- 1Department of Molecular Medicine, Institute of Basic Medical Sciences, University of Oslo, Oslo, Norway
- 2Department of Research and Development, Division of Emergencies and Critical Care, Oslo University Hospital, Oslo, Norway
- 3Institute of Cytology, Russian Academy of Sciences, Saint Petersburg, Russia
- 4Department of Cardiothoracic Surgery, Oslo University Hospital, Oslo, Norway
- 5Sanifit Therapeutics, Palma de Mallorca, Spain
- 6Almazov National Medical Research Centre, Saint Petersburg, Russia
- 7Department of Woman and Children Health, Karolinska Institute, Stockholm, Sweden
- 8Norwegian Center for Stem Cell Research, Oslo University Hospital and University of Oslo, Oslo, Norway
- 9Institute of Immunology, Oslo University Hospital, Oslo, Norway
- 10Hybrid Technology Hub - Centre of Excellence, Institute of Basic Medical Sciences, University of Oslo, Oslo, Norway
- 11Department of Pediatric Research, Oslo University Hospital, Oslo, Norway
- 12Department of Pulmonary Diseases, Oslo University Hospital, Oslo, Norway
- 13Institute of Clinical Medicine, University of Oslo, Oslo, Norway
Aortic valve stenosis secondary to aortic valve calcification is the most common valve disease in the Western world. Calcification is a result of pathological proliferation and osteogenic differentiation of resident valve interstitial cells. To develop non-surgical treatments, the molecular and cellular mechanisms of pathological calcification must be revealed. In the current overview, we present methods for evaluation of calcification in different ex vivo, in vitro and in vivo situations including imaging in patients. The latter include echocardiography, scanning with computed tomography and magnetic resonance imaging. Particular emphasis is on translational studies of calcific aortic valve stenosis with a special focus on cell culture using human primary cell cultures. Such models are widely used and suitable for screening of drugs against calcification. Animal models are presented, but there is no animal model that faithfully mimics human calcific aortic valve disease. A model of experimentally induced calcification in whole porcine aortic valve leaflets ex vivo is also included. Finally, miscellaneous methods and aspects of aortic valve calcification, such as, for instance, biomarkers are presented.
1 Introduction
Calcific aortic valve disease (CAVD) is a slowly progressing disorder starting with non-symptomatic thickening and sclerosis of valve leaflets. Severe calcification causes deformation of the valve tissue and ultimately aortic stenosis (AS). It is the most common form of valve disease in the Western world and it will become an increasing health burden with an ageing populations (Stewart et al., 1997; Nkomo et al., 2006; Takkenberg et al., 2008; Osnabrugge et al., 2013).
Calcification of the aortic valve is an active, proliferative process that results from inflammation, fibrosis and bone matrix formation (Rajamannan et al., 2011; Mathieu et al., 2014; Rutkovskiy et al., 2017), mediated by the signaling pathways common to valvulogenesis and osteogenesis (Dutta and Lincoln, 2018). Currently, the main therapeutic option is open heart surgery and replacement with a valve prosthesis, although endovascular or minimally invasive techniques are being introduced for an increasing number of patients. Considering the costs and risks involved in surgical and endovascular replacement, pharmacological inhibition of CAVD ought to be a priority of research (Bhatia et al., 2016).
The basic mechanisms of aortic valve calcification are still poorly understood. Unraveling the cellular and molecular mechanisms of valve calcification may open up new therapeutic options, which is why researchers are in need of reliable and relevant models (Pawade et al., 2015). The valve interstitial cells (VIC) are believed to play a key role in the calcification process and VIC in culture appear to be the most relevant in vitro model for valve calcification (Takkenberg et al., 2008; Mathieu et al., 2014; Rutkovskiy et al., 2017). This is particularly true due to the lack of good animal models–the pathophysiology of aortic stenosis is quite unique to humans (Sider et al., 2011; Gomez Stallons et al., 2016). Experimentally induced calcification in VIC is an accurate and affordable model of in vitro aortic valve calcification. It is also suitable for screening potential pharmacological inhibitors. Different techniques or variants of in vitro models that are used in investigations leading to inconsistencies across the studies (Goto et al., 2019). This is also true for ex vivo and in vivo models of the disease, where the methods vary wildly.
Presently available models to study aortic valve calcification are not optimal, however, they are what we have for now. Exact techniques for measuring the amount of calcification as well as exact content of trace elements are important parts of research studies. Good methods for evaluating aortic valve calcification by different imaging techniques have become increasingly important due to catheter-based implantation of valve prostheses. Exact information about the calcification of the valve and valvular annulus are decisive for a successful result. The purpose of this article is to give an overview of methods for investigating cellular and molecular mechanisms of aortic valve calcification as well as techniques to measure the amount of calcium and calcification. Cultures of VIC have been given special attention as they are extensively used to study the cellular and molecular mechanisms of calcification. Furthermore, we present an overview of different in vitro, ex vivo, and in vivo models to study calcification as well as methods to investigate calcification and CAVD in patients.
2 Morphology of the Aortic Valve
Human aortic valve leaflets have three main layers: the fibrosa facing the aorta, the spongiosa in the middle and the ventricularis facing the ventricle. This tri-layer structure is populated with VIC, and the surface is covered with a monolayer of valve endothelial cells (VEC). VICs are able to synthesize and regulate remodeling of extracellular matrix components. VEC function as a barrier, a signaling interface, they produce a number of substances that potentially regulate VIC, and may have an active role in valve calcification (Tao et al., 2012; Rattazzi and Pauletto, 2015). Injury to the valve endothelium might even be the trigger of the whole process. In a healthy valve, VIC are quiescent and have characteristics akin to fibroblasts. Under certain conditions VIC can undergo differentiation to either myofibroblasts or osteoblast-like cells (Rutkovskiy et al., 2017). At the time of surgery, approximately 13% of stenotic valves have inclusions of osteoblasts and osteoclasts along with organized lamellar bone matrix. Around 83% have signs of dystrophic calcification, possibly mediated by myofibroblasts (Mohler et al., 2001). While the exact mechanism is unclear, the myofibroblasts may contract the extracellular matrix, creating cellular aggregates (nodules), where the cells undergo apoptosis. This leads to calcium phosphate precipitation around apoptotic bodies (Chen and Simmons, 2011) and turns micro-calcification into macro-calcification.
3 Measuring Amounts of Calcium
3.1 In Cell Cultures
3.1.1 Alizarin Red Staining and Quantification of Calcification in Cell Cultures
Alizarin Red (1.2-dihydroxyanthraquinone) staining is the most common method used to assess calcification in cell culture. It stains mineralized matrix, binding to different bivalent ions, mostly calcium. Although it is not the most accurate method to detect calcium content, it is optimal in terms of time consumption, simplicity, and cost (Bowler and Merryman, 2015). Alizarin Red provides a visual picture of calcium distribution in cell culture. It is also possible to quantify the signal by extracting the dye with acetic acid and measuring its concentration spectroscopically. Another common method for identification of calcium deposits is von Kossa staining. The latter dye unlike Alizarin Red reacts with phosphates and carbonates in calcium deposits (Puchtler and Meloan, 1978; Prins et al., 2014). An alternative method for quantifying calcification is cetylpyridinium chloride extraction. This method is less labor intensive, but less sensitive than Alizarin Red (Gregory et al., 2004). Another method to quantify calcium in cell cultures is the colorimetric method. This method needs solubilization of calcium deposits with HCl. The calcium content of HCl supernatants is then determined colorimetrically using commerical kits. This technique can also be used for tissue biopsies (Jono et al., 2000; Poggio et al., 2014; Hortells et al., 2015; Gayrard et al., 2020). In addition to that, many other calcium deposits detection methods have been developed including fluorescent and peptide−based dyes (Lee et al., 2012; Macri-Pellizzeri et al., 2018; Sim et al., 2018).
3.2 Ex vivo
3.2.1 Microscopy
Standard light microscopy today has a limited place in the analysis of mineral content. However, some information may be obtained with polarized light microscopy. For instance, ectopic deposits and amorphous masses may be characterized as containing apatite (Cottignoli et al., 2015a). More detailed information about mineral content can be detected by confocal microscopy collecting Raman spectra. This has been used in a few studies attempting to characterize the mineral content of calcified valves, sometimes in combination with infrared spectra (Mangialardo et al., 2012) or powder X-ray diffraction (Gourgas et al., 2020). Raman spectra can assess the crystallinity of mineral deposits, and peaks in the spectra suggest that the main mineral in calcified valves is carbonated hydroxyapatite (Gourgas et al., 2020). Unfortunately, however, there are severe limitations as to how much qualitative and quantitative information can be obtained regarding mineral content and composition of calcified aortic valves using techniques based on light microscopy.
3.2.2 Electron Microscopy
Both scanning and transmission electron microscopy have been used in several studies to characterize the biomineralization of calcified aortic valves and in particular their morphology (Mangialardo et al., 2012; Danilchenko et al., 2013; Cottignoli et al., 2015a; Cottignoli et al., 2015b; Gourgas et al., 2020). These techniques also show disturbances in the organic parts of the leaflets and the extracellular matrix such as disorganized bundles of collagen fibers. It is also described “the presence of biological niches within the calcified extracellular matrix, small, unfilled cavities inside rock that may be formed through a variety of processes” (Cottignoli et al., 2015b). These techniques also show details of different shapes of the crystalline structure: semispherical, laminar crystals, and spherical particles that make the calcified masses. The masses are described as bioapatite and also form needle or rod like crystals (Cottignoli et al., 2015b). Electron microscopy is the method with the highest resolution, but it is very labor-intensive, making its routine application difficult. However, additional methods are necessary for qualitative studies of calcification and crystals.
3.2.3 Micro-Computed Tomography (Micro-CT)
A novel method to describe the morphology and density of calcification and minerals in explanted aortic valves is micro-CT (Orzechowska et al., 2014). Micro-CT has a resolution of one micron; it is suitable for studying porosity, bone thickness, density, particle size, fiber orientation, etc. The level of x−ray signal attenuation is proportional to the material density and thickness. This could be interpreted as different levels of calcification. Soft tissue presents with very low attenuation calcifications, while bone matrix causes high attenuation, which creates high contrast images. Using micro-CT it is possible to assess the amount of calcified tissue in relation to the total volume of valve leaflet or the whole valve. In a study of explanted aortic valves, micro-CT showed a strong correlation between the amount of calcification and the severity of aortic stenosis (Chitsaz et al., 2012). Another study using micro-CT identified aortic valve deposits as B-type carbonate-containing hydroxyapatite (Orzechowska et al., 2014). In general, however, this technique does not give detailed information on the mineral composition, rather information on material density. Thus, it may give ratios between soft tissue and more calcified (harder) tissue.
3.2.4 Inductively Coupled Plasma Optical Emission Spectrometry and Inductively Coupled Plasma Mass Spectrometry (ICP-OES and ICP-MS)
The information obtained from this technique (chemical analysis) is not comparable to the others, as the approaches described above are able to study the crystal morphology, particle size, etc. However, ICP-OES and ICP-MS are techniques used for elemental analysis concentration (Hanć et al., 2011). There are some drawbacks, such as, it is a destructive technique: you have to digest the samples before analysis.
These techniques are superior for quantifying calcium (see 4.2.2.) and a broad series of trace elements (Heitkemper et al., 1994; Baralkiewicz et al., 2007; Sneddon and Vincent, 2008; Kreitals and Watling, 2014; Paraskova et al., 2015). In particular, these techniques have been quite extensively used for industrial purposes with ramifications for biology and forensic medicine (Carpenter, 1985). In veterinary medicine this technique has been used, to measure 14 trace elements from bovine liver biopsies (Braselton et al., 1997). Recently, there has been a shift towards the utilization of ICP-MS which offers a low detection limit combined with high sample throughput. ICP-MS also offers analysis of at least 25 trace elements in a biological sample. With a few exceptions, the lower detection limit of trace element and minerals is 1 nmol/L or less (Wilschefski and Baxter, 2019).
3.2.5 Miscellaneous, Minerals and Crystals of Calcified Aortic Valves
Among other methods used for characterization of biomineralogy and chemical composition are X-ray microanalysis coupled with energy-dispersive X-ray (Danilchenko et al., 2013) and direct chemical analysis, X-ray diffraction and Fourier transform infrared (Prieto et al., 2011). However, these techniques are usually used as adjunctive methods and in combinations with other techniques. Until quite recently, there was little knowledge regarding the analysis of the exact composition of calcified aortic valves. However, modern techniques have taught us more about the composition, structure, and formation of calcified valves. Mineralogical analyses of calcified valves to gain information about crystallization may be important and possibly an underestimated part of understanding the calcification process.
3.3 Imaging in vivo
Reliable imaging of the aortic valve has become increasingly important in recent years in parallel with the increase of catheter-based valve replacements (Bettinger et al., 2017; Francone et al., 2020; Mittal and Marcus, 2021). Usually a multimodality approach is recommended for evaluation of calcification, the characteristics of the valve itself and the aortic root. There are excellent reviews discussing imaging far beyond the scope of this overview (Salemi and Worku, 2017; Pawade et al., 2019; Francone et al., 2020; Ternacle and Clavel, 2020; Tzolos et al., 2020; Fletcher et al., 2021).
3.3.1 Echocardiography
Echocardiography is the standard clinical basis of all heart valve evaluations. It is safe, not expensive, widely available, and non-invasive. The key assessment criteria of aortic stenosis are combination of aortic valve area, mean gradient across the valve, and peak flow velocity. Details of the method including its limitations are beyond the scope of this review (Chong et al., 2019). Important to note is that echocardiography cannot quantify calcium. However, echocardiography is the standard technique to evaluate valve function, degeneration, leaflet stiffness due to fibrosis, and calcification of the aortic valve with the development of aortic stenosis. Transesophageal echocardiography provides better imaging than transthoracic, in particular to differentiate between tri- and bicuspid aortic valves (Yousry et al., 2012; Yousry et al., 2015).
3.3.2 Computed Tomography (CT)
CT is the method to choose to evaluate and quantify aortic valve calcification as calcium score measured by Agatston score “which accounts for both the density and volume of CT-measured calcium and correlates closely with the weight of calcium in explanted aortic valves” (Kang et al., 2010). Aortic valve calcium score also correlates well with calcific aortic valve disease progression and prognosis (Messika-Zeitoun et al., 2007; Nguyen et al., 2015) and is closely associated with severity of aortic stenosis measure by echocardiography (Cowell et al., 2003; Cueff et al., 2011; Tastet et al., 2017). For exact evaluation of the role of assessment of aortic valve calcification by CT, it is necessary to be aware of a series of pitfalls as described by Pawade et al. (Pawade et al., 2019). This is particularly important in younger patients (<51 years) with bicuspid aortic valves where Shen et al. found no correlation between mean gradient across the aortic valve and aortic valve calcium density (Shen et al., 2017). Several studies have also shown that women have lower calcification loads than men for the same aortic stenosis severity (Aggarwal et al., 2013; Gourgas et al., 2020). Standard CT cannot detect the early stages with micro-calcification, it can only visualize confluent areas of macro-calcification (Bailey et al., 2016). However, recently contrast-enhanced CT has been shown to be able to assess not only calcium, but also non-calcific (fibrotic) aortic valve composition, allowing assessment of early CAVD (Cartlidge et al., 2021; Grodecki et al., 2021).
3.3.3 Magnetic Resonance Imaging (MRI)
MRI is not routinely used and is far less widespread than CT. It is rather a supplementary imaging technique. However, it has an increasing role in the planning of endovascular aortic valve procedures (Mittal and Marcus, 2021). In the recent consensus document by the European Society of Cardiovascular Radiology, it is explicitly stated that MRI have many potential advantages in such situations (Francone et al., 2020). This includes all necessary measurements of the valve and the aortic root as well as evaluation of ventricular function and the aorta. Furthermore, MRI has some distinct advantages to avoid the use of contrast in cases with severe kidney failure. Unfortunately, it does not provide reliable calcium score.
3.3.4 Positron Emission Tomography (PET)
PET uses radioactive isotopes, which concentrate in regions with high metabolic activity, thus being able to detect changes on the molecular and cellular level before anatomic changes occur. 18F-sodiumfluoride accumulation was found to correlate with calcification in the aortic valve (Dweck et al., 2014) and it is able to detect micro-calcification in the vasculature (Vancheri et al., 2019) and in valves (Hutcheson et al., 2014). 18F-sodiumfluoride binds to hydroxyapatite on calcified nodules and is quantitatively shown to be associated with faster progression of CAVD (Dweck et al., 2014; Jenkins et al., 2015). Furthermore, accumulation of 18F-sodiumfluoride is also associated with bio-prosthetic aortic valve degeneration (Cartlidge et al., 2019). So far PET is primarily a research tool and less used in clinical investigations, partly due to costs and lower availability in clinical practice. However, PET may be helpful to develop our understanding of aortic stenosis, both its molecular background as well as its development, risk stratification, and progression in patients (Pai et al., 2006; Rojulpote et al., 2020). In particular, it may be a powerful tool when it localizes the process in 3D, in combination with CT and/or MRI (Tzolos et al., 2020).
4 Collection of Human Aortic Valves for Cell Isolation
For studies in cell cultures, cells from human aortic valves are preferred in order to eliminate species differences. Exceptions are relevant when in vivo animal experiments are performed or when using transgenic models. Calcified human aortic valves are fairly easy to obtain if the laboratory is situated in the proximity of a cardiac surgery unit. Calcified aortic valves can be harvested from patients with aortic valve stenosis undergoing aortic valve replacement. Cells from a valve can be freshly isolated and usually retain moderate to high degree of viability. The degree of cell viability/quantity/proliferation for each donor is individual. It depends among others on the level of the valve calcification where severe calcified valves result in the inferior cell isolation yield. Of note: patients with rheumatic aortic valve stenosis, a late inflammatory complication of group A Streptococcal pharyngitis, represent a totally different disease (Wallby et al., 2013) which is not included or discussed here.
Healthy valves are less readily available. There are several potential sources of non-calcified human aortic valves (Rutkovskiy et al., 2017). The ideal one is from donor hearts that were considered unsuitable for transplantation. Other possibilities include valves from explanted hearts of heart transplant recipients.
For isolation of cells from explanted aortic valves, timing is critical, in particular for the isolation of VEC. By placing the leaflets in saline immediately after excision and keeping at +4 C enhances viability, opening a window of several hours for VEC isolation. VIC are less sensitive to time before isolation, however, we recommend isolation of VIC within the first 24 h. According to our experience with aortic valve cells from autopsy material acceptable viability can be expected for up to 24 h post mortem which is in accordance of what has been reported earlier (Gall et al., 1998). Gender may also be important: valves from men have more advanced calcification at the same age as women, whereas stenotic valves from women have increased levels of fibrosis compared to men (Aggarwal et al., 2013). It is also important to avoid mixing bicuspid (BAV) and tricuspid (TAV) aortic valves since there are differences in the molecular and cellular mechanisms that underlie calcification of BAV and TAV (Kostina et al., 2018).
5 Endothelial and Interstitial Cells From Human Aortic Valves
Handling of cells and cell cultures are presented in more details than other techniques here because it is probably the most widely spread and concise method to study the basic cellular and molecular events of aortic valve calcification. Additionally, cell culture models are also used for initial screening of potential inhibitory drugs (Dutta et al., 2021; Natorska et al., 2021; Parra-Izquierdo et al., 2021; Wang et al., 2021).
5.1 Isolation and Culture
The most widely used and reproducible techniques for isolation of VEC and VIC have been derived from methods that originally employed porcine material (Gould and Butcher, 2010). The quality of plastic on which the cell culture is seeded is crucial for good VIC growth. Normal practice is to use standard cell growth medium containing DMEM supplemented with 10–15% fetal bovine serum (FBS) and antibiotics. The day after isolation, the VICs are usually visible, and they present a fibroblast-like morphology (Figure 1). During cultivation, the media is changed twice a week until a confluence of 70–80% is attained (usually within 1 week, see Figure 1). At this point the VIC are harvested using trypsin/EDTA and seeded at a high density (we recommend to not exceed ratio of 1:2), which is a crucial factor for survival of VICs isolated from calcified valves. It is recommended to passage VICs after achieving density around 90–95%. VECs are usually isolated by swabbing cells from the surface of the leaflet or via vortexing collagenase−treated leaflets. On the second day in culture, the rosette-like colonies of VECs should form. VECs are grown until a confluence of 70–80% is attained (usually within 1 week, Figure 1). VEC then passaged at a ratio of 1:3. Primary cells in culture are known to change particular properties with each passage, thus the number of passages is important to report (Yperman et al., 2004; Goto et al., 2019). An important precaution with regards to the culture of primary VECs and VICs is to ensure they are mycoplasma-free, therefore all cultures should be kept in a quarantine area until a mycoplasma test has been conducted.
