- 1Xiangya School of Pharmaceutical Sciences, Central South University, Changsha, China
- 2Hunan Provincial Key Laboratory of Cardiovascular Research, Xiangya School of Pharmaceutical Sciences, Central South University, Changsha, China
- 3Department of Pharmacy, Xiangya Hospital, Central South University, Changsha, China
- 4National Clinical Research Center for Geriatric Disorders, Xiangya Hospital, Central South University, Changsha, China
Mitochondria, as one of the most critical subcellular organelles of cancer cells, are very vulnerable and often on the verge of oxidative stress. The classic chemodynamic therapy (CDT) directly employs endogenous chemical energy to trigger reactive oxygen species (ROS) burst and destroy tumor cells. However, the effectiveness of CDT is restricted by the limited diffusion distance and short half-life of ROS. From this perspective, the treatment method (mitochondria-targeting chemodynamic therapy nanodrugs, M-CDT nanodrugs) that can generate high levels of ROS at the mitochondrial site is extremely efficient and promising for cancer treatment. Currently, many emerging M-CDT nanodrugs have been demonstrated excellent spatial specificity and anti-cancer efficacy. In this minireview, we review various proof-of-concept researches based on different M-CDT nanodrugs designs to overcome the limits of the efficacy of CDT, mainly divided into four strategies: supplying H2O2, non-H2O2 dependent CDT, eliminating GSH and enhancing by hyperthermia therapy (HT). These well-designed M-CDT nanodrugs greatly increase the efficacy of CDT. Finally, the progress and potential of M-CDT nanodrugs are discussed, as well as their limitations and opportunities.
Introduction
Many new therapeutic targets and emerging therapies have been developed to find the Achilles heel of cancer cells (de Lázaro and Mooney, 2021). However, most therapy methods cannot completely kill malignant tumors or prevent the formation and recurrence of metastatic tumors (Chen et al., 2020). Mitochondria, as one of the most critical organelles in cancer cells, play a vital role in the energy supply, calcium homeostasis regulation, and signal transduction of cancer cells (Jiang et al., 2021). Mitochondria are also the key regulators of the apoptosis pathway. Damaged mitochondria release pro-apoptotic proteins (like cytochrome c) from the mitochondrial membrane space to the cytoplasm to trigger apoptosis in a typical apoptotic pathway (Bock and Tait, 2020). Moreover, the mitochondria of cancer cells usually undergo metabolic reprogramming for rapid proliferation and invasion, that is, they are more inclined to glycolysis than oxidative phosphorylation to produce ATP (Martínez-Reyes and Chandel, 2021). This process also produces a higher level of reactive oxygen species (ROS), which makes the mitochondria themselves on the verge of oxidative stress (Hayes et al., 2020). ROS has strong oxidative activity and can cause severe oxidative damage (Qin et al., 2021). Therefore, it is an extremely efficient strategy to promote mitochondrial damage to kill cancer cells by increasing the level of ROS in the mitochondrial region (Guo et al., 2021).
ROS-based cancer therapy such as chemodynamic therapies (CDT), photodynamic therapy (PDT), and sonodynamic therapy (SDT) have attracted substantial attention in recent years with the advantages of noninvasiveness, high tumor specificity, minimal toxicity, and low drug resistance (Wang et al., 2021a; Gao et al., 2020a). Among them, CDT has more unique advantages than other methods because it directly employs endogenous H2O2 in tumor site to generate toxic ROS through Fenton/Fenton-like reactions (Eq. 1) or other ways to kill cancer cells with no need for external energy input or O2 (Tian et al., 2021). However, ROS is a short-lived and efficient “killer” with a lifetime of less than 0.1 ms and a diffusion range of only 10–20 nm (Zhang et al., 2019). The initial location of ROS production has a significant impact on the therapeutic effect of CDT. CDT agents with targeting mitochondria can produce a high level of ROS in situ on mitochondria to cause severely mitochondrial damage and further trigger cell death (Zeng et al., 2021). However, the transport of CDT agents to the mitochondria of cancer cells is a huge bottleneck. In addition, the effect of CDT is greatly limited by the high concentration of glutathione (GSH), the unsustainable supply of H2O2, and the low Fenton reaction rate at the tumor site (Wang et al., 2020). Fortunately, the mitochondrial membrane potential of cancer cells is much higher than that in normal cells (∼−220 mV vs. ∼ −140 mV) (Fantin et al., 2006; Bagkos et al., 2014), which means mitochondria targeting groups with positive electricity and lipophilicity can be preferentially enriched into mitochondria of cancer cells (Shen et al., 2020; Xie et al., 2021). Moreover, the rapid development of nanodrugs has brought new hope for an ideal CDT agent delivery system. Well-designed nanodrugs can greatly increase the penetration of CDT agents at tumor sites by the enhanced permeation and retention (EPR) effect or active targeting (Li et al., 2021a; Nadar et al., 2021). Currently, many emerging CDT nanodrugs with mitochondria-targeting (M-CDT nanodrugs) have recently been demonstrated excellent spatial specificity and anti-cancer efficacy. More importantly, the therapeutic efficacy of M-CDT nanodrugs is further increased by GSH depletion (Wang et al., 2021b), H2O2 supplementation (Sun et al., 2021), non-H2O2 dependent CDT (Zhang et al., 2019), and combination hyperthermia therapy. This minireview focuses on the latest progress of M-CDT nanodrugs because of the significance of M-CDT nanodrugs and many advances in this research field. We divide these M-CDT nanodrugs into two parts to comprehensively summarize: Firstly, self-enhanced M-CDT nanodrugs with H2O2 supplementation, non-H2O2 dependent CDT, and GSH depletion; Secondly, HT enhanced M-CDT nanodrugs (Figure 1; Table 1). Finally, we propose the future development direction and challenges of M-CDT nanodrugs. This review provides the most advanced treatment methods and valuable information for cancer treatment.
