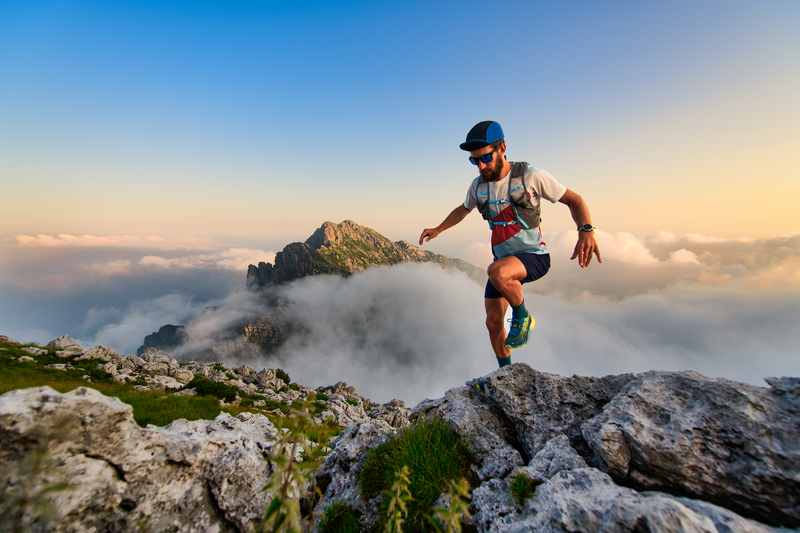
95% of researchers rate our articles as excellent or good
Learn more about the work of our research integrity team to safeguard the quality of each article we publish.
Find out more
ORIGINAL RESEARCH article
Front. Pharmacol. , 03 February 2022
Sec. Pharmacology of Ion Channels and Channelopathies
Volume 13 - 2022 | https://doi.org/10.3389/fphar.2022.821758
Cannabidiol (CBD), a major non-psychotropic cannabinoid found in the Cannabis plant, has been shown to exert anti-nociceptive, anti-psychotic, and anti-convulsant effects and to also influence the cardiovascular system. In this study, the effects of CBD on major ion currents were investigated using the patch-clamp technique in rabbit ventricular myocytes. CBD inhibited voltage-gated Na+ and Ca2+ channels with IC50 values of 5.4 and 4.8 µM, respectively. In addition, CBD, at lower concentrations, suppressed ion currents mediated by rapidly and slowly activated delayed rectifier K+ channels with IC50 of 2.4 and 2.1 µM, respectively. CBD, up to 10 μM, did not have any significant effect on inward rectifier IK1 and transient outward Ito currents. The effects of CBD on these currents developed gradually, reaching steady-state levels within 5–8 min, and recoveries were usually slow and partial. Hill coefficients higher than unity in concentration-inhibition curves suggested multiple CBD binding sites on these channels. These findings indicate that CBD affects cardiac electrophysiology by acting on a diverse range of ion channels and suggest that caution should be exercised when CBD is administered to carriers of cardiac channelopathies or to individuals using drugs known to affect the rhythm or the contractility of the heart.
Cannabis cultivars have been used since ancient times for recreational and medicinal purposes. Cannabis plant extracts contain over 100 structurally related, highly lipophilic terpenoid derivatives, collectively designated as phytocannabinoids (Pertwee et al., 2010). Among these phytocannabinoids, cannabidiol (CBD) is of great pharmacological interest because of its lack of potency on cannabinoid type 1 and type 2 receptors that are thought to mediate the psychotropic activity of the cannabis plant, an effect mainly mediated by (-)-trans-Δ9-Tetrahydrocannabinol (Mechoulam and Hanus, 2002; Pertwee et al., 2010). Cannabidiol has been shown to exert anti-nociceptive, anti-psychotic, and anti-convulsant effects (Hill et al., 2012; Vitale et al., 2021). More recently it has been approved by the US Food and Drug Administration for the treatment of patients with refractory epilepsy, e.g., Lennox–Gastaut and Dravet syndromes (Vitale et al., 2021). However, the specific molecular and cellular mechanisms underlying the effects of CBD remain largely unknown.
In earlier studies, CBD, in the concentration range of 1–30 μM, has been shown to modulate the functions of ligand-gated ion channels including nicotinic α7 (Mahgoub et al., 2013), 5-HT3 (Yang et al., 2010), glycine (Ahrens et al., 2009), and GABAA (Bakas et al., 2017) receptors as well as voltage-gated Na+ (Ghovanloo et al., 2018), L-type Ca2+ (Ali et al., 2015), T-type Ca2+ (Ross et al., 2008) and K+ (Orvos et al., 2020) channels. Drugs interacting with such a large repertoire of ion channels can legitimately be suspected to cause cardiac safety concerns due to their arrhythmogenic potential. Recently, CBD was found to affect action potential durations (Ali et al., 2015; Le Marois et al., 2020; Orvos et al., 2020; Topal et al., 2021) by acting on cardiac Na+ (Le Marois et al., 2020; Orvos et al., 2020), L-type Ca2+ (Ali et al., 2015; Le Marois et al., 2020) and K+ (Le Marois et al., 2020; Orvos et al., 2020; Topal et al., 2021) channels in rat, rabbit, and dog cardiomyocytes and in heterologous expression systems, such as HEK293 and COS cells. In this study, we investigated the effects of CBD on voltage-gated K+, Na+ and Ca2+ channels in rabbit ventricular cardiomyocytes, as a single assay system, and discussed our findings comparatively.
Male New Zealand white rabbits (ca. 2–2.5 kg) were anaesthetized by injection of sodium pentobarbitone (40 mg/kg) into a marginal ear vein and killed by removal of the heart.
