- Department of Oncology, West China Hospital of Sichuan University, Chengdu, China
The discovery of oncogenes and immune checkpoints has revolutionized the treatment of melanoma in the past 10 years. However, the current PD-L1 checkpoints lack specificity for tumors and target normal cells expressing PD-L1, thus reducing the efficacy on malignant melanoma and increasing the side effects. In addition, the treatment options for primary or secondary drug-resistant melanoma are limited. Bispecific antibodies bind tumor cells and immune cells by simultaneously targeting two antigens, enhancing the anti-tumor targeting effect and cytotoxicity and reducing drug-resistance in malignant melanoma, thus representing an emerging strategy to improve the clinical efficacy. This review focused on the treatment of malignant melanoma by bispecific antibodies and summarized the effective results of the experiments that have been conducted, also discussing the different aspects of these therapies. The role of the melanoma epitopes, immune cell activation, cell death and cytotoxicity induced by bispecific antibodies were evaluated in the clinical or preclinical stage, as these therapies appear to be the most suitable in the treatment of malignant melanoma.
Background
Melanoma is considered a multifactorial disease due to trauma, sunlight, biology, immunity, and genetic susceptibility. It has a predominant cutaneous localization, as compared with other cancers. Thus, early detection is still a key factor in reducing mortality (Lai et al., 2018). Melanoma spreads easily and metastasizes rapidly, and patients with metastases are almost always incurable disease, resulting in the death of most of these patients (Xie et al., 2022). The high risk of metastasis is the cause of the complexity of melanoma treatment.
Generally, melanoma is treated using comprehensive treatments. When detected at an early stage, the tumor can be removed by local resection, extensive local resection, and lymphadenectomy (Saltman et al., 2010). However, the effect of the surgical treatment is very limited in patients with advanced stages and tumor metastases, and comprehensive treatments including radiotherapy and drug therapy, are indispensable (Cherobin et al., 2018). Current chemotherapeutic drugs are of limited effect for most patients with advanced melanoma (Kozovska et al., 2016). Until recently, dacarbazine has been considered as the standard chemotherapeutic drug for metastatic melanoma, although the best effect is a partial remission (Koller et al., 2016). Driver mutations of melanoma often occur in the regulation of proliferation, metabolism, apoptosis and cell cycle control (Scolyer et al., 2011). BRAF and MAPK mutation are considered early carcinogenic events in melanoma (Davis et al., 2019). Striking BRAF inhibitors, including vemurafenib and dabrafenib, are effective in metastatic or advanced BRAF-mutated melanoma patients (Chapman et al., 2011). Although BRFA inhibitors are quite effective in BRAF-mutated melanoma patients, most of the patients develop a secondary resistance after an effective treatment. Then, immunomodulators emerged to treat melanoma, including IFN and IL-2, providing a promising approach for further research. One of the immunomodifiers developed for metastatic melanoma is interleukin 2 (IL2), which promotes the expansion of melanoma-specific T cells (Byrne and Fisher, 2017). In addition, the immunosuppressive molecules CD200 and immune checkpoint proteins expressed on melanoma cells, such as CTLA-4 and PD-L1, have also been identified as immunotherapeutic candidates (Talebian et al., 2012). Currently, three immune checkpoint inhibitors are used to treat melanoma, including the anti-CTLA-4 antibody ipilimumab and the two anti-PD-1 antibodies nivolumab and pembrolizumab (Topalian et al., 2015). Iplimumab treatment results in a long-lasting survival of up to 10 years in 20% of advanced melanoma cases, representing a great improvement compared to the median survival of less than 1 year in patients without immunotherapy (Sharma and Allison, 2015; Topalian et al., 2015). Although checkpoint inhibitors are promising treatments, the activation of the immune system surely causes a variety of side effects (Davis et al., 2019). In addition, the problem of immunotherapy resistance still needs to be resolved. Therefore, the exploration of new bispecific antibodies should be immediate.
Basic Characteristics of Bispecific Antibodies
Although monoclonal antibodies are clinically effective in treating solid tumors and hematological malignancies, bispecific antibodies can have altered forms and binding part of the molecule to enable the combination of various anti-tumor functions. These alterations include changes in size, binding valence and geometric shapes. Changes in the binding part, including linker length or domain composition, may affect the function of the bispecific antibodies. According to different functional requirements, bispecific antibodies are designed to have different advantages in anti-tumor therapy (Lim et al., 2021). According to the assembly of bispecific antibody building blocks, the format of the molecule is divided into no Fc and Fc. Bispecific antibody fragments without Fc can easily combine multiple antibody fragments (Goebeler et al., 2016). However, these formats do not have Fc regions, their plasma half-life is very short and lacks Fc-mediated functions, including immune-mediated cell death. In addition, the lack of the Fc segment in the composition of bispecific antibodies may lead to a decreased stability of the antibodies and promote their mutual aggregation, thus affecting their biological functions (Kontermann and Brinkmann, 2015; Ayyar et al., 2016). In addition to the shortcomings mentioned above, they are simple in structure, small in size, with a good tissue permeability, and easy to produce (Labrijn et al., 2013). Bispecific antibodies with Fc structure have a long half-life in plasma and Fc-mediated cytotoxicity. The functions include complement-dependent cytotoxicity, Ab-dependent phagocytosis, and the induction of antibody-dependent cellular cytotoxicity. Another advantage is that its purification process is easy, with high stability and good solubility (Li et al., 2020). In conclusion, bispecific antibodies modified by different methods can achieve specific useful functions, including the regulation of immunogenicity, effector function and antibody half-life. Bispecific antibodies also kill tumor cells expressing specific targets, including internal tumor antigens and increase the number of effector T cells in tumor lesions (Weisbart et al., 2012; Ma et al., 2019; de Jong et al., 2021).
Thus, bispecific antibodies have a great potential in the treatment of melanoma, and large clinical trials with enough data are needed to clarify the best position of these drugs in the treatment paradigm and their effects on patients at an advanced stage of melanoma. The checkpoint inhibitors of melanoma, T cell co-stimulation, the target of multiple melanoma epitopes and melanoma intracellular targets are interesting areas that require further research in bispecific antibodies. Thus, this review explores the current status and future treatment prospects of bispecific antibodies in melanoma. Bispecific antibodies are divided into four groups based on the mechanism of action: 1) Tumor-targeted immunomodulators, 2) tumor-targeted death, 3) engaging immune cells and 4) regulation of intracellular targets.
Potential targets and current antibodies under development are summarized in Figures 1–4 and Table 1.
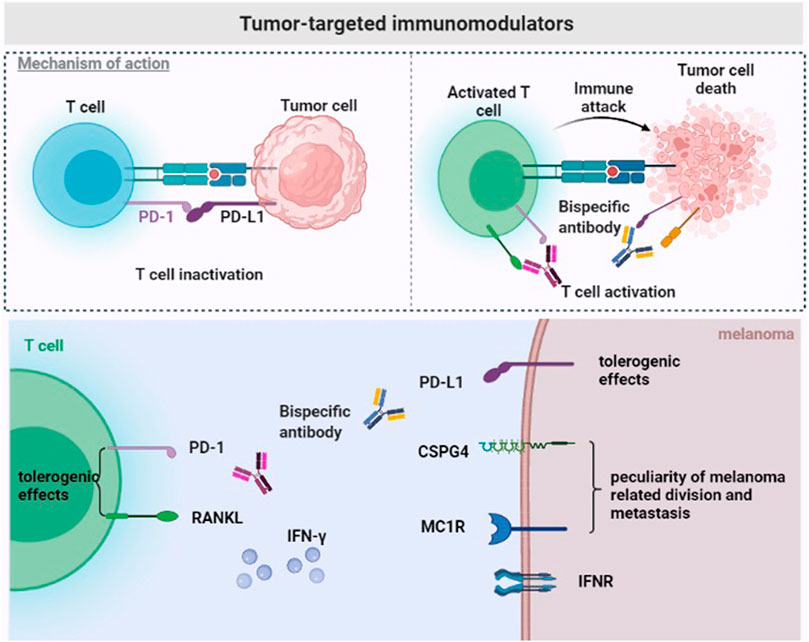
FIGURE 1. Tumor-targeted immunomodulators. The immunosuppressive tumor microenvironment of melanoma. Bispecific antibodies exert anti-tumor effects by binding to tumor-specific antigens (CSPG4/MC1R) and PD-L1, or to double immune checkpoints (RANKL and PD-1) on the surface of T cells.