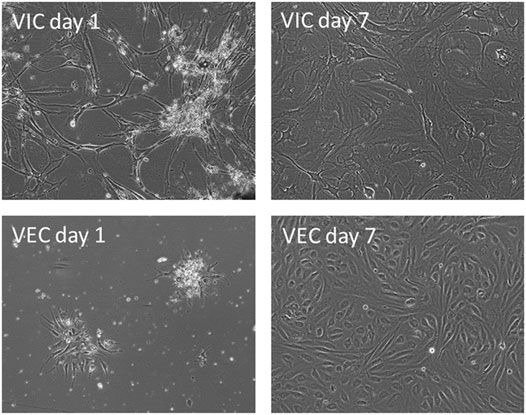
FIGURE 1. Interstitial (VIC) and endothelial (VEC) cells isolated from human aortic valves shown on first and seventh days after isolation. Phase contrast microscopy.
5.2 Purification and Characterization
Isolated VEC from calcified valves will invariably be contaminated with VICs, which will threaten to outgrow them over time. Therefore, an enrichment step is highly recommended, for instance, magnetic-activated cell sorting 1 week after initial isolation (Gould and Butcher, 2010). Human VEC can be enriched using the surface markers PECAM-1/CD31 (Platelet endothelial cell adhesion molecule-1), providing a discriminatory marker for enrichment. The main steps or the cell isolation procedure are summarized in Figure 2. Following enrichment, it is recommended to assess the purity of the respective cell populations using markers that allow the delineation of each cell type. The VEC population may be assessed using flow cytometry against the endothelial marker CD31 (Gould and Butcher, 2010) and/or von Willebrand factor (vWF) (Gould and Butcher, 2010) and VE-cadherin (Farrar and Butcher, 2014) (Figure 3). We routinely observe high purity, with 95% of the population being CD31 positive (Figure 3). Flow cytometry data are well in line with the immunocytochemistry staining. The cell population should not exhibit alpha-smooth muscle actin expression (αSMA) in immunocytochemistry assessment (Figure 3). However, using flow cytometry we observed that approximately 7.5% cells are positive for αSMA. This can suggest either VIC culures are contaminated or the presence of cells bearing both markers, such as VEC undergoing mesenchymal transition (Figure 4).
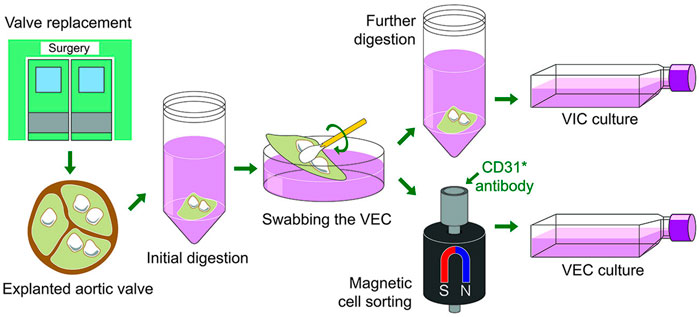
FIGURE 2. A schematic overview of the main steps of the calcified human aortic valves collection, digestion and valvular interstitial and endothelial cells isolation.
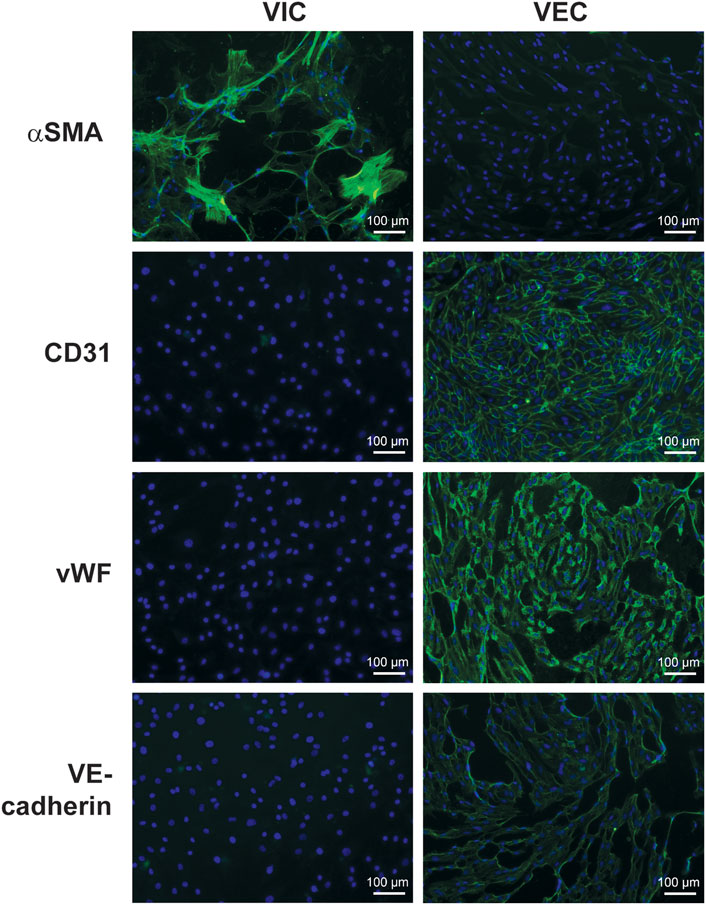
FIGURE 3. Immunofluorescence staining for fibroblastic and endothelial markers in valve interstitial (VIC) and endothelial cells (VEC). Cells were isolated from human aortic valves with calcification (n = 3) and separated by magnetic-activated cell sorting. The pictures show expression of alpha-smooth muscle actin (αSMA) in VIC and cluster of differentiation 31 (CD31), vascular endothelial cadherin (VE-Cadherin), and von Willebrand factor (vWF) in VEC. The nuclei were stained with Hoechst 3342 (blue).
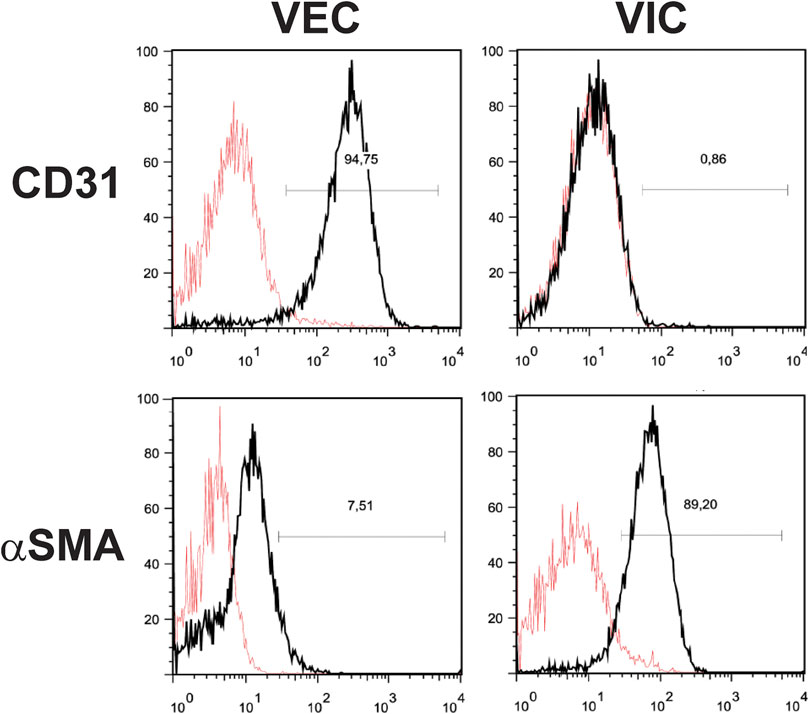
FIGURE 4. Fluorescence-activated cell sorting (FACS) analysis of valve endothelial (VEC) and interstitial cells (VIC). Cells were isolated from aortic valves with calcification (n = 3). A representative image shows expression of the typical fibroblastic marker protein alpha-smooth muscle actin (αSMA) in VIC, but not in VEC and expression of typical endothelial cell marker CD31 in VEC, but not in VIC.
αSMA is common marker used to separate human VIC population from VECs (Gould and Butcher, 2010) and is useful to assess the purity of the VIC population. The expression of αSMA is low in healthy human valves, and relatively high in calcified valves (Olsson et al., 1994). Higher expression of αSMA is associated with myofibroblastic differentiation, which is one of the hallmarks of CAVD. It is suggested that cultured VICs can spontaneously differentiate into myofibroblast-like cells as they increase αSMA expression with time (Pho et al., 2008; Monzack and Masters, 2011; Latif et al., 2015a; Porras et al., 2017). The spontaneous myofibroblast differentiation is believed to be a result of the novel physical environment, which influences cells via mechanoreceptors. It is well established that rigid substrates promote myofibroblast differentiation in fibroblasts. It may be related to the physical properties of the substrate (culture plastic) as stiffer substrates are known to promote myofibroblast phenotype (Yip et al., 2009). Some authors propose culturing VIC in “fibroblast medium” to potentially reduce expression of myofibroblastic markers such as αSMA, transgelin, and extra domain-A fibronectin (Latif et al., 2015a; Porras et al., 2017). Alternatively, one can try to use cells with low passage numbers to be as close to the original phenotype as possible.
Using flow cytometry, we observed that approximately 90% of VIC from calcified aortic valves were positive for αSMA (Figure 4). The presence of αSMA was further verified by immunostaining (Figure 3). Possible contamination with VEC in the VIC population was assessed by immunostaining for vWF, CD31, and VE-cadherin (Figure 3). This can be further validated using flow cytometry for CD31 (Figure 4). There has been efforts to find additional markers that are highly expressed in human VICs, for example, vimentin (Latif et al., 2007; Latif et al., 2015a), prolyl-4-hydroxilase (Taylor et al., 2000; Osman et al., 2006a) and markers of bone-morrow mesenchymal stem cells: fibroblast surface antigen (CD90) (Latif et al., 2007; Latif et al., 2015a) and CD44 (Latif et al., 2007). The relevance of these markers for separation of human VICs from VEC have not been confirmed. We observed expression of vimentin and CD90 in both human VEC and VIC populations and do not recommend the use of these markers for purification of human VIC. It has also been suggested that calponin can be utilized as a VIC marker, especially associated with progression of CAVD (Plazyo et al., 2018; Bogdanova et al., 2019).
5.3 2D or 3D Culture Models of Human Aortic Valve Cells
5.3.1 Interstitial Cells
2D models of culturing VIC and VEC are most common, although 3D cultures are used as well. 3D cultures have been suggested to have some advantages providing a more physiologically relevant model for the cells because VIC are influenced by their microenvironment (Hjortnaes et al., 2015; Ravi et al., 2015; Hjortnaes et al., 2016; van der Valk et al., 2018; Bracco Gartner et al., 2019). This is highlighted by Hjortnaes and co-workers when cell are cultured in 3D hybrid hydrogels composed of hyaluronic acid and gelatin (Hanć et al., 2011). They state the following: “The elastic modulus of 3D hydrogels used in our study (∼20kPA) corresponds to the perceived modulus of the fibrosa as measured by micropipette aspiration up to 21 kPA” Furthermore, “We previously showed that the 3D hydrogel platform maintains a quiescent VIC phenotype identified in healthy heart valves, thus providing a platform to study phenotypic changes associated with CAVD” as well as “The 3D approach presented in this work can maintain healthy quiescent VIC population and thus can model the entire cellular process”. However, there are several limitations of the 3D model. The hydrogel platform is static and the composition of their hydrogel is different when compared to the in vivo extracellular matrix. The 3D cultures are more difficult to subject to mechanical stimulation and the diffusion through the gel should be taken into account when performing chemical stimulations. Although 3D platform may in some ways be attractive, 2D cultures for VIC are still leading in the field due to simplicity and standardization. For general use, the superiority of 3D cultures use can so far be discussed.
5.3.2 Co-Cultures of Valve Endothelial and Interstitial Cell
Recent studies of the molecular and cellular mechanisms of CAVD have emphasized the importance of VEC-VIC interactions. Porcine VIC have reduced expression of the myofibroblastic gene αSMA when co-cultured with VECs (Butcher and Nerem, 2006), implying that VECs are involved in the regulation and maintenance of the VIC phenotype. This is also corroborated by several studies demonstrating that VECs inhibited myofibroblastic or osteogenic differentiation of porcine VIC in co-culture (Kennedy et al., 2009; Richards et al., 2013; Gould et al., 2014), suggesting an important role of VEC-VIC interaction for cellular valve homeostasis. Dysfunction or denudation of VECs, have also been implicated as an initiator of VIC transformation leading to calcification (Leopold, 2012; Gomel et al., 2018; Hulin et al., 2018). It was recently suggested that VEC isolated from different sides of the valve have a different effect on the VIC calcification through cadherin-11 (Johnson and Merryman, 2021).
Static 3D co-cultures of human VEC and VIC are suitable for studies of cell type interactions. We seeded VIC pre-mixed with collagen and when the gels were cast, VEC were seeded on top. The endpoints included gene expression changes, as well as the contraction of collagen gels by the VIC, being a function of their myofibroblast differentiation. More details on this topic are provided in Section 5.4.3. We also have positive experience with 2D co-cultures where the VIC are seeded at 90% density and then VEC are seeded directly on top of the VIC in the amount that is sufficient to achieve the same density in the top monolayer.
An interesting version of 3D co-cultures was recently reported by van der Valk et al., where they engineered a 3D-bioprinted model of a human aortic valve (van der Valk et al., 2018). In this study the aortic leaflet tissue was mechanically tested after micro-dissection of different layers. Leaflets were then constructed by bioprinting of 3D hydrogels with encapsulated human VIC. The hydrogels had been tuned to duplicate specific mechanical characteristics of the leaflets. It is too early to conclude how helpful this model is due to limited usage data.
5.4 Osteogenic Differentiation of Valve Interstitial Cells
Osteoblast- and osteoclast-like cells have been identified histologically in human calcified aortic valves (Mohler et al., 2001), but not in healthy aortic valves. Many markers that are attributed to osteoblasts have been found in valves of patients with CAVD and the majority of these markers are also expressed by VIC differentiated into osteoblast-like cells in vitro (Osman et al., 2007; Galeone et al., 2013; Zhang et al., 2014). The most common formulation of osteogenic medium that triggers calcification and expression of osteogenic markers in human VICs include beta-glycerophosphate, dexamethasone and ascorbic acid, which can be substituted with vitamin D (Osman et al., 2006a; Osman et al., 2006b; Osman et al., 2007; Babu et al., 2008; Galeone et al., 2013). Beta-glycerophosphate is the most potent component of most osteogenic media, as it donates a phosphate group to calcium ions to form calcium phosphate crystals, the main ingredient in mineral bone matrix. Beta-glycerophosphate induces transdifferentiation into osteoblast-like cells, thus increasing osteoblast activity and subsequent calcification (Babu et al., 2008). Dexamethasone stimulates both osteogenic and adipogenic differentiation depending on its concentration. The typical concentration that induces osteogenic differentiation is 0.1 µM, whereas higher concentrations are used to induce adipogenic differentiation (Zhao et al., 2018). Ascorbic acid is an additional cofactor that facilitates osteogenic differentiation by increasing collagen I synthesis (Ishikawa et al., 2004) and secretion (Langenbach and Handschel, 2013). The length of treatment in the majority of studies is 21 days (Osman et al., 2006a; Osman et al., 2006b; Osman et al., 2007; Babu et al., 2008). Basic osteogenic medium can be supplemented with BMP2, which has been demonstrated to be important for valve calcification (Zhang et al., 2014). It has been shown that treatment of human VIC with ATP (Osman et al., 2006a); BMP2 (Bone morphogenetic protein 2), BMP4, BMP7, TGFβ-1 or TGFβ-3 (Osman et al., 2006b) for 21 days can activate expression of alkaline phosphatase (ALP), which is a marker of late-stage osteoblastic differentiation. In our hands, the strongest effect was obtained when VICs were stimulated for 21 days with a basic osteogenic medium containing standard cell growth medium (DMEM, 10% FBS) supplemented with 10 mM beta-glycerophosphate, 0.1 µM dexamethasone and 50 µM ascorbic acid. This regimen induced reproducible and robust calcification (Bogdanova et al., 2019).
Another popular formulation of medium that promotes osteogenic differentiation of human VIC (termed “pro-calcifying medium”) include DMEM supplemented with 5% FBS, 2 mM NaH2PO4 and 50 μg/ml ascorbic acid (Bouchareb et al., 2015; Rogers et al., 2017; Schlotter et al., 2018). Gotto et al. (Goto et al., 2019) showed that the calcification potential of human VIC decreased with passage number in osteogenic medium, but not in pro-calcifying medium. Passage-dependent calcification of VIC cultured in osteogenic medium is regulated by abundance of tissue non-specific alkaline phosphatase (TNAP), an enzyme that hydrolyzes β-glycerophosphate to inorganic phosphate, which can be incorporated into calcium phosphate crystals promoting calcification. TNAP also plays a key role in mineralization by degrading inorganic pyrophosphate (calcification inhibitor) and providing free inorganic phosphate to induce calcification (Hui and Tenenbaum, 1998). Pro-calcifying medium contains inorganic phosphate and therefore does not require TNAP for the calcification process in VIC (Goto et al., 2019). Proteomic analysis of human VICs revealed induced expression of fibrosis- and calcification-related proteins under treatment with both osteogenic and pro-calcifying medium compared to control cells without stimulation, some of these proteins were shared between the two treatment groups (Schlotter et al., 2018). Further studies are required to gain a better understanding of which of the culture media best reflect the natural conditions of aortic valve calcification.
5.4.1 Myofibroblastic Differentiation of Valve Interstitial Cells
Myofibroblasts are defined as fibroblasts that have some properties of smooth muscle cells and are characterized by the presence of stress fibers composed mainly of αSMA, providing the ability to contract the extracellular matrix (Tomasek et al., 2002). The myofibroblast-like cells play an important role in extracellular matrix remodeling in the pathogenesis of aortic valve calcification (Liu et al., 2007). High expression of αSMA is a well described marker of myofibroblasts (Tomasek et al., 2002) which is increased in calcified aortic valves (Olsson et al., 1994). A recent study has proposed that MAPK/ERK as a potential pathway involved in myofibroblast calcification in CAVD (Gonzalez Rodriguez et al., 2021). In addition to αSMA, Calponin and SM22 (Transgelin) are established markers to identify myofibroblast-like cells in human (Latif et al., 2015b; Porras et al., 2017; Kostina et al., 2018).
TGFβ-1 (Transforming growth factor beta 1) is highly expressed in diseased aortic valve leaflets and has been the most extensively studied cytokine in relation to VIC activation and aortic valve calcification (Jian et al., 2003; Walker et al., 2004; Merryman et al., 2007; Hutcheson et al., 2012). As stated above, the majority of animal VICs are positive for αSMA, and its expression varies with the degree of myofibroblastic differentiation. In calcified valves this phenotype is usually widespread, but even then TGFβ-1 added to cultures can further promote it and thereby enhance αSMA expression (Walker et al., 2004; Kennedy et al., 2009; Monzack et al., 2009; Chen et al., 2011; Quinlan and Billiar, 2012). There appears to be species differences with respect to timing of myofibroblastic differentiation in porcine (Cushing et al., 2008) and ovine (Jian et al., 2002; Walker et al., 2004) VIC. After treatment with TGFβ-1 in low-serum medium, αSMA was detected after 24 h in ovine VIC (Gwanmesia et al., 2010), while αSMA was not detected until day 5 in porcine VIC (Gu and Masters, 2010). In our experience VIC isolated from both healthy and calcified human aortic valves have increased expression of αSMA and Calponin, analyzed by flow cytometry, after 4 days of stimulation with a myofibroblastic medium (DMEM, 1% FBS and 5 ng/ml TGFβ-1). Furthermore, stimulated cells from healthy valves are characterized by higher expression of these myofibroblastic markers indicating more prominent myofibroblastic differentiation in comparison with cells from calcified valves. In conclusion, a dynamic increase in αSMA and Calponin expression is a reliable myofibroblastic differentiation marker for human VIC isolated from healthy and calcified aortic valves.
5.4.2 Role of Extracellular Matrix in Myofibroblastic Differentiation
The extracellular matrix plays a key role in the regulation of VIC phenotype and function, including the processes of differentiation (Gwanmesia et al., 2010). Moreover, it is speculated that TGFβ-1 may bind to components of the extracellular matrix and this interaction may be essential for its signaling (Chen and Simmons, 2011; Jenkins, 2008; Wipff and Hinz, 2008). Disruption of the extracellular matrix in valve leaflets in turn alters TGFβ-1 signaling in VIC, leading to remodeling and valve disease (Chen and Simmons, 2011; Jenkins, 2008; Wipff and Hinz, 2008). In vitro it has been demonstrated that different coatings on conventional tissue culture plates influence myofibroblastic differentiation in different ways (Chen and Simmons, 2011; Hinton and Yutzey, 2011). Collagen and laminin coatings increase both the calcification process and induction of αSMA in ovine VIC, whereas fibronectin has an opposite effect (Gwanmesia et al., 2010). Laminin, heparin, and fibrin, but not collagen or fibronectin promote nodule formation in porcine VIC (Rodriguez and Masters, 2009). Another factor that influences the differentiation of VIC into myofibroblasts is the rigidity of the matrix (mechanical properties). By varying the concentration of collagen in a 3-dimensional model, very different effects were observed: compliant matrices contribute to osteogenic differentiation and calcification, whereas stiff matrices promote myofibroblastic differentiation and calcification through apoptosis (Yip et al., 2009; Quinlan and Billiar, 2012; Wyss et al., 2012). In addition, the effect of TGFβ-1 on αSMA expression is proportional to the matrix stiffness (Chen et al., 2011). In 2D cultures, stiff substrates such as tissue culture plastic may be sufficient to promote VIC differentiation to myofibroblasts (Kennedy et al., 2009; Benton et al., 2008). A summary of coatings employed for myofibroblastic differentiation of animal VIC and their effect is shown in Table 1. Laminin and collagen are the most commonly used coating surfaces for culture of myofibroblasts (Monzack et al., 2009; Rodriguez and Masters, 2009; Yip et al., 2009; Gwanmesia et al., 2010; Chen et al., 2011; Quinlan and Billiar, 2012; Wyss et al., 2012). Figure 5 shows the comparison of αSMA expression in cells cultured either on laminin or collagen coating after stimulation with myofibroblastic medium.