This study was carried out in accordance with the recommendations in the Guide for the Care and Use of Laboratory Animals of the National Institutes of Health and approved by the Animal Care Committee of Bogomoletz Institute of Physiology of National Academy of Science of Ukraine. Ventricular myocytes were prepared by slight modification of the method described earlier (Al Kury et al., 2014a; Ali et al., 2015). Briefly, hearts were mounted for retrograde perfusion according to the Langendorff method and perfused at a constant flow of 10 ml g heart−1 min−1 and at 37°C with a solution containing (mM): 130 NaCl, 5.4 KCl, 1.4 MgCl2, 0.75 CaCl2, 0.4 NaH2PO4, 5 HEPES, 10 glucose, 20 taurine, and 10 creatine set to pH 7.3 with NaOH. When the heart had stabilized, perfusion was continued for 4 min with Ca2+-free isolation solution containing 0.1 mM EGTA, and then for 6 min with cell isolation solution containing 0.05 mM Ca2+, 0.8 mg/ml collagenase (type 1; Worthington Biochemical Corp, United States) and 0.075 mg/ml protease (type X1 V; Sigma, Taufkirchen, Germany). Ventricles were excised from the heart, minced and gently shaken in collagenase-containing isolation solution supplemented with 1% BSA. Cells were filtered from this solution at 4 min intervals and resuspended in isolation solution containing 0.75 mM Ca2+.
Myocytes were dispersed and allowed to settle for at least 1 h at room temperature (22–24°C) prior to their use. Measurements were performed only in quiescent myocytes with clear-cut striations. The whole-cell patch-clamp technique was used to evaluate individual ionic currents using an Axopatch 200B amplifier (Molecular Devices, Downington, PA, United States) linked to an A/D interface (Digidata 1,322; Molecular Devices). Currents were filtered at 5 kHz and acquired using Axon pCLAMP 8.2 (Molecular Devices, Downington, PA, United States). Heat-polished borosilicate glass pipettes (World Precision Instruments, Sarasota, FL, United States) with tip resistance of 1–3 MΩ were used to establish GΩ seals and continuity with the intracellular medium.
Cell capacitance (Cm) was calculated by integrating the area under an uncompensated capacity transient elicited by a 10 mV depolarizing pulse from a holding potential of −80 mV.
The total series resistance (Rs) between the pipette interior and the cell membrane in the whole-cell configuration was calculated from the estimates of Cm and the time constant (τc) of the capacitative current decay using the equation τc = Rs x Cm. The mean Rs for the pathway between the pipette and the cell membrane after rupture of the membrane seal was calculated to be 2.36 ± 0.09 MΩ. After establishment of whole-cell configuration and measurement of Cm, the Rs was compensated by 50–60%. Junction potentials under our conditions were approximately −3 mV and were not corrected.
The dialyzing internal pipette contained (in mM): 135 KCl, 10 NaCl, 10 HEPES, 5 Mg-ATP, 10 μM cyclic AMP; titrated with KOH to pH 7.2. The control perfusate was a modified Tyrode solution containing (in mM): 137 NaCl, 5.4 KCl, 1 MgCl2, 2 CaCl2, 10 HEPES, 10 glucose; titrated with NaOH to pH 7.4. Extracellular solution for recordings of Na+ currents consisted of (in mM): 100 TEACl, 40 NaCl, 10 glucose, 1 MgCl2, 5 CsCl, 0.1 CaCl2, 10 HEPES [adjusted to pH 7.3 with CsOH; (Al Kury et al., 2014b)], and 10 µM nifedipine included to suppress L-type Ca2+ current. Intracellular solution contained (in mM) 135 CsCl, 5 NaCl, 10, EGTA, 10 HEPES, and 1 MgATP (adjusted to pH 7.25 with CsOH). For the recording of Ca2+ currents, the whole-cell bath solution contained (in mM): 95 NaCl, 50 TEACl, 2 MgCl2, 2 CaCl2, 10 HEPES and 10 glucose (adjusted to pH 7.35 with NaOH). The pipette solution contained (in mM): 140 CsCl, 10 TEACl, 2.0 MgCl2, 2 HEPES 1 MgATP and 10 EGTA (adjusted to pH 7.25 with CsOH). For recording K+ currents, the external solution contained (in mM) 140 N-methyl-D-glucamine, 5.4 KCl, 0.1 CaCl2, 1 MgCl2, 0.5 CdCl2, 10 glucose, and 10 HEPES (pH 7.3 with NaOH). The pipette solution for IK recordings contained (in mM) 120 potassium glutamate, 20 KCl, 2 MgCl2, 10 HEPES, 5 EGTA, 2 Mg-ATP, and 2 QX314; adjusted to pH 7.4 with KOH (Rose et al., 2005). When IKr was recorded, IKs was inhibited by using 1 µM selective IKs blocker HMR 1556 (Tocris, Minneapolis, MN, United States). During IKs measurements, IKr was blocked by 1 µM dofetilide (Sigma, St. Louis, MO, United States), and the bath solution contained 0.1 µM forskolin (Sigma, St. Louis, MO, United States). For recordings of Ito, 0.5 mM CdCl2 and 20 µM tetrodotoxin (TTX) were included to eliminate IL-Ca and INa, respectively. In pharmacological verification of Na+ and Ca2+ currents, bath applications of nifedipine (10 μM; n = 4) and TTX (20 μM, n = 4) completely inhibited IL-Ca and INa, respectively. Experiments were performed at room temperature (22–24°C). Changes of external solutions and application of drugs were performed using a multi-line perfusion system with a common outflow connected to the recording chamber. A perfusion rate of 2 ml/min was used routinely in a recoding chamber with a volume of 200 µl.