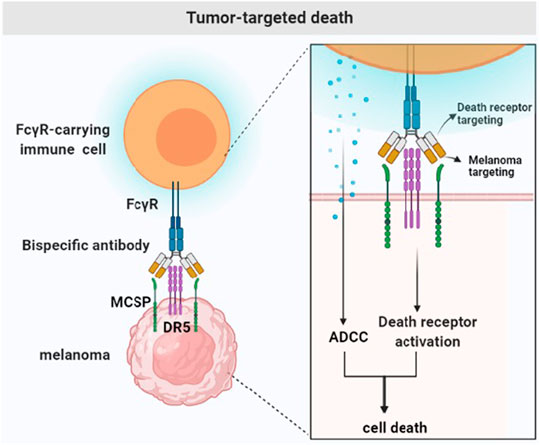
FIGURE 2. Tumor-targeted death. Tumor drug resistance is due to the prevention of the initiation or execution of the cell death signal. Bispecific antibodies bind to the tumor-specific antigen (MCSP) to activate the death signaling pathway (DR5) and FcγR-carrying immune cells.
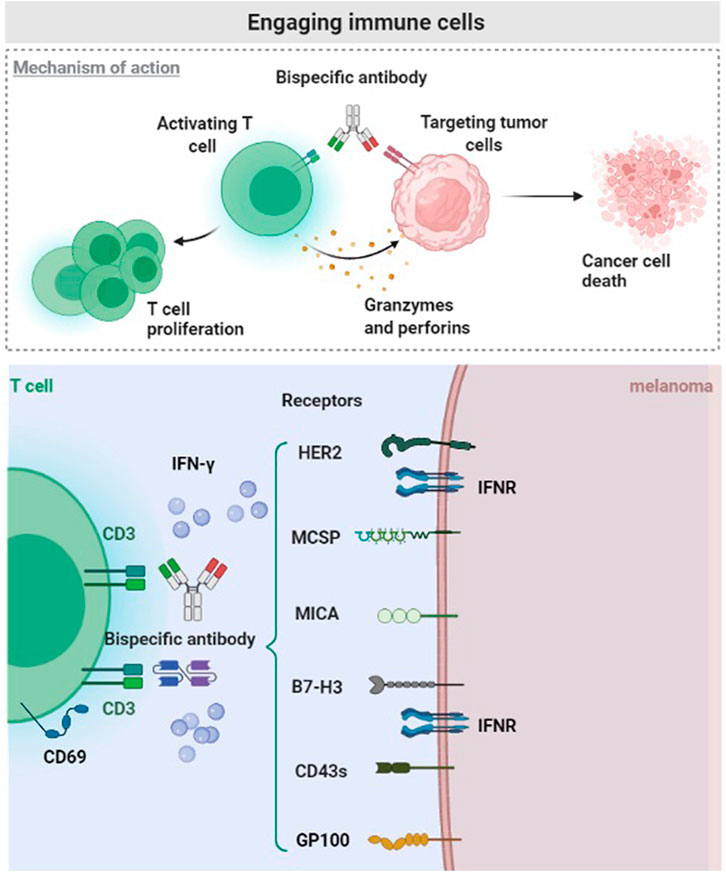
FIGURE 3. Engaging immune cells. The malignant melanoma microenvironment hinders the transport, infiltration and activation of T cells. CD3 related Bispecific antibodies bind tumor-specific antigen (HER2, MCSP, MICA, B7-H3, CD43s, and Glycoprotein 100), potentially promoting the intratumoral infiltration and response of T cells.
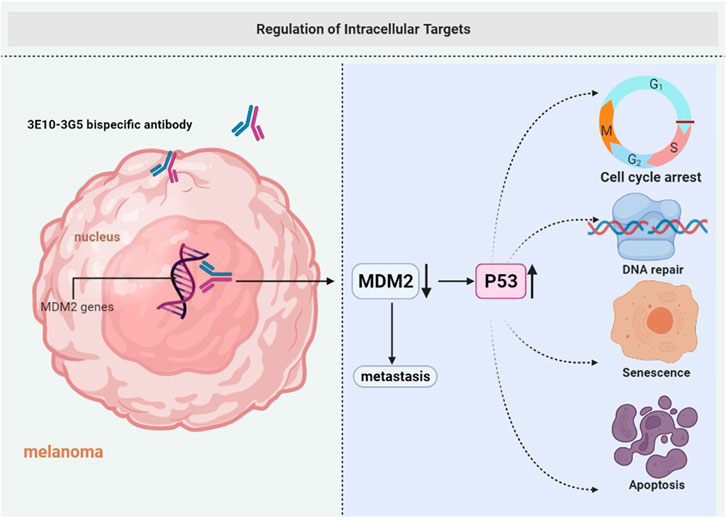
FIGURE 4. Regulation of intracellular targets. Cell membranes are impermeable to most macromolecules. 3E10-3G5 bispecific antibody retains cell-penetrating and MDM2-binding activities, increases tumor p53 expression, and inhibits the growth of tumors.
Tumor-Targeted Immunomodulators
Although the current PD1/PD-L1 blocking antibody approved by the FDA shows significant therapeutic effects, especially in patients with melanoma and non-small cell lung cancer, these antibodies lack tumor-specific selectivity. Therefore, they arbitrarily activate T cells, including autoreactive T cells that are harmful to the body.
Chondroitin sulfate proteoglycan 4 (CSPG4), a type 1 transmembrane protein, is composed of a 280 kDa glycoprotein and a 450 kDa chondroitin sulfate proteoglycan and is also called MCSP. CSPG4 is highly expressed in various refractory malignant tumors, such as triple-negative breast cancer, glioblastoma, mesothelioma, and melanoma (Wang et al., 2010; Svendsen et al., 2011; Rivera et al., 2012). A study reported that CSPG4 is overexpressed in more than 90% of melanoma lesions and is not present in healthy adult tissues. Therefore, it represents a significant target antigen to combat malignant tumors. The bispecific antibody PD-L1xCSPG4 may improve the selectivity, effectiveness and safety of the therapies against malignant tumors overexpressing CSPG4, including melanoma. Importantly, some results indicate that the bispecific antibody PD-L1xCSPG4 induces T cell activation by targeting the CSPG4 antigen. In this case, PDL1xCSPG4 enhances the ability of T cells to secrete IFN–γ, thus exerting an anti-tumor effect (Koopmans et al., 2019) (Figure 1).
A report shows that the bispecific antibody avelumab composed of a melanocyte stimulating hormone (α-MSH) analog and PD-L1 antibody inhibits checkpoints on the tumor microenvironment and prevents systemic immune activation. Melanocortin 1 receptor (MC1R) is a marker of the risk of melanoma overexpressed in more than 80% of melanomas (Rosenkranz et al., 2013). It belongs to the melanocortin receptor group and binds to α-MSH to cause a biological response. The high MC1R expression not only represents a molecular characteristic of melanoma, but also has important implications for tumor cell division and metastasis. The bispecific antibody avelumab composed of α-MSH analog and PD-L1 antibody may exert a cytotoxic effect, reverse the immune suppression in the tumor environment, and reduce side effects (Zhang et al., 2019) (Figure 1).
Bintrafusp alfa works by targeting PD-L1 through IgG, and the peptide chain ends are fused with the extracellular domain of two TGF-β receptor II molecules, designed to “capture TGF-β” in the tumor microenvironment. According to the results of phase I clinical trials, bispecific antibodies are similar to other checkpoint inhibitors in safety but they have a better objective and effective clinical remission rate (Lind et al., 2020).
