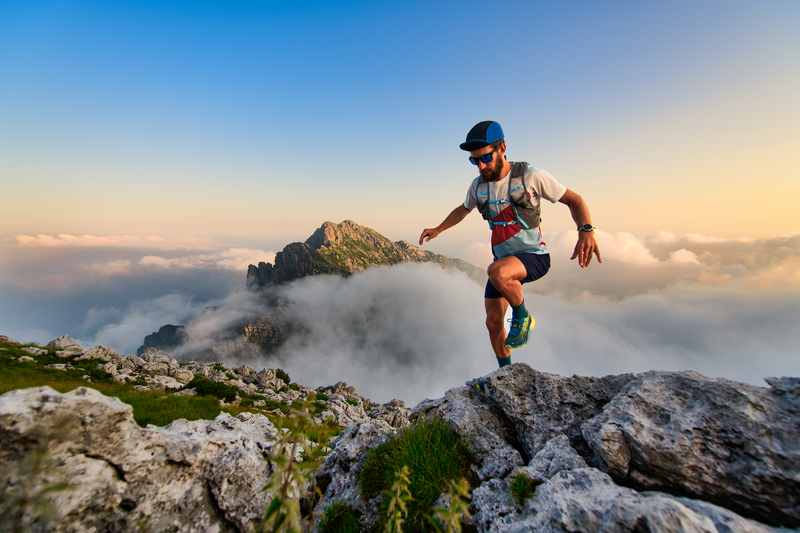
94% of researchers rate our articles as excellent or good
Learn more about the work of our research integrity team to safeguard the quality of each article we publish.
Find out more
ORIGINAL RESEARCH article
Front. Pharmacol. , 20 April 2022
Sec. Pharmacology of Anti-Cancer Drugs
Volume 13 - 2022 | https://doi.org/10.3389/fphar.2022.849364
Nineteen erlotinib derivatives bearing different 1,2,3-triazole moieties were designed, synthesized, and evaluated for their potential against different cancer cell lines. The structures of the synthesized compounds were confirmed via 1H NMR, 13C NMR, and HR MS. Preliminary antitumor activity assay results suggested that some compounds showed remarkable inhibitory activity against different cancer cell lines including the corresponding drug-resistant ones. Among these compounds, 3d was the most promising one with an IC50 of 7.17 ± 0.73 μM (KYSE70TR), 7.91 ± 0.61 μM (KYSE410TR), 10.02 ± 0.75 μM (KYSE450TR), 5.76 ± 0.3 3 μM (H1650TR), and 2.38 ± 0.17 μM (HCC827GR). A preliminary mechanism study suggested that compound 3d suppressed cancer cell proliferation through the EGFR-TK pathway.
Epidermal growth factor receptor (EGFR) is closely related to carcinogenesis of different cancers (Wells, 1989; Mendelsohn, 1992; Brand et al., 2006). Many important cellular functions such as cell growth, proliferation, and cell death can be controlled by epidermal growth factor receptor tyrosine kinase (EGFR-TK) (Thomas, 2003). Uncontrollable cell growth and malignant cell proliferation can occur upon the overexpression of EGFR-TK (Murtuza et al., 2019), and inhibiting the high expression of EGFR-TK has been proven to be an effective measure to reduce tumor growth and proliferation (Roskoski, 2014). As a consequence, targeting EGFR has become a prolific field of research such as anticancer (Cohen et al., 2003; Zhang et al., 2017), kidney inflammation and damage (Rayego-Mateos et al., 2018), and anti-inflammation against LPS-stimulated production of NO in peritoneal macrophages (Elkamhawy et al., 2019). Especially, significant progresses were achieved in the treatment of non-small-cell lung cancers using EGFR-targeting therapeutics (Kelloff et al., 1996; Mukherji and Spicer, 2009; Ou, 2012; Liao et al., 2015; Minari et al., 2016; Chen et al., 2019; Wright and Goss, 2019; Rebuzzi et al., 2020). These include different generations of EGFR-TKIs as early as gefitinib (Culy and Faulds, 2002), erlotinib (Kim and Murren, 2002), or the most recent EGFR-TKIs such as rociletinib (Chuang et al., 2016) or osimertinib (Greig, 2016).
While such EGFR-TKIs can significantly improve the life quality and the median survival rate of patients and show better performance in terms of progression-free survival rate or objective response rate, such therapeutics also suffer problems such as drug resistance after a period of administration (Spaans and Goss, 2014; Karlsen et al., 2021; Zhao et al., 2021), and developing new EGFR-TK targeting chemical entities with reduced drug resistance but similar antitumor activity is highly desirable (Zhang et al., 2014; Maione et al., 2015; Tsubata et al., 2021).
We are interested in developing new chemical entities using known therapeutics as lead compounds (Mao LF. et al., 2020; Mao L. et al., 2020; Wang et al., 2020). Herein, we present our preliminary results on the preparation of new erlotinib derivatives, and the antitumor activity of the prepared compounds against different cancer cell lines. Successfully marketed drugs generally showed good druggability such as good pharmacokinetic property, ideal solubility or high activity, and drug discovery process could be effectively facilitated using a known drug as a lead compound.
Erlotinib (Figure 1) is a classical EGFR-TKI approved for the treatment of advanced non-small-cell lung cancer (NSCLC) (Kim and Murren, 2002; Schettino et al., 2008). Compared with traditional chemotherapeutics, erlotinib can improve the median survival rate of patients and exhibit better performance in terms of progression-free survival rate, objective response rate, quality of life, and tolerability (Mathew et al., 2015).
In addition, erlotinib was also effective in the treatment of esophageal cancer (Choi et al., 2012). Esophageal cancer is a common malignant cancer with high morbidity and mortality, and 5-year survival rate for esophageal cancer patients is lower than that of lung cancer (Hiroshi et al., 2005; Wei et al., 2020). A study showed that 40–80% of esophageal cancer patients are diagnosed with high expression of EGFR (Hirsch et al., 2017), and erlotinib can be used as an adjuvant therapy for the treatment of esophageal cancer in combination with radiotherapy and chemotherapy (Sutter et al., 2005; Zhong et al., 2020). However, drug resistance and adverse reactions have become prominent issues after a period of erlotinib treatment (Sutter et al., 2010; Whitley et al., 2012), and development of new EGFR-TKIs is highly desirable to maintain the antitumor activity of the drug on the one hand, and to tackle the drug resistance problem on the other (Chen et al., 2020).
Nitrogen-containing heterocyclic compounds are widely present in nature and have played key roles in drug discovery (DeSimone et al., 2004; Costantino, 2006). Especially, 1,2,3-triazole moieties are an important category of nitrogen-containing heterocycles in the design of biologically active molecules (Thomopoulou et al., 2015). 1,2,3-Triazole has a large dipole moment and can form a variety of non-covalent interactions with different functional groups. The structural characteristics of 1,2,3-triazoles make them ideal surrogates for amides, esters or carboxylic acids, and compounds bearing triazole moieties often showed broad-spectrum biological activities such as antibacterial (Röhrig et al., 2012; Mao et al., 2017), antimalarial, antifungal, antiviral (Hong et al., 2010), anti-tuberculosis, and antitumor activities (Qi et al., 2020).
On the basis of these rationales, different 1,2,3-triazole moieties were introduced to erlotinib in an attempt to maintaining the antitumor activity of the parent drug and in some extent tackling the drug resistance problem of the drug (Figure 2).
The preparation of the target compounds is illustrated in Scheme 1. Erlotinib (2) was obtained after the reaction of 4-chloro-6,7-bis(methoxyethoxy)quinazolinone with 3-aminophenylacetylene (Chandregowda et al., 2007a, b, c). The target compounds 3a-3s were obtained via the click reaction of erlotinib with different azido compounds (Xu et al., 2016) (Table 1). The reaction conditions of these operations were mild, and the reactions were easy to carry out. The structures of the key intermediates and all target compounds were confirmed by nuclear magnetic resonance (1H NMR and 13C NMR) and high-resolution mass spectrometry (HR MS). The purity of the key compounds was checked with HPLC.
A total of five tumor cell lines including three esophageal cancer cell lines (KYSE70, KYSE410, and KYSE450) and two lung cancer cell lines (H1650 and HCC827) were chosen for evaluating the anticancer activity of the newly synthesized erlotinib derivatives. Three types of esophageal cancer cell lines KYSE70TR, KYSE410TR, and KYSE450TR which were resistant to paclitaxel, and NSCLC cell lines HCC827GR and H1650TR, which were resistant to gefitinib and paclitaxel, respectively, were also tested for the comparison purpose. The resistance indices of these cell lines are shown in Table 2.