Cannabidiol (CBD) was from Sigma (St. Louis, MO, United States). It was dissolved in 100% DMSO, and final concentrations were diluted from stock solutions. Stocks were kept at −20°C until their use. The control solution also contained the same quantities of the stock vehicle (e.g., 0.0001% of DMSO v/v at 1 μM of CBD). Reagents and chemicals used in our experiments were purchased from Sigma-Aldrich (St. Louis, MO, United States).
All cumulative results are expressed as mean ± SEM as indicated.
Statistical significance among groups was determined using pair-wise comparisons (Mann-Whitney U-Test) followed by Bonferroni Post-hoc analysis. Statistical analysis of the data was performed using Origin 7.0 software (OriginLab Corp, Northampton, MA, United States). Currents were measured in each cell before and after exposure to the drug. The number of tested cells for each type of ion current varied between 5 and 14. p < 0.05 was considered statistically significant.
Inward INa was elicited by incremental 10 mV step depolarizations applied from a holding potential of −80 mV with 40 mМ Na+ outside and Cs+ as the major intracellular cation. INa started to activate at -50 mV and reached maximal amplitude at −30 mV. At more positive potentials, INa gradually decreased and reversed its direction at an apparent reversal potential of around +60 mV. Bath application of CBD (0.3–30 µM) caused a gradually increasing suppression of INa. The effect of CBD was detectable at 2–3 min and reached a steady-state level within 10–15 min (n = 6). The recovery was partial during the experiments lasting up to 20–25 min. Traces of INa within the voltage range of −80 mV to +60 mV are presented before (control) and 10 min after 10 µM CBD in Figure 1A. Cannabidiol inhibited INa without causing significant changes in the I-V relationship. The current–voltage (I-V) relationship for INa is illustrated in Figure 1B. Cannabidiol inhibited INa without changing the threshold, peak and reversal potentials. The concentration-response curve is presented in Figure 1C. The IC50 value and Hill coefficient were 5.4 µM and 2.6, respectively (n = 6–8).
FIGURE 1. Cannabidiol suppresses the INa in rabbit ventricular myocytes (A) Original current traces elicited by voltages in the range of −60 mV to +60 mV in control conditions (on the left) and 10 min after exposure to 10 μM CBD (on the right). The pulse protocol to activate INa is presented in the inset (B) Current-voltage relations of INa in the absence and presence of 10 μM CBD are presented with filled and open circles, respectively (n = 6) (C) Concentration-inhibition curve for CBD suppression of INa (n = 6–8).
We have also investigated the effect of CBD on L-type Ca2+ currents (IL,Ca). IL,Ca was recorded in the presence of intracellular Cs+ and extracellular TEA+ to suppress K+ currents while retaining 95 mM Na+ in the extracellular solution. Elimination of contaminating Na+ current during the recording of IL,Ca was achieved by applying voltage step-pulses from a relatively depolarized potential of −50 mV, which produced steady-state INa inactivation (Al Kury et al., 2014b). Application of CBD (0.3–30 µM) caused a steadily progressing inhibition of INa. The effect of CBD was detectable at 2–3 min and reached a steady-state level within 5–10 min (n = 6). The recovery was partial during the experiments lasting up to 20 min. Traces of current elicited in a voltage range from −40 to +60 are presented in control (on the left) and 10 min after 10 µM CBD application (Figure 2A). In response to step depolarizations, IL,Ca had much slower kinetics and activated at more positive potentials than INa. Inward INa started to appear at −30 mV, reached a maximum at around +10 mV, and approached zero at about +50 mV (Figure 2B). Cannabidiol inhibited INa in a concentration-dependent manner with IC50 value of 4.8 µM and Hill coefficient of 1.8 (n = 5–8; Figure 2C).
FIGURE 2. Cannabidiol inhibits the IL-Ca in rabbit ventricular myocytes (A) Original current traces elicited by voltages in the range of −50 mV to +50 mV in control conditions (on the left) and after 10 min exposure to 10 μM CBD (on the right). The pulse protocol to evoke IL-Ca is presented in the inset (B) Current-voltage relations of IL-Ca in the absence and presence of 10 μM CBD are presented with filled and open circles, respectively (n = 6) (C) Concentration-inhibition curve for CBD suppression of IL-Ca (n = 5–8).
In most cases the delayed rectifier K+ current in mammalian ventricular myocytes is composed of two components, a slowly activating IKs, and a rapidly activating but inwardly rectifying IKr (Sanguinetti and Jurkiewicz, 1990).
The slowly activating delayed-rectifier K+ current (IKs) was measured as the time-dependent current accompanying 2.5 s pulses from 0 to +80 mV following a 1 s conditioning pulse from −60 to 0 mV to activate and inactivate INa and ICa. Application of CBD (0.1–10 µM) caused a gradually developing inhibition of IKs. The effect of CBD was detectable at 2–3 min and reached a steady-state level within 5–10 min. Figure 3A presents current traces recorded between 0 mV and +80 mV in control (on the left) and 10 min after 3 µM CBD application. Figure 2B presents the effect of 10 µM CBD on the current-voltage relationship of IKs (n = 6). The effect of CBD on IKs was not voltage-dependent as it suppressed IKs to the same extent at all potentials. Concentration-dependent inhibition of IK is presented in Figure 3C. The IC50 value and Hill coefficient were 2.1 µM and 1.7, respectively (n = 4–7).
