- Institute of Digestive Diseases, Longhua Hospital, Shanghai University of Traditional Chinese Medicine, Shanghai, China
Huazhi-Rougan (HZRG) formula is a Traditional Chinese medicine prescription, and has been widely used to treat non-alcoholic fatty liver disease (NAFLD) and its progressive form non-alcoholic steatohepatitis (NASH). However, the anti-NASH effects and the underlying mechanisms of HZRG have not yet been characterized. Here we showed that 4-week HZRG treatment alleviated methionine-choline-deficiency (MCD) diet-induced NASH in C57BL/6J mice, as evidenced by the improvement of hepatic steatosis and inflammation, as well as the decrease of serum levels of alanine and aspartate transaminases. Fecal 16S rDNA sequencing indicated that HZRG reduced the enrichment of pathogenic bacteria and increased the abundance of bacteria gena that are involved in bile acid (BA) conversation. The alteration of fecal and serum BA profile suggested that HZRG enhanced fecal BA excretion, and reduced the reabsorption of toxic secondary BA species (LCA, DCA, HCA). We further analyzed the BA receptors and transporters, and found that HZRG inhibited the expression of ileal bile acid transporter, and organic solute transporter subunit β, and increased the expression of intestinal tight junction proteins (ZO-1, Occludin, Claudin-2). The modulation of gut dysbiosis and BA profile, as well as the improvement of the intestinal environment, may contribute to the decrease of the p-65 subunit of NF-κB phosphorylation, liver F4/80 positive macrophages, inflammatory cytokine IL-1β and TNF-α expression. In conclusion, HZRG treatment enhances fecal BA excretion via inhibiting BA transporters, modulates BA profiles, gut dysbiosis as well as the intestinal environment, thus contributing to the beneficial effect of HZRG on NASH mice.
Introduction
Nonalcoholic fatty liver disease (NAFLD) is emerging as the leading chronic liver disease, and is considered the hepatic manifestation of metabolic syndrome, which affects more than a quarter of the world population (Younossi et al., 2018). Nonalcoholic steatohepatitis (NASH) is the progressive form of NAFLD, and is characterized by liver steatosis, inflammation, with or without fibrosis. NASH plays a pivotal role in the progression of metabolic syndrome and the development of certain tumors, thus attracting numerous pharmaceutical companies to be active in the drug development market (Anstee et al., 2019). There are many drugs in the pipeline that hold promise for treating NASH, however, approved pharmacological therapy for NASH is not available due to the complicated pathophysiological mechanisms (Negi et al., 2022). Therefore, new treatment strategies for NASH are urgently needed.
Bile acid (BA) receptors emerged as promising drug targets for NASH in recent years (Friedman et al., 2018). BAs are solely synthesized in the liver, and are considered to be associated with the pathogenesis and management of NASH. BAs are detergent molecules that aid in fat and vitamin absorption. BA synthesis takes place in the liver from cholesterol, and occurs via both classical and alternative pathways. The classical pathway is initiated by the enzyme cholesterol 7α-hydroxylase (CYP7A1), and physiologically accounts for approximately 75% of BA production, whereas the alternative pathway is catalyzed by the enzyme sterol-27-hydroxylase (CYP27A1), and contributes about 25% of BA production. Chenodeoxycholic acid (CDCA) and cholic acid (CA) are primary BAs produced in humans, and their ratio is determined by the enzyme sterol 12α-hydroxylase (CYP8B1). CDCA in mice can further generate muricholic acids (MCAs) (Gustafsson et al., 1981). Primary BAs are then conjugated with glycine or taurine in the hepatocytes, stored in the gall bladder, and released into the duodenum upon fat ingestion. BAs facilitate fat absorption within the ileum. After conducting their functions, most BAs are reabsorbed in the distal ileum via the ileal bile acid transporter (IBAT), while the remains are excreted into the colon where billions of bacteria and microorganisms are colonized. The microbial metabolism of BAs begins in deconjugation, removing the taurine or glycine from BAs, this process is conducted by bile salt hydrolase-producing bacteria. The deconjugation of BAs is of great importance because it can counteract BA toxicity and promote secondary BA production in the colon. In humans, lithocholic acid (LCA) that derived from CDCA and deoxycholic acid (DCA) from CA are the two major secondary BAs, while MCA can be further converted into hyocholicacid (HCA) and hyodeoxycholic acid (HDCA) in rodents (Wahlstrom et al., 2017).
BA homeostasis is tightly regulated by enterohepatic signaling, whereas BA accumulation causes a series of diseases including inflammatory bowel disease, cholestatic hepatitis, primary biliary cirrhosis (Fiorucci et al., 2021). Clinical investigation reported that the serum level of BAs is relatively higher in NASH patients compared with healthy controls (Sydor et al., 2020), suggesting that the alteration of the BA pool in the development of NASH. Since IBAT is in charging of the efficient BA reabsorption, IBAT inhibitors that prevent BA accumulation are promising agents in improving NASH (Yamauchi et al., 2021).
Huazhi-Rougan (HZRG) formula is a Chinese patent drug designed according to the theories of Traditional Chinese medicine (TCM). Targeting the TCM pathogenesis of damp-heat of NAFLD, HZRG has been widely used to treat NAFLD and its complications. Previous studies demonstrated that HZRG treatment significantly improved the CT value, hyperlipidemia, and reduced serum ALT and AST levels in NAFLD patients (Wang et al., 2021a). However, the underlying mechanisms are largely unknown.
The present study aimed to examine the effects of HZRG on NASH mice. We demonstrated that 4-week HZRG treatment improved liver lipid accumulation, injury and inflammation in mice fed a methionine- and choline-deficient (MCD) diet. We further identified that HZRG enhanced fecal BA excretion via inhibiting IBAT, and the modulation of BA profiles, gut dysbiosis as well as the intestinal environment all contributed to the beneficial effects of HZRG on NASH mice.
Materials and Methods
Preparation of Huazhi-Rougan Granule
HZRG granule is a patent TCM drug, composed of 16 herbal or medicinal fungi species: Artemisia scoparia Waldst. & Kitam. (Yin-Chen), Cassia abbreviata Oliv. (Jue-Ming-Zi), Rheum officinale Baill. (Da-Huang), Alisma orientale (Sam.) Juz. (Ze-Xie), Polyporus umbellatus (Pers.) Fries. (Zhu-Ling), Crataegus pinnatifida Bunge. (Shan-Zha), Atractylodes lancea (Thunb.) DC. (Cang-Shu), Atractylis macrocephala (Koidz.) Hand. -Mazz. (Bai-Shu), Citrus reticulata Blanco (Chen-Pi), Trichosanthes kirilowii Maxim. (Gua-Lou), Ligustrum lucidum W. T. Aiton (Nv-Zhen-Zi), Eclipta prostrata (L.) L. (Mo-Han-Lian), Lycium barbarum L. (Gou-Qi-Zi), Cirsium setosum (Willd.) Besser (Xiao-Ji), Bupleurum chinense DC. (Chai-Hu), and Glycyrrhiza uralensis Fisch. (Gan-Cao). The ratio of each component in the formula was defined based on a previous study (Wang et al., 2021a). The granules were purchased from Shandong New Time Pharmaceutical CO., Ltd. The chemical constituents in HZRG granules were analyzed based on UPLC-Q-TOF/MS approach (Hu et al., 2019).