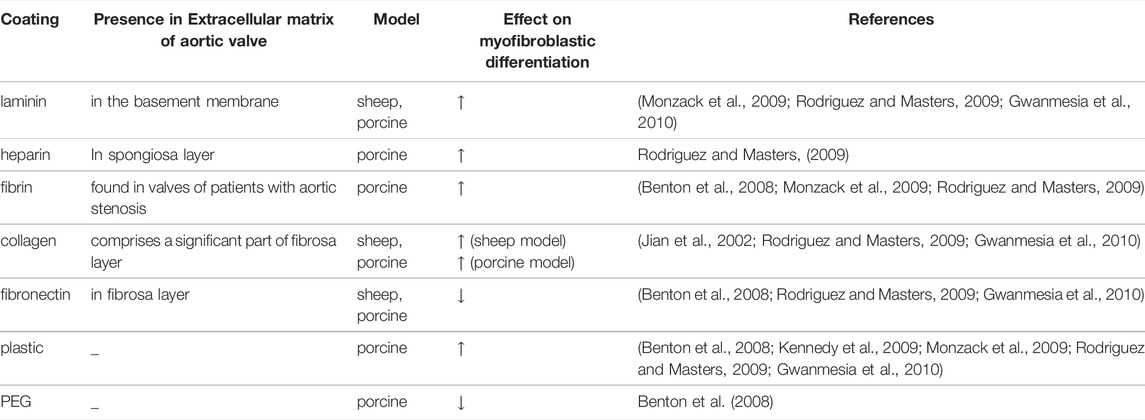
TABLE 1. The effect of different coatings on myofibroblastic differentiation of cultured valve interstitial cells from different species.
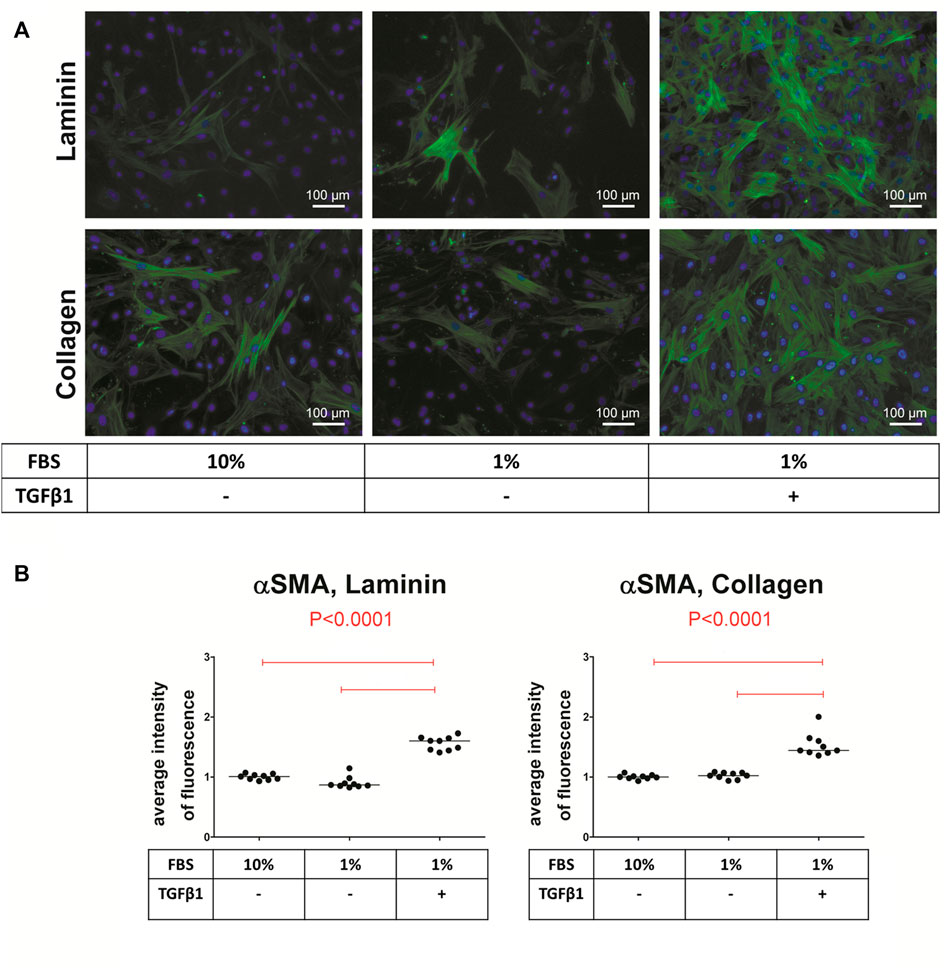
FIGURE 5. Immunofluorescence staining of valve interstitial cells (VIC) for alpha-smooth muscle actin (αSMA). (A) VIC were isolated from aortic valves with calcification (n = 9) and cultured for 14 days on either laminin or collagen, with 10% FBS without TGFβ-1, with 1% FBS without TGFβ-1 or with TGFβ-1. αSMA (green), cell nuclei (Hoechst 3342/blue). (B) Quantification of αSMA fluorescence, shown as scatter plot with median. Statistical differences were tested using ANOVA followed by Tukey test. Overall p-values from ANOVA analysis are shown in red.
5.4.3 Myofibroblastic Contractility of Valve Interstitial Cells in 3D Cultures
Actin-myosin cytoskeleton of myofibroblasts is connected with components of extracellular matrix via cellular transmembrane receptors, the integrins, allowing cells to contract the surrounding extracellular matrix (Parizi et al., 2000). In order to provide relevant models that reflect the in vivo situation in humans with contraction inside the leaflets, VIC isolated from calcified valves can be incorporated into a 3D cell culture system, based on collagen gel (Bond et al., 2007). This allows measuring the contractility of VIC-derived myofibroblasts, which in turn demonstrates their functional attributes (Butcher and Nerem, 2004; Cushing et al., 2008). αSMA expression induced by TGF-β1 stimulation correlates with gel contraction confirming the contractile phenotype of VIC (Hinz et al., 2001). Blocking αSMA polymerization with cytochalasin D attenuates TGF-β1–induced contraction (Walker et al., 2004). These results confirm that VIC contract collagen gel due to their differentiation into myofibroblast-like cells.
The collagen gel constructs, in which the VIC are encapsulated (Cushing et al., 2008), may be created with 2 mg/ml collagen I, 5x DMEM (10% FBS 0.1M NaOH) before VIC are added. After polymerization the gels can be gently detached from the wells (floating model), otherwise the gels are kept attached to the well (stressed model). Whereas the floating model is believed to mimic normal connective tissue, stressed model mimics wound healing situation where cells are under mechanical load transferred from extracellular matrix. To stimulate the human VIC to differentiate into myofibroblasts, the gels containing the cells are treated with DMEM supplemented with 1% FBS and 5 ng/ml TGF-β1. Imaging of floating collagen gels are acquired every 24 h (Figure 6A). Collagen gel size is measured and percent contraction is calculated as the change in area from the initial area at time zero. Using this model, we have shown that collagen cell constructs from healthy valves contracted more strongly than if cells were from calcified valves after stimulation with TGF-β1, suggesting higher potential to differentiate into myofibroblasts (Fletcher et al., 2021). A schematic overview of the gel contraction and stressed model formation is shown in Figure 7. Although treatment with 10% FBS does not change expression of myofibroblastic marker αSMA in human VIC compared to treatment with 1% FBS, we noticed that treatments with 10% FBS or 1% FBS without TGFβ1 have different effects on gel contractility of human VIC (Bogdanova et al., 2018). A possible explanation is that serum contains factor(s) that can promote myofibroblast contraction (Parizi et al., 2000; Latif et al., 2015a; Porras et al., 2017). Treatment of floating collagen gel constructs with high-serum (10% FBS) leads to significantly greater collagen gel contractility compared to low-serum (1% FBS) and contracted collagen gel at the same level as stimulation by low-serum (1% FBS) together with TGFβ1 (Figure 6B). In conclusion, collagen gel contraction is a relevant method to characterize functional attributes of human VIC. However, when results are interpreted, it is important to take into consideration factors discussed above that influences collagen gel contractility.
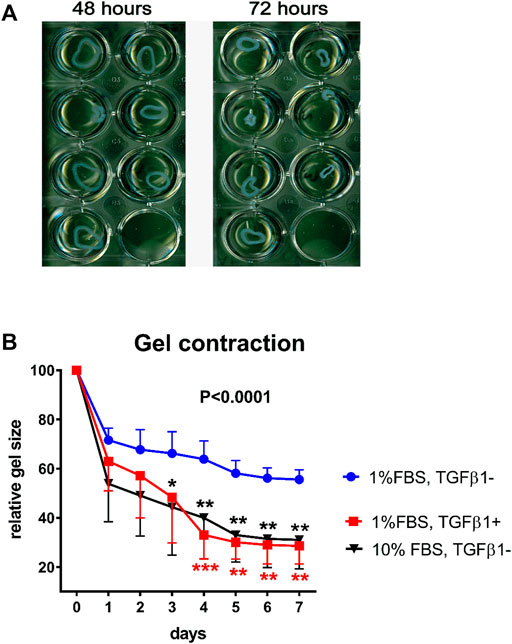
FIGURE 6. Valve interstitial cells in 3D collagen cultures. Contraction of collagen gel containing cultured interstitial cells from calcified aortic valves (n = 4) and treated with 1% FBS with or without TGF-β1 over a period of 7 days. Panel (A) shows how the cultures contract and become smaller. Panel (B) shows the collective data of gel contraction of VIC from healthy aortic valves (n = 4) under stimulation with low-serum (1% FBS) with or without TGF-β1 or with high-serum (10% FBS) without TGF-β1. Gel sizes on day 0 were considered as 100%. Data were analyzed by two-way ANOVA with repeated measures. Differences between treatments with 1% FBS with TGF-β1 (shown in red stars) or 10% without TGF-β1 (shown in black stars) compared to 1% FBS without TGFβ1 were determined with Sidak’s multiple comparison post-test, *indicates 0.01 < p ≤ 0.05, ** indicates 0.001 < p ≤ 0.01, *** indicates 0.0001 < p ≤ 0.001. Values are expressed as mean ± SD. Overall p-value from two-way ANOVA is shown in bold.
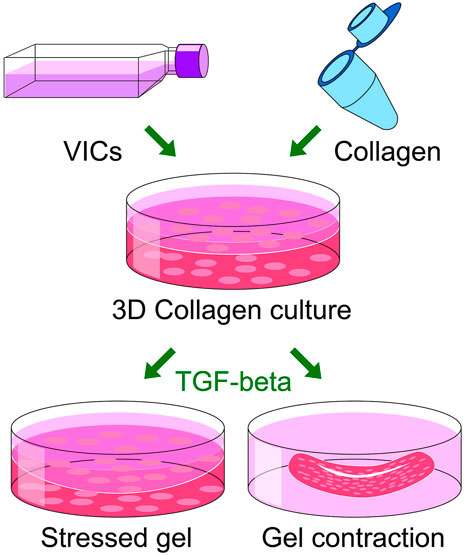
FIGURE 7. A schematic overview of two 3D cell culture system (stressed gel and gel contraction), based on collagen gel that are being used for measuring the contractility of VIC-derived myofibroblasts.
5.5 Genetic Modification of Valve Endothelial and Interstitial Cells
Both VEC and VIC can be genetically engineered providing tools for unraveling the underlying mechanisms of calcification. We have tested two main gene delivery approaches: 1. Transfection of siRNA using N-TER Nanoparticle delivery system, and 2. Transduction with lentivirus. For the siRNA transfection we assessed the ability of VIC to take up a FITC conjugated siRNA, providing a convenient way to monitor efficiency. Both 10% FBS and serum-free approaches can be used for N-TER Nanoparticle delivery into VIC. Serum free conditions in our hands provided the highest efficiency of transfection with minimal cell death (Figure 8A). VEC appeared to be more susceptible than VIC to lentiviral entry, with approximately 77% of cell being transduced as assessed by GFP expression, while VIC had a transduction efficiency of 47% in comparison to HEK293 (Figure 8B).
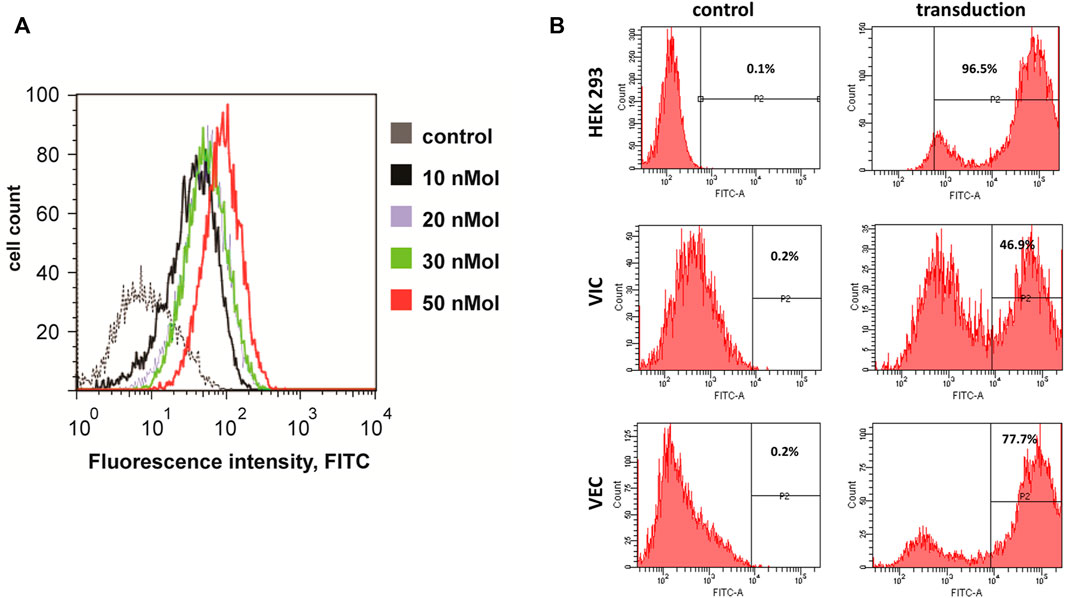
FIGURE 8. Genetic engineering of valve endothelial (VEC) and interstitial cells (VIC). (A) Representative example of fluorescence - activated cell sorting (FACS) analysis of VIC from a calcified aortic valve and transfected with siRNA to mRNA conjugated with fluorescein isothiocyanate (FITC). Transfection kit was used with different concentrations of nanoparticle formulation solution (NFS). Dotted line - negative control (transfected with scrambled siRNA), black line–transfected with 10 nM NFS, blue–20 nM NFS, green -30 nM NFS, red -50 nM NFS (86.5% of cells transfected with siRNA). (B) Representative example of fluorescence–activated cell sorting (FACS) analysis of human embryonic kidney cells 293 (HEK293), aortic valve interstitial and endothelial cells from a calcified aortic valve without transduction or transduced with lentiviral construct that encoded green fluorescent protein (GFP).
6 Animal Models
There is generally a lack of animal models that accurately reflect human aortic valve stenosis (Sider et al., 2011). However, animal models are needed to investigate any kind of cardiovascular and soft tissue calcification. In particular to evaluate the effects as well as toxicity of drugs that potentially can inhibit calcification.
6.1 Subcutaneous Implantation of Cusps
One extensively used model to study calcification, is implantation of cusp tissue–or other biological materials - in a subcutaneous pouch of rats or rabbits (Fishbein et al., 1982; Levy et al., 1983; Schoen et al., 1985; Mako and Vesely, 1997). This model has been used to evaluate how different preservation techniques influence calcification in cusps of bio-prosthetic heart valves, but also in other biological materials used for implantation, such as pericardial patches. Calcification develops in about 8 weeks when the material tested is explanted (Kennedy et al., 2009; Richards et al., 2013; Gould et al., 2014; Hulin et al., 2018). This model is something between an in vitro and in vivo model and it is easy to prepare. Although being an un-physiological model, it is suitable for studying inhibition of calcification.
6.2 Aortic Valve Leaflets in Culture
As a more complex model than cell culture, culturing aortic valve leaflets may be a good alternative. In the model hierarchy it brings the investigation one step up from the cell cultures. Unfortunately, this is a model where the use of human tissues is less feasible. Healthy human valves are difficult to obtain, and culturing calcified valves may cause problems of interpretations for analysis of calcification. One possibility is to use parts of explanted calcified valves without macroscopic calcification; another possibility would be to use autopsy material. However, the most common practice is to use porcine aortic valve leaflets, either as parts of leaflets or as whole leaflets in culture medium (Sauren et al., 1983; Xing et al., 2004; Konduri et al., 2005; Balachandran et al., 2006; Chester et al., 2008; El-Hamamsy et al., 2009). Most of these studies have focused on the mechanical, biological or contractile properties of valve leaflet tissue. The best results are achieved with pig leaflets which better reflect the human morphology than mice and rat leaflets (Hinton et al., 2008). Rodent valves are also smaller and provide less material for molecular analysis. Several studies induced calcification in pig aortic leaflets. In one, calcification was induced by cyclic stretch for 2 weeks combined with a high concentration of osteogenic medium (Balachandran et al., 2010). Including mechanical stress may add some similarities to the human situation. In another, Rathan et al. induced calcification in porcine aortic leaflets by adding phosphate plus inorganic pyrophosphatase for 8 days (Rathan et al., 2014). Chester et al. (2021) developed another model with whole leaflets where calcification is not induced by osteogenic media, but uses the combination of lipopolysaccharide and inorganic phosphate, to initiate and drive the calcification process by an inflammatory response. One of the advantages is the extensive histological investigation of the calcifying leaflets–both qualitative and quantitative (Chester et al., 2021).
6.2.1 A Novel Model of Calcification ex vivo in Whole Valve Leaflets
We have developed a reliable model of cultured whole leaflets from porcine valves (Zabirnyk et al., 2020). Shortly, after animal sacrifice in an authorized abattoir, the hearts are transported on ice to the laboratory where the aortic valve leaflets are dissected free. The whole leaflets are maintained in individual wells of low attachment cell culture plates to avoid cell migration and loss of leaflet integrity. In standard pro-osteogenic media the leaflets shrunk from a leaflet to a ball-like shape due to myofibroblast contraction and with negligible accumulation of calcium deposits. Using anti-myofibroblastic growth medium (low-glucose DMEM, 2% FBS, FGF2 (fibroblast growth factor 2) and insulin), pro-osteogenic stimulation caused strong accumulation of calcium. This formulation prevented leaflets from shrinking, probably by inhibiting myofibroblastic transition of VICs. A schematic overview of the above described culturing pig leaflets in two different growth medias is presented in Figure 9.
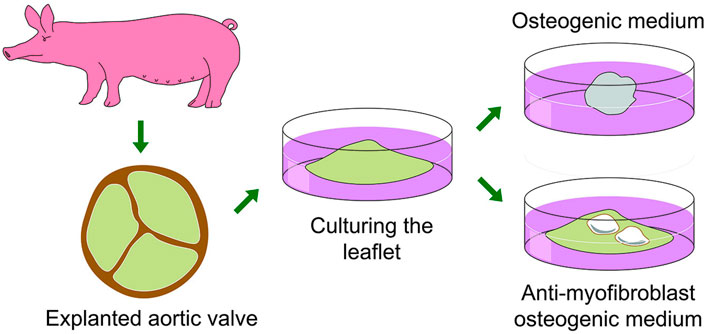
FIGURE 9. A schematic overview of the whole pig leaflet cultivation and calcification model with the effect of standard osteogenic and anti−myofibroblast media being used.
The validity of the ex vivo leaflet models is solely based on the amount of calcium accumulated in the valves. A limitation is that these leaflet models cannot be expected to closely mimic calcification in patients, a process slowly developing over several years. Histological characterization of the leaflets would be valuable. The in vitro leaflets cannot obtain the structure of calcified human valves that have ingrowth of vasculature and containing inflammatory cells and bioactive substances derived from the blood stream plus fibrosis. However, what determines the stiffness of a leaflet giving rise to aortic stenosis is fibrosis and the amount of calcification/calcium in the valve.