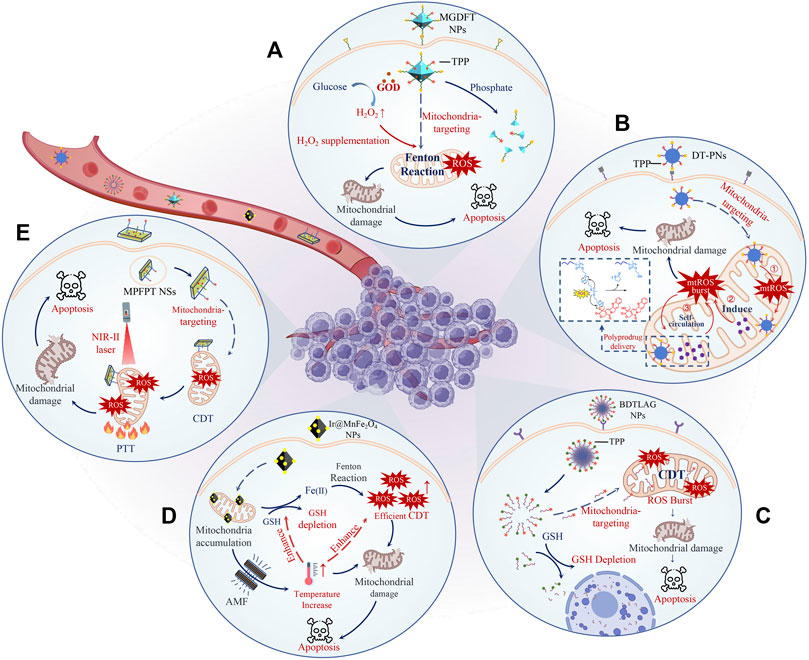
FIGURE 1. Schematic illustration of representative M-CDT nanodrugs. The therapeutic efficacy of M-CDT nanodrugs is further increased by H2O2 supplementation (A), non-H2O2 dependent CDT (B), GSH depletion (C), and combination with MHT (D) or PTT (E). To be specific, MGDFT NPs catalyzed the decomposition of glucose into H2O2 and gluconic acid through loaded GOD, significantly increasing the level of H2O2 (A). DT-PNs delivered non-H2O2 CDT agents to mitochondria through a poly-prodrug delivery strategy, triggering a ROS burst in situ and efficiently killing tumor cells (B). BDTLAG NPs rapidly consumed GSH and released CDT agents through the reaction of disulfide bonds with GSH, which significantly improved the tumor killing effect of ROS (C). Ir@MnFe2O4 NPs generated high temperatures locally in the mitochondria under the AMF, greatly promoting the rate of Fenton reaction and thus producing a high level of ROS to induce mitochondria damage (D). MPFPT NSs with high photothermal conversion efficiency efficiently accelerated the rate of the Fenton reaction by heating mitochondria, indicating the superiority of PTT-CDT with mitochondria targeting (E).
Self-Enhanced M-CDT Nanodrugs
Cancer cells have a much higher level of H2O2 (up to 100 mM) than normal cells (20 nM), which is also the first prerequisite for CDT treatment (Tang et al., 2020). However, H2O2 cannot be continuously supplied, which greatly limits the effect of CDT (Xuan et al., 2020). In addition, cancer cells have high concentrations of GSH to balance the oxidative stress caused by ROS (Langford et al., 2018). The ROS can easily be eliminated by the GSH-Glutathione peroxidase system during CDT treatment. Currently, three strategies are developed to greatly improve the efficacy of M-CDT nanodrugs: supplying H2O2, non-H2O2 dependent CDT, and eliminating GSH in cancer cells.
H2O2 is still insufficient for continuous ·OH generation during Fenton reaction-based CDT. Therefore, M-CDT nanodrugs with H2O2-supplement are advantageous for maintaining continuous oxidative damage (Cheng et al., 2022). For example, Peng et al. (2021) designed phosphate-degradable nanoparticles based on metal-organic frameworks nanocarriers (MGDFT NPs) with glucose oxidase (GOD) and doxorubicin (DOX) for CDT of cancer. Mil-101 (Fe) was selected as the nanocarrier for DOX and GOD, as well as the iron source for the Fenton reaction. Then Mil-101 (Fe) was modified by folic acid (FA) and triphenylphosphonium (TPP) to get a sequential targeting efficiency to cancer cells and mitochondria in cancer cells, respectively. Furthermore, MIL-101(Fe) was phosphate-sensitive and easy to degrade in cancer cells with the high concentration of phosphate, and MGDFT NPs disintegrated quickly to release iron ions and GOD. GOD catalyzed efficiently the reaction of glucose and O2 to produce gluconic acid and H2O2, which supplied the Fenton reaction substrate and prolonged CDT treatment duration. The level of ROS produced by MGDFT NPs was 4.4 times that of the NPs without GOD in 4T1 cells. The growth of cancer cells was successfully inhibited by MGDFT NPs without obvious side effects. Similarly, Sun et al. (2021) developed M-CDT nanodrugs loaded with GOD and lonidamine (FG/T-Nanoprodrug) for the chemotherapy and CDT combination therapy. The FG/T-Nanoprodrug was prepared in two steps: First, a T-Prodrug containing polylysine, TPP, and lonidamine self-assembled to form nanoparticles; Secondly, GOD and ferrocene (a Fenton reaction catalyst) were linked to the surface of nanoparticles to prepare the FG/T-Nanoprodrug. The FG/T-Nanoprodrug entered the mitochondria of cancer cells mediated by TPP and then released lonidamine and GOD. Lonidamine, as a hexokinase inhibitor (the rate-limiting enzyme of glycolysis), reduced glucose utilization by inhibiting mitochondrial energy metabolism, which allowed more glucose to be converted by GOD into H2O2 in cancer cells. The FG/T-Nanoprodrug continuously produced abundant ROS to destroy mitochondria and release cytochrome c. Tumor tissues treated with the FG/T-Nanoprodrug had a 7-fold increase of ROS levels compared to the saline group after 7 days. The FG/T-Nanoprodrug demonstrated extremely strong tumor suppression and ablation in a tumor xenograft model.