Animal and Diet
Forty male C57BL/6J mice of 6-week age were purchased from Gempharmatech Experimental Animal Technology Co. Ltd. (Jiangsu, China), and placed in the specific-pathogen-free environment at constant temperature (22 ± 2°C) and humidity (55 ± 15%), and 24 h light/dark alternation. The mice were divided into four groups after 1-week acclimatization: control group (n = 10) received chow diet (Research Diet, C17040502), NASH group (n = 10) received MCD diet (Research Diet, A02082002B), HZRG high dose (HRH, 6 g/kg/d) and low dose (HRL, 3 g/kg/d) groups (n = 10 per group) received MCD diet plus HZRG administration. The low dose of HZRG was equivalent to the effective clinical dose, while the double-dose was defined as a high dose. The drugs were dissolved in 0.5% carboxymethyl cellulose sodium solution (CMC-Na) and administered to the mice by gavage (0.1 ml/10 g body weight) once a day for 4 weeks, the control and NASH mice were given equivalent 0.5% CMC-Na solution. At the end of the experiment, mice were anesthetized via 2% pentobarbital sodium injection (1.5 ml/kg). Blood was collected to separate serum for biological analysis. A portion of the liver was fixed in 4% paraformaldehyde solution. Intestine, cecal feces, and the rest of liver portions were snap-frozen in liquid nitrogen and then stored at −80°C refrigerator. All mice were received humane care during this experiment, and the experiment was approved by the Animal Experiment Ethics Committee of Gempharmatech CO., Ltd. IACUC (Approval number: GPTAP20200721-2).
Liver Histopathology
Liver pathological alterations were presented by the established method of our lab (Li et al., 2021). In brief, liver tissues were fixed, then dehydrated and embedded in paraffin. Paraffin-embedded tissue was cut into 4 μm sections and stained with hematoxylin-eosin (H&E) according to the standard process (Kohypath, Shanghai, China). For Oil Red O (ORO) staining, frozen liver tissues were embedded in Tissue-Tek OCT Compound (Sakura, Tokyo, Japan), cut into ∼8 μm sections, and stained with ORO reagent (Sigma, St. Louis, MO, United States). For immunohistochemical (IHC) analysis, anti-F4/80 (70076 s, cell signaling technology) primary antibody, and biotinylated goat anti-rabbit IgG (BOSTER, SA1022) were applied. Images were captured under a Nikon Eclipse 50i microscope (Nikon, Tokyo, Japan) with a magnification of ×200.
Analysis of Serum and Liver Biochemical Parameters
The serum alanine aminotransferase (ALT), aspartate aminotransferase (AST), and were analyzed by a TBA-40FR Fully Automatic Biochemical Analyzer (TOSHIBA, Japan) according to the manufacturer’s protocol. Serum tumor necrosis factor-alpha (TNF-α) was detected using a mouse ELISA kit (mlbio, Shanghai, China). The liver tissue in ethanol was homogenized to collect supernatant for detecting TC and TG contents in the liver with certain kits (Nanjing Jiancheng Bioengineering Institute).
16S rDNA Sequence
Cecal feces of mice were collected for 16S rDNA analysis of gut microbiota. Microbial genome DNA was extracted using the Qiagen QIAamp DNA Stool Mini Kit (Qiagen, Dusseldorf, Germany), and quantified and characterized by the NanoDrop 2000°C spectrophotometer and agarose gel electrophoresis, respectively. The V3-V4 region of the bacterial 16S ribosomal RNA was amplified by PCR and used for the following analysis. The sequencing and analysis were performed as previously reported (Shu et al., 2021).
Bile Acid Profile Analysis
The BA profile of fecal and serum sample was quantified by ultra-performance liquid-chromatography coupled with triple quadrupole mass spectrometry (UPLC-TQMS, Waters, Milford, MA) according to the previous method (Shu et al., 2021).
Western Blot
Liver and intestinal samples were homogenized in RIPA buffer added with protease and phosphatase inhibitors. Full centrifugation at low temperature (15 min at 12,000 g) to obtain supernatant, and the protein concentration was quantified by BCA kit (Epizyme, Shanghai, China), proteins electrophoresis using the 10% sodium dodecyl sulfate-polyacrylamide gel and transferred onto 0.45 μm PVDF membranes (Millipore, United States). Subsequently, the PVDF membranes were socked in 5% skim milk containing 140 mmol/L NaCl, 20 mmol/L Tris-HCl (pH 7.5), and 0.1% Tween 20°at room temperature for 60 min, and incubated with primary antibodies at 4°C overnight: FXR mouse monoclonal antibody (72105S, CST, United States), TGR5 rabbitpolyclonal antibody (72,608, Abcam, United States), ZO-1 (ab216880, Abcam, United States), Occludin (ab 216,327, Abcam, United States), Claudin 2 (ab53032, Abcam, United States), P-P65 rabbit monoclonal antibody (3031S, CST, United States), P65 rabbit monoclonal antibody (8242S, CST, United States), β-actin (Hua-an Biotech Inc., Hangzhou, China), and then incubated with horseradish peroxidase-conjugated secondary antibodies at room temperature for another 60 min. The protein bands were visualized by an ECL chemiluminescence detection kit (WBKLS0500, Millipore, United States) with an enhanced chemiluminescence system (Tanon 5200, Shanghai, China).
Real-Time Quantitative PCR
Liver tissues were homogenized in TRIzol reagent (Invitrogen Corp, Carlsbad, CA, United States) and the total RNA was isolated. RNA concentration was measured using a NanoDrop 2000°C spectrophotometer, and was reversely transcribed into complementary DNA by reverse transcription kit (Accurate Biology, Shanghai, China). The PCR primers (Shanjin Biotech, Shanghai, China) showed in Table 1. GAPDH was used as the internal control, and the expression of the target gene was normalized to GAPDH expression, and the relative expression was calculated by the 2−ΔΔT method.
Statistical Analysis
All the data are collected and expressed as mean ± standard deviation (SD). Statistical analysis was performed using a one-way analysis of variance (ANOVA). Independent-sample t test was used to compare differences between two groups. Mann-Whitney U tests and Spearman correlation were performed using SPSS 26.0 software. p < 0.05 was considered of statistical significance.
Results
Chemical Profiling of Huazhi-Rougan
A total of 100 constituents have been identified or tentatively characterized in HZRG granule (compounds of 16 medicinal) under positive or negative ion mode (Figure 1 and Table 2). Among them, 20 constituents attributed to Lycium barbarum L. (Gou-Qi-Zi), 13 attributed to Eclipta prostrata (L.) L. (Mo-Han-Lian), 11 attributed to Ligustrum lucidum W. T. Aiton (Nv-Zhen-Zi), six attributed to Atractylodes lancea (Thunb.) DC. (Cang-Shu), 8 attributed to Rheum officinale Baill. (Da-Huang), 11 attributed to Citrus reticulata Blanco (Chen-Pi), eight attributed to Glycyrrhiza uralensis Fisch. (Gan-Cao), three attributed to Crataegus pinnatifida Bunge. (Shan-Zha), five attributed to Bupleurum chinense DC. (Chai-Hu), four attributed to Trichosanthes kirilowii Maxim. (Gua-Lou), four attributed to Polyporus umbellatus (Pers.) Fries. (Zhu-Ling), three attributed to Artemisia scoparia Waldst. and Kitam. (Yin-Chen), three attributed to Atractylis macrocephala (Koidz.) Hand. -Mazz. (Bai-Shu), and two was attributed to Cirsium setosum (Willd.) Besser (Xiao-Ji) (Table 2). Collectively, the dominant constituents in HZRG granule belong to flavonoids, alkaloids and lactones.