At first, MTT experiments were carried out to study the cytotoxicity of 3a-3s against five cell lines using erlotinib as a control. The results expressed in IC50 values are shown in Table 3. KYSE410 cells were most sensitive to erlotinib with an IC50 value of 5.00 ± 0.46 μM. KYSE450 cells and two lung cancer cell lines (H1650 and HCC827) also showed some effects following erlotinib treatment, with IC50 values of 7.60 ± 0.51 μM, 14.00 ± 1.19 μM, and 11.81 ± 1.02 μM, respectively. The KYSE70 cell line was not very sensitive to erlotinib, but some erlotinib 1,2,3-triazole derivatives had good inhibitory activities against KYSE70 cells. For example, compounds 3a, 3b, 3c, 3d, 3g, 3j, 3m, and 3r showed IC50 values less than 10 μM, which were 5.85 ± 0.28 μM, 9.03 ± 0.82 μM, 5.35 ± 0.34 μM, 5.43 ± 0.21 μM, 5.46 ± 0.23 μM, 3.72 ± 0.33 μM, 3.92 ± 0.15 μM, and 5.85 ± 0.52 μM, respectively. Compounds with IC50 values less than 10 μM against KYSE410 cells included 3a (9.70 ± 0.75 μM), 3d (6.91 ± 0.40 μM), 3m (5.85 ± 0.20 μM), and 3r (8.74 ± 0.74 μM). Compounds with IC50 values less than 10 μM against KYSE450 cells included 3a (5.27 ± 0.31 μM), 3c (5.47 ± 0.29 μM), 3d (4.23 ± 0.19 μM), 3e (6.25 ± 0.35 μM), 3j (3.60 ± 0.27 μM), 3m (4.05 ± 0.21 μM), and 3r (8.20 ± 0.67 μM). Compounds with IC50 values less than 10 μM against H1650 cells included 3c (5.72 ± 0.33 μM), 3d (2.99 ± 0.13 μM), 3j (4.98 ± 0.17 μM), and 3m (4.27 ± 0.19 μM). Compounds with IC50 values less than 10 μM against HCC827 cells included 3d (8.17 ± 0.42 μM), 3j (6.27 ± 0.42 μM), 3k (6.18 ± 0.61 μM), 3l (4.61 ± 0.28 μM), and 3m (8.44 ± 0.37 μM). These preliminary results suggested that compounds 3d and 3m showed good inhibitory activities against these tumor cell lines with IC50 values less than 10 μM. Compound 3d was more suitable for further study due to the easy availability of the key azide raw material. The human esophageal epithelial cell, namely SHEE, was chosen for MTT assay to evaluate the toxicity of the compound against normal cells. As shown in Table 2, SHEE showed poor sensitivity to 3a, 3d, 3e, 3m, 3p, and 3r, with IC50 values of over 50 μM. These preliminary results suggested that compounds 3d, 3m, and 3r may be used as lead compounds for the development of chemotherapeutic agents for esophageal cancer. Compound 3d was chosen for further study due to its good performance and easier preparation.
Next, 3d and erlotinib were tested for their activity against three drug-resistant esophageal cancer cell lines (KYSE70TR, KYSE410TR, and KYSE450TR) and two drug-resistant lung cancer cell lines (H1650TR and HCC827GR). The results expressed by IC50 values are shown in Table 4. The preliminary results suggested that the inhibitory effect of erlotinib on drug-resistant tumor cells was not significant and the IC50 values were over 10 μM for all cases. In contrast, 3d showed good inhibitory effect on five drug-resistant tumor cell lines with IC50 values of 7.17 ± 0.73 μM, 7.91 ± 0.61 μM, 10.02 ± 0.75 μM, 5.76 ± 0.33 μM, and 2.38 ± 0.17 μM, respectively.
TABLE 4. Anti-proliferative activities of compounds 3d and erlotinib against drug-resistant cancer cell lines.
Plate clone experiments of 3d and erlotinib were also carried out to study the tumor response (Von Hoff et al., 1980), and the results similar to MTT experiments were observed (Figure 3). The inhibition effects of 3d on both cancer cell lines and the corresponding drug-resistant ones were more significant than that of erlotinib.
FIGURE 3. Plate clone results of 3d and erlotinib. (A) and (B) The proliferation inhibitionof 3d and erlotinib on KYSE450 and KYSE450TRcells. (C) and (D) The proliferation inhibitionof 3d and erlotinibon HCC827 and HCC827GRcells. Unpaired Student’s t test was used in Plate clone. *p< 0.05, **p< 0.01, ***p< 0.001. Error bars represent the mean ± SD.
To clarify whether the inhibitory effects of these compounds on cell proliferation were related to apoptosis, compound 3d, which showed strong inhibitory effects on the proliferation of esophageal cancer cell lines, were chosen for further study. KYSE450 and KYSE450T cells were treated with DMSO or different concentrations of 3d and erlotinib for 48 h, the cells were stained with Annexin V and PI, and the proportion of apoptotic cells was detected with flow cytometry. The results are shown in Figure 4.
FIGURE 4. Cell apoptosis induced by compound 3d and erlotinib. (A) Cell apoptosis induced by compound 3d in KYSE450 cell and KYSE450TR cell, compared with cells treated with 0.1% DMSO; (B) Cell apoptosis induced by erlotinib in KYSE450 cell and KYSE450TR cell,compared with cells treated with 0.1% DMSO. Unpaired Student’s t test was used inCell apoptosis. *p< 0.05, **p< 0.01, **p< 0.001. Error bars represent the mean ± SD.
Results in Figure 4A showed that the proportion of KYSE450 apoptotic cells treated with 3d was 24.50% (5 μM) and 69.64% (10 μM), while the proportion of KYSE450TR apoptotic cells treated with 3d was 44.12% (5 μM) and 48.77% (10 μM). Results in Figure 4B showed that the proportion of KYSE450 apoptotic cells treated with erlotinib was 7.04% (5 μM) and 8.94% (10 μM), while the proportion of KYSE450TR apoptotic cells treated with erlotinib was 10.16% (5 μM) and 16.68% (10 μM). These preliminary results suggested that compound 3d could induce apoptosis of esophageal cancer KYSE450 cells and drug-resistant KYSE450TR cells in a concentration-dependent manner, and the performance of compound 3d on these two cells was better than that of erlotinib.
To investigate whether the mechanism of 3d-induced KYSE450 cell apoptosis was related to mitochondrial apoptosis, protein electrophoresis was carried out to measure the protein levels of apoptosis-related marker proteins caspase-3, cytochrome-c, and PARP. The results are presented in Figure 5.
KYSE450 cells were treated with 3d for 6, 12, and 24 h, respectively. Analysis of total cell proteins showed that in KYSE450 cells, the caspase-3 and cytochrome-c protein levels of 3d (4 μM) group were higher than those in the control at 0 h after administration. The results suggest that 3d can regulate KYSE450 cell apoptosis through the mitochondrial pathway. The cleaved PARP protein levels of the 3d group were higher than those of the control at 0 h after administration, suggesting that compound 3d may regulate KYSE450 apoptosis through DNA injury.
To assess whether the inhibitory effect of these compounds on the proliferation of esophageal cancer cells was related to cell cycle arrest, cancer cells were treated with DMSO or different concentrations of 3d and erlotinib. Then, the cell cycle phases were evaluated with flow cytometry (Figure 6). In comparison with the control, the 3d group showed that the ratio of KYSE450 cells in the G0/G1 phase increased after 24 h with the increase in concentration (3.5, 7, 14, and 28 μM) in comparison with the control. However, the change in the ratio of S-phase cells and the ratio of G2/M-phase cells were not remarkable. When the cells were treated with erlotinib, the ratio of KYSE450 cells in the G0/G1 phase increased significantly at 3.5 μM and increased further with the concentration (3.5, 7, 14, and 28 μM) but did not change significantly with the further increase in concentration. The ratio of S-phase cells and the ratio of G2/M-phase cells decreased significantly at 3.5 μM but did not change significantly when the concentration was further increased. Therefore, both compound 3d and erlotinib inhibited KYSE450 cells in the G0/G1 phase.
FIGURE 6. KYSE450 cell cycle arrests induced by compound 3d and erlotinib in comparison with the cells treated with 0.1% DMSO.
For the drug resistance cell line KYSE450TR, the cell cycle results showed that the ratio of KYSE450TR cells in the G0/G1 phase increased after treatment with compound 3d for 24 h with the increase in concentration (3.5, 7, 14, and 28 μM) in comparison with the control (Figure 7). However, the ratio of S-phase cells significantly reduced, and the ratio of G2/M-phase cells increased in a concentration-dependent manner. When cells were treated with erlotinib, the ratio of KYSE450TR cells in the G0/G1 phase increased significantly at 3.5 μM and further increased with concentration (3.5, 7, 14, and 28 μM) but did not change significantly when the concentration was further increased. The ratio of S-phase cells decreased significantly at 3.5 μM, and the change was significant with the increase in concentration. The ratio of cells in the G2/M phase increased to a small degree with the increase in concentration. Therefore, compound 3d inhibited KYSE450TR cells in the G2/M phase, whereas erlotinib arrested KYSE450TR cells in the G0/G1 phase.
FIGURE 7. Cell cycle arrests induced by compound 3d and erlotinib in KYSE450TR, compared with the cells treated with 0.1% DMSO.
