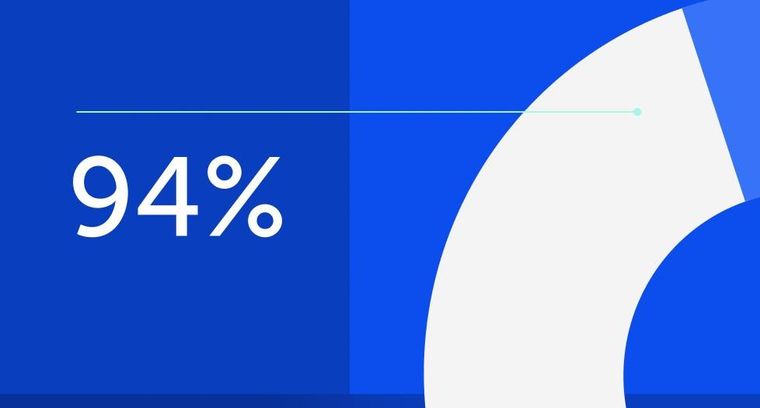
94% of researchers rate our articles as excellent or good
Learn more about the work of our research integrity team to safeguard the quality of each article we publish.
Find out more
ORIGINAL RESEARCH article
Front. Pharmacol., 16 March 2022
Sec. Translational Pharmacology
Volume 13 - 2022 | https://doi.org/10.3389/fphar.2022.842921
This article is part of the Research TopicPharmacokinetics, Pharmacodynamics (PK/PD) of Antibiotics: A Reality CheckView all 9 articles
The reduction in antimicrobial activity at high bacterial counts is a microbiological phenomenon known as the inoculum effect (IE). In a previous in vitro study, a significant IE was observed for polymyxin B (PMB) against a clinical isolate of Acinetobacter baumannii, and well described by a new pharmacokinetic-pharmacodynamic model. Few in vivo studies have investigated the impact of inoculum size on survival or antibiotic efficacy. Therefore, our objective was to confirm the influence of inoculum size of this A. baumannii clinical isolate on PMB in vivo effect over time. Pharmacokinetics and pharmacodynamics of PMB after a single subcutaneous administration (1, 15 and 40 mg/kg) were studied in a neutropenic murine thigh infection model. The impact of A. baumannii inoculum size (105, 106 and 107 CFU/thigh) on PMB efficacy was also evaluated. In vivo PMB PK was well described by a two-compartment model including saturable absorption from the subcutaneous injection site and linear elimination. The previous in vitro PD model was modified to adequately describe the decrease of PMB efficacy with increased inoculum size in infected mice. The IE was modeled as a decrease of 32% in the in vivo PMB bactericidal effect when the starting inoculum increases from 105 to 107 CFU/thigh. Although not as important as previously characterized in vitro an IE was confirmed in vivo.
Acinetobacter baumannii is an opportunistic Gram-negative pathogen responsible for severe clinical infections encountered in intensive care units (ICUs) worldwide, such as acquired pneumonia and bacteremia but also urinary tract infections, meningitis and infections of traumatic wounds (García-Garmendia et al., 2001; Garnacho et al., 2003; Gil-Perotin et al., 2012; El-Saed et al., 2013; Garnacho-Montero and Timsit, 2019). Carbapenems are used as the first-line treatments for A. baumannii infections (Wong et al., 2017). However, due to the increase of A. baumannii strains resistant to carbapenems, other antibiotics such as polymyxins [colistin (CST) and polymyxin B (PMB)] may be used as last-line treatments (Wong et al., 2017; Garnacho-Montero and Timsit, 2019).
High bacterial counts may alter antibiotherapy success due to an inoculum effect (IE) (Chastre and Fagon, 2002; Koenig and Truwit, 2006; Kalanuria et al., 2014), corresponding to a reduction of the antibiotic activity as bacterial count increases (Harada et al., 2014; Lenhard and Bulman, 2019). This phenomenon has been described in vitro for ß-lactam antibiotics used against ß-lactamases-producing bacteria such as Escherichia coli and Klebsiella pneumoniae (Bedenić et al., 2001; Harada et al., 2014; Smith and Kirby, 2018; Lenhard and Bulman, 2019). It has also been reported in vitro with other antibiotics and bacteria, including glycopeptides used against Staphylococcus aureus (Rio-Marques et al., 2014), fluoroquinolones against S. aureus and Pseudomonas aeruginosa (Mizunaga et al., 2005), aminoglycosides against S. aureus and E. coli (Li and Ma, 1998) or PMB against P. aeruginosa (Tam et al., 2005). We have recently documented for the first time, an inoculum effect of A. baumannii on polymyxin B in vitro using static time-kill experiments and PKPD modeling (Akrong et al., 2021). The IE required a 17-fold increase of the PMB concentration to reach 50% of maximal effect (EC50) as the initial inoculum increased from 105 to 108 CFU/ml.
Yet the IE of A. baumannii on polymyxins in vivo remains to be investigated. Indeed, Lin et al. observed a decrease in the efficacy of nebulized colistin when the initial inoculum of A. baumannii increased from 107 to 108 CFU/lung (Lin et al., 2018). But IE was only investigated on rare occasions in vivo, with antibiotics such as marbofloxacin (Ferran et al., 2009), piperacillin-tazobatam (Harada et al., 2014), ertapenem (Maglio et al., 2005), meropenem (Mizunaga et al., 2005) or colistin (Fantin et al., 2019) against various Gram-negative pathogens such as E. coli, K. pneumoniae or P. aeruginosa but never against A. baumannii. In these in vivo studies, the impact of inoculum size was evaluated based on bacterial counts at 24 or 48 h after the start of antibiotic therapy (Maglio et al., 2004; Maglio et al., 2005; Ferran et al., 2009; Lee et al., 2013; Harada et al., 2014), or on the survival of infected animals (Mizunaga et al., 2005; Fantin et al., 2019). However, to our knowledge, no PKPD modeling of the impact of inoculum size on antibiotic activity has ever been performed in vivo.