6.2.2 Measurements of Calcium in Leaflets
After the cultivation for 4 weeks with osteogenic differentiation, the amount of calcium accumulation is assessed. Alizarin Red staining is a good method in cell cultures, but it is not suitable as the multilayer tissue nonspecifically absorbs the dye. One way is to section the leaflet and semi-quantitatively assess the regions stained with Alizarin Red, however this method is rather inaccurate. After comparing several methods of quantifying calcium accumulation, the ICP-OES or ICP-MS appeared to be the most reliable and accurate method in whole leaflet models after tissue digestion. An example of calcium accumulation in the leaflets cultivated in growth and osteogenic media measured by ICP-OES is shown in Figure 10.
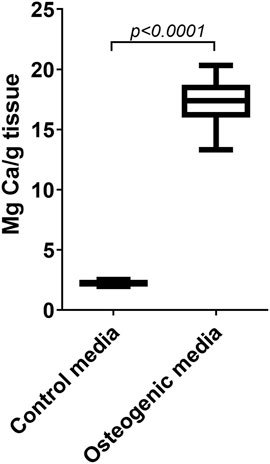
FIGURE 10. Induced calcification in porcine whole leaflets cultivated ex vivo. Calcium accumulation in whole pig leaflets cultivated for 4 weeks in control growth media and in osteogenic media. The amount of calcium was measured by inductively coupled plasma optical emission spectroscopy. The data are shown as box plots and wiskers with 5–95% percentiles.
6.3 Animal Models in vivo
As already stated above animal models are a necessary instrument for studying the underlying mechanisms of disease and its treatment. Unfortunately, CAVD is a disease with an unmet need for good animal models despite numerous proposed. The most commonly used animals for modelling CAVD are mice, rat, rabbit and porcine, however, only the latter is able to develop CAVD spontaneously (Sider et al., 2011). Below we have provided an overview of the most commonly used animal models.
6.3.1 Mouse Models
The majority of animal models of CAVD have been developed in mice. This is because of their cost-efficiency, rapid breeding and, most importantly, the availability of genetically modified variants. Regrettably, mouse models have significant limitations. Neither mouse nor rat aortic valve leaflets have the tri-layer structure akin to the human leaflet, only several layers of cells (Hinton et al., 2008). Wild-type mice do not develop aortic valve stenosis, however, a diet-based model has been reported with mild to moderate aortic stenosis (Drolet et al., 2006). A better alternative is transgenic mouse models. Until recently, the most commonly used mouse models contained a single gene mutation which affected lipid metabolism, the low-density lipoprotein receptor (Ldlr−/−) and apolipoprotein E deficient mice (ApoE −/−). These mice developed significant aortic valve calcification and some signs of CAVD when fed a high cholesterol diet. However, these models do not develop hemo-dynamically significant aortic valve stenosis (Rajamannan, 2014). To achieve stenosis the complex hypercholesterolemic mouse model with mutations in both Ldlr and ApoB100 (Apolipoprotein B100) (Ldlr −/−/ApoB100/100 mice) is necessary. It is efficiency is significantly increased if fed with a high cholesterol Western diet over 12 months (Weiss et al., 2006; Miller et al., 2009; Miller et al., 2010). This model is further developed by addition of a conditional knockout of the microsomal triglyceride transfer protein (Mttp) which plays a critical role in production of apolipoprotein B-containing lipoproteins (Ldlr−/−/Apob100/100/Mttpfl/fl/Mx1-Cre+/+)–the so-called Reversa model. This allows controlled onset of hyperlipidemia during the experimental aortic valve stenosis development (Miller et al., 2009). A recent study reports an improved of Ldlr −/−/ApoB100/100 mouse model that develops aortic stenosis earlier − after 6 month with high fat diet treatment - and gives insight into the role of platelet-derived TGF-β1 in CAVD progression (Varshney et al., 2019).
Several non-hyperlipidemic models offer features of CAVD including aortic valve leaflet calcification, but they lack the development of aortic stenosis. They include mice containing mutations in MGP (Matrix Gla protein) (Luo et al., 1997), EGFR (Epidermal growth factor receptor) (Barrick et al., 2009), Klotho (Cheek et al., 2012), RBPJk (Recombination Signal Binding Protein For Immunoglobulin Kappa J Region) (Nus et al., 2011) and IL1RN (Interleukin 1 Receptor Antagonist) (Isoda et al., 2010). Interestingly, despite strong calcification, the combination of high fat diet and vitamin D supplementation does not enhance the aortic stenosis phenotype of the EGFR mouse (Colleville et al., 2019).
Some genetic mouse models resemble human congenital aortic valve defects, which present with increased occurrence of CAVD. Bicuspid aortic valves are reported in mice containing mutations in eNOS (Endothelial nitric oxide synthase) (Lee et al., 2000), Notch1(Notch Receptor 1) (Nigam and Srivastava, 2009), Postn (Periostin) (Tkatchenko et al., 2009). A unicuspid aortic valve with some signs of CAVD was reported in a novel mouse model heterozygous for a dominant loss-of-function mutation in EGFR (Egfr Vel/+) (Weiss et al., 2018).
In addition to dietary and genetic mouse models, an in vivo valve injury model was developed by insertion of a spring guide wire into the left ventricle via the right common carotid artery under echocardiographic guidance, and scratching the leaflets with the body of the wire (Honda et al., 2014). This model was recently improved to achieve either mild, moderate or severe cusp injury to enable a more reproducible study of different stages of CAVD (Niepmann et al., 2019). It is important to notice that this direct injury models demonstrate typical clinical features of CAVD including inflammation, valve thickening, fibrosis and calcification combined with hemo-dynamically significant aortic stenosis as well as regurgitation (in severe injury). This model together with the Ldlr-deficient, ApoB100-only mice (Ldlr −/−/ApoB100/100) model appears to be the most relevant murine models of CAVD to date.
6.3.2 Rat Models
The rat aortic valve leaflets like the mouse, are not optimal for studies on CAVD because they consist of several cell layers without the tri-layered structure of human aortic valves (Grauss et al., 2003) (see above). A common model of vascular calcification and CAVD in rats is based on intravenous treatment with Warfarin, however, these rats do not develop hemo-dynamically significant aortic stenosis (Price et al., 1998) and warfarin-induced aortic valve calcification differs from the naturally occurring (Venardos et al., 2022). Such rats are phenotypically similar to MGP mutant mice, suggesting similar underlying mechanisms (Tsang et al., 2016). Mirroring the fact that renal failure is also associated with CAVD in humans, several uremic rat models induced by nephrectomy or high-adenine diet develop aortic valve calcification (Shuvy et al., 2008; Roosens et al., 2013a; Roosens et al., 2013b). Furthermore, vitamin D treatment causes vascular and aortic valve calcification in rats, but without aortic stenosis (Roosens et al., 2011). All taken together, rats do not represent an appropriate experimental model of aortic valve calcification with aortic stenosis.
6.3.3 Rabbit Models
Rabbits have both advantages and disadvantages as model for CAVD. They have the tri-layer leaflet composition similar to humans, several similarities in lipoprotein metabolism, and natural mutant and transgenic strains are available. Most frequently a hypercholesterolemic diet is administered to cause CAVD (Guerraty and Mohler Iii, 2007). A 40-weeks treatment with such diet induces early development of aortic stenosis (Cimini et al., 2005). When a hypercholesterolemic diet was coupled with vitamin D-induced hypercalcemia, significant calcium deposition developed in addition to aortic stenosis (Drolet et al., 2003; Guerraty and Mohler Iii, 2007). Another study demonstrated that a hypercholesterolemic and vitamin D2-supplemented diet caused leaflet thickening, calcification, matrix disorganization, and aortic stenosis (Marechaux et al., 2009). This combination appears to provide a better model of CAVD than hypercholesterolemic or vitamin D diets alone (Roosens et al., 2013a). However, a rabbit model using high-cholesterol diet is limited by liver dysfunction and high mortality rates due to cholesterol overload (Hara et al., 2018). In contrast, rabbit genetic models that have alterations in the Ldlr and/or apolipoprotein-encoding genes result in hypercholesterolemia even under a cholesterol-free, limited fat diet without cholesterol overload (Sider et al., 2011). Such a model is the Watanabe heritable hyperlipidemic rabbits that develop valve thickening, calcification, aortic stenosis and calcification-related gene activation (Hara et al., 2018). In addition to the hypercholesterolemic models, a hypertensive rabbit model develops increased valve thickness and mild aortic stenosis (Cuniberti et al., 2006).
6.3.4 Pig Models
The pig has tri-layered aortic valve leaflets similar to humans. Unlike mouse, rat and rabbit models, pigs are prone to naturally develop valvular atherosclerotic lesions (Skold et al., 1966). Swine develop valvular lesions and early signs of CAVD when fed with a high-fat/high-cholesterol diet for 5 months (Sider et al., 2014). Aortic valve calcification has been shown to be restricted to the aortic side in early CAVD in a porcine models with hypercholesterolemic diet (Guerraty et al., 2010). The Rapacz-familial hypercholesterolemic swine mutants develop leaflet thickening, increased lipid oxidation and infiltration of macrophages, however, further stimulation is needed to develop more advanced stages of CAVD with aortic stenosis (Porras et al., 2015). Additional swine models with lipid metabolism mutations used in atherosclerosis research may have a potential to be used in CAVD research (Sider et al., 2011). Tsang et al. have published a detailed review on the pig as a model for cardiovascular disease including CAVD (Tsang et al., 2016).
6.3.5 Other Animal Models
There are other animal models available, although potentially useful, they are not commonly used. For example, naturally occurring and experimental aortic stenosis has been investigated in dogs (Copeland et al., 1974; Kim et al., 1986; Ahlstrom Ast et al., 2008). Sheep are routinely used as a big animal model to investigate calcification of biological aortic valve prosthesis and homografts in vivo (Kheradvar et al., 2017; Theodoridis et al., 2017; Bester et al., 2018). Apparently, calcification occurs very rapidly in sheep compared to humans.
7 Multiomnics
7.1 Proteomics as an Example of Multi-Omnics Approaches
Multi-omnic approaches with proteomics, metabolomics and transcriptomics have recently gained momentum in aortic valve calcification investigations (Schlotter et al., 2018). Here as an example we have listed several approaches to perform proteomics analysis in CAVD research. Several groups have targeted proteome changes in human plasma during the development of calcific aortic valve disease for better understanding the basic mechanisms and to discover biomarkers (Gil-Dones et al., 2012; Satoh et al., 2015; Mourino-Alvarez et al., 2016; Olkowicz et al., 2017; Ljungberg et al., 2018). Additionally, gaining access to the plasma of both CAVD patients and healthy controls is rather straight-forward. This approach may be useful for the identification of biomarkers of CAVD in the blood of patients. The later aim is especially important because of the current lack of screening for early detection of CAVD. Targeting known problems of the proteome complexity in plasma, Gil−Dones et al. (Monzack and Masters, 2011) suggested improved protocols for plasma proteomics analysis in CAVD research.
An ex vivo modification of the plasma proteome analysis in patients with calcified aortic valves was reported as a secretome proteomics analysis from the explanted whole human leaflets kept for some time in growth media (Alvarez-Llamas et al., 2013; de la Cuesta et al., 2013). This approach allows one to mimic the secretome entering the circulation from aortic leaflets without interference from other tissues. Another common approach is to perform proteomics on the whole human leaflets explanted during the surgery or autopsy after lysate of the valve leaflets (Martin-Rojas et al., 2015; Weisell et al., 2019). A protein extraction procedure optimization was reported for this approach (Gil-Dones et al., 2010). The use of more advanced proteomics technique such as MALDI-imaging mass spectrometry offers the advantage to investigate the pathophysiological changes taking place in calcified aortic valves while retaining the histopathological context. This allows the simultaneous mapping of hundreds of peptides and proteins present in tissue sections with a lateral resolution of approximately 50–75 microns (Martin-Rojas et al., 2015).
Direct analysis of whole leaflets explanted from humans is most relevant to in vivo assessment, however, it has an important drawback as the analysis is performed on all cell types within the valve. Several groups partly overcome this problem by performing macro- (Matsumoto et al., 2012; Suzuki et al., 2016) and microdissection (Schlotter et al., 2018), subdividing the valve into calcified and non−calcified regions.
Another approach is to isolate and propagate in vitro VICs and subsequently perform proteomic analysis on human (Yu et al., 2018; Goto et al., 2019), bovine (Renato et al., 2013) or rat (Cui et al., 2017) cell cultures. Some authors have reported clonogenic sub−fractioning of the isolated and cultivated bovine VIC prior to proteomics analysis (Bertacco et al., 2010; Rattazzi et al., 2020). Unfortunately, gene studies in cultured VIC are influenced by the culture process per se. At the same time, omics analysis of calcified whole leaflets are “impure” containing material from several cell types in addition to VIC: VEC, vascular cells including smooth muscle cells from vascular ingrowth, as well as macrophages and other inflammatory cells. A combination of the above-mentioned proteomics approaches (whole leaflet, secretome, cell cultures, and plasma proteomics analysis) reveals more data than individual approaches (Martin-Rojas et al., 2017). Microarray and RNA sequencing with transcriptomics, in particular if combined with proteomics, might provide valuable information about signaling of the calcification process.
8 Biomarkers of Aortic Valve Calcification
To identify high-risk asymptomatic patients with aortic stenosis has become a major topic of interest during the last years. However, detailed discussion of risks and indications for surgical intervention is beyond the scope of this article (see review by Lindman et al. (Lindman et al., 2020)). Among a jungle of advanced and sometimes costly imaging modalities which may be predictive of disease progression and mortality in aortic stenosis (Nchimi et al., 2018), a blood sample for measuring circulating biomarkers is a simple, inexpensive, and easily available method to provide information about the stage and possible risks of asymptomatic aortic stenosis. Even if biomarkers represent indirect assessment, they might possibly be helpful to identify progression of CAVD and asymptomatic patients who then would benefit from aortic valve replacement.
Most interest has been focused on natriuretic peptides, in particular brain-natriuretic peptide (BNP) and its pro-hormone N-terminal pro B-type natriuretic peptide (NT-proBNP) as possible biomarkers of aortic stenosis (Weber et al., 2004; Steadman et al., 2010; Clavel et al., 2014; Auensen et al., 2017; Small et al., 2017). The biomarker does not reflect calcification per se, but it provides diagnostic and prognostic information about myocardial remodeling as a consequence of aortic stenosis. Marked increased levels of BNP may reflect irreversible injury to the myocardium and has been shown to predict worse outcome in patients after transcatheter aortic valve interventions (O'Neill et al., 2015). The same is the case with cardiac troponins (Köhler et al., 2016). BNP is the only biomarker in the circulation accepted to have prognostic value in the guidelines of the European Society for Cardiology and the European Association for Cardio-Thoracic Surgery (but not for the American Heart Association or the American College of Cardiology).
Recently, a series of other potential biomarkers in the circulation have brokered interest, such as for instance von Willebrand Factor (vWF) due to high shear stress in aortic stenosis (Van Belle et al., 2019). Plasma levels and function of vWF is reduced in parallel with severity of aortic stenosis. The levels are normalized after transcatheter aortic valve intervention, but did not normalize if a paravalvular leakage was present (Van Belle et al., 2016).
Of particular interests for this review are biomarkers that may be directly related to the calcification process. This includes microRNAs (Oury et al., 2016), fetuin-A (Di Minno et al., 2017), osteopontin (Sainger et al., 2013), osteoprogeterin (Ueland et al., 2011), and MGP (Ueland et al., 2010). Notch may have an important role in aortic valve calcification (Kostina et al., 2018) and the Notch ligand Delta-1 is elevated and associated with mortality in patients with symptomatic aortic stenosis (Abraityte et al., 2015). Elmariah et al. suggested that a panel of multiple biomarkers including age, NT-proBNP, vWF, and fetuin-A would be valuable for the identification of high-risk patients with aortic stenosis and for timely valve intervention (Elmariah et al., 2018). MacGrogan et al also suggested that a set of several genes in blood provided a “gene signature” predicting aortic valve calcification (MacGrogan et al., 2020).
So far neither guidelines of the American Heart Association, the American College of Cardiology, the European Society of Cardiology, nor the European Association for Cardio-Thoracic Surgery include these biomarkers as valuable for evaluation of patients with CAVD. The role of biomarkers as a guide to more aggressive aortic valve replacement in asymptomatic patients has yet to be investigated. It might well be in the future a profile of several biomarkers may be useful. A full discussion of the field is beyond the scope of this review, however, several concise and recent reviews have been published on this topic (Redfors et al., 2017; Small et al., 2017; Patel and Kumbhani, 2018; Toutouzas et al., 2019; Oury et al., 2020).
9 Calcium Phosphate Protein Particles
Circulating calcium phosphate protein particles might be important both for the understanding of the processes leading to calcification and for the development of therapy for both valvular and vascular calcifications. Such particles have not been found in the circulation of healthy individuals, but exist in the circulation of patients with some inflammatory diseases (Smith et al., 2013). The number of particles in the blood can be reduced by sodium thiosulphate which has been suggested to reduce vascular calcification (Cai et al., 2013). Fetuin-A is a key player in the formation of calcium phosphate protein particles. This protein is an endogenous inhibitor of soft tissue calcification by inhibiting formation of calcium phosphate (Heiss et al., 2010). Once a mineral nuclei is formed, fetuin-A binds to the apatite surface and inhibits the formation of larger entities (Price and Lim, 2003). The nanoparticles consisting of calcium phosphate crystals may be a way to clear calcium and inhibit calcification; they are cleared from the circulation in the liver and the spleen, a process which is dependent on scavenger receptors on phagocyte surfaces (Herrmann et al., 2012). The role of calcium phosphate protein particles in soft tissue calcification is uncertain, however, in pro-calcific situations, the particles may have structural transformation into larger particles with a crystalline core and initiate calcification (Jahnen-Dechent et al., 2011). Using nano-analytical electron microscopy techniques, Bertazzo et al. found such mineralized particles on the aortic valve even before calcification of the valve (Bertazzo et al., 2013). The presence of these particles might perhaps even initiate CAVD (Bertazzo and Gentleman, 2017). This is in line with findings that crystallinity of hydroxyapatite in 3D cultures with VEC and VIC increase calcium accumulation (Richards et al., 2018). Detailed methods for studying calcium phosphate particles in human serum, on tissues, and in tissues include ultracentrifugation, gel filtration, scanning and transmission electron microscopy, measurements of calcium and phosphate, energy-dispersive X-ray spectroscopy, selected area electron diffraction analyses, and material science technology in general (Price and Lim, 2003; Bertazzo et al., 2013).
10 Discussion
The process of aortic valve calcification is still far from elucidated. In this overview we try to cover presently used methods to study CAVD, from translational studies in cell cultures to patient studies. With the lack of good animal models, translational studies in cell cultures are by far the most frequently used model to clarify the cellular and molecular mechanisms of calcification. Consequently, this is the only model where more detailed techniques were presented. Cells from human aortic valves should be used in order to avoid species differences. Cell models are also suitable for screening of potentially inhibitory drugs. There is an unmet need for good models of aortic valve calcification in animals where the structure of the valve leaflet is similar to the structure of human aortic valve. Moreover, we know too little about the mineral structure of calcified valves including its role. With increasing use of endovascular implantation of aortic valve prostheses, good imaging of the aortic ostium and the valve has become more and more important. Possibly, MRI should be used more extensively. There is also a need for good biomarkers. Unfortunately, although there are suggestions for several biomarkers, it is highly uncertain how they should be used. Biomarkers cannot replace imaging because the structure and degree of stenosis are decisive for clinical decisions.
Author Contributions
MB established cell techniques and wrote parts of the manuscript, AZ contributed to cell techniques, established whole valve leaflet model, revised the manuscript and wrote some parts, AM supervised techniques and revised the manuscript, KE contributed to cell techniques, AK supervised and revised the manuscript, J-PEK; MK managed logistics and sampling of aortic valves and revised manuscript, KS supervised work and revised manuscript, MP measured calcium in tissue and revised the manuscript, RS contributed with techniques, GS supervised on cell methodology and revised manuscript, JV initiated and supervised the project, wrote some parts and revised manuscript, AR established cell techniques, co-supervised the project and revised manuscript.
Funding
This work has been funded by The South-Eastern Health Authorities by a postdoc scholarship to AR. AZ has been the recipient of a Scientia Fellow scholarship funded by the European Union and the Faculty of Medicine, University of Oslo, and has at present a postdoc scholarship from the Norwegian Health Association. Further funding has been received by the University of Oslo (including PhD scholarship to MB), The Norwegian Health Association, and by Russian Science Foundation (grant # 18-14-00152). GJS was partly supported by the Research Council of Norway through its Center of Excellence funding scheme (project number 262613).
Conflict of Interest
Author MP was employed by Sanifit Therapeutics.
The remaining authors declare that the research was conducted in the absence of any commercial or financial relationships that could be construed as a potential conflict of interest.
