- Department of Nephrology, Shanghai Municipal Hospital of Traditional Chinese Medicine, Shanghai University of Traditional Chinese Medicine, Shanghai, China
As an increasing public health concern worldwide, acute kidney injury (AKI) is characterized by rapid deterioration of kidney function. Although continuous renal replacement therapy (CRRT) could be used to treat severe AKI, effective drug treatment methods for AKI are largely lacking. Tetramethylpyrazine (TMP) is an active ingredient of Chinese herb Ligusticum wallichii (Chuan Xiong) with antioxidant and anti-inflammatory functions. In recent years, more and more clinical and experimental studies suggest that TMP might effectively prevent AKI. The present article reviews the potential mechanisms of TMP against AKI. Through search and review, a total of 23 studies were finally included. Our results indicate that the undergoing mechanisms of TMP preventing AKI are mainly related to reducing oxidative stress injury, inhibiting inflammation, preventing apoptosis of intrinsic renal cells, and regulating autophagy. Meanwhile, given that AKI and chronic kidney disease (CKD) are very tightly linked by each other, and AKI is also an important inducement of CKD, we thus summarized the potential of TMP impeding the progression of CKD through anti-renal fibrosis.
Introduction
Acute kidney injury (AKI) is characterized by an abrupt loss of renal function, mainly manifested by increased serum creatinine (sCr) levels and decreased urine output. The duration of AKI is generally less than 7 days, and the functional criteria are: increase in sCr by ≥50% within 7 days or increase in sCr by ≥ 0.3 mg/dl (26.5 μmol/L) within 2 days or oliguria for ≥6 h (Khwaja, 2012). A meta-analysis combined research data from 3,585,911 people from most areas north of the equator. The results reported that the combined morbidity and related mortality of AKI in adults were 21.6% and 23.9%, respectively, and 33.7% and 13.8% in children, respectively (Susantitaphong et al., 2013). Due to different medical resources, the cause and incidence of AKI vary greatly among different countries. In high-income countries, AKI is mostly hospital-acquired, mainly in elderly patients with multiple organ failure. In low- and middle-income countries, AKI mainly occurs as a complication of a single disease, and approximately 77% of AKI is community-acquired (Mehta et al., 2015; Hoste et al., 2018). The global burden of AKI-related mortality has exceeded the burden of breast cancer, heart failure or diabetes, and its medical burden is increasing (Lewington et al., 2013). In addition, AKI is associated with progressive chronic kidney disease (CKD) and the following end-stage renal disease (ESRD), which further aggravates the harm of AKI. Many studies have reported some chemical and biological agents have beneficial effects on AKI but there is still a lack of accepted therapeutic drugs so far (Yang et al., 2016; Ronco et al., 2019). AKI patients not only have an increased risk of recent mortality and cardiovascular events, but also have a long-term risk of CKD (See et al., 2019). After the occurrence of AKI, if the kidney tissue is repaired excessively, repaired incompletely, or the damage persists, it might lead to renal dysfunction and renal fibrosis. The progression of AKI to CKD is a complex process involving the regulation of multiple cells and multiple signaling pathways, such as inflammatory damage, G2/M cell cycle arrest, oxidative stress and apoptosis, and these processes ultimately lead to or aggravate renal fibrosis (Vernon et al., 2010; He et al., 2017; Liu et al., 2017; Dong et al., 2018).
Tetramethylpyrazine (ligustrazine, TMP) is the active ingredient and characteristic alkaloid of the Chinese herbal medicine Ligusticum wallichii (Chuan Xiong) (Figure 1). TMP has the effects of inhibiting platelet aggregation, reducing blood viscosity, increasing coronary flow, scavenging free radicals, protecting cerebral vessels, and expanding renal vessels (Zou et al., 2018). The pyrazine ring on the TMP molecule is the key group for its pharmacological effect, but the methyl group in its side chain is easily excreted by oxidative metabolism, which leads to the short half-life of TMP and weakens its pharmacological effect (Wang et al., 2019). Pharmacokinetic studies have shown that after oral or intravenous injection, TMP is mainly distributed in tissues such as liver, brain, kidney, and small intestine, and is eventually excreted from urine through the kidney (Lou et al., 1986; Pan et al., 2021). In view of its anti-oxidative and anti-inflammatory effects, TMP is widely used in cardiovascular and cerebrovascular diseases (Zhao et al., 2016). To date, many studies have focused on the benefits of TMP in a variety of animal or cell models of AKI (Li et al., 2019). Through years of exploration, our team has also confirmed that TMP and Chinese herbal formulas containing Chuan Xiong have an intervention effect in AKI caused by contrast mediums (Gong, 2018; Norgren and Gong, 2018; Gong, 2020). TMP also could function as anti-renal fibrosis and be used in clinical to treat renal fibrosis and CKD. Although there are many reports on TMP effects in AKI, no systematic summary is available. Based on the evaluation of the evidence supporting this hypothesis, we mainly reviewed the therapeutic effects and mechanisms of TMP on AKI. Considering the close relationship between AKI and CKD, the present study also briefly summarized the effects of TMP on renal fibrosis and CKD.
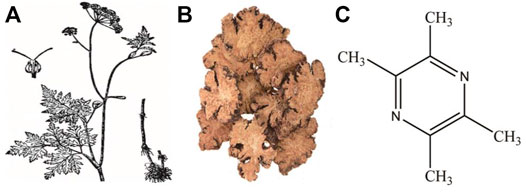
FIGURE 1. Illustration of Ligusticum wallichii plant (A), decoction pieces (B) and chemical structure of TMP (C).