FIGURE 3. Cannabidiol suppresses the IKs component of the delayed rectifying IK (A) Original current traces recorded in control conditions (on the left) and after 10 min exposure to 3 μM CBD (on the right) in rabbit cardiomyocytes. The pulse protocol to activate IKs is presented in the inset. Only solid lines in the voltage protocols are presented in current traces. Dotted line indicates 0 mV baseline (B) Current-voltage relations of IKs in the absence and presence of 3 μM CBD are presented with filled and open circles, respectively (n = 5) (C) Concentration-inhibition curve for CBD inhibition of IKs (n = 4–7).
The other member of the delayed rectifier family, IKr was quantified by activating IKr in the range of −40 mV and +50 mV and measuring the deactivating tail currents in response to repolarizing test pulse of −40 mV in rabbit cardiomyocytes. IKr tails were measured as the time-dependent currents which reached to maximal at +30 mV upon repolarizing the membrane −40 mV from positive potentials. The difference in currents measured (peak of the tail current minus the current at the beginning of test potential) was taken as an estimate of IKr. In order to increase the proportion of IKr, we inhibited IKs by 1 µM of selective IKs blocker HMR 1556 and omitted cAMP and Ca2+ from the solution to further suppress the contribution of the IKs component. Bath application of CBD (0.1–10 µM) caused inhibition of IKr which was detectable at 2–3 min and reached a steady-state level within 5 min. Traces of currents elicited at voltages ranging from −40 to +50 mV are shown in the absence (control) and 10 min presence of 3 μM CBD in Figure 4A, effects of CBD on the I-V relationship of IKr are presented in Figure 4B (n = 7). The concentration-dependent effect of CBD is shown in Figure 4C. The IC50 value and Hill coefficient were 2.4 µM and 2.7, respectively (n = 5–8).
FIGURE 4. Cannabidiol suppresses the IKr tail current. Original traces of currents recorded in rabbit cardiomyocytes are presented in panel (A). IKr tails were measured as a time-dependent component of the tail current activated in response to membrane repolarization. Recordings in control and after 10 min in the presence of 3 μM CBD are presented on the left and right panels, respectively. The pulse protocol to evoke IKr is presented in the inset (B) Current-voltage relation showing the cumulative effect of 3 μM CBD. Data points represent the averages of currents recorded with the same protocol described above in 6 cells before (control, filled circles) and after (open circles) CBD application (C) Concentration-inhibition curve for CBD inhibition of IKr tails (n = 5–8).
Using 300 ms test pulses from −80 mV to +60 mV applied at 5 s intervals, Ito was defined as the initial transient peak of the current minus the maintained current at the end of the pulse. Application of CBD up to 10 µM did not cause a significant effect on the amplitudes and kinetics of Ito. Current traces activated in the range of −40 mV to +60 mV in control (on the left) and 10 min after 10 µM CBD applicated are shown in Figure 5A. The I-V relationships of Ito in the absence and presence of 10 µM CBD are presented in Figure 5B (n = 6). Figure 5C demonstrates the lack of a CBD effect on Ito (n = 4–6 cells).
FIGURE 5. The effects of cannabidiol on the transient outward Ito in rabbit ventricular myocytes (A) Original traces of Ito obtained in response to incremental depolarizations from a holding potential of −80 mV up to +60 mV are presented in controls (on the left) and after 10 min exposure to 10 μM CBD. The pulse protocol to activate Ito is presented in the inset (B) Averages of current-voltage relation of Ito in the absence (filled circles) and presence of CBD (open circles) are presented (n = 6) (C) The effect of increasing CBD concentrations on Ito (n = 4–6).
IK1 was activated from a holding potential of −90 mV to test potentials between −120 and 0 mV. The outward component of IK1 reached a maximum at around −50 mV generating a negative slope between −50 and −10 mV (Figure 6B). The amplitude of IKl at each voltage was determined by measuring the peak current relative to zero current. After 10 min bath application, CBD up to 10 μM had little effect on IK1 at voltages between 0 and −120 mV. The I-V relationships of IK1 in the absence and presence of 10 µM CBD are presented in Figure 6B (4–5). Figure 6C shows the lack of a CBD effect on the IK1 (n = 3–5 cells).
FIGURE 6. Cannabidiol does not have a significant effect on IK1 (A) Original current traces recorded in control conditions (on the left) and after10 min exposure to 10 μM CBD (on the right) in a rabbit cardiomyocyte. The pulse protocol to evoke IK1 is presented in the inset (B) Current-voltage relations of IK1 in the absence and presence of 10 μM CBD are presented with filled and open circles, respectively (n = 6) (C) The effect of increasing CBD concentrations on IK1 (n = 4–6).
The main finding of this study is that CBD appears to be particularly effective in suppressing the delayed rectifier currents IKr and IKs. At higher concentrations, CBD inhibits inward Na+ and L-type Ca2+ channels. On the other hand, the transient outward current Ito and inward rectifier IK1 are significantly less sensitive to CBD.
In earlier studies, action potential duration (APD) was either increased in guinea pig, rabbit, and dog cardiomyocytes (Orvos et al., 2020; Topal et al., 2021) or decreased in rat ventricular myocytes (Ali et al., 2015) and rabbit Purkinje fibers (Le Marois et al., 2020). Although action potential measurements were not conducted in the present study, our results indicate that inhibitory effects of low CBD concentrations on outward K+ conductances are counteracted by the inhibition of Na+ and Ca2+ conductances at high concentrations of CBD, and indirectly confirm the finding of earlier studies showing the increased duration of action potentials at relatively low CBD concentrations and no change of the duration at higher CBD concentrations in rabbit papillary muscle cardiomyocytes (Orvos et al., 2020). In the present study, CBD inhibited outward IKr and IKs currents with IC50 values of 2.4 and 2.1 µM, respectively, and inward INa and IL-Ca with 4.8 and 5.4 µM. The potency of CBD observed in our study was slightly higher than the ones reported in earlier studies (Orvos et al., 2020; Topal et al., 2021), and it is likely due to temperature differences between the present study (room temperature) and earlier work (at 37°C). For example, CBD inhibition of INa has been shown to be significantly increased at lower temperatures (Ghovanloo et al., 2018). Similarly, the potency of CBD on IL-Ca [at room temperature, IC50 ≈ 1 μM; (Ali et al., 2015)] is significantly higher than the one recorded at 37°C [IC50 > 10 μM; (Orvos et al., 2020)].