Publisher’s Note
All claims expressed in this article are solely those of the authors and do not necessarily represent those of their affiliated organizations, or those of the publisher, the editors and the reviewers. Any product that may be evaluated in this article, or claim that may be made by its manufacturer, is not guaranteed or endorsed by the publisher.
Acknowledgments
Professor Arnt Fiane, chairman of the Department of Cardiothoracic Surgery, Oslo University Hospital, generously made sampling of heart valves possible. Torunn Flatebø and Kristin Larsen Sand provided technical assistance, Kristin Larsen Sand also helped with the production of Figure 4.
References
Abraityte, A., Gullestad, L., Askevold, E. T., Nymo, S., Dahl, C. P., Aakhus, S., et al. (2015). The Notch Ligand Delta-like 1 Is Elevated and Associated with Mortality in Patients with Symptomatic Aortic Stenosis. Int. J. Cardiol. 180, 18–20. doi:10.1016/j.ijcard.2014.11.111
Aggarwal, S. R., Clavel, M. A., Messika-Zeitoun, D., Cueff, C., Malouf, J., Araoz, P. A., et al. (2013). Sex Differences in Aortic Valve Calcification Measured by Multidetector Computed Tomography in Aortic Stenosis. Circ. Cardiovasc Imaging 6 (1), 40–47. doi:10.1161/CIRCIMAGING.112.980052
Ahlstrom Ast, C., Höglund, K., Hult, P., Häggström, J., Kvart, C., and Ask, P. (2008). Assessing Aortic Stenosis Using Sample Entropy of the Phonocardiographic Signal in Dogs. IEEE Trans. Biomed. Eng. 55 (8), 2107–2109. doi:10.1109/TBME.2008.923767
Alvarez-Llamas, G., Martín-Rojas, T., de la Cuesta, F., Calvo, E., Gil-Dones, F., Dardé, V. M., et al. (2013). Modification of the Secretion Pattern of Proteases, Inflammatory Mediators, and Extracellular Matrix Proteins by Human Aortic Valve Is Key in Severe Aortic Stenosis. Mol. Cell Proteomics 12 (9), 2426–2439. doi:10.1074/mcp.M113.027425
Auensen, A., Hussain, A. I., Falk, R. S., Walle-Hansen, M. M., Bye, J., Pettersen, K. I., et al. (2017). Associations of Brain-Natriuretic Peptide, High-Sensitive Troponin T, and High-Sensitive C-Reactive Protein with Outcomes in Severe Aortic Stenosis. PLoS One 12 (6), e0179304. doi:10.1371/journal.pone.0179304
Babu, A. N., Meng, X., Zou, N., Yang, X., Wang, M., Song, Y., et al. (2008). Lipopolysaccharide Stimulation of Human Aortic Valve Interstitial Cells Activates Inflammation and Osteogenesis. Ann. Thorac. Surg. 86 (1), 71–76. doi:10.1016/j.athoracsur.2008.03.008
Bailey, G., Meadows, J., and Morrison, A. R. (2016). Imaging Atherosclerotic Plaque Calcification: Translating Biology. Curr. Atheroscler. Rep. 18 (8), 51. doi:10.1007/s11883-016-0601-6
Balachandran, K., Konduri, S., Sucosky, P., Jo, H., and Yoganathan, A. P. (2006). An Ex Vivo Study of the Biological Properties of Porcine Aortic Valves in Response to Circumferential Cyclic Stretch. Ann. Biomed. Eng. 34 (11), 1655–1665. doi:10.1007/s10439-006-9167-8
Balachandran, K., Sucosky, P., Jo, H., and Yoganathan, A. P. (2010). Elevated Cyclic Stretch Induces Aortic Valve Calcification in a Bone Morphogenic Protein-dependent Manner. Am. J. Pathol. 177 (1), 49–57. doi:10.2353/ajpath.2010.090631
Baralkiewicz, D., Gramowska, H., Hanć, A., and Krzyzaniak, I. (2007). A Comparison of ICP-OES and ICP-MS in the Determination of Elements in Lake Water. At. Spectrosc. -Norwalk Connecticut- 28, 164–170.
Barrick, C. J., Roberts, R. B., Rojas, M., Rajamannan, N. M., Suitt, C. B., O'Brien, K. D., et al. (2009). Reduced EGFR Causes Abnormal Valvular Differentiation Leading to Calcific Aortic Stenosis and Left Ventricular Hypertrophy in C57BL/6J but Not 129S1/SvImJ Mice. Am. J. Physiol. Heart Circ. Physiol. 297 (1), H65–H75. doi:10.1152/ajpheart.00866.2008
Benton, J. A., Kern, H. B., and Anseth, K. S. (2008). Substrate Properties Influence Calcification in Valvular Interstitial Cell Culture. J. Heart Valve Dis. 17 (6), 689–699.
Bertacco, E., Millioni, R., Arrigoni, G., Faggin, E., Iop, L., Puato, M., et al. (2010). Proteomic Analysis of Clonal Interstitial Aortic Valve Cells Acquiring a Pro-calcific Profile. J. Proteome Res. 9 (11), 5913–5921. doi:10.1021/pr100682g
Bertazzo, S., and Gentleman, E. (2017). Aortic Valve Calcification: a Bone of Contention. Eur. Heart J. 38 (16), 1189–1193. doi:10.1093/eurheartj/ehw071
Bertazzo, S., Gentleman, E., Cloyd, K. L., Chester, A. H., Yacoub, M. H., and Stevens, M. M. (2013). Nano-analytical Electron Microscopy Reveals Fundamental Insights into Human Cardiovascular Tissue Calcification. Nat. Mater 12 (6), 576–583. doi:10.1038/nmat3627
Bester, D., Botes, L., van den Heever, J. J., Kotze, H., Dohmen, P., Pomar, J. L., et al. (2018). Cadaver Donation: Structural Integrity of Pulmonary Homografts Harvested 48 H Post Mortem in the Juvenile Ovine Model. Cell Tissue Bank. 19 (4), 743–754. doi:10.1007/s10561-018-9729-7
Bettinger, N., Khalique, O. K., Krepp, J. M., Hamid, N. B., Bae, D. J., Pulerwitz, T. C., et al. (2017). Practical Determination of Aortic Valve Calcium Volume Score on Contrast-Enhanced Computed Tomography Prior to Transcatheter Aortic Valve Replacement and Impact on Paravalvular Regurgitation: Elucidating Optimal Threshold Cutoffs. J. Cardiovasc Comput. Tomogr. 11 (4), 302–308. doi:10.1016/j.jcct.2017.04.009
Bhatia, N., Basra, S. S., Skolnick, A. H., and Wenger, N. K. (2016). Aortic Valve Disease in the Older Adult. J. Geriatr. Cardiol. 13 (12), 941–944. doi:10.11909/j.issn.1671-5411.2016.12.004
Bogdanova, M., Kostina, A., Zihlavnikova Enayati, K., Zabirnyk, A., Malashicheva, A., Stensløkken, K. O., et al. (2018). Inflammation and Mechanical Stress Stimulate Osteogenic Differentiation of Human Aortic Valve Interstitial Cells. Front. Physiol. 9 (1635), 1635. doi:10.3389/fphys.2018.01635
Bogdanova, M., Zabirnyk, A., Malashicheva, A., Enayati, K. Z., Karlsen, T. A., Kaljusto, M. L., et al. (2019). Interstitial Cells in Calcified Aortic Valves Have Reduced Differentiation Potential and Stem Cell-like Properties. Sci. Rep. 9 (1), 12934. doi:10.1038/s41598-019-49016-0
Bond, W. S., Roberts, E. L., and Warnock, J. N. (2007). Evaluation of Porcine Aortic Valve Interstitial Cell Activity Using Different Serum Types in Two- and Three-Dimensional Culture. Tissue Eng. 13 (2), 343–349. doi:10.1089/ten.2006.0166
Bouchareb, R., Mahmut, A., Nsaibia, M. J., Boulanger, M. C., Dahou, A., Lépine, J. L., et al. (2015). Autotaxin Derived from Lipoprotein(a) and Valve Interstitial Cells Promotes Inflammation and Mineralization of the Aortic Valve. Circulation 132 (8), 677–690. doi:10.1161/CIRCULATIONAHA.115.016757
Bowler, M. A., and Merryman, W. D. (2015). In Vitro models of Aortic Valve Calcification: Solidifying a System. Cardiovasc Pathol. 24 (1), 1–10. doi:10.1016/j.carpath.2014.08.003
Bracco Gartner, T. C. L., Deddens, J. C., Mol, E. A., Magin Ferrer, M., van Laake, L. W., Bouten, C. V. C., et al. (2019). Anti-fibrotic Effects of Cardiac Progenitor Cells in a 3D-Model of Human Cardiac Fibrosis. Front. Cardiovasc Med. 6, 52. doi:10.3389/fcvm.2019.00052
Braselton, W. E., Stuart, K. J., Mullaney, T. P., and Herdt, T. H. (1997). Biopsy Mineral Analysis by Inductively Coupled Plasma-Atomic Emission Spectroscopy with Ultrasonic Nebulization. J. Vet. Diagn Invest 9 (4), 395–400. doi:10.1177/104063879700900409
Butcher, J. T., and Nerem, R. M. (2004). Porcine Aortic Valve Interstitial Cells in Three-Dimensional Culture: Comparison of Phenotype with Aortic Smooth Muscle Cells. J. Heart Valve Dis. 13 (3), 478–485.
Butcher, J. T., and Nerem, R. M. (2006). Valvular Endothelial Cells Regulate the Phenotype of Interstitial Cells in Co-culture: Effects of Steady Shear Stress. Tissue Eng. 12 (4), 905–915. doi:10.1089/ten.2006.12.905
Cai, M. M., Smith, E. R., Brumby, C., McMahon, L. P., and Holt, S. G. (2013). Fetuin-A-containing Calciprotein Particle Levels Can Be Reduced by Dialysis, Sodium Thiosulphate and Plasma Exchange. Potential Therapeutic Implications for Calciphylaxis? Nephrol. Carlt. 18 (11), 724–727. doi:10.1111/nep.12137
Carpenter, R. C. (1985). The Analysis of Some Evidential Materials by Inductively Coupled Plasma-Optical Emission Spectrometry. Forensic Sci. Int. 27 (3), 157–163. doi:10.1016/0379-0738(85)90152-5
Cartlidge, T. R., Bing, R., Kwiecinski, J., Guzzetti, E., Pawade, T. A., Doris, M. K., et al. (2021). Contrast-enhanced Computed Tomography Assessment of Aortic Stenosis. Heart 107 (23), 1905–1911. doi:10.1136/heartjnl-2020-318556
Cartlidge, T. R. G., Doris, M. K., Sellers, S. L., Pawade, T. A., White, A. C., Pessotto, R., et al. (2019). Detection and Prediction of Bioprosthetic Aortic Valve Degeneration. J. Am. Coll. Cardiol. 73 (10), 1107–1119. doi:10.1016/j.jacc.2018.12.056
Cheek, J. D., Wirrig, E. E., Alfieri, C. M., James, J. F., and Yutzey, K. E. (2012). Differential Activation of Valvulogenic, Chondrogenic, and Osteogenic Pathways in Mouse Models of Myxomatous and Calcific Aortic Valve Disease. J. Mol. Cell Cardiol. 52 (3), 689–700. doi:10.1016/j.yjmcc.2011.12.013
Chen, J. H., Chen, W. L., Sider, K. L., Yip, C. Y., and Simmons, C. A. (2011). β-Catenin Mediates Mechanically Regulated, Transforming Growth Factor-Β1-Induced Myofibroblast Differentiation of Aortic Valve Interstitial Cells. Arterioscler. Thromb. Vasc. Biol. 31 (3), 590–597. doi:10.1161/ATVBAHA.110.220061
Chen, J. H., and Simmons, C. A. (2011). Cell-matrix Interactions in the Pathobiology of Calcific Aortic Valve Disease: Critical Roles for Matricellular, Matricrine, and Matrix Mechanics Cues. Circ. Res. 108 (12), 1510–1524. doi:10.1161/CIRCRESAHA.110.234237
Chester, A. H., Kershaw, J. D., Sarathchandra, P., and Yacoub, M. H. (2008). Localisation and Function of Nerves in the Aortic Root. J. Mol. Cell Cardiol. 44 (6), 1045–1052. doi:10.1016/j.yjmcc.2008.03.014
Chester, A. H., Sarathchandra, P., McCormack, A., and Yacoub, M. H. (2021). Organ Culture Model of Aortic Valve Calcification. Front. Cardiovasc Med. 8, 734692. doi:10.3389/fcvm.2021.734692
Chitsaz, S., Gundiah, N., Blackshear, C., Tegegn, N., Yan, K. S., Azadani, A. N., et al. (2012). Correlation of Calcification on Excised Aortic Valves by Micro-computed Tomography with Severity of Aortic Stenosis. J. Heart Valve Dis. 21 (3), 320–327.
Chong, A., Senior, R., and Wahi, S. (2019). Contemporary Imaging of Aortic Stenosis. Heart Lung Circ. 28 (9), 1310–1319. doi:10.1016/j.hlc.2019.05.177
Cimini, M., Boughner, D. R., Ronald, J. A., Aldington, L., and Rogers, K. A. (2005). Development of Aortic Valve Sclerosis in a Rabbit Model of Atherosclerosis: an Immunohistochemical and Histological Study. J. Heart Valve Dis. 14 (3), 365–375.
Clavel, M. A., Malouf, J., Michelena, H. I., Suri, R. M., Jaffe, A. S., Mahoney, D. W., et al. (2014). B-type Natriuretic Peptide Clinical Activation in Aortic Stenosis: Impact on Long-Term Survival. J. Am. Coll. Cardiol. 63 (19), 2016–2025. doi:10.1016/j.jacc.2014.02.581
Colleville, B., Perzo, N., Avinée, G., Dumesnil, A., Ziegler, F., Billoir, P., et al. (2019). Impact of High-Fat Diet and Vitamin D3 Supplementation on Aortic Stenosis Establishment in Waved-2 Epidermal Growth Factor Receptor Mutant Mice. J. Integr. Med. 17 (2), 107–114. doi:10.1016/j.joim.2019.01.010
Copeland, J. G., Maron, B. J., Luka, N. L., Ferrans, V. J., and Michaelis, L. L. (1974). Experimental Production of Aortic Valvular Stenosis. Short-Term and Long-Term Studies in Dogs. J. Thorac. Cardiovasc Surg. 67 (3), 371–379. doi:10.1016/s0022-5223(19)40509-6
Cottignoli, V., Cavarretta, E., Salvador, L., Valfré, C., and Maras, A. (2015). Morphological and Chemical Study of Pathological Deposits in Human Aortic and Mitral Valve Stenosis: a Biomineralogical Contribution. Pathol. Res. Int. 2015, 342984. doi:10.1155/2015/342984
Cottignoli, V., Relucenti, M., Agrosì, G., Cavarretta, E., Familiari, G., Salvador, L., et al. (2015). Biological Niches within Human Calcified Aortic Valves: Towards Understanding of the Pathological Biomineralization Process. Biomed. Res. Int. 2015, 542687. doi:10.1155/2015/542687
Cowell, S. J., Newby, D. E., Burton, J., White, A., Northridge, D. B., Boon, N. A., et al. (2003). Aortic Valve Calcification on Computed Tomography Predicts the Severity of Aortic Stenosis. Clin. Radiol. 58 (9), 712–716. doi:10.1016/s0009-9260(03)00184-3
Cueff, C., Serfaty, J. M., Cimadevilla, C., Laissy, J. P., Himbert, D., Tubach, F., et al. (2011). Measurement of Aortic Valve Calcification Using Multislice Computed Tomography: Correlation with Haemodynamic Severity of Aortic Stenosis and Clinical Implication for Patients with Low Ejection Fraction. Heart 97 (9), 721–726. doi:10.1136/hrt.2010.198853
Cui, L., Rashdan, N. A., Zhu, D., Milne, E. M., Ajuh, P., Milne, G., et al. (2017). End Stage Renal Disease-Induced Hypercalcemia May Promote Aortic Valve Calcification via Annexin VI Enrichment of Valve Interstitial Cell Derived-Matrix Vesicles. J. Cell Physiol. 232 (11), 2985–2995. doi:10.1002/jcp.25935
Cuniberti, L. A., Stutzbach, P. G., Guevara, E., Yannarelli, G. G., Laguens, R. P., and Favaloro, R. R. (2006). Development of Mild Aortic Valve Stenosis in a Rabbit Model of Hypertension. J. Am. Coll. Cardiol. 47 (11), 2303–2309. doi:10.1016/j.jacc.2005.12.070
Cushing, M. C., Mariner, P. D., Liao, J. T., Sims, E. A., and Anseth, K. S. (2008). Fibroblast Growth Factor Represses Smad-Mediated Myofibroblast Activation in Aortic Valvular Interstitial Cells. FASEB J. 22 (6), 1769–1777. doi:10.1096/fj.07-087627
Danilchenko, S., Kuznetsov, V., Stanislavov, A., Kalinkevich, A., Starikov, V., Moskalenko, R., et al. (2013). The Mineral Component of Human Cardiovascular Deposits: Morphological, Structural and Crystal-Chemical Characterization. Cryst. Res. Technol. 48. doi:10.1002/crat.201200443
de la Cuesta, F., Alvarez-Llamas, G., Gil-Dones, F., Darde, V. M., Calvo, E., López, J. A., et al. (2013). Secretome of Human Aortic Valves. Methods Mol. Biol. 1005, 237–243. doi:10.1007/978-1-62703-386-2_19
Di Minno, A., Zanobini, M., Myasoedova, V. A., Valerio, V., Songia, P., Saccocci, M., et al. (2017). Could Circulating Fetuin A Be a Biomarker of Aortic Valve Stenosis? Int. J. Cardiol. 249, 426–430. doi:10.1016/j.ijcard.2017.05.040
Drolet, M. C., Arsenault, M., and Couet, J. (2003). Experimental Aortic Valve Stenosis in Rabbits. J. Am. Coll. Cardiol. 41 (7), 1211–1217. doi:10.1016/s0735-1097(03)00090-1
Drolet, M. C., Roussel, E., Deshaies, Y., Couet, J., and Arsenault, M. (2006). A High Fat/high Carbohydrate Diet Induces Aortic Valve Disease in C57BL/6J Mice. J. Am. Coll. Cardiol. 47 (4), 850–855. doi:10.1016/j.jacc.2005.09.049
Dutta, P., Kodigepalli, K. M., LaHaye, S., Thompson, J. W., Rains, S., Nagel, C., et al. (2021). KPT-330 Prevents Aortic Valve Calcification via a Novel C/EBPβ Signaling Pathway. Circ. Res. 128 (9), 1300–1316. doi:10.1161/CIRCRESAHA.120.318503
Dutta, P., and Lincoln, J. (2018). Calcific Aortic Valve Disease: a Developmental Biology Perspective. Curr. Cardiol. Rep. 20 (4), 21. doi:10.1007/s11886-018-0968-9
Dweck, M. R., Jenkins, W. S., Vesey, A. T., Pringle, M. A., Chin, C. W., Malley, T. S., et al. (2014). 18F-sodium Fluoride Uptake Is a Marker of Active Calcification and Disease Progression in Patients with Aortic Stenosis. Circ. Cardiovasc Imaging 7 (2), 371–378. doi:10.1161/CIRCIMAGING.113.001508
El-Hamamsy, I., Balachandran, K., Yacoub, M. H., Stevens, L. M., Sarathchandra, P., Taylor, P. M., et al. (2009). Endothelium-dependent Regulation of the Mechanical Properties of Aortic Valve Cusps. J. Am. Coll. Cardiol. 53 (16), 1448–1455. doi:10.1016/j.jacc.2008.11.056
Elmariah, S., McCarthy, C., Ibrahim, N., Furman, D., Mukai, R., Magaret, C., et al. (2018). Multiple Biomarker Panel to Screen for Severe Aortic Stenosis: Results from the CASABLANCA Study. Open Heart 5 (2), e000916–e. doi:10.1136/openhrt-2018-000916
Farrar, E. J., and Butcher, J. T. (2014). Heterogeneous Susceptibility of Valve Endothelial Cells to Mesenchymal Transformation in Response to TNFα. Ann. Biomed. Eng. 42 (1), 149–161. doi:10.1007/s10439-013-0894-3