RANK (TNFRSF11a) and RANKL (TNFSF11) belong to the tumor necrosis factor superfamily (Anderson et al., 1997). Recently, the RANK signal in bone marrow cells is considered as tolerogenic under various conditions (Williamson et al., 2002; Demoulin et al., 2015; Fujimura et al., 2015). This suggests that RANKL/RANK antagonists can be potentially used as an anti-tumor therapy through immune activation. In addition, RANK and RANKL are overexpressed in tumor cells and different immune cell types in the tumor microenvironment. RANK is overexpressed in tumor infiltrating bone marrow cells, and RANKL is overexpressed in effector T cells and tumor cells (Lacey et al., 2012; Ahern et al., 2018a). RANKL/RANK blockade induces tumor- associated myeloid cell suppression and increases the number of tumor infiltrating lymphocytes to reverse T cell dysfunction in the tumor microenvironment. According to other reports, the simultaneous effect of anti-RANKL and immune checkpoint blockers enhances the anti-tumor effect in various mouse tumor types (such as prostate, fibrosarcoma and melanoma). This treatment method controls subcutaneous tumor growth or metastasis and, more importantly, in an anti-PD-1 resistant environment (Ahern et al., 2017; Bakhru et al., 2017; Ahern et al., 2018b). The superior therapeutic effect of the bispecific antibody anti-RANKL/PD-1 compared with the simultaneous action of two antibodies anti-RANKL and anti-PD-1 may be due to better biodistribution of anti-RANKL/PD-1, which the bispecific antibody to elevated levels of RANKL and PD-1 expressed in the TME, including, but not limited to, co-expression of both target antigens on CD8+TILs. The increased affinity obtained by the simultaneous co-target of two antigens may lead to a more precise targeting of tumors and a greater degree of immune checkpoint blockade. In addition, “real world” clinical evidence derived from case reports and retrospective clinical analysis of patients with advanced melanoma shows that the simultaneous inhibition of RANKL and PD-L1 promotes the efficacy of immune checkpoint inhibitors (Liede et al., 2018). Anti-RANKL/PD-1 especially shows an effective inhibition of tumor growth in an immune checkpoint inhibitor resistant environment, providing a new treatment strategy against tumors with advanced drug-resistance (Dougall et al., 2019) (Figure 1).
Tumor-Targeted Death
An important factor leading to tumor resistance is the inhibition of the activation or conduction of cell death signals. Therefore, drugs need to induce cell death to destroy drug resistance, especially in cohorts of patients with poor prognosis (Hanahan and Weinberg, 2011). Death receptor-5 (DR5) is overexpressed in melanoma cells and activates cell death signals by binding to death ligand cytokines. Tumor necrosis factor (TNF), tumor necrosis factor-related apoptosis-inducing ligand (TRAIL) and CD95 ligand provides a direct induction of cell death. Among these death ligand cytokines, TRAIL has attracted considerable interest because of its specificity for transformed cells (Leverkus et al., 2000). TRAIL represents an attractive treatment for receptor-mediated apoptosis. It has attracted considerable attention due to its selective killing of tumor cells (Pan et al., 1997) and induction of apoptosis when binding to its own receptor, because TRAIL provides a strategy to directly trigger cell death. According to reports, the bispecific, tetravalent antibody MCSPxDR5 targets MCSP and simultaneously activates death receptor 5 (DR5, TRAIL-R2). The bispecific antibody is specific to MCSP-positive melanoma cells and activates the DR5-dependent death signaling pathway and exerts a cytotoxic activity. The cross-linking of antibodies to Fcγ receptors further increases the cytotoxic potential without affecting the selectivity. This method uses three coordinated processes: The binding of death receptor agonists in malignant tumor cells, the effective activation of cell death signals, and the recruitment of immune cells. Therefore, MCSPxDR5 can guide the anti-DR5 apoptotic function on melanoma cells, effectively and selectively killing melanoma cells expressing MCSP and DR5 (Szegezdi and Leverkus, 2016) (Figure 2).
Most of the therapeutic antibodies targeting ovarian cancer are largely disappointing due to the limited cytotoxicity of tumor-specific antibodies. Bispecific targeted cytotoxicity activator specifically binds to folate receptor α-1 (FOLR1) and DR5 to achieve “cis” and “trans” cytotoxicity. The DR5 signaling pathway is activated in vivo by interacting with FcγRIIB, and FOLR1 anchoring represents the basis in the maintenance of high levels of tumor-specific cell apoptosis (Shivange et al., 2018).
Engaging Immune Cells
The tumor microenvironment in solid tumors hinders the transport and infiltration of T cells. CD3 related bispecific antibodies may promote T cell infiltration and HLA-independent T cell activation in tumors. All T cells express the CD3 antigen on the surface.
Many experiments proved that T cell-based therapies produce partial or complete responses to advanced melanoma, including T-cell gene therapy and adoptive T-cell therapy (Berry et al., 2009). MCSP expression varies in different melanoma cell lines, but 94% of MCSP expression is positive overall. The expression of MCSP antigen in melanoma cells is a promising target for immunotherapy, according to the characteristics of bispecific T cell junction (BiTE) (Torisu-Itakura et al., 2011). The tumor-specific antigen binds to one arm, and the CD3 subunit of T cell receptor binds to the other arm of the bispecific antibody, effectively stimulating T cell activation. Therefore, BiTE antibody directly activates T cells and does not require dendritic cells to produce specific T cell clones or antigen presentation (Baeuerle et al., 2009; Schmidt et al., 2011). MCSP-BiTE antibody co-cultured with peripheral mononuclear blood cells or CD8+ T cells mediates the lysis of MCSP positive melanoma cells (Torisu-Itakura et al., 2011) (Figure 3).
Immune checkpoint blockade achieved compelling results in malignant tumors and shows broad prospects (Larkin et al., 2015). B7-H3 is another immune checkpoint, and it is a member of the B7/CD28 immunoglobulin superfamily, also known as CD276. It is a type I transmembrane glycoprotein and a promising immunosuppressant (Steinberger et al., 2004). Importantly, B7-H3 is an inhibitory receptor for T cell activation and it is less expressed in healthy tissues, but overexpressed in various human malignancies (Wang et al., 2014; Picarda et al., 2016). In addition to the promotion of immune evasion by the tumor, B7-H3 is also related to tumor proliferation, apoptosis, adhesion, angiogenesis, invasion and metastasis (Castellanos et al., 2017). The overexpression of B7-H3 in melanoma is negatively related to the survival rate of patients, it participates in many metastatic-related pathways, and promotes tumor progression (Tekle et al., 2012; Wang et al., 2013). Studies confirmed that B7-H3 is a promising target for the activation of T cell immunotherapy. One arm of B7-H3 bispecific antibody recognizes tumor-specific antigens and the other arm binds immune cells. B7-H3 bispecific antibody activated T cells and produce higher levels of IFN-γ when interacting with melanoma cells, consequently exerting a tumor-killing effect (Ma et al., 2019) (Figure 3).
CD43 is a mucin-like transmembrane protein composed of serine and threonine residues (Tuccillo et al., 2014). It is considered as a marker of hematopoietic cells and is highly expressed in acute myeloid leukemia and myelodysplastic syndromes (Rosenstein et al., 1999). It is also highly expressed in various solid malignant tumors, and it is related to tumor promotion (Balikova et al., 2012; Batdorf et al., 2017). More importantly, CD43s is overexpressed in melanoma cell lines and patients’ melanoma samples. The synthesis of the bispecific T-cell activator based on AT1413 and CD3 where AT1413 is a ligand for CD43s is now possible. Two functional T-cell engager forms of AT1413 were synthesized, and the dual bivalent b T-cell engager format is approximately 50 times more effective than the unit price KiH format. These T-cell engagers effectively activate T cells to kill melanoma cells (de Jong et al., 2021) (Figure 3).
The epidermal growth factor receptor 2 (HER2), also known as erbB2, belongs to the epidermal growth factor receptor family. HER2 is highly expressed in various malignant tumors, such as female reproductive system malignancies and digestive tract malignancies. Many studies demonstrated that HER2 is expressed during the progression and metastasis of melanoma while it is not expressed in normal melanocytes. According to reports, HER2 was detected in 10 metastatic melanoma patients, and 8 of them showed a high expression (Bodey et al., 1997). A preclinical study evaluates the specific cytotoxic effect of Malme-3M-luc cells by anti-CD3 x anti-HER2 bispecific antibody (HER2Bi-Ab). High levels of the activation marker CD69 and secretion of considerable higher levels of IFN-γ are produced by HER2Bi-armed ATC. Therefore, HER2Bi-Ab not only binds to tumor-specific antigens, but also activates T cells and promotes cytokine secretion (Ma et al., 2013) (Figure 3).