Therefore, our objectives were, first to evaluate whether the IE that was observed in vitro with A. baumannii and PMB could be detected in vivo. Second, if an in vivo IE was revealed, we aimed to assess the capability of the PKPD model developed with in vitro data (Akrong et al., 2021) to describe the newly produced in vivo data.
Polymyxin B sulfate (PMB) and cyclophosphamide monohydrate obtained from Sigma-Aldrich (Merck KGaA, Saint-Quentin Fallavier, France) were used to prepare solutions in sterile conditions. During this study, all chemicals and reagents used were analytical grade.
A clinical strain of A. baumannii (CS01), isolated from a patient with a meningitis (Seville, Spain) before treatment with CST, was used during this study (López-Rojas et al., 2013). Before each experiment, the strain was cultured in 5 ml of cation adjusted Muller-Hinton broth II (MHB) (Biomérieux, Marcy-l’Etoile, France) and incubated overnight at 37 ± 2°C with constant shaking (150–170 rpm). This overnight suspension was diluted 1:50 in MHB and was incubated with constant shaking at 35°C during 2 h until an OD600nm of 0.26 was achieved (Ultrospec10, Biochrom Ltd., Cambridge, United Kingdom), corresponding to a bacterial count of 108 CFU/ml in exponential growth phase. The bacterial suspension was centrifuged (3,000 rpm, 6 min), broth was removed and replaced by the same volume of sterile saline solution. This suspension was then diluted to obtain inocula of 107 and 106 CFU/ml. Samples of the inoculation solutions were serially diluted on saline, plated on Muller-Hinton agar plates (Biomérieux, Marcy-l’Etoile, France) and incubated overnight at 37°C.
Animal experiments were carried out according to the EC Directive 2010/63/EU. They were approved by the local ethics committee (COMETHEA) and registered by the French Ministry of Higher Education and Research (approval numbers: 2019022216097190 and 2017072415099072). Five-week-old male Swiss RjOrl mice (n = 296) weighing 34 ± 2 g (mean ± standard deviation [SD]) were obtained from Janvier Labs (Saint-Berthevin, France). All animals were acclimatized in ventilated racks in a temperature-regulated environment with a 12 h light-dark cycle, with free access to food and water for a minimum of 5 days before the beginning of the experiment. Neutropenia was induced by intraperitoneal administrations of cyclophosphamide at 150 and 100 mg/kg, 4 days and 1 day prior to experimental infection, respectively (Landersdorfer et al., 2018). Thigh infection was induced by intramuscular administration of 0.1 ml of a bacterial suspension of 106, 107 or 108 CFU/ml (corresponding to 105, 106 and 107 CFU/thigh, respectively), into one of the posterior thigh muscles. Thus, three groups of mice were distinguished according to the inoculum injected (n = 76, 146 and 74 for 105, 106 and 107 CFU/thigh, respectively) Each group was divided into two subgroups: treated (n = 48, 116 and 45 for 105, 106 and 107 CFU/thigh, respectively) and control mice (n = 28, 30 and 29 for 105, 106 and 107 CFU/thigh, respectively).
Two hours after bacterial inoculation, mice received either a single subcutaneous administration of PMB (1, 15 or 40 mg/kg for mice infected with 106 and 107 CFU/thigh, and 15 or 40 mg/kg for mice infected with 105 CFU/thigh), or a subcutaneous administration of saline solution (control group).
The PK of PMB was determined in neutropenic mice infected with the bacterial inoculum size of 106 CFU/thigh (n = 56). Mice were anesthetized by isoflurane (AbbVie, Rungis, France) inhalation (3%) for 5 min at each sampling time. Blood samples were collected by intracardiac puncture into heparinized tubes up to 24 h after PMB administration for a total of 7 time points per dose level (n = 3 animals per time point). Plasma was separated from the whole blood after centrifugation at 4,000 rpm for 10 min at 4°C and divided into two samples. The first sample was used to determine total PMB concentrations and the second one (0.15 ml) was ultrafiltered (4000 rpm for 30 min at room temperature) using Centrifree® ultrafiltration devices from Millipore (Merck KGaA) to determine unbound PMB concentrations and consequently protein binding. The non-specific binding of PMB to the membrane of the Centrifree® ultrafiltration devices (Sader et al., 2012) was determined by ultrafiltration of PMB solutions in phosphate buffer (pH7.2) at concentrations ranging from 0.2 to 7.5 mg/L and was used to correct ultrafiltrate concentrations. Plasma samples and ultrafiltrates were stored at −20°C until further analysis. Total and unbound PMB concentrations were determined by a liquid chromatography tandem mass spectrometry (LS-MS/MS) method (Supplemental Material).