To investigate whether the mechanism of 3d suppressing cancer cell proliferation was related to the EGFR-TK pathway, surface plasmon resonance (SPR) experiments were carried out to study the interaction between 3d and erlotinib with the EGFR. The results are presented in Figure 8. As these results showed, 3d can bind to the EGFR wild-type protein (H672-E410) and EGFR mutant protein (672-1210, L858R) with a KD(M) value of 6.88 × 10−6 and 3.12 × 10−6, respectively. The KD(M) value for erlotinib and EGFR was 1.97 × 10−6. These preliminary results also suggested that 3d suppressed cancer cells proliferation through the EGFR-TK pathway.
FIGURE 8. Surface plasmon resonance experiments of 3d and erlotinib. (A) Binding sensorgrams for 3d interaction with immobilized wild-type of EGFR. The KD(M) value between 3d and wild-type EGFR is 6.88 × 10−6. (B) Binding sensorgrams for 3d interaction with immobilized mutant protein of EGFR. The KD(M) value between 3d and mutant protein EGFR is 3.12 × 10−6. (C) Binding sensorgrams for erlotinib interaction with immobilized wild-type of EGFR. The KD(M) value between erlotinib and wild-type EGFR is 1.97 × 10−6.
In summary, erlotinib derivatives bearing 1,2,3-triazole moieties were designed, synthesized, and evaluated for their activity against different cancer cell lines. Several of these compounds exhibited remarkable antitumor activity than erlotinib against one or more cancer cell lines. Among these compounds, 3d demonstrated good cytotoxicity against all ten cancer cell lines. The underlying mechanisms of 3d-induced cancer cell deaths were mitochondrial apoptosis and cell cycle arrest. In addition, a preliminary study on the interaction between 3d and EGFR suggested that 3d can bind to both wild-type protein (H672-E410) and mutant protein (672-1210, L858R). Taking these results together, erlotinib–1,2,3-triazole derivative 3d could induce apoptosis and arrest cell cycle, and the combination of erlotinib and 1,2,3-triazole might be a successful strategy for the development of new EGFR inhibitors for cancer therapy.
All reagents and solvents were obtained from commercially available sources and were used as received. 1H NMR and 13C NMR spectra were acquired in DMSO-d6 solution using a Bruker 600 spectrometer. Chemical shifts (δ) were given in parts per million with tetramethylsilane as internal reference. Coupling constants were expressed in hertz. High-resolution mass spectra (HRMS) measurements were carried out using a Bruker MicrOTOF-Q II mass spectrometer.
Compound 2 (3 g, 0.01 mol) was suspended in isopropanol alcohol (50 ml). 3-Aminophenylacetylene (1.2 g, 0.01 mol) was added to the solution. The suspension was stirred at 85°C for 6 h under nitrogen. Solid gradually formed, and the course of the reaction was monitored with TLC. After the completion of the reaction, the reaction mixture was transferred to ice water, and the mixture was stirred for half an hour. The solid was collected by filtration and was washed twice with isopropanol (30 ml) to give 2.1 g of erlotinib. 1H NMR (600 MHz, DMSO-d6): δ 9.48 (s, 1H, NH), 8.51 (s, 1H, CH), 8.00 (s, 1H, Ar-H), 7.91 (d, J = 9.5 Hz, 1H, Ar-H), 7.87 (s, 1H, Ar-H), 7.41 (t, J = 7.9 Hz, 1H, Ar-H), 7.27–7.17 (m, 2H, Ar-H), 4.31–4.29 (m, 4H, CH2CH2), 4.21 (s, 1H, CH), 3.80–3.75 (m, 4H, CH2CH2), 3.38 (s, 3H, CH3), 3.36 (s, 3H, CH3); 13C NMR (150 MHz, DMSO-d6): 156.6, 154.1, 153.2, 148.6, 147.4, 140.2, 129.3, 126.8, 125.2, 123.0, 122.2, 109.3, 108.6, 103.6, 83.9, 81.0, 70.5, 70.5, 68.8, 68.5, 58.8, and 58.8; HRMS (ESI)m/z: calcd for C22H23O4N3Na (M + Na)+ 416.1581, found 416.1585.
Aryl azide (1.2 mmol) and erlotinib (1.0 mmol) were added to 30 ml of mixed solvent (water: t-butanol = 2:1). The reaction was carried out in the presence of cuprous iodide (0.1 mmol) at 80°C. After completion of the reaction (monitored by TLC), the mixture was extracted with dichloromethane (20 ml × 3). The combined organic phase was washed successively with water and brine, dried over sodium sulfate, and concentrated in vacuo. The residue was purified through column chromatography (VCH2Cl2/VMeOH = 30:1) to give the desired 3a-3s (Figure 9).
FIGURE 9. Synthetic routes to erlotinib–1,2,3-triazole derivatives. Conditions: (A) isopropanol alcohol, 85°C for 6 h, and (B) CuI, 80°C.
Purity 99%; m. p. 89–92°C; 1H NMR (600 MHz, DMSO-d6): δ 9.56 (s, 1H, NH), 8.67 (s, 1H, CH), 8.49 (s, 1H, CH), 8.27 (s, 1H, Ar-H), 7.95–7.86 (m, 2H, Ar-H), 7.56 (d, J = 7.7 Hz, 1H, Ar-H), 7.51–7.28 (m, 6H, Ar-H), 7.24 (s, 1H, Ar-H), 5.67 (s, 2H, CH2), 4.33–4.29 (m, 4H, CH2CH2), 3.81–3.75 (m, 4H, CH2CH2), 3.39 (s, 3H, CH3), 3.36 (s, 3H, CH3). 13C NMR (150 MHz, DMSO-d6): 156.8, 154.0, 153.4, 148.5, 147.4, 147.1, 140.5, 136.5, 131.3, 129.4, 129.3, 128.6, 128.4, 122.2, 122.1, 120.7, 119.2, 109.4, 108.6, 103.6, 70.6, 70.5, 68.8, 68.5, 58.8, 58.8, 53.5; HR MS (ESI) m/z: calcd for C29H30O4N6Na (M + Na)+ 549.2221, found 549.2231.
Purity 98%; m. p. 93–96°C; 1H NMR (600 MHz, DMSO-d6): δ 9.63 (s, 1H, NH), 8.64 (s, 1H, CH), 8.54 (s, 1H, CH), 8.32 (s, 1H, Ar-H), 8.06–7.90 (m, 3H, Ar-H), 7.63 (d, J = 7.7 Hz, 1H, Ar-H), 7.50 (dd, J = 16.4, 8.0 Hz, 2H, Ar-H), 7.28 (s, 1H, Ar-H), 7.20 (dd, J = 11.8, 7.6 Hz, 2H, Ar-H), 5.75 (s, 2H, CH2), 4.37–4.34 (m, 4H, CH2CH2), 3.85–3.80 (m, 4H, CH2CH2), 3.43 (s, 3H, CH3), 3.41 (s, 3H, CH3). 13C NMR (150 MHz, DMSO-d6): 156.8, 154.0, 153.3, 148.5, 147.3, 146.9, 140.5, 140.0, 138.3, 131.3, 130.8, 130.1, 129.4, 129.3, 122.5, 122.3, 120.8, 119.3, 109.4, 108.5, 103.7, 99.7, 70.5, 70.5, 68.8, 68.5, 58.8, 58.8, 58.0; HR MS (ESI) m/z: calcd for C29H29O4N6INa (M + Na)+ 675.1187, found 675.1196.
Purity 99%; m. p. 94–97°C; 1H NMR (600 MHz, DMSO-d6): δ 9.60 (s, 1H, NH), 8.63 (s, 1H, CH), 8.50 (s, 1H, CH), 8.27 (s, 1H, Ar-H), 7.98–7.84 (m, 2H, Ar-H), 7.72 (d, J = 7.9 Hz, 1H, Ar-H), 7.58 (d, J = 7.7 Hz, 1H, Ar-H), 7.46 (dt, J = 11.5, 7.7 Hz, 2H, Ar-H), 7.34 (t, J = 8.3 Hz, 1H, Ar-H), 7.29–7.16 (m, 2H, Ar-H), 5.76 (s, 2H, CH2), 4.33–4.29 (m, 4H, CH2CH2), 3.81–3.75 (m, 4H, CH2CH2), 3.38 (s, 3H, CH3), 3.36 (s, 3H, CH3). 13C NMR (150 MHz, DMSO-d6): 156.8, 154.1, 153.3, 148.5, 147.2, 146.9, 140.4, 135.2, 133.4, 131.3, 131.0, 129.9, 129.5, 128.8, 123.3, 122.5, 122.4, 120.9, 119.3, 109.4, 108.5, 103.7, 87.3, 70.6, 70.5, 68.8, 68.5, 58.8, 58.8, 53.6; HR MS (ESI) m/z: calcd for C29H29O4N6BrNa (M + Na)+ 627.1331, found 627.1336.