Fishbein, M. C., Levy, R. J., Ferrans, V. J., Dearden, L. C., Nashef, A., Goodman, A. P., et al. (1982). Calcifications of Cardiac Valve Bioprostheses. Biochemical, Histologic, and Ultrastructural Observations in a Subcutaneous Implantation Model System. J. Thorac. Cardiovasc Surg. 83 (4), 602–609. doi:10.1016/s0022-5223(19)37251-4
Fletcher, A. J., Singh, T., Syed, M. B. J., and Dweck, M. R. (2021). Imaging Aortic Valve Calcification: Significance, Approach and Implications. Clin. Radiol. 76 (1), 15–26. doi:10.1016/j.crad.2020.04.007
Francone, M., Budde, R. P. J., Bremerich, J., Dacher, J. N., Loewe, C., Wolf, F., et al. (2020). CT and MR Imaging Prior to Transcatheter Aortic Valve Implantation: Standardisation of Scanning Protocols, Measurements and Reporting-A Consensus Document by the European Society of Cardiovascular Radiology (ESCR). Eur. Radiol. 30 (5), 2627–2650. doi:10.1007/s00330-019-06357-8
Galeone, A., Brunetti, G., Oranger, A., Greco, G., Di Benedetto, A., Mori, G., et al. (2013). Aortic Valvular Interstitial Cells Apoptosis and Calcification Are Mediated by TNF-Related Apoptosis-Inducing Ligand. Int. J. Cardiol. 169 (4), 296–304. doi:10.1016/j.ijcard.2013.09.012
Gall, K. L., Smith, S. E., Willmette, C. A., and O'Brien, M. F. (1998). Allograft Heart Valve Viability and Valve-Processing Variables. Ann. Thorac. Surg. 65 (4), 1032–1038. doi:10.1016/s0003-4975(98)00085-x
Gayrard, N., Muyor, K., Notarnicola, C., Duranton, F., Jover, B., and Argilés, À. (2020). Optimisation of Cell and Ex Vivo Culture Conditions to Study Vascular Calcification. PLoS One 15 (3), e0230201. doi:10.1371/journal.pone.0230201
Gil-Dones, F., Darde, V. M., Alonso-Orgaz, S., Lopez-Almodovar, L. F., Mourino-Alvarez, L., Padial, L. R., et al. (2012). Inside Human Aortic Stenosis: a Proteomic Analysis of Plasma. J. Proteomics 75 (5), 1639–1653. doi:10.1016/j.jprot.2011.11.036
Gil-Dones, F., Martin-Rojas, T., Lopez-Almodovar, L. F., de la Cuesta, F., Darde, V. M., Alvarez-Llamas, G., et al. (2010). Valvular Aortic Stenosis: a Proteomic Insight. Clin. Med. Insights Cardiol. 4, 1–7. doi:10.4137/cmc.s3884
Gomel, M. A., Lee, R., and Grande-Allen, K. J. (2018). Comparing the Role of Mechanical Forces in Vascular and Valvular Calcification Progression. Front. Cardiovasc Med. 5, 197. doi:10.3389/fcvm.2018.00197
Gomez Stallons, M. V., Wirrig-Schwendeman, E. E., Fang, M., Cheek, J. D., Alfieri, C. M., Hinton, R. B., et al. (2016). “Molecular Mechanisms of Heart Valve Development and Disease,” in Etiology and Morphogenesis of Congenital Heart Disease: From Gene Function and Cellular Interaction to Morphology. Tokyo2016. Editors T. Nakanishi, R. R. Markwald, H. S. Baldwin, B. B. Keller, D. Srivastava, and H. Yamagishi, 145–151. doi:10.1007/978-4-431-54628-3_18
Gonzalez Rodriguez, A., Schroeder, M. E., Grim, J. C., Walker, C. J., Speckl, K. F., Weiss, R. M., et al. (2021). Tumor Necrosis Factor-α Promotes and Exacerbates Calcification in Heart Valve Myofibroblast Populations. FASEB J. official Publ. Fed. Am. Soc. Exp. Biol. 35 (3), e21382. doi:10.1096/fj.202002013rr
Goto, S., Rogers, M. A., Blaser, M. C., Higashi, H., Lee, L. H., Schlotter, F., et al. (2019). Standardization of Human Calcific Aortic Valve Disease In Vitro Modeling Reveals Passage-dependent Calcification. Front. Cardiovasc Med. 6, 49. doi:10.3389/fcvm.2019.00049
Gould, R. A., and Butcher, J. T. (2010). Isolation of Valvular Endothelial Cells. J. Vis. Exp. 46. doi:10.3791/2158
Gould, S. T., Matherly, E. E., Smith, J. N., Heistad, D. D., and Anseth, K. S. (2014). The Role of Valvular Endothelial Cell Paracrine Signaling and Matrix Elasticity on Valvular Interstitial Cell Activation. Biomaterials 35 (11), 3596–3606. doi:10.1016/j.biomaterials.2014.01.005
Gourgas, O., Khan, K., Schwertani, A., and Cerruti, M. (2020). Differences in Mineral Composition and Morphology between Men and Women in Aortic Valve Calcification. Acta Biomater. 106, 342–350. doi:10.1016/j.actbio.2020.02.030
Grauss, R. W., Hazekamp, M. G., van Vliet, S., Gittenberger-de Groot, A. C., and DeRuiter, M. C. (2003). Decellularization of Rat Aortic Valve Allografts Reduces Leaflet Destruction and Extracellular Matrix Remodeling. J. Thorac. Cardiovasc Surg. 126 (6), 2003–2010. doi:10.1016/s0022-5223(03)00956-5
Gregory, C. A., Gunn, W. G., Peister, A., and Prockop, D. J. (2004). An Alizarin Red-Based Assay of Mineralization by Adherent Cells in Culture: Comparison with Cetylpyridinium Chloride Extraction. Anal. Biochem. 329 (1), 77–84. doi:10.1016/j.ab.2004.02.002
Grodecki, K., Tamarappoo, B. K., Huczek, Z., Jedrzejczyk, S., Cadet, S., Kwiecinski, J., et al. (2021). Non-calcific Aortic Tissue Quantified from Computed Tomography Angiography Improves Diagnosis and Prognostication of Patients Referred for Transcatheter Aortic Valve Implantation. Eur. Heart J. Cardiovasc Imaging 22 (6), 626–635. doi:10.1093/ehjci/jeaa304
Gu, X., and Masters, K. S. (2010). Regulation of Valvular Interstitial Cell Calcification by Adhesive Peptide Sequences. J. Biomed. Mater Res. A 93 (4), 1620–1630. doi:10.1002/jbm.a.32660
Guerraty, M., and Mohler Iii, E. R. (2007). Models of Aortic Valve Calcification. J. Investig. Med. 55 (6), 278–283. doi:10.2310/6650.2007.00012
Guerraty, M. A., Grant, G. R., Karanian, J. W., Chiesa, O. A., Pritchard, W. F., and Davies, P. F. (2010). Hypercholesterolemia Induces Side-specific Phenotypic Changes and Peroxisome Proliferator-Activated Receptor-Gamma Pathway Activation in Swine Aortic Valve Endothelium. Arterioscler. Thromb. Vasc. Biol. 30 (2), 225–231. doi:10.1161/ATVBAHA.109.198549
Gwanmesia, P., Ziegler, H., Eurich, R., Barth, M., Kamiya, H., Karck, M., et al. (2010). Opposite Effects of Transforming Growth Factor-Β1 and Vascular Endothelial Growth Factor on the Degeneration of Aortic Valvular Interstitial Cell Are Modified by the Extracellular Matrix Protein Fibronectin: Implications for Heart Valve Engineering. Tissue Eng. Part A 16 (12), 3737–3746. doi:10.1089/ten.TEA.2010.0304
Hanć, A., Komorowicz, I., Iskra, M., Majewski, W., and Barałkiewicz, D. (2011). Application of Spectroscopic Techniques: ICP-OES, LA-ICP-MS and Chemometric Methods for Studying the Relationships between Trace Elements in Clinical Samples from Patients with Atherosclerosis Obliterans. Anal. Bioanal. Chem. 399 (9), 3221–3231. doi:10.1007/s00216-011-4729-5
Hara, T., Tsukada, N., Okano, M., Ishida, T., Hirata, K. I., and Shiomi, M. (2018). Progression of Calcific Aortic Valve Sclerosis in WHHLMI Rabbits. Atherosclerosis 273, 8–14. doi:10.1016/j.atherosclerosis.2018.03.044
Heiss, A., Pipich, V., Jahnen-Dechent, W., and Schwahn, D. (2010). Fetuin-A Is a Mineral Carrier Protein: Small Angle Neutron Scattering Provides New Insight on Fetuin-A Controlled Calcification Inhibition. Biophys. J. 99 (12), 3986–3995. doi:10.1016/j.bpj.2010.10.030
Heitkemper, D. T., Kaine, L. A., Jackson, D. S., and Wolnik, K. A. (1994). Practical Applications of Element-specific Detection by Inductively Coupled Plasma Atomic Emission Spectroscopy and Inductively Coupled Plasma Mass Spectrometry to Ion Chromatography of Foods. J. Chromatogr. A 671 (1-2), 101–108. doi:10.1016/0021-9673(94)80227-0
Herrmann, M., Schäfer, C., Heiss, A., Gräber, S., Kinkeldey, A., Büscher, A., et al. (2012). Clearance of Fetuin-A-Ccontaining Calciprotein Particles Is Mediated by Scavenger Receptor-A. Circ. Res. 111 (5), 575–584. doi:10.1161/CIRCRESAHA.111.261479
Hinton, R. B., Alfieri, C. M., Witt, S. A., Glascock, B. J., Khoury, P. R., Benson, D. W., et al. (2008). Mouse Heart Valve Structure and Function: Echocardiographic and Morphometric Analyses from the Fetus through the Aged Adult. Am. J. Physiol. Heart Circ. Physiol. 294 (6), H2480–H2488. doi:10.1152/ajpheart.91431.2007
Hinton, R. B., and Yutzey, K. E. (2011). Heart Valve Structure and Function in Development and Disease. Annu. Rev. Physiol. 73, 29–46. doi:10.1146/annurev-physiol-012110-142145
Hinz, B., Celetta, G., Tomasek, J. J., Gabbiani, G., and Chaponnier, C. (2001). Alpha-smooth Muscle Actin Expression Upregulates Fibroblast Contractile Activity. Mol. Biol. Cell 12 (9), 2730–2741. doi:10.1091/mbc.12.9.2730
Hjortnaes, J., Camci-Unal, G., Hutcheson, J. D., Jung, S. M., Schoen, F. J., Kluin, J., et al. (2015). Directing Valvular Interstitial Cell Myofibroblast-like Differentiation in a Hybrid Hydrogel Platform. Adv. Healthc. Mater 4 (1), 121–130. doi:10.1002/adhm.201400029
Hjortnaes, J., Goettsch, C., Hutcheson, J. D., Camci-Unal, G., Lax, L., Scherer, K., et al. (2016). Simulation of Early Calcific Aortic Valve Disease in a 3D Platform: A Role for Myofibroblast Differentiation. J. Mol. Cell Cardiol. 94, 13–20. doi:10.1016/j.yjmcc.2016.03.004
Honda, S., Miyamoto, T., Watanabe, T., Narumi, T., Kadowaki, S., Honda, Y., et al. (2014). A Novel Mouse Model of Aortic Valve Stenosis Induced by Direct Wire Injury. Arterioscler. Thromb. Vasc. Biol. 34 (2), 270–278. doi:10.1161/ATVBAHA.113.302610
Hortells, L., Sosa, C., Millán, Á., and Sorribas, V. (2015). Critical Parameters of the In Vitro Method of Vascular Smooth Muscle Cell Calcification. PLoS One 10 (11), e0141751. doi:10.1371/journal.pone.0141751
Hui, M., and Tenenbaum, H. C. (1998). New Face of an Old Enzyme: Alkaline Phosphatase May Contribute to Human Tissue Aging by Inducing Tissue Hardening and Calcification. Anat. Rec. 253 (3), 91–94. doi:10.1002/(SICI)1097-0185(199806)253:3<91:AID-AR5>3.0.CO;2-H
Hulin, A., Hego, A., Lancellotti, P., and Oury, C. (2018). Advances in Pathophysiology of Calcific Aortic Valve Disease Propose Novel Molecular Therapeutic Targets. Front. Cardiovasc Med. 5, 21. doi:10.3389/fcvm.2018.00021
Hutcheson, J. D., Maldonado, N., and Aikawa, E. (2014). Small Entities with Large Impact: Microcalcifications and Atherosclerotic Plaque Vulnerability. Curr. Opin. Lipidol. 25 (5), 327–332. doi:10.1097/MOL.0000000000000105
Hutcheson, J. D., Ryzhova, L. M., Setola, V., and Merryman, W. D. (2012). 5-HT(2B) Antagonism Arrests Non-canonical TGF-Β1-Induced Valvular Myofibroblast Differentiation. J. Mol. Cell Cardiol. 53 (5), 707–714. doi:10.1016/j.yjmcc.2012.08.012
Ishikawa, S., Iwasaki, K., Komaki, M., and Ishikawa, I. (2004). Role of Ascorbic Acid in Periodontal Ligament Cell Differentiation. J. Periodontol. 75 (5), 709–716. doi:10.1902/jop.2004.75.5.709
Isoda, K., Matsuki, T., Kondo, H., Iwakura, Y., and Ohsuzu, F. (2010). Deficiency of Interleukin-1 Receptor Antagonist Induces Aortic Valve Disease in BALB/c Mice. Arterioscler. Thromb. Vasc. Biol. 30 (4), 708–715. doi:10.1161/ATVBAHA.109.201749
Jahnen-Dechent, W., Heiss, A., Schäfer, C., and Ketteler, M. (2011). Fetuin-A Regulation of Calcified Matrix Metabolism. Circ. Res. 108 (12), 1494–1509. doi:10.1161/CIRCRESAHA.110.234260
Jenkins, G. (2008). The Role of Proteases in Transforming Growth Factor-Beta Activation. Int. J. Biochem. Cell Biol. 40 (6-7), 1068–1078. doi:10.1016/j.biocel.2007.11.026
Jenkins, W. S., Vesey, A. T., Shah, A. S., Pawade, T. A., Chin, C. W., White, A. C., et al. (2015). Valvular (18)F-Fluoride and (18)F-Fluorodeoxyglucose Uptake Predict Disease Progression and Clinical Outcome in Patients with Aortic Stenosis. J. Am. Coll. Cardiol. 66 (10), 1200–1201. doi:10.1016/j.jacc.2015.06.1325
Jian, B., Narula, N., Li, Q. Y., Mohler, E. R., and Levy, R. J. (2003). Progression of Aortic Valve Stenosis: TGF-Beta1 Is Present in Calcified Aortic Valve Cusps and Promotes Aortic Valve Interstitial Cell Calcification via Apoptosis. Ann. Thorac. Surg. 75 (2), 457–465. doi:10.1016/s0003-4975(02)04312-6
Jian, B., Xu, J., Connolly, J., Savani, R. C., Narula, N., Liang, B., et al. (2002). Serotonin Mechanisms in Heart Valve Disease I: Serotonin-Induced Up-Regulation of Transforming Growth Factor-Beta1 via G-Protein Signal Transduction in Aortic Valve Interstitial Cells. Am. J. Pathol. 161 (6), 2111–2121. doi:10.1016/s0002-9440(10)64489-6
Johnson, C. L., and Merryman, W. D. (2021). Side-specific Valvular Endothelial-Interstitial Cell Mechano-Communication via Cadherin-11. J. Biomech. 119, 110253. doi:10.1016/j.jbiomech.2021.110253
Jono, S., Peinado, C., and Giachelli, C. M. (2000). Phosphorylation of Osteopontin Is Required for Inhibition of Vascular Smooth Muscle Cell Calcification. J. Biol. Chem. 275 (26), 20197–20203. doi:10.1074/jbc.M909174199
Kang, D. H., Park, S. J., Rim, J. H., Yun, S. C., Kim, D. H., Song, J. M., et al. (2010). Early Surgery versus Conventional Treatment in Asymptomatic Very Severe Aortic Stenosis. Circulation 121 (13), 1502–1509. doi:10.1161/CIRCULATIONAHA.109.909903
Kennedy, J. A., Hua, X., Mishra, K., Murphy, G. A., Rosenkranz, A. C., and Horowitz, J. D. (2009). Inhibition of Calcifying Nodule Formation in Cultured Porcine Aortic Valve Cells by Nitric Oxide Donors. Eur. J. Pharmacol. 602 (1), 28–35. doi:10.1016/j.ejphar.2008.11.029
Kheradvar, A., Zareian, R., Kawauchi, S., Goodwin, R. L., and Rugonyi, S. (2017). Animal Models for Heart Valve Research and Development. Drug Discov. Today Dis. Models 24, 55–62. doi:10.1016/j.ddmod.2018.04.001
Kim, K. M., Chang, S. H., Trump, B. F., and Spurgeon, H. (1986). Calcification in Aging Canine Aortic Valve. Scan Electron Microsc. (Pt 3), 1151–1156.
Köhler, W. M., Freitag-Wolf, S., Lambers, M., Lutz, M., Niemann, P. M., Petzina, R., et al. (2016). Preprocedural but Not Periprocedural High-Sensitive Troponin T Levels Predict Outcome in Patients Undergoing Transcatheter Aortic Valve Implantation. Cardiovasc Ther. 34 (6), 385–396. doi:10.1111/1755-5922.12208
Konduri, S., Xing, Y., Warnock, J. N., He, Z., and Yoganathan, A. P. (2005). Normal Physiological Conditions Maintain the Biological Characteristics of Porcine Aortic Heart Valves: an Ex Vivo Organ Culture Study. Ann. Biomed. Eng. 33 (9), 1158–1166. doi:10.1007/s10439-005-5506-4
Kostina, A., Shishkova, A., Ignatieva, E., Irtyuga, O., Bogdanova, M., Levchuk, K., et al. (2018). Different Notch Signaling in Cells from Calcified Bicuspid and Tricuspid Aortic Valves. J. Mol. Cell Cardiol. 114, 211–219. doi:10.1016/j.yjmcc.2017.11.009
Kreitals, N. M., and Watling, R. J. (2014). Multi-element Analysis Using Inductively Coupled Plasma Mass Spectrometry and Inductively Coupled Plasma Atomic Emission Spectroscopy for Provenancing of Animals at the Continental Scale. Forensic Sci. Int. 244, 116–121. doi:10.1016/j.forsciint.2014.08.016
Langenbach, F., and Handschel, J. (2013). Effects of Dexamethasone, Ascorbic Acid and β-glycerophosphate on the Osteogenic Differentiation of Stem Cells In Vitro. Stem Cell Res. Ther. 4 (5), 117. doi:10.1186/scrt328
Latif, N., Quillon, A., Sarathchandra, P., McCormack, A., Lozanoski, A., Yacoub, M. H., et al. (2015). Modulation of Human Valve Interstitial Cell Phenotype and Function Using a Fibroblast Growth Factor 2 Formulation. PLoS One 10 (6), e0127844. doi:10.1371/journal.pone.0127844
Latif, N., Sarathchandra, P., Chester, A. H., and Yacoub, M. H. (2015). Expression of Smooth Muscle Cell Markers and Co-activators in Calcified Aortic Valves. Eur. Heart J. 36 (21), 1335–1345. doi:10.1093/eurheartj/eht547
Latif, N., Sarathchandra, P., Thomas, P. S., Antoniw, J., Batten, P., Chester, A. H., et al. (2007). Characterization of Structural and Signaling Molecules by Human Valve Interstitial Cells and Comparison to Human Mesenchymal Stem Cells. J. Heart Valve Dis. 16 (1), 56–66.
Lee, J. S., Morrisett, J. D., and Tung, C. H. (2012). Detection of Hydroxyapatite in Calcified Cardiovascular Tissues. Atherosclerosis 224 (2), 340–347. doi:10.1016/j.atherosclerosis.2012.07.023
Lee, T. C., Zhao, Y. D., Courtman, D. W., and Stewart, D. J. (2000). Abnormal Aortic Valve Development in Mice Lacking Endothelial Nitric Oxide Synthase. Circulation 101 (20), 2345–2348. doi:10.1161/01.cir.101.20.2345
Leopold, J. A. (2012). Cellular Mechanisms of Aortic Valve Calcification. Circ. Cardiovasc Interv. 5 (4), 605–614. doi:10.1161/CIRCINTERVENTIONS.112.971028
Levy, R. J., Schoen, F. J., Levy, J. T., Nelson, A. C., Howard, S. L., and Oshry, L. J. (1983). Biologic Determinants of Dystrophic Calcification and Osteocalcin Deposition in Glutaraldehyde-Preserved Porcine Aortic Valve Leaflets Implanted Subcutaneously in Rats. Am. J. Pathol. 113 (2), 143–155.