Claireet al. developed and characterized a novel BiTE molecule specific for the NKG2D ligand. NKG2D plays a role in recognizing the oncogenic transformation of cells (Lanier, 2015). The expression of NKG2D ligand is controlled at the transcription, translation and post-translational levels. The expression of NKG2D ligand on the cell surface increases when the cell is under infection, transformation or other stress state (Del Toro-Arreola et al., 2011). Major histocompatibility complex class I-related chain A (MICA) is one of the ligands of NKG2D and is widely expressed on many malignant tumors, including those of the genitourinary system, gastrointestinal tract, and respiratory system. MICA is overexpressed in 75% of skin melanoma cases and 50% of metastatic melanoma cases (Shi et al., 2014). It becomes promising targets for immunotherapy because their expression in normal tissues is not abundant. T cells anchor tumors with high expression of MICA. Therefore, B2-OKT3 BiTE can target MICA on tumor cells and CD3ε on T cells through the tandem scFv BiTE format, which specifically induces T cells to kill tumor cells (Godbersen et al., 2017) (Figure 3).
Uveal melanoma is a disease different from skin melanoma. The 1-year overall survival rate for patients with metastatic uveal melanoma is approximately 50%. Glycoprotein 100 (Gp-100) is a 100 kDa molecule specific for melanocytes of the skin, mucosa and retina. It is a glycosylated transmembrane protein involved in melanosome maturation, which are organelles that transport melanin and they are highly expressed in normal melanocytes and melanoma cells (Middleton et al., 2020; Martinez-Perez et al., 2021). Different approaches for treating melanoma with Gp-100 as an antigen have been developed, including Gp-100-based vaccines, mRNA electroporation of dendritic cells, and fusion proteins named IMCGp-100 or tebentafusp. Tebentafusp is a first-in-class anti-Gp-100 immune mobilizing antibody. This bispecific fusion protein composed of a soluble affinity-enhanced TCR and an anti-CD3 scFv, targets tumor cells that express a peptide of Gp-100 presented by HLA*A0201, creating an immune synapse that kills targeted tumor cells (Middleton et al., 2020). Tebentafusp treatment used in a randomized phase 3 clinical trial was positively correlated with the overall survival of metastatic uveal melanoma (Nathan et al., 2021) (Figure 3).
Currently, CD3-related bispecific antibodies are already used in clinical or preclinical trials of other tumors. The treatment options for HER2-negative breast cancer are limited. Phase I studies showed that anti-CD3 x anti-HER2 bispecific antibodies (HER2 BATs) activate T cells, representing a promising approach in the treatment of HER2-positive patients. A phase II trial evaluated the efficacy and immune response of HER2 BAT as an immuno-consolidation therapy after chemotherapy in HER2-negative patients. The use of HER2 BAT for immune consolidation after chemotherapy in a number of treated HER2 patients increases the number of stable patients and the median overall survival, as well as the adaptive and innate anti-tumor response (Lum et al., 2021). B cell maturation antigen is an effective target for relapsed or refractory multiple myeloma. Teclistamab binds to B cell maturation antigen and CD3 to form a bispecific antibody that redirects T cells to multiple myeloma cells. At the recommended dose in the phase 2 clinical trial, Teclistamab was maintained above the target dose level to achieve a consistent T cell activation. It shows high safety, good tolerability and curative effect in patients with relapsed or refractory multiple myeloma (Usmani et al., 2021).
Regulation of Intracellular Targets
Antibodies play an important role in tumor therapy, but their use in targeting intracellular tumor antigens is difficult. Cell membranes are impermeable to most macromolecules, thus, the modulation of intracellular target antigens is primarily based on the use of small molecules that can passively diffuse into cells. However, small molecule inhibitors are prone to off-target effects, leading to a significant toxicity (Zhang et al., 2009). Several different strategies have been explored to facilitate the passage of macromolecular proteins across cell membranes, including electroporation, microinjection, cell penetrating peptides, and liposomes (Singh et al., 2019).
In addition to the above-mentioned, a cell-penetrating monoclonal antibody 3E10 was developed as an intracellular delivery vehicle for the intracellular and intranuclear delivery of antibodies to overcome the limitation of intracellular targets. It is a rare monoclonal anti-DNA antibody that penetrates living cells and localizes in the nucleus without causing any appreciable damage to the cells (Hansen et al., 2007a). The monoclonal antibody 3E10 and its single-chain variable fragment (3E10 scFv) have been developed as intracellular delivery systems for macromolecules (Weisbart et al., 2004; Hansen et al., 2007b). The inhibition of MDM2 in melanoma immunotherapy increases active p53 expression and is associated with the death of human melanoma cell lines (Verhaegen et al., 2012). Since MDM2 is an important target in melanoma, the monoclonal antibody 3G5 is chosen for intracellular transport. The mAb 3G5 binds MDM2 and blocks MDM2 binding to p53. The 3E10-3G5 bispecific antibody retains cell-penetrating and MDM2-binding activities, increases tumor p53 expression, and inhibits the growth of MDM2-associated tumors (Weisbart et al., 2012) (Figure 4).
Immunotherapy is a promising chemotherapy against resistant leukemia. However, it is limited by the ability of targeting lineage-specific cell surface antigens. A large number of leukemia-related targets become accessible by targeting intracellular antigens. A novel T-cell bispecific (TCB) antibody was generated using CrossMab and Knob-in-pore technology, containing a bivalent T-cell receptor-like binding domain that recognizes RMFPNAPYL derived from intracellular tumor antigens peptide in the context of human leukocyte antigen (HLA) A*02, Wilms’ tumor 1 (WT1). The binding to CD3ε leads to the recruitment of T cells regardless of T cell receptor specificity. WT1-TCB promotes an efficient in vitro, ex vivo and in vivo killing of acute myelocytic leukemia cell lines and primary acute myelocytic leukemia. These results lead to the initiation of a phase I trial in patients with r/r AML (Augsberger et al., 2021).
Conclusion
Clinicians still face great challenges when faced with malignant melanoma, especially in patients with advanced cancer. Immunotherapy has improved the prognosis of melanoma over the past decade. Monoclonal antibodies exert their effects through various mechanisms of action (Redman et al., 2015). For example, antibodies directly block the binding of angiogenic factors to their receptors, or block cell mitosis through membrane tyrosine kinases. In addition, antibodies can stimulate the immune system of the patient and exert cytotoxic effects on the tumor, including antibody-dependent cellular phagocytosis, antibody-dependent cytotoxicity, and complement-mediated cytotoxicity (Agus et al., 2002; Strome et al., 2007; Sullivan and Brekken, 2010; Azoury et al., 2015). However, monoclonal antibodies used in some patients have been found as ineffective or inducing the progression of the disease. Thus, more effective treatment methods should be explored. The concept of bispecific antibodies first appeared in the 1980s (Raso and Griffin, 1981), and they can realize multiple antitumor functions among the above-mentioned regarding monoclonal antibodies. Bispecific antibodies have the specificity of two antibodies; thus, they can bind tightly to two different target molecules or cell types (Szegezdi and Leverkus, 2016). Adaptive immunity needs to initiate an antigen-specific response. The key step in this process is to activate T cells, which recognize and kill antigen-specific cells. Antigen presenting cells capture and process antigens, then enter the lymph nodes and present the processed antigens to major histocompatibility complex I/II antigen-specific T cell molecules. The complex T cell activation process leads to immune inactivation, resulting in the immunosuppression of the tumor microenvironment. The CD3 subunit is a protein for T cell activation, and it does not use cell antigen presentation of antigen presenting cells to simplify T cell activation. The bispecific antibody activates T cells while producing cytotoxicity through this mechanism. Blinatumomab (CD3 ×B lymphocyte antigen CD19) used for the treatment of B-cell acute lymphoblastic leukemia is an effective and advanced bispecific antibody (Lim et al., 2021). The PD1/PD-L1 blocking antibody currently approved by the FDA shows a significant therapeutic activity, especially in patients with advanced NSCLC and melanoma. The combination of immune checkpoints with specific antigens on immune cells or tumor cells form bispecific antibodies is a promising approach (Bhandaru and Rotte, 2019). CSPG4 and MC1R are surface antigens often expressed on melanoma cells, and they participate in many biological behaviors of melanoma. Therefore, these two tumor-specific antigens are used as target antigens for the development of new antibodies and vaccines (Bluemel et al., 2010). They can form bispecific antibodies with immune checkpoint to achieve more precise targeting, which may increase the anti-tumor effect while reducing the side effects of immunotherapy. One of the key aspects of tumor drug resistance is to block the induction or conduction of cell death signals. Drugs directly induce cell death to break drug resistance, thus activating the death receptors expressed in melanoma cells to achieve effective therapeutic effects. The cell membrane resists the passage of macromolecular proteins, resulting in the inability of antibodies to bind to the internal antigens of tumor cells. Bispecific antibodies can be used as carriers on the one hand and be combined with intracellular antigens on the other hand, so as to achieve a wider range of biological effects produced by antigen-antibody binding.