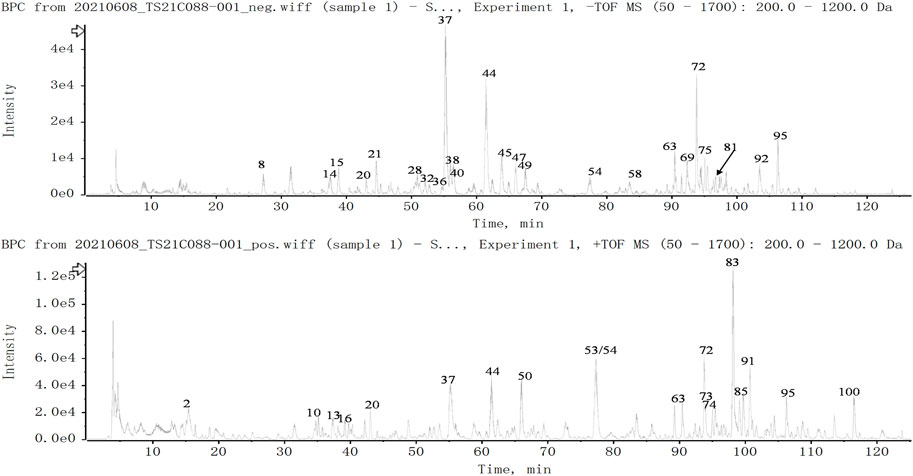
FIGURE 1. Total ion chromatogram of constituents in HZRG. Agilent 1290 UPLC system was applied to analyze the chemical profiling of HZRG granules, data were collected under both negative ion mode and positive ion mode, and processed by Analyst Ver. 1.6 software.
Huazhi-Rougan Attenuates Non-Alcoholic Steatohepatitis in Methionine-Choline-Deficiency Mice
To investigate whether HZRG has an effect on NASH, we used MCD-fed mice as a NASH model. MCD-fed mice were treated with either low dose, high dose of HZRG, or vehicle for 4 weeks. MCD-fed mice showed a significant decrease in body weight and the liver/body weight ratio in comparison to control mice, but no statistical difference was found among treated groups (Figures 2A,B). MCD feeding induced obvious steatosis, inflammatory cell infiltration in liver sections as evidenced by H&E staining and ORO staining, and both high and low dose HZRG treatment significantly improved liver steatosis and reduced the infiltration of inflammatory cells (Figure 2C). The quantification of hepatic lipids revealed that HZRG treatment also decreased liver TG content, which was consistent with the pathological change (Figure 2D). However, the liver TC content showed no statistical difference among groups (Figure 2E). HZRG also significantly decreased serum ALT and AST levels in MCD-fed mice (Figures 2F,G), indicating the protective effects against liver damage. Collectively, these results suggest that HZRG attenuates NASH in MCD-fed mice, and the high dose was superior to the low dose HZRG.
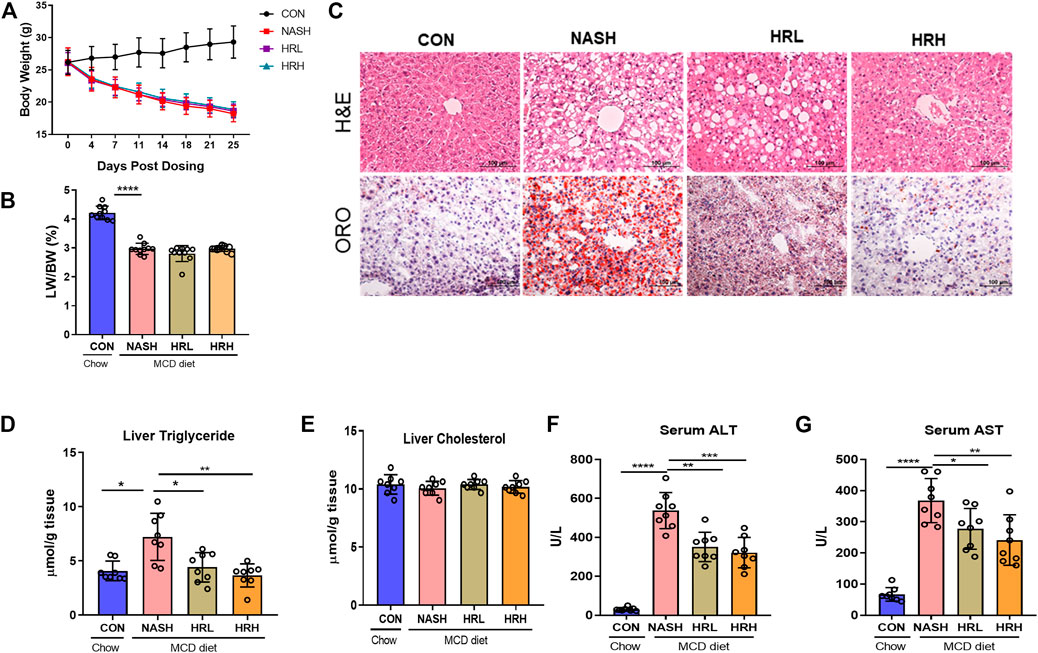
FIGURE 2. Effects of HZRG on MCD induced NASH mice. (A) Body weight; (B) liver/body weight ratio; (C) Representative H&E and ORO staining images of liver sections (magnification ×200); (D,E) Liver TG and TC content; (F,G) Serum ALT and AST levels. Data are expressed as mean ± SD, 10 animals were allocated for each group. *p < 0.05, **p < 0.01; ***p < 0.001 between groups.
Huazhi-Rougan Alleviates Methionine-Choline-Deficiency-Induced Gut Dysbiosis
Gut dysbiosis plays a pivotal role in the development and progression of NASH, and modulation of gut microbiota is a potential therapeutic strategy for NASH. HZRG contains various phytochemicals, such as flavonoids and alkaloids, which are known to regulate dysbiosis. We examined the effects of HZRG on the structure of gut microbiota by performing bacterial 16S rDNA sequence in feces, and observed a distinct clustering of microbiota for control, NASH, and HZH treatment groups using weighted (Figure 3A) and unweighted (Figure 3B) UniFrac-based principal coordinates analysis (PCoA), respectively. The comparison among the three groups revealed 1874 operational Taxonomic Units (OTUs) in the control group, 906 OTUs in the NASH group, and 858 OTUs in the HZRG-treated group, and a total of 234 OTUs shared all the samples (Figure 3C). At the phylum level, Firmicutes, Bacteroidetes, Actinobactiria, and Verrucomicrobia were the dominant four phyla (Figure 3D). The Family-level analysis revealed that the MCD diet increased the relative abundance of Peptostreptococcaceae, Atopobiaceae, Enterobacteriaceae, Erysipelotrichaceae, and Streptococcaceae, whereas HZRG decreased the relative abundance of these microbiomes. Notably, HZRG specifically increased the enrichment of Lactobacillaceae, Bifidobacteriaceae, Clostruduaceae, Chostridiales VadinBB60, Corynebacteriaceae, Solanales, Propionibacteriaceae, Micrococcaceae, and Satphylococcaceae (Figure 3E). In addition, the comparison was also conducted in genus and species levels (Supplementary Figure S1) Meanwhile, the functional prediction analysis based on PICRUST (phylogenetic investigation of communities by reconstruction of unobserved states) suggested that BA biosynthesis was the most obvious pathway upon HZRG treatment (Figure 3F). These results indicated that HZRG modulates the gut microbiota of NASH mice, resulting in the alleviation of dysbiosis in MCD-fed mice.