Non-H2O2 dependent CDT completely avoids the bottleneck problem that H2O2 cannot be continuously supplied in cancer cells (Gao et al., 2020b). Some chemotherapy drugs can directly stimulate the generation of endogenous ROS by interacting with the mitochondrial respiratory chain complex, which is considered to have CDT effect as well (Wang et al., 2021c; Wang et al., 2021d). For example, camptothecin (CPT), as an atypical CDT agent, can not only inhibit DNA topoisomerase I but stimulate the production of endogenous mitochondrial ROS to hyperpolarize mitochondria (Sánchez-Alcázar et al., 2000; Sen et al., 2004). Recently, Zhang et al. (2019) developed ROS-responsive polyprodrug nanoreactors with dual-targeting properties (DT-PNs) for cancer therapy. DT-PNS was self-assembled with two polyprodrug amphiphiles connected with multi-CPTs through the ROS-responsive thioketal linkage: cRGD-PDMA-bPCPTSM and TPP-PDMA-bPCPTSM. The decorated cyclic Arg-Gly-Asp (cRGD) peptide and TPP targeted the overexpressed integrins on the surface of cancer cells and mitochondria, respectively. As a result, DT-PNs internalized by cancer cells precisely anchored the mitochondria and released the initial CPT in response to endogenous upregulated mitochondrial ROS, while the CPT released in situ further triggered mitochondrial ROS, and finally formed a CPT-ROS circulation to generate a ROS burst in mitochondria. The self-circulation of CPT release and ROS burst led to mitochondrial-dependent apoptosis and efficiently killed cancer cells.
Very recently, Wang et al. (2021b) constructed a sequential multi-stage targeting nanoparticles (BDTLAG NPs) to increase the effect of non-H2O2 dependent CDT by GSH depletion. The BDTLAG NPs consisted of three parts: two mitochondria targeting CDT agents (TPP-PEG2K-LND and TPP-PEG2K-TOS) and a GSH scavenging group (Bio-PEG2K-S-S-CPT). The TPP group mediated the mitochondria targeting of lonidamine (LND) and α-tocopheryl succinate (α-TOS), both of which interfered with the mitochondrial energy metabolism to stimulate endogenous ROS production. Importantly, the Bio-PEG2K-S-S-CPT part of BDTLAG NPs actively targeted cancer cells because the surface of the cancer cells expresses biotin (Bio) receptors. The disulfide bond in the BDTLAG NPs broke to release CPT, LND, and TOS by reacting with GSH in cancer cells. The effect of depletion GSH was measured by the released CPT. Bio-PEG2K-S-S-CPT effectively removed GSH in cancer cells, and the CPT released by Bio-PEG2K-S-S-CPT was 6.8 times higher than that by the group without GSH response in cancer cells. At the same time, LND and TOS entered the mitochondria and caused high concentrations of ROS in situ on mitochondria, which led to mitochondria damage to induce the death of cancer cells. Compared with the CPT group and the BDG NPs group (without mitochondria targeting), BDTLAG NPs had the best tumor suppression effect because of their mitochondria targeting and efficient GSH depletion.
HT Enhanced M-CDT Nanodrugs
The slower reaction rate of CDT based on Fenton reaction is also a major factor limitation of the effectiveness of CDT (Cao et al., 2021). The high temperature from hyperthermia therapy (HT) can improve the rate of the Fenton/Fenton-like reaction to greatly increase the efficacy of CDT (Liu et al., 2019; Danewalia and Singh, 2021). Recently, Shen et al. (2020) reported MnFe2O4 NPs functionalized with Ir (III) complexes (Ir@MnFe2O4 NPs) for magnetic hyperthermia therapy (MHT) enhanced CDT. Cyclometalated iridium (III) complexes effectively targeted tumor cells inner mitochondrial membrane with high membrane potential. MnFe2O4 with superparamagnetism responded quickly to external magnetic fields and had excellent magnetothermal-properties including high magnetothermal conversion efficiency and magnetothermal stability. Therefore, Ir@MnFe2O4 NPs were internalized into cancer cells via the EPR effect and localized in mitochondria with help of cyclometalated iridium (III) complexes. GSH in cancer cells reduced the Fe (III) to Fe (II) on Ir@MnFe2O4 NPs surfaces to catalyze the Fenton reaction. More importantly, Ir@MnFe2O4 NPs generated high temperatures locally in the mitochondria under the alternating magnetic field (AMF), which produced a high level of ROS to induce mitochondria damage by greatly promoting the rate of the Fenton reaction. Ir@MnFe2O4NPs + AMF demonstrated a much stronger effect of inhibiting tumor growth than Ir@MnFe2O4NPs and AMF due to the synergistic effect of MHT and CDT.