Future Outlooks/Challenges
Although bispecific antibodies are superior to monoclonal antibodies in terms of therapeutic effects, their structure is more complex, with large molecular weight and strong immunogenicity, which may induce the production of anti-drug antibodies (ADA) and lead to undesirable consequences. ADA can destroy or eliminate antibody activity by removing it from the circulation or blocking its function. The factors that determine the immunogenicity of bispecific antibodies are diverse and complex (Harding et al., 2010), and it is generally believed that immunogenicity is closely related to the homology of endogenous substances. The lower the homology of proteins to the corresponding endogenous counterpart, the higher its immunogenic potential (Husain and Ellerman, 2018). Co-administration of methotrexate and TNF-α antibody may reduce the rate of ADA formation in inflammatory bowel disease (Sasson et al., 2021). It has also been reported that avoiding antibiotic therapy may reduce the risk of ADA during anti-TNF therapy (Gorelik et al., 2021). However, reports on ADA treatment in cancer therapy are rare. Therefore, further studies are needed to reduce their immunogenicity to make bispecific antibodies with broader application prospects.
Furthermore, bispecific antibody fragments lacking the Fc region require continuous infusion to maintain a constant blood concentration because of their short half-life (Portell et al., 2013). Two popular bispecific antibody platforms successfully tried to add the Fc region to overcome this challenge. Another way to extend the half-life is to add albumin or albumin binding entities (Kontermann, 2011).
Cytokine release syndrome and neurotoxicity are the two main aspects of bispecific antibody toxicity. The first consists of the release of cytokines mediated by the activation of immune cells in the body, which is a systemic inflammatory response (Li et al., 2019), while the biological mechanism of neurotoxicity remains unclear (Stein et al., 2019). Both cytokine release syndrome and neurotoxicity are reduced by gradual administration of dexamethasone, administration of dexamethasone before the therapy, or temporary treatment discontinuation (Clynes and Desjarlais, 2019; Klinger et al., 2020). Our aim in the future is to explore more in-depth multivalent and multispecific antibodies, which could widen the way of bispecific antibodies on treating melanoma.
Author Contributions
JT collected the appropriate literature and wrote the manuscript. YG drew the figure and made the table. XM revised the manuscript. All authors agreed to be responsible for the content of the work.
Conflict of Interest
The authors declare that the research was conducted in the absence of any commercial or financial relationships that could be construed as a potential conflict of interest.
Publisher’s Note
All claims expressed in this article are solely those of the authors and do not necessarily represent those of their affiliated organizations, or those of the publisher, the editors and the reviewers. Any product that may be evaluated in this article, or claim that may be made by its manufacturer, is not guaranteed or endorsed by the publisher.
References
Agus, D. B., Akita, R. W., Fox, W. D., Lewis, G. D., Higgins, B., Pisacane, P. I., et al. (2002). Targeting Ligand-Activated ErbB2 Signaling Inhibits Breast and Prostate Tumor Growth. Cancer cell 2, 127–137. doi:10.1016/S1535-6108(02)00097-1
Ahern, E., Harjunpää, H., Barkauskas, D., Allen, S., Takeda, K., Yagita, H., et al. (2017). Co-administration of RANKL and CTLA4 Antibodies Enhances Lymphocyte-Mediated Antitumor Immunity in Mice. Clin. Cancer Res. 23, 5789–5801. doi:10.1158/1078-0432.ccr-17-0606
Ahern, E., Harjunpää, H., O'Donnell, J. S., Allen, S., Dougall, W. C., Teng, M. W. L., et al. (2018b). RANKL Blockade Improves Efficacy of PD1-PD-L1 Blockade or Dual PD1-PD-L1 and CTLA4 Blockade in Mouse Models of Cancer. Oncoimmunology 7, e1431088. doi:10.1080/2162402x.2018.1431088
Ahern, E., Smyth, M. J., Dougall, W. C., and Teng, M. W. L. (2018a). Roles of the RANKL-RANK axis in Antitumour Immunity - Implications for Therapy. Nat. Rev. Clin. Oncol. 15, 676–693. doi:10.1038/s41571-018-0095-y
Anderson, D. M., Maraskovsky, E., Billingsley, W. L., Dougall, W. C., Tometsko, M. E., Roux, E. R., et al. (1997). A Homologue of the TNF Receptor and its Ligand Enhance T-Cell Growth and Dendritic-Cell Function. Nature 390, 175–179. doi:10.1038/36593
Augsberger, C., Hänel, G., Xu, W., Pulko, V., Hanisch, L. J., Augustin, A., et al. (2021). Targeting Intracellular WT1 in AML with a Novel RMF-peptide-MHC-specific T-Cell Bispecific Antibody. Blood 138, 2655–2669. doi:10.1182/blood.2020010477
Ayyar, B. V., Arora, S., and O'Kennedy, R. (2016). Coming-of-Age of Antibodies in Cancer Therapeutics. Trends Pharmacol. Sci. 37, 1009–1028. doi:10.1016/j.tips.2016.09.005
Azoury, S. C., Straughan, D. M., and Shukla, V. (2015). Immune Checkpoint Inhibitors for Cancer Therapy: Clinical Efficacy and Safety. Curr. Cancer Drug Targets 15, 452–462. doi:10.2174/156800961506150805145120
Baeuerle, P. A., Kufer, P., and Bargou, R. (2009). BiTE: Teaching Antibodies to Engage T-Cells for Cancer Therapy. Curr. Opin. Mol. Ther. 11, 22–30.
Bakhru, P., Zhu, M. L., Wang, H. H., Hong, L. K., Khan, I., Mouchess, M., et al. (2017). Combination central Tolerance and Peripheral Checkpoint Blockade Unleashes Antimelanoma Immunity. JCI insight 2, e93265. doi:10.1172/jci.insight.93265
Balikova, A., Jääger, K., Viil, J., Maimets, T., and Kadaja-Saarepuu, L. (2012). Leukocyte Marker CD43 Promotes Cell Growth in Co-operation with β-catenin in Non-hematopoietic Cancer Cells. Int. J. Oncol. 41, 299–309. doi:10.3892/ijo.2012.1440
Batdorf, B. H., Kroft, S. H., Hosking, P. R., Harrington, A. M., Mackinnon, A. C., and Olteanu, H. (2017). Evaluation of CD43 Expression in Non-hematopoietic Malignancies. Ann. Diagn. Pathol. 29, 23–27. doi:10.1016/j.anndiagpath.2017.04.010
Berry, L. J., Moeller, M., and Darcy, P. K. (2009). Adoptive Immunotherapy for Cancer: the Next Generation of Gene-Engineered Immune Cells. Tissue antigens 74, 277–289. doi:10.1111/j.1399-0039.2009.01336.x
Bhandaru, M., and Rotte, A. (2019). Monoclonal Antibodies for the Treatment of Melanoma: Present and Future Strategies. Methods Mol. Biol. 1904, 83–108. doi:10.1007/978-1-4939-8958-4_4
Bluemel, C., Hausmann, S., Fluhr, P., Sriskandarajah, M., Stallcup, W. B., Baeuerle, P. A., et al. (2010). Epitope Distance to the Target Cell Membrane and Antigen Size Determine the Potency of T Cell-Mediated Lysis by BiTE Antibodies Specific for a Large Melanoma Surface Antigen. Cancer Immunol. Immunother. 59, 1197–1209. doi:10.1007/s00262-010-0844-y
Bodey, B., Bodey, B., Gröger, A. M., Luck, J. V., Siegel, S. E., Taylor, C. R., et al. (1997). Clinical and Prognostic Significance of the Expression of the C-erbB-2 and C-erbB-3 Oncoproteins in Primary and Metastatic Malignant Melanomas and Breast Carcinomas. Anticancer Res. 17, 1319–1330.
Byrne, E. H., and Fisher, D. E. (2017). Immune and Molecular Correlates in Melanoma Treated with Immune Checkpoint Blockade. Cancer 123, 2143–2153. doi:10.1002/cncr.30444
Castellanos, J. R., Purvis, I. J., Labak, C. M., Guda, M. R., Tsung, A. J., Velpula, K. K., et al. (2017). B7-H3 Role in the Immune Landscape of Cancer. Am. J. Clin. Exp. Immunol. 6, 66–75.