Mice (n = 240) were sacrificed at 6 different time points: just prior to the start of the therapy (0 h) and at 2, 4, 6, 8 and 24 h after PMB administration. A total of 3-6 mice were sacrificed at each time point. Thigh muscles were collected and homogenized with 1 ml of sterile saline solution using potters Elvehjem-type tissue grinders (Thermo Fischer Scientific, Illkirch-Graffenstaden, France). Homogenates were serially diluted in saline, plated on Muller-Hinton agar plates and incubated overnight at 37°C. Bacterial colonies were counted and expressed as log10 numbers of CFU/thigh. The lower limit of quantification (LOQ) was set to 800 CFU/ml corresponding to 2.9 log10 CFU/thigh.
A PKPD model was developed in two steps to quantify the exposure-effect relationship of PMB in infected mice. First, time courses of total and unbound PMB concentrations were modeled and then, PK parameters were fixed during development of the PD part of the PKPD model.
Different structural models including one, two or three compartments, linear, nonlinear (Michaelis-Menten) or parallel linear/nonlinear elimination were evaluated to describe PK data. Models with linear and nonlinear absorption were also tested. Additive, proportional and exponential residual error models were explored.
The structural model for the bacterial population included one compartment representing drug-susceptible growing bacteria. A logistic function was used to model the self-limiting growth observed in vivo:
Where, B (CFU/thigh) is the drug-susceptible bacterial population, knet (h−1) is the apparent (net) growth rate constant and Bmax (CFU/thigh) the maximum bacterial count reached in the tissue. The residual error was additive on a log10 scale for bacterial counts (log10 CFU/thigh).
Predicted unbound plasma concentrations were linked to the bacterial sub-model using a mathematical function to characterize PMB antimicrobial effect (kPMB) such as:
Where multiple functions (linear, power, basic Emax or a sigmoidal Emax function) for kPMB were tested.
Empirical mathematical functions (i.e. linear, exponential and power) describing the relationship between kPMB and inoculum size were tested as a way to include the impact of inoculum size on PMB bactericidal activity.”
Model selection was based on objective function value (OFV) and goodness of fit (GOF) plots. When two models were nested, a decrease in OFV of at least 3.84 (chi square 1df p = 0.05) was needed to select the most complex model. Visual predictive checks (VPCs) based on 1,000 simulations were drawn after stratification on the PMB dose and the starting inoculum to evaluate the predictive performance of the model and were taken into account for model selection. Data below the LOQ were taken into account during parameter estimation by applying Beal’s M3 method (Beal, 2001). Parameter estimation was performed using NONMEM software (ICON, Dublin, Ireland) version 7.4.2 using the LAPLACIAN algorithm. Uncertainty around population parameters was estimated using the sampling importance resampling (SIR) technique (Dosne et al., 2016).
To evaluate the impact of outliers on parameter estimates and model-based inferences, a sensitivity analysis was performed. Briefly, model estimation was performed with the complete dataset and with a reduced dataset excluding outliers. Parameter estimates were compared and simulations of expected bacterial counts for all tested experimental conditions (i.e. all inocula and dosing regimens) were performed with the two parameter estimate sets.
A saturable non-specific binding (NSB) of PMB to the ultrafiltration membrane, translating to a non-linear decrease of PMB NSB when PMB concentration increased, was observed (Supplementary Figure S1) and could be described by the following equation:
Where NSB corresponds to the non-specific binding (%) and UF to PMB concentrations in ultrafiltrates (mg/L). Equation 3 was used to correct ultrafiltrates concentrations, as follows:
Total and unbound PMB plasma concentrations versus time profiles in thigh-infected mice are shown on Supplementary Figure S2. Unbound concentrations in mice receiving a subcutaneous dose of 1 mg/kg were all below LOQ (unbound LOQ = 0.62 mg/L after correction by the non-specific binding). The plasma peak was smoother and delayed as the dose increased, with a time to peak (Tmax) of 0.5 h for a dose of 1 mg/kg and 2 h for a dose of 40 mg/kg. Total PMB peak concentration (Cmax) did not change proportionally with dose, but increased only 15-fold (from 0.92 ± 0.14 (mean ± SD) to 13.75 ± 0.81 mg/L) when the dose increased from 1 to 40 mg/kg, attesting for some degree of PK non-linearity across this range of PMB subcutaneous doses.
Total and unbound plasma PMB concentrations versus time were best fitted by a two-compartment model with saturable absorption from the injection site, and linear elimination (Figure 1). Parameter estimates with their corresponding uncertainties are summarized in Table 1. PMB plasma protein binding was concentration independent within the observed range of total concentrations (0.20–14.56 mg/L) and the unbound fraction, estimated to be 17% (Table 1), was used for unbound concentrations fitting. GOF plots (Supplementary Figure S3) and VPCs (Figure 2) demonstrate that the selected model adequately predicted the mean tendency and dispersion of the total plasma data across the investigated dose range. For unbound concentrations, the model slightly overestimates and underestimates peak concentrations after the 15 and 40 mg/kg doses respectively (Supplementary Figure S3).
FIGURE 1. Schematic representation of the final pharmacokinetic-pharmacodynamic model characterizing the inoculum effect of A. baumannii on PMB bactericidal activity (kPMB). SC, subcutaneous; knet, apparent growth rate constant of bacteria; kslope, kill rate constant due to PMB; kslope,med, kill rate constant for a theoretical starting inoculum of 6 log10 CFU/thigh; kinoc, constant describing the inoculum effect on kslope; Cu, unbound PMB concentration; γ, power parameter for PMB effect.