Purity 98%; m. p. 102–105°C; 1H NMR (600 MHz, DMSO-d6): δ 9.58 (s, 1H, NH), 8.72 (s, 1H, CH), 8.49 (s, 1H, CH), 8.28 (s, 1H, Ar-H), 7.99–7.89 (m, 2H, Ar-H), 7.86 (s, 1H, Ar-H), 7.64 (s, 2H, Ar-H), 7.57 (d, J = 7.6 Hz, 1H, Ar-H), 7.47 (t, J = 7.9 Hz, 1H, Ar-H), 7.24 (s, 1H, Ar-H), 5.70 (s, 2H, CH2), 4.33–4.29 (m, 4H, CH2CH2), 3.81–3.75 (m, 4H, CH2CH2), 3.39 (s, 3H, CH3), 3.36 (s, 3H, CH3); 13C NMR (150 MHz, DMSO-d6): 156.8, 154.0, 153.3, 148.5, 147.4, 147.2, 140.8, 140.5, 133.7, 131.2, 130.6, 129.5, 123.2, 122.4, 120.8, 119.3, 109.4, 108.6, 103.7, 70.6, 70.5, 68.8, 68.5, 58.8, 58.8, 52.0; HR MS(ESI) m/z: calcd for C29H29O4N6Br2Na (M + Na)+ 683.0612, found 683.0624.
Purity 99%; m. p. 85–88°C; 1H NMR (600 MHz, DMSO-d6): δ 9.61 (s, 1H, NH), 8.66 (s, 1H, CH), 8.27 (s, 1H, CH), 8.01 (s, 1H, Ar-H), 7.91 (d, J = 7.9 Hz, 1H, Ar-H), 7.57 (d, J = 7.6 Hz, 1H, Ar-H), 7.46 (t, J = 7.9 Hz, 1H, Ar-H), 7.32 (t, J = 7.9 Hz, 2H, Ar-H), 6.97 (s, 1H, Ar-H), 6.93 (d, J = 7.9 Hz, 2H, Ar-H), 5.63 (s, 2H, CH2), 4.33–4.31 (m, 4H, CH2CH2), 3.80–3.76 (m, 4H, CH2CH2), 3.76 (s, 3H, OCH3), 3.39 (s, 3H, CH3), 3.37 (s, 3H, CH3).13C NMR (150 MHz, DMSO-d6): 159.9, 156.7, 154.0, 148.6, 147.0, 140.4, 137.9, 131.4, 130.4, 129.5, 122.3, 122.1, 120.8, 120.5, 119.3, 114.2, 113.9, 108.9, 103.8, 87.7, 70.6, 70.5, 68.8, 68.5, 58.8, 58.8, 55.6, 53.4, 22.5; HR MS(ESI) m/z: calcd for C30H33O5N6 (M + H)+ 557.2512, found 557.2508.
Purity 98%; m. p. 83–86°C; 1H NMR (600 MHz, DMSO-d6): δ 9.62 (s, 1H, NH), 9.11 (s, 1H, CH), 8.51 (s, 1H, CH), 8.39 (s, 1H, Ar-H), 8.04–7.86 (m, 3H, Ar-H), 7.73–7.59 (m, 3H, Ar-H), 7.56–7.46 (m, 2H, Ar-H), 7.25 (s, 1H, Ar-H), 4.34–4.30 (m, 4H, CH2CH2), 3.82–3.76 (m, 4H, CH2CH2), 3.39 (s, 3H, CH3), 3.37 (s, 3H, CH3). 13C NMR (150 MHz, DMSO-d6): 156.8, 155.2, 154.0, 153.4, 148.5, 147.4, 140.6, 131.8, 130.7, 129.6, 126.5, 126.0, 123.4, 122.7, 121.0, 119.4, 117.7, 117.6, 109.4, 108.6, 103.7, 70.6, 70.5, 68.8, 68.5, 58.8, 58.8; HR MS (ESI) m/z: calcd for C28H27O4N6FNa (M + Na)+ 553.1970, found 553.1979.
Purity 97%; m. p. 88–91°C; 1H NMR (600 MHz, DMSO-d6): δ 9.73 (s, 1H, NH), 9.32 (s, 1H, CH), 8.57 (s, 1H, CH), 8.38 (s, 1H, Ar-H), 8.14–7.86 (m, 4H, Ar-H), 7.67 (d, J = 7.6 Hz, 1H, Ar-H), 7.52 (dt, J = 12.5, 8.3 Hz, 3H, Ar-H), 7.26 (s, 1H, Ar-H), 4.34–4.30 (m, 4H, CH2CH2), 3.82–3.76 (m, 4H, CH2CH2), 3.39 (s, 3H, CH3), 3.37 (s, 3H, CH3); 13C NMR (150 MHz, DMSO-d6): 162.9, 161.3, 156.9, 156.9, 154.2, 153.1, 148.6, 147.8, 140.4, 133.7, 130.9, 129.6, 122.8, 122.8, 120.4, 119.6, 117.3, 117.2, 108.3, 103.7, 70.5, 70.5, 68.8, 68.5, 58.8, 58.8; HR MS(ESI) m/z: calcd for C28H27O4N6FNa (M + Na)+ 553.1970, found 553.1979.
Purity 99%; m. p. 131–134°C; 1H NMR (600 MHz, DMSO-d6): δ 9.62 (s, 1H, NH), 9.08 (s, 1H, CH), 8.51 (s, 1H, CH), 8.40 (s, 1H, Ar-H), 7.94 (d, J = 10.6 Hz, 2H, Ar-H), 7.85–7.79 (m, 2H, Ar-H), 7.70–7.61 (m, 3H, Ar-H), 7.52 (t, J = 7.9 Hz, 1H, Ar-H), 7.25 (s, 1H, Ar-H), 4.34–4.30 (m, 4H, CH2CH2), 3.81–3.76 (m, 4H, CH2CH2), 3.39 (s, 3H, CH3), 3.37 (s, 3H, CH3); 13C NMR (150 MHz, DMSO-d6): 156.8, 154.0, 153.4, 148.5, 147.4, 146.9, 140.6, 135.0, 131.0, 130.8, 129.6, 129.1, 129.0, 128.9, 124.1, 122.6, 121.0, 119.4, 109.4, 108.6, 103.7, 70.6, 70.5, 68.8, 68.5, 58.8, 58.8; HR MS(ESI) m/z: calcd for C28H27O4N6ClNa (M + Na)+ 569.1675, found 569.1678.
Purity 97%; m. p. 93–97°C; 1H NMR (600 MHz, DMSO-d6): δ 9.63 (s, 1H, NH), 9.05 (s, 1H, CH), 8.51 (s, 1H, CH), 8.40 (s, 1H, Ar-H), 8.00–7.89 (m, 3H, Ar-H), 7.77 (dd, J = 7.8, 1.5 Hz, 1H, Ar-H), 7.67 (t, J = 7.7 Hz, 2H, Ar-H), 7.60 (t, J = 8.6 Hz, 1H, Ar-H), 7.52 (t, J = 7.9 Hz, 1H, Ar-H), 7.25 (s, 1H, Ar-H), 4.34–4.30 (m, 4H, CH2CH2), 3.81–3.76 (m, 4H, CH2CH2), 3.39 (s, 3H, CH3), 3.37 (s, 3H, CH3); 13C NMR (150 MHz, DMSO-d6): 156.8, 154.1, 153.3, 148.5, 147.4, 146.9, 140.6, 136.7, 134.1, 132.5, 130.9, 129.6, 129.4, 129.2, 124.2, 122.6, 121.0, 119.4, 109.4, 108.6, 103.7, 100.0, 70.6, 70.5, 68.8, 68.5, 58.8, 58.8; HR MS(ESI) m/z: calcd for C28H27O4N6BrNa (M + Na)+ 613.1169, found 613.1180.
Purity 98%; m. p. 105–108°C; 1H NMR (600 MHz, DMSO-d6): δ 9.63 (s, 1H, NH), 9.37 (s, 1H, CH), 8.51 (s, 1H, CH), 8.38 (s, 1H, Ar-H), 7.96 (dd, J = 16.5, 7.6 Hz, 4H, Ar-H), 7.86 (d, J = 8.8 Hz, 2H, Ar-H), 7.66 (d, J = 7.6 Hz, 1H, Ar-H), 7.53 (t, J = 7.9 Hz, 1H, Ar-H), 7.25 (s, 1H, Ar-H), 4.34–4.30 (m, 4H, CH2CH2), 3.82–3.76 (m, 4H, CH2CH2), 3.39 (s, 3H, CH3), 3.37 (s, 3H, CH3); 13C NMR (150 MHz, DMSO-d6): 156.8, 154.0, 153.4, 148.5, 147.9, 147.4, 140.6, 136.3, 133.3, 130.8, 129.6, 122.7, 122.3, 121.8, 121.0, 120.1, 119.4, 109.4, 108.6, 103.6, 70.6, 70.5, 68.8, 68.5, 58.8, 58.8; HR MS(ESI) m/z: calcd for C28H27O4N6BrNa (M + Na)+ 613.1169, found 613.1177.
