathway Enrichment Analysis
DAVID was used to carry out GO analysis to elucidate the function of 217 candidate targets in biological process, cellular component, and molecular function. One hundred and three GO terms with FDR <0.05 were significantly enriched: 73 in biological process, 11 in cellular component, and 19 in molecular function. The top 10 GO terms enriched in each sub-ontology are shown in Figure 4. Regulation of apoptotic process, regulation of transcription, nucleoplasm, nucleus, protein binding, and damaged DNA binding were the highly enriched GO terms. Anticancer agents activate several pathways simultaneously, positively or negatively regulating the death process (Solary et al., 2000). Regulation of apoptosis is the mechanism by which most chemotherapeutic drugs induce tumor cell death. Genetic alterations induce cancer and always result in dysregulated transcriptional programs. Almost every DNA, RNA, and protein component controlled by normal transcription is influenced by recurrent somatic mutations in tumor cells (Bradner et al., 2017). DNA binding of a new compound is an important aspect of its therapeutic potential for anticancer (Thangavel et al., 2018). Because of reversible binding or formation of covalent bonds with deoxyribonucleic acid, small DNA-interacting anticancer drugs abrogate the interaction between DNA and transcription factors in gene promoters (José, 2018). Therefore, G-Rh2 may reduce A549 cell viability by intervening in biological processes and affecting cellular components and molecular functions.
The KEGG pathway analysis was performed by KOBAS and 143 significantly enriched pathways (corrected p < 0.005) including pathways in cancer, the PI3K-Akt signaling pathway, proteoglycans in cancer, focal adhesion, the Jak-STAT signaling pathway, the FoxO signaling pathway, and apoptosis were obtained. Figure 5 shows the top 20 enriched pathways. The most significantly enriched pathways were related to pathways in cancer, followed by the PI3K-Akt signaling pathway. The PI3K-Akt signaling pathway is crucial in the development of many types of tumors (Lorusso, 2016; Zhang et al., 2017). Cell proliferation, growth, cell cycle, apoptosis, and protein synthesis are regulated by the PI3K-Akt signaling pathway (Zhu et al., 2018). Also, activation of the PI3K-Akt signaling pathway promotes cancer cell proliferation, survival, and angiogenesis. The PI3K-Akt signaling pathway is activated in cancer and is a potential therapeutic target (Chen et al., 2017). Xie et al. indicated that the PI3K-Akt signaling pathway is important in lung cancer and found that ginsenoside Rg3 promoted apoptosis by inhibiting the ratio of p-PI3K/PI3K and p-Akt/Akt in A549 cells (Xie et al., 2017). Our results suggest that G-Rh2 inhibits the viability of A549 cells by inducing apoptosis via the PI3K-Akt signaling pathway. In addition, the Wnt/β-catenin, mTOR, VEGF, EGFR, and metabolic signaling pathways are important in lung cancer (Cho, 2013).
Gene-Pathway Network Analysis
The significantly enriched pathways and genes were used to construct a gene-pathway network. As shown in Figure 6, 143 pathways and 191 genes were identified. Betweenness centrality (BC) was used to carry out the topological analysis. In the network, metabolic pathways had the highest BC, followed by pathways in cancer, the neurotrophin signaling pathway, HTLV-1 infection, and the PI3K-Akt signaling pathway. PIK3CA had the highest BC and several other genes including IRS1, AKT1, NFKB1, MAPK9, and POLD1 had larger BC. PIK3CA is one of the most commonly mutated oncogenes in human cancer and is used in the development of PI3 kinase inhibitors, which can be used as targeted therapies for cancers with these mutations (Jelovac et al., 2014). The PI3K signaling pathway is activated in cancers and is concerned with oncogenesis and cancer progression (Hennessy et al., 2005). PIK3CA mutations have been focused on as potential biomarkers of PI3K pathway activation (Ito et al., 2017). Activation of mutations and genomic amplification of the PIK3CA gene are closely related to increased PI3K activity in lung cancer (Yamamoto et al., 2008). Somatic mutations in the IA PI3K catalytic subunit p110α, encoded by PIK3CA, activate the PI3K signaling pathway (Sawa et al., 2017). IRS1 regulates many cancer-cell processes and PI3K within malignant cells (Houghton et al., 2010; Porter et al., 2013). A549 cell survival, proliferation, malignancy, and metastasis are suppressed by Akt1 knockdown (Jere et al., 2009). Also, constitutional activation of the PI3K-Akt signaling pathway can be caused by oncogenic mutations in the AKT1 gene, increasing the malignant potential of the affected cells. Our results indicate that G-Rh2 induces A549-cell apoptosis mainly by regulating the expression of PIK3CA, thus inhibiting activation of the PI3K-Akt signaling pathway. Also, regulation of IRS1, AKT1, NFKB1, MAPK9, and POLD1 may explain the G-Rh2-mediated inhibition of the viability of A549 cells.