FIGURE 2. VPCs of the final PK model for total (red) and unbound (blue) PMB plasma concentrations, stratified by dose. Circles represent observed data, solid lines represent the median of the simulations and the colored-shaded areas depict the 80% prediction intervals for 1,000 simulated profiles. Dashed lines correspond to the limits of quantification (0.1 mg/L and 0.62 mg/L for total (red) and unbound (blue) concentrations, respectively). Note the different axis scales.
All untreated infected animals in the control group survived after 24 h. The time courses of bacterial loads after a single dose of PMB at 15 and 40 mg/kg are shown in Figure 3 for the three inocula. At the start of PMB treatment (2 h post-infection), bacterial counts were equal to 5.8 ± 0.4, 7.1 ± 0.5 and 8.0 ± 0.5 log10 CFU/thigh in mice infected with 1.5 × 105, 1.4 × 106 and 1.3 × 107 CFU/thigh inoculum, respectively. For the 105 CFU/thigh inoculum, bacterial counts in the untreated control group increased for the first 6 h for all animals but two patterns are seen at 8 and 24 h post-infection. For half of the studied mice bacteria reached a plateau at 8 h (8.2 ± 0.6 log10 CFU/thigh). For the other half, unexpectedly low bacterial counts were observed (8 h: 4.2 ± 0.9 log10 CFU/thigh and 24 h: 3.6 ± 0.7 log10 CFU/thigh). At higher inocula, bacterial counts in the untreated control group increased until a plateau was reached 8 h (8.3 ± 0.3 log10 CFU/thigh) and 4 h (8.9 ± 0.1 log10 CFU/thigh) after infection with the 106 and 107 CFU/thigh inoculum, respectively. When infected mice were treated with PMB at 1 mg/kg, no differences in bacterial counts with the control group were observed (data not shown). For the 105 CFU/thigh inocula high bacterial load reductions of 2.6 ± 1.2 log10 CFU/thigh were observed 24 h after administration of 15 mg/kg PMB while a moderate efficacy of PMB was observed at 15 mg/kg for the two highest inocula (Figure 3). In contrast high reductions of 2.5 ± 2.3, 3.5 ± 0.9 and 2.1 ± 1.4 log10 CFU/thigh were observed 24 h after administration of 40 mg/kg PMB for 105, 106 and 107 CFU/thigh inocula respectively.
FIGURE 3. VPCs of the final PKPD model for bacterial counts, stratified by dose of PMB and starting inoculum. Circles represent experimental data, solid lines the median of simulated data and, colored areas depict the 80% prediction intervals for 1,000 simulated profiles. Dashed lines correspond to the limit of quantification (2.9 log10 CFU/thigh).
The time course of bacterial counts was adequately described by the model depicted on Figure 1. Parameter estimates with their corresponding uncertainties are summarized in Table 2. VPCs of the final model are shown on Figure 3 and GOF plots on Supplementary Figure S4. PMB bactericidal effect (kPMB) was best described by a power function:
Where kslope corresponds to the kill rate constant due to PMB (L/mg.h), Cu the unbound PMB concentration (mg/L) and
The inoculum effect was incorporated in the model as a decrease of kslope with increasing theoretical starting inoculum using a linear function:
Where kslope,med is the kill rate constant for a theoretical starting inoculum of 6 log10 CFU/thigh corresponding to the median of the starting inoculum tested in the present study (5, 6 or 7 log10 CFU/thigh) and kinoc is the constant describing the inoculum effect on kslope.
A decrease of kslope from 1.19 to 0.81 L/mg.h (−32%) was predicted for a starting inoculum increasing from 5 to 7 log10 CFU/thigh respectively. kPMB at various PMB concentrations was derived from Equation 5, using for each starting inoculum the corresponding kslope value, and converted into initial killing half-lives (IK-HL) (Table 3), as previously performed with the in vitro model (Akrong et al., 2021).
TABLE 3. Model derived initial killing half-lives (min) at various unbound PMB concentrations and starting inocula.
The data-points identified as outliers were samples with less than 105 CFU/thigh from the control group infected with inoculum 105 CFU/thigh at times 8 and 24 h. With the sensitivity analysis results, it was observed that these outliers were not influential regarding parameter estimates and simulations under the final model. Detailed results can be found in supplemental material (Supplementary Table S1 and Supplementary Figure S5).
These new study results can be compared with those previously obtained in relatively similar conditions, at least from PK standpoint (Landersdorfer et al., 2018). In both studies PMB was administered subcutaneously to neutropenic mice infected with K. pneumoniae (Landersdorfer et al., 2018) or A. baumannii, leading to saturable absorption rate. However although Landersdorfer et al. used a model with parallel linear and saturable absorption, an Emax model was sufficient to provide satisfactory fit of our data. Noticeably, while this nonlinear absorption was important to consider for PK and then PKPD modeling of these animal data, it would not be relevant in clinical practice since PMB is administered intravenously and not subcutaneously. Another difference was observed between these two studies in terms of elimination. We observed linear elimination characterized by a clearance value, whereas Landersdorfer et al. described their data, with again a model including parallel linear and saturable pathways. Doses ranging were comparable between these two studies (from 2 to 32 mg/kg for Landersdorfer et al. and from 1 to 40 mg/kg for us), and peak concentrations observed in Landersdorfer et al study at the highest dose, were close to 20 mg/L and therefore only slightly higher than in our study (15 mg/L).