Local high temperature through near-infrared light is also an effective strategy to enhance the efficacy of CDT (Zhou et al., 2019; Li et al., 2021b). For example, Jin et al. (2020) recently reported dual-targeted core-shell nanoplatforms (PTFHD nanoplatforms) for photothermal therapy (PTT) enhanced CDT. The PTFHD nanoplatforms were composed of hyaluronic acid (HA) and TPP co-modified polydopamine (PDA) nanoparticles loaded with DOX and Fe ions. HA and TPP mediated the sequential multistage-targeting of PTFHD nanoplatforms from cancer cells to their mitochondria. The PTFHD nanoplatforms catalyzed Fenton reaction by Fe ions in mitochondria after enzymolysis of HA shell and exposure of PDA-TPP-Fe core. PDA was a high-efficiency photothermal agent with high photothermal conversion efficiency. The PTFHD nanoplatforms lead to the local high temperature of mitochondria site under the irradiation of near-infrared laser (808 nm), which greatly promoted the rate of the Fenton reaction and damages the mitochondria. The second near-infrared (1,000–1,350 nm, NIR-II) has deeper tissue penetration and higher maximum allowable exposure than the first near-infrared (750–1,000 nm, NIR-I) light (Wang et al., 2021e; Liu et al., 2021; Zhang et al., 2021). Very recently, Li et al. (2021c) developed mitochondria-targeted MoS2@PDA-Fe@PEG/TPP nanosheets (MPFPT NSs) for the combination therapy of NIR-II-activated PTT and CDT. MPFPT NSs had a high photothermal conversion efficiency at NIR-II (34.9%). In addition, the Fe doped in MPFPT NSs was adopted as a Fenton reaction catalyst. MPFPT NSs significantly led to mitochondrial dysfunction and cancer cell apoptosis through TPP-mediated mitochondria targeting under NIR-II light (1,064 nm). The MPFPTNSs had a better tumor suppressive effect than similar nanoagents without mitochondria targeting ability or relying on single-mode therapy thanks to the superiority of PTT-CDT with mitochondria targeting.
Iron-based Fenton reaction has a low reaction rate. In addition, the optimal pH value for iron-based Fenton reaction is usually pH 2-5, and the reaction rate is low in the neutral intracellular environment of tumor cells (pH7.4) (Bao et al., 2021). The Fenton reaction rate of Cu(I) (1 × 104 M−1s−1) is much higher than that of Fe (II) (76 M−1s−1) (Hu et al., 2019; Li et al., 2019; Lin et al., 2019). Meanwhile, the pH range required for the reaction of Cu(I) is wider than that of Fe(II), which can be carried out in weakly acidic and neutral media (Ma et al., 2019). Recently, Li et al. (2020) proposed an exo/endogenous dual-augmented mitochondria targeting nanoplatform (CuO@AuCu-TPP) for PTT-enhanced CDT by Cu(I) mediated Fenton reaction. The CuO@AuCu was prepared by in-situ reduction of chloroauric acid on Cu2O truncated octahedron. In this process, the Cu(I) in truncated octahedron was converted to Cu (II), and chloroauric acid was reduced to small gold nanoparticles on the surface of Cu2O truncated octahedrons. The CuO@AuCu were further modified with TPP (CuO@AuCu-TPP) to target the mitochondria of cancer cells. The aggregates of small gold nanoparticles on the surface of CuO@AuCu-TPP were adopted as a powerful photothermal agent because of their strong near-infrared absorption. Cu (II) was reduced into Cu (I) by GSH after CuO@AuCu-TPP were enriched in mitochondria, which deleted GSH and initiated a rapid Fenton reaction. Moreover, ROS generation efficiency of the CuO@AuCu-TPP further increased by 4.6 times under the irradiation of near-infrared light (808 nm). The CuO@AuCu-TPP possessed an excellent antitumor effect due to the PTT enhanced CDT and GSH depletion in Balb/c mice tumor models.
Conclusion and Prospect
Traditional anticancer drugs including CDT agents are limited by their poor pharmacokinetic properties, such as low bioavailability, short half-life and low stability. Fortunately, nanotechnology can effectively improve the pharmacokinetic characteristics of traditional drugs by changing the size, shape and material characteristics of nanomaterials (Liu et al., 2012; Chen et al., 2021; Zhao et al., 2022; Zhu et al., 2022). Especially, well-designed nanodrugs can greatly increase the penetration of drugs at tumor sites and improve their pharmacokinetic and pharmacodynamic properties by the EPR effect. After decades of development, some nanodrugs have been approved for clinical cancer treatment, such as liposomes, polymer micelles, and inorganic nanomaterials (Cheng et al., 2021). However, most of these nanodrugs are carried with traditional chemotherapeutic drugs. Fundamentally, these nanodrugs are difficult to solve the resistance of cancer cells to traditional chemotherapeutic drugs, and have limited efficacy for cancer. Mitochondria are probably the most vulnerable part of cancer cells, because mitochondria not only play an irreplaceable core role in cancer cells but are also a key link in the apoptosis pathway of cancer cells. Therefore, mitochondria are likely to be the Achilles heel of cancer cells. Compared with non-mitochondria-targeting treatments, M-CDT nanodrugs exhibit higher therapeutic effect with lower dosage of drugs via precise and severe damage to mitochondria. More importantly, M-CDT nanodrugs are expected to solve the resistance of traditional drugs, because the resistance of cancer cells is generally ATP-dependent. For example, P-glycoproteins use ATP produced by mitochondria to pump a variety of anti-cancer drugs with different structures and different molecular weights out of the cancer cell, which is why cancer cells acquire multiple drug resistance. These emerging M-CDT nanodrugs destroy mitochondria very efficiently and fundamentally eliminate the multi-drug resistance of cancer cells. In short, M-CDT nanodrugs have great advantages over traditional drugs and conventional CDT nanodrugs, and have demonstrated amazing effects of cancer treatment. However, M-CDT nanodrugs still have some challenges to overcome.