Purity 98%; m. p. 87–90°C; 1H NMR (600 MHz, DMSO-d6): δ 9.62 (s, 1H, NH), 8.94 (s, 1H, CH), 8.50 (s, 1H, CH), 8.36 (s, 1H, Ar-H), 7.98–7.91 (m, 2H, Ar-H), 7.69 (dd, J = 21.0, 7.7 Hz, 2H, Ar-H), 7.58 (t, J = 7.9 Hz, 1H, Ar-H), 7.50 (t, J = 7.9 Hz, 1H, Ar-H), 7.36 (d, J = 8.2 Hz, 1H, Ar-H), 7.25 (s, 1H, Ar-H), 7.19 (t, J = 7.6 Hz, 1H, Ar-H), 4.34–4.30 (m, 4H, CH2CH2), 3.90 (s, 3H, OCH3), 3.82–3.76 (m, 4H, CH2CH2), 3.39 (s, 3H, CH3), 3.37 (s, 3H, CH3); 13C NMR (150 MHz, DMSO-d6): 156.8, 154.0, 153.4, 152.3, 148.5, 147.4, 146.6, 140.5, 131.3, 131.2, 129.5, 126.4, 126.2, 123.9, 122.5, 121.3, 121.0, 119.4, 113.4, 109.4, 108.6, 103.7, 68.8, 68.5, 58.8, 58.5, 56.6; HR MS(ESI) m/z: calcd for C29H30O5N6Na (M + Na)+ 565.2170, and found 565.2172.
Purity 97%; m. p. 95–98°C; 1H NMR (600 MHz, DMSO-d6): δ 9.63 (s, 1H, NH), 9.28 (s, 1H, CH), 8.51 (s, 1H, CH), 8.37 (s, 1H, Ar-H), 7.95 (d, J = 9.9 Hz, 2H, Ar-H), 7.87 (d, J = 8.3 Hz, 2H, Ar-H), 7.67 (d, J = 7.6 Hz, 1H, Ar-H), 7.52 (t, J = 7.9 Hz, 1H, Ar-H), 7.45 (d, J = 8.3 Hz, 2H, Ar-H), 7.25 (s, 1H, Ar-H), 4.34–4.30 (m, 4H, CH2CH2), 3.82–3.76 (m, 4H, CH2CH2), 3.39 (s, 3H, CH3), 3.37 (s, 3H, CH3), 2.51 (s, 3H, CH3); 13C NMR (150 MHz, DMSO-d6): 156.8, 154.0, 153.4, 148.5, 147.7, 147.4, 140.5, 138.8, 134.8, 131.0, 130.7, 129.5, 122.6, 121.0, 120.3, 120.0, 119.4, 109.4, 108.6, 103.6, 70.6, 70.5, 68.8, 68.5, 58.8, 58.8, 21.0; HR MS(ESI) m/z: calcd for C29H31O4N6 (M + H)+ 527.2401, found 527.2410.
Purity 99%; m. p. 98–101°C; 1H NMR (600 MHz, DMSO-d6): δ 9.64 (s, 1H, NH), 9.60 (s, 1H, CH), 8.83 (t, J = 2.1 Hz, 1H, CH), 8.50 (d, J = 12.4 Hz, 2H, Ar-H), 8.41 (s, 1H, Ar-H), 8.37 (d, J = 7.5 Hz, 1H, Ar-H), 7.99–7.94 (m, 3H, Ar-H), 7.69 (d, J = 7.8 Hz, 1H, Ar-H), 7.55 (t, J = 7.9 Hz, 1H, Ar-H), 7.25 (s, 1H, Ar-H), 4.34–4.30 (m, 4H, CH2CH2), 3.82–3.76 (m, 4H, CH2CH2), 3.39 (s, 3H, CH3), 3.37 (s, 3H, CH3); 13C NMR (150 MHz, DMSO-d6): 156.8, 154.0, 153.4, 149.0, 148.5, 147.4, 140.6, 137.7, 132.0, 130.6, 129.6, 126.4, 123.6, 122.9, 121.0, 120.6, 119.5, 115.0, 109.4, 108.6, 103.6, 70.6, 70.5, 68.8, 68.5, 58.8, 58.8; HR MS(ESI) m/z: calcd for C28H27O6N7Na (M + Na)+ 580.1915, found 580.1923.
Purity 98%; m. p. 110–114°C; 1H NMR (600 MHz, DMSO-d6): δ 9.63 (s, 1H, NH), 9.36 (s, 1H, CH), 8.51 (s, 1H, CH), 8.37 (s, 1H, Ar-H), 7.95 (d, J = 10.1 Hz, 2H, Ar-H), 7.67 (d, J = 7.7 Hz, 1H, Ar-H), 7.55 (d, J = 36.4 Hz, 4H, Ar-H), 7.25 (s, 1H, Ar-H), 7.08 (d, J = 10.2 Hz, 1H, Ar-H), 4.34–4.30 (m, 4H, CH2CH2), 4.17 (q, J = 7.0 Hz, 2H, CH2), 3.82–3.76 (m, 4H, CH2CH2), 3.39 (s, 3H, CH3), 3.37 (s, 3H, CH3), 1.39 (t, J = 7.0 Hz, 3H, CH3); 13C NMR (150 MHz, DMSO-d6): 159.9, 156.8, 154.0, 153.4, 148.5, 147.8, 147.4, 140.6, 138.1, 131.3, 130.9, 129.5, 122.7, 121.0, 120.1, 119.4, 115.2, 112.2, 109.4, 108.6, 106.4, 103.6, 70.6, 70.5, 68.8, 68.5, 64.1, 58.8, 58.8, 15.0; HR MS(ESI) m/z: calcd for C30H32O5N6Na (M + Na)+ 579.2326, found 579.2332.
Purity 96%; m. p. 137–140°C; 1H NMR (600 MHz, DMSO-d6): δ 9.63 (s, 1H, NH), 9.34 (s, 1H, CH), 8.50 (s, 1H, CH), 8.38 (s, 1H, Ar-H), 7.99 (d, J = 7.6 Hz, 2H, Ar-H), 7.94 (d, J = 9.8 Hz, 2H, Ar-H), 7.66 (dd, J = 16.3, 8.6 Hz, 3H, Ar-H), 7.53 (d, J = 18.2 Hz, 2H, Ar-H), 7.24 (s, 1H, Ar-H), 4.34–4.29 (m, 4H, CH2CH2), 3.81–3.76 (m, 4H, CH2CH2), 3.39 (s, 3H, CH3), 3.36 (s, 3H, CH3); 13C NMR (150 MHz, DMSO-d6): 156.8, 154.0, 153.4, 148.5, 147.8, 147.4, 140.6, 137.1, 130.9, 130.4, 129.5, 129.2, 122.7, 121.0, 120.4, 120.1, 119.4, 109.4, 108.6, 103.6, 70.6, 70.5, 68.8, 68.5, 58.8, 58.8; HR MS(ESI) m/z: calcd for C28H28O4N6Na (M + Na)+ 535.2064, found 535.2069.
Purity 97%; m. p. 113–116°C; 1H NMR (400 MHz, DMSO-d6): δ 9.62 (s, 1H, NH), 9.05 (s, 1H, CH), 8.51 (s, 1H, CH), 8.39 (s, 1H, Ar-H), 8.08 (d, J = 7.1 Hz, 1H, Ar-H), 7.93 (d, J = 61.3 Hz, 5H, Ar-H), 7.65 (d, J = 7.8 Hz, 1H, Ar-H), 7.52 (t, J = 7.9 Hz, 1H, Ar-H), 7.24 (s, 1H, Ar-H), 4.34–4.29 (m, 4H, CH2CH2), 3.81–3.75 (m, 4H, CH2CH2), 3.39 (s, 3H, CH3), 3.37 (s, 3H, CH3); 13C NMR (100 Hz, DMSO-d6): 156.8, 154.0, 153.3, 148.5, 147.3, 146.9, 140.6, 134.5, 131.7, 130.7, 129.8, 129.6, 127.9, 125.5, 125.2, 124.7, 122.7, 121.9, 121.0, 119.4, 109.3, 108.5, 103.5, 70.5, 70.5, 68.7, 68.4, 58.8, 58.8; HR MS (ESI) m/z: calcd for C29H27O4N6F3Na (M + Na)+ 603.1938, found 603.1945.
Purity 98%; m. p. 85–88°C; 1H NMR (400 MHz, DMSO-d6): δ 9.66 (s, 1H, NH), 8.88 (s, 1H, CH), 8.55 (s, 1H, CH), 8.39 (s, 1H, Ar-H), 8.02–7.92 (m, 2H, Ar-H), 7.70 (d, J = 7.8 Hz, 1H, Ar-H), 7.63 (d, J = 8.7 Hz, 1H, Ar-H), 7.54 (t, J = 7.9 Hz, 1H, Ar-H), 7.29 (s, 1H, Ar-H), 6.92 (d, J = 2.5 Hz, 1H, Ar-H), 6.78 (dd, J = 8.8, 2.6 Hz, 1H, Ar-H), 4.39–4.34 (m, 4H, CH2CH2), 3.93 (s, 3H, OCH3), 3.92 (s, 3H, OCH3), 3.87–3.80 (m, 4H, CH2CH2), 3.44 (s, 3H, CH3), 3.42 (s, 3H, CH3); 13C NMR (100 MHz, DMSO-d6): 161.7, 156.8, 154.0, 153.7, 153.3, 148.5, 146.4, 140.5, 131.2, 129.4, 127.4, 124.0, 122.4, 120.9, 119.6, 119.3, 108.6, 105.7, 103.6, 99.9, 70.5, 70.5, 68.7, 68.4, 58.8, 58.8, 56.6, 56.1; HR MS (ESI) m/z: calcd for C30H32O6N6Na (M + Na)+ 595.2276, found 595.2285.