Plasma protein binding results also demonstrate some discrepancies between the two studies. Although both studies showed extensive binding, close to 80 and 90%, our estimated unbound fraction (0.17 on average) was twice the previously reported value at 0.086 (Landersdorfer et al., 2018). This discrepancy may be explained by differences in methodology. We used ultra-filtration after correction for non-specific adsorption on membranes to determine unbound fraction individually in infected mice, whereas the previous study used ultracentrifugation and pooled plasma sampled drawn from infected mice and spiked with PMB. The latter methodology may seem more suitable for the determination of protein binding of drugs, such as PMB, that exhibit significant nonspecific binding to laboratory material including ultrafiltration membranes (Cheah et al., 2015). Although, ultrafiltration has also been used to determine unbound concentrations of daptomycin, another antibiotic known to adhere to ultrafiltration membranes, after evaluation of non-specific binding by regression methods (Kim et al., 2008; Grégoire et al., 2019). This two-fold difference in unbound fractions should complicate PKPD modeling comparisons between these two studies, but not the IE investigated during this new study.
PKPD results of this new study could not be compared with Landersdorfer et al., not only because the bacterial species were different, but also because we have performed repeated measurements of bacterial counts over time, to describe a bacterial count versus time profile, which constitutes a major originality of our study. We have first compared the bacterial count versus time profiles at various initial inocula, with those predicted by combining our PK model in mice previously discussed, with the PD model that we previously developed to characterize the IE in vitro (Akrong et al., 2021). Such promising simulations can be found in publications that apply modelling to in vitro time-kill and/or hollow-fiber data (Yadav et al., 2015; Ly et al., 2016; Mohamed et al., 2016; Kristoffersson et al., 2019). However in vivo data are missing to evaluate the predictive ability of those simulations. In the present study, in vivo data show (Figure 4) that initial CFU decay with time is less rapid than predicted by the in vitro PD model, but more importantly the rapid regrowth observed in vitro was no more apparent in vivo. These in vitro—in vivo discrepancies invite caution when making recommendations based on predictions of models based solely on in vitro data. The reasons for these discrepancies should be further investigated and the importance of performing in vivo experiments is reinforced. These differences in model are schematically represented on Supplementary Figure S6.
FIGURE 4. Bacterial counts (CFU/thigh) versus time profiles at various initial inocula predicted by combining the PMB mice PK model, with the PD model previously developed to characterize the inoculum effect in vitro (Akrong et al., 2021). Solid circles represent experimental data and solid lines represent model predictions.
An innovative aspect of this study was the refinement of a PKPD model, based on the model developed after in vitro TK experiments conducted with A. baumannii and PMB (Akrong et al., 2021). The comparison between PD parameter values obtained after in vitro and in vivo data fitting indicated that the apparent growth rate constant was lower in vivo (0.594 h−1) (Table 2) than in vitro (1.62 h−1) (Akrong et al., 2021), as previously shown for E. coli (0.76 versus 1.30 h−1) (de Araujo et al., 2011). The different growth behavior between in vitro and in vivo has been associated in literature to the different environmental conditions, with higher volumes and nutritional factors more abundantly available in vitro than in vivo leading to a medium favorable to bacterial growth (Gloede et al., 2010; Mouton, 2018). As an illustration, the bactericidal effect of PMB characterized by the typical killing rates (kPMB), described by a power function in vivo and by a sigmoid Emax model in vitro, were respectively equal to 1.25 h−1 and 6.55 h−1 for initial inocula of 106 CFU/thigh and 106 CFU/ml and PMB concentration of 4 mg/L, that corresponds almost to the unbound peak concentration of PMB after a dose of 40 mg/kg. Similarly, a two times smaller maximum killing effect was previously observed for piperacillin against E. coli in a murine thigh infection model as compared to in vitro (de Araujo et al., 2011).
In both in vitro and in vivo PKPD models, a decrease in PMB killing effect was related to the baseline inoculum and was modeled either as a decrease of the in vivo PMB killing rate constant (kslope) or as an increase of the in vitro half-maximal effective concentration of PMB (EC50), making PMB IE difficult to compare between in vitro and in vivo. In vivo IK-HL were derived from Equation 5 for each starting inoculum and various PMB concentrations to better illustrate the consequences of IE on PMB activity (Table 3). In the present study, the modelling suggests that the in vivo IE is moderate and not concentration dependent with a mean IK-HL 48% higher at 107 CFU/thigh compared with 105 CFU/thigh inoculum (48 vs. 32.4 min). In contrast, our in vitro model suggested that the IE was PMB-concentration dependent and attenuated at high PMB concentrations (Akrong et al., 2021). As an example, at a PMB concentration equal to 4 mg/L, in vitro IK-HL increased by 60% (from 5 to 8 min) when the starting inoculum increased from 105 to 107 CFU/ml, whereas it increased by 140% (from 10 to 24 min) for a PMB concentration 16 times lower (0.25 mg/L).
The clinical relevance of in vitro IE has been questioned in previous studies (Maglio et al., 2004; Maglio et al., 2005; Fantin et al., 2019). Indeed, in the case of cefepime (Maglio et al., 2004) and ertapenem (Maglio et al., 2005) in vitro elevations of E. coli MICs were seen with an initial inoculum at 107 CFU/ml compared to 105 CFU/ml, while no IE was observed in vivo (neutropenic mouse thigh infection model). On the other hand, Fantin et al. observed an in vivo IE in mice infected with E. coli and treated by colistin (Fantin et al., 2019).
In conclusion, a PKPD model has been successfully developed to characterize the in vivo IE of A. baumannii on PMB, which confirms the IE observed in vitro. The PKPD model previously developed from in vitro TKC data was modified to take into account the intrinsic differences between in vitro and in vivo experimental infection models. The comparison between in vitro and in vivo PKPD parameters was not straightforward, especially due to the absence of in vivo regrowth. Yet although less pronounced than in vitro, the initial inoculum size of A. baumannii had a real impact on in vivo PMB activity.