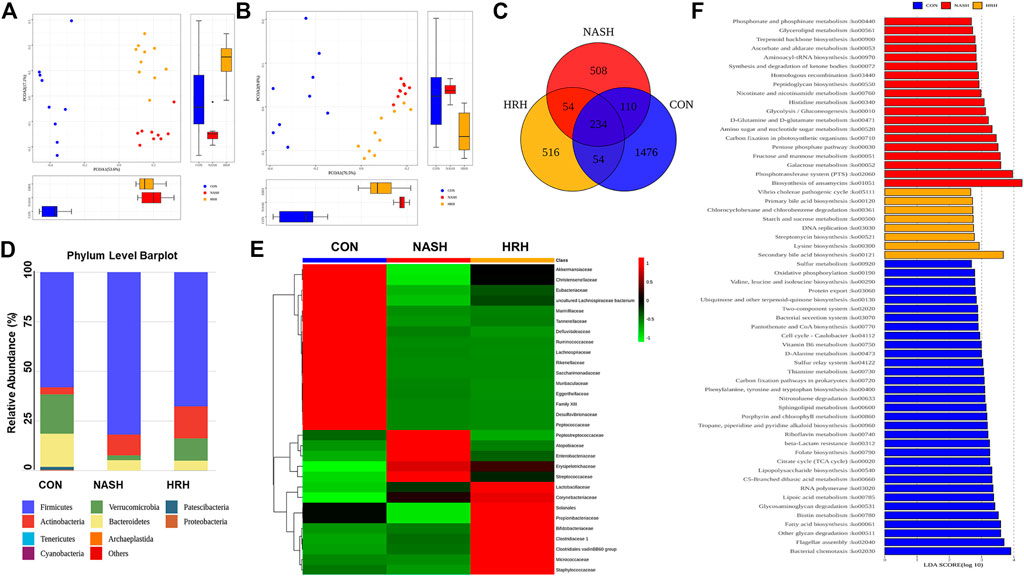
FIGURE 3. HZRG modulates gut microbiota in NASH mice. (A) PCoA analysis based on the weighted UniFrac analysis of OTUs; (B) PCoA analysis based on the unweighted UniFrac analysis of OTUs; (C) Venn Diagram; (D) Mean Phylum abundance of OTUs; (E) Mean family abundance of OTUs; (F) Pathways that based on PICRUST functional prediction. Data are expressed as mean ± SD of 9 or 10 animals per group.
Huazhi-Rougan Enhances Fecal Bile Acid Excretion
To assess BA profile alternation in response to the of gut microbiota, a UPLC/TQMS based targeted metabolomics approach was applied to analyze the fecal BAs in mice. The results revealed that the total level of fecal BAs was dramatically elevated upon HZRG treatment in NASH mice, although the total BA level between control mice and NASH mice was not statistically different (Figure 4A). By analyzing BA composition, we found that the relative abundance of secondary BAs was significantly decreased in NASH mice, whereas HZRG intervention increased the percent of fecal secondary BAs (Figure 4B). The BA profiling showed that HZRG treatment significantly increased the secondary BA species such as LCA, ketoLCAs (6,7-keto, 6-keto, 7-keto), HCA and βDCA in NASH mice (Figures 4C,D). The changes in total fecal BA level along with the increased content of secondary BAs suggested that HZRG promoted fecal BA excretion, especially the secondary BA species. Transformation into secondary BAs largely depends on the action of gut microbiota. To explore the correlation of HZRG modulated fecal BAs and gut microbiota, a Spearman correlation was conducted between the relative abundance of the 21 differential microbial species and the 11 BA species in the NASH and HZRG groups. All of the 11 BAs had at least one significant correlation with a microbe (Figure 4E). βUCA was negatively correlated with microbial Genus Erysipelatoclostridium, Dubosiella, Coriobacteriaceae UCG-002 and Romboutsia, whereas all the other differentiate BAs (DCA, HCA, LCA, ketoLCAs, etc) were positively correlated with these microbial species.
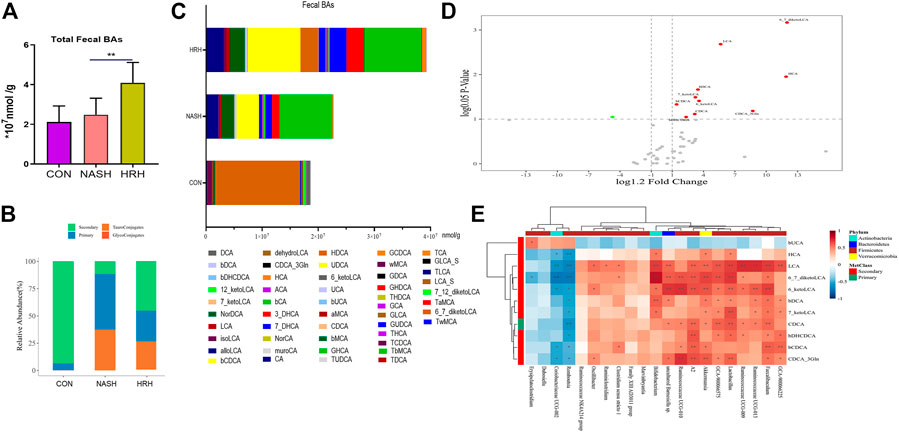
FIGURE 4. HZRG enhances fecal BA excretion. (A) The total fecal BA concentration; (B) The alteration of BA classification; (C) Fecal BA profile; (D) Volcano plot shows the change of BA species upon HZRG intervention (Red-increase, green-decrease); (E) Spearman correlation analysis of the relative abundance of microbial species and the BAs. Data are expressed as mean ± SD, n = 10 per group. *p < 0.05, **p < 0.01between groups.
Huazhi-Rougan Inhibits Bile Acid Reabsorption
Physiologically, fecal BA reabsorption and excretion are in dynamic balance. BA undergoes continuous enterohepatic circulation, and 95% of BAs are re-absorbed at the ileum in each circle, thus the BA transport process determines the alteration of fecal BAs. IBAT is the chief mediator of intestinal BA absorption in charging transporting BA from the lumen to the enterocytes. The absorbed BAs in enterocytes then secret into the portal circulation via the basolateral BA transporters organic solute transporter subunit α (OST) α, OSTβ, multidrug-resistance protein (MRP) two and MRP3 (Figure 5A). BA receptors are reported to regulate the transporters, so we examined FXR and TGR5 expression in the intestine. Although the protein expression of FXR and TGR5 was decreased in NASH mice, HZRG intervention did not affect their expression, indicating the regulation of HZRG on BA receptors was limited (Figure 5B). The mRNA expression of IBAT and OSTβ was significantly increased in NASH mice compared to control mice, and HZRG intervention significantly decreased their expression. However, other transporters such as OSTα, MRP2, and MRP3 did not show statistical differences among groups (Figure 5C).