Categories and Pathology of AKI
Although the cause of the disease is extremely complex, AKI is usually regarded as a single disease. Generally, it is divided into three categories based on anatomical location: pre-renal, intrinsic, and post-renal. In recent years, this simple classification method of AKI has been replaced by more specific etiological categories, since different etiologies often mean different pathological mechanisms (Bellomo et al., 2012). Related causes in the latter etiological categories include drugs, sepsis, toxins, cardiorenal, obstruction, hepatorenal, and renal hypoperfusion (Figure 2) (Privratsky et al., 2018; Huang et al., 2019; Jentzer et al., 2020; Simonetto et al., 2020; Molema et al., 2021). In terms of pathological manifestations, AKI is generally described as damage to renal tubular epithelial cells and vascular system (Linkermann et al., 2014; Sancho-Martínez et al., 2015). Due to pathological factors, a variety of stresses occur in AKI, including hypoxia, nutrient deprivation, energy consumption, oxidative damage, genotoxic stress, and endoplasmic reticulum stress. These stresses eventually affect renal tubular epithelial cells by causing oxidative stress damage, inflammation, necrosis, mitochondrial dysfunction, apoptosis, and autophagy (Sureshbabu et al., 2015; Cybulsky 2017; Kimura et al., 2017). Renal hypoperfusion is due to the lack of oxygen and nutrition in the nephrons, which activates the damage and death of epithelial cells through necrosis or apoptosis, ultimately leading to endothelial injury, inflammatory activation, and renal dysfunction (Makris and Spanou 2016). Nephrotoxic drugs and toxins have direct cytotoxic effects on renal tubular epithelial cells and endothelial cells. In addition, they impair hemodynamics and deposit metabolites (Yatim and Oberbarnscheidt, 2015; Wu and Huang, 2018). In sepsis, the reduction of effective circulating blood volume leads to a reduction in renal blood flow and oxygen delivery. Simultaneously, it is accompanied by immune inflammation and activation of the coagulation cascade (Peerapornratana et al., 2019). Although the mechanisms of renal hypoperfusion, nephrotoxic drugs, sepsis, and other causes of AKI are different, they all involve the pathophysiological links of hemodynamic changes, oxidative stress injury, and inflammation.
Methods
This study required a systematic search of electronic databases to identify studies to determine the renal protective effect of TMP on AKI. The search was performed using PubMed and Embase. The following combination of terms were used as search keywords: “Tetramethylpyrazine” OR “Ligustrazine” AND “kidney injury” OR “Renal Injury” OR “Nephrotoxicity” OR “Renal ischemia.” The specified exclusion criteria included: a) case reports, clinical studies, case series, editorials, and reviews; b) research on tetramethylpyrazine derivatives; and c) articles not written in English. A summary of the literature search process is presented in Figure 3.
Results
Studies Characteristics and Mechanism
In total, 98 potentially relevant studies were screened. Ultimately, 23 experimental studies met the inclusion and exclusion criteria (Table 1). In Table 1, there are 17 in vivo studies, 2 in vitro studies, and four both. Of the 23 studies, 12 were on nephrotoxic drugs and toxins, seven on ischemia-reperfusion, two on contrast mediums, one on sepsis, and one on severe burns. These studies involve the use of TMP, including oral, intravenous, and intraperitoneal injections. Based on the results of these studies, TMP had a therapeutic effect on AKI caused by a variety of etiologies. In terms of mechanism, TMP could alleviate AKI by reducing oxidative stress, inflammation, mitochondrial and other organelle damage, or affecting cytoprotective mechanisms such as autophagy or apoptosis (Figure 4). The target highlighted by the red dashed line in the figure represents the key link for TMP to exert its effect. These mechanisms are described in detail below.
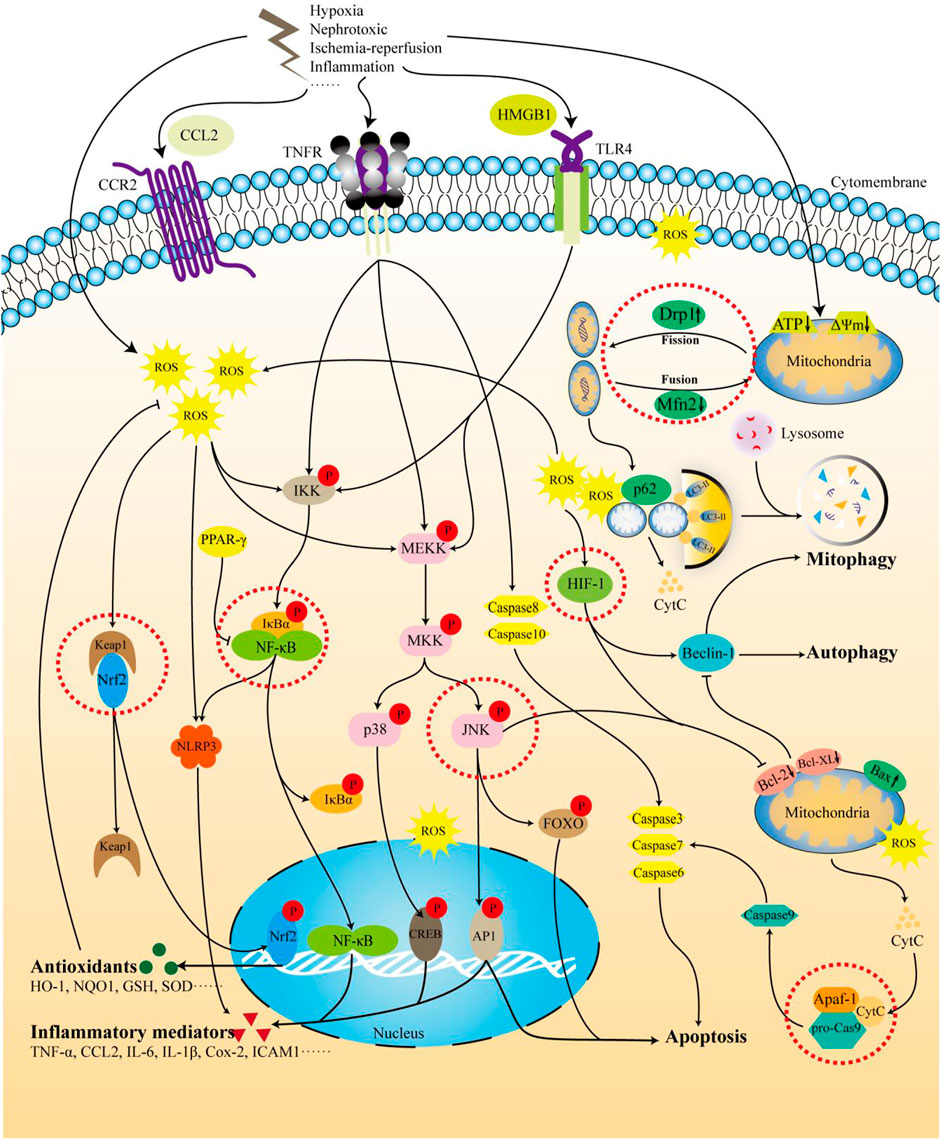
FIGURE 4. The mechanism of TMP intervention in AKI. The figure summarizes the molecular pathways of TMP treatment of AKI involved in this review. Receptors such as TNR, TLR, and CCR2 are stimulated by nephrotoxic drugs, LPS, I/R, and inflammatory factors. In addition, hypoxia and I/R can also directly affect the mitochondrial quality control process and membrane potential, leading to the generation of ROS. The activation of the above receptors and the production of intracellular ROS can activate downstream pathways, further triggering inflammation, apoptosis, and autophagy, and ultimately leading to kidney damage. TMP can target Nfr2 and HIF-1 to activate the expression of antioxidant factors and enhance cell tolerance to oxidative stress. TMP can also inhibit TLR4 and TNFR or, by activating PPAR-γ, further inhibit the NF-κB pathway and reduce inflammation. In addition to the targeted inhibition of caspase-8/3/6/7 through the TNFR pathway, TMP can also affect mitochondrial-related apoptosis by inhibiting the ERK/JNK pathway. There is still controversy regarding the regulation of autophagy by TMP. It is generally believed that TMP activates the autophagy process and eliminates damaged mitochondria by targeting mitochondrial quality control, ultimately reducing cell damage.