Chapman, P. B., Hauschild, A., Robert, C., Haanen, J. B., Ascierto, P., Larkin, J., et al. (2011). Improved Survival with Vemurafenib in Melanoma with BRAF V600E Mutation. N. Engl. J. Med. 364, 2507–2516. doi:10.1056/NEJMoa1103782
Cherobin, A. C. F. P., Wainstein, A. J. A., Colosimo, E. A., Goulart, E. M. A., and Bittencourt, F. V. (2018). Prognostic Factors for Metastasis in Cutaneous Melanoma. Bras Dermatol. 93, 19–26. doi:10.1590/abd1806-4841.20184779
Clynes, R. A., and Desjarlais, J. R. (2019). Redirected T Cell Cytotoxicity in Cancer Therapy. Annu. Rev. Med. 70, 437–450. doi:10.1146/annurev-med-062617-035821
Davis, L. E., Shalin, S. C., and Tackett, A. J. (2019). Current State of Melanoma Diagnosis and Treatment. Cancer Biol. Ther. 20, 1366–1379. doi:10.1080/15384047.2019.1640032
de Jong, G., Bartels, L., Kedde, M., Verdegaal, E. M. E., Gillissen, M. A., Levie, S. E., et al. (2021). Melanoma Cells Can Be Eliminated by Sialylated CD43 × CD3 Bispecific T Cell Engager Formats In Vitro and In Vivo. Cancer Immunol. Immunother. 70, 1569–1581. doi:10.1007/s00262-020-02780-9
Del Toro-Arreola, S., Arreygue-Garcia, N., Aguilar-Lemarroy, A., Cid-Arregui, A., Jimenez-Perez, M., Haramati, J., et al. (2011). MHC Class I-Related Chain A and B Ligands Are Differentially Expressed in Human Cervical Cancer Cell Lines. Cancer Cel Int 11, 15. doi:10.1186/1475-2867-11-15
Demoulin, S. A., Somja, J., Duray, A., Guénin, S., Roncarati, P., Delvenne, P. O., et al. (2015). Cervical (Pre)neoplastic Microenvironment Promotes the Emergence of Tolerogenic Dendritic Cells via RANKL Secretion. Oncoimmunology 4, e1008334. doi:10.1080/2162402x.2015.1008334
Dougall, W. C., Roman Aguilera, A., and Smyth, M. J. (2019). Dual Targeting of RANKL and PD-1 with a Bispecific Antibody Improves Anti-tumor Immunity. Clin. Transl Immunol. 8, e01081. doi:10.1002/cti2.1081
Fujimura, T., Kambayashi, Y., Furudate, S., Asano, M., Kakizaki, A., and Aiba, S. (2015). Receptor Activator of NF-Κb Ligand Promotes the Production of CCL17 from RANK+ M2 Macrophages. J. Invest. Dermatol. 135, 2884–2887. doi:10.1038/jid.2015.209
Godbersen, C., Coupet, T. A., Huehls, A. M., Zhang, T., Battles, M. B., Fisher, J. L., et al. (2017). NKG2D Ligand-Targeted Bispecific T-Cell Engagers Lead to Robust Antitumor Activity against Diverse Human Tumors. Mol. Cancer Ther. 16, 1335–1346. doi:10.1158/1535-7163.mct-16-0846
Goebeler, M. E., Knop, S., Viardot, A., Kufer, P., Topp, M. S., Einsele, H., et al. (2016). Bispecific T-Cell Engager (BiTE) Antibody Construct Blinatumomab for the Treatment of Patients with Relapsed/Refractory Non-hodgkin Lymphoma: Final Results from a Phase I Study. J. Clin. Oncol. 34, 1104–1111. doi:10.1200/jco.2014.59.1586
Gorelik, Y., Freilich, S., Gerassy-Vainberg, S., Pressman, S., Friss, C., Blatt, A., et al. (2022). Antibiotic Use Differentially Affects the Risk of Anti-drug Antibody Formation during Anti-tnfα Therapy in Inflammatory Bowel Disease Patients: a Report from the Epi-IIRN. Gut 71, 287–295. doi:10.1136/gutjnl-2021-325185
Hanahan, D., and Weinberg, R. A. (2011). Hallmarks of Cancer: the Next Generation. Cell 144, 646–674. doi:10.1016/j.cell.2011.02.013
Hansen, J. E., Fischer, L. K., Chan, G., Chang, S. S., Baldwin, S. W., Aragon, R. J., et al. (2007b). Antibody-mediated P53 Protein Therapy Prevents Liver Metastasis In Vivo. Cancer Res. 67, 1769–1774. doi:10.1158/0008-5472.can-06-3783
Hansen, J. E., Tse, C. M., Chan, G., Heinze, E. R., Nishimura, R. N., and Weisbart, R. H. (2007a). Intranuclear Protein Transduction through a Nucleoside Salvage Pathway. J. Biol. Chem. 282, 20790–20793. doi:10.1074/jbc.C700090200
Harding, F. A., Stickler, M. M., Razo, J., and DuBridge, R. B. (2010). The Immunogenicity of Humanized and Fully Human Antibodies: Residual Immunogenicity Resides in the CDR Regions. mAbs 2, 256–265. doi:10.4161/mabs.2.3.11641
Husain, B., and Ellerman, D. (2018). Expanding the Boundaries of Biotherapeutics with Bispecific Antibodies. BioDrugs 32, 441–464. doi:10.1007/s40259-018-0299-9
Klinger, M., Zugmaier, G., Nägele, V., Goebeler, M. E., Brandl, C., Stelljes, M., et al. (2020). Adhesion of T Cells to Endothelial Cells Facilitates Blinatumomab-Associated Neurologic Adverse Events. Cancer Res. 80, 91–101. doi:10.1158/0008-5472.can-19-1131
Koller, K. M., Wang, W., Schell, T. D., Cozza, E. M., Kokolus, K. M., Neves, R. I., et al. (2016). Malignant Melanoma-The Cradle of Anti-neoplastic Immunotherapy. Crit. Rev. Oncol. Hematol. 106, 25–54. doi:10.1016/j.critrevonc.2016.04.010
Kontermann, R. E., and Brinkmann, U. (2015). Bispecific Antibodies. Drug Discov. Today 20, 838–847. doi:10.1016/j.drudis.2015.02.008
Kontermann, R. E. (2011). Strategies for Extended Serum Half-Life of Protein Therapeutics. Curr. Opin. Biotechnol. 22, 868–876. doi:10.1016/j.copbio.2011.06.012
Koopmans, I., Hendriks, M. A. J. M., van Ginkel, R. J., Samplonius, D. F., Bremer, E., and Helfrich, W. (2019). Bispecific Antibody Approach for Improved Melanoma-Selective PD-L1 Immune Checkpoint Blockade. J. Invest. Dermatol. 139, 2343–e3. e2343. doi:10.1016/j.jid.2019.01.038
Kozovska, Z., Gabrisova, V., and Kucerova, L. (2016). Malignant Melanoma: Diagnosis, Treatment and Cancer Stem Cells. Neoplasma 63, 510–517. doi:10.4149/neo_2016_403
Labrijn, A. F., Meesters, J. I., de Goeij, B. E., van den Bremer, E. T., Neijssen, J., van Kampen, M. D., et al. (2013). Efficient Generation of Stable Bispecific IgG1 by Controlled Fab-Arm Exchange. Proc. Natl. Acad. Sci. U S A. 110, 5145–5150. doi:10.1073/pnas.1220145110
Lacey, D. L., Boyle, W. J., Simonet, W. S., Kostenuik, P. J., Dougall, W. C., Sullivan, J. K., et al. (2012). Bench to Bedside: Elucidation of the OPG-RANK-RANKL Pathway and the Development of Denosumab. Nat. Rev. Drug Discov. 11, 401–419. doi:10.1038/nrd3705
Lai, V., Cranwell, W., and Sinclair, R. (2018). Epidemiology of Skin Cancer in the Mature Patient. Clin. Dermatol. 36, 167–176. doi:10.1016/j.clindermatol.2017.10.008
Lanier, L. L. (2015). NKG2D Receptor and its Ligands in Host Defense. Cancer Immunol. Res. 3, 575–582. doi:10.1158/2326-6066.cir-15-0098
Larkin, J., Chiarion-Sileni, V., Gonzalez, R., Grob, J. J., Cowey, C. L., Lao, C. D., et al. (2015). Combined Nivolumab and Ipilimumab or Monotherapy in Untreated Melanoma. N. Engl. J. Med. 373, 23–34. doi:10.1056/NEJMc150966010.1056/NEJMoa1504030
Leverkus, M., Neumann, M., Mengling, T., Rauch, C. T., Bröcker, E. B., Krammer, P. H., et al. (2000). Regulation of Tumor Necrosis Factor-Related Apoptosis-Inducing Ligand Sensitivity in Primary and Transformed Human Keratinocytes. Cancer Res. 60, 553–559.