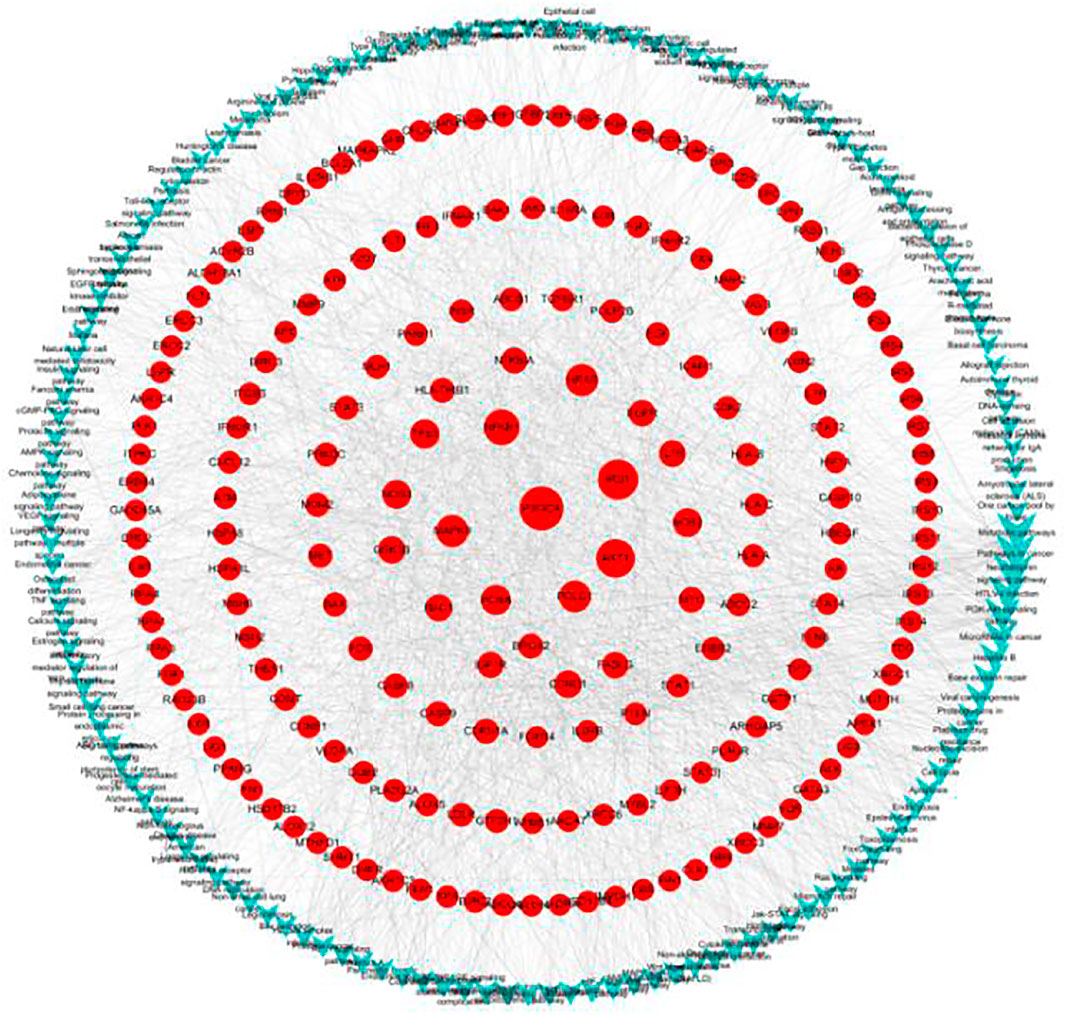
FIGURE 6. Gene-pathway network for G-Rh2 in lung cancer. Red circles, target genes; blue V-shapes, pathways. Size is proportional to betweenness centrality.
G-Rh2 Inhibits A549 Cell Viability and Promotes Apoptosis
G-Rh2 suppresses cell proliferation, causes G1-phase arrest, enhances the activity of capase-3, and induces apoptosis in A549 cells (An et al., 2013). To validate the anti-proliferation effect of G-Rh2 on A549 cells, a MTT assay was carried out. A549 cells were treated with G-Rh2 at different concentrations for 24 h or 48 h. As shown in Figure 7, G-Rh2 significantly inhibited A549 cells proliferation in a dose-dependent manner. Based on the results of MTT assay, IC50 of G-Rh2 on A549 cells at 24 and 48 h were calculated respectively as 42.75 and 36.25 µM which was consist with previous report (Zhang et al., 2011).