TMP Relieves Oxidative Stress Injury
Oxidative stress reflects a state of imbalance between the formation of reactive oxygen and nitrogen and antioxidant system. Oxidative stress occurs when the production of pro-oxidants or reactive oxygen species (ROS) exceeds the endogenous antioxidant capacity (Sies et al., 2017). ROS are several active molecules and free radicals derived from molecular oxygen, including superoxide anions (O2−) and hydroxyl radicals (OH). At high concentrations, ROS can be toxic to macromolecules, including lipids, proteins, and DNA, leading to the destruction of the integrity and capacity of the cell structure (Davies, 1987; Sies, 1997). Oxidative stress is an important pathological mechanism of AKI caused by various etiologies. In AKI induced by ischemia-reperfusion injury, sepsis, and contrast mediums, changes in renal hemodynamics can lead to increased ROS production. In the hypoxic state, electron transfer in the mitochondrial respiratory chain is obstructed, causing electron leakage. The leaked electrons combine with oxygen to generate a large amount of active oxygen (Granata et al., 2015; Kusirisin et al., 2020). Cisplatin and aminoglycoside drugs can induce mitochondrial dysfunction and increase the production of ROS and can also react with thiol-containing molecules, including glutathione (GSH). The consumption or inactivation of GSH and related antioxidants leads to the intracellular accumulation of endogenous ROS (Kruidering et al., 1997; Sureshbabu et al., 2015). Other studies have also shown that oxidative stress plays a key role in the development of AKI. For example, in a mouse model of renal I/R injury, heme oxygenase-1 knockout (HO-1−/−) mice were found to be more sensitive to I/R injury, while increasing the incidence of renal injury and mortality rate (Tracz et al., 2007). Oxidative stress further leads to downstream effects such as inflammatory damage, necrosis, and apoptosis. During oxidative stress, TMP mainly inhibits ROS generation and activates the antioxidant system. Liu et al. studied the protective effect of TMP on cisplatin-induced nephrotoxicity in rats, using ligustrazine for 7 consecutive days of intraperitoneal injection, starting from 2 days before a single intravenous injection of cisplatin. The results showed that cisplatin increased the levels of MDA, NOS, and NO, while the levels of GSH, GST, and SOD decreased. These changes were reversed by TMP treatment (Liu et al., 2008). Nrf2 is an important regulator of the antioxidant system that can neutralize the activation of cellular oxidative stress. Under basic conditions, the Keap1/Nrf2 complex is easily degraded by ubiquitination. However, under oxidative stress conditions, Keap1 is oxidized, and Nrf2 is introduced into the nucleus and binds to the antioxidant response element in the gene promoter region to initiate the transcription of a series of antioxidant factors (Saito, 2013; Suzuki and Yamamoto, 2015). Michel et al. found that TMP pretreatment significantly activated the Nrf2 defense pathway in rats with nephrotoxicity induced by cisplatin indicated by the increase in levels of Nrf2 and downstream antioxidant enzymes such as HO-1 and NQO1 in the kidney. This also shows that TMP inhibits cisplatin-induced oxidative stress by activating the Nrf2 defense mechanism (Michel and Menze, 2019). However, the regulation of Nrf2 and HO-1 signals by TMP is obviously complex. For example, as a response biomarker for arsenic exposure in various types of cells, HO-1 was observed downregulated by TMP pretreatment in arsenic-induced nephrotoxicity cell model, so did Nrf2 (Gong et al., 2016). We speculated that the reasons for the above contradictory results are multifaceted and complicated. The protective effect of Nrf2 in the kidney is affected by its activation degree and duration, and there might be a delicate balance. Studies have shown that in mice with renal tubule-specific knockout of Keap1, moderate activation of Nrf2 might reduce the damage caused by ischemia or nephrotoxic substances, while excessive and continuous activation of Nrf2 loses this protective effect (Noel et al., 2016; Tan et al., 2016; Nezu et al., 2017). Moreover, the transcription of the HO-1 gene is complicated and might not only be regulated by Nrf2. For example, sodium arsenite has been shown to cause BACH1-specific HO-1 induction independent of Nrf2 (Reichard et al., 2016). Additionally, there is a functional κB element in the promoter of mouse HO-1 gene, which might be the mechanism of HO-1 upregulation in vivo mediated by NF-κB subunits p50 and p65 (Li et al., 2009). Our previously data indicated clearly that arsenic-induced HO-1 expression is mediated by multiple pathways, and the corresponding transcription factors includes Nrf2, NF-κB AP-1, p38 MAPK, and JNK (but not ERK) (Gong et al., 2016). As an organ rich in mitochondria, kidney is very susceptible to oxidative stress mediated damage, thus reducing mitochondrial-derived ROS might be another important way to protect kidney against oxidative stress injury (Gorin, 2016). Our previous study also found that TMP could improve abnormal mitochondrial dynamics and regulate mitochondrial damage in contrast-induced nephropathy (CIN) (Gong et al., 2019). In addition, oxidative stress also interacts with a variety of pathological processes in the AKI process, including inflammation and apoptosis, which are discussed below. Therefore, TMP has the therapeutic potential of antagonizing oxidative stress in AKI caused by various etiologies.