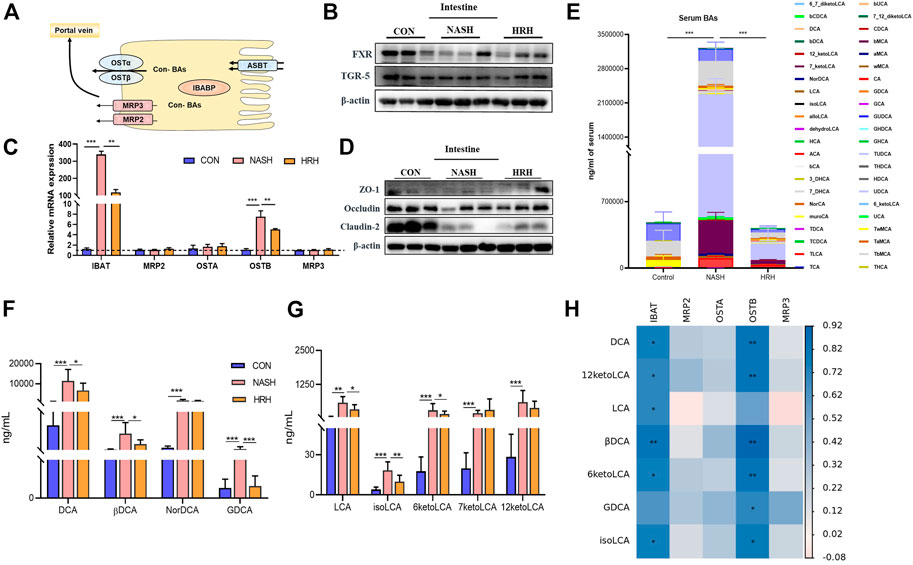
FIGURE 5. HZRG inhibits BA reabsorption. (A) The model of BA transport in the intestine; (B) The protein expression of intestinal BA receptors; (C) The mRNA expression of BA transporters; (D) Intestinal tight junction protein expression; (E) Serum BA profile; (F,G) The alteration of secondary BA species; (H) The correlation of altered secondary BAs and the genes regulating BA transport. Data are expressed as mean ± SD, n = 10 per group. *p < 0.05, **p < 0.01; ***p < 0.001 between groups.
Suppression of BA transport indicates less BA absorption. Accumulation of toxic BAs may destroy the intestinal barrier. To verify the effect of HZRG on BA absorption, we first detected the tight junction proteins that are associated with intestinal permeability, and found that the expression of tight junction protein Occludin and Claudin was decreased in NASH mice, whereas HZRG intervention counteracted the decrease (Figure 5D). We further investigated the serum BA profiles of the mice to verify the regulation of HZRG on BA reabsorption. The total serum BA was significantly increased in NASH mice compared to control mice, whereas HZRG treatment reversed the increase of total serum BA level (Figure 5E). And the alteration of total serum BAs among 44 detected BA species was mostly attributed to the changes of secondary BA species. Most of the toxic DCA species showed an increase in NASH mice, and HZRG treatment significantly decrease the concentration of DCA, βDCA and GDCA (Figure 5F). Correspondingly, treatment of HZRG also decreased LCA, isoLCA and 6-ketoLCA levels of the NASH mice (Figure 5G). The altered secondary BAs were correlated with BA transport genes IBAT and OSTB (Figure 5H). Therefore, the decrease of serum secondary BAs upon HZRG treatment indicated lower reabsorption from the intestine, which was consistent with the alteration of fecal BA concentrations.
Huazhi-Rougan Attenuates Hepatic Inflammation
The suppression of toxic BA reabsorption along with the attenuation of the intestinal environment by HZRG is supposed to improve hepatic inflammation. HZRG significantly decreased the serum TNF-α level in NASH mice (Figure 6A). We also investigated a classic inflammatory pathway, and found that HZRG decreased the phosphorylation of nuclear factor-kappaB (NF-kB p65) (Figure 6B), indicating the inhibition of NF-kB activation. Consistently, the expression of macrophage marker F4/80 was increased in the liver of NASH mice, whereas HZRG treatment significantly reduced the expression of macrophage marker (Figure 6C). In addition, the mRNA of liver inflammatory cytokines TNF-α and interleukin-1 beta (IL-1β) also decreased upon HZRG treatment (Figures 6D,E). These results suggested that HZRG attenuated hepatic inflammation.
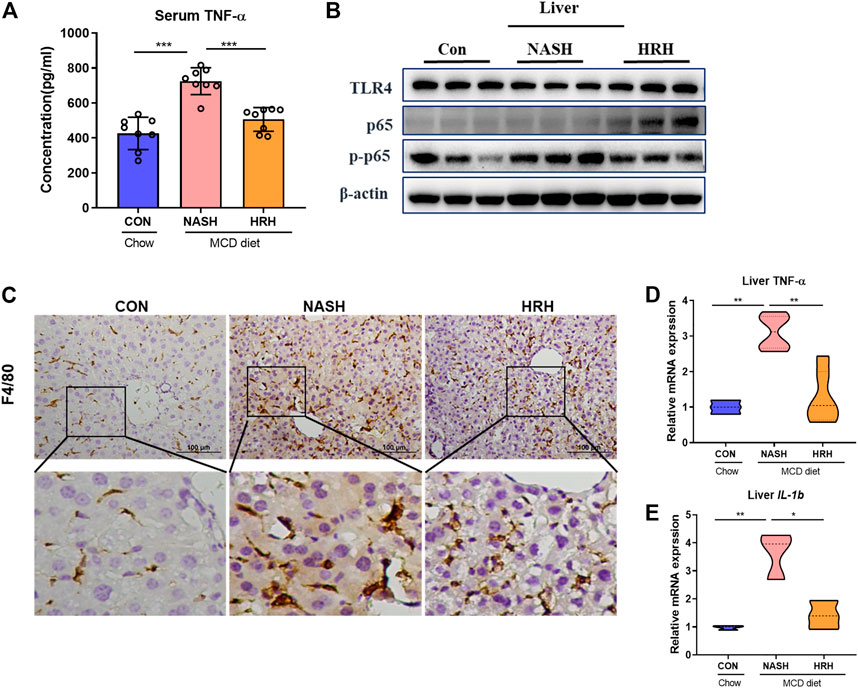
FIGURE 6. HZRG suppressed hepatic inflammation. (A) The serum concentration of TNFα; (B) The protein expression of liver TLR4, P-P65, P65. (C) IHC staining of liver macrophage biomarker F4/80; (D,E) The mRNA expression of liver Tnfα were and IL-1β. Data were shown as mean ± SD, *p < 0.05, **p < 0.01 between groups.
Discussion
TCM intervention is an important strategy for NASH treatment. The philosophy of TCM theories is different from modern medicine, which emphasizes an overview of the entire situation. The lack of recognized drugs is largely attributed to the complicated pathological mechanisms of NASH, and decades of works on exploring NASH drugs highlight that single target medicines are difficult to solve all problems (Friedman et al., 2018). The prescriptions with more than one medicinal that based on TCM theory have been widely used in Asian regions. In recent years, accumulating evidence has demonstrated the beneficial role of Chinese prescriptions in the treatment of NAFLD and NASH. HZRG prescription has been made as standard granules form, which is prescribed for NAFLD patients for decades. In the current study, we found that HZRG ameliorates the NASH phenotype induced by MCD diet feeding, the beneficial effects of HZRG are related to the modulation of gut dysbiosis and fecal BA excretion via inhibit IBAT expression (Figure 7).