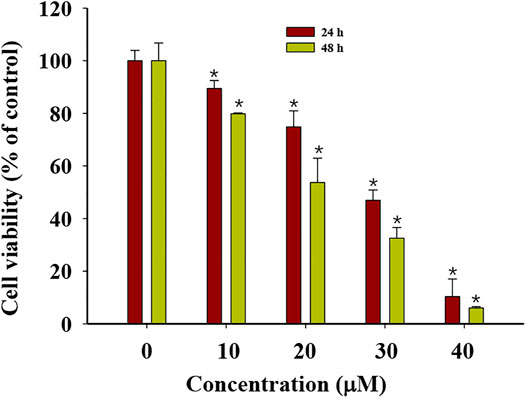
FIGURE 7. Cytotoxicity of G-Rh2 to A549 cells. Cells were treated with G-Rh2 for 24 and 48 h and viability was evaluated by MTT assay. Values are means ± SD (n = 3).
Apoptosis, an orderly process of programmed cell death, is central to development of cancers. It involves of the activation, expression and regulation of a series of genes, and inhibits the growth of tumor cells. Apoptosis may not the main way for the death of many cancers response to common treatments (Brown and Attardi, 2005). G-Rh2 were reported to induces apoptosis of many cancer cells, such as human epidermoid carcinoma A431cells (Park et al., 2010), human malignant melanoma A375-S2 cells (Fei et al., 2002), and hepatocellular carcinoma HepG2 cells (Zhang et al., 2019). In order to determine whether G-Rh2 can regulates A549 cell death by inducing apoptosis in this study, A549 cells treated with 10, 20, and 40 μM G-Rh2 were stained by Annexin-PI based on a flow cytometry. As shown in Figure 8, the apoptosis rate increased significantly from 4.4% in the control group to 15.92, 23.72, and 78.7% at 10, 20, and 40 μM G-Rh2, respectively. It is indicate that G-Rh2 can significantly induce A549 cells apoptosis to regulate this lung cancer cell death, and is consistent with literatures (Cheng et al., 2005; Wang et al., 2018).
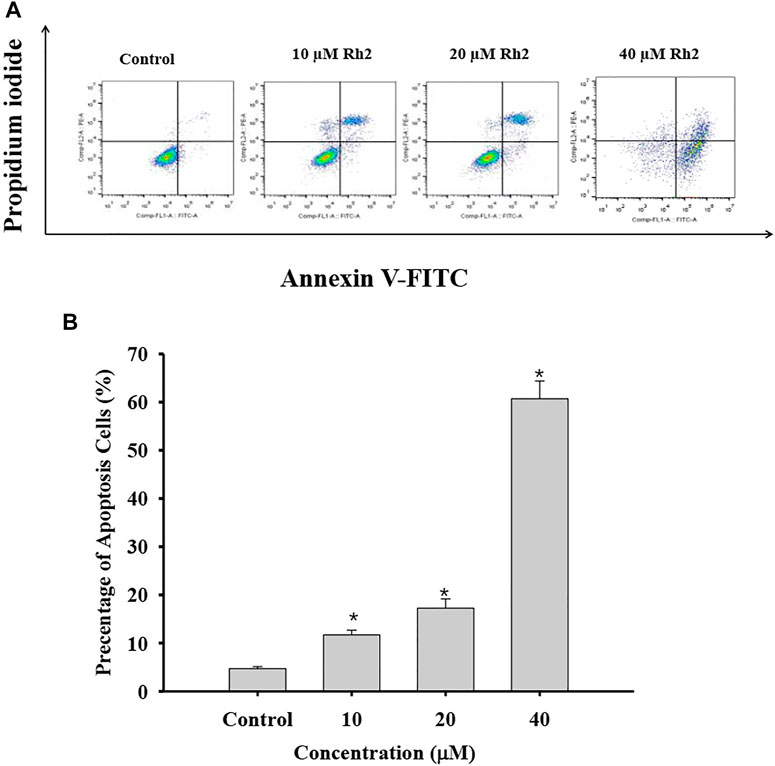
FIGURE 8. Effect of G-Rh2 on apoptosis of A549 cells. (A) Cells were treated with G-Rh2, and the apoptotic rate was determined by Annexin V-FITC/PI staining. (B) Percentage of apoptotic cells, Values are means ± SD (n = 3).
The PI3K/Akt signaling pathway operates by phosphorylation and dephosphorylation of the substrate-level (Sang and Li, 2007; Yu et al., 2021). PI3K, phosphoinositide 3-kinase, a lipid kinase family protein, which was constructed by two subunit including a regulatory subunit (P85) and a catalytic subunit (P110). When the growth factors binds to their receptor tyrosine kinase (RTK) or G protein-coupled receptors (GPCR), PI3K isoforms were stimulated and catalyzes the production of phosphatidylinositol-3,4,5-triphosphate (PIP3) at the cell membrane. PIP3, serves as a secondary messenger, is in turn to help the phosphorylation and activation of PDK1 and AKT. AKT is a serine/threonine kinase, once activated, it controls key cellular processes including the inhibition of apoptosis (Liu et al., 2009), and promotes cell survival by activating CREB and NF-κB activity (Park et al., 2010). Therefore, PI3K/Akt as a most commonly activated signaling pathway in human cancer, presents both an opportunity and a challenge for cancer therapy (Liu et al., 2009). In the present study, G-Rh2 was confirmed to down regulate the phosphorylation of P85, PDK1, Akt and IκBα in A549 cells by western blot analyses (Figure 9). The inactivation of P85, PDK1 and Akt by G-Rh2 treatment indicating that G-Rh2 can induces cancer cells to apoptosis via inhibiting the PI3K/Akt signaling pathway. Similarly, the inactivation of IκBα means G-Rh2 suppress the cell survival via inhibiting the NF-κB signaling pathway and to induces cancer cells death. These results are consistent with that deduced from network pharmacology analyses.
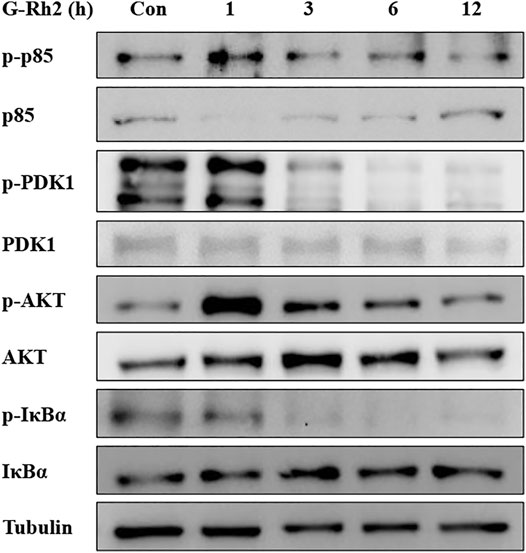
FIGURE 9. Effects of G-Rh2 on apoptosis-related proteins and their phosphorylation levels in A549 cells. Cells were treated with G-Rh2 for 0, 1, 3, 6, and 12 h. The protein levels of p85/p-p85, PDK1/p-PDK1, Akt/p-Akt and IκBa/p-IκBa were measured by Western blotting.
Conclusion
The potential targets and signaling pathways of G-Rh2 on human lung cancer were predicted in this work by network pharmacology approach. According to the network pharmacology analyses results, 217 genes/proteins were predicted as potential targets for G-Rh2. Besides, bioinformatics analysis on the predicted targets revealed that over 140 pathways, of which PI3K-Akt signaling pathway is top signaling pathway involved in the underlying mechanisms of G-Rh2. Confirmatory experiments showed that G-Rh2 inhibits proliferation of human lung cancer A549 cells, and induces cells apoptosis via inhibiting the PI3K-Akt signaling pathway. It is consistent to the predicted results in the network pharmacology analyses.
Data Availability Statement
The raw data supporting the conclusion of this article will be made available by the authors, without undue reservation.
Author Contributions
LW and JZ contributed to design of the study. CS and JZ carried out the network pharmacology analyses. ZH, YH and YX performed the experiments. JZ, CS and JZ wrote and the manuscript. YY, NL, and CS contributed to manuscript revision. All authors read and approved the submitted version.
Conflict of Interest
Author NL is employed by Beijing Increasepharm Safety and Efficacy Co., Ltd.
The remaining authors declare that the research was conducted in the absence of any commercial or financial relationships that could be construed as a potential conflict of interest.
Publisher’s Note
All claims expressed in this article are solely those of the authors and do not necessarily represent those of their affiliated organizations, or those of the publisher, the editors and the reviewers. Any product that may be evaluated in this article, or claim that may be made by its manufacturer, is not guaranteed or endorsed by the publisher.
References
Albano, D., Benenati, M., Bruno, A., Bruno, F., Calandri, M., Caruso, D., et al. (2021). Imaging Side Effects and Complications of Chemotherapy and Radiation Therapy: a Pictorial Review from Head to Toe. Insights Imaging 12, 1–28. doi:10.1186/s13244-021-01017-2
An, I. S., An, S., Kwon, K. J., Kim, Y. J., and Bae, S. (2013). Ginsenoside Rh2 Mediates Changes in the microRNA Expression Profile of Human Non-small Cell Lung Cancer A549 Cells. Oncol. Rep. 29 (2), 523–528. doi:10.3892/or.2012.2136
Bradner, J. E., Hnisz, D., and Young, R. A. (2017). Transcriptional Addiction in Cancer. Cell. 168 (4), 629–643. doi:10.1016/j.cell.2016.12.013
Brown, J. M., and Attardi, L. D. (2005). The Role of Apoptosis in Cancer Development and Treatment Response. Nat. Rev. Cancer 5, 231–237. doi:10.1038/nrc1560
Chen, Q., Duan, X., Fan, H., Xu, M., Tang, Q., Zhang, L., et al. (2017). Oxymatrine Protects against DSS-Induced Colitis via Inhibiting the PI3K/AKT Signaling Pathway. Int. Immunopharmacol. 53, 149–157. doi:10.1016/j.intimp.2017.10.025
Cheng, C. C., Yang, S. M., Huang, C. Y., Chen, J. C., Chang, W. M., and Hsu, S. L. (2005). Molecular Mechanisms of Ginsenoside Rh2-Mediated G1 Growth Arrest and Apoptosis in Human Lung Adenocarcinoma A549 Cells. Cancer Chemother. Pharmacol. 55, 531–540. doi:10.1007/s00280-004-0919-6
Cho, W. C. (2013). Targeting Signaling Pathways in Lung Cancer Therapy. Expert Opin. Ther. Targets 17 (2), 107–111. doi:10.1517/14728222.2013.729043
David, G., Aurélien, G., Matthias, W., Antoine, D., Olivier, M., and Vincent, Z. (2014). Swisstargetprediction: a Web Server for Target Prediction of Bioactive Small Molecules. Nucleic Acids Res. 42 (Web Server issue), W32–W38. doi:10.1093/nar/gku293
Fei, X. F., Wang, B. X., Tashiro, S., Li, T. J., Ma, J. S., and Ikejima, T. (2002). Apoptotic Effects of Ginsenoside Rh2 on Human Malignant Melanoma A375-S2 Cells. Acta Pharmacol. Sin. 23 (4), 315–322. doi:10.1016/S0300-483X(02)00008-2
Ge, G., Yan, Y., and Cai, H. (2017). Ginsenoside Rh2 Inhibited Proliferation by Inducing ROS Mediated ER Stress Dependent Apoptosis in Lung Cancer Cells. Biol. Pharm. Bull. 40, 2117–2124. doi:10.1248/bpb.b17-00463
Han, S., Jeong, A. J., Yang, H., Bin Kang, K., Lee, H., Yi, E. H., et al. (2016). Ginsenoside 20(S)-Rh2 Exerts Anti-cancer Activity through Targeting IL-6-induced JAK2/STAT3 Pathway in Human Colorectal Cancer Cells. J. Ethnopharmacol. 194, 83–90. doi:10.1016/j.jep.2016.08.039
Hanna, J. M., and Onaitis, M. W. (2013). Cell of Origin of Lung Cancer. J. Carcinog. 12, 6–12. doi:10.4103/1477-3163.109033
Hayashi, H., Kurata, T., and Nakagawa, K. (2011). Gemcitabine: Efficacy in the Treatment of Advanced Stage Nonsquamous Non-small Cell Lung Cancer. Clin. Med. Insights Oncol. 5, 177–184. doi:10.4137/CMO.S6252
Hennessy, B. T., Smith, D. L., RamDebra, P. T., LuPrahlad, Y., and Mills, G. B. (2005). Exploiting the PI3K/AKT Pathway for Cancer Drug Discovery. Nat. Rev. Drug Discov. 4 (12), 988–1004. doi:10.1038/nrd1902
Houghton, A. M., Rzymkiewicz, D. M., Ji, H., Gregory, A. D., Egea, E. E., Metz, H. E., et al. (2010). Neutrophil Elastase-Mediated Degradation of IRS-1 Accelerates Lung Tumor Growth. Nat. Med. 16 (2), 219–223. doi:10.1038/nm.2084
Huang, da. W., Sherman, B. T., and Lempicki, R. A. (2009). Systematic and Integrative Analysis of Large Gene Lists Using David Bioinformatics Resources. Nat. Protoc. 4 (1), 44–57. doi:10.1038/nprot.2008.211
Huang, Q. M., Liu, Y. H., and Suzuki, M. (2021). Role of POLD4 in Smoking- Induced Lung Cancer. J. Mod. Oncol. 29 (24), 4271–4275. doi:10.3969/j.issn.1672-4992.2021.24.002
Ito, C., Nishizuka, S. S., Ishida, K., Uesugi, N., Sugai, T., Tamura, G., et al. (2017). Analysis of PIK3CA Mutations and PI3K Pathway Proteins in Advanced Gastric Cancer. J. Surg. Res. 212, 195–204. doi:10.1016/j.jss.2017.01.018
Jelovac, D., Beaver, J. A., Balukrishna, S., Wong, H. Y., Toro, P. V., Cimino-Mathews, A., et al. (2014). A PIK3CA Mutation Detected in Plasma from a Patient with Synchronous Primary Breast and Lung Cancers. Hum. Pathol. 45 (4), 880–883. doi:10.1016/j.humpath.2013.10.016
Jere, D., Jiang, H. L., Kim, Y. K., Arote, R., Choi, Y. J., Yun, C. H., et al. (2009). Chitosan-graft-polyethylenimine for Akt1 siRNA Delivery to Lung Cancer Cells. Int. J. Pharm. 378 (1-2), 194–200. doi:10.1016/j.ijpharm.2009.05.046
José, P. (2018). Challenging Transcription by Dna-Binding Antitumor Drugs. Biochem. Pharmacol. 155, 336–345. doi:10.1016/j.bcp.2018.07.030
Keiser, M. J., Roth, B. L., Armbruster, B. N., Ernsberger, P., Irwin, J. J., and Shoichet, B. K. (2007). Relating Protein Pharmacology by Ligand Chemistry. Nat. Biotechnol. 25 (2), 197–206. doi:10.1038/nbt1284
Li, Y., Wang, J., Xiao, Y., Wang, Y., Chen, S., Yang, Y., et al. (2015). A Systems Pharmacology Approach to Investigate the Mechanisms of Action of Semen Strychni and Tripterygium Wilfordii Hook F for Treatment of Rheumatoid Arthritis. J. Ethnopharmacol. 175, 301–314. doi:10.1016/j.jep.2015.09.016
Lim, S. M., Hong, M. H., and Kim, H. R. (2020). Immunotherapy for Non-small Cell Lung Cancer: Current Landscape and Future Perspectives. Immune Netw. 20, e10–14. doi:10.4110/in.2020.20.e10
Liu, P., Cheng, H., Roberts, T. M., and Zhao, J. J. (2009). Targeting the Phosphoinositide 3-kinase Pathway in Cancer. Nat. Rev. Drug Discov. 8, 627–644. doi:10.1038/nrd2926
Liu, Y., Yu, S., Xing, X., Qiao, J., Yin, Y., Wang, J., et al. (2021). Ginsenoside Rh2 Stimulates the Production of Mitochondrial Reactive Oxygen Species and Induces Apoptosis of Cervical Cancer Cells by Inhibiting Mitochondrial Electron Transfer Chain Complex. Mol. Med. Rep. 24 (6), 873. doi:10.3892/mmr.2021.12513
Lorusso, P. M. (2016). Inhibition of the Pi3k/akt/mtor Pathway in Solid Tumors. J. Clin. Oncol. 34 (31), 3803–3815. doi:10.1200/JCO.2014.59.0018
Martin, A., Ochagavia, M. E., Rabasa, L. C., Miranda, J., Fernandez-de-Cossio, J., and Bringas, R. (2010). BisoGenet: A New Tool for Gene Network Building, Visualization and Analysis. BMC Bioinformatics 11, 91. doi:10.1186/1471-2105-11-91
Park, E. K., Lee, E. J., Lee, S. H., Koo, K. H., Sung, J. Y., Hwang, E. H., et al. (2010). Induction of Apoptosis by the Ginsenoside Rh2 by Internalization of Lipid Rafts and Caveolae and Inactivation of Akt. Br. J. Pharmacol. 160, 1212–1223. doi:10.1111/j.1476-5381.2010.00768.x
Park, S. Y., Park, J. H., Kim, H. S., Lee, C. Y., Lee, H. J., Kang, K. S., et al. (2018). Systems-level Mechanisms of Action of Panax Ginseng: a Network Pharmacological Approach. J. Ginseng Res. 42 (1), 98–106. doi:10.1016/j.jgr.2017.09.001
Peng, G. S., Yi, N. Q., Qi, G. M., and Chi, J. (2018). Identifying Influential Genes in Protein–Protein Interaction Networks. Inf. Sci. (454-455), 229–241. doi:10.1016/j.ins.2018.04.078
Porter, H. A., Perry, A., Kingsley, C., Tran, N. L., and Keegan, A. D. (2013). IRS1 Is Highly Expressed in Localized Breast Tumors and Regulates the Sensitivity of Breast Cancer Cells to Chemotherapy, while IRS2 Is Highly Expressed in Invasive Breast Tumors. Cancer Lett. 338 (2), 239–248. doi:10.1016/j.canlet.2013.03.030
Sang, C. L., and Li, Q. (2007). Research Progress of NF-Κb and its Relationship with Lung Cancer. Chin. J. Pract. Intern. Med. 27 (16), 1313–1315. doi:10.3969/j.issn.1005-2194.2007.16.029
Sawa, K., Koh, Y., Kawaguchi, T., Kambayashi, S., Asai, K., Mitsuoka, S., et al. (2017). PIK3CA Mutation as a Distinctive Genetic Feature of Non-small Cell Lung Cancer with Chronic Obstructive Pulmonary Disease: a Comprehensive Mutational Analysis from a Multi-Institutional Cohort. Lung Cancer 112, 96–101. doi:10.1016/j.lungcan.2017.07.039
Scott, D. E., Bayly, A. R., Abell, C., and Skidmore, J. (2016). Small Molecules, Big Targets: Drug Discovery Faces the Protein-Protein Interaction Challenge. Nat. Rev. Drug Discov. 15 (8), 533–550. doi:10.1038/nrd.2016.29
Shi, X., Jing, L. I., Ran, J., Xiong, W., Haixing, L. I., Guo, P., et al. (2017). Ginsenoside Rh2 Induced Human Colorectal Cancer Cell Apoptosis through PI3K/AKT/GSK-3βpathway. Chin. Pharmacol. Bull. 33 (1), 114–119. doi:10.3969/j.issn.1001-1978.2017.01.020
Solary, E., Droin, N., Bettaieb, A., Corcos, L., Dimanche-Boitrel, M. T., and Garrido, C. (2000). Positive and Negative Regulation of Apoptotic Pathways by Cytotoxic Agents in Hematological Malignancies. Leukemia 14 (10), 1833–1849. doi:10.1038/sj.leu.2401902
Song, X., Zhang, Y., Dai, E., Du, H., and Wang, L. (2019). Mechanism of Action of Celastrol against Rheumatoid Arthritis: A Network Pharmacology Analysis. Int. Immunopharmacol. 74, 105725. doi:10.1016/j.intimp.2019.105725
Szklarczyk, D., Santos, A., von Mering, C., Jensen, L. J., Bork, P., and Kuhn, M. (2016). STITCH 5: Augmenting Protein-Chemical Interaction Networks with Tissue and Affinity Data. Nucleic Acids Res. 44 (Database issue), D380–D384. doi:10.1093/nar/gkv1277
Thangavel, T., Sparkes, H. A., and Karuppannan, N. (2018). Quinoline Based Pd(II) Complexes: Synthesis, Characterization and Evaluation of Dna/protein Binding, Molecular Docking and In Vitro Anticancer Activity. Inorg. Chim. Acta. 482, 229–239. doi:10.1016/j.ica.2018.06.003
Tong-Lin Wu, T., Tong, Y. C., Chen, I. H., Niu, H. S., Li, Y., and Cheng, J. T. (2018). Induction of Apoptosis in Prostate Cancer by Ginsenoside Rh2. Oncotarget 9 (13), 11109–11118. doi:10.18632/oncotarget.24326
Wang, Y., Xu, H., Lu, Z., Yu, X., Lv, C., Tian, Y., et al. (2018). Pseudo-ginsenoside Rh2 Induces A549 Cells Apoptosis via the Ras/Raf/ERK/p53 Pathway. Exp. Ther. Med. 15, 4916–4924. doi:10.3892/etm.2018.6067
Wang, Y. S., Lin, Y., Li, H., Li, Y., Song, Z., and Jin, Y. H. (2017). The Identification of Molecular Target of (20S) Ginsenoside Rh2 for its Anti-cancer Activity. Sci. Rep. 7 (1), 12408–12412. doi:10.1038/s41598-017-12572-4
Wild, C., Weiderpass, E., and Stewart, B. W. (2020). World Cancer Report: Cancer Research for Cancer Prevention. Lyon, France: IARC Press.
Wong, A. S., Che, C. M., and Leung, K. W. (2015). Recent Advances in Ginseng as Cancer Therapeutics: a Functional and Mechanistic Overview. Nat. Prod. Rep. 32 (2), 256–272. doi:10.1039/c4np00080c
Xia, W., Yihang, S., Shiwei, W., Shiliang, L., Weilin, Z., and Xiaofeng, L. E. A. (2017). Pharmmapper 2017 Update: a Web Server for Potential Drug Target Identification with a Comprehensive Target Pharmacophore Database. Nucleic Acids Res. 45 (W1), W356–W360. doi:10.1093/nar/gkx374
Xie, C., Mao, X., Huang, J., Ding, Y., Wu, J., Dong, S., et al. (2011). KOBAS 2.0: a Web Server for Annotation and Identification of Enriched Pathways and Diseases. Nucleic Acids Res. 39 (Suppl. l_2), W316–W322. doi:10.1093/nar/gkr483
Xie, Q., Wen, H., Zhang, Q., Zhou, W., Lin, X., Xie, D., et al. (2017). Inhibiting PI3K-AKt Signaling Pathway Is Involved in Antitumor Effects of Ginsenoside Rg3 in Lung Cancer Cell. Biomed. Pharmacother. 85, 16–21. doi:10.1016/j.biopha.2016.11.096
Yamamoto, H., Shigematsu, H., Nomura, M., Lockwood, W. W., Sato, M., Okumura, N., et al. (2008). PIK3CA Mutations and Copy Number Gains in Human Lung Cancers. Cancer Res. 68 (17), 6913–6921. doi:10.1158/0008-5472.CAN-07-5084
Yu, T., Yu, W. J., and Wang, H. Y. (2021). Research Progress on the Role of PI3K/AKT Signaling Pathway in Non-small Cell Lung Cancer. Chin. J. Clin. Res. 34 (02), 248–250+254. doi:10.13429/j.cnki.cjcr.2021.02.026
Zhang, C., Yu, H., and Hou, J. (2011). [Effects of 20 (S) -ginsenoside Rh2 and 20 (R) -ginsenoside Rh2 on Proliferation and Apoptosis of Human Lung Adenocarcinoma A549 Cells]. Zhongguo Zhong Yao Za Zhi 36 (12), 1670–1674. doi:10.4268/cjcmm20111228
Zhang, H., Song, P., Huang, H., Kim, E., and Kim, M. (2021). Anticancer Effects and Potential Mechanisms of Ginsenoside Rh2 in Various Cancer Types (Review). Oncol. Rep. 45 (4), 1–10. doi:10.3892/or.2021.7984
Zhang, J., Li, W., Yuan, Q., Zhou, J., Zhang, J., Cao, Y., et al. (2019). Transcriptome Analyses of the Anti-proliferative Effects of 20(S)-Ginsenoside Rh2 on HepG2 Cells. Front. Pharmacol. 10, 1331. doi:10.3389/fphar.2019.01331
Zhang, Y., Kwok-Shing Ng, P., Kucherlapati, M., Chen, F., Liu, Y., Tsang, Y. H., et al. (2017). A Pan-Cancer Proteogenomic Atlas of PI3K/AKT/mTOR Pathway Alterations. Cancer Cell. 31 (6), 820–e3. doi:10.1016/j.ccell.2017.04.013
Zhao, R. L., and He, Y. M. (2018). Network Pharmacology Analysis of the Anti-cancer Pharmacological Mechanisms of Ganoderma Lucidum Extract with Experimental Support Using Hepa1-6-Bearing C57 BL/6 Mice. J. Ethnopharmacol. 210, 287–295. doi:10.1016/j.jep.2017.08.041
Zhou, Z., Chen, B., Chen, S., Lin, M., Chen, Y., Jin, S., et al. (2020). Applications of Network Pharmacology in Traditional Chinese Medicine Research. Evid. Based Complement. Altern. Med. 2020 (6), 1646905–1646907. doi:10.1155/2020/1646905
Keywords: ginsenoside Rh2, network pharmacology, lung cancer, A549 cells, PI3K-Akt signaling pathway
Citation: Song C, Yuan Y, Zhou J, He Z, Hu Y, Xie Y, Liu N, Wu L and Zhang J (2022) Network Pharmacology-Based Prediction and Verification of Ginsenoside Rh2-Induced Apoptosis of A549 Cells via the PI3K/Akt Pathway. Front. Pharmacol. 13:878937. doi: 10.3389/fphar.2022.878937
Received: 18 February 2022; Accepted: 20 April 2022;
Published: 04 May 2022.
Edited by:
Bing Chun Yan, Yangzhou University, ChinaReviewed by:
Rongjie Zhao, Qiqihar Medical University, ChinaSun Eun Choi, Kangwon National University, South Korea
Copyright © 2022 Song, Yuan, Zhou, He, Hu, Xie, Liu, Wu and Zhang. This is an open-access article distributed under the terms of the Creative Commons Attribution License (CC BY). The use, distribution or reproduction in other forums is permitted, provided the original author(s) and the copyright owner(s) are credited and that the original publication in this journal is cited, in accordance with accepted academic practice. No use, distribution or reproduction is permitted which does not comply with these terms.
*Correspondence: Nan Liu, bmFubGl1MDMwNEAxNjMuY29t; Lei Wu, d3VsZWk4NTgxOTZAMTYzLmNvbQ==; Ji Zhang, emhhbmdqaUBoeXRjLmVkdS5jbg==
†These authors have contributed equally to this work and share first authorship