Simulation with LabHEART, a computer model of rabbit ventricular action potentials (Puglisi and Bers, 2001), revealed that suppression of IKs and IKr alone at low (≤2 µM) concentrations of CBD as expected, causes a marked prolongation (29%) of APD, whereas integration of inhibition of INa and IL-Ca at higher CBD concentrations (≥5 µM) results in 27% shortening of APD (Figure 7), which is qualitatively in agreement with experimental data in rat and rabbit cardiomyocytes (Ali et al., 2015; Le Marois et al., 2020; Orvos et al., 2020; Topal et al., 2021). Both increase and decrease of APD can potentially have arrhythmogenic effects depending mainly on the underlying pathophysiological mechanisms. Our LabHEART simulation of rabbit cardiomyocytes paced at 5 Hz indicates that both low and high concentrations of CBD may induce arrhythmic effects (Supplementary Figure S1). On the other hand, CBD has been shown to suppresses ischemia-induced ventricular arrhythmias (Walsh et al., 2010; Gonca and Darıcı, 2015) and exert cardioprotective effects in several earlier studies (for a review (Kicman and Toczek, 2020)). Importantly, the inhibition of L-type Ca2+ channels is likely to cause the negative inotropic effects of CBD observed in earlier studies (Ali et al., 2015; Kicman and Toczek, 2020). In line with these reports, high, but not low, concentrations of CBD markedly depressed intracellular Ca2+ transients, and tension development in LabHEART simulation (Supplementary Figure S2).
FIGURE 7. Effects of low and high concentrations of CBD on the simulated action potentials using LabHEART simulations. At low (≤2 µM) CBD concentrations, inhibition of IKr and IKs was incorporated into the model. At high (≥5 µM) CBD concentrations, inhibitions of IKr, IKs, INa, and IL-Ca were incorporated into the model. At low concentrations, CBD induced a marked prolongation (29%) of action potential duration (APD), while at high concentrations, it significantly shortened (27%) the APD.
Plasma concentrations of CBD following intraperitoneal, oral, and intravenous administrations have been studied previously (Lodzki et al., 2003; Varvel et al., 2006; Deiana et al., 2012). Commonly used doses of CBD (3–30 mg/kg I.V.; mouse) are found to promote mean plasma CBD levels of 0.42–11.8 μM, respectively (Varvel et al., 2006). In another study, following oral and intraperitoneal CBD administration (120 mg/kg; rat), maximal plasma levels of CBD were 6.4 and 8.3 µM, respectively. Since CBD is a highly lipophilic compound with a LogP (octanol–water partition coefficient) value ranging between 6 and 8 (Lodzki et al., 2003), its membrane concentration is expected to be considerably higher than blood levels. In an earlier study, it has been shown that perfusion of isolated rat hearts with buffer containing [3H]-CBD results in strong accumulation of radioactivity in the tissue (Smiley et al., 1976). Therefore, the functional modulation of ion channels by the concentration ranges demonstrated in this study (2.3–5.4 µM) is likely to be pharmacologically relevant.
It is likely that CBD, a highly lipophilic agent, first dissolves into the lipid membrane and then diffuses into a non-annular lipid space to inhibit the ion channels. Consistent with this assumption, the effect of CBD on ion channels tested in this study reached a maximal level within several minutes (5–10 min) of application. Similarly, actions of several lipophilic modulators, such as capsaicin (Lundbaek et al., 2005; Alzaabi et al., 2019; Nebrisi et al., 2020), endocannabinoids (Oz et al., 2003; Spivak et al., 2007), and general anesthetics (Zhang et al., 1997; Jackson et al., 2008), on various ion channels require 5–20 min to reach their maxima, suggesting that the binding site(s) for these allosteric modifiers is/are located inside the lipid membrane and require/s a relatively slow (in minutes) time course to modulate the functions of these channels. From this aspect, it appears that alone or the combination of two mechanisms can describe the lipophilic actions of CBD (Oz, 2006; Ghovanloo and Ruben, 2021). First, CBD, like other lipophilic molecules, partitions into the lipid bilayer and alters the biophysical properties of the membrane by reducing stiffness, changing phase preference, membrane curvature and fluidity (Bach et al., 1976; Hillard et al., 1985; Ghovanloo et al., 2021). Secondly, CBD can bind directly to ion channel domains embedded in the cell membrane (Ghovanloo et al., 2021; Ghovanloo and Ruben, 2021). As a result of these mechanisms, it is likely that these hydrophobic agents, such as CBD, affect the energy requirements for gating-related conformational changes in ion channels (Spivak et al., 2007).
Collectively, our results suggest that the administration of CBD to carriers of congenital Na+, Ca2+, or K+ channelopathies, or its coadministration with other drugs known to affect cardiac electrophysiology should be cautioned. Moreover, the combined inhibition of Na+ and Ca2+ currents by CBD could counteract the positive inotropic effects of some heart failure drugs and could impede its use in conjunction with antiarrhythmic drugs. However, antiarrhythmic effects of CBD in ischemia-induced arrhythmia models have also been reported (Walsh et al., 2010; Gonca and Darıcı, 2015).