TMP Improves Inflammation
Inflammation is a physiological process that protects the body from acute damages such as ischemia, pathogens, or toxins. Inflammation is believed to play an important role in the pathogenesis of AKI. Basically, all immune cells, such as neutrophils, monocytes/macrophages, and NK cells are involved in the pathogenesis of AKI to varying degrees (Rabb et al., 2016). Activation of the inflammatory process in AKI is caused by multiple pathways. In models of ischemia, sepsis, and nephrotoxicity, the initial damage occurs in the tubular epithelium and vascular endothelial cells (Akcay et al., 2009). The above-mentioned damage induces the production of inflammatory mediators such as inflammatory factors, chemokines, and adhesion factors (TNF-α, TGF-β, IL-6, IL-1β, IL-18, CCL2, MCP-1, ICAM-1, and P-selectin), which help recruit leukocytes to the kidney. Neutrophils, macrophages, and lymphocytes infiltrate the injury site (McWilliam et al., 2021). In addition, oxidative stress can promote inflammation, and cell damage caused by inflammation further aggravates oxidative stress (Tucker et al., 2015). In the tetracycline-induced AKI rat model, the use of mitochondrial-targeted antioxidants significantly reduced the accumulation of dendritic cells and T cells in the kidney tissue, suggesting that mitochondrial-derived ROS are involved in antigen presentation and T-cell activation (Gentle et al., 2013). Under oxidative stress, NADPH oxidase (NOX) can interact with Toll-like receptor 4 (TLP4) to directly activate the nuclear transcription factor NF-κB pathway, leading to an increase in the transcription of downstream inflammatory mediators and further increasing inflammation (El-Benna et al., 2016). Most of the studies in Table 1 show an inhibitory effect on the level of inflammatory mediators, and the regulation of the NF-κB pathway is the key to TMP. NLRP3 is a member of the nucleotide-binding oligomerization domain-like receptor protein family (NLRPs) and is a common inflammasome. It promotes the maturation of the pro-inflammatory factors IL-1β and IL-18 by activating caspase-1 (Liston and Masters, 2017; Shi et al., 2017). The expression of signal sensing receptors such as TLRs and TNFRs and downstream gene expression proteins such as NF-κB and ROS is involved in the activation of NLRP3 (Xue et al., 2019). Many studies have shown that the NLRP3 inflammasome and its downstream apoptosis and inflammation play important roles in the occurrence and development of AKI (Bakker et al., 2014; Shen et al., 2016). Sun et al. explored the protective effect of TMP on renal ischemia-reperfusion injury in rats and its potential mechanism. The expression level of NLRP3 in NRK-52E cells increased after hypoxia and glucose deprivation, and decreased significantly after TMP treatment (Sun et al., 2020). As an important member of the CC subfamily of chemokines, CCL2 is also called monocyte chemotactic protein-1 (MCP-1). CCL2 is formed under pathological conditions such as pro-inflammatory stimuli (IL-8, TNF-α, and LPS stimulus). It usually binds to the extracellular specific ligand CCR2 to mediate the migration and activation of a variety of inflammatory cells (Kawaguchi-Niida et al., 2013). Our previous study found that the abundance of CCL2 and CCR2 in the renal tubules of rats with contrast-induced nephropathy (CIN) increased, accompanied by an increase in the concentration of IL-6 and TNF-α in the kidney and serum, and TMP could inhibit the CCL2/CCR2 pathway activation (Gong et al., 2019). The peroxisome proliferator-activated receptor (PPAR) is a member of the superfamily of nuclear transcription factors activated by ligands (Wu et al., 2018). PPAR-γ can inhibit the inflammatory response by competing with the inflammatory signaling pathway and the production of inflammatory mediators such as activator protein-1 (AP-1) and NF-κB (Ju et al., 2020). Studies have found that PPAR-γ expression is significantly reduced in cisplatin-induced acute kidney injury in rats, and TMP administration can significantly improve this change (Michel and Menze, 2019). In summary, TMP is a promising anti-inflammatory agent for treating AKI.
TMP Inhibits Apoptosis
Apoptosis refers to the biochemical process of cell breakdown by a set of specific proteins that interact with each other and program death-inducing signals. Unlike necrosis, apoptosis does not cause inflammation (Kønig et al., 2019). When a cell receives an apoptosis signal, it activates the initial caspases through different signaling pathways, reactivates the effector caspases, and degrades related substrates, eventually leading to cell apoptosis (Chota et al., 2021). Since there are many apoptotic signaling pathways, the upstream regulation of caspases is also different. Bcl-2 family molecules are involved in upstream regulatory pathways for the reception and transmission of apoptosis signals. They mainly regulate apoptosis via the mitochondrial pathway. When pro-apoptotic proteins receive apoptosis signals, they can release cytochrome C (Cytc) from the mitochondria to activate downstream caspases, then causing apoptosis (Singh et al., 2019). The permeability of the mitochondrial membrane is regulated by Bcl-2 family proteins. In renal epithelial cells, Bcl-2 members Bax and Bak cause an increase in membrane permeability, while Bcl-2 and Bcl-XL antagonize this “membrane attack” effect (Youle and Strasser, 2008). Intrarenal stress and ischemia both increase the ratio of Bax/Bcl2, which is the main determinant of cell death (Chien et al., 2005; Liu and Baliga, 2005). In most AKI models, the adjustment effect of TMP on the ratio of Bax/Bcl2 has been confirmed in many studies. Juan et al. showed that gentamicin significantly induced apoptosis in NRK-52E cells in a dose-dependent manner. TMP pretreatment can inactivate the activities of caspase-3, caspase-8, and caspase-9 stimulated by gentamicin, inhibit the release of Cytc, and increase the expression of Bcl-XL (Juan et al., 2007). Although renal tubular cell apoptosis is often reported in various AKI models, the upstream signaling pathways leading to apoptosis may be different (Havasi and Borkan, 2011; Linkermann et al., 2013). Although there are different initiation mechanisms, most apoptotic pathways cluster on the mitochondria. The endogenous mitochondrial apoptotic pathway begins with oxidative stress. ROS and other stress products enter the mitochondria with the Bax/Bcl-2 protein complex, promote the increase in mitochondrial permeability with other pro-apoptotic genes, and then release Cytc (Galluzzi et al., 2018). Therefore, the anti-oxidative stress ability of TMP can regulate the mitochondrial apoptosis pathway from the source. There is also a close relationship between apoptosis and mitochondrial dynamics. Previous studies have shown that in the early stage of apoptosis, Bax is transferred from the cytoplasm to the mitochondria before the caspases are activated, and, at the same time, dynein-related protein 1 (DRP1) is also transferred from the cytoplasm to the mitochondrial division site and then mediates mitochondrial division (Suen et al., 2008). Inhibiting the activity of Drp1 not only inhibits mitochondrial division but also inhibits the activation and apoptosis of caspases (Hoppins and Nunnari, 2012). In addition, high expression of mitochondrial outer membrane fusion proteins Mfn1 and Mfn2 can also inhibit apoptosis (Jian et al., 2018). Our previous found that TMP could improve abnormal mitochondrial dynamics by upregulating Mfn2 and downregulating Drp1 and alleviating the apoptosis of tubule epithelial cells caused by contrast agents (Gong et al., 2019). In addition, the external pathway of apoptosis mediated by TNFR may also be involved in renal tubular cell apoptosis in ischemic and septic AKI (Cunningham et al., 2002; Linkermann et al., 2014). TNFR knockout mice are resistant to cisplatin-induced AKI, supporting this pathogenesis (Ramesh and Reeves, 2004). TMP can simultaneously regulate the upstream ligand (TNF-α) and downstream signaling pathways (JNK and NF-κB) of the TNFR-mediated apoptosis pathway.