Li, H., Er Saw, P., and Song, E. (2020). Challenges and Strategies for Next-Generation Bispecific Antibody-Based Antitumor Therapeutics. Cell Mol Immunol 17, 451–461. doi:10.1038/s41423-020-0417-8
Li, J., Piskol, R., Ybarra, R., Chen, Y. J., Li, J., Slaga, D., et al. (2019). CD3 Bispecific Antibody-Induced Cytokine Release Is Dispensable for Cytotoxic T Cell Activity. Sci. Transl Med. 11, eaax8861. doi:10.1126/scitranslmed.aax8861
Liede, A., Hernandez, R. K., Wade, S. W., Bo, R., Nussbaum, N. C., Ahern, E., et al. (2018). An Observational Study of Concomitant Immunotherapies and Denosumab in Patients with Advanced Melanoma or Lung Cancer. Oncoimmunology 7, e1480301. doi:10.1080/2162402x.2018.1480301
Lim, S. M., Pyo, K. H., Soo, R. A., and Cho, B. C. (2021). The Promise of Bispecific Antibodies: Clinical Applications and Challenges. Cancer Treat. Rev. 99, 102240. doi:10.1016/j.ctrv.2021.102240
Lind, H., Gameiro, S. R., Jochems, C., Donahue, R. N., Strauss, J., Gulley, J. L., et al. (2020). Dual Targeting of TGF-β and PD-L1 via a Bifunctional Anti-PD-l1/tgf-βrii Agent: Status of Preclinical and Clinical Advances. J. Immunother. Cancer 8, e000433. doi:10.1136/jitc-2019-000433
Lum, L. G., Al-Kadhimi, Z., Deol, A., Kondadasula, V., Schalk, D., Tomashewski, E., et al. (2021). Phase II Clinical Trial Using Anti-CD3 × Anti-HER2 Bispecific Antibody Armed Activated T Cells (HER2 BATs) Consolidation Therapy for HER2 Negative (0-2+) Metastatic Breast Cancer. J. Immunother. Cancer 9, e002194. doi:10.1136/jitc-2020-002194
Ma, J., Han, H., Liu, D., Li, W., Feng, H., Xue, X., et al. (2013). HER2 as a Promising Target for Cytotoxicity T Cells in Human Melanoma Therapy. PloS one 8, e73261. doi:10.1371/journal.pone.0073261
Ma, J., Shang, T., Ma, P., Sun, X., Zhao, J., Sun, X., et al. (2019). Bispecific Anti-CD3 X Anti-B7-H3 Antibody Mediates T Cell Cytotoxic Ability to Human Melanoma In Vitro and In Vivo. Invest. New Drugs 37, 1036–1043. doi:10.1007/s10637-018-00719-7
Martinez-Perez, D., Viñal, D., Solares, I., Espinosa, E., and Feliu, J. (2021). Gp-100 as a Novel Therapeutic Target in Uveal Melanoma. Cancers (Basel) 13, 5968. doi:10.3390/cancers13235968
Middleton, M. R., McAlpine, C., Woodcock, V. K., Corrie, P., Infante, J. R., Steven, N. M., et al. (2020). Tebentafusp, A TCR/Anti-CD3 Bispecific Fusion Protein Targeting Gp100, Potently Activated Antitumor Immune Responses in Patients with Metastatic Melanoma. Clin. Cancer Res. 26, 5869–5878. doi:10.1158/1078-0432.ccr-20-1247
Nathan, P., Hassel, J. C., Rutkowski, P., Baurain, J. F., Butler, M. O., Schlaak, M., et al. (2021). Overall Survival Benefit with Tebentafusp in Metastatic Uveal Melanoma. N. Engl. J. Med. 385, 1196–1206. doi:10.1056/NEJMoa2103485
Pan, G., Ni, J., Wei, Y.-F., Yu, G.-l., Gentz, R., and Dixit, V. M. (1997). An Antagonist Decoy Receptor and a Death Domain-Containing Receptor for TRAIL. SCIENCE 277, 815–818. doi:10.1126/science.277.5327.815
Picarda, E., Ohaegbulam, K. C., and Zang, X. (2016). Molecular Pathways: Targeting B7-H3 (CD276) for Human Cancer Immunotherapy. Clin. Cancer Res. 22, 3425–3431. doi:10.1158/1078-0432.ccr-15-2428
Portell, C. A., Wenzell, C. M., and Advani, A. S. (2013). Clinical and Pharmacologic Aspects of Blinatumomab in the Treatment of B-Cell Acute Lymphoblastic Leukemia. Clin. Pharmacol. 5, 5–11. doi:10.2147/cpaa.s42689
Raso, V., and Griffin, T. (1981). Hybrid Antibodies with Dual Specificity for the Delivery of Ricin to Immunoglobulin-Bearing Target Cells. Cancer Res. 41, 2073–2078.
Redman, J. M., Hill, E. M., AlDeghaither, D., and Weiner, L. M. (2015). Mechanisms of Action of Therapeutic Antibodies for Cancer. Mol. Immunol. 67, 28–45. doi:10.1016/j.molimm.2015.04.002
Rivera, Z., Ferrone, S., Wang, X., Jube, S., Yang, H., Pass, H. I., et al. (2012). CSPG4 as a Target of Antibody-Based Immunotherapy for Malignant Mesothelioma. Clin. Cancer Res. 18, 5352–5363. doi:10.1158/1078-0432.ccr-12-0628
Rosenkranz, A. A., Slastnikova, T. A., Durymanov, M. O., and Sobolev, A. S. (2013). Malignant Melanoma and Melanocortin 1 Receptor. Biochemistry (Mosc) 78, 1228–1237. doi:10.1134/s0006297913110035
Rosenstein, Y., Santana, A., and Pedraza-Alva, G. (1999). CD43, a Molecule with Multiple Functions. Immunol. Res. 20, 89–99. doi:10.1007/bf02786465
Saltman, B. E., Ganly, I., Patel, S. G., Coit, D. G., Brady, M. S., Wong, R. J., et al. (2010). Prognostic Implication of sentinel Lymph Node Biopsy in Cutaneous Head and Neck Melanoma. Head Neck 32, 1686–1692. doi:10.1002/hed.21390
Sasson, S. C., Wilkins, L. E., Watson, R. A., Jolly, C., Brain, O., Klenerman, P., et al. (2021). Identification of Neutralising Pembrolizumab Anti-drug Antibodies in Patients with Melanoma. Sci. Rep. 11, 19253. doi:10.1038/s41598-021-98700-7
Schmidt, P., Kopecky, C., Hombach, A., Zigrino, P., Mauch, C., and Abken, H. (2011). Eradication of Melanomas by Targeted Elimination of a Minor Subset of Tumor Cells. Proc. Natl. Acad. Sci. U S A. 108, 2474–2479. doi:10.1073/pnas.1009069108
Scolyer, R. A., Long, G. V., and Thompson, J. F. (2011). Evolving Concepts in Melanoma Classification and Their Relevance to Multidisciplinary Melanoma Patient Care. Mol. Oncol. 5, 124–136. doi:10.1016/j.molonc.2011.03.002
Sharma, P., and Allison, J. P. (2015). Immune Checkpoint Targeting in Cancer Therapy: toward Combination Strategies with Curative Potential. Cell 161, 205–214. doi:10.1016/j.cell.2015.03.030
Shi, P., Yin, T., Zhou, F., Cui, P., Gou, S., and Wang, C. (2014). Valproic Acid Sensitizes Pancreatic Cancer Cells to Natural Killer Cell-Mediated Lysis by Upregulating MICA and MICB via the PI3K/Akt Signaling Pathway. BMC cancer 14, 370. doi:10.1186/1471-2407-14-370
Shivange, G., Urbanek, K., Przanowski, P., Perry, J. S. A., Jones, J., Haggart, R., et al. (2018). A Single-Agent Dual-Specificity Targeting of FOLR1 and DR5 as an Effective Strategy for Ovarian Cancer. Cancer cell 34, 331–e11. e311. doi:10.1016/j.ccell.2018.07.005
Singh, K., Ejaz, W., Dutta, K., and Thayumanavan, S. (2019). Antibody Delivery for Intracellular Targets: Emergent Therapeutic Potential. Bioconjug. Chem. 30, 1028–1041. doi:10.1021/acs.bioconjchem.9b00025