HZRG is composed of 16 medicinal species that contain more than 100 ingredients. At this stage our analysis provides detailed qualitive analysis of the diversity of phytochemicals present. Any future clinical study should provide quantitative data on major constituents in the formula and perhaps information on their pharmacokinetics. According to TCM theory, the prescription is designed to clear Damp-Heat, while the best way to discard the pathogen is to promote their excretion. Analysis of HZRG revealed that the formula is rich in flavonoids, alkaloid and benzene, which were with low bioavailability, indicating the compounds might be abundantly detained in the intestine. Actually, the therapeutic effects of a series of TCM formulas are reported to be associated with the modulation of gut microbiota. Consistently, we found that HZRG modulated the gut dysbiosis, decreased the Firmicutes/Bacteroidetes (F/B) ratio in NASH mice. The F/B ratio increase is a feature of metabolic diseases, and regimens decreasing the ratio are reported to reverse the dysfunction (Spychala et al., 2018). The abundance of Atopobiaceae is reported to be associated with multidrug-resistant organism colonization in nursing home residents (Ducarmon et al., 2021). Enrichment of Erysipelotrichaceae, Erysipelotrichaceae, and Streptococcaceae was found in inflammatory bowel disease, obesity, NAFLD patients and animals, whereas decrease their abundant is associated with the alleviation of colitis (Lupp et al., 2007; Etxeberria et al., 2015; Monk et al., 2016; Sookoian et al., 2020). Consistently, we found that abundance of these pathogenic bacteria was all increased in NASH mice, where HZRG intervention significantly decreased their enrichment. HZRG specifically increased the enrichment of Lactobacillaceae, Bifidobacteriaceae, Clostruduaceae, Chostridiales VadinBB60 group, Corynebacteriaceae, Solanales, Propionibacteriaceae, Micrococcaceae, and Satphylococcaceae. The secondary BAs are formed from primary BAs, and numerous bacterial genera in the gut are involved in the BA transformation. Bacteroides, Clostridium, Lactobacillus, and Bifidobacterium are confirmed to carry out the deconjugation, oxidation and epimerization of BAs, and subsequently, Bacteroides, Clostridium, Eubacterium, Lactobacillus and Escherichia convert the unconjugated CDCA and CA into the secondary LCA and DCA (Ridlon et al., 2006). In our study, we noticed the increase of bacterial genera that contributes to secondary BA formation in HZRG treatment mice, which was consistent with the fecal BA profile.
Ileal BA transport is an efficient system, accounting for 95% intraluminal BA reabsorption at the terminal ileum. IBAT, also known as apical sodium-dependent bile acid transporter (ASBT), and SLC10A2, is mainly expressed in the apical membrane of ileal enterocytes. IBAT determines the size of the BA pool and regulates the homeostasis of lipid metabolism. By reducing gut-derived BAs entering the liver, IBAT inhibitors may potentially reduce the liver damage in cholestatic liver disease, and IBAT inhibitors, such as maralixibat and odevixibat, are in clinical programs for treating pediatric cholestatic liver diseases (Karpen et al., 2020). In diet-induced NAFLD mice, IBAT inhibitor IMB17-15 is reported to alter the intestinal BA composition and mediate intestinal FGF15/19 pathway, which contributes to the improvement of NAFLD phenotype in mice (Ge et al., 2019). Another IBAT inhibitor SC-435 is found to significantly reduce the liver fat of NAFLD mice, which is associated with liver FXR activation and lipid synthesis inhibition (Rao et al., 2016; Rao et al., 2020). Thus, IBAT inhibition presents as a new type of treatment strategy for NASH and related complications. In comparison to the currently under-testing FXR agonist obeticholic acid on NASH, specific inhibition of IBAT may reduce itching and other side effects (Li and Chiang, 2020).
The secondary BAs LCA and DCA are hydrophobic and unconjugated, and considered to be cytotoxic, which are related to intestinal barrier damage and liver cell injury. It is reported that the excretion of the LCA and DCA in feces is increased upon the application of IBAT inhibitor volixibat. Consistently, obese and overweight adults who orally take volixibat showed improved dyslipidemia accompanied by increased fecal BA excretion (Salic et al., 2019). Inhibition of IBAT also leads to increased BA delivery to the colon, which accelerates colonic transit and increases colonic secretion, thus regarded as a promising regimen for chronic constipation (Chedid et al., 2018). In the present study, we found that HZRG obviously suppressed the expression of IBAT in the intestine, and simultaneously reduced the expression of MRP2/3 and OSTα/β, the molecules that control BA transport back the portal vein.
BAs obtain potential cytotoxic effects on extra-hepatic tissues. The enterohepatic circulation functions to safely store and then promptly deliver BAs in high concentration to the intestinal lumen for digestion and absorption of lipids. The gut microbiota dysbiosis promoted BA homeostasis disbalance, characterized by the accumulation of LCA and DCA in feces (Guo et al., 2021). Dysregulated expression of the ASBT and OSTα/β may lead to BA accumulation and injury in liver epithelial cells. Higher levels of intestinal secondary BAs (DCA and LCA) are associated with the down-regulation of tight junction proteins, indicating that DCA and LCA impair gut barrier function (Pi et al., 2020). ASBT knockout mice showed more than 5-fold fecal BA excretion, reduced whole body BA pool size, and altered BA pool composition. Inactivation of OSTα in mice demonstrated the almost same extent of BA pool size as ASBT knockout mice (Dawson et al., 2003; Rao et al., 2008). Loss-of-function ASBT mutations in humans yield a classical primary BA malabsorption phenotype without ileal histological changes, suggesting IBAT inhibition potentiates protective effects on the intestinal environment (Oelkers et al., 1997).
The alteration of BAs may also regulate the intestinal immune response (Wang et al., 2021b). Interleukin -17 is a main pro-inflammatory cytokine involved in gut dysbiosis, and this role is highlighted by recent data indicating that the IL-17/IL-17R axis drives intestinal neutrophil migration, limits gut dysbiosis and attenuates LPS translocation to the circulation and tissue, resulting in protection to high-fat diet-induced mice (Perez et al., 2019). Actually, there is a previous study emphasizing the IL-17 role in this setting. Specifically, the association found between the amount of visceral fat and circulating levels of eotaxin on the one hand, and intima-media thickness on the other, could reinforce the hypothesis that IL-17, released by the visceral adipose tissue, induces eotaxin secretion via the smooth muscle cells present in the atheromatosus vessels of patients suffering from obesity-related NAFLD (Tarantino et al., 2014). Therefore, the role of BAs in maintaining the IL-17/IL-17R axis is also contribute to the protection effects of HZRG on NASH.
In conclusion, the current study demonstrates that HZRG treatment counteracts MCD-induced liver steatosis and inflammation, the beneficial effects of HZRG are associated with the modulation of gut dysbiosis and promotion of fecal BA excretion (Figure 7). Our findings expand the current knowledge of microbiota-BA interaction, and provide evidence that HZRG administration reduces pathogenic microbiota, enhances fecal BA excretion, and reduces secondary BA accumulation in the intestine.
Data Availability Statement
The datasets presented in this study can be found in online repositories. The names of the repository/repositories and accession number(s) can be found below: https://www.ncbi.nlm.nih.gov/bioproject/, PRJNA799458.
Ethics Statement
The animal study was reviewed and approved by Animal Experiment Ethics Committee of Gempharmatech CO., Ltd. IACUC (Approval number: GPTAP20200721-2).
Author Contributions
LZ and GJ developed the idea and designed the research. CL, SY, and XL carried out experiments, collected the samples. ML analyzed the data, LZ and CL interpreted the data, drafted the manuscript, GJ supervised the process, and critically revised the manuscript. All data were generated in-house, and the authors all have read and approved the submitted version.
Funding
The work was supported by the grants from the National Natural Science Foundation of China (No. 82174250). The funders had no role in study design, data collection and analysis, decision to publish, or preparation of the manuscript.
Conflict of Interest
The authors declare that the research was conducted in the absence of any commercial or financial relationships that could be construed as a potential conflict of interest.
Publisher’s Note
All claims expressed in this article are solely those of the authors and do not necessarily represent those of their affiliated organizations, or those of the publisher, the editors and the reviewers. Any product that may be evaluated in this article, or claim that may be made by its manufacturer, is not guaranteed or endorsed by the publisher.