Purity 98%; m. p. 100–103°C; 1H NMR (400 MHz, DMSO-d6): δ 9.66 (s, 1H, NH), 8.98 (s, 1H, CH), 8.55 (s, 1H, CH), 8.40 (s, 1H, OH), 7.99 (d, J = 12.3 Hz, 2H, Ar-H), 7.73 (dd, J = 14.5, 7.8 Hz, 2H, Ar-H), 7.63 (t, J = 7.9 Hz, 1H, Ar-H), 7.55 (t, J = 7.9 Hz, 1H, Ar-H), 7.41 (d, J = 7.7 Hz, 1H, Ar-H), 7.29 (s, 1H, Ar-H), 7.24 (t, J = 7.6 Hz, 1H, Ar-H), 4.39–4.34 (m, 4H, CH2CH2), 3.95 (s, 3H, CH3), 3.87–3.80 (m, 4H, CH2CH2), 3.44 (s, 3H, CH3), 3.42 (s, 3H, CH3); 13C NMR (100 MHz, DMSO-d6): 156.8, 154.0, 153.3, 152.2, 148.5, 147.3, 146.6, 140.5, 131.4, 131.1, 129.5, 126.4, 126.1, 123.9, 122.5, 121.3, 121.0, 119.3, 113.4, 109.4, 108.5, 103.6, 70.5, 70.5, 68.7, 68.4, 58.8, 58.8, 56.6; HR MS (ESI) m/z: calcd for C29H30O5N6Na (M + Na)+ 565.2170, found 565.2175.
Purity 97%; m. p. 109–112°C; 1H NMR (600 MHz, DMSO-d6): δ 9.56 (s, 1H, NH), 8.53 (s, 1H, CH), 8.49 (s, 1H, CH), 8.24 (s, 1H, Ar-H), 7.93 (s, 1H, Ar-H), 7.89 (d, J = 8.9 Hz, 1H, Ar-H), 7.51 (d, J = 7.7 Hz, 1H, Ar-H), 7.45 (t, J = 7.8 Hz, 1H, Ar-H), 7.29 (t, J = 7.4 Hz, 2H, Ar-H), 7.22 (dd, J = 13.1, 6.9 Hz, 4H, Ar-H), 4.68 (t, J = 7.3 Hz, 2H, CH2), 4.33–4.29 (m, 4H,CH2CH2), 3.81–3.75 (m, 4H,CH2CH2), 3.38 (s, 3H, CH3), 3.36 (s, 3H, CH3), 3.24 (t, J = 7.3 Hz, 2H, CH2); 13C NMR (150 MHz, DMSO-d6): 156.8, 154.0, 153.3, 148.5, 147.4, 146.5, 140.5, 138.1, 131.5, 129.4, 129.1, 127.0, 122.1, 121.8, 120.6, 119.1, 109.4, 108.6, 103.6, 70.6, 70.5, 68.8, 68.5, 58.8, 58.8, 51.1, 36.0; HR MS(ESI) m/z: calcd for C30H32O4N6Na (M + Na)+ 563.2377, found 563.2381.
The human cancer cells H1650/H1650TR, HCC827/HCC827GR, KYSE70/KYSE70TR, KYSE410/KYSE410TR, and KYSE450/KYSE450TR were cultured in the RPMI-1640 complete growth medium containing 100 U/mL of penicillin–streptomycin and 10% FBS. The cells were incubated at 37°C with 5% of CO2. The compounds were dissolved in DMSO to make a 50 mM stock solution and were diluted to the concentration of working solutions with the complete growth medium before administration.
Cells were seeded in 96-well plates with densities of 2,200–2,500 cells/well in 100 μL. One day after seeding, the concentration of the test compounds between 0 and 50 μM, 0.1% DMSO was added to cells as control. Approximately 2200-2500 transfected cells in 100 μL were incubated in quintuplicate in 96-well plates. After 48 h, MTT was added and incubated in the plate for 1–4 h in the incubator. The absorbance at 490 nm was measured using a microplate reader (Thermo).
Cells were seeded in 6-well plates with a density of 200 cells/well and cultured overnight for attachment. The cells were exposed to 3d or erlotinib of various concentrations (0, 2.5, 5, and 10 µM) separately for 10 days. Medium with or without compounds was changed every 48 h. When colony formation was visible, the medium was discharged. Then, the colonies were washed with cold PBS, fixed with 4% paraformaldehyde (PFA) for at least 30 min, and then stained with 0.2% crystal violet solution in 100% ethanol for 20 min.
Cell apoptosis analysis was carried out by flow cytometry using the Annexin V/PI apoptosis methods. Briefly, KYSE450/KYSE450TR (2 × 104- 3×104/well) cells were incubated in 6-well plates for 48 h and then treated with 0.1% DMSO (as control), either compound 3d or erlotinib at various concentrations for 48 h, respectively. The cells were harvested and incubated with 250 μL of 1 × Annexin V binding buffer containing 5 μL of PI and 5 μL of FITC Annexin V (final concentration 1.8 μg/ml, Biolegend cat: 640945) for 15 min at room temperature in the dark. Then 200 µL of 1 × binding buffer was added for flow cytometry analysis (BD FACSCalibur™ Flow Cytometer).
KYSE450 cells (3 × 105/well) were incubated overnight in 10 square petri dishes and then treated with compound 3d at 4 μM for 0, 6, 12, and 24 h. Cells treated with 0.1% DMSO were used as control. Then, the cells were harvested, and total proteins were extracted. Total proteins were separated by 12% SDS polyacrylamide gel electrophoresis and transferred onto PVDF membranes. The membrane was blocked for 1 h, then incubated overnight with a 1:1000 dilution of anti-caspase-3, anti-cytochrome-c, and anti-PARP, or 1 : 3,000 dilution of anti-β-action primary antibody at 4°C. Finally, 1 : 3,000 anti-rabbit secondary antibodies were incubated for 2 h at room temperature. Protein bands were developed by chemiluminescence.
KYSE450 and KYSE450TR cells were plated in 6-well plates with a density of 1 × 105 cells/well and cultured overnight to attach. The cells were treated with 3d or erlotinib at different concentrations (0, 3.5, 7, 14, and 28 μM) for 24 h. After trypsinization treatment, the cells were collected by centrifugation. The cell pellet was re-suspended in 70% ethanol at −20°C for at least 3 h. The cells were washed with PBS and was re-suspended in 250 μL of 0.6% tricine with renease A for 1 h, then stained in PI (final concentration 1.8 μg/ml, Biolegend cat: 640945) for 15 min in the dark. Cells were re-transferred to the BD FACSCalibur™ Flow Cytometer. All analyses were performed with FlowJo software v105.3.6.
Surface plasmon resonance experiments were carried out to evaluate the interaction between 3d with the EGFR wild-type (0.468 μg/μL, Active MOTIF, cat: 31165) and the EGFR mutant type (672-1210, L858R, Active MOTIF, cat: 81200). The interaction of erlotinib with wild-type EGFR was also studied. Biacore T-200 (GE healthcare, Waukesha, WI, United States) equipment was used for the study. First, the EGFR wild-type (0.468 μg/μL, Active MOTIF, cat: 31165) and the EGFR mutant type (672-1210, L858R, Active MOTIF, cat: 81200) were covalently immobilized at densities of 2000 response units onto a CM5 sensor chip. Then, 3d dissolved in DMSO was injected at concentrations between 0.064 and 5,000 nM at 25°C. The final doses of DMSO did not exceed 1% (v/v). During the interaction, the changes in the refractive index were measured in real time to allow the plotting of the results of interaction as response units versus time. The interaction results were analyzed with BIA evaluation 3.0 software.
The original contributions presented in the study are included in the article/Supplementary Material; further inquiries can be directed to the corresponding authors.
All authors listed have made a substantial, direct, and intellectual contribution to the work and approved it for publication.
This study was supported by the Doctoral Foundation of Henan University of Science and Technology (No. 13480044, XC), and Tianjin Research Innovation Project for Postgraduate Students (No. 2019YJSB077, LM).
The authors declare that the research was conducted in the absence of any commercial or financial relationships that could be construed as a potential conflict of interest.
All claims expressed in this article are solely those of the authors and do not necessarily represent those of their affiliated organizations, or those of the publisher, the editors, and the reviewers. Any product that may be evaluated in this article, or claim that may be made by its manufacturer, is not guaranteed or endorsed by the publisher.