Lindman, B. R., Dweck, M. R., Lancellotti, P., Généreux, P., Piérard, L. A., O'Gara, P. T., et al. (2020). Management of Asymptomatic Severe Aortic Stenosis: Evolving Concepts in Timing of Valve Replacement. JACC Cardiovasc Imaging 13 (2 Pt 1), 481–493. doi:10.1016/j.jcmg.2019.01.036
Liu, A. C., Joag, V. R., and Gotlieb, A. I. (2007). The Emerging Role of Valve Interstitial Cell Phenotypes in Regulating Heart Valve Pathobiology. Am. J. Pathol. 171 (5), 1407–1418. doi:10.2353/ajpath.2007.070251
Ljungberg, J., Janiec, M., Bergdahl, I. A., Holmgren, A., Hultdin, J., Johansson, B., et al. (2018). Proteomic Biomarkers for Incident Aortic Stenosis Requiring Valvular Replacement. Circulation 138 (6), 590–599. doi:10.1161/CIRCULATIONAHA.117.030414
Luo, G., Ducy, P., McKee, M. D., Pinero, G. J., Loyer, E., Behringer, R. R., et al. (1997). Spontaneous Calcification of Arteries and Cartilage in Mice Lacking Matrix GLA Protein. Nature 386 (6620), 78–81. doi:10.1038/386078a0
MacGrogan, D., Martínez-Poveda, B., Desvignes, J. P., Fernandez-Friera, L., Gomez, M. J., Gil Vilariño, E., et al. (2020). Identification of a Peripheral Blood Gene Signature Predicting Aortic Valve Calcification. Physiol. Genomics 52 (12), 563–574. doi:10.1152/physiolgenomics.00034.2020
Macri-Pellizzeri, L., De Melo, N., Ahmed, I., Grant, D., Scammell, B., and Sottile, V. (2018). Live Quantitative Monitoring of Mineral Deposition in Stem Cells Using Tetracycline Hydrochloride. Tissue Eng. Part C Methods 24 (3), 171–178. doi:10.1089/ten.TEC.2017.0400
Mako, W. J., and Vesely, I. (1997). In Vivo and In Vitro Models of Calcification in Porcine Aortic Valve Cusps. J. Heart Valve Dis. 6 (3), 316–323.
Mangialardo, S., Cottignoli, V., Cavarretta, E., Salvador, L., Postorino, P., and Maras, A. (2012). Pathological Biominerals: Raman and Infrared Studies of Bioapatite Deposits in Human Heart Valves. Appl. Spectrosc. 66 (10), 1121–1127. doi:10.1366/12-06606
Marechaux, S., Corseaux, D., Vincentelli, A., Richardson, M., Ung, A., Susen, S., et al. (2009). Identification of Tissue Factor in Experimental Aortic Valve Sclerosis. Cardiovasc Pathol. 18 (2), 67–76. doi:10.1016/j.carpath.2007.12.014
Martin-Rojas, T., Mourino-Alvarez, L., Alonso-Orgaz, S., Rosello-Lleti, E., Calvo, E., Lopez-Almodovar, L. F., et al. (2015). iTRAQ Proteomic Analysis of Extracellular Matrix Remodeling in Aortic Valve Disease. Sci. Rep. 5, 17290. doi:10.1038/srep17290
Martin-Rojas, T., Mourino-Alvarez, L., Gil-Dones, F., de la Cuesta, F., Rosello-Lleti, E., Laborde, C. M., et al. (2017). A Clinical Perspective on the Utility of Alpha 1 Antichymotrypsin for the Early Diagnosis of Calcific Aortic Stenosis. Clin. Proteomics 14, 12. doi:10.1186/s12014-017-9147-z
Mathieu, P., Boulanger, M. C., and Bouchareb, R. (2014). Molecular Biology of Calcific Aortic Valve Disease: towards New Pharmacological Therapies. Expert Rev. Cardiovasc Ther. 12 (7), 851–862. doi:10.1586/14779072.2014.923756
Matsumoto, K., Satoh, K., Maniwa, T., Araki, A., Maruyama, R., and Oda, T. (2012). Noticeable Decreased Expression of Tenascin-X in Calcific Aortic Valves. Connect. Tissue Res. 53 (6), 460–468. doi:10.3109/03008207.2012.702818
Merryman, W. D., Lukoff, H. D., Long, R. A., Engelmayr, G. C., Hopkins, R. A., and Sacks, M. S. (2007). Synergistic Effects of Cyclic Tension and Transforming Growth Factor-Beta1 on the Aortic Valve Myofibroblast. Cardiovasc Pathol. 16 (5), 268–276. doi:10.1016/j.carpath.2007.03.006
Messika-Zeitoun, D., Bielak, L. F., Peyser, P. A., Sheedy, P. F., Turner, S. T., Nkomo, V. T., et al. (2007). Aortic Valve Calcification: Determinants and Progression in the Population. Arterioscler. Thromb. Vasc. Biol. 27 (3), 642–648. doi:10.1161/01.ATV.0000255952.47980.c2
Miller, J. D., Weiss, R. M., Serrano, K. M., Brooks, R. M., Berry, C. J., Zimmerman, K., et al. (2009). Lowering Plasma Cholesterol Levels Halts Progression of Aortic Valve Disease in Mice. Circulation 119 (20), 2693–2701. doi:10.1161/CIRCULATIONAHA.108.834614
Miller, J. D., Weiss, R. M., Serrano, K. M., Castaneda, L. E., Brooks, R. M., Zimmerman, K., et al. (2010). Evidence for Active Regulation of Pro-osteogenic Signaling in Advanced Aortic Valve Disease. Arterioscler. Thromb. Vasc. Biol. 30 (12), 2482–2486. doi:10.1161/ATVBAHA.110.211029
Mittal, T. K., and Marcus, N. (2021). Imaging Diagnosis of Aortic Stenosis. Clin. Radiol. 76 (1), 3–14. doi:10.1016/j.crad.2020.04.008
Mohler, E. R., Gannon, F., Reynolds, C., Zimmerman, R., Keane, M. G., and Kaplan, F. S. (2001). Bone Formation and Inflammation in Cardiac Valves. Circulation 103 (11), 1522–1528. doi:10.1161/01.cir.103.11.1522
Monzack, E. L., Gu, X., and Masters, K. S. (2009). Efficacy of Simvastatin Treatment of Valvular Interstitial Cells Varies with the Extracellular Environment. Arterioscler. Thromb. Vasc. Biol. 29 (2), 246–253. doi:10.1161/ATVBAHA.108.179218
Monzack, E. L., and Masters, K. S. (2011). Can Valvular Interstitial Cells Become True Osteoblasts? A Side-By-Side Comparison. J. Heart Valve Dis. 20 (4), 449–463.
Mourino-Alvarez, L., Baldan-Martin, M., Gonzalez-Calero, L., Martinez-Laborde, C., Sastre-Oliva, T., Moreno-Luna, R., et al. (2016). Patients with Calcific Aortic Stenosis Exhibit Systemic Molecular Evidence of Ischemia, Enhanced Coagulation, Oxidative Stress and Impaired Cholesterol Transport. Int. J. Cardiol. 225, 99–106. doi:10.1016/j.ijcard.2016.09.089
Natorska, J., Kopytek, M., and Undas, A. (2021). Aortic Valvular Stenosis: Novel Therapeutic Strategies. Eur. J. Clin. Invest 51 (7), e13527. doi:10.1111/eci.13527
Nchimi, A., Dibato, J. E., Davin, L., Schoysman, L., Oury, C., and Lancellotti, P. (2018). Predicting Disease Progression and Mortality in Aortic Stenosis: A Systematic Review of Imaging Biomarkers and Meta-Analysis. Front. Cardiovasc Med. 5, 112. doi:10.3389/fcvm.2018.00112
Nguyen, V., Cimadevilla, C., Estellat, C., Codogno, I., Huart, V., Benessiano, J., et al. (2015). Haemodynamic and Anatomic Progression of Aortic Stenosis. Heart 101 (12), 943–947. doi:10.1136/heartjnl-2014-307154
Niepmann, S. T., Steffen, E., Zietzer, A., Adam, M., Nordsiek, J., Gyamfi-Poku, I., et al. (2019). Graded Murine Wire-Induced Aortic Valve Stenosis Model Mimics Human Functional and Morphological Disease Phenotype. Clin. Res. Cardiol. 108 (8), 847–856. doi:10.1007/s00392-019-01413-1
Nigam, V., and Srivastava, D. (2009). Notch1 Represses Osteogenic Pathways in Aortic Valve Cells. J. Mol. Cell Cardiol. 47 (6), 828–834. doi:10.1016/j.yjmcc.2009.08.008
Nkomo, V. T., Gardin, J. M., Skelton, T. N., Gottdiener, J. S., Scott, C. G., and Enriquez-Sarano, M. (2006). Burden of Valvular Heart Diseases: a Population-Based Study. Lancet 368 (9540), 1005–1011. doi:10.1016/S0140-6736(06)69208-8
Nus, M., MacGrogan, D., Martínez-Poveda, B., Benito, Y., Casanova, J. C., Fernández-Avilés, F., et al. (2011). Diet-induced Aortic Valve Disease in Mice Haploinsufficient for the Notch Pathway Effector RBPJK/CSL. Arterioscler. Thromb. Vasc. Biol. 31 (7), 1580–1588. doi:10.1161/ATVBAHA.111.227561
O'Neill, B. P., Guerrero, M., Thourani, V. H., Kodali, S., Heldman, A., Williams, M., et al. (2015). Prognostic Value of Serial B-type Natriuretic Peptide Measurement in Transcatheter Aortic Valve Replacement (From the PARTNER Trial). Am. J. Cardiol. 115 (9), 1265–1272. doi:10.1016/j.amjcard.2015.01.561
Olkowicz, M., Debski, J., Jablonska, P., Dadlez, M., and Smolenski, R. T. (2017). Application of a New Procedure for Liquid Chromatography/mass Spectrometry Profiling of Plasma Amino Acid-Related Metabolites and Untargeted Shotgun Proteomics to Identify Mechanisms and Biomarkers of Calcific Aortic Stenosis. J. Chromatogr. A 1517, 66–78. doi:10.1016/j.chroma.2017.08.024
Olsson, M., Rosenqvist, M., and Nilsson, J. (1994). Expression of HLA-DR Antigen and Smooth Muscle Cell Differentiation Markers by Valvular Fibroblasts in Degenerative Aortic Stenosis. J. Am. Coll. Cardiol. 24 (7), 1664–1671. doi:10.1016/0735-1097(94)90172-4
Orzechowska, S., Wróbel, A., Goncerz, G., Podolec, P., and Rokita, E. (2014). Physicochemical and Micro-tomographic Characterization of Inorganic Deposits Associated with Aortic Stenosis. J. Heart Valve Dis. 23 (1), 40–47.
Osman, L., Chester, A. H., Amrani, M., Yacoub, M. H., and Smolenski, R. T. (2006). A Novel Role of Extracellular Nucleotides in Valve Calcification: a Potential Target for Atorvastatin. Circulation 114 (1 Suppl. l), I566–I572. doi:10.1161/CIRCULATIONAHA.105.001214
Osman, L., Chester, A. H., Sarathchandra, P., Latif, N., Meng, W., Taylor, P. M., et al. (2007). A Novel Role of the Sympatho-Adrenergic System in Regulating Valve Calcification. Circulation 116 (11 Suppl. l), I282–I287. doi:10.1161/CIRCULATIONAHA.106.681072
Osman, L., Yacoub, M. H., Latif, N., Amrani, M., and Chester, A. H. (2006). Role of Human Valve Interstitial Cells in Valve Calcification and Their Response to Atorvastatin. Circulation 114 (1 Suppl. l), I547–I552. doi:10.1161/CIRCULATIONAHA.105.001115
Osnabrugge, R. L., Mylotte, D., Head, S. J., Van Mieghem, N. M., Nkomo, V. T., LeReun, C. M., et al. (2013). Aortic Stenosis in the Elderly: Disease Prevalence and Number of Candidates for Transcatheter Aortic Valve Replacement: a Meta-Analysis and Modeling Study. J. Am. Coll. Cardiol. 62 (11), 1002–1012. doi:10.1016/j.jacc.2013.05.015
Oury, C., Côté, N., and Clavel, M. A. (2020). Biomarkers Associated with Aortic Stenosis and Structural Bioprosthesis Dysfunction. Cardiol. Clin. 38 (1), 47–54. doi:10.1016/j.ccl.2019.09.005
Oury, C., Servais, L., Bouznad, N., Hego, A., Nchimi, A., and Lancellotti, P. (2016). MicroRNAs in Valvular Heart Diseases: Potential Role as Markers and Actors of Valvular and Cardiac Remodeling. Int. J. Mol. Sci. 17 (7). doi:10.3390/ijms17071120
Pai, R. G., Kapoor, N., Bansal, R. C., and Varadarajan, P. (2006). Malignant Natural History of Asymptomatic Severe Aortic Stenosis: Benefit of Aortic Valve Replacement. Ann. Thorac. Surg. 82 (6), 2116–2122. doi:10.1016/j.athoracsur.2006.07.043
Paraskova, J. V., Jørgensen, C., Reitzel, K., Pettersson, J., Rydin, E., and Sjöberg, P. J. (2015). Speciation of Inositol Phosphates in Lake Sediments by Ion-Exchange Chromatography Coupled with Mass Spectrometry, Inductively Coupled Plasma Atomic Emission Spectroscopy, and 31P NMR Spectroscopy. Anal. Chem. 87 (5), 2672–2677. doi:10.1021/ac5033484
Parizi, M., Howard, E. W., and Tomasek, J. J. (2000). Regulation of LPA-Promoted Myofibroblast Contraction: Role of Rho, Myosin Light Chain Kinase, and Myosin Light Chain Phosphatase. Exp. Cell Res. 254 (2), 210–220. doi:10.1006/excr.1999.4754
Parra-Izquierdo, I., Sánchez-Bayuela, T., Castaños-Mollor, I., López, J., Gómez, C., San Román, J. A., et al. (2021). Clinically Used JAK Inhibitor Blunts dsRNA-Induced Inflammation and Calcification in Aortic Valve Interstitial Cells. FEBS J. 288 (22), 6528–6542. doi:10.1111/febs.16026
Patel, N., and Kumbhani, D. J. (2018). Clinical Implications of Serum Biomarkers of Cardiac Stress in Aortic Stenosis. Curr. Heart Fail Rep. 15 (5), 281–286. doi:10.1007/s11897-018-0403-y
Pawade, T., Sheth, T., Guzzetti, E., Dweck, M. R., and Clavel, M. A. (2019). Why and How to Measure Aortic Valve Calcification in Patients with Aortic Stenosis. JACC Cardiovasc Imaging 12 (9), 1835–1848. doi:10.1016/j.jcmg.2019.01.045
Pawade, T. A., Newby, D. E., and Dweck, M. R. (2015). Calcification in Aortic Stenosis: The Skeleton Key. J. Am. Coll. Cardiol. 66 (5), 561–577. doi:10.1016/j.jacc.2015.05.066
Pho, M., Lee, W., Watt, D. R., Laschinger, C., Simmons, C. A., and McCulloch, C. A. (2008). Cofilin Is a Marker of Myofibroblast Differentiation in Cells from Porcine Aortic Cardiac Valves. Am. J. Physiol. Heart Circ. Physiol. 294 (4), H1767–H1778. doi:10.1152/ajpheart.01305.2007
Plazyo, O., Liu, R., Moazzem Hossain, M., and Jin, J. P. (2018). Deletion of Calponin 2 Attenuates the Development of Calcific Aortic Valve Disease in ApoE-/- Mice. J. Mol. Cell Cardiol. 121, 233–241. doi:10.1016/j.yjmcc.2018.07.249
Poggio, P., Branchetti, E., Grau, J. B., Lai, E. K., Gorman, R. C., Gorman, J. H., et al. (2014). Osteopontin-CD44v6 Interaction Mediates Calcium Deposition via Phospho-Akt in Valve Interstitial Cells from Patients with Noncalcified Aortic Valve Sclerosis. Arterioscler. Thromb. Vasc. Biol. 34 (9), 2086–2094. doi:10.1161/ATVBAHA.113.303017
Porras, A. M., Shanmuganayagam, D., Meudt, J. J., Krueger, C. G., Hacker, T. A., Rahko, P. S., et al. (2015). Development of Aortic Valve Disease in Familial Hypercholesterolemic Swine: Implications for Elucidating Disease Etiology. J. Am. Heart Assoc. 4 (10), e002254. doi:10.1161/JAHA.115.002254
Porras, A. M., van Engeland, N. C., Marchbanks, E., McCormack, A., Bouten, C. V., Yacoub, M. H., et al. (2017). Robust Generation of Quiescent Porcine Valvular Interstitial Cell Cultures. J. Am. Heart Assoc. 6 (3). doi:10.1161/JAHA.116.005041
Price, P. A., Faus, S. A., and Williamson, M. K. (1998). Warfarin Causes Rapid Calcification of the Elastic Lamellae in Rat Arteries and Heart Valves. Arterioscler. Thromb. Vasc. Biol. 18 (9), 1400–1407. doi:10.1161/01.atv.18.9.1400
Price, P. A., and Lim, J. E. (2003). The Inhibition of Calcium Phosphate Precipitation by Fetuin Is Accompanied by the Formation of a Fetuin-Mineral Complex. J. Biol. Chem. 278 (24), 22144–22152. doi:10.1074/jbc.M300744200
Prieto, R. M., Gomila, I., Söhnel, O., Costa-Bauza, A., Bonnin, O., and Grases, F. (2011). Study on the Structure and Composition of Aortic Valve Calcific Deposits. Etiological Aspects. Jbpc 02, 19–25. doi:10.4236/jbpc.2011.21003
Prins, H. J., Braat, A. K., Gawlitta, D., Dhert, W. J., Egan, D. A., Tijssen-Slump, E., et al. (2014). In Vitro induction of Alkaline Phosphatase Levels Predicts In Vivo Bone Forming Capacity of Human Bone Marrow Stromal Cells. Stem Cell Res. 12 (2), 428–440. doi:10.1016/j.scr.2013.12.001
Puchtler, H., and Meloan, S. N. (1978). Demonstration of phosphates in calcium deposits: a modification of von Kossa's reaction. Histochemistry 56 (3-4), 177–185. doi:10.1007/BF00495978
Quinlan, A. M., and Billiar, K. L. (2012). Investigating the Role of Substrate Stiffness in the Persistence of Valvular Interstitial Cell Activation. J. Biomed. Mater Res. A 100 (9), 2474–2482. doi:10.1002/jbm.a.34162
Rajamannan, N. M., Evans, F. J., Aikawa, E., Grande-Allen, K. J., Demer, L. L., Heistad, D. D., et al. (2011). Calcific Aortic Valve Disease: Not Simply a Degenerative Process: A Review and Agenda for Research from the National Heart and Lung and Blood Institute Aortic Stenosis Working Group. Executive Summary: Calcific Aortic Valve Disease-2011 Update. Circulation 124 (16), 1783–1791. doi:10.1161/CIRCULATIONAHA.110.006767
Rajamannan, N. M. (2014). Molecular Biology of Valvular Heart Disease2014. Springer London, 1–150. doi:10.1007/978-1-4471-6350-3
Rathan, S., Yoganathan, A. P., and O'Neill, C. W. (2014). The Role of Inorganic Pyrophosphate in Aortic Valve Calcification. J. Heart Valve Dis. 23 (4), 387–394.
Rattazzi, M., Donato, M., Bertacco, E., Millioni, R., Franchin, C., Mortarino, C., et al. (2020). l-Arginine Prevents Inflammatory and Pro-calcific Differentiation of Interstitial Aortic Valve Cells. Atherosclerosis 298, 27–35. doi:10.1016/j.atherosclerosis.2020.02.024
Rattazzi, M., and Pauletto, P. (2015). Valvular Endothelial Cells: Guardians or Destroyers of Aortic Valve Integrity? Atherosclerosis 242 (2), 396–398. doi:10.1016/j.atherosclerosis.2015.07.034
Ravi, M., Paramesh, V., Kaviya, S. R., Anuradha, E., and Solomon, F. D. (2015). 3D Cell Culture Systems: Advantages and Applications. J. Cell Physiol. 230 (1), 16–26. doi:10.1002/jcp.24683
Redfors, B., Furer, A., Lindman, B. R., Burkhoff, D., Marquis-Gravel, G., Francese, D. P., et al. (2017). Biomarkers in Aortic Stenosis: A Systematic Review. Struct. Heart 1 (1-2), 18–30. doi:10.1080/24748706.2017.1329959
Renato, M., Bertacco, E., Franchin, C., Arrigoni, G., and Rattazzi, M. (2013). Proteomic Analysis of Interstitial Aortic Valve Cells Acquiring a Pro-calcific Profile. Methods Mol. Biol. 1005, 95–107. doi:10.1007/978-1-62703-386-2_8
Richards, J., El-Hamamsy, I., Chen, S., Sarang, Z., Sarathchandra, P., Yacoub, M. H., et al. (2013). Side-specific Endothelial-dependent Regulation of Aortic Valve Calcification: Interplay of Hemodynamics and Nitric Oxide Signaling. Am. J. Pathol. 182 (5), 1922–1931. doi:10.1016/j.ajpath.2013.01.037
Richards, J. M., Kunitake, J. A. M. R., Hunt, H. B., Wnorowski, A. N., Lin, D. W., Boskey, A. L., et al. (2018). Crystallinity of Hydroxyapatite Drives Myofibroblastic Activation and Calcification in Aortic Valves. Acta Biomater. 71, 24–36. doi:10.1016/j.actbio.2018.02.024
Rodriguez, K. J., and Masters, K. S. (2009). Regulation of Valvular Interstitial Cell Calcification by Components of the Extracellular Matrix. J. Biomed. Mater Res. A 90 (4), 1043–1053. doi:10.1002/jbm.a.32187
Rogers, M. A., Maldonado, N., Hutcheson, J. D., Goettsch, C., Goto, S., Yamada, I., et al. (2017). Dynamin-Related Protein 1 Inhibition Attenuates Cardiovascular Calcification in the Presence of Oxidative Stress. Circ. Res. 121 (3), 220–233. doi:10.1161/CIRCRESAHA.116.310293
Rojulpote, C., Borja, A. J., Zhang, V., Aly, M., Koa, B., Seraj, S. M., et al. (2020). Role of 18F-NaF-PET in Assessing Aortic Valve Calcification with Age. Am. J. Nucl. Med. Mol. Imaging 10 (1), 47–56.