Supplementary Material
The Supplementary Material for this article can be found online at: https://www.frontiersin.org/articles/10.3389/fphar.2022.833414/full#supplementary-material
References
Anstee, Q. M., Reeves, H. L., Kotsiliti, E., Govaere, O., and Heikenwalder, M. (2019). From NASH to HCC: Current Concepts and Future Challenges. Nat. Rev. Gastroenterol. Hepatol. 16 (7), 411–428. doi:10.1038/s41575-019-0145-7
Chedid, V., Vijayvargiya, P., and Camilleri, M. (2018). Elobixibat for the Treatment of Constipation. Expert Rev. Gastroenterol. Hepatol. 12 (10), 951–960. doi:10.1080/17474124.2018.1522248
Dawson, P. A., Haywood, J., Craddock, A. L., Wilson, M., Tietjen, M., Kluckman, K., et al. (2003). Targeted Deletion of the Ileal Bile Acid Transporter Eliminates Enterohepatic Cycling of Bile Acids in Mice. J. Biol. Chem. 278 (36), 33920–33927. doi:10.1074/jbc.M306370200
Ducarmon, Q. R., Terveer, E. M., Nooij, S., Bloem, M. N., Vendrik, K. E. W., Caljouw, M. A. A., et al. (2021). Microbiota-associated Risk Factors for Asymptomatic Gut Colonisation with Multi-Drug-Resistant Organisms in a Dutch Nursing Home. Genome Med. 13 (1), 54. doi:10.1186/s13073-021-00869-z
Etxeberria, U., Arias, N., Boqué, N., Macarulla, M. T., Portillo, M. P., Martínez, J. A., et al. (2015). Reshaping Faecal Gut Microbiota Composition by the Intake of Trans-resveratrol and Quercetin in High-Fat Sucrose Diet-Fed Rats. J. Nutr. Biochem. 26 (6), 651–660. doi:10.1016/j.jnutbio.2015.01.002
Fiorucci, S., Carino, A., Baldoni, M., Santucci, L., Costanzi, E., Graziosi, L., et al. (2021). Bile Acid Signaling in Inflammatory Bowel Diseases. Dig. Dis. Sci. 66 (3), 674–693. doi:10.1007/s10620-020-06715-3
Friedman, S. L., Neuschwander-Tetri, B. A., Rinella, M., and Sanyal, A. J. (2018). Mechanisms of NAFLD Development and Therapeutic Strategies. Nat. Med. 24 (7), 908–922. doi:10.1038/s41591-018-0104-9
Ge, M. X., Niu, W. X., Ren, J. F., Cai, S. Y., Yu, D. K., Liu, H. T., et al. (2019). A Novel ASBT Inhibitor, IMB17-15, Repressed Nonalcoholic Fatty Liver Disease Development in High-Fat Diet-Fed Syrian Golden Hamsters. Acta Pharmacol. Sin. 40 (7), 895–907. doi:10.1038/s41401-018-0195-3
Guo, Q., Tang, Y., Li, Y., Xu, Z., Zhang, D., Liu, J., et al. (2021). Perinatal High-Salt Diet Induces Gut Microbiota Dysbiosis, Bile Acid Homeostasis Disbalance, and NAFLD in Weanling Mice Offspring. Nutrients 13 (7). 2135. doi:10.3390/nu13072135
Gustafsson, B. E., Angelin, B., Björkhem, I., Einarsson, K., and Gustafsson, J. A. (1981). Effects of Feeding Chenodeoxycholic Acid on Metabolism of Cholesterol and Bile Acids in Germ-free Rats. Lipids 16 (4), 228–233. doi:10.1007/BF02535021
Hu, L., Yao, Z., Qin, Z., Liu, L., Song, X., Dai, Y., et al. (2019). In Vivo metabolic Profiles of Bu-Zhong-Yi-Qi-Tang, a Famous Traditional Chinese Medicine Prescription, in Rats by Ultra-high-performance Liquid Chromatography Coupled with Quadrupole Time-Of-Flight Tandem Mass Spectrometry. J. Pharm. Biomed. Anal. 171, 81–98. doi:10.1016/j.jpba.2019.04.001
Karpen, S. J., Kelly, D., Mack, C., and Stein, P. (2020). Ileal Bile Acid Transporter Inhibition as an Anticholestatic Therapeutic Target in Biliary Atresia and Other Cholestatic Disorders. Hepatol. Int. 14 (5), 677–689. doi:10.1007/s12072-020-10070-w
Li, C., Zhou, W., Li, M., Shu, X., Zhang, L., and Ji, G. (2021). Salvia-Nelumbinis Naturalis Extract Protects Mice against MCD Diet-Induced Steatohepatitis via Activation of Colonic FXR-FGF15 Pathway. Biomed. Pharmacother. 139, 111587. doi:10.1016/j.biopha.2021.111587
Li, T., and Chiang, J. Y. L. (2020). Bile Acid-Based Therapies for Non-alcoholic Steatohepatitis and Alcoholic Liver Disease. Hepatobiliary Surg. Nutr. 9 (2), 152–169. doi:10.21037/hbsn.2019.09.03
Lupp, C., Robertson, M. L., Wickham, M. E., Sekirov, I., Champion, O. L., Gaynor, E. C., et al. (2007). Host-mediated Inflammation Disrupts the Intestinal Microbiota and Promotes the Overgrowth of Enterobacteriaceae. Cell Host Microbe 2 (2), 204–129. doi:10.1016/j.chom.2007.06.01010.1016/j.chom.2007.08.002
Monk, J. M., Lepp, D., Zhang, C. P., Wu, W., Zarepoor, L., Lu, J. T., et al. (2016). Diets Enriched with Cranberry Beans Alter the Microbiota and Mitigate Colitis Severity and Associated Inflammation. J. Nutr. Biochem. 28, 129–139. doi:10.1016/j.jnutbio.2015.10.014
Negi, C. K., Babica, P., Bajard, L., Bienertova-Vasku, J., and Tarantino, G. (2022). Insights into the Molecular Targets and Emerging Pharmacotherapeutic Interventions for Nonalcoholic Fatty Liver Disease. Metabolism 126, 154925. doi:10.1016/j.metabol.2021.154925
Oelkers, P., Kirby, L. C., Heubi, J. E., and Dawson, P. A. (1997). Primary Bile Acid Malabsorption Caused by Mutations in the Ileal Sodium-dependent Bile Acid Transporter Gene (SLC10A2). J. Clin. Invest. 99 (8), 1880–1887. doi:10.1172/JCI119355
Pérez, M. M., Martins, L. M. S., Dias, M. S., Pereira, C. A., Leite, J. A., Gonçalves, E. C. S., et al. (2019). Interleukin-17/interleukin-17 Receptor axis Elicits Intestinal Neutrophil Migration, Restrains Gut Dysbiosis and Lipopolysaccharide Translocation in High-Fat Diet-Induced Metabolic Syndrome Model. Immunology 156 (4), 339–355. doi:10.1111/imm.13028
Pi, Y., Mu, C., Gao, K., Liu, Z., Peng, Y., and Zhu, W. (2020). Increasing the Hindgut Carbohydrate/Protein Ratio by Cecal Infusion of Corn Starch or Casein Hydrolysate Drives Gut Microbiota-Related Bile Acid Metabolism to Stimulate Colonic Barrier Function. mSystems 5 (3). e00176-20. doi:10.1128/mSystems.00176-20
Rao, A., Haywood, J., Craddock, A. L., Belinsky, M. G., Kruh, G. D., and Dawson, P. A. (2008). The Organic Solute Transporter Alpha-Beta, Ostalpha-Ostbeta, Is Essential for Intestinal Bile Acid Transport and Homeostasis. Proc. Natl. Acad. Sci. U. S. A. 105 (10), 3891–3896. doi:10.1073/pnas.0712328105