TMP Adjusts Autophagy
Autophagy is a process in which a double-membrane autophagosome encapsulates cytoplasm, organelles, and protein polymers and is transported to lysosomes for catabolism (Youle and Narendra, 2011). Under normal physiological conditions, low levels of basal autophagy maintain cell homeostasis by removing damaged proteins and organelles. The autophagy pathway is upregulated in stress states such as cell starvation, hypoxia, and endoplasmic reticulum stress (Feng et al., 2014). Autophagy is non-selective, but it can also selectively degrade damaged organelles such as mitophagy to clear damaged mitochondria. The formation of autophagosomes depends on the coordination of autophagy-related proteins, which mainly include the ULK1/2 complex, Beclin-1/class III PI3K complex, and autophagy-related genes (ATG). LC3-II is located in pre-autophagosomes and autophagosomes, and its level increases with the increase in autophagosome membranes. The Beclin-1/class III PI3K complex promotes the nucleation of autophagosomes on the phagocytic vesicle membrane. Both LC3-II and Beclin-1 are markers for autophagy detection (Dancourt and Melia, 2014). There are many reports on the link between AKI and autophagy, most of which indicate the protective effect of autophagy on AKI. Studies have found that the expression of LC3 in proximal tubule cells of ATG5-deficient mice after renal I/R injury is inhibited, suggesting that basic autophagy has a protective effect against renal injury caused by I/R injury (Kimura et al., 2011). In the CI-AKI rat and cell models established with iohexol, it was found that the expression of autophagy marker LC3-II in renal tubular epithelial cells increased, the mitochondrial damage of renal tubular cells increased after the use of autophagy inhibitors, and apoptosis increased (Ko et al., 2016). Although most studies have found that autophagy activated in renal tubular epithelial cells of various AKI plays a protective role, a few studies have suggested that autophagy aggravates cell damage in AKI. Chen et al. found that TMP could reduce renal I/R damage by enhancing autophagy, indicated by increased LC3-II/I ratio and Beclin-1 in kidney tissue (Chen et al., 2020). Another study found that TMP reduced inflammation in renal I/R injury and was related to the activation of autophagy (Jiang et al., 2020). Interestingly, in a study on CI-AKI, we found that the mechanism by which TMP protected the kidney from contrast agent damage was partly related to the inhibition of autophagy (Gong et al., 2019). The reason for this apparently contradictory result may be related to the different AKI models. Some studies have reported that autophagy induces cell metabolism imbalance and induces cell death in renal tubular epithelial cells induced by contrast agents, and this result can be attenuated by curcumin (Buyuklu et al., 2014). This shows that the role of autophagy in AKI is still controversial. As an upstream regulator of autophagy induction, ROS not only induces autophagy through the mitochondrial pathway but also induces mitophagy through the signaling pathway mediated by HIF-1 (Scherz-Shouval and Elazar, 2007; Zhang et al., 2008). The regulation of oxidative stress and HIF-1 by TMP is also one of the ways to regulate autophagy. In addition, there is an interaction between autophagy and apoptosis. In response to stress such as hypoxia, autophagy can prevent cells from triggering the apoptotic pathway by degrading misfolded proteins and damaged organelles. The inhibitory effect of Bcl-2 family proteins on autophagy in renal tubular cells has been confirmed in many experiments. In Bcl-2/GFP-LC3 transgenic mice, autophagy induced by ischemia-reperfusion was attenuated (Isaka et al., 2009). Studies have shown that enhancing the expression of Bcl-XL in the kidney is sufficient to inhibit autophagy induction and apoptosis (Chien et al., 2007). Regarding the mechanism by which Bcl-2 downregulates autophagy, it is generally believed that Bcl-2 family proteins bind Beclin-1 through the BH3 domain, blocking the necessary process of autophagosome formation. The details of the simultaneous regulation of autophagy and apoptosis by TMP are still unclear, and this may be a promising research direction. In short, the mechanism by which TMP interferes with autophagy in AKI is unclear, and there are still controversies.
The Potential of TMP Prevents CKD and Renal Fibrosis
AKI and CKD are very tightly linked by each other. Many studies suggested that AKI is also an important inducement of chronic kidney disease (CKD) (Horne et al., 2017; See et al., 2019). There are many published data of TMP against CKD as well as renal fibrosis. In China, several TMP injections have been used to treat CKD in clinical, especially in diabetic nephropathy patients (Wang et al., 2012). Cao et al. reported that TMP had an inhibitory effect on the proliferation of human renal interstitial fibroblasts in a time- and concentration-dependent manner (Cao et al., 2006). Unilateral ureteral obstruction (UUO) model is a classic model for studying renal fibrosis, Yuan et al. reported that TMP treatment could reduce the score of interstitial collagen deposition, the density of macrophages, and the mRNA expressions of TGF-β1 and CTGF in this rat model (Yuan et al., 2012). The matrix accumulation caused by the reduction of the ratio of MMPS/TIMPS is the basic pathophysiological process of renal interstitial fibrosis (Kelly et al., 2010). Studies had found that TMP could inhibit the high expression of TIMP-1 and the imbalance of MMP-9/TIMP-1 ratio in UUO model rats, and thereby slow the progression of renal fibrosis (Li et al., 2017). TGF-β/Smad3 is the main pathway of renal fibrosis, and Smad7 could block the phosphorylation of Smad3, thereby limiting the effect of TGF-β (Meng et al., 2016; Chen et al., 2018). The results of Lu et al. showed that TMP could reduce the content of TGF-β1 in kidney tissue and restore the expression levels of Smad reverse regulators Smad7 and SnoN protein (Lu et al., 2009). In addition, aristolochic acid is very toxic to kidney, which would cause tubulointerstitial damage and renal fibrosis, and TMP has been reported to reduce the kidney damage caused by aristolochic acid in rats (Wang et al., 2006).