The raw data supporting the conclusions of this article will be made available by the authors, without undue reservation.
The animal study was reviewed and approved by the local ethics committee (COMETHEA) and registered by the French Ministry of Higher Education and Research (approval numbers: 2019022216097190 and 2017072415099072).
AC, GA, JB, WC and SM contributed to conception and design of the study. AC, GA, JM, LP, HM, JB performed experiments. AC and VA-C performed PKPD modelling. AC and GA wrote the first draft of the manuscript. AC, GA, VA-C, WC and SM wrote sections of the manuscript. All authors contributed to manuscript revision, read, and approved the submitted version.
The financial support of GA was provided by the Nouvelle Aquitaine Region and Inserm. This work had benefited from the facilities and expertise of PREBIOS platform (University of Poitiers).
The authors declare that the research was conducted in the absence of any commercial or financial relationships that could be construed as a potential conflict of interest.
All claims expressed in this article are solely those of the authors and do not necessarily represent those of their affiliated organizations, or those of the publisher, the editors and the reviewers. Any product that may be evaluated in this article, or claim that may be made by its manufacturer, is not guaranteed or endorsed by the publisher.
The Supplementary Material for this article can be found online at: https://www.frontiersin.org/articles/10.3389/fphar.2022.842921/full#supplementary-material
Akrong, G., Chauzy, A., Aranzana-Climent, V., Lacroix, M., Deroche, L., Prouvensier, L., et al. (2022). A New Pharmacokinetic-Pharmacodynamic Model to Characterize the Inoculum Effect of Acinetobacter Baumannii on Polymyxin B In Vitro. Antimicrob. Agents Chemother. 66, e0178921. doi:10.1128/AAC.01789-21
Beal, S. L. (2001). Ways to Fit a PK Model with Some Data below the Quantification Limit. J. Pharmacokinet. Pharmacodyn. 28, 481–504. doi:10.1023/a:1012299115260
Bedenić, B., Beader, N., and Zagar, Z. (2001). Effect of Inoculum Size on the Antibacterial Activity of Cefpirome and Cefepime against Klebsiella pneumoniae Strains Producing SHV Extended-Spectrum Beta-Lactamases. Clin. Microbiol. Infect. 7, 626–635. doi:10.1046/j.1198-743x.2001.x
Chastre, J., and Fagon, J. Y. (2002). Ventilator-associated Pneumonia. Am. J. Respir. Crit. Care Med. 165, 867–903. doi:10.1164/ajrccm.165.7.2105078
Cheah, S. E., Wang, J., Nguyen, V. T., Turnidge, J. D., Li, J., and Nation, R. L. (2015). New Pharmacokinetic/pharmacodynamic Studies of Systemically Administered Colistin against Pseudomonas aeruginosa and Acinetobacter Baumannii in Mouse Thigh and Lung Infection Models: Smaller Response in Lung Infection. J. Antimicrob. Chemother. 70, 3291–3297. doi:10.1093/jac/dkv267
de Araujo, B. V., Diniz, A., Palma, E. C., Buffé, C., and Dalla Costa, T. (2011). PK-PD Modeling of β-lactam Antibiotics: In Vitro or In Vivo Models? J. Antibiot. (Tokyo) 64, 439–446. doi:10.1038/ja.2011.29
Dosne, A. G., Bergstrand, M., Harling, K., and Karlsson, M. O. (2016). Improving the Estimation of Parameter Uncertainty Distributions in Nonlinear Mixed Effects Models Using Sampling Importance Resampling. J. Pharmacokinet. Pharmacodyn. 43, 583–596. doi:10.1007/s10928-016-9487-8
El-Saed, A., Balkhy, H. H., Al-Dorzi, H. M., Khan, R., Rishu, A. H., and Arabi, Y. M. (2013). Acinetobacter Is the Most Common Pathogen Associated with Late-Onset and Recurrent Ventilator-Associated Pneumonia in an Adult Intensive Care Unit in Saudi Arabia. Int. J. Infect. Dis. 17, e696–701. doi:10.1016/j.ijid.2013.02.004
Fantin, B., Poujade, J., Grégoire, N., Chau, F., Roujansky, A., Kieffer, N., et al. (2019). The Inoculum Effect of Escherichia coli Expressing Mcr-1 or Not on Colistin Activity in a Murine Model of Peritonitis. Clin. Microbiol. Infect. 25, 1563–e8. doi:10.1016/j.cmi.2019.08.021
Ferran, A. A., Kesteman, A. S., Toutain, P. L., and Bousquet-Mélou, A. (2009). Pharmacokinetic/pharmacodynamic Analysis of the Influence of Inoculum Size on the Selection of Resistance in Escherichia coli by a Quinolone in a Mouse Thigh Bacterial Infection Model. Antimicrob. Agents Chemother. 53, 3384–3390. doi:10.1128/AAC.01347-08
García‐Garmendia, J. L., Ortiz‐Leyba, C., Garnacho‐Montero, J., Jiménez‐Jiménez, F. J., Pérez‐Paredes, C., Barrero‐Almodóvar, A. E., et al. (2001). Risk Factors forAcinetobacter baumanniiNosocomial Bacteremia in Critically Ill Patients: A Cohort Study. Clin. Infect. Dis. 33, 939–946. doi:10.1086/322584