Rao, A., Kosters, A., Mells, J. E., Zhang, W., Setchell, K. D., Amanso, A. M., et al. (2016). Inhibition of Ileal Bile Acid Uptake Protects against Nonalcoholic Fatty Liver Disease in High-Fat Diet-Fed Mice. Sci. Transl. Med. 8 (357), 357ra122. doi:10.1126/scitranslmed.aaf4823
Rao, A., van de Peppel, I. P., Gumber, S., Karpen, S. J., and Dawson, P. A. (2020). Attenuation of the Hepatoprotective Effects of Ileal Apical Sodium Dependent Bile Acid Transporter (ASBT) Inhibition in Choline-Deficient L-Amino Acid-Defined (CDAA) Diet-Fed Mice. Front. Med. (Lausanne) 7, 60. doi:10.3389/fmed.2020.00060
Ridlon, J. M., Kang, D. J., and Hylemon, P. B. (2006). Bile Salt Biotransformations by Human Intestinal Bacteria. J. Lipid Res. 47 (2), 241–259. doi:10.1194/jlr.R500013-JLR200
Salic, K., Kleemann, R., Wilkins-Port, C., McNulty, J., Verschuren, L., and Palmer, M. (2019). Apical Sodium-dependent Bile Acid Transporter Inhibition with Volixibat Improves Metabolic Aspects and Components of Non-alcoholic Steatohepatitis in Ldlr-/-.Leiden Mice. PLoS One 14 (6), e0218459. doi:10.1371/journal.pone.0218459
Shu, X., Li, M., Cao, Y., Li, C., Zhou, W., Ji, G., et al. (2021). Berberine Alleviates Non-alcoholic Steatohepatitis through Modulating Gut Microbiota Mediated Intestinal FXR Activation. Front. Pharmacol. 12, 750826. doi:10.3389/fphar.2021.750826
Sookoian, S., Salatino, A., Castaño, G. O., Landa, M. S., Fijalkowky, C., Garaycoechea, M., et al. (2020). Intrahepatic Bacterial Metataxonomic Signature in Non-alcoholic Fatty Liver Disease. Gut 69 (8), 1483–1491. doi:10.1136/gutjnl-2019-318811
Spychala, M. S., Venna, V. R., Jandzinski, M., Doran, S. J., Durgan, D. J., Ganesh, B. P., et al. (2018). Age-related Changes in the Gut Microbiota Influence Systemic Inflammation and Stroke Outcome. Ann. Neurol. 84 (1), 23–36. doi:10.1002/ana.25250
Sydor, S., Best, J., Messerschmidt, I., Manka, P., Vilchez-Vargas, R., Brodesser, S., et al. (2020). Altered Microbiota Diversity and Bile Acid Signaling in Cirrhotic and Noncirrhotic NASH-HCC. Clin. Transl. Gastroenterol. 11 (3), e00131. doi:10.14309/ctg.0000000000000131
Tarantino, G., Costantini, S., Finelli, C., Capone, F., Guerriero, E., La Sala, N., et al. (2014). Is Serum Interleukin-17 Associated with Early Atherosclerosis in Obese Patients? J. Transl. Med. 12, 214. doi:10.1186/s12967-014-0214-1
Wahlström, A., Kovatcheva-Datchary, P., Ståhlman, M., Bäckhed, F., and Marschall, H. U. (2017). Crosstalk between Bile Acids and Gut Microbiota and its Impact on Farnesoid X Receptor Signalling. Dig. Dis. 35 (3), 246–250. doi:10.1159/000450982
Wang, T., Song, J., Hu, J., Feng, S., Zhang, H., Wang, H., et al. (2021a). Efficacy and Safety of Huazhi Rougan Granule in the Treatment of Non-alcoholic Fatty Liver: a Systematic Review and Meta-Analysis. Ann. Palliat. Med. 10, 12969–12984. doi:10.21037/apm-20-1613
Wang, Z., Chen, J., Chen, Z., Xie, L., and Wang, W. (2021). Clinical Effects of Ursodeoxycholic Acid on Patients with Ulcerative Colitis May Improve via the Regulation of IL-23-IL-17 axis and the Changes of the Proportion of Intestinal Microflora. Saudi J. Gastroenterol. 27 (3), 149–157. doi:10.4103/sjg.SJG_462_20
Yamauchi, R., Takedatsu, H., Yokoyama, K., Yamauchi, E., Kawashima, M., Nagata, T., et al. (2021). Elobixibat, an Ileal Bile Acid Transporter Inhibitor, Ameliorates Non-alcoholic Steatohepatitis in Mice. Hepatol. Int. 15 (2), 392–404. doi:10.1007/s12072-020-10107-0
Younossi, Z., Anstee, Q. M., Marietti, M., Hardy, T., Henry, L., Eslam, M., et al. (2018). Global Burden of NAFLD and NASH: Trends, Predictions, Risk Factors and Prevention. Nat. Rev. Gastroenterol. Hepatol. 15 (1), 11–20. doi:10.1038/nrgastro.2017.109
Glossary
BA bile acid
CYP7A1 cholesterol 7α-hydroxylase
CYP27A1 sterol-27-hydroxylase
CDCA Chenodeoxycholic acid
CA Cholic acid
CYP8B1 sterol 12α-hydroxylase
MCAs muricholic acids
LCA ithocholic acid
DCA deoxycholic acid
HCA hyocholicacid
HDCA hyodeoxycholic acid
IBAT ileal bile acid transporter
TCM Traditional Chinese medicine
MCD methionine-and choline-deficient
CMC-Na carboxymethyl cellulose sodium
H&E hematoxylineosin
ORO Oil Red O
ALT alanine aminotransferase
AST aspartate aminotransferase
TNF-α tumor necrosis factor-alpha
TG triglyceride
TC total cholesterol
RT-qPCR Real-time quantitative PCR
PCoA principal coordinates analysis
OSTβ organic solute transporter subunit β
MRP2 Multidrug Resistanceassociated Protein 2
MRP3 Multidrug Resistanceassociated Protein 3
NF-kB nuclear factor-kappaB
IL-1β Interlukin-1 beta
F/B Firmicutes/Bacteroidetes
ASBT apical sodium-dependent bile acid transporter
SLC10A2 Solute Carrier Family 10 Member 2
Keywords: huazhi-rougan formula, non-alcoholic steatohepatitis, bile acid excretion, gut microbiota, ileal bile acid transporter
Citation: Li C, Yu S, Li X, Cao Y, Li M, Ji G and Zhang L (2022) Medicinal Formula Huazhi-Rougan Attenuates Non-Alcoholic Steatohepatitis Through Enhancing Fecal Bile Acid Excretion in Mice. Front. Pharmacol. 13:833414. doi: 10.3389/fphar.2022.833414
Received: 11 December 2021; Accepted: 16 May 2022;
Published: 01 June 2022.
Edited by:
John Thor Arnason, University of Ottawa, CanadaReviewed by:
Guoxiang Xie, University of Hawaii Cancer Center, United StatesGiovanni Tarantino, University of Naples Federico II, Italy
Copyright © 2022 Li, Yu, Li, Cao, Li, Ji and Zhang. This is an open-access article distributed under the terms of the Creative Commons Attribution License (CC BY). The use, distribution or reproduction in other forums is permitted, provided the original author(s) and the copyright owner(s) are credited and that the original publication in this journal is cited, in accordance with accepted academic practice. No use, distribution or reproduction is permitted which does not comply with these terms.
*Correspondence: Guang Ji, amdAc2h1dGNtLmVkdS5jbg==; Li Zhang, emhhbmdsaS5obEAxNjMuY29t
†These authors share first authorship