Conclusion and Perspective
Considering the importance of oxidative stress and inflammation in AKI, the application of TMP in AKI treatment deserves attention. The present study mainly focuses on the experimental research of TMP in preventing AKI, and aims to synthesize the current knowledge in this field, concurrently, this study also briefly sums up the effects of TMP against renal fibrosis and CKD. Based on the collected data, TMP not only improves kidney function, reduces the level of kidney injury markers (including kim-1, CysC, UNAG, and UGGT), but also decreases the degree of pathological damage in kidney. Although the pathological mechanisms of AKI caused by various factors are different, the preventive effects of TMP against AKI are inseparable from the following four processes: oxidative stress, inflammatory mediators, apoptosis, and autophagy. These data support the potential application of TMP as a new therapeutic drug for AKI. It should be noted that these data mainly are preclinical studies, and the clinical application of TMP in AKI treatment still needs more rigorous clinical research data. As mentioned above, our group has been focusing on the basic experimental research of TMP against CI-AKI for more than 10 years (Gong et al., 2013, 2019; Gong, 2018; Norgren and Gong, 2018), and we hope these basic experimental research data should promote the followed clinical research progress of TMP treating CI-AKI and other types of AKI, not just for anti-renal fibrosis and the treatment of CKD.
Author Contributions
XG and JL designed the work of review. JL and XG reviewed the literature available on this topic and wrote the paper. XG and JL revised the manuscript. All authors approved the paper for publication. As the leader of the project team, XG won the research fundings supporting this manuscript.
Funding
This project is supported by the National Natural Science Foundation of China (No.82074387 and No.81873280) and Shanghai Municipal Science and Technology Commission Project (No.20Y21902200).
Conflict of Interest
The authors declare that the research was conducted in the absence of any commercial or financial relationships that could be construed as a potential conflict of interest.
Publisher’s Note
All claims expressed in this article are solely those of the authors and do not necessarily represent those of their affiliated organizations, or those of the publisher, the editors and the reviewers. Any product that may be evaluated in this article, or claim that may be made by its manufacturer, is not guaranteed or endorsed by the publisher.
Acknowledgments
Meanwhile, the authors would like to thank Zongping Li and Zhiyong Song for advice and helpful discussion.
References
Akcay, A., Nguyen, Q., and Edelstein, C. L. (2009). Mediators of Inflammation in Acute Kidney Injury. Mediators Inflamm. 2009, 137072. doi:10.1155/2009/137072
Ali, B. H., Al-Moundhri, M., Eldin, M. T., Nemmar, A., Al-Siyabi, S., and Annamalai, K. (2008). Amelioration of Cisplatin-Induced Nephrotoxicity in Rats by Tetramethylpyrazine, a Major Constituent of the Chinese Herb Ligusticum Wallichi. Exp. Biol. Med. (Maywood) 233 (7), 891–896. doi:10.3181/0711-RM-315
Ali, B. H., Al-Salam, S., Al-Husseini, I., and Nemmar, A. (2009). Comparative Protective Effect of N-Acetyl Cysteine and Tetramethylpyrazine in Rats with Gentamicin Nephrotoxicity. J. Appl. Toxicol. 29 (4), 302–307. doi:10.1002/jat.1409
Bakker, P. J., Butter, L. M., Claessen, N., Teske, G. J., Sutterwala, F. S., Florquin, S., et al. (2014). A Tissue-specific Role for Nlrp3 in Tubular Epithelial Repair after Renal Ischemia/reperfusion. Am. J. Pathol. 184 (7), 2013–2022. doi:10.1016/j.ajpath.2014.04.005
Bellomo, R., Kellum, J. A., and Ronco, C. (2012). Acute Kidney Injury. Lancet 380 (9843), 756–766. doi:10.1016/S0140-6736(11)61454-2
Buyuklu, M., Kandemir, F. M., Ozkaraca, M., Set, T., Bakirci, E. M., and Topal, E. (2014). Protective Effect of Curcumin against Contrast Induced Nephropathy in Rat Kidney: what Is Happening to Oxidative Stress, Inflammation, Autophagy and Apoptosis? Eur. Rev. Med. Pharmacol. Sci. 18 (4), 461–470. doi:10.1016/j.jep.2013.12.015
Cao, L., Sun, X., Yu, G., Guo, Q., Zhang, X., and Xu, K. (2006). “Effects of Ligustrazine on the Proliferation and Morphology of Human Renal Interstitial Fibroblasts. Phytother Res.26 1936–1937+1942. doi:10.1002/ptr.3630
Chen, J. L., Zhou, T., Chen, W. X., Zhu, J. S., Chen, N. W., Zhang, M. J., et al. (2003). Effect of Tetramethylpyrazine on P-Selectin and Hepatic/renal Ischemia and Reperfusion Injury in Rats. World J. Gastroenterol. 9 (7), 1563–1566. doi:10.3748/wjg.v9.i7.1563
Chen, L., Yang, T., Lu, D. W., Zhao, H., Feng, Y. L., Chen, H., et al. (2018). Central Role of Dysregulation of TGF-β/Smad in CKD Progression and Potential Targets of its Treatment. Biomed. Pharmacother. 101, 670–681. doi:10.1016/j.biopha.2018.02.090
Chen, P., Li, J., Liu, S., and Wei, R. (2020). Tetramethylpyrazine Attenuates Renal Ischemia-Reperfusion Injury in Rats through Inhibiting Inflammation and Oxidative Stress and Enhancing Autophagy. Latin Am. J. Pharm. 39 (6), 1094–1099.