The Supplementary Material for this article can be found online at: https://www.frontiersin.org/articles/10.3389/fphar.2022.849364/full#supplementary-material
Andrews Wright, N. M., and Goss, G. D. (2019). Third-generation Epidermal Growth Factor Receptor Tyrosine Kinase Inhibitors for the Treatment of Non-small Cell Lung Cancer. Transl Lung Cancer Res. 8, S247–S264. doi:10.21037/tlcr.2019.06.01
Brand, F. X., Ravanel, N., Gauchez, A. S., Pasquier, D., Payan, R., Fagret, D., et al. (2006). Prospect for Anti-HER2 Receptor Therapy in Breast Cancer. Anticancer Res. 26, 463–470.
Chandrasekara Reddy, G., Chandregowda, V., and Venkateswara Rao, G. (2007c). One-Pot Conversion of 2-Nitrobenzonitriles to Quinazolin-4(3h)-Ones and Synthesis of Gefitinib and Erlotinib Hydrochloride. Heterocycles 71, 39–48. doi:10.3987/COM-06-10884
Chandregowda, V., Rao, G. V., and Reddy, G. C. (2007b). Improved Synthesis of Gefitinib and Erlotinib Hydrochloride‐ Anticancer Agents. Synth. Commun. 37, 3409–3415. doi:10.1080/00397910701483761
Chandregowda, V., Venkateswara Rao, G., and Chandrasekara Reddy, G. (2007a). Convergent Approach for Commercial Synthesis of Gefitinib and Erlotinib. Org. Process. Res. Dev. 11, 813–816. doi:10.1021/op700054p
Chen, S., He, Y., Liu, J., Chen, X., Yu, J., Li, W., et al. (2019). Third-Generation TKI Resistance Due to SCLC Transformation: A Case Report and Brief Review. Onco Targets Ther. 12, 11305–11311. doi:10.2147/ott.s228301
Chen, X., Wang, Y., Zhang, L., and Gao, Y. (2020). Hydroxysafflor Yellow A of Carthamus Tinctorius L., Represses the Malignant Development of Esophageal Cancer Cells via Regulating NF-Κb Signaling Pathway. Cell Biochem Biophys 78, 511–520. doi:10.1007/s12013-020-00934-1
Choi, M., Razzaque, S., and Kim, R. (2012). Esophageal Cancer, Bevacizumab, Erlotinib. Clin. Adv. Hematol. Oncol. H O 10, 430–437.
Chuang, J. C., Salahudeen, A. A., and Wakelee, H. A. (2016). Rociletinib, a Third Generation EGFR Tyrosine Kinase Inhibitor: Current Data and Future Directions. Expert Opin. Pharmacother. 17, 989–993. doi:10.1517/14656566.2016.1162786
Cohen, M. H., Williams, G. A., Sridhara, R., Chen, G., and Pazdur, R. (2003). FDA Drug Approval Summary: Gefitinib (ZD1839) (Iressa) Tablets. Oncologist 8, 303–306. doi:10.1634/theoncologist.8-4-303
Costantino, L., and Barlocco, D. (2006). Privileged Structures as Leads in Medicinal Chemistry. Curr. Med. Chem. 13, 65–85. doi:10.2174/092986706775197999
Culy, C. R., and Faulds, D. (2002). Gefitinib. Drugs 62, 2237–2248. doi:10.2165/00003495-200262150-00008
DeSimone, R. W., Currie, K. S., Mitchell, S. A., Darrow, J. W., and Pippin, D. A. (2004). Privileged Structures: Applications in Drug Discovery. Comb. Chem. High Throughput Screen. 7, 473–494. doi:10.2174/1386207043328544
Elkamhawy, A., Hassan, A. H. E., Paik, S., Sup Lee, Y., Lee, H. H., Shin, J. S., et al. (2019). EGFR Inhibitors from Cancer to Inflammation: Discovery of 4-Fluoro-N-(4-(3-(trifluoromethyl)phenoxy)pyrimidin-5-Yl)benzamide as a Novel Anti-inflammatory EGFR Inhibitor. Bioorg. Chem. 86, 112–118. doi:10.1016/j.bioorg.2019.01.017
Greig, S. L. (2016). Osimertinib: First Global Approval. Drugs 76, 263–273. doi:10.1007/s40265-015-0533-4
Hirsch, F. R., Scagliotti, G. V., Mulshine, J. L., Kwon, R., Curran, W. J., Wu, Y. L., et al. (2017). Lung Cancer: Current Therapies and New Targeted Treatments. Lancet 389, 299–311. doi:10.1016/s0140-6736(16)30958-8
Hong, V., Steinmetz, N. F., Manchester, M., and Finn, M. G. (2010). Labeling Live Cells by Copper-Catalyzed Alkyne-Aazide Click Chemistry. Bioconjug. Chem. 21, 1912–1916. doi:10.1021/bc100272z
Karlsen, E. A., Kahler, S., Tefay, J., Joseph, S. R., and Simpson, F. (2021). Epidermal Growth Factor Receptor Expression and Resistance Patterns to Targeted Therapy in Non-small Cell Lung Cancer: A Review. Cells 10, 1206. doi:10.3390/cells10051206
Kelloff, G. J., Fay, J. R., Steele, V. E., Lubet, R. A., Boone, C. W., Crowell, J. A., et al. (1996). Epidermal Growth Factor Receptor Tyrosine Kinase Inhibitors as Potential Cancer Chemopreventives. Cancer Epidemiol. Biomarkers Prev. 5, 657–666. doi:10.1097/00008469-199612002-00012
Kim, T. E., and Murren, J. R. (2002). Erlotinib OSI/Roche/Genentech. Curr. Opin. Investig. Drugs 3, 1385–1395.
Liao, B. C., Lin, C. C., and Yang, J. C. (2015). Second and Third-Generation Epidermal Growth Factor Receptor Tyrosine Kinase Inhibitors in Advanced Nonsmall Cell Lung Cancer. Curr. Opin. Oncol. 27, 94–101. doi:10.1097/cco.0000000000000164
Maione, P., Sacco, P. C., Sgambato, A., Casaluce, F., Rossi, A., and Gridelli, C. (2015). Overcoming Resistance to Targeted Therapies in NSCLC: Current Approaches and Clinical Application. Ther. Adv. Med. Oncol. 7, 263–273. doi:10.1177/1758834015595048
Mao, L., Sun, G., Zhao, J., Xu, G., Yuan, M., and Li, Y. M. (2020b). Design, Synthesis and Antitumor Activity of Icotinib Derivatives. Bioorg. Chem. 105, 104421. doi:10.1016/j.bioorg.2020.104421
Mao, L.-F., Xu, G.-Q., Sun, B., Jiang, Y.-Q., Dong, W.-P., Zhang, S.-T., et al. (2017). Design, Synthesis and Antibacterial Evaluation of Novel 1,2,3-Triazole Derivatives Incorporating 3′-Deoxythymidine. J. Chem. Res. 41, 645–649. doi:10.3184/174751917X15094552081189
Mao, L. F., Wang, Y. W., Zhao, J., Xu, G. Q., Yao, X. J., and Li, Y. M. (2020a). Discovery of Icotinib-1,2,3-Triazole Derivatives as Ido1 Inhibitors. Front. Pharmacol. 11, 579024. doi:10.3389/fphar.2020.579024
Mathew, M. P., Tan, E., Saeui, C. T., Bovonratwet, P., Liu, L., Bhattacharya, R., et al. (2015). Metabolic Glycoengineering Sensitizes Drug-Resistant Pancreatic Cancer Cells to Tyrosine Kinase Inhibitors Erlotinib and Gefitinib. Bioorg. Med. Chem. Lett. 25, 1223–1227. doi:10.1016/j.bmcl.2015.01.060
Mendelsohn, J. (1992). Epidermal Growth Factor Receptor as a Target for Therapy with Antireceptor Monoclonal Antibodies. J. Natl. Cancer Inst., 125–131.