Roosens, B., Bala, G., Droogmans, S., Van Camp, G., Breyne, J., and Cosyns, B. (2013). Animal Models of Organic Heart Valve Disease. Int. J. Cardiol. 165 (3), 398–409. doi:10.1016/j.ijcard.2012.03.065
Roosens, B., Bala, G., Gillis, K., Remory, I., Droogmans, S., Somja, J., et al. (2013). Echocardiographic Integrated Backscatter for Detecting Progression and Regression of Aortic Valve Calcifications in Rats. Cardiovasc Ultrasound 11, 4. doi:10.1186/1476-7120-11-4
Roosens, B., Droogmans, S., Hostens, J., Somja, J., Delvenne, E., Hernot, S., et al. (2011). Integrated Backscatter for the In Vivo Quantification of Supraphysiological Vitamin D(3)-induced Cardiovascular Calcifications in Rats. Cardiovasc Toxicol. 11 (3), 244–252. doi:10.1007/s12012-011-9118-y
Rutkovskiy, A., Malashicheva, A., Sullivan, G., Bogdanova, M., Kostareva, A., Stensløkken, K. O., et al. (2017). Valve Interstitial Cells: The Key to Understanding the Pathophysiology of Heart Valve Calcification. J. Am. Heart Assoc. 6 (9). doi:10.1161/JAHA.117.006339
Sainger, R., Grau, J. B., Branchetti, E., Poggio, P., Lai, E., Koka, E., et al. (2013). Comparison of Transesophageal Echocardiographic Analysis and Circulating Biomarker Expression Profile in Calcific Aortic Valve Disease. J. Heart Valve Dis. 22 (2), 156–165.
Salemi, A., and Worku, B. M. (2017). Standard Imaging Techniques in Transcatheter Aortic Valve Replacement. J. Thorac. Dis. 9 (Suppl. 4), S289–s98. doi:10.21037/jtd.2017.03.114
Satoh, K., Yamada, K., Maniwa, T., Oda, T., and Matsumoto, K. (2015). Monitoring of Serial Presurgical and Postsurgical Changes in the Serum Proteome in a Series of Patients with Calcific Aortic Stenosis. Dis. Markers 2015, 694120. doi:10.1155/2015/694120
Sauren, A. A., van Hout, M. C., van Steenhoven, A. A., Veldpaus, F. E., and Janssen, J. D. (1983). The Mechanical Properties of Porcine Aortic Valve Tissues. J. Biomech. 16 (5), 327–337. doi:10.1016/0021-9290(83)90016-7
Schlotter, F., Halu, A., Goto, S., Blaser, M. C., Body, S. C., Lee, L. H., et al. (2018). Spatiotemporal Multi-Omics Mapping Generates a Molecular Atlas of the Aortic Valve and Reveals Networks Driving Disease. Circulation 138 (4), 377–393. doi:10.1161/CIRCULATIONAHA.117.032291
Schoen, F. J., Levy, R. J., Nelson, A. C., Bernhard, W. F., Nashef, A., and Hawley, M. (1985). Onset and Progression of Experimental Bioprosthetic Heart Valve Calcification. Lab. Invest 52 (5), 523–532.
Shen, M., Tastet, L., Capoulade, R., Larose, É., Bédard, É., Arsenault, M., et al. (2017). Effect of Age and Aortic Valve Anatomy on Calcification and Haemodynamic Severity of Aortic Stenosis. Heart 103 (1), 32–39. doi:10.1136/heartjnl-2016-309665
Shuvy, M., Abedat, S., Beeri, R., Danenberg, H. D., Planer, D., Ben-Dov, I. Z., et al. (2008). Uraemic Hyperparathyroidism Causes a Reversible Inflammatory Process of Aortic Valve Calcification in Rats. Cardiovasc Res. 79 (3), 492–499. doi:10.1093/cvr/cvn088
Sider, K. L., Blaser, M. C., and Simmons, C. A. (2011). Animal Models of Calcific Aortic Valve Disease. Int. J. Inflam. 2011, 364310. doi:10.4061/2011/364310
Sider, K. L., Zhu, C., Kwong, A. V., Mirzaei, Z., de Langé, C. F., and Simmons, C. A. (2014). Evaluation of a Porcine Model of Early Aortic Valve Sclerosis. Cardiovasc Pathol. 23 (5), 289–297. doi:10.1016/j.carpath.2014.05.004
Sim, A. M., Rashdan, N. A., Cui, L., Moss, A. J., Nudelman, F., Dweck, M. R., et al. (2018). A Novel Fluorescein-Bisphosphonate Based Diagnostic Tool for the Detection of Hydroxyapatite in Both Cell and Tissue Models. Sci. Rep. 8 (1), 17360. doi:10.1038/s41598-018-35454-9
Skold, B. H., Getty, R., and Ramsey, F. K. (1966). Spontaneous Atherosclerosis in the Arterial System of Aging Swine. Am. J. Vet. Res. 27 (116), 257–273.
Small, A., Kiss, D., Giri, J., Anwaruddin, S., Siddiqi, H., Guerraty, M., et al. (2017). Biomarkers of Calcific Aortic Valve Disease. Arterioscler. Thromb. Vasc. Biol. 37 (4), 623–632. doi:10.1161/ATVBAHA.116.308615
Smith, E. R., Cai, M. M., McMahon, L. P., Pedagogos, E., Toussaint, N. D., Brumby, C., et al. (2013). Serum Fetuin-A Concentration and Fetuin-A-Containing Calciprotein Particles in Patients with Chronic Inflammatory Disease and Renal Failure. Nephrol. Carlt. 18 (3), 215–221. doi:10.1111/nep.12021
Sneddon, J., and Vincent, M. D. (2008). ICP-OES and ICP-MS for the Determination of Metals: Application to Oysters. Anal. Lett. 41 (8), 1291–1303. doi:10.1080/00032710802013991
Steadman, C. D., Ray, S., Ng, L. L., and McCann, G. P. (2010). Natriuretic Peptides in Common Valvular Heart Disease. J. Am. Coll. Cardiol. 55 (19), 2034–2048. doi:10.1016/j.jacc.2010.02.021
Stewart, B. F., Siscovick, D., Lind, B. K., Gardin, J. M., Gottdiener, J. S., Smith, V. E., et al. (1997). Clinical Factors Associated with Calcific Aortic Valve Disease. Cardiovascular Health Study. J. Am. Coll. Cardiol. 29 (3), 630–634. doi:10.1016/s0735-1097(96)00563-3
Suzuki, H., Chikada, M., Yokoyama, M. K., Kurokawa, M. S., Ando, T., Furukawa, H., et al. (2016). Aberrant Glycosylation of Lumican in Aortic Valve Stenosis Revealed by Proteomic Analysis. Int. Heart J. 57 (1), 104–111. doi:10.1536/ihj.15-252
Takkenberg, J. J., Rajamannan, N. M., Rosenhek, R., Kumar, A. S., Carapetis, J. R., and Yacoub, M. H. (2008). The Need for a Global Perspective on Heart Valve Disease Epidemiology. The SHVD Working Group on Epidemiology of Heart Valve Disease Founding Statement. J. Heart Valve Dis. 17 (1), 135–139.
Tao, G., Kotick, J. D., and Lincoln, J. (2012). Heart Valve Development, Maintenance, and Disease: the Role of Endothelial Cells. Curr. Top. Dev. Biol. 100, 203–232. doi:10.1016/B978-0-12-387786-4.00006-3
Tastet, L., Enriquez-Sarano, M., Capoulade, R., Malouf, J., Araoz, P. A., Shen, M., et al. (2017). Impact of Aortic Valve Calcification and Sex on Hemodynamic Progression and Clinical Outcomes in AS. J. Am. Coll. Cardiol. 69 (16), 2096–2098. doi:10.1016/j.jacc.2017.02.037
Taylor, P. M., Allen, S. P., and Yacoub, M. H. (2000). Phenotypic and Functional Characterization of Interstitial Cells from Human Heart Valves, Pericardium and Skin. J. Heart Valve Dis. 9 (1), 150–158.
Ternacle, J., and Clavel, M. A. (2020). Assessment of Aortic Stenosis Severity: A Multimodality Approach. Cardiol. Clin. 38 (1), 13–22. doi:10.1016/j.ccl.2019.09.004
Theodoridis, K., Tudorache, I., Cebotari, S., Calistru, A., Meyer, T., Sarikouch, S., et al. (2017). * Six-Year-Old Sheep as a Clinically Relevant Large Animal Model for Aortic Valve Replacement Using Tissue-Engineered Grafts Based on Decellularized Allogenic Matrix. Tissue Eng. Part C Methods 23 (12), 953–963. doi:10.1089/ten.tec.2017.0163
Tkatchenko, T. V., Moreno-Rodriguez, R. A., Conway, S. J., Molkentin, J. D., Markwald, R. R., and Tkatchenko, A. V. (2009). Lack of Periostin Leads to Suppression of Notch1 Signaling and Calcific Aortic Valve Disease. Physiol. Genomics 39 (3), 160–168. doi:10.1152/physiolgenomics.00078.2009
Tomasek, J. J., Gabbiani, G., Hinz, B., Chaponnier, C., and Brown, R. A. (2002). Myofibroblasts and Mechano-Regulation of Connective Tissue Remodelling. Nat. Rev. Mol. Cell Biol. 3 (5), 349–363. doi:10.1038/nrm809
Toutouzas, K., Stathogiannis, K., Latsios, G., Synetos, A., Drakopoulou, M., Penesopoulou, V., et al. (2019). Biomarkers in Aortic Valve Stenosis and Their Clinical Significance in Transcatheter Aortic Valve Implantation. Curr. Med. Chem. 26 (1), 864–827. doi:10.2174/0929867324666170727110241
Tsang, H. G., Rashdan, N. A., Whitelaw, C. B., Corcoran, B. M., Summers, K. M., and MacRae, V. E. (2016). Large Animal Models of Cardiovascular Disease. Cell Biochem. Funct. 34 (3), 113–132. doi:10.1002/cbf.3173
Tzolos, E., Andrews, J. P., and Dweck, M. R. (2020). Aortic Valve Stenosis-Multimodality Assessment with PET/CT and PET/MRI. Br. J. Radiol. 93 (1113), 20190688. doi:10.1259/bjr.20190688
Ueland, T., Aukrust, P., Dahl, C. P., Husebye, T., Solberg, O. G., Tønnessen, T., et al. (2011). Osteoprotegerin Levels Predict Mortality in Patients with Symptomatic Aortic Stenosis. J. Intern Med. 270 (5), 452–460. doi:10.1111/j.1365-2796.2011.02393.x
Ueland, T., Gullestad, L., Dahl, C. P., Aukrust, P., Aakhus, S., Solberg, O. G., et al. (2010). Undercarboxylated Matrix Gla Protein Is Associated with Indices of Heart Failure and Mortality in Symptomatic Aortic Stenosis. J. Intern Med. 268 (5), 483–492. doi:10.1111/j.1365-2796.2010.02264.x
Van Belle, E., Rauch, A., Vincent, F., Robin, E., Kibler, M., Labreuche, J., et al. (2016). Von Willebrand Factor Multimers during Transcatheter Aortic-Valve Replacement. N. Engl. J. Med. 375 (4), 335–344. doi:10.1056/NEJMoa1505643
Van Belle, E., Vincent, F., Rauch, A., Casari, C., Jeanpierre, E., Loobuyck, V., et al. (2019). von Willebrand Factor and Management of Heart Valve Disease: JACC Review Topic of the Week. J. Am. Coll. Cardiol. 73 (9), 1078–1088. doi:10.1016/j.jacc.2018.12.045
van der Valk, D. C., van der Ven, C. F. T., Blaser, M. C., Grolman, J. M., Wu, P. J., Fenton, O. S., et al. (2018). Engineering a 3D-Bioprinted Model of Human Heart Valve Disease Using Nanoindentation-Based Biomechanics. Nanomater. (Basel) 8 (5). doi:10.3390/nano8050296
Vancheri, F., Longo, G., Vancheri, S., Danial, J. S. H., and Henein, M. Y. (2019). Coronary Artery Microcalcification: Imaging and Clinical Implications. Diagn. (Basel) 9 (4). doi:10.3390/diagnostics9040125
Varshney, R., Murphy, B., Woolington, S., Ghafoory, S., Chen, S., Robison, T., et al. (2019). Inactivation of Platelet-Derived TGF-Β1 Attenuates Aortic Stenosis Progression in a Robust Murine Model. Blood Adv. 3 (5), 777–788. doi:10.1182/bloodadvances.2018025817
Venardos, N., Gergen, A. K., Jarrett, M., Weyant, M. J., Reece, T. B., Meng, X., et al. (2022). Warfarin Induces Calcification of the Aortic Valve through Extracellular Signal-Regulated Kinase 1/2 and β-catenin Signaling. Ann. Thorac. Surg. 133 (9), 824–835. doi:10.1016/j.athoracsur.2021.03.099
Walker, G. A., Masters, K. S., Shah, D. N., Anseth, K. S., and Leinwand, L. A. (2004). Valvular Myofibroblast Activation by Transforming Growth Factor-Beta: Implications for Pathological Extracellular Matrix Remodeling in Heart Valve Disease. Circ. Res. 95 (3), 253–260. doi:10.1161/01.RES.0000136520.07995.aa
Wallby, L., Steffensen, T., Jonasson, L., and Broqvist, M. (2013). Inflammatory Characteristics of Stenotic Aortic Valves: A Comparison between Rheumatic and Nonrheumatic Aortic Stenosis. Cardiol. Res. Pract. 2013, 895215. doi:10.1155/2013/895215
Wang, C., Xia, Y., Qu, L., Liu, Y., Liu, X., and Xu, K. (2021). Cardamonin Inhibits Osteogenic Differentiation of Human Valve Interstitial Cells and Ameliorates Aortic Valve Calcification via Interfering in the NF-Κb/nlrp3 Inflammasome Pathway. Food Funct. 12 (23), 11808–11818. doi:10.1039/d1fo00813g
Weber, M., Arnold, R., Rau, M., Brandt, R., Berkovitsch, A., Mitrovic, V., et al. (2004). Relation of N-Terminal Pro-B-type Natriuretic Peptide to Severity of Valvular Aortic Stenosis. Am. J. Cardiol. 94 (6), 740–745. doi:10.1016/j.amjcard.2004.05.055
Weisell, J., Ohukainen, P., Näpänkangas, J., Ohlmeier, S., Bergmann, U., Peltonen, T., et al. (2019). Heat Shock Protein 90 Is Downregulated in Calcific Aortic Valve Disease. BMC Cardiovasc Disord. 19 (1), 306. doi:10.1186/s12872-019-01294-2
Weiss, R. M., Chu, Y., Brooks, R. M., Lund, D. D., Cheng, J., Zimmerman, K. A., et al. (2018). Discovery of an Experimental Model of Unicuspid Aortic Valve. J. Am. Heart Assoc. 7 (13). doi:10.1161/JAHA.117.006908
Weiss, R. M., Ohashi, M., Miller, J. D., Young, S. G., and Heistad, D. D. (2006). Calcific Aortic Valve Stenosis in Old Hypercholesterolemic Mice. Circulation 114 (19), 2065–2069. doi:10.1161/CIRCULATIONAHA.106.634139
Wilschefski, S. C., and Baxter, M. R. (2019). Inductively Coupled Plasma Mass Spectrometry: Introduction to Analytical Aspects. Clin. Biochem. Rev. 40 (3), 115–133. doi:10.33176/AACB-19-00024
Wipff, P. J., and Hinz, B. (2008). Integrins and the Activation of Latent Transforming Growth Factor Beta1 - an Intimate Relationship. Eur. J. Cell Biol. 87 (8-9), 601–615. doi:10.1016/j.ejcb.2008.01.012
Wyss, K., Yip, C. Y., Mirzaei, Z., Jin, X., Chen, J. H., and Simmons, C. A. (2012). The Elastic Properties of Valve Interstitial Cells Undergoing Pathological Differentiation. J. Biomech. 45 (5), 882–887. doi:10.1016/j.jbiomech.2011.11.030
Xing, Y., He, Z., Warnock, J. N., Hilbert, S. L., and Yoganathan, A. P. (2004). Effects of Constant Static Pressure on the Biological Properties of Porcine Aortic Valve Leaflets. Ann. Biomed. Eng. 32 (4), 555–562. doi:10.1023/b:abme.0000019175.12013.8f
Yip, C. Y., Chen, J. H., Zhao, R., and Simmons, C. A. (2009). Calcification by Valve Interstitial Cells Is Regulated by the Stiffness of the Extracellular Matrix. Arterioscler. Thromb. Vasc. Biol. 29 (6), 936–942. doi:10.1161/ATVBAHA.108.182394
Yousry, M., Rickenlund, A., Petrini, J., Gustavsson, T., Prahl, U., Liska, J., et al. (2012). Real-time Imaging Required for Optimal Echocardiographic Assessment of Aortic Valve Calcification. Clin. Physiol. Funct. Imaging 32 (6), 470–475. doi:10.1111/j.1475-097X.2012.01153.x
Yousry, M., Rickenlund, A., Petrini, J., Jenner, J., Liska, J., Eriksson, P., et al. (2015). Aortic Valve Type and Calcification as Assessed by Transthoracic and Transoesophageal Echocardiography. Clin. Physiol. Funct. Imaging 35 (4), 306–313. doi:10.1111/cpf.12166
Yperman, J., De Visscher, G., Holvoet, P., and Flameng, W. (2004). Molecular and Functional Characterization of Ovine Cardiac Valve-Derived Interstitial Cells in Primary Isolates and Cultures. Tissue Eng. 10 (9-10), 1368–1375. doi:10.1089/ten.2004.10.1368
Yu, B., Khan, K., Hamid, Q., Mardini, A., Siddique, A., Aguilar-Gonzalez, L. P., et al. (2018). Pathological Significance of Lipoprotein(a) in Aortic Valve Stenosis. Atherosclerosis 272, 168–174. doi:10.1016/j.atherosclerosis.2018.03.025
Zabirnyk, A., Perez, M. D. M., Blasco, M., Stensløkken, K. O., Ferrer, M. D., Salcedo, C., et al. (2020). A Novel Ex Vivo Model of Aortic Valve Calcification. A Preliminary Report. Front. Pharmacol. 11, 568764. doi:10.3389/fphar.2020.568764
Zhang, X. W., Zhang, B. Y., Wang, S. W., Gong, D. J., Han, L., Xu, Z. Y., et al. (2014). Twist-related Protein 1 Negatively Regulated Osteoblastic Transdifferentiation of Human Aortic Valve Interstitial Cells by Directly Inhibiting Runt-Related Transcription Factor 2. J. Thorac. Cardiovasc Surg. 148 (4), 1700–e1. doi:10.1016/j.jtcvs.2014.02.084
Keywords: aortic valve, interstitial cells, endothelial cells, calcification, animal models, calcified aortic valve disease, imaging
Citation: Bogdanova M, Zabirnyk A, Malashicheva A, Semenova D, Kvitting J-PE, Kaljusto M-L, Perez MdM, Kostareva A, Stensløkken K-O, Sullivan GJ, Rutkovskiy A and Vaage J (2022) Models and Techniques to Study Aortic Valve Calcification in Vitro, ex Vivo and in Vivo. An Overview. Front. Pharmacol. 13:835825. doi: 10.3389/fphar.2022.835825
Received: 14 December 2021; Accepted: 29 April 2022;
Published: 02 June 2022.
Edited by:
Paolo Poggio, Monzino Cardiology Center (IRCCS), ItalyReviewed by:
Najma Latif, The Magdi Yacoub Institute, United KingdomVeronika Myasoedova, Monzino Cardiology Center (IRCCS), Italy
Copyright © 2022 Bogdanova, Zabirnyk, Malashicheva, Semenova, Kvitting, Kaljusto, Perez, Kostareva, Stensløkken, Sullivan, Rutkovskiy and Vaage. This is an open-access article distributed under the terms of the Creative Commons Attribution License (CC BY). The use, distribution or reproduction in other forums is permitted, provided the original author(s) and the copyright owner(s) are credited and that the original publication in this journal is cited, in accordance with accepted academic practice. No use, distribution or reproduction is permitted which does not comply with these terms.
*Correspondence: Arsenii Zabirnyk, YXJzZW5paS56YWJpcm55a0BtZWRpc2luLnVpby5ubw==
†These authors have contributed equally to this work and share first authorship
‡These authors have contributed equally to this work