First, M-CDT nanodrugs with the classic iron-based Fenton reaction face the bottleneck of insufficient reaction rate. Iron is a ubiquitous element in the human body and has excellent biocompatibility. However, the biggest problem lies in its low efficiency under the conditions of the cancer microenvironment. Hyperthermia can increase the reaction rate, but it brings complexity to the treatment and does not fundamentally solve the problem. In addition, other transition metals such as Cu, Pt, etc. are adopted to increase the reaction rate. However, the biocompatibility of transition metals is far less than that of iron (Ai et al., 2021). Reducing the pH of cancer cells is a very promising solution. The deduction of pH in tumor cells reinforces the response rate of the classic Fenton reaction-based CDT and simultaneously induces mitochondrial calcium overload, and these two resulting effects synergistically cause mitochondrial dysfunction and therefore tumor cell death (Bao et al., 2021). Secondly, for non-H2O2-dependent M-CDT nanodrugs, precise control of CDT drugs release on the mitochondria is a great challenge. Most of these CDT drugs can produce ROS only when they interfere with the mitochondrial respiratory chain in the inner mitochondrial membrane. In addition, these CDT drugs generally belong to traditional high toxic chemotherapy drugs, and their uncontrollable release can cause great side effects. Third, cancer cells themselves have sophisticated antioxidant defense systems, such as abundant GSH and antioxidant enzyme systems, etc (Su et al., 2021). Therefore, M-CDT nanodrugs often need to intervene in the various factor of antioxidant defense systems. Integrating multiple functions on M-CDT nanodrugs is still a great challenge.
Nevertheless, M-CDT nanodrugs are very promising in cancer treatment. M-CDT nanodrugs will be undergoing rapid development with a deeper understanding of tumor pathology and treatment methods. We hope that a new way for the clinical transformation of the M-CDT nanodrugs will be opened through the optimized design of M-CDT nanodrugs.
Author Contributions
All authors listed have made a substantial, direct, and intellectual contribution to the work and approved it for publication.
Funding
This work was supported by the National Natural Science Foundation of China (No. 81974508, 21974134), the Hunan Science Fund for Distinguished Young Scholar of China (No. 2021JJ10067), Innovation-Driven Project of Central South University (No. 202045005), Hunan Provincial Natural Science Foundation of China (No. 2021JJ31066), Changsha Science and Technology Project (No. kq2001048), Key Research Project of Ningxia Hui Autonomous Region in 2021 of China (Major Project) (No. 2021BEG01001).
Conflict of Interest
The authors declare that the research was conducted in the absence of any commercial or financial relationships that could be construed as a potential conflict of interest.
Publisher’s Note
All claims expressed in this article are solely those of the authors and do not necessarily represent those of their affiliated organizations, or those of the publisher, the editors and the reviewers. Any product that may be evaluated in this article, or claim that may be made by its manufacturer, is not guaranteed or endorsed by the publisher.
References
Ai, K., Huang, J., Xiao, Z., Yang, Y., Bai, Y., and Peng, J. (2021). Localized Surface Plasmon Resonance Properties and Biomedical Applications of Copper Selenide Nanomaterials. Mater. Today Chem. 20, 100402. doi:10.1016/j.mtchem.2020.100402
Bagkos, G., Koufopoulos, K., and Piperi, C. (2014). A New Model for Mitochondrial Membrane Potential Production and Storage. Med. Hypotheses 83, 175–181. doi:10.1016/j.mehy.2014.05.001
Bao, W., Liu, M., Meng, J., Liu, S., Wang, S., Jia, R., et al. (2021). MOFs-based Nanoagent Enables Dual Mitochondrial Damage in Synergistic Antitumor Therapy via Oxidative Stress and Calcium Overload. Nat. Commun. 12, 6399. doi:10.1038/s41467-021-26655-4
Bock, F. J., and Tait, S. W. G. (2020). Mitochondria as Multifaceted Regulators of Cell Death. Nat. Rev. Mol. Cel Biol 21, 85–100. doi:10.1038/s41580-019-0173-8
Cao, W., Jin, M., Yang, K., Chen, B., Xiong, M., Li, X., et al. (2021). Fenton/Fenton-like Metal-Based Nanomaterials Combine with Oxidase for Synergistic Tumor Therapy. J. Nanobiotechnol 19, 325. doi:10.1186/s12951-021-01074-1