Garnacho, J., Sole-Violan, J., Sa-Borges, M., Diaz, E., and Rello, J. (2003). Clinical Impact of Pneumonia Caused by Acinetobacter Baumannii in Intubated Patients: A Matched Cohort Study. Crit. Care Med. 31, 2478–2482. doi:10.1097/01.CCM.0000089936.09573.F3
Garnacho-Montero, J., and Timsit, J. F. (2019). Managing Acinetobacter Baumannii Infections. Curr. Opin. Infect. Dis. 32, 69–76. doi:10.1097/QCO.0000000000000518
Gil-Perotin, S., Ramirez, P., Marti, V., Sahuquillo, J. M., Gonzalez, E., Calleja, I., et al. (2012). Implications of Endotracheal Tube Biofilm in Ventilator-Associated Pneumonia Response: a State of Concept. Crit. Care 16, R93. doi:10.1186/cc11357
Gloede, J., Scheerans, C., Derendorf, H., and Kloft, C. (2010). In Vitro pharmacodynamic Models to Determine the Effect of Antibacterial Drugs. J. Antimicrob. Chemother. 65, 186–201. doi:10.1093/jac/dkp434
Grégoire, N., Marchand, S., Ferrandière, M., Lasocki, S., Seguin, P., Vourc’h, M., et al. (2019). Population Pharmacokinetics of Daptomycin in Critically Ill Patients with Various Degrees of Renal Impairment. J. Antimicrob. Chemother. 74, 117–125. doi:10.1093/jac/dky374
Harada, Y., Morinaga, Y., Kaku, N., Nakamura, S., Uno, N., Hasegawa, H., et al. (2014). In Vitro and In Vivo Activities of Piperacillin-Tazobactam and Meropenem at Different Inoculum Sizes of ESBL-Producing Klebsiella pneumoniae. Clin. Microbiol. Infect. 20, O831–O839. doi:10.1111/1469-0691.12677
Kalanuria, A. A., Ziai, W., Zai, W., and Mirski, M. (2014). Ventilator-associated Pneumonia in the ICU. Crit. Care 18, 208. doi:10.1186/cc13775
Kim, A., Suecof, L. A., Sutherland, C. A., Gao, L., Kuti, J. L., and Nicolau, D. P. (2008). In Vivo Microdialysis Study of the Penetration of Daptomycin into Soft Tissues in Diabetic versus Healthy Volunteers. Antimicrob. Agents Chemother. 52, 3941–3946. doi:10.1128/AAC.00589-08
Koenig, S. M., and Truwit, J. D. (2006). Ventilator-Associated Pneumonia: Diagnosis, Treatment, and Prevention. Clin. Microbiol. Rev. 19, 637–657. doi:10.1128/CMR.00051-05
Kristoffersson, A. N., Bissantz, C., Okujava, R., Haldimann, A., Walter, I., Shi, T., et al. (2019). A Novel Mechanism-Based Pharmacokinetic–Pharmacodynamic (PKPD) Model Describing Ceftazidime/avibactam Efficacy against β-lactamase-producing Gram-Negative Bacteria. J. Antimicrob. Chemother. 75, 400–408. doi:10.1093/jac/dkz440
Landersdorfer, C. B., Wang, J., Wirth, V., Chen, K., Kaye, K. S., Tsuji, B. T., et al. (2018). Pharmacokinetics/pharmacodynamics of Systemically Administered Polymyxin B against Klebsiella pneumoniae in Mouse Thigh and Lung Infection Models. J. Antimicrob. Chemother. 73, 462–468. doi:10.1093/jac/dkx409
Lee, D. G., Murakami, Y., Andes, D. R., and Craig, W. A. (2013). Inoculum Effects of Ceftobiprole, Daptomycin, Linezolid, and Vancomycin with Staphylococcus aureus and Streptococcus Pneumoniae at Inocula of 10(5) and 10(7) CFU Injected into Opposite Thighs of Neutropenic Mice. Antimicrob. Agents Chemother. 57, 1434–1441. doi:10.1128/AAC.00362-12
Lenhard, J. R., and Bulman, Z. P. (2019). Inoculum Effect of β-lactam Antibiotics. J. Antimicrob. Chemother. 74, 2825–2843. doi:10.1093/jac/dkz226
Li, R. C., and Ma, H. H. (1998). Parameterization of Inoculum Effect via Mathematical Modeling: Aminoglycosides against Staphylococcus aureus and Escherichia coli. J. Chemother. 10, 203–207. doi:10.1179/joc.1998.10.3.203
Lin, Y. W., Zhou, Q. T., Han, M. L., Chen, K., Onufrak, N. J., Wang, J., et al. (2018). Elucidating the Pharmacokinetics/Pharmacodynamics of Aerosolized Colistin against Multidrug-Resistant Acinetobacter Baumannii and Klebsiella pneumoniae in a Mouse Lung Infection Model. Antimicrob. Agents Chemother. 62, e01790. doi:10.1128/AAC.01790-17
López-Rojas, R., McConnell, M. J., Jiménez-Mejías, M. E., Domínguez-Herrera, J., Fernández-Cuenca, F., and Pachón, J. (2013). Colistin Resistance in a Clinical Acinetobacter Baumannii Strain Appearing after Colistin Treatment: Effect on Virulence and Bacterial Fitness. Antimicrob. Agents Chemother. 57, 4587–4589. doi:10.1128/AAC.00543-13
Ly, N. S., Bulman, Z. P., Bulitta, J. B., Baron, C., Rao, G. G., Holden, P. N., et al. (2016). Optimization of Polymyxin B in Combination with Doripenem to Combat Mutator Pseudomonas aeruginosa. Antimicrob. Agents Chemother. 60, 2870–2880. doi:10.1128/AAC.02377-15