The raw data supporting the conclusions of this article will be made available by the authors, without undue reservation.
The animal study was reviewed and approved by The Animal Care Committee of Bogomoletz Institute of Physiology of National Academy of Science of Ukraine.
DI, WS, and MO conducted experiments, performed the statistical analysis, and prepared the figures. EYD simulated the action potentials and prepared the figures. DEL, GP, and MO contributed to the conception and design of the study. MO wrote the manuscript. All authors contributed to manuscript revision, read, and approved the submitted version.
The authors declare that the research was conducted in the absence of any commercial or financial relationships that could be construed as a potential conflict of interest.
All claims expressed in this article are solely those of the authors and do not necessarily represent those of their affiliated organizations, or those of the publisher, the editors and the reviewers. Any product that may be evaluated in this article, or claim that may be made by its manufacturer, is not guaranteed or endorsed by the publisher.
Authors thank Dr. Donald Bers and Dr. Jose Puglisi of University of California, Davis CA, United States of America for their help and guidance in using LabHEART v5.3.
The Supplementary Material for this article can be found online at: https://www.frontiersin.org/articles/10.3389/fphar.2022.821758/full#supplementary-material
Supplementary Figure S1 | Effects of low and high concentrations of cannabidiol on the simulated trains of action potentials elicited at stimulation frequency of 5 Hz using LabHEART simulations. (A) Trains of action potentials stimulated at 5 Hz under control conditions in the LabHEART simulation program. (B) At low (≤2 µM) CBD concentrations, inhibition of IKr and IKs was incorporated into the model. (C) At high (≥4 µM) CBD concentrations, inhibitions of IKr, IKs, INa, and IL-Ca were incorporated into the model. At low concentrations, CBD induced trains of incomplete partial depolarization waves, while at high concentrations, an early afterdepolarization-like arrhythmic pattern was notable.
Supplementary Figure S2 | Effects of low and high concentrations of cannabidiol on the simulated intracellular Ca2+ transients and force development during a single twitch response in LabHEART. (A) At low (≤2 µM) CBD concentrations, incorporation of IKr and IKs inhibition caused a slight delay in the decay phase of Ca2+ transient. At high (≥4 µM) CBD concentrations, incorporations of IKr, IKs, INa, and IL-Ca inhibitions induced a marked suppression of Ca2+ transient. (B) At low (≤2 µM) CBD concentrations, incorporation of IKr and IKs inhibition caused a slight delay in the decay phase of force development. At high (≥4 µM) CBD concentrations, incorporations of IKr, IKs, INa, and IL-Ca inhibitions induced a marked inhibition of force development during a simulated twitch response of rabbit ventricular cardiomyocyte.
Ahrens, J., Demir, R., Leuwer, M., De La Roche, J., Krampfl, K., Foadi, N., et al. (2009). The Nonpsychotropic Cannabinoid Cannabidiol Modulates and Directly Activates Alpha-1 and Alpha-1-Beta glycine Receptor Function. Pharmacology 83, 217–222. doi:10.1159/000201556
Al Kury, L. T., Voitychuk, O. I., Ali, R. M., Galadari, S., Yang, K. H., Howarth, F. C., et al. (2014a). Effects of Endogenous Cannabinoid Anandamide on Excitation-Contraction Coupling in Rat Ventricular Myocytes. Cell Calcium 55, 104–118. doi:10.1016/j.ceca.2013.12.005
Al Kury, L. T., Voitychuk, O. I., Yang, K. H., Thayyullathil, F. T., Doroshenko, P., Ramez, A. M., et al. (2014b). Effects of the Endogenous Cannabinoid Anandamide on Voltage-dependent Sodium and Calcium Channels in Rat Ventricular Myocytes. Br. J. Pharmacol. 171, 3485–3498. doi:10.1111/bph.12734
Ali, R. M., Al Kury, L. T., Yang, K. H., Qureshi, A., Rajesh, M., Galadari, S., et al. (2015). Effects of Cannabidiol on Contractions and Calcium Signaling in Rat Ventricular Myocytes. Cell Calcium 57, 290–299. doi:10.1016/j.ceca.2015.02.001
Alzaabi, A. H., Howarth, L., El Nebrisi, E., Syed, N., Susan Yang, K. H., Howarth, F. C., et al. (2019). Capsaicin Inhibits the Function of α7-nicotinic Acetylcholine Receptors Expressed in Xenopus Oocytes and Rat Hippocampal Neurons. Eur. J. Pharmacol. 857, 172411. doi:10.1016/j.ejphar.2019.172411
Bach, D., Raz, A., and Goldman, R. (1976). The Interaction of Hashish Compounds with Planar Lipid Bilayer Membranes (BLM). Biochem. Pharmacol. 25, 1241–1244. doi:10.1016/0006-2952(76)90084-8