Stein, A. S., Schiller, G., Benjamin, R., Jia, C., Zhang, A., Zhu, M., et al. (2019). Neurologic Adverse Events in Patients with Relapsed/refractory Acute Lymphoblastic Leukemia Treated with Blinatumomab: Management and Mitigating Factors. Ann. Hematol. 98, 159–167. doi:10.1007/s00277-018-3497-0
Steinberger, P., Majdic, O., Derdak, S. V., Pfistershammer, K., Kirchberger, S., Klauser, C., et al. (2004). Molecular Characterization of Human 4Ig-B7-H3, a Member of the B7 Family with Four Ig-like Domains. J. Immunol. 172, 2352–2359. doi:10.4049/jimmunol.172.4.2352
Strome, S. E., Sausville, E. A., and Mann, D. (2007). A Mechanistic Perspective of Monoclonal Antibodies in Cancer Therapy beyond Target-Related Effects. Oncologist 12, 1084–1095. doi:10.1634/theoncologist.12-9-1084
Sullivan, L. A., and Brekken, R. A. (2010). The VEGF Family in Cancer and Antibody-Based Strategies for Their Inhibition. mAbs 2, 165–175. doi:10.4161/mabs.2.2.11360
Svendsen, A., Verhoeff, J. J., Immervoll, H., Brøgger, J. C., Kmiecik, J., Poli, A., et al. (2011). Expression of the Progenitor Marker NG2/CSPG4 Predicts Poor Survival and Resistance to Ionising Radiation in Glioblastoma. Acta Neuropathol. 122, 495–510. doi:10.1007/s00401-011-0867-2
Szegezdi, E., and Leverkus, M. (2016). Guiding the Killer and Bringing in Accomplices: Bispecific Antibody Treatment for Malignant Melanoma. J. Invest. Dermatol. 136, 362–364. doi:10.1016/j.jid.2015.12.002
Talebian, F., Liu, J. Q., Liu, Z., Khattabi, M., He, Y., Ganju, R., et al. (2012). Melanoma Cell Expression of CD200 Inhibits Tumor Formation and Lung Metastasis via Inhibition of Myeloid Cell Functions. PloS one 7, e31442. doi:10.1371/journal.pone.0031442
Tekle, C., Nygren, M. K., Chen, Y. W., Dybsjord, I., Nesland, J. M., Maelandsmo, G. M., et al. (2012). B7-H3 Contributes to the Metastatic Capacity of Melanoma Cells by Modulation of Known Metastasis-Associated Genes. Int. J. Cancer 130, 2282–2290. doi:10.1002/ijc.26238
Topalian, S. L., Drake, C. G., and Pardoll, D. M. (2015). Immune Checkpoint Blockade: a Common Denominator Approach to Cancer Therapy. Cancer cell 27, 450–461. doi:10.1016/j.ccell.2015.03.001
Torisu-Itakura, H., Schoellhammer, H. F., Sim, M. S., Irie, R. F., Hausmann, S., Raum, T., et al. (20111997). Redirected Lysis of Human Melanoma Cells by a MCSP/CD3-bispecific BiTE Antibody that Engages Patient-Derived T Cells. J. Immunother. 34, 597–605. doi:10.1097/CJI.0b013e3182307fd8
Tuccillo, F. M., de Laurentiis, A., Palmieri, C., Fiume, G., Bonelli, P., Borrelli, A., et al. (20142014). Aberrant Glycosylation as Biomarker for Cancer: Focus on CD43. Biomed. Res. Int. 2014, 742831. doi:10.1155/2014/742831
Usmani, S. Z., Garfall, A. L., van de Donk, N. W. C. J., Nahi, H., San-Miguel, J. F., Oriol, A., et al. (2021). Teclistamab, a B-Cell Maturation Antigen × CD3 Bispecific Antibody, in Patients with Relapsed or Refractory Multiple Myeloma (MajesTEC-1): a Multicentre, Open-Label, Single-Arm, Phase 1 Study. The Lancet 398, 665–674. doi:10.1016/s0140-6736(21)01338-6
Verhaegen, M., Checinska, A., Riblett, M. B., Wang, S., and Soengas, M. S. (2012). E2F1-dependent Oncogenic Addiction of Melanoma Cells to MDM2. Oncogene 31, 828–841. doi:10.1038/onc.2011.277
Wang, J., Chong, K. K., Nakamura, Y., Nguyen, L., Huang, S. K., Kuo, C., et al. (2013). B7-H3 Associated with Tumor Progression and Epigenetic Regulatory Activity in Cutaneous Melanoma. J. Invest. Dermatol. 133, 2050–2058. doi:10.1038/jid.2013.114
Wang, L., Kang, F. B., and Shan, B. E. (2014). B7-H3-mediated Tumor Immunology: Friend or Foe? Int. J. Cancer 134, 2764–2771. doi:10.1002/ijc.28474
Wang, X., Osada, T., Wang, Y., Yu, L., Sakakura, K., Katayama, A., et al. (2010). CSPG4 Protein as a New Target for the Antibody-Based Immunotherapy of Triple-Negative Breast Cancer. J. Natl. Cancer Inst. 102, 1496–1512. doi:10.1093/jnci/djq343
Weisbart, R. H., Gera, J. F., Chan, G., Hansen, J. E., Li, E., Cloninger, C., et al. (2012). A Cell-Penetrating Bispecific Antibody for Therapeutic Regulation of Intracellular Targets. Mol. Cancer Ther. 11, 2169–2173. doi:10.1158/1535-7163.mct-12-0476-t
Weisbart, R. H., Wakelin, R., Chan, G., Miller, C. W., and Koeffler, P. H. (2004). Construction and Expression of a Bispecific Single-Chain Antibody that Penetrates Mutant P53 colon Cancer Cells and Binds P53. Int. J. Oncol. 25, 1113–1118. doi:10.1016/j.hoc.2004.06.011
Williamson, E., Bilsborough, J. M., and Viney, J. L. (2002). Regulation of Mucosal Dendritic Cell Function by Receptor Activator of NF-Kappa B (RANK)/RANK Ligand Interactions: Impact on Tolerance Induction. J. Immunol. 169, 3606–3612. doi:10.4049/jimmunol.169.7.3606
Xie, R., Li, B., Jia, L., and Li, Y. (2022). Identification of Core Genes and Pathways in Melanoma Metastasis via Bioinformatics Analysis. Int. J. Mol. Sci. 23, 794. doi:10.3390/ijms23020794
Zhang, J., Yang, P. L., and Gray, N. S. (2009). Targeting Cancer with Small Molecule Kinase Inhibitors. Nat. Rev. Cancer 9, 28–39. doi:10.1038/nrc2559
Keywords: bispecific antibody, melanoma, intracellular target, immunotherapy, cytotoxicity
Citation: Tang J, Gong Y and Ma X (2022) Bispecific Antibodies Progression in Malignant Melanoma. Front. Pharmacol. 13:837889. doi: 10.3389/fphar.2022.837889
Received: 17 December 2021; Accepted: 28 February 2022;
Published: 23 March 2022.
Edited by:
Albrecht Reichle, University Medical Center Regensburg, GermanyReviewed by:
Andrey A. Rosenkranz, Lomonosov Moscow State University, RussiaJizu Yi, Wuhan YZY Biopharma, China
Copyright © 2022 Tang, Gong and Ma. This is an open-access article distributed under the terms of the Creative Commons Attribution License (CC BY). The use, distribution or reproduction in other forums is permitted, provided the original author(s) and the copyright owner(s) are credited and that the original publication in this journal is cited, in accordance with accepted academic practice. No use, distribution or reproduction is permitted which does not comply with these terms.
*Correspondence: Youling Gong, Z29uZ3lvdWxpbmdAaG90bWFpbC5jb20=; Xuelei Ma, ZHJtYXh1ZWxlaUBnbWFpbC5jb20=
†These authors have contributed equally to this work and share last authorship