Chen, J., Zhu, Y., Wu, C., and Shi, J. (2020). Nanoplatform-based cascade Engineering for Cancer Therapy. Chem. Soc. Rev. 49, 9057–9094. doi:10.1039/d0cs00607f
Chen, L., Huang, Q., Zhao, T., Sui, L., Wang, S., Xiao, Z., et al. (2021). Nanotherapies for Sepsis by Regulating Inflammatory Signals and Reactive Oxygen and Nitrogen Species: New Insight for Treating COVID-19. Redox Biol. 45, 102046. doi:10.1016/j.redox.2021.102046
Cheng, Y., Ji, Y., and Ouyang, D. (2022). FC-BBR/IND-induced Glucose Oxidase Nanodrugs for Targeted Combination Therapy. Int. J. Pharm. 611, 121349. doi:10.1016/j.ijpharm.2021.121349
Cheng, Z., Li, M., Dey, R., and Chen, Y. (2021). Nanomaterials for Cancer Therapy: Current Progress and Perspectives. J. Hematol. Oncol. 14, 85. doi:10.1186/s13045-021-01096-0
Danewalia, S. S., and Singh, K. (2021). Bioactive Glasses and Glass-Ceramics for Hyperthermia Treatment of Cancer: State-Of-Art, Challenges, and Future Perspectives. Mater. Today Bio 10, 100100. doi:10.1016/j.mtbio.2021.100100
de Lázaro, I., and Mooney, D. J. (2021). Obstacles and Opportunities in a Forward Vision for Cancer Nanomedicine. Nat. Mater. 20, 1469–1479. doi:10.1038/s41563-021-01047-7
Fantin, V. R., St-Pierre, J., and Leder, P. (2006). Attenuation of LDH-A Expression Uncovers a Link between Glycolysis, Mitochondrial Physiology, and Tumor Maintenance. Cancer cell 9, 425–434. doi:10.1016/j.ccr.2006.04.023
Gao, D., Guo, X., Zhang, X., Chen, S., Wang, Y., Chen, T., et al. (2020). Multifunctional Phototheranostic Nanomedicine for Cancer Imaging and Treatment. Mater. Today Bio 5, 100035. doi:10.1016/j.mtbio.2019.100035
Gao, F., Wang, F., Nie, X., Zhang, Z., Chen, G., Xia, L., et al. (2020). Mitochondria-targeted Delivery and Light Controlled Release of Iron Prodrug and CO to Enhance Cancer Therapy by Ferroptosis. New J. Chem. 44, 3478–3486. doi:10.1039/c9nj05860e
Guo, X., Yang, N., Ji, W., Zhang, H., Dong, X., Zhou, Z., et al. (2021). Mito-Bomb: Targeting Mitochondria for Cancer Therapy. Adv. Mater. 33, 2007778. doi:10.1002/adma.202007778
Hayes, J. D., Dinkova-Kostova, A. T., and Tew, K. D. (2020). Oxidative Stress in Cancer. Cancer cell 38, 167–197. doi:10.1016/j.ccell.2020.06.001
Hou, H., Huang, X., Wei, G., Xu, F., Wang, Y., and Zhou, S. (2019). Fenton Reaction-Assisted Photodynamic Therapy for Cancer with Multifunctional Magnetic Nanoparticles. ACS Appl. Mater. Inter. 11, 29579–29592. doi:10.1021/acsami.9b09671
Hu, P., Wu, T., Fan, W., Chen, L., Liu, Y., Ni, D., et al. (2017). Near Infrared-Assisted Fenton Reaction for Tumor-specific and Mitochondrial DNA-Targeted Photochemotherapy. Biomaterials 141, 86–95. doi:10.1016/j.biomaterials.2017.06.035
Hu, R., Fang, Y., Huo, M., Yao, H., Wang, C., Chen, Y., et al. (2019). Ultrasmall Cu2-xS Nanodots as Photothermal-Enhanced Fenton Nanocatalysts for Synergistic Tumor Therapy at NIR-II Biowindow. Biomaterials 206, 101–114. doi:10.1016/j.biomaterials.2019.03.014
Jiang, L., Zhou, S., Zhang, X., Li, C., Ji, S., Mao, H., et al. (2021). Mitochondrion-specific Dendritic Lipopeptide Liposomes for Targeted Sub-cellular Delivery. Nat. Commun. 12, 2390. doi:10.1038/s41467-021-22594-2
Jin, R., Wang, Q., Dou, G., Bai, Y., Liu, S., Cai, B., et al. (2020). Stimuli Responsive Nanoplatform with Mitochondria-specific Multiple Model Therapeutics for Effective Tumor Treatment. Applied Materials Today 21, 100883. doi:10.1016/j.apmt.2020.100883
Langford, T. F., Huang, B. K., Lim, J. B., Moon, S. J., and Sikes, H. D. (2018). Monitoring the Action of Redox-Directed Cancer Therapeutics Using a Human Peroxiredoxin-2-Based Probe. Nat. Commun. 9, 3145. doi:10.1038/s41467-018-05557-y
Li, X., Hao, S., Han, A., Yang, Y., Fang, G., Liu, J., et al. (2019). Intracellular Fenton Reaction Based on Mitochondria-Targeted Copper(II)-peptide Complex for Induced Apoptosis. J. Mater. Chem. B 7, 4008–4016. doi:10.1039/c9tb00569b
Li, W., Wang, S., Ren, C., Liu, P., Lu, Q., Yang, L., et al. (2020). Exo/endogenous Dual-Augmented Chemodynamic Therapy Based on Bio-Reducible and Bio-Breakable Copper (Ⅱ)-based Truncated Octahedron. Chem. Eng. J. 396, 125280. doi:10.1016/j.cej.2020.125280
Li, S. L., Jiang, P., Jiang, F. L., and Liu, Y. (2021). Recent Advances in Nanomaterial-Based Nanoplatforms for Chemodynamic Cancer Therapy. Adv. Funct. Mater. 31, 2100243. doi:10.1002/adfm.202100243