Bakas, T., Van Nieuwenhuijzen, P. S., Devenish, S. O., Mcgregor, I. S., Arnold, J. C., and Chebib, M. (2017). The Direct Actions of Cannabidiol and 2-arachidonoyl Glycerol at GABAA Receptors. Pharmacol. Res. 119, 358–370. doi:10.1016/j.phrs.2017.02.022
Deiana, S., Watanabe, A., Yamasaki, Y., Amada, N., Arthur, M., Fleming, S., et al. (2012). Plasma and Brain Pharmacokinetic Profile of Cannabidiol (CBD), Cannabidivarine (CBDV), Δ⁹-tetrahydrocannabivarin (THCV) and Cannabigerol (CBG) in Rats and Mice Following Oral and Intraperitoneal Administration and CBD Action on Obsessive-Compulsive Behaviour. Psychopharmacology (Berl) 219, 859–873. doi:10.1007/s00213-011-2415-0
Ghovanloo, M.-R., and Ruben, P. C. (2021). Cannabidiol and Sodium Channel Pharmacology: General Overview, Mechanism, and Clinical Implications. Neuroscientist, 107385842110170. doi:10.1177/10738584211017009
Ghovanloo, M. R., Choudhury, K., Bandaru, T. S., Fouda, M. A., Rayani, K., Rusinova, R., et al. (2021). Cannabidiol Inhibits the Skeletal Muscle Nav1.4 by Blocking its Pore and by Altering Membrane Elasticity. J. Gen. Physiol. 153, e202012701. doi:10.1085/jgp.202012701
Ghovanloo, M. R., Shuart, N. G., Mezeyova, J., Dean, R. A., Ruben, P. C., and Goodchild, S. J. (2018). Inhibitory Effects of Cannabidiol on Voltage-dependent Sodium Currents. J. Biol. Chem. 293, 16546–16558. doi:10.1074/jbc.RA118.004929
Gonca, E., and Darıcı, F. (2015). The Effect of Cannabidiol on Ischemia/reperfusion-Induced Ventricular Arrhythmias: the Role of Adenosine A1 Receptors. J. Cardiovasc. Pharmacol. Ther. 20, 76–83. doi:10.1177/1074248414532013
Hill, A. J., Williams, C. M., Whalley, B. J., and Stephens, G. J. (2012). Phytocannabinoids as Novel Therapeutic Agents in CNS Disorders. Pharmacol. Ther. 133, 79–97. doi:10.1016/j.pharmthera.2011.09.002
Hillard, C. J., Harris, R. A., and Bloom, A. S. (1985). Effects of the Cannabinoids on Physical Properties of Brain Membranes and Phospholipid Vesicles: Fluorescence Studies. J. Pharmacol. Exp. Ther. 232, 579–588.
Jackson, S. N., Singhal, S. K., Woods, A. S., Morales, M., Shippenberg, T., Zhang, L., et al. (2008). Volatile Anesthetics and Endogenous Cannabinoid Anandamide Have Additive and Independent Inhibitory Effects on Alpha(7)-Nicotinic Acetylcholine Receptor-Mediated Responses in Xenopus Oocytes. Eur. J. Pharmacol. 582, 42–51. doi:10.1016/j.ejphar.2007.12.023
Kicman, A., and Toczek, M. (2020). The Effects of Cannabidiol, a Non-intoxicating Compound of Cannabis, on the Cardiovascular System in Health and Disease. Int. J. Mol. Sci. 21, 6740. doi:10.3390/ijms21186740
Le Marois, M., Ballet, V., Sanson, C., Maizières, M. A., Carriot, T., Chantoiseau, C., et al. (2020). Cannabidiol Inhibits Multiple Cardiac Ion Channels and Shortens Ventricular Action Potential Duration In Vitro. Eur. J. Pharmacol. 886, 173542. doi:10.1016/j.ejphar.2020.173542
Lodzki, M., Godin, B., Rakou, L., Mechoulam, R., Gallily, R., and Touitou, E. (2003). Cannabidiol-transdermal Delivery and Anti-inflammatory Effect in a Murine Model. J. Control. Release 93, 377–387. doi:10.1016/j.jconrel.2003.09.001
Lundbaek, J. A., Birn, P., Tape, S. E., Toombes, G. E., Søgaard, R., Koeppe, R. E., et al. (2005). Capsaicin Regulates Voltage-dependent Sodium Channels by Altering Lipid Bilayer Elasticity. Mol. Pharmacol. 68, 680–689. doi:10.1124/mol.105.013573
Mahgoub, M., Keun-Hang, S. Y., Sydorenko, V., Ashoor, A., Kabbani, N., Al Kury, L., et al. (2013). Effects of Cannabidiol on the Function of α7-nicotinic Acetylcholine Receptors. Eur. J. Pharmacol. 720, 310–319. doi:10.1016/j.ejphar.2013.10.011
Mechoulam, R., and Hanus, L. (2002). Cannabidiol: an Overview of Some Chemical and Pharmacological Aspects. Part I: Chemical Aspects. Chem. Phys. Lipids 121, 35–43. doi:10.1016/s0009-3084(02)00144-5
Nebrisi, E. E., Prytkova, T., Lorke, D. E., Howarth, L., Alzaabi, A. H., Yang, K. S., et al. (2020). Capsaicin Is a Negative Allosteric Modulator of the 5-HT3 Receptor. Front. Pharmacol. 11, 1274. doi:10.3389/fphar.2020.01274
Orvos, P., Pászti, B., Topal, L., Gazdag, P., Prorok, J., Polyák, A., et al. (2020). The Electrophysiological Effect of Cannabidiol on hERG Current and in guinea-pig and Rabbit Cardiac Preparations. Sci. Rep. 10, 16079. doi:10.1038/s41598-020-73165-2
Oz, M., Ravindran, A., Diaz-Ruiz, O., Zhang, L., and Morales, M. (2003). The Endogenous Cannabinoid Anandamide Inhibits Alpha7 Nicotinic Acetylcholine Receptor-Mediated Responses in Xenopus Oocytes. J. Pharmacol. Exp. Ther. 306, 1003–1010. doi:10.1124/jpet.103.049981
Oz, M. (2006). Receptor-independent Actions of Cannabinoids on Cell Membranes: Focus on Endocannabinoids. Pharmacol. Ther. 111, 114–144. doi:10.1016/j.pharmthera.2005.09.009