Maglio, D., Banevicius, M. A., Sutherland, C., Babalola, C., Nightingale, C. H., and Nicolau, D. P. (2005). Pharmacodynamic Profile of Ertapenem against Klebsiella pneumoniae and Escherichia coli in a Murine Thigh Model. Antimicrob. Agents Chemother. 49, 276–280. doi:10.1128/AAC.49.1.276-280.2005
Maglio, D., Ong, C., Banevicius, M. A., Geng, Q., Nightingale, C. H., and Nicolau, D. P. (2004). Determination of the In Vivo Pharmacodynamic Profile of Cefepime against Extended-Spectrum-Beta-Lactamase-Producing Escherichia coli at Various Inocula. Antimicrob. Agents Chemother. 48, 1941–1947. doi:10.1128/AAC.48.6.1941-1947.2004
Mizunaga, S., Kamiyama, T., Fukuda, Y., Takahata, M., and Mitsuyama, J. (2005). Influence of Inoculum Size of Staphylococcus aureus and Pseudomonas aeruginosa on In Vitro Activities and In Vivo Efficacy of Fluoroquinolones and Carbapenems. J. Antimicrob. Chemother. 56, 91–96. doi:10.1093/jac/dki163
Mohamed, A. F., Kristoffersson, A. N., Karvanen, M., Nielsen, E. I., Cars, O., and Friberg, L. E. (2016). Dynamic Interaction of Colistin and Meropenem on a WT and a Resistant Strain of Pseudomonas aeruginosa as Quantified in a PK/PD Model. J. Antimicrob. Chemother. 71, 1279–1290. doi:10.1093/jac/dkv488
Mouton, J. W. (2018). Soup with or without Meatballs: Impact of Nutritional Factors on the MIC, Kill-Rates and Growth-Rates. Eur. J. Pharm. Sci. 125, 23–27. doi:10.1016/j.ejps.2018.09.008
Rio-Marques, L., Hartke, A., and Bizzini, A. (2014). The Effect of Inoculum Size on Selection of In Vitro Resistance to Vancomycin, Daptomycin, and Linezolid in Methicillin-Resistant Staphylococcus aureus. Microb. Drug Resist. 20, 539–543. doi:10.1089/mdr.2014.0059
Sader, H. S., Rhomberg, P. R., Flamm, R. K., and Jones, R. N. (2012). Use of a Surfactant (Polysorbate 80) to Improve MIC Susceptibility Testing Results for Polymyxin B and Colistin. Diagn. Microbiol. Infect. Dis. 74, 412–414. doi:10.1016/j.diagmicrobio.2012.08.025
Smith, K. P., and Kirby, J. E. (2018). The Inoculum Effect in the Era of Multidrug Resistance: Minor Differences in Inoculum Have Dramatic Effect on MIC Determination. Antimicrob. Agents Chemother. 62, e00433. doi:10.1128/AAC.00433-18
Tam, V. H., Schilling, A. N., and Nikolaou, M. (2005). Modelling Time-Kill Studies to Discern the Pharmacodynamics of Meropenem. J. Antimicrob. Chemother. 55, 699–706. doi:10.1093/jac/dki086
Wong, D., Nielsen, T. B., Bonomo, R. A., Pantapalangkoor, P., Luna, B., and Spellberg, B. (2017). Clinical and Pathophysiological Overview of Acinetobacter Infections: a Century of Challenges. Clin. Microbiol. Rev. 30, 409–447. doi:10.1128/CMR.00058-16
Keywords: inoculum effect, polymyxin B (PMB), in vivo, PKPD, modelling, Acinetobacter baumannii
Citation: Chauzy A, Akrong G, Aranzana-Climent V, Moreau J, Prouvensier L, Mirfendereski H, Buyck JM, Couet W and Marchand S (2022) PKPD Modeling of the Inoculum Effect of Acinetobacter baumannii on Polymyxin B in vivo. Front. Pharmacol. 13:842921. doi: 10.3389/fphar.2022.842921
Received: 24 December 2021; Accepted: 28 February 2022;
Published: 16 March 2022.
Edited by:
Markus Zeitlinger, Medical University of Vienna, AustriaReviewed by:
Hanna Evelina Sidjabat, Griffith University, AustraliaCopyright © 2022 Chauzy, Akrong, Aranzana-Climent, Moreau, Prouvensier, Mirfendereski, Buyck, Couet and Marchand. This is an open-access article distributed under the terms of the Creative Commons Attribution License (CC BY). The use, distribution or reproduction in other forums is permitted, provided the original author(s) and the copyright owner(s) are credited and that the original publication in this journal is cited, in accordance with accepted academic practice. No use, distribution or reproduction is permitted which does not comply with these terms.
*Correspondence: William Couet, d2lsbGlhbS5jb3VldEB1bml2LXBvaXRpZXJzLmZy
Disclaimer: All claims expressed in this article are solely those of the authors and do not necessarily represent those of their affiliated organizations, or those of the publisher, the editors and the reviewers. Any product that may be evaluated in this article or claim that may be made by its manufacturer is not guaranteed or endorsed by the publisher.
Research integrity at Frontiers
Learn more about the work of our research integrity team to safeguard the quality of each article we publish.