Li, X., Yuan, H. J., Tian, X. M., Tang, J., Liu, L. F., and Liu, F. Y. (2021). Biocompatible Copper Sulfide-Based Nanocomposites for Artery Interventional Chemo-Photothermal Therapy of Orthotropic Hepatocellular Carcinoma. Mater. Today Bio 12, 100128. doi:10.1016/j.mtbio.2021.100128
Li, X., Xiao, H., Xiu, W., Yang, K., Zhang, Y., Yuwen, L., et al. (2021). Mitochondria-Targeting MoS2-Based Nanoagents for Enhanced NIR-II Photothermal-Chemodynamic Synergistic Oncotherapy. ACS Appl. Mater. Inter. 13, 55928–55938. doi:10.1021/acsami.1c18311
Lin, K., Lin, Z., Li, Y., Zheng, Y., and Zhang, D. (2019). Ultrasound-induced Reactive Oxygen Species Generation and Mitochondria-specific Damage by Sonodynamic Agent/metal Ion-Doped Mesoporous Silica. RSC Adv. 9, 39924–39931. doi:10.1039/c9ra08142a
Liu, Y., Ai, K., and Lu, L. (2012). Nanoparticulate X-ray Computed Tomography Contrast Agents: From Design Validation to In Vivo Applications. Acc. Chem. Res. 45, 1817–1827. doi:10.1021/ar300150c
Liu, X., Liu, Y., Wang, J., Wei, T., and Dai, Z. (2019). Mild Hyperthermia-Enhanced Enzyme-Mediated Tumor Cell Chemodynamic Therapy. ACS Appl. Mater. Inter. 11, 23065–23071. doi:10.1021/acsami.9b08257
Liu, S., Li, Y., Zhang, J., Zhang, H., Wang, Y., Chuah, C., et al. (2021). A Two-In-One Janus NIR-II AIEgen with Balanced Absorption and Emission for Image-Guided Precision Surgery. Mater. Today Bio 10, 100087. doi:10.1016/j.mtbio.2020.100087
Ma, B., Wang, S., Liu, F., Zhang, S., Duan, J., Li, Z., et al. (2019). Self-Assembled Copper-Amino Acid Nanoparticles for In Situ Glutathione "AND" H2O2 Sequentially Triggered Chemodynamic Therapy. J. Am. Chem. Soc. 141, 849–857. doi:10.1021/jacs.8b08714
Martínez-Reyes, I., and Chandel, N. S. (2021). Cancer Metabolism: Looking Forward. Nat. Rev. Cancer 21, 669–680. doi:10.1038/s41568-021-00378-6
Nadar, R. A., Franssen, G. M., Van Dijk, N. W. M., Codee-van der Schilden, K., de Weijert, M., Oosterwijk, E., et al. (2021). Bone Tumor-Targeted Delivery of Theranostic 195mPt-Bisphosphonate Complexes Promotes Killing of Metastatic Tumor Cells. Mater. Today Bio 9, 100088. doi:10.1016/j.mtbio.2020.100088
Peng, H., Qin, Y. T., Feng, Y. S., He, X. W., Li, W. Y., and Zhang, Y. K. (2021). Phosphate-Degradable Nanoparticles Based on Metal-Organic Frameworks for Chemo-Starvation-Chemodynamic Synergistic Antitumor Therapy. ACS Appl. Mater. Inter. 13, 37713–37723. doi:10.1021/acsami.1c10816
Qin, X., Wu, C., Niu, D., Qin, L., Wang, X., Wang, Q., et al. (2021). Peroxisome Inspired Hybrid Enzyme Nanogels for Chemodynamic and Photodynamic Therapy. Nat. Commun. 12, 5243. doi:10.1038/s41467-021-25561-z
Sánchez-Alcázar, J. A., Ault, J. G., Khodjakov, A., and Schneider, E. (2000). Increased Mitochondrial Cytochrome C Levels and Mitochondrial Hyperpolarization Precede Camptothecin-Induced Apoptosis in Jurkat Cells. Cell Death Differ 7, 1090–1100. doi:10.1038/sj.cdd.4400740
Sen, N., Das, B. B., Ganguly, A., Mukherjee, T., Tripathi, G., Bandyopadhyay, S., et al. (2004). Camptothecin Induced Mitochondrial Dysfunction Leading to Programmed Cell Death in Unicellular Hemoflagellate Leishmania Donovani. Cel Death Differ 11, 924–936. doi:10.1038/sj.cdd.4401435
Shen, J., Rees, T. W., Zhou, Z., Yang, S., Ji, L., and Chao, H. (2020). A Mitochondria-Targeting Magnetothermogenic Nanozyme for Magnet-Induced Synergistic Cancer Therapy. Biomaterials 251, 120079. doi:10.1016/j.biomaterials.2020.120079
Su, X., Cao, Y., Liu, Y., Ouyang, B., Ning, B., Wang, Y., et al. (2021). Localized Disruption of Redox Homeostasis Boosting Ferroptosis of Tumor by Hydrogel Delivery System. Mater. Today Bio 12, 100154. doi:10.1016/j.mtbio.2021.100154
Sun, M., Wang, C., Lv, M., Fan, Z., and Du, J. (2021). Mitochondrial-targeting Nanoprodrugs to Mutually Reinforce Metabolic Inhibition and Autophagy for Combating Resistant Cancer. Biomaterials 278, 121168. doi:10.1016/j.biomaterials.2021.121168
Tang, Y., Ji, Y., Yi, C., Cheng, D., Wang, B., Fu, Y., et al. (2020). Self-accelerating H2O2-Responsive Plasmonic Nanovesicles for Synergistic Chemo/starving Therapy of Tumors. Theranostics 10, 8691–8704. doi:10.7150/thno.45392
Tian, Q., Xue, F., Wang, Y., Cheng, Y., An, L., Yang, S., et al. (2021). Recent Advances in Enhanced Chemodynamic Therapy Strategies. Nano Today 39, 101162. doi:10.1016/j.nantod.2021.101162
Wang, X., Zhong, X., Liu, Z., and Cheng, L. (2020). Recent Progress of Chemodynamic Therapy-Induced Combination Cancer Therapy. Nano Today 35, 100946. doi:10.1016/j.nantod.2020.100946