Chien, C. T., Chiang-Ting, C., Chang, T. C., Tsai, C. C. Y., Ching-Yi, T., Shyue, S. K., et al. (2005). Adenovirus-mediated Bcl-2 Gene Transfer Inhibits Renal Ischemia/reperfusion Induced Tubular Oxidative Stress and Apoptosis. Am. J. Transpl. 5 (6), 1194–1203. doi:10.1111/j.1600-6143.2005.00826.x
Chien, C. T., Shyue, S. K., and Lai, M. K. (2007). Bcl-xL Augmentation Potentially Reduces Ischemia/reperfusion Induced Proximal and Distal Tubular Apoptosis and Autophagy. Transplantation 84 (9), 1183–1190. doi:10.1111/j.1600-6143.2005.00826.x10.1097/01.tp.0000287334.38933.e3
Chota, A., George, B. P., and Abrahamse, H. (2021). Interactions of Multidomain Pro-apoptotic and Anti-apoptotic Proteins in Cancer Cell Death. Oncotarget 12 (16), 1615–1626. doi:10.18632/oncotarget.28031
Cunningham, P. N., Dyanov, H. M., Park, P., Wang, J., Newell, K. A., and Quigg, R. J. (2002). Acute Renal Failure in Endotoxemia Is Caused by TNF Acting Directly on TNF Receptor-1 in Kidney. J. Immunol. 168 (11), 5817–5823. doi:10.4049/jimmunol.168.11.5817
Cybulsky, A. V. (2017). Endoplasmic Reticulum Stress, the Unfolded Protein Response and Autophagy in Kidney Diseases. Nat. Rev. Nephrol. 13 (11), 681–696. doi:10.1038/nrneph.2017.129
Dancourt, J., and Melia, T. J. (2014). Lipidation of the Autophagy Proteins LC3 and GABARAP Is a Membrane-Curvature Dependent Process. Autophagy 10 (8), 1470–1471. doi:10.4161/auto.29468
Davies, K. J. (1987). Protein Damage and Degradation by Oxygen Radicals. I. General Aspects. J. Biol. Chem. 262 (20), 9895–9901. doi:10.0000/PMID303687510.1016/s0021-9258(18)48018-0
Dong, W., Li, Z., Chen, Y., Zhang, L., Ye, Z., Liang, H., et al. (2018). Necrostatin-1 Attenuates Sepsis-Associated Acute Kidney Injury by Promoting Autophagosome Elimination in Renal Tubular Epithelial Cells. Mol. Med. Rep. 17 (2), 3194–3199. doi:10.3892/mmr.2017.8214
El-Benna, J., Hurtado-Nedelec, M., Marzaioli, V., Marie, J. C., Gougerot-Pocidalo, M. A., and Dang, P. M. (2016). Priming of the Neutrophil Respiratory Burst: Role in Host Defense and Inflammation. Immunol. Rev. 273 (1), 180–193. doi:10.1111/imr.12447
Feng, L., Ke, N., Cheng, F., Guo, Y., Li, S., Li, Q., et al. (2011). The Protective Mechanism of Ligustrazine against Renal Ischemia/reperfusion Injury. J. Surg. Res. 166 (2), 298–305. doi:10.1016/j.jss.2009.04.005
Feng, L., Xiong, Y., Cheng, F., Zhang, L., Li, S., and Li, Y. (2004). Effect of Ligustrazine on Ischemia-Reperfusion Injury in Murine Kidney. Transpl. Proc 36 (7), 1949–1951. doi:10.1016/j.transproceed.2004.07.050
Feng, Y., He, D., Yao, Z., and Klionsky, D. J. (2014). The Machinery of Macroautophagy. Cell Res 24 (1), 24–41. doi:10.1038/cr.2013.168
Galluzzi, L., Vitale, I., Aaronson, S. A., Abrams, J. M., Adam, D., Agostinis, P., et al. (2018). Molecular Mechanisms of Cell Death: Recommendations of the Nomenclature Committee on Cell Death 2018. Cell Death Differ 25 (3), 486–541. doi:10.1038/s41418-017-0012-4
Gao, C., Peng, H., Wang, S., and Zhang, X. (2012). Effects of Ligustrazine on Pancreatic and Renal Damage after Scald Injury. Burns 38 (1), 102–107. doi:10.1016/j.burns.2011.04.022
Gentle, M. E., Shi, S., Daehn, I., Zhang, T., Qi, H., Yu, L., et al. (2013). Epithelial Cell TGFβ Signaling Induces Acute Tubular Injury and Interstitial Inflammation. J. Am. Soc. Nephrol. 24 (5), 787–799. doi:10.1681/ASN.2012101024
Gong, X., Duan, Y., Zheng, J., Ye, Z., and Hei, T. K. (20192019). Tetramethylpyrazine Prevents Contrast-Induced Nephropathy via Modulating Tubular Cell Mitophagy and Suppressing Mitochondrial Fragmentation, CCL2/CCR2-Mediated Inflammation, and Intestinal Injury. Oxid Med. Cel Longev 2019, 7096912. doi:10.1155/2019/7096912
Gong, X., Ivanov, V. N., Davidson, M. M., and Hei, T. K. (2015). Tetramethylpyrazine (TMP) Protects against Sodium Arsenite-Induced Nephrotoxicity by Suppressing ROS Production, Mitochondrial Dysfunction, Pro-inflammatory Signaling Pathways and Programed Cell Death. Arch. Toxicol. 89 (7), 1057–1070. doi:10.1007/s00204-014-1302-y
Gong, X., Ivanov, V. N., and Hei, T. K. (2016). 2,3,5,6-Tetramethylpyrazine (TMP) Down-Regulated Arsenic-Induced Heme Oxygenase-1 and ARS2 Expression by Inhibiting Nrf2, NF-Κb, AP-1 and MAPK Pathways in Human Proximal Tubular Cells. Arch. Toxicol. 90 (9), 2187–2200. doi:10.1007/s00204-015-1600-z
Gong, X., Wang, Q., Tang, X., Wang, Y., Fu, D., Lu, H., et al. (2013). Tetramethylpyrazine Prevents Contrast-Induced Nephropathy by Inhibiting P38 MAPK and FoxO1 Signaling Pathways. Am. J. Nephrol. 37 (3), 199–207. doi:10.1159/000347033
Gong, X. Z. (2020). Chinese Medicine Might Be A Promising Way for A Solution to Arsenic Nephrotoxicity. Chin. J. Integr. Med. 26 (2), 83–87. doi:10.1007/s11655-019-3210-8
Gong, X. Z. (2018). Recent Advances in Chinese Medicine for Contrast-Induced Nephropathy. Chin. J. Integr. Med. 24 (1), 6–9. doi:10.1007/s11655-017-2906-x
Gorin, Y. (2016). The Kidney: An Organ in the Front Line of Oxidative Stress-Associated Pathologies. Antioxid. Redox Signal. 25 (12), 639–641. doi:10.1089/ars.2016.6804