Pertwee, R. G., Howlett, A. C., Abood, M. E., Alexander, S. P., Di Marzo, V., Elphick, M. R., et al. (2010). International Union of Basic and Clinical Pharmacology. LXXIX. Cannabinoid Receptors and Their Ligands: beyond CB₁ and CB₂. Pharmacol. Rev. 62, 588–631. doi:10.1124/pr.110.003004
Puglisi, J. L., and Bers, D. M. (2001). LabHEART: an Interactive Computer Model of Rabbit Ventricular Myocyte Ion Channels and Ca Transport. Am. J. Physiol. Cel. Physiol. 281, C2049–C2060. doi:10.1152/ajpcell.2001.281.6.C2049
Rose, J., Armoundas, A. A., Tian, Y., Disilvestre, D., Burysek, M., Halperin, V., et al. (2005). Molecular Correlates of Altered Expression of Potassium Currents in Failing Rabbit Myocardium. Am. J. Physiol. Heart Circ. Physiol. 288, H2077–H2087. doi:10.1152/ajpheart.00526.2003
Ross, H. R., Napier, I., and Connor, M. (2008). Inhibition of Recombinant Human T-type Calcium Channels by Delta9-tetrahydrocannabinol and Cannabidiol. J. Biol. Chem. 283, 16124–16134. doi:10.1074/jbc.M707104200
Sanguinetti, M. C., and Jurkiewicz, N. K. (1990). Two Components of Cardiac Delayed Rectifier K+ Current. Differential Sensitivity to Block by Class III Antiarrhythmic Agents. J. Gen. Physiol. 96, 195–215. doi:10.1085/jgp.96.1.195
Smiley, K. A., Karler, R., and Turkanis, S. A. (1976). Effects of Cannabinoids on the Perfused Rat Heart. Res. Commun. Chem. Pathol. Pharmacol. 14, 659–675.
Spivak, C. E., Lupica, C. R., and Oz, M. (2007). The Endocannabinoid Anandamide Inhibits the Function of Alpha4beta2 Nicotinic Acetylcholine Receptors. Mol. Pharmacol. 72, 1024–1032. doi:10.1124/mol.107.036939
Topal, L., Naveed, M., Orvos, P., Pászti, B., Prorok, J., Bajtel, Á., et al. (2021). The Electrophysiological Effects of Cannabidiol on Action Potentials and Transmembrane Potassium Currents in Rabbit and Dog Cardiac Ventricular Preparations. Arch. Toxicol. 95, 2497–2505. doi:10.1007/s00204-021-03086-0
Varvel, S. A., Wiley, J. L., Yang, R., Bridgen, D. T., Long, K., Lichtman, A. H., et al. (2006). Interactions between THC and Cannabidiol in Mouse Models of Cannabinoid Activity. Psychopharmacology (Berl) 186, 226–234. doi:10.1007/s00213-006-0356-9
Vitale, R. M., Iannotti, F. A., and Amodeo, P. (2021). The (Poly)Pharmacology of Cannabidiol in Neurological and Neuropsychiatric Disorders: Molecular Mechanisms and Targets. Int. J. Mol. Sci. 22, 4876. doi:10.3390/ijms22094876
Walsh, S. K., Hepburn, C. Y., Kane, K. A., and Wainwright, C. L. (2010). Acute Administration of Cannabidiol In Vivo Suppresses Ischaemia-Induced Cardiac Arrhythmias and Reduces Infarct Size when Given at Reperfusion. Br. J. Pharmacol. 160, 1234–1242. doi:10.1111/j.1476-5381.2010.00755.x
Yang, K. H., Galadari, S., Isaev, D., Petroianu, G., Shippenberg, T. S., and Oz, M. (2010). The Nonpsychoactive Cannabinoid Cannabidiol Inhibits 5-hydroxytryptamine3A Receptor-Mediated Currents in Xenopus laevis Oocytes. J. Pharmacol. Exp. Ther. 333, 547–554. doi:10.1124/jpet.109.162594
Keywords: cannabidiol, cannabinoid, ion channels, rabbit, ventricular myocytes
Citation: Isaev D, Shabbir W, Dinc EY, Lorke DE, Petroianu G and Oz M (2022) Cannabidiol Inhibits Multiple Ion Channels in Rabbit Ventricular Cardiomyocytes. Front. Pharmacol. 13:821758. doi: 10.3389/fphar.2022.821758
Received: 24 November 2021; Accepted: 04 January 2022;
Published: 03 February 2022.
Edited by:
Maria Cristina D'Adamo, LUM GiuseppeDeGennaro University, ItalyReviewed by:
Anna Bukiya, University of Tennessee Health Science Center (UTHSC), United StatesCopyright © 2022 Isaev, Shabbir, Dinc, Lorke, Petroianu and Oz. This is an open-access article distributed under the terms of the Creative Commons Attribution License (CC BY). The use, distribution or reproduction in other forums is permitted, provided the original author(s) and the copyright owner(s) are credited and that the original publication in this journal is cited, in accordance with accepted academic practice. No use, distribution or reproduction is permitted which does not comply with these terms.
*Correspondence: Murat Oz, YWhtZXQub3pAa3UuZWR1Lmt3
Disclaimer: All claims expressed in this article are solely those of the authors and do not necessarily represent those of their affiliated organizations, or those of the publisher, the editors and the reviewers. Any product that may be evaluated in this article or claim that may be made by its manufacturer is not guaranteed or endorsed by the publisher.
Research integrity at Frontiers
Learn more about the work of our research integrity team to safeguard the quality of each article we publish.