Wang, X. Q., Wang, W., Peng, M., and Zhang, X. Z. (2021). Free Radicals for Cancer Theranostics. Biomaterials 266, 120474. doi:10.1016/j.biomaterials.2020.120474
Wang, N., Liu, C., Yao, W., Zhou, H., Yu, S., Chen, H., et al. (2021). A Traceable, Sequential Multistage-Targeting Nanoparticles Combining Chemo/Chemodynamic Therapy for Enhancing Antitumor Efficacy. Adv. Funct. Mater. 31, 2101432. doi:10.1002/adfm.202101432
Wang, N., Liu, C., Yao, W., Wang, X., Zhou, H., Chen, H., et al. (2021). A Sequential Multistage-Targeted Nanoparticles for MR Imaging and Efficient Chemo/chemodynamic Synergistic Therapy of Liver Cancer. Applied Materials Today 24, 101147. doi:10.1016/j.apmt.2021.101147
Wang, N., Liu, C., Yao, W., Zhou, H., Yu, S., Chen, H., et al. (2021). Endogenous Reactive Oxygen Species Burst Induced and Spatiotemporally Controlled Multiple Drug Release by Traceable Nanoparticles for Enhancing Antitumor Efficacy. Biomater. Sci. 9, 4968–4983. doi:10.1039/d1bm00668a
Wang, J., Sui, L., Huang, J., Miao, L., Nie, Y., Wang, K., et al. (2021). MoS2-based Nanocomposites for Cancer Diagnosis and Therapy. Bioact Mater. 6, 4209–4242. doi:10.1016/j.bioactmat.2021.04.021
Wen, Y. L., Chen, L., Leng, F., Yang, Z. Y., and Yu, C. (2021). Bimetallic Au-Ag Nanocages Extended TPP Conjugate Structure for Self-Enhancing Therapy of Tumors. J. Nanostructure Chem. doi:10.1007/s40097-021-00457-y
Xie, L., Wang, L., Guan, R., Ji, L., and Chao, H. (2021). Anti-metastasis and Anti-proliferation Effect of Mitochondria-Accumulating Ruthenium(II) Complexes via Redox Homeostasis Disturbance and Energy Depletion. J. Inorg. Biochem. 217, 111380. doi:10.1016/j.jinorgbio.2021.111380
Xuan, W., Xia, Y., Li, T., Wang, L., Liu, Y., and Tan, W. (2020). Molecular Self-Assembly of Bioorthogonal Aptamer-Prodrug Conjugate Micelles for Hydrogen Peroxide and pH-independent Cancer Chemodynamic Therapy. J. Am. Chem. Soc. 142, 937–944. doi:10.1021/jacs.9b10755
Zeng, Z., Fang, C., Zhang, Y., Chen, C.-X., Zhang, Y.-F., and Zhang, K. (2021). Mitochondria-Targeted Nanocarriers Promote Highly Efficient Cancer Therapy: A Review. Front. Bioeng. Biotechnol. 9, 1060. doi:10.3389/fbioe.2021.784602
Zhang, W., Hu, X., Shen, Q., and Xing, D. (2019). Mitochondria-specific Drug Release and Reactive Oxygen Species Burst Induced by Polyprodrug Nanoreactors Can Enhance Chemotherapy. Nat. Commun. 10, 1704. doi:10.1038/s41467-019-09566-3
Zhang, Y., Zhang, S., Zhang, Z., Ji, L., Zhang, J., Wang, Q., et al. (2021). Recent Progress on NIR-II Photothermal Therapy. Front. Chem. 9, 728066. doi:10.3389/fchem.2021.728066
Zhao, T., Wu, W., Sui, L., Huang, Q., Nan, Y., Liu, J., et al. (2022). Reactive Oxygen Species-Based Nanomaterials for the Treatment of Myocardial Ischemia Reperfusion Injuries. Bioactive Mater. 7, 47–72. doi:10.1016/j.bioactmat.2021.06.006
Zhou, W., Guan, H., Sun, K., Xing, Y., and Zhang, J. (2019). FeOOH/Polypyrrole Nanocomposites with an Islands-In-Sea Structure toward Combined Photothermal/Chemodynamic Therapy. ACS Appl. Bio Mater. 2, 2708–2714. doi:10.1021/acsabm.9b00435
Keywords: chemodynamic therapy, mitochondria-targeting, nanomaterials, cancer therapy, reactive oxygen species
Citation: Chen Q, Li N, Wang X, Yang Y, Xiang Y, Long X, Zhang J, Huang J, Chen L and Huang Q (2022) Mitochondria-Targeting Chemodynamic Therapy Nanodrugs for Cancer Treatment. Front. Pharmacol. 13:847048. doi: 10.3389/fphar.2022.847048
Received: 01 January 2022; Accepted: 17 January 2022;
Published: 10 January 2022.
Edited by:
Zeming Liu, Huazhong University of Science and Technology, ChinaReviewed by:
Wu-Yi Sun, Anhui Medical University, ChinaWensheng Chen, Texas A&M University, United States
Copyright © 2022 Chen, Li, Wang, Yang, Xiang, Long, Zhang, Huang, Chen and Huang. This is an open-access article distributed under the terms of the Creative Commons Attribution License (CC BY). The use, distribution or reproduction in other forums is permitted, provided the original author(s) and the copyright owner(s) are credited and that the original publication in this journal is cited, in accordance with accepted academic practice. No use, distribution or reproduction is permitted which does not comply with these terms.
*Correspondence: Qiong Huang, cWlvbmdodWFuZ0Bjc3UuZWR1LmNu
†These authors have contributed equally to this work