Minari, R., Bordi, P., and Tiseo, M. (2016). Third-generation Epidermal Growth Factor Receptor-Tyrosine Kinase Inhibitors in T790M-Positive Non-small Cell Lung Cancer: Review on Emerged Mechanisms of Resistance. Transl Lung Cancer Res. 5, 695–708. doi:10.21037/tlcr.2016.12.02
Mukherji, D., and Spicer, J. (2009). Second-generation Epidermal Growth Factor Tyrosine Kinase Inhibitors in Non-small Cell Lung Cancer. Expert Opin. Investig. Drugs 18, 293–301. doi:10.1517/13543780902762843
Murtuza, A., Bulbul, A., Shen, J. P., Keshavarzian, P., Woodward, B. D., Lopez-Diaz, F. J., et al. (2019). Novel Third-Generation EGFR Tyrosine Kinase Inhibitors and Strategies to Overcome Therapeutic Resistance in Lung Cancer. Cancer Res. 79, 689–698. doi:10.1158/0008-5472.CAN-18-1281
Ou, S. H. (2012). Second-generation Irreversible Epidermal Growth Factor Receptor (EGFR) Tyrosine Kinase Inhibitors (TKIs): A Better Mousetrap? A Review of the Clinical Evidence. Crit. Rev. Oncol. Hematol. 83, 407–421. doi:10.1016/j.critrevonc.2011.11.010
Qi, Z. Y., Hao, S. Y., Tian, H. Z., Bian, H. L., Hui, L., and Chen, S. W. (2020). Synthesis and Biological Evaluation of 1-(benzofuran-3-Yl)-4-(3,4,5-Trimethoxyphenyl)-1h-1,2,3-Triazole Derivatives as Tubulin Polymerization Inhibitors. Bioorg. Chem. 94, 103392. doi:10.1016/j.bioorg.2019.103392
Rayego-Mateos, S., Rodrigues-Diez, R., Morgado-Pascual, J. L., Valentijn, F., Valdivielso, J. M., Goldschmeding, R., et al. (2018). Role of Epidermal Growth Factor Receptor (EGFR) and its Ligands in Kidney Inflammation and Damage. Mediators Inflamm. 2018, 8739473. doi:10.1155/2018/8739473
Rebuzzi, S. E., Alfieri, R., La Monica, S., Minari, R., Petronini, P. G., and Tiseo, M. (2020). Combination of EGFR-TKIs and Chemotherapy in Advanced EGFR Mutated NSCLC: Review of the Literature and Future Perspectives. Crit. Rev. Oncol. Hematol. 146, 102820. doi:10.1016/j.critrevonc.2019.102820
Röhrig, U. F., Majjigapu, S. R., Grosdidier, A., Bron, S., Stroobant, V., Pilotte, L., et al. (2012). Rational Design of 4-Aryl-1,2,3-Triazoles for Indoleamine 2,3-Dioxygenase 1 Inhibition. J. Med. Chem. 55, 5270–5290. doi:10.1021/jm300260v
Roskoski, R. (2014). The ErbB/HER Family of Protein-Tyrosine Kinases and Cancer. Pharmacol. Res. 79, 34–74. doi:10.1016/j.phrs.2013.11.002
Schettino, C., Bareschino, M. A., Ricci, V., and Ciardiello, F. (2008). Erlotinib: an EGF Receptor Tyrosine Kinase Inhibitor in Non-small-cell Lung Cancer Treatment. Expert Rev. Respir. Med. 2, 167–178. doi:10.1586/17476348.2.2.167
Spaans, J. N., and Goss, G. D. (2014). Drug Resistance to Molecular Targeted Therapy and its Consequences for Treatment Decisions in Non-small-cell Lung Cancer. Front. Oncol. 4, 190. doi:10.3389/fonc.2014.00190
Sutter, A., Höpfner, M., Hüther, A., Maaser, K., and Scherübl, H. (2005). Targeting the Epidermal Growth Factor Receptor by Erlotinib (Tarceva) for the Treatment of Esophageal Cancer. Z. Gastroenterol. 43, P371. doi:10.1055/s-2005-920154
Sutter, A. P., Höpfner, M., Huether, A., Maaser, K., and Scherübl, H. (2010). Targeting the Epidermal Growth Factor Receptor by Erlotinib (Tarceva) for the Treatment of Esophageal Cancer. Int. J. Cancer 118, 1814–1822. doi:10.1002/ijc.21512
Thomas, M. (2003). Epidermal Growth Factor Receptor Tyrosine Kinase Inhibitors: Application in Non-small Cell Lung Cancer. Cancer Nurs. 26, 21S–25S. doi:10.1097/00002820-200312001-00006
Thomopoulou, P., Sachs, J., Teusch, N., Mariappan, A., Gopalakrishnan, J., and Schmalz, H. G. (2015). New Colchicine-Derived Triazoles and Their Influence on Cytotoxicity and Microtubule Morphology. ACS Med. Chem. Lett. 7, 188–191. doi:10.1021/acsmedchemlett.5b00418
Tsubata, Y., Tanino, R., and Isobe, T. (2021). Current Therapeutic Strategies and Prospects for EGFR Mutation-Positive Lung Cancer Based on the Mechanisms Underlying Drug Resistance. Cells 10, 3192. doi:10.3390/cells10113192
Von Hoff, D. D., Casper, J., Bradley, E., Trent, J. M., Hodach, A., Reichert, C., et al. (1980). Direct Cloning of Human Neuroblastoma Cells in Soft agar Culture. Cancer Res. 40, 3591–3597.
Wada, H., Doki, Y., Nishioka, K., Ishikawa, O., Kabuto, T., Yano, M., et al. (2005). Clinical Outcome of Esophageal Cancer Patients with History of Gastrectomy. J. Surg. Oncol. 89, 67–74. doi:10.1002/jso.20194
Wang, W. J., Mao, L. F., Lai, H. L., Wang, Y. W., Jiang, Z. B., Li, W., et al. (2020). Dolutegravir Derivative Inhibits Proliferation and Induces Apoptosis of Non-small Cell Lung Cancer Cells via Calcium Signaling Pathway. Pharmacol. Res. 161, 105129. doi:10.1016/j.phrs.2020.105129
Wei, W. T., Wang, L., Liang, J. X., Wang, J. F., Li, Q., and Zeng, J. (2020). LncRNA EIF3J-AS1 Enhanced Esophageal Cancer Invasion via Regulating AKT1 Expression through Sponging miR-373-3p. Sci. Rep. 10, 13969. doi:10.1038/s41598-020-70886-2
Wells, A. (1989). The Epidermal Growth Factor Receptor and its Ligands. Cancer Treat. Res. 47, 143–168. doi:10.1007/978-1-4613-1599-5_6
Whitley, A. C., Jackson, J., Trummell, H., Bonner, J., and Yang, E. S. (2012). Erlotinib Induces Synthetic Lethality with the Poly(ADP-Ribose) Polymerase (PARP) Inhibitor ABT-888 in Esophageal Cancer. Int. J. Radiat. Oncology*Biology*Physics 84, S165–S166. doi:10.1016/j.ijrobp.2012.07.428
Xu, G., Zhao, J., Jiang, Y., Zhang, P., and Li, W. (2016). Design, Synthesis and Antifungal Activity of Novel Indole Derivatives Linked with the 1,2,3-Triazole Moiety via the CuAAC Click Reaction. J. Chem. Res. 40, 269–272. doi:10.3184/174751916x14597828245275
Zhang, H. Q., Gong, F. H., Ye, J. Q., Zhang, C., Yue, X. H., Li, C. G., et al. (2017). Design and Discovery of 4-Anilinoquinazoline-Urea Derivatives as Dual TK Inhibitors of EGFR and VEGFR-2. Eur. J. Med. Chem. 125, 245–254. doi:10.1016/j.ejmech.2016.09.039
Zhang, W., Lei, P., Dong, X., and Xu, C. (2014). The New Concepts on Overcoming Drug Resistance in Lung Cancer. Drug Des. Devel Ther. 8, 735–744. doi:10.2147/dddt.s60672
Zhao, Y., Wang, H., and He, C. (2021). Drug Resistance of Targeted Therapy for Advanced Non-small Cell Lung Cancer Harbored EGFR Mutation: from Mechanism Analysis to Clinical Strategy. J. Cancer Res. Clin. Oncol. 147, 3653–3664. doi:10.1007/s00432-021-03828-8
Keywords: erlotinib, EGFR, anticancer, drug-resistant cancer cell lines, 1,2,3-triazole
Citation: Mao L-f, Wang Z-Z, Wu Q, Chen X, Yang J-X, Wang X and Li Y-M (2022) Design, Synthesis, and Antitumor Activity of Erlotinib Derivatives. Front. Pharmacol. 13:849364. doi: 10.3389/fphar.2022.849364
Received: 06 January 2022; Accepted: 28 February 2022;
Published: 20 April 2022.
Edited by:
Ruiwen Zhang, University of Houston, United StatesReviewed by:
Somaia Abd El-Karim, National Research Centre, EgyptCopyright © 2022 Mao, Wang, Wu, Chen, Yang, Wang and Li. This is an open-access article distributed under the terms of the Creative Commons Attribution License (CC BY). The use, distribution or reproduction in other forums is permitted, provided the original author(s) and the copyright owner(s) are credited and that the original publication in this journal is cited, in accordance with accepted academic practice. No use, distribution or reproduction is permitted which does not comply with these terms.
*Correspondence: Jian-Xue Yang, RG9jeWp4MTk2OUAxMjYuY29t; Xin Wang, d2FuZ3hpbm5rQG5hbmthaS5lZHUuY24=; Yue-Ming Li, eW1saUBuYW5rYWkuZWR1LmNu
†These authors have contributed equally to this work
Disclaimer: All claims expressed in this article are solely those of the authors and do not necessarily represent those of their affiliated organizations, or those of the publisher, the editors and the reviewers. Any product that may be evaluated in this article or claim that may be made by its manufacturer is not guaranteed or endorsed by the publisher.
Research integrity at Frontiers
Learn more about the work of our research integrity team to safeguard the quality of each article we publish.