Granata, S., Dalla Gassa, A., Tomei, P., Lupo, A., and Zaza, G. (2015). Mitochondria: a New Therapeutic Target in Chronic Kidney Disease. Nutr. Metab. (Lond) 12, 49. doi:10.1186/s12986-015-0044-z
Havasi, A., and Borkan, S. C. (2011). Apoptosis and Acute Kidney Injury. Kidney Int. 80 (1), 29–40. doi:10.1038/ki.2011.120
He, L., Wei, Q., Liu, J., Yi, M., Liu, Y., Liu, H., et al. (2017). AKI on CKD: Heightened Injury, Suppressed Repair, and the Underlying Mechanisms. Kidney Int. 92 (5), 1071–1083. doi:10.1016/j.kint.2017.06.030
Hoppins, S., and Nunnari, J. (2012). Cell Biology. Mitochondrial Dynamics and Apoptosis-Tthe ER Connection. Science 337 (6098), 1052–1054. doi:10.1126/science.1224709
Horne, K. L., Packington, R., Monaghan, J., Reilly, T., and Selby, N. M. (2017). Three-year Outcomes after Acute Kidney Injury: Results of a Prospective Parallel Group Cohort Study. BMJ Open 7 (3), e015316. doi:10.1136/bmjopen-2016-015316
Hoste, E. A. J., Kellum, J. A., Selby, N. M., Zarbock, A., Palevsky, P. M., Bagshaw, S. M., et al. (2018). Global Epidemiology and Outcomes of Acute Kidney Injury. Nat. Rev. Nephrol. 14 (10), 607–625. doi:10.1038/s41581-018-0052-0
Huang, J., Li, J., Lyu, Y., Miao, Q., and Pu, K. (2019). Molecular Optical Imaging Probes for Early Diagnosis of Drug-Induced Acute Kidney Injury. Nat. Mater. 18 (10), 1133–1143. doi:10.1038/s41563-019-0378-4
Isaka, Y., Suzuki, C., Abe, T., Okumi, M., Ichimaru, N., Imamura, R., et al. (2009). Bcl-2 Protects Tubular Epithelial Cells from Ischemia/reperfusion Injury by Dual Mechanisms. Transpl. Proc 41 (1), 52–54. doi:10.1016/j.transproceed.2008.10.026
Jentzer, J. C., Bihorac, A., Brusca, S. B., Del Rio-Pertuz, G., Kashani, K., Kazory, A., et al. (2020). Contemporary Management of Severe Acute Kidney Injury and Refractory Cardiorenal Syndrome: JACC Council Perspectives. J. Am. Coll. Cardiol. 76 (9), 1084–1101. doi:10.1016/j.jacc.2020.06.070
Jian, F., Chen, D., Chen, L., Yan, C., Lu, B., Zhu, Y., et al. (2018). Sam50 Regulates PINK1-Parkin-Mediated Mitophagy by Controlling PINK1 Stability and Mitochondrial Morphology. Cell Rep 23 (10), 2989–3005. doi:10.1016/j.celrep.2018.05.015
Jiang, G., Xin, R., Yuan, W., Zhang, L., Meng, X., Sun, W., et al. (2020). Ligustrazine Ameliorates Acute Kidney Injury through Downregulation of NOD2-mediated I-nflammation. Int. J. Mol. Med. 45 (3), 731–742. doi:10.3892/ijmm.2020.4464
Ju, Z., Su, M., Hong, J., Kim, E., and Jung, J. H. (2020). Anti-inflammatory Effects of an Optimized PPAR-γ Agonist via NF-Κb Pathway Inhibition. Bioorg. Chem. 96, 103611. doi:10.1016/j.bioorg.2020.103611
Juan, S. H., Chen, C. H., Hsu, Y. H., Hou, C. C., Chen, T. H., Lin, H., et al. (2007). Tetramethylpyrazine Protects Rat Renal Tubular Cell Apoptosis Induced by Gentamicin. Nephrol. Dial. Transpl. 22 (3), 732–739. doi:10.1093/ndt/gfl699
Kawaguchi-Niida, M., Yamamoto, T., Kato, Y., Inose, Y., and Shibata, N. (2013). MCP-1/CCR2 Signaling-Mediated Astrocytosis Is Accelerated in a Transgenic Mouse Model of SOD1-Mutated Familial ALS. Acta Neuropathol. Commun. 1, 21. doi:10.1186/2051-5960-1-21
Kelly, B. C., Markle, L. S., Vickers, J. L., Petitt, M. S., Raimer, S. S., and McNeese, C. (2010). The Imbalanced Expression of Matrix Metalloproteinases in Nephrogenic Systemic Fibrosis. J. Am. Acad. Dermatol. 63 (3), 483–489. doi:10.1016/j.jaad.2009.09.006
Khwaja, A. (2012). KDIGO Clinical Practice Guidelines for Acute Kidney Injury. Nephron Clin. Pract. 120 (4), c179–84. doi:10.1159/000339789
Kimura, T., Isaka, Y., and Yoshimori, T. (2017). Autophagy and Kidney Inflammation. Autophagy 13 (6), 997–1003. doi:10.1080/15548627.2017.1309485
Kimura, T., Takabatake, Y., Takahashi, A., Kaimori, J. Y., Matsui, I., Namba, T., et al. (2011). Autophagy Protects the Proximal Tubule from Degeneration and Acute Ischemic Injury. J. Am. Soc. Nephrol. 22 (5), 902–913. doi:10.1681/ASN.2010070705
Ko, G. J., Bae, S. Y., Hong, Y. A., Pyo, H. J., and Kwon, Y. J. (2016). Radiocontrast-induced Nephropathy Is Attenuated by Autophagy through Regulation of Apoptosis and Inflammation. Hum. Exp. Toxicol. 35 (7), 724–736. doi:10.1177/0960327115604198
Kønig, S. M., Rissler, V., Terkelsen, T., Lambrughi, M., and Papaleo, E. (2019). Alterations of the Interactome of Bcl-2 Proteins in Breast Cancer at the Transcriptional, Mutational and Structural Level. Plos Comput. Biol. 15 (12), e1007485. doi:10.1371/journal.pcbi.1007485
Kruidering, M., Van de Water, B., de Heer, E., Mulder, G. J., and Nagelkerke, J. F. (1997). Cisplatin-induced Nephrotoxicity in Porcine Proximal Tubular Cells: Mitochondrial Dysfunction by Inhibition of Complexes I to IV of the Respiratory Chain. J. Pharmacol. Exp. Ther. 280 (2), 638–649. doi:10.1016/j.bbi.2007.10.008
Kuang, W., Zhang, X., Zhu, W., and Lan, Z. (2017). Ligustrazine Modulates Renal Cysteine Biosynthesis in Rats Exposed to Cadmium. Environ. Toxicol. