- 1Department of Cardiology, Daping Hospital, Third Military Medical University, Chongqing, China
- 2Chongqing Key Laboratory for Hypertension Research, Chongqing Cardiovascular Clinical Research Center, Chongqing Institute of Cardiology, Chongqing, China
- 3State Key Laboratory of Trauma, Burns and Combined Injury, Daping Hospital, Army Medical University, Chongqing, China
- 4Cardiovascular Research Center of Chongqing College, Chinese Academy of Sciences, University of Chinese Academy of Sciences, Chongqing, China
- 5Department of Cardiology, Chongqing General Hospital, Chongqing, China
- 6Heart Center of Fujian Province, Union Hospital, Fujian Medical University, Fuzhou, China
Pulmonary ischemia/reperfusion (IR) injury is the leading cause of acute lung injury, which is mainly attributed to reactive oxygen species (ROS) induced cell injuries and apoptosis. Since rosmarinic acid (RA) has been identified as an antioxidant natural ester, this natural compound might protect against pulmonary IR injury. In this study, the mice were given RA daily (50, 75, or 100 mg/kg) by gavage for 7 days before the pulmonary IR injury. We found that hypoxemia, pulmonary edema, and serum inflammation cytokines were aggravated in pulmonary IR injury. RA pretreatment (75 and 100 mg/kg) effectively reversed these parameters, while 50 mg/kg RA pretreatment was less pronounced. Our data also indicated RA pretreatment mitigated the upregulation of pro-oxidant NADPH oxidases (NOX2 and NOX4) and the downregulation of anti-oxidant superoxide dismutases (SOD1 and SOD2) upon IR injury. In vitro studies showed RA preserved the viability of anoxia/reoxygenation (AR)-treated A549 cells (a human lung epithelial cell line), and the results showed the protective effect of RA started at 5 μM concentration, reached its maximum at 15 μM, and gradually decreased at 20–25 μM. Besides, RA pretreatment (15 μM) greatly reduced the lactate dehydrogenase release levels subjected to AR treatment. Moreover, the results of our research revealed that RA eliminated ROS production and reduced alveolar epithelial cell apoptosis through activating the phosphatidylinositol 3 kinase (PI3K)/protein kinase B (Akt) signaling pathway, which was supported by using wortmannin, because in the presence of wortmannin, the RA-mediated protection was blocked. Meanwhile, wortmannin also reversed the protective effects of RA in mice. Together, our results demonstrate the beneficial role of RA in pulmonary IR injury via PI3K/Akt-mediated anti-oxidation and anti-apoptosis, which could be a promising therapeutic intervention for pulmonary IR injury.
Introduction
Acute lung injury is a common and severe disease with an incidence rate of 86.2 per 100,000 person-years and a mortality rate of 40% (Johnson and Matthay, 2010). Pulmonary ischemia/reperfusion (IR) injury is a pathological process observed in several clinical conditions, including infarction, thrombolytic therapy, and cardiopulmonary bypass (Eltzschig and Eckle, 2011), which is characterized by the injury of pulmonary alveolar epithelium and vascular endothelium due to reactive oxygen species (ROS) accumulation and inflammation during blood flow restoration (Oshima et al., 2016). The therapy of pulmonary IR damage mostly consists of nitric oxide inhalation, administration of prostaglandin 1, and complement inhibition as anti-inflammatory treatments, and their effectiveness has been proven in clinical studies (Aoe et al., 1994; Bacha et al., 1996; Zamora et al., 1999). In terms of scavenging oxidative stress, N-acetylcysteine was found to be effective in alleviating oxidative stress and improving the survival rate in transplant patients in a phase II clinical research (D’Amico et al., 2013). Meanwhile, other antioxidants, including methylene blue, melatonin, and creatine, have also been shown to attenuate pulmonary IR injury (Inci et al., 2002; Abreu et al., 2014; Almeida et al., 2016). However, those methods are not widely used due to their limited clinic effects or obvious side effects.
Rosmarinic acid (RA), as a common ester, exists in a variety of medicinal species within the plant genus Lamiaceae, such as basil, sage, rosemary, mint, and Perilla frutescens (Takano et al., 2004). Meanwhile, RA possesses the most potent antioxidant activity in hydroxycinnamic acids, and is commonly used to treat diseases such as peptic ulcers, arthritis, cataracts, cancer, rheumatoid arthritis, and bronchial asthma in clinics (Soobrattee et al., 2005; Petersen, 2013). Many physiological actions of RA, including anti-oxidation and anti-inflammation, have previously been described (Rašković et al., 2014). For instance, studies have shown that RA protects the heart, liver, and brain against IR injury through its antioxidant activity (Ramalho et al., 2014; Han et al., 2017; Zhang et al., 2017). It has been reported that RA could directly scavenge free radicals through the catechol hydroxyl group, and also play an indirect role by regulating the content of NADPH oxidases (NOX) and superoxide dismutases (SOD) (Imai et al., 2019; Liang et al., 2020). Thus, RA possesses the potential to protect the lung against IR injury through its antioxidant activity.
However, this possibility and the mechanism need further investigation. In the current study, we aimed to investigate the function and mechanism of RA in pulmonary IR injury. We provided evidence that RA could protect the lung against IR injury by reducing oxidative damage, inhibiting cell apoptosis and alleviating inflammation, which were regulated by the phosphatidylinositol 3 kinase (PI3K)/protein kinase B (Akt) signaling pathway. This study also provides a potential treatment or prevention drug for pulmonary IR injury.
Materials and Methods
Materials
RA was obtained from Aladdin, Shanghai, China. Schwartz Micro Serrefines were purchased from FineScienceTools, Germany. Kits for measurement of interleukin-1β (IL-1β), interleukin-6 (IL-6), lactate dehydrogenase (LDH), malondialdehyde (MDA), SOD, and NOX were from the Nanjing Jiancheng Bioengineering Institute, Nanjing, China. Wortmannin, AKT inhibitor VIII, hematoxylin and eosin (H&E) staining kit, Western and IP cell lysates, BCA protein assay kit, Dihydroethidium (DHE) kit, and Cell Counting Kit-8 (CCK-8) kit were obtained from Beyotime, Jiangsu, China. Protease inhibitor cocktails, and 4′, 6-diamino-2-phenyl-indole (DAPI) were obtained from Solarbio, Beijing, China. Nitro-cellulose membranes were purchased from BioTrace NT Nitrocellulose, PALL, United States. The In Situ Cell Death Detection Kit, POD was purchased from Roche, Mannheim, Germany. Rabbit anti-active caspase-3 antibody, rabbit anti-PI3K antibody, rabbit anti-p-PI3K antibody, rabbit anti-p-AKT antibody, and rabbit anti-BCL-2 antibody were from Cell Signaling Technology, Danvers, MA, United States. Rabbit anti-AKT antibody, rabbit anti-NOX2 antibody, rabbit anti-NOX4 antibody, rabbit anti-BAX antibody, and mouse anti-GAPDH antibody were obtained from ProteinTech, Wuhan, China. Rabbit anti-SOD1 antibody, and rabbit anti-SOD2 antibody were from Abcam, Cambridge, United Kingdom. Fluorescent-labeled goat anti-rabbit IgG, and fluorescent-labeled goat anti-mouse IgG were obtained from LI-COR Biotechnology, Lincoln, Nebraska, NE, United States.
Animals
C57BL/6J male mice aged 10–12 weeks (weight 20–25 g) were obtained from the Experimental Animal Center of Daping Hospital (Chongqing, China). Mice were kept at room temperature (∼23°C) under a standard 12-h light/dark cycle.
Animal handling and surgical procedures were carried out in accordance with local institutional regulations and approved by the Experimental Animal Committee of Daping Hospital. All procedures were approved by the Research Council and Animal Care and the Use Committee of Daping Hospital, the Third Military Medical University (ethical approval number: AMUWEC20213011).
Induction of Pulmonary Ischemia/Reperfusion Injury Model and Experimental Groups
The mouse model of pulmonary IR injury was carried out in accordance with the published protocol (Chen et al., 2017). Briefly, mice were anesthetized with an intraperitoneal injection of sodium pentobarbital (50 mg/kg) and placed on a heating pad to maintain body temperature. After tracheotomy, mice were ventilated with the Harvard ventilator (Hugo Sachs Elektronik) at a tidal ventilation of 7 ml/kg. The respiratory rate was set at around 100 breaths/min. After thoracotomy, lung ischemia was induced by clamping the left lung hilum using a Schwartz Micro Serrefine for 1 h, followed by 1 h of reperfusion. Subsequently, blood samples were immediately collected from the left ventricle for arterial blood gas analysis (Gem primer 3000, Instrumentation Laboratory). The oxygenation index (OI) was calculated by the ratio of partial pressure of oxygen (PaO2) to fraction of inspired oxygen (FiO2) (Xu et al., 2020; Wang et al., 2021). Meanwhile, other blood samples were placed at room temperature for 2 h. Then the blood samples were centrifuged for 3,000 rpm (30 min) to collect serum for enzyme-linked immunosorbent assay measurement of IL-1β and IL-6. Meanwhile, the left lungs were harvested and kept for further analysis.
Adult male mice were randomly subdivided into four groups: saline, RA 50 mg/kg, RA 75 mg/kg, RA 100 mg/kg, with 12 mice in each group. Then the mice were randomly divided into two parts in each group, one received sham operation, the other received pulmonary IR operation. According to the preliminary experiment, the optimal administration dose was 75 mg/kg in vivo (Joardar et al., 2019; Quan et al., 2021). Thereafter, the mice were randomly divided into four groups: Sham + Saline, Sham + RA 75 mg/kg, IR + Saline, and IR + RA 75 mg/kg, with six mice in each group (Lan et al., 2017). Furthermore, we used the PI3K inhibitor Wort (wortmannin, 1 mg/kg, intraperitoneally, 30 min before operation) in the pulmonary IR model (Yang et al., 2013; Liu et al., 2018b). Mice were divided into six groups: Sham + Saline, Sham + Wort, IR + Saline, IR + Wort, IR + RA 75 mg/kg, and IR + RA 75 mg/kg + Wort. In the Sham + RA 75 mg/kg, IR + RA 75 mg/kg, and IR + RA 75 mg/kg + Wort groups, mice were pretreated with RA (i.g.) for 7 days, and the same quantity of solvent was added to the sham or IR groups as RA 75 mg/kg pretreatment groups (Cao et al., 2019; Joardar et al., 2019).
Cell Culture and Anoxia/Reoxygenation Treatment
A549, a lung epithelial cell line, was obtained from Procell Life Science & Technology in Wuhan and cultured in Dulbecco’s modified Eagle’s medium/high glucose with 10% FBS. Besides, RLE-6TN, a rat lung alveolar type II epithelial cell line, was cultured in Ham’s F-12 medium with 2 mM L-glutamine supplemented with bovine pituitary extract (0.01 mg/ml), insulin (0.005 mg/ml), insulin-like growth factor (2.5 ng/ml), transferrin (1.25 μg/ml), epidermal growth factor (2.5 ng/ml), and 10% FBS. The two cell lines were cultured in 1% v/v penicillin/streptomycin (Beyotime, Jiangsu, China) at 37°C.
After pretreatment with RA for 24 h (or saline as control), the cells were exposed to an anoxic chamber with 5% CO2 and 95% N2 at 37°C for 2 h followed by reoxygenation for up to 2 h (Jia et al., 2014; Chen et al., 2017). For inhibiting the PI3K/Akt signaling pathway, the PI3K inhibitor (wortmannin, 1 μM) or AKT inhibitor (AKT inhibitor VIII, 10 μM) was given to cells after replacing the medium with RA and incubated for 30 min before anoxia/reoxygenation (AR) (Xu et al., 2008; Liu et al., 2020). Cells were divided into three groups: Control + Saline, AR + Saline, and AR + RA to explore the effect of RA subjected to AR treatment, and then divided into four groups: Control + Saline, AR + Saline, AR + RA, and AR + RA + Wort to investigate the role of RA in PI3K/Akt-mediated protection.
Wet/Dry Lung Weight Ratio Determination
The left lungs of different groups were weighed immediately after being removed to determine the wet weight, and then placed in an oven at 80°C for 24 h and re-weighed as dry weight to calculate the wet/dry lung weight ratio.
Histological Analysis
The left lung samples were fixed in 4% paraformaldehyde for 1–2 days at 4°C and then dehydrated in ethanol. Thereafter, the samples were clarified in xylene and embedded in paraffin. Subsequently, samples were cut into 4-μm-thick sections and stained with an H&E staining kit, and the images were captured by the software viewpoint M8 Digital Scanning Microscopy System (PreciPoint, Freising, Germany). Subsequently, an experienced histologist from the Department of Virology of Daping Hospital scored on a four-point scale (Takıl et al., 2003) under blind conditions. Each section was randomly selected 10 microscope fields (×400) for histological analysis.
Western Blot Analysis
Protein samples were extracted from lung tissues or A549 cells with Western and IP cell lysates containing protease inhibitor cocktails. The whole protein samples were collected and the concentrations were measured using a BCA protein assay kit. Tissue homogenates (50 mg of protein) were separated by 10% SDS-PAGE and transferred onto nitro-cellulose membranes. The blots were then washed with tris-buffered saline with Tween 20 (TBST), blocked with 5% milk powder in TBST buffer for 1 h, and incubated with the appropriate primary antibodies at appropriate dilutions. The blotted membranes were probed with the rabbit anti-active caspase-3 antibody (1:1,000), the rabbit anti-PI3K antibody (1:1,000), rabbit anti-p-PI3K antibody (1:1,000), rabbit anti-AKT antibody (1:1,000), rabbit anti-p-AKT antibody (1:1,000), rabbit anti-NOX2 antibody (1:500), rabbit anti-NOX4 antibody (1:500), rabbit anti-SOD1 antibody (1:1,000), rabbit anti-SOD2 antibody (1:1,000), rabbit anti-BAX antibody (1:1,000), rabbit anti-BCL-2 antibody (1:1,000), mouse anti-GAPDH antibody (1:10,000) at 4°C overnight. Then, the membranes were washed and primary antibodies were detected with fluorescent-labeled goat anti-rabbit IgG (1:15,000) or fluorescent-labeled goat anti-mouse IgG (1:15,000), and the bands were visualized by the Odyssey Western Blot Detection System (LI-COR Biotechnology, Lincoln, Nebraska, NE, United States). The images were analyzed using the Odyssey Application Software to obtain integrated intensities.
TUNEL Assay
TUNEL assays were performed with the In Situ Cell Death Detection Kit, POD according to the manufacturer’s instructions. To visualize all nuclei, slides were incubated with DAPI. Finally, the Olympus DP80 digital camera (Olympus, Tokyo, Japan) was used to capture fluorescence images and the percentage of TUNEL-positive cells was calculated.
Cell Viability Assay
Cell viability was assayed using CCK-8 (Beyotime, Jiangsu, China) according to the manufacturer’s protocol. Briefly, A549 cells were cultured in a 96-well plate, pretreated with RA (5, 10, 15, 20, 25 μM) or without RA for 24 h, and PBS was used as control. After normoxia or AR treatment, 10 μl CCK-8 solution/well was added and the cells were incubated for another 60 min at 37°C. The amount of formazan dye generated by cellular dehydrogenase activity was measured by absorbance at 450 nm with a microplate reader (ThermoFisher Scientific, Waltham, MA, United States).
Lactate Dehydrogenase Detection
LDH is a stable cytoplasmic enzyme that can be quickly released into the cell culture medium when the plasma membrane is damaged, and is regarded as a marker of cell damage (Kumar et al., 2018). After RA (15 μM) pretreatment for 24 h, A549 cells were cultured in 96-well plates and then subjected to AR treatment. The LDH detection kit was used to measure the amount of LDH released from the cells, according to the manufacturer’s instructions (Dong et al., 2013).
Dihydroethidium Staining
For detection of the superoxide anions, A549 cells in 24-well plates and tissue sections were incubated with 10 μM DHE at 37°C for 30 min. After being rinsed in PBS for three times, the fluorescence images were captured by an Olympus DP80 digital camera (Olympus, Tokyo, Japan) and analyzed using the Olympus Cellsens software.
Detection of Malondialdehyde, Superoxide Dismutase and NADPH Oxidase
The levels of MDA, activities of SOD and NOX in lung tissues and A549 cells were detected by the colorimetric method using commercially available kits purchased from the Jiancheng Bioengineering Institute. All procedures were performed according to the manufacturers’ instructions.
Statistical Analysis
Data were presented as mean ± standard deviation (SD) values. The data were analyzed using GraphPad PRISM software version 8.0 (GraphPad Software, Inc., San Diego, CA, United States) and SPSS 26.0 software (SPSS Inc., Chicago, IL, United States). Statistical significance was determined by the ANOVA test, followed by Sidak’s multiple comparisons test or Tukey’s multiple comparisons test for multi-group (>2) comparison. The statistic of the survival rate was analyzed by Kaplan-Meier curves and compared with the log-rank test followed by Bonferroni multiple comparison to determine the significance of difference. Differences were considered statistically significant when p < 0.05 as specified in the figure legends.
Result
Rosmarinic Acid Protects Against Pulmonary Ischemia/Reperfusion Injury
To explore the role of RA in pulmonary IR injury, the C57BL/6J mice were given different doses of RA (50, 75, or 100 mg/kg B.W/day) by gavage for 7 days before the pulmonary IR injury (Figure 1A). We measured the wet/dry lung weight ratio as an evaluation of pulmonary edema. RA pretreatment with 50, 75, and 100 mg/kg significantly reduced pulmonary edema in mice subjected to IR injury, with optimal effectiveness at 75 and 100 mg/kg, while 50 mg/kg RA pretreatment was less pronounced (Figure 1B). Hypoxemia is another hallmark associated with lung function, which is indicated by PaO2, partial pressure of carbon dioxide (PaCO2) and OI (Jia et al., 2014, 53; Chen et al., 2017). The arterial blood gas analysis showed PaCO2 was increased while PaO2 and OI were decreased in mice subjected to pulmonary IR injury, while RA pretreatment (75 and 100 mg/kg) effectively prevented lung dysfunction (Figures 1C,D). Thus, we selected 75 mg/kg RA as the optimal therapeutic concentration in the following experiment. Moreover, the H&E staining and lung injury score revealed that the RA pretreatment reduced lung edema, blood cell exudation, and inflammatory cells infiltration in IR-induced lung injury (Figures 1E,F). The levels of proinflammatory cytokines IL-1β and IL-6 were elevated in serum derived from IR injury mice, and RA pretreatment reversed the cytokines elevation (Figure 1G). Besides, we found that RA pretreatment significantly improved the survival of mice that were subjected to IR injury (Figure 1H). These results demonstrate that RA pretreatment has protective effects on lung function after IR injury by alleviating inflammation.
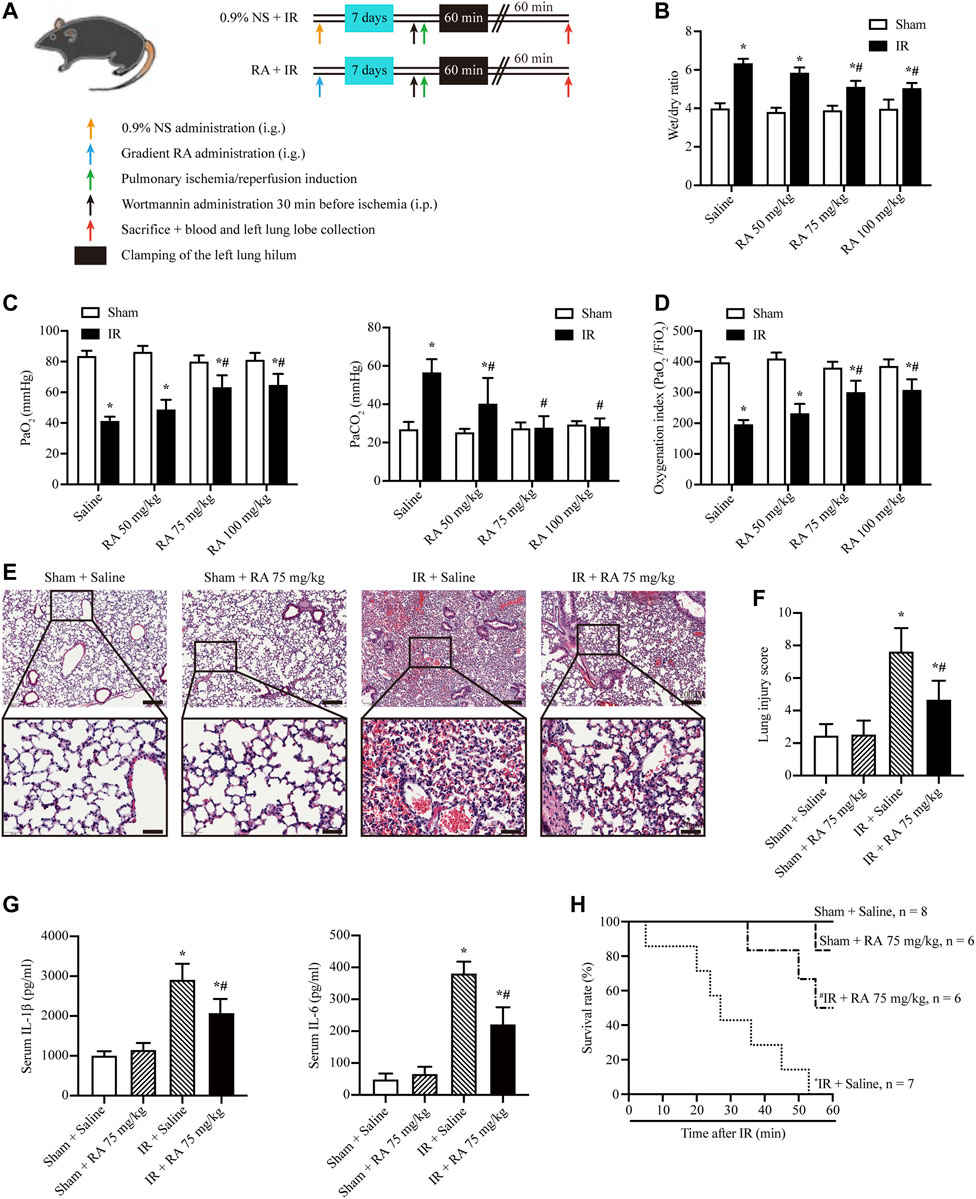
FIGURE 1. RA protects against pulmonary IR injury. (A) Schematic diagram of IR-induced lung injury in mice (RA: rosmarinic acid; IR: ischemia/reperfusion; NS: normal saline). (B) The wet/dry lung weight ratio of excised lungs from IR injury mice following 7 days of pretreatment with different dosages of RA was used to assess lung edema (n = 6; *p < 0.05 vs. Sham-Saline; #p < 0.05 vs. IR-Saline). (C) lung hypoxemia was evaluated as PaO2 and PaCO2 during IR injury (n = 6; *p < 0.05 vs. Sham-Saline; #p < 0.05 vs. IR-Saline). (D) OI (PaO2/FiO2) was calculated as a ratio to examine the respiratory dysfunction (n = 6; *p < 0.05 vs. Sham-Saline; #p < 0.05 vs. IR-Saline). (E) Histopathological changes in the IR-injured lung of mice pretreated with or without RA (scale bars, upper: 200 μm, lower: 50 μm). (F) The lung injury score of pulmonary tissues. Each group of staining was repeated at least 5 times, and 15 fields of view were selected for histopathological evaluation in each slice (n = 6; *p < 0.05 vs. Sham-Saline; #p < 0.05 vs. IR-Saline). (G) Serum concentrations of interleukin-1β (IL-1β) and interleukin-6 (IL-6) were measured using enzyme-linked immunosorbent assay (n = 6; *p < 0.05 vs. Sham-Saline; #p < 0.05 vs. IR-Saline). (H) The animal survival rate after pulmonary IR injury with RA pretreatment (n = 7 in IR + Saline group, n = 8 in Sham + Saline group, n = 6 in other groups; *p < 0.05 vs. Sham + Saline; #p < 0.05 vs. IR + Saline).
Rosmarinic Acid Attenuates Ischemia/Reperfusion-Increased Oxidative Stress and Cell Apoptosis in Mice
Besides inflammation, we investigated the effect of RA pretreatment on IR-induced ROS levels and cell apoptosis in the lung tissues. Our results showed that RA pretreatment reduced IR-induced ROS and MDA elevations in the lungs (Figures 2A,B). Moreover, we revealed that pro-oxidant enzymes, NOX2 and NOX4, were upregulated while anti-oxidant enzymes, SOD1 and SOD2, were downregulated upon pulmonary IR injury. Noticeably, all these expressions of enzymes were reversed by RA pretreatment (Figures 2C–G). Our data also revealed that RA pretreatment reduced the increased number of apoptotic cells and the boosted activity of active caspase 3 upon IR injury (Figures 2H,I). Overall, these results suggest that RA pretreatment protects pulmonary function through anti-oxidation and anti-apoptosis.
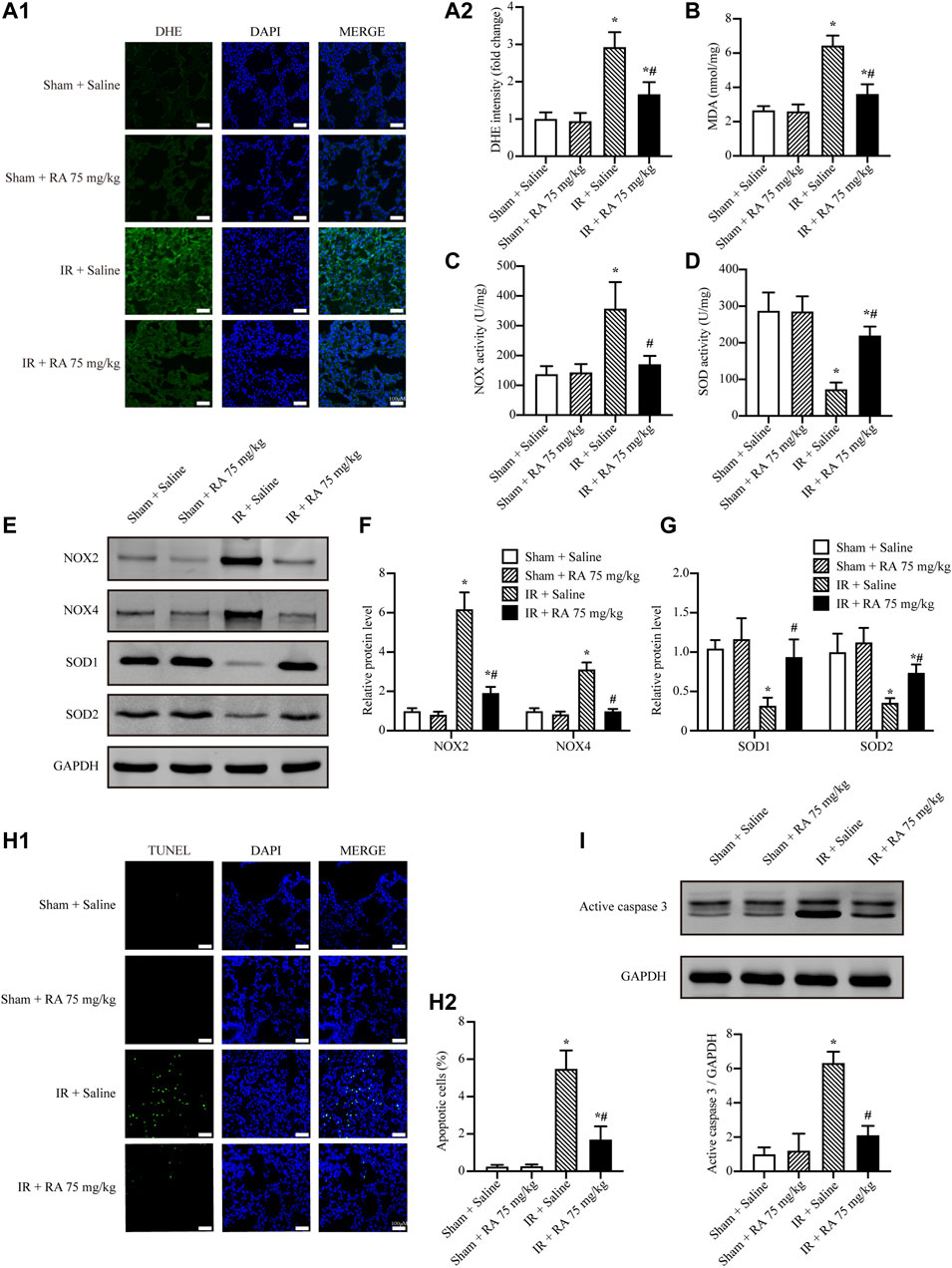
FIGURE 2. RA attenuates IR-increased oxidative stress and cell apoptosis in mice. (A1,A2) Representative micrographs of lung sections stained with DHE and semi-quantification analysis of fluorescence intensity indicated RA pretreatment reduced the ROS levels subjected to pulmonary IR injury (RA: rosmarinic acid; IR: ischemia/reperfusion; n = 6; *p < 0.05 vs. Sham + Saline; #p < 0.05 vs. IR + Saline; scale bars: 50 μm). (B) The expressions of MDA in lung tissues were detected (n = 6; *p < 0.05 vs. Sham + Saline; #p < 0.05 vs. IR + Saline). (C,D) The activities of NOX and SOD in lung tissues were detected (n = 6; *p < 0.05 vs. Sham + Saline; #p < 0.05 vs. IR + Saline). (E–G) NOX2, NOX4, SOD1 and SOD2 levels were detected using Western blots. The immunoblots were calculated by densitometric analysis using GAPDH as the internal reference (n = 3; *p < 0.05 vs. Sham + Saline; #p < 0.05 vs. IR + Saline). (H1,H2) TUNEL staining and statistical analysis showed RA pretreatment reduced cell apoptosis induced by IR injury (n = 6; *p < 0.05 vs. Sham + Saline; #p < 0.05 vs. IR + Saline; scale bar, 50 μm). (I) Representative blots and analysis results showed the active caspase 3 was elevated after IR injury and reversed by RA pretreatment (n = 3; *p < 0.05 vs. Sham + Saline; #p < 0.05 vs. IR + Saline).
Rosmarinic Acid Reduces Oxidative Stress and Apoptosis Induced by Anoxia/Reoxygenation Injury in A549 Cells
Alveolar epithelial cells are considered to be the principal target in pulmonary IR injury (Nicolls and Laubach, 2014). Thus, we further investigated the effect of RA on A549, a human lung epithelial cell line, which is used to investigate the function of alveolar epithelial cells (Giard et al., 1973). Here we determined the toxicity of RA in A549 cells at basal conditions, finding no obviously toxic effect of RA on cell viability after 24 h (Supplementary Figure S1A). Furthermore, based on the results of cell viability, we found RA pretreatment reduced AR-induced cell death, and the result showed the protective effect of RA started at 5 μM concentration, reached its maximum at 15 μM, and gradually decreased at 20–25 μM (Supplementary Figure S1B), which was consistent with previous research (Luan et al., 2013; Coelho et al., 2017). Thus, we selected 15 μM for subsequent experiments, finding that RA pretreatment (15 μM) greatly reduced cell death in A549 cells subjected to AR treatment measured by the LDH assay (Figure 3A). Meanwhile, it revealed the ability of RA to reduce AR-induced ROS levels, assessed by DHE staining and the MDA detection kit (Figures 3B,C). Similar results were obtained by LDH assay and DHE staining of RLE-6TN cells, a rat lung alveolar type II epithelial cell line (Supplementary Figures S2A,B). We also showed the increased SOD activity and decreased NOX activity were involved in the decreased ROS levels by RA in A549 cells (Figures 3D,E). Additionally, RA pretreatment reduced AR-induced cell apoptosis, as indicated by TUNEL staining (Figure 3F). Consistent with lung tissues, these data imply that RA protects pulmonary alveolar epithelial cells against oxidative damage and apoptosis through diminishing ROS accumulation.
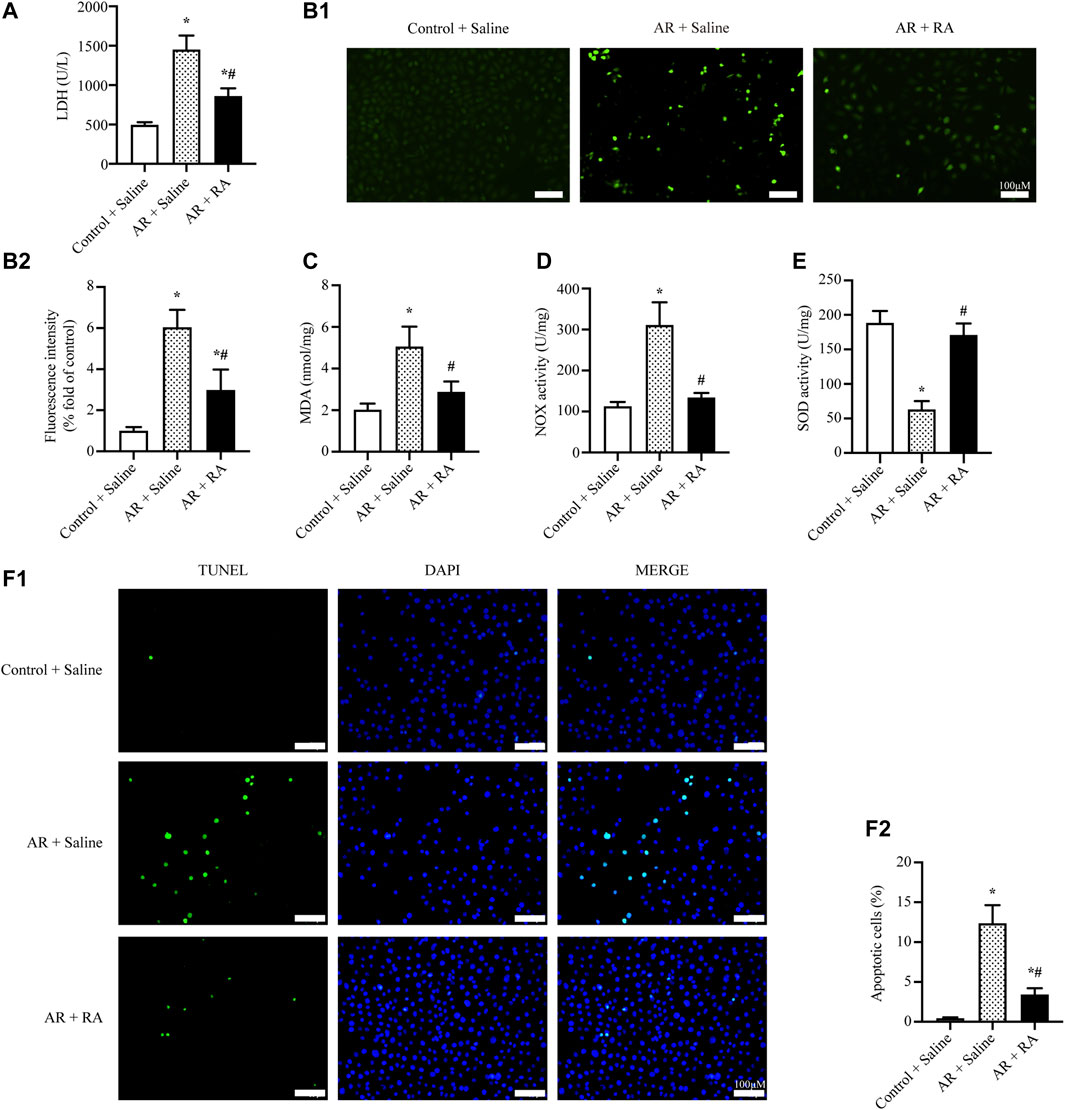
FIGURE 3. RA reduces oxidative stress and apoptosis induced by AR injury in A549 cells. (A) The release of LDH from A549 cells was reduced with RA (15 μM) pretreatment during AR injury (RA: rosmarinic acid; AR: anoxia/reoxygenation; n = 3; *p < 0.05 vs. Control + Saline; #p < 0.05 vs. AR + Saline). (B1,B2) Representative images and statistical analysis of DHE staining indicated that RA (15 μM) pretreatment reduced ROS content in AR-induced A549 cells (n = 3; *p < 0.05 vs. Control + Saline; #p < 0.05 vs. AR + Saline; scale bars: 100 μm). (C–E) The level of MDA and the activity of NOX were reduced while the activity of SOD was increased with RA (15 μM) pretreatment in AR-induced A549 cells (n = 3; *p < 0.05 vs. Control + Saline; #p < 0.05 vs. AR + Saline). (F1,F2) Representative pictures and the statistical results of the TUNEL assay illustrated that A549 cell apoptosis was alleviated with RA (15 μM) pretreatment when subjected to AR injury (n = 3; *p < 0.05 vs. Control + Saline; #p < 0.05 vs. AR + Saline; scale bars: 100 μm).
PI3K/Akt Signaling Pathway is Involved in the Protective Effect of Rosmarinic Acid on Oxidative Stress and Apoptosis Against Anoxia/Reoxygenation Injury
RA could activate PI3K (Vlavcheski et al., 2017), and the PI3K/Akt pathway has been considered to play a vital role in the regulation of oxidative stress and apoptosis. Thus, we further administrated the PI3K inhibitor, wortmannin (1 μM) and Akt inhibitor VIII (10 μM) to explore whether RA protects AR injury through the PI3K/Akt signaling pathway, which was consistent with previous research (Xu et al., 2008; Liu et al., 2020). The cell viability assay indicated Wortmannin (1 μM) and Akt inhibitor VIII (10 μM) partially reversed the protective effects of RA on AR-induced A549 cell damage (Supplementary Figure S3). Furthermore, the phosphorylation levels of PI3K and Akt increased during AR injury, which were further increased by RA pretreatment, as compared with AR. However, in the presence of wortmannin, the increased phosphorylation of PI3K and Akt was inhibited (Figures 4A,B). Additionally, compared with AR injury, RA pretreatment downregulated the expression of pro-oxidative enzymes NOX2 and NOX4 and upregulated the expression of anti-oxidative enzymes SOD1 and SOD2, which were blocked by wortmannin (Figures 4C,D). These data indicate that RA might enhance Akt phosphorylation to regulate NOX and SOD activities. Similarly, RA pretreatment increased the anti-apoptotic protein B-cell lymphoma-2 (BCL-2) but decreased pro-apoptotic BCL2-Associated X (BAX) and, thus, inhibited the activity of active caspase 3, which were all blocked by wortmannin treatment (Figures 4E,F). Therefore, RA depends on activating the PI3K/Akt signaling pathway to inhibit oxidative stress and apoptosis in pulmonary alveolar epithelial cells.
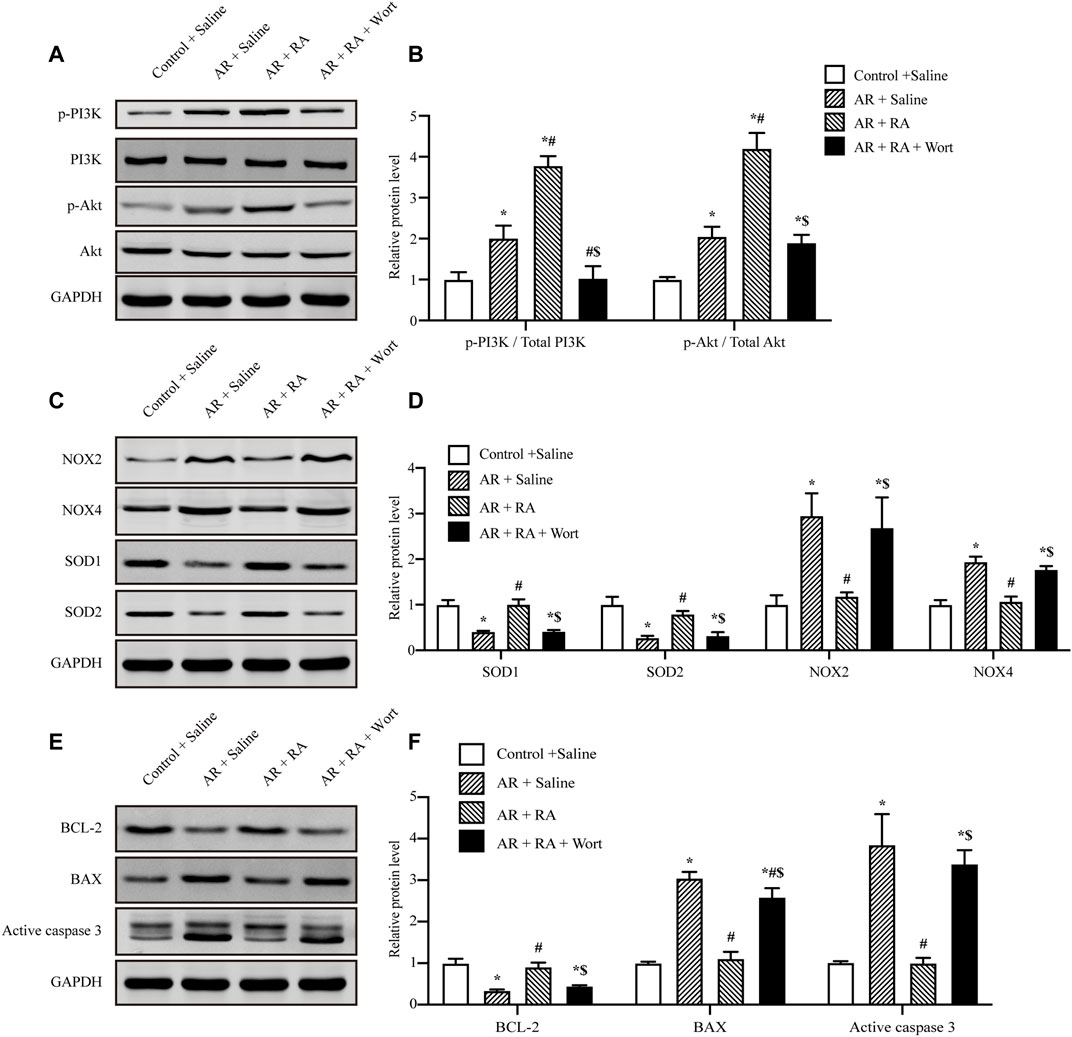
FIGURE 4. PI3K/Akt signaling pathway is involved in the protective effect of RA on oxidative stress and apoptosis against AR injury. (A,B) AR-induced PI3K and Akt phosphorylation increased with RA pretreatment (15 μM), but was blocked by wortmannin (1 μM). The immunoblots were calculated by densitometric analysis (RA: rosmarinic acid; AR: anoxia/reoxygenation; Wort: wortmannin; p-: phosphorylated; n = 3; *p < 0.05 vs. Control + Saline; #p < 0.05 vs. AR + Saline; $p < 0.05 vs. AR + RA). (C,D) Western blots were used to detect NOX2, NOX4, SOD1 and SOD2 levels. The immunoblots were calculated by densitometric analysis using GAPDH as the internal reference (n = 3; *p < 0.05 vs. Control + Saline; #p < 0.05 vs. AR + Saline; $p < 0.05 vs. AR + RA). (E,F) BCL-2, BAX and active caspase 3 levels were detected using Western blots. The immunoblots were calculated by densitometric analysis using GAPDH as the internal reference (n = 3; *p < 0.05 vs. Control + Saline; #p < 0.05 vs AR + Saline; $p < 0.05 vs. AR + RA).
These above data demonstrate that RA protects pulmonary IR injury in vivo, and the mechanism might be attributed to the PI3K/Akt signaling pathway to regulate ROS content in vitro (A549 cells). We further explored the role of the PI3K/Akt signaling pathway in vivo. Our results indicated that RA pretreatment alone reduced pulmonary edema, hypoxemia, and inflammation cytokines (IL-1β and IL-6) of the lung tissues following IR injury. The H&E staining indicated that the pretreatment of RA protected the structural integrity of lung tissue subjected to IR injury. In the presence of wortmannin alone (1 mg/kg), there was no significant effect on normal and IR-injured lung tissues, while the protective effect of RA was compromised in the presence of wortmannin and RA during IR injury (1 mg/kg) (Figures 5A–G).
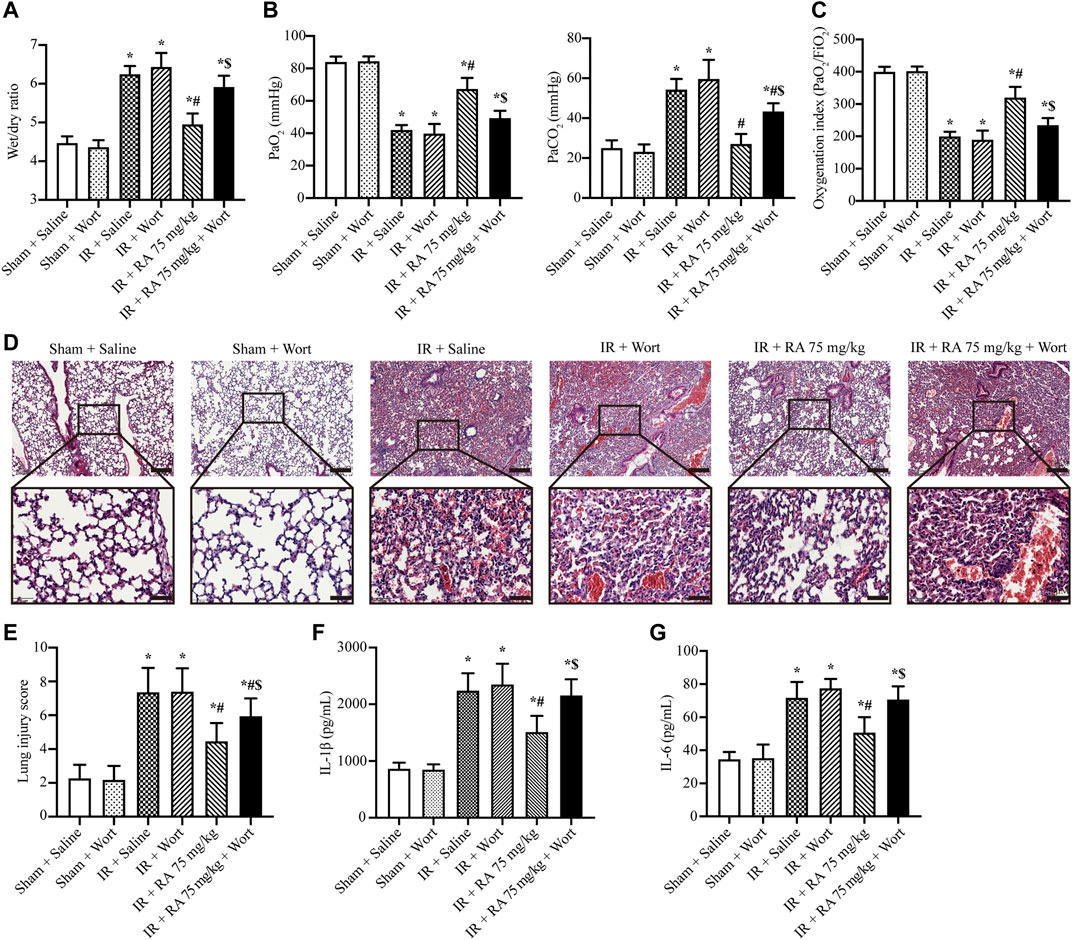
FIGURE 5. Effect of PI3K/Akt signaling pathway on the protection of pulmonary IR injury. (A) The wet/dry lung weight ratio of the excised lungs from mice demonstrated the effect of RA and wortmannin (1 mg/kg) administration on lung edema in IR mice (IR: ischemia/reperfusion; RA: rosmarinic acid; Wort: wortmannin; n = 6; *p < 0.05 vs. Sham + Saline; #p < 0.05 vs. IR + Saline; $p < 0.05 vs. IR + RA). (B) Lung hypoxemia was evaluated as plasma PaO2 and PaCO2 (n = 6; *p < 0.05 vs. Sham + Saline; #p < 0.05 vs. IR + Saline; $p < 0.05 vs. IR + RA). (C) OI (PaO2/FiO2) was calculated as a ratio to examine the respiratory dysfunction (n = 6; *p < 0.05 vs. Sham + Saline; #p < 0.05 vs. IR + Saline; $p < 0.05 vs. IR + RA). (D) Histopathological changes in IR-injured lungs of mice indicating RA pretreatment preserved the integrity of the lung structure, while wortmannin (1 mg/kg) reduced the protective effect (scale bars, upper: 200 μm, lower: 50 μm). (E) The lung injury score of pulmonary tissues. Each group of staining was repeated at least 5 times, and 15 fields of view were selected for histopathological evaluation in each slice (n = 6; *p < 0.05 vs. Sham + Saline; #p < 0.05 vs. IR + Saline; $p < 0.05 vs. IR + RA). The effects of RA on IL-1β (F), IL-6 (G) levels in lung tissues during IR injury (n = 6; *p < 0.05 vs. Sham + Saline; #p < 0.05 vs. IR + Saline; $p < 0.05 vs. IR + RA).
Discussion
Acute lung injury induced by pulmonary IR occurs in many clinical situations and becomes life-threatening in approximately 20% of patients (Sun et al., 2008). IR-induced pulmonary damage is characterized by nonspecific alveolar damage, pulmonary edema, and hypoxemia in clinics (Tang and Zhuang, 2019). At present, treatment is limited and narrowed to symptomatic and supportive care, which exhibits limited therapeutic effects. In recent years, natural compounds obtained from plants have received considerable attention. For instance, RA, possesses potent biological effects, has been shown to protect against IR injury in the heart and liver (Ramalho et al., 2014; Quan et al., 2021). However, the role of RA in pulmonary IR injury has not been reported yet. Thus, our study tried to explore the role of RA in the lung. Besides, more and more published reports have indicated the use of “drug preconditioning” or “drug pretreatment” to protect against limited operations. For instance, one study has implied a 7-day metformin pretreatment regimen significantly reduces postprocedural myocardial injury and improves 1-year clinical outcomes in patients undergoing percutaneous coronary intervention (Dirnagl et al., 2003; Li et al., 2014). In previous studies, pretreatment with folic acid for 1 week has been confirmed to ameliorate heart IR injury (Moens et al., 2008), while mice preconditioned with RA for 7 days show a prophylactic cardioprotective effect during myocardial IR injury (Quan et al., 2021). Thus, we pretreated mice with RA for 7 days and induced a pulmonary IR model. Our results found that 75 and 100 mg/kg RA pretreatment significantly alleviated hypoxemia and mitigated lung edema in pulmonary IR injury. Since the protective effects of the two concentrations on lung function and survival were approximately the same, we chose the relatively lower concentration of 75 mg/kg for further experiments. Lung function is largely dependent on its structural integrity, which is sensitive to inflammatory damage (Liu et al., 2018a). Therefore, we performed pathological staining and revealed that RA pretreatment reduced edema, inflammatory cells infiltration and blood cell exudation. Meanwhile, the levels of serum and lung tissue inflammatory factors were also reduced with RA pretreatment in IR injury. These were consistent with the findings that RA pretreatment inhibits the inflammatory cytokines through activating peroxisome proliferator-activated receptor-gamma (PPARγ) and down-regulating the nuclear factor kappa B (NF-κB)-mediated signaling pathway in the rat myocardial IR injury (Han et al., 2017). Besides, RA pretreatment improved the survival rate of mice subjected to IR injury. Thus, we conclude that RA improves lung tissue structure and function subjected to pulmonary IR injury by reducing inflammation damage.
During the pathological process of IR injury, massive ROS are produced as the initiating factor (Eltzschig and Eckle, 2011; Weissmann et al., 2012). For example, high levels of ROS directly oxidize cell membrane lipid to produce MDA and cause cell membrane structure injury (Kocaturk et al., 2020). In our study, we found that IR injury significantly increased ROS and MDA content in lung tissues and epithelial cells, but they were reduced by RA pretreatment. Previous studies have also shown RA protects against oxidative stress-induced liver and brain damage (Baranauskaite et al., 2020). Besides, lung tissue damage is the consequence not only of the direct effect of IR injury but also of ROS-mediated apoptosis (Valko et al., 2007; den Hengst et al., 2010). In our study, the decreased anti-apoptotic protein BCL-2, increased pro-apoptotic proteins BAX and caspase 3 activity in alveolar epithelial cells were inversed by RA pretreatment. These results were consistent with the previously reported study on the inhibition of apoptosis of RA (Lee et al., 2008). The above information indicates that RA protects lung alveolar epithelial cells and tissues, which are dependent on reducing IR-caused oxidative damage and apoptosis.
Furthermore, it’s widely accepted that the content of ROS is mainly regulated by the balance between ROS production and scavenging (Cui et al., 2018). The disrupted redox balance causes oxidative damage to lung tissue. NOX, especially NOX2 and NOX4, are the main source of cellular ROS production (Bedard and Krause, 2007; Katsuyama et al., 2011). On the other hand, cytoplasmic SOD1 and mitochondrial SOD2 are two of the most important endogenous ROS scavenging enzymes (Gerdprasert et al., 2019; Xie et al., 2020). In this study, RA pretreatment upregulated the expression and activities of antioxidant enzymes while downregulating the two pro-oxidant enzymes during IR injury, indicating that changes in the expression of oxidative kinases generally led to strong driving or damped alterations of enzyme activities and, ultimately, a low level of ROS. This result was similar to a previous study of RA on asthma (Liang et al., 2020). RA possesses the potential to eliminate ROS by chemical process in many diseases (Jang et al., 2018). However, the ROS elimination process needs further investigation in pulmonary IR injury. Summarily, RA protects pulmonary IR injury, at least in part, via increasing SOD1/2 activities and reducing NOX2/4 activities to reduce ROS content, subsequently alleviating oxidative damage and apoptosis.
The antioxidant effect of RA has been widely reported, and RA is even reported to be the most potent antioxidant among the hydroxycinnamic acids, which is the subclass of phenols (Soobrattee et al., 2005). However, the mechanism of RA regulation of NOX and SOD is unclear. Previous studies have reported that Akt activation inhibits the activity of NOX (Ying and Xiong, 2010) and upregulates the activity of SOD (Chen et al., 2019). In general, Akt acts as a downstream factor of PI3K, which is mediated by phosphoinositide-dependent kinase-1 (PDK-1) (Manning and Toker, 2017). Meanwhile, RA is a cinnamic acid derivative (Abd Aziz et al., 2021), and studies have shown that cinnamic acid could activate PI3K via binding to G-protein coupled receptors (GPCR) and Epidermal Growth Factor Receptor (EGFR) (Li et al., 2011; Kopp et al., 2014; Fruman et al., 2017). Therefore, we speculate that RA has a similar function in activating the PI3K/Akt signaling pathway, which is regarded as a critical event for survival and proliferation in various IR models (Dhanasekaran et al., 2008; Liu et al., 2020), while these effects could be abolished by coadministration with the PI3K specific inhibitor wortmannin, which binds to the p110 catalytic subunit of PI3K and irreversibly inhibits the enzyme (Ui et al., 1995; Ihara et al., 2020). Actually, previous studies have confirmed that the PI3K/Akt signaling pathway could be activated by RA (Vlavcheski et al., 2017). In our studies, we first assessed the expression and activities of NOX2/4 and SOD1/2 in lung tissues subjected to IR injury. Then we used alveolar epithelial cells to validate this phenomenon. Mechanistically, we found the PI3K/Akt signaling pathway was activated in AR treatment, which could be further activated by RA pretreatment with upregulated SODs activities and downregulated NOXs activities. Therefore, the ROS-induced apoptosis signal was also reduced. However, this activation effect of the PI3K/Akt signaling pathway and its downstream regulation of oxidative stress and apoptosis was removed in the presence of the PI3K inhibitor wortmannin in vitro. Moreover, in order to further clarify the clinical significance, mice were injected with wortmannin intraperitoneally 30 min before the IR injury. We evaluated a series of indicators, including pulmonary hypoxemia (PaO2, PaCO2, OI), lung edema, pathological staining, and levels of inflammatory factors in lung tissues. The analysis showed the protective effect of RA in pulmonary IR was abolished when PI3K was inhibited by wortmannin. These data indicate that RA could regulate NOXs and SODs activities through the PI3K/Akt signaling pathway.
Intriguingly, the changes in Akt phosphorylation in different animal models are variable. In our study, we detected that Akt phosphorylation was increased when subjected to IR injury. This phenomenon might be a compensatory response to stress. Previous studies found that short-term exposure to stress increases Akt activity, while long-term stress exposure inhibits the Akt signaling pathway (Chen et al., 2007; Tsai and Weissman, 2010). In our study, pulmonary IR injury was a relatively short-term injury (including 1 h for ischemia and 1 h for reperfusion), and phosphorylation of Akt was detected to upregulate during IR. The upregulation of Akt phosphorylation upon IR stress seems to be insufficient to inhibit NOX and compensate for SOD. When RA pretreatment, it further activates the PI3K/Akt signaling pathway, which ultimately inhibits NOXs, restores SODs, and protects lung IR.
The current study has several limitations. First, the potential mechanisms for RA-induced PI3K/AKT activation remain largely unknown. Although previous studies pointed out the cinnamic acid which derivates RA could activate PI3K by GPCR and EGFR, we still need further studies to reveal the underlying mechanisms. Second, since the cell viability analysis found RA improves cell survival in a dosage-dependent manner under AR insult, the pharmacokinetic parameters of RA are still unclear. And a further randomized control trial study could be performed to verify the therapeutical effect of RA in a further cohort.
Conclusion
Our study found RA inhibits the activities of NOXs and increases the activities of SODs through activating the PI3K/Akt signaling pathway, thereby eliminating ROS, inhibiting oxidative damage and apoptosis, ultimately protecting lung structure and function in pulmonary IR injury. This study provides insight into a better explanation of the mechanism of RA, which may be used as a potential target for the treatment of clinical pulmonary IR injury.
Data Availability Statement
The original contributions presented in the study are included in the article/Supplementary Material, further inquiries can be directed to the corresponding authors.
Ethics Statement
The animal study was reviewed and approved by Research Council and Animal Care and the Use Committee of Daping Hospital, the Third Military Medical University (ethical approval number: AMUWEC20213011).
Author Contributions
WL, CZ, and KC designed the study. WL, TY, CZ, and KC wrote the manuscript. CZ and KC acquired funding for the study. CZ and KC supervised the study. WL, YT, SC, HL, XL, SQ, CZ, KC acquired and analyzed the data. The order of co–first authors was determined by the volume of work each contributed to the study.
Funding
These studies were supported in part by grants from the National Natural Science Foundation of China (81930008, 31730043), National Key R&D Program of China (2018YFC1312700), Program of Innovative Research Team by National Natural Science Foundation (81721001).
Conflict of Interest
The authors declare that the research was conducted in the absence of any commercial or financial relationships that could be construed as a potential conflict of interest.
Publisher’s Note
All claims expressed in this article are solely those of the authors and do not necessarily represent those of their affiliated organizations, or those of the publisher, the editors and the reviewers. Any product that may be evaluated in this article, or claim that may be made by its manufacturer, is not guaranteed or endorsed by the publisher.
Acknowledgments
We would like to thanks Yu Li for her useful discussion and Yue Zhou (Southwest Medical University) for pathological section analysis.
Supplementary Material
The Supplementary Material for this article can be found online at: https://www.frontiersin.org/articles/10.3389/fphar.2022.860944/full#supplementary-material
References
Abd Aziz, N. A., Hasham, R., Sarmidi, M. R., Suhaimi, S. H., and Idris, M. K. H. (2021). A Review on Extraction Techniques and Therapeutic Value of Polar Bioactives from Asian Medicinal Herbs: Case Study on Orthosiphon Aristatus, Eurycoma Longifolia and Andrographis Paniculata. Saudi Pharm. J. 29, 143–165. doi:10.1016/j.jsps.2020.12.016
Abreu, M. D. M., Pazetti, R., Almeida, F. M., Correia, A. T., Parra, E. R., Silva, L. P., et al. (2014). Methylene Blue Attenuates Ischemia-Rreperfusion Injury in Lung Transplantation. J. Surg. Res. 192, 635–641. doi:10.1016/j.jss.2014.07.043
Almeida, F. M., Oliveira-Junior, M. C., Souza, R. A., Petroni, R. C., Soto, S. F., Soriano, F. G., et al. (2016). Creatine Supplementation Attenuates Pulmonary and Systemic Effects of Lung Ischemia and Reperfusion Injury. J. Heart Lung Transpl. 35, 242–250. doi:10.1016/j.healun.2015.06.012
Aoe, M., Trachiotis, G. D., Okabayashi, K., Manchester, J. K., Lowry, O. H., Cooper, J. D., et al. (1994). Administration of Prostaglandin E1 after Lung Transplantation Improves Early Graft Function. Ann. Thorac. Surg. 58, 655–661. doi:10.1016/0003-4975(94)90723-4
Bacha, E. A., Hervé, P., Murakami, S., Chapelier, A., Mazmanian, G. M., de Montpreville, V., et al. (1996). Lasting Beneficial Effect of Short-Term Inhaled Nitric Oxide on Graft Function after Lung Transplantation. Paris-Sud University Lung Transplantation Group. J. Thorac. Cardiovasc. Surg. 112, 590–598. doi:10.1016/S0022-5223(96)70040-5
Baranauskaite, J., Sadauskiene, I., Liekis, A., Kasauskas, A., Lazauskas, R., Zlabiene, U., et al. (2020). Natural Compounds Rosmarinic Acid and Carvacrol Counteract Aluminium-Induced Oxidative Stress. Molecules 25, 1807. doi:10.3390/molecules25081807
Bedard, K., and Krause, K. H. (2007). The NOX Family of ROS-Generating NADPH Oxidases: Physiology and Pathophysiology. Physiol. Rev. 87, 245–313. doi:10.1152/physrev.00044.2005
Cao, W., Mo, K., Wei, S., Lan, X., Zhang, W., and Jiang, W. (2019). Effects of Rosmarinic Acid on Immunoregulatory Activity and Hepatocellular Carcinoma Cell Apoptosis in H22 Tumor-Bearing Mice. Korean J. Physiol. Pharmacol. 23, 501–508. doi:10.4196/kjpp.2019.23.6.501
Chen, J. X., Zeng, H., Tuo, Q. H., Yu, H., Meyrick, B., and Aschner, J. L. (2007). NADPH Oxidase Modulates Myocardial Akt, ERK1/2 Activation, and Angiogenesis after Hypoxia-Reoxygenation. Am. J. Physiol. Heart Circ. Physiol. 292, H1664–H1674. doi:10.1152/ajpheart.01138.2006
Chen, K., Xu, Z., Liu, Y., Wang, Z., Li, Y., Xu, X., et al. (2017). Irisin Protects Mitochondria Function during Pulmonary Ischemia/Reperfusion Injury. Sci. Transl. Med. 9, eaao6298. doi:10.1126/scitranslmed.aao6298
Chen, X. J., Ren, S. M., Dong, J. Z., Qiu, C. G., Chen, Y. W., and Tao, H. L. (2019). Ginkgo Biloba Extract-761 Protects Myocardium by Regulating Akt/Nrf2 Signal Pathway. Drug Des. Devel. Ther. 13, 647–655. doi:10.2147/DDDT.S191537
Coelho, V. R., Viau, C. M., Staub, R. B., De Souza, M. S., Pflüger, P., Regner, G. G., et al. (2017). Rosmarinic Acid Attenuates the Activation of Murine Microglial N9 Cells through the Downregulation of Inflammatory Cytokines and Cleaved Caspase-3. Neuroimmunomodulation 24, 171–181. doi:10.1159/000481095
Cui, Y., Wang, Y., Li, G., Ma, W., Zhou, X. S., Wang, J., et al. (2018). The Nox1/Nox4 Inhibitor Attenuates Acute Lung Injury Induced by Ischemia-Reperfusion in Mice. PLOS ONE 13, e0209444. doi:10.1371/journal.pone.0209444
D'Amico, F., Vitale, A., Piovan, D., Bertacco, A., Ramirez Morales, R., Chiara Frigo, A., et al. (2013). Use of N-Acetylcysteine during Liver Procurement: a Prospective Randomized Controlled Study. Liver Transpl. 19, 135–144. doi:10.1002/lt.23527
den Hengst, W. A., Gielis, J. F., Lin, J. Y., Van Schil, P. E., De Windt, L. J., and Moens, A. L. (2010). Lung Ischemia-Reperfusion Injury: a Molecular and Clinical View on a Complex Pathophysiological Process. Am. J. Physiol. Heart Circ. Physiol. 299, H1283–H1299. doi:10.1152/ajpheart.00251.2010
Dhanasekaran, A., Gruenloh, S. K., Buonaccorsi, J. N., Zhang, R., Gross, G. J., Falck, J. R., et al. (2008). Multiple Antiapoptotic Targets of the PI3K/Akt Survival Pathway Are Activated by Epoxyeicosatrienoic Acids to Protect Cardiomyocytes from Hypoxia/Anoxia. Am. J. Physiol. Heart Circ. Physiol. 294, H724–H735. doi:10.1152/ajpheart.00979.2007
Dirnagl, U., Simon, R. P., and Hallenbeck, J. M. (2003). Ischemic Tolerance and Endogenous Neuroprotection. Trends Neurosci. 26, 248–254. doi:10.1016/S0166-2236(03)00071-7
Dong, L., Liu, Y., Lu, Y., Zhang, L., Man, N., Cao, L., et al. (2013). Tuning Magnetic Property and Autophagic Response for Self-Assembled Ni-Co Alloy Nanocrystals. Adv. Funct. Mater. 23, 5930–5940. doi:10.1002/adfm.201203767
Eltzschig, H. K., and Eckle, T. (2011). Ischemia and Reperfusion--From Mechanism to Translation. Nat. Med. 17, 1391–1401. doi:10.1038/nm.2507
Fruman, D. A., Chiu, H., Hopkins, B. D., Bagrodia, S., Cantley, L. C., and Abraham, R. T. (2017). The PI3K Pathway in Human Disease. Cell 170, 605–635. doi:10.1016/j.cell.2017.07.029
Gerdprasert, O., Choomchuay, N., Chantong, B., Sutanthavibul, N., Meksuriyen, D., and Nusuetrong, P. (2019). Phikud Navakot Modulates the Level of Pro-Inflammatory Mediators and the Protein Expression of SOD1 and 2 and the Nrf2/HO-1 Signaling Pathway in Rats with Acute Myocardial Infarction. Evid. Based Complement. Alternat. Med. 2019, 4823645. doi:10.1155/2019/4823645
Giard, D. J., Aaronson, S. A., Todaro, G. J., Arnstein, P., Kersey, J. H., Dosik, H., et al. (1973). In Vitro cultivation of Human Tumors: Establishment of Cell Lines Derived from a Series of Solid Tumors. J. Natl. Cancer Inst. 51, 1417–1423. doi:10.1093/jnci/51.5.1417
Han, J., Wang, D., Ye, L., Li, P., Hao, W., Chen, X., et al. (2017). Rosmarinic Acid Protects against Inflammation and Cardiomyocyte Apoptosis during Myocardial Ischemia/Reperfusion Injury by Activating Peroxisome Proliferator-Activated Receptor Gamma. Front. Pharmacol. 8, 456. doi:10.3389/fphar.2017.00456
Ihara, M., Shichijo, K., Takeshita, S., and Kudo, T. (2020). Wortmannin, a Specific Inhibitor of Phosphatidylinositol-3-Kinase, Induces Accumulation of DNA Double-Strand Breaks. J. Radiat. Res. 61, 171–176. doi:10.1093/jrr/rrz102
Imai, K., Nakanishi, I., Ohkubo, K., Ohno, A., Mizuno, M., Fukuzumi, S., et al. (2019). Synthesis and Radical-Scavenging Activity of C-Methylated Fisetin Analogues. Bioorg. Med. Chem. 27, 1720–1727. doi:10.1016/j.bmc.2019.02.033
Inci, I., Inci, D., Dutly, A., Boehler, A., and Weder, W. (2002). Melatonin Attenuates Posttransplant Lung Ischemia-Reperfusion Injury. Ann. Thorac. Surg. 73, 220–225. doi:10.1016/S0003-4975(01)03101-0
Jang, Y. G., Hwang, K. A., and Choi, K. C. (2018). Rosmarinic Acid, a Component of Rosemary Tea, Induced the Cell Cycle Arrest and Apoptosis through Modulation of HDAC2 Expression in Prostate Cancer Cell Lines. Nutrients 10, 1784. doi:10.3390/nu10111784
Jia, Y., Chen, K., Lin, P., Lieber, G., Nishi, M., Yan, R., et al. (2014). Treatment of Acute Lung Injury by Targeting MG53-Mediated Cell Membrane Repair. Nat. Commun. 5, 4387. doi:10.1038/ncomms5387
Joardar, S., Dewanjee, S., Bhowmick, S., Dua, T. K., Das, S., Saha, A., et al. (2019). Rosmarinic Acid Attenuates Cadmium-Induced Nephrotoxicity via Inhibition of Oxidative Stress, Apoptosis, Inflammation and Fibrosis. Int. J. Mol. Sci. 20, 2027. doi:10.3390/ijms20082027
Johnson, E. R., and Matthay, M. A. (2010). Acute Lung Injury: Epidemiology, Pathogenesis, and Treatment. J. Aerosol Med. Pulm. Drug Deliv. 23, 243–252. doi:10.1089/jamp.2009.0775
Katsuyama, M., Matsuno, K., and Yabe-Nishimura, C. (2011). Physiological Roles of NOX/NADPH Oxidase, the Superoxide-Generating Enzyme. J. Clin. Biochem. Nutr. 50, 9–22. doi:10.3164/jcbn.11-06SR
Kocaturk, H., Bedir, F., Altay, M. S., Bakan, E., Suleyman, B., Yazici, G. N., et al. (2020). The Effect of Desloratadine on Ischemia Reperfusion Induced Oxidative and Inflammatory Renal Injury in Rats. Ren. Fail. 42, 531–538. doi:10.1080/0886022X.2020.1769656
Kopp, C., Singh, S. P., Regenhard, P., Müller, U., Sauerwein, H., and Mielenz, M. (2014). Trans-Cinnamic Acid Increases Adiponectin and the Phosphorylation of AMP-Activated Protein Kinase through G-Protein-Coupled Receptor Signaling in 3T3-L1 Adipocytes. Int. J. Mol. Sci. 15, 2906–2915. doi:10.3390/ijms15022906
Kumar, P., Nagarajan, A., and Uchil, P. D. (2018). Analysis of Cell Viability by the Lactate Dehydrogenase Assay. Cold Spring Harb. Protoc. 2018 (6), pdb.prot095497. doi:10.1101/pdb.prot095497
Lan, C. C., Peng, C. K., Tang, S. E., Huang, K. L., and Wu, C. P. (2017). Carbonic Anhydrase Inhibitor Attenuates Ischemia-Reperfusion Induced Acute Lung Injury. PLoS One 12, e0179822. doi:10.1371/journal.pone.0179822
Lee, H. J., Cho, H. S., Park, E., Kim, S., Lee, S. Y., Kim, C. S., et al. (2008). Rosmarinic Acid Protects Human Dopaminergic Neuronal Cells against Hydrogen Peroxide-Induced Apoptosis. Toxicology 250, 109–115. doi:10.1016/j.tox.2008.06.010
Li, D. D., Lv, P. C., Zhang, H., Zhang, H. J., Hou, Y. P., Liu, K., et al. (2011). The Combination of 4-Anilinoquinazoline and Cinnamic Acid: A Novel Mode of Binding to the Epidermal Growth Factor Receptor Tyrosine Kinase. Bioorg. Med. Chem. 19, 5012–5022. doi:10.1016/j.bmc.2011.06.044
Li, J., Xu, J. P., Zhao, X. Z., Sun, X. J., Xu, Z. W., and Song, S. J. (2014). Protective Effect of Metformin on Myocardial Injury in Metabolic Syndrome Patients Following Percutaneous Coronary Intervention. Cardiology 127, 133–139. doi:10.1159/000355574
Liang, Z., Wu, L., Deng, X., Liang, Q., Xu, Y., Deng, R., et al. (2020). The Antioxidant Rosmarinic Acid Ameliorates Oxidative Lung Damage in Experimental Allergic Asthma via Modulation of NADPH Oxidases and Antioxidant Enzymes. Inflammation 43, 1902–1912. doi:10.1007/s10753-020-01264-3
Liu, C., Chen, K., Wang, H., Zhang, Y., Duan, X., Xue, Y., et al. (2020). Gastrin Attenuates Renal Ischemia/Reperfusion Injury by a PI3K/Akt/Bad-Mediated Anti-Apoptosis Signaling. Front. Pharmacol. 11, 540479. doi:10.3389/fphar.2020.540479
Liu, H., Tao, S., Ma, H., Jin, J., Jing, J., Yao, L., et al. (2018a). Functional Changes of Airway Epithelial Cells and Mitochondria in Rat Models of Asthenic Lung and Phlegm Blocking Combined with Cough Variant Asthma. Exp. Ther. Med. 16 (6), 5021–5024. doi:10.3892/etm.2018.6863
Liu, Y. Y., Sun, C., Xue, F. S., Yang, G. Z., Li, H. X., Liu, Q., et al. (2018b). Effect of Autophagy Inhibition on the Protection of Ischemia Preconditioning against Myocardial Ischemia/Reperfusion Injury in Diabetic Rats. Chin. Med. J. (Engl) 131, 1702–1709. doi:10.4103/0366-6999.235867
Luan, H., Kan, Z., Xu, Y., Lv, C., and Jiang, W. (2013). Rosmarinic Acid Protects against Experimental Diabetes with Cerebral Ischemia: Relation to Inflammation Response. J. Neuroinflammation 10, 28. doi:10.1186/1742-2094-10-28
Manning, B. D., and Toker, A. (2017). AKT/PKB Signaling: Navigating the Network. Cell 169, 381–405. doi:10.1016/j.cell.2017.04.001
Moens, A. L., Champion, H. C., Claeys, M. J., Tavazzi, B., Kaminski, P. M., Wolin, M. S., et al. (2008). High-Dose Folic Acid Pretreatment Blunts Cardiac Dysfunction during Ischemia Coupled to Maintenance of High-Energy Phosphates and Reduces Postreperfusion Injury. Circulation 117, 1810–1819. doi:10.1161/CIRCULATIONAHA.107.725481
Nicolls, M. R., and Laubach, V. E. (2014). Traumatic Brain Injury: Lungs in a RAGE. Sci. Transl. Med. 6, 252fs34. doi:10.1126/scitranslmed.3010259
Oshima, Y., Sakamoto, S., Yamasaki, K., Mochida, S., Funaki, K., Moriyama, N., et al. (2016). Desflurane Inhalation before Ischemia Increases Ischemia-Reperfusion-Induced Vascular Leakage in Isolated Rabbit Lungs. SpringerPlus 5, 2031. doi:10.1186/s40064-016-3741-9
Petersen, M. (2013). Rosmarinic Acid: New Aspects. Phytochem. Rev. 12, 207–227. doi:10.1007/s11101-013-9282-8
Quan, W., Liu, H. X., Zhang, W., Lou, W. J., Gong, Y. Z., Yuan, C., et al. (2021). Cardioprotective Effect of Rosmarinic Acid against Myocardial Ischaemia/Reperfusion Injury via Suppression of the NF-κB Inflammatory Signalling Pathway and ROS Production in Mice. Pharm. Biol. 59, 222–231. doi:10.1080/13880209.2021.1878236
Ramalho, L. N., Pasta, Â. A., Terra, V. A., Augusto, M., Sanches, S. C., Souza-Neto, F. P., et al. (2014). Rosmarinic Acid Attenuates Hepatic Ischemia and Reperfusion Injury in Rats. Food Chem. Toxicol. 74, 270–278. doi:10.1016/j.fct.2014.10.004
Rašković, A., Milanović, I., Pavlović, N., Ćebović, T., Vukmirović, S., and Mikov, M. (2014). Antioxidant Activity of Rosemary (Rosmarinus Officinalis L.) Essential Oil and its Hepatoprotective Potential. BMC Complement. Altern. Med. 14, 225. doi:10.1186/1472-6882-14-225
Soobrattee, M. A., Neergheen, V. S., Luximon-Ramma, A., Aruoma, O. I., and Bahorun, T. (2005). Phenolics as Potential Antioxidant Therapeutic Agents: Mechanism and Actions. Mutat. Res. 579, 200–213. doi:10.1016/j.mrfmmm.2005.03.023
Sun, J., Yang, D., Li, S., Xu, Z., Wang, X., and Bai, C. (2008). Effects of Curcumin or Dexamethasone on Lung Ischaemia-Reperfusion Injury in Rats. Eur. Respir. J. 33, 398–404. doi:10.1183/09031936.00142407
Takano, H., Osakabe, N., Sanbongi, C., Yanagisawa, R., Inoue, K., Yasuda, A., et al. (2004). Extract of Perilla Frutescens Enriched for Rosmarinic Acid, a Polyphenolic Phytochemical, Inhibits Seasonal Allergic Rhinoconjunctivitis in Humans. Exp. Biol. Med. (Maywood) 229, 247–254. doi:10.1177/153537020422900305
Takıl, A., Umuroğlu, T., Göğüş, Y. F., Etı, Z., Yildizelı, B., and Ahiskali, R. (2003). Histopathologic Effects of Lipid Content of Enteral Solutions after Pulmonary Aspiration in Rats. Nutrition 19, 666–669. doi:10.1016/S0899-9007(03)00057-1
Tang, J., and Zhuang, S. (2019). Histone Acetylation and DNA Methylation in Ischemia/Reperfusion Injury. Clin. Sci. (Lond) 133, 597–609. doi:10.1042/CS20180465
Tsai, Y. C., and Weissman, A. M. (2010). The Unfolded Protein Response, Degradation from Endoplasmic Reticulum and Cancer. Genes Cancer 1, 764–778. doi:10.1177/1947601910383011
Ui, M., Okada, T., Hazeki, K., and Hazeki, O. (1995). Wortmannin as a Unique Probe for an Intracellular Signalling Protein, Phosphoinositide 3-Kinase. Trends Biochem. Sci. 20, 303–307. doi:10.1016/S0968-0004(00)89056-8
Valko, M., Leibfritz, D., Moncol, J., Cronin, M. T., Mazur, M., and Telser, J. (2007). Free Radicals and Antioxidants in Normal Physiological Functions and Human Disease. Int. J. Biochem. Cel Biol. 39, 44–84. doi:10.1016/j.biocel.2006.07.001
Vlavcheski, F., Naimi, M., Murphy, B., Hudlicky, T., and Tsiani, E. (2017). Rosmarinic Acid, a Rosemary Extract Polyphenol, Increases Skeletal Muscle Cell Glucose Uptake and Activates AMPK. Molecules 22, 1669. doi:10.3390/molecules22101669
Wang, L. F., Sun, Y. Y., Pan, Q., Yin, Y. Q., Tian, X. M., Liu, Y., et al. (2021). Diminazen Aceturate Protects Pulmonary Ischemia-Reperfusion Injury via Inhibition of ADAM17-Mediated Angiotensin-Converting Enzyme 2 Shedding. Front. Pharmacol. 12, 713632. doi:10.3389/fphar.2021.713632
Weissmann, N., Sydykov, A., Kalwa, H., Storch, U., Fuchs, B., Mederos y Schnitzler, M., et al. (2012). Activation of TRPC6 Channels Is Essential for Lung Ischaemia-Reperfusion Induced Oedema in Mice. Nat. Commun. 3, 649. doi:10.1038/ncomms1660
Xie, C., Ge, M., Jin, J., Xu, H., Mao, L., Geng, S., et al. (2020). Mechanism Investigation on Bisphenol S-Induced Oxidative Stress and Inflammation in Murine RAW264.7 Cells: The Role of NLRP3 Inflammasome, TLR4, Nrf2 and MAPK. J. Hazard. Mater. 394, 122549. doi:10.1016/j.jhazmat.2020.122549
Xu, X., Chua, C. C., Gao, J., Chua, K. W., Wang, H., Hamdy, R. C., et al. (2008). Neuroprotective Effect of Humanin on Cerebral Ischemia/Reperfusion Injury Is Mediated by a PI3K/Akt Pathway. Brain Res. 1227, 12–18. doi:10.1016/j.brainres.2008.06.018
Xu, Y., Li, X., Cheng, Y., Yang, M., and Wang, R. (2020). Inhibition of ACSL4 Attenuates Ferroptotic Damage after Pulmonary Ischemia-Reperfusion. FASEB J. 34, 16262–16275. doi:10.1096/fj.202001758R
Yang, C., Zhao, T., Lin, M., Zhao, Z., Hu, L., Jia, Y., et al. (2013). Helix B Surface Peptide Administered after Insult of Ischemia Reperfusion Improved Renal Function, Structure and Apoptosis through Beta Common Receptor/Erythropoietin Receptor and PI3K/Akt Pathway in a Murine Model. Exp. Biol. Med. (Maywood) 238, 111–119. doi:10.1258/ebm.2012.012185
Ying, W., and Xiong, Z. G. (2010). Oxidative Stress and NAD+ in Ischemic Brain Injury: Current Advances and Future Perspectives. Curr. Med. Chem. 17, 2152–2158. doi:10.2174/092986710791299911
Zamora, M. R., Davis, R. D., Keshavjee, S. H., Schulman, L., Levin, J., Ryan, U., et al. (1999). Complement Inhibition Attenuates Human Lung Transplant Reperfusion Injury: A Multicenter Trial. Chest 116, 46S. doi:10.1378/chest.116.suppl_1.46S
Keywords: rosmarinic acid, pulmonary ischemia/reperfusion injury, oxidative stress, apoptosis, PI3K/AKT
Citation: Luo W, Tao Y, Chen S, Luo H, Li X, Qu S, Chen K and Zeng C (2022) Rosmarinic Acid Ameliorates Pulmonary Ischemia/Reperfusion Injury by Activating the PI3K/Akt Signaling Pathway. Front. Pharmacol. 13:860944. doi: 10.3389/fphar.2022.860944
Received: 24 January 2022; Accepted: 30 March 2022;
Published: 11 May 2022.
Edited by:
Bhagavatula Moorthy, Baylor College of Medicine, United StatesReviewed by:
Zhenhua Zeng, Southern Medical University, ChinaMona Fouad Mahmoud, Zagazig University, Egypt
Copyright © 2022 Luo, Tao, Chen, Luo, Li, Qu, Chen and Zeng. This is an open-access article distributed under the terms of the Creative Commons Attribution License (CC BY). The use, distribution or reproduction in other forums is permitted, provided the original author(s) and the copyright owner(s) are credited and that the original publication in this journal is cited, in accordance with accepted academic practice. No use, distribution or reproduction is permitted which does not comply with these terms.
*Correspondence: Ken Chen, Y2tfdG1tdUBzaW5hLmNvbQ==; Chunyu Zeng, Y2h1bnl1emVuZzAxQDE2My5jb20=
†These authors have contributed equally to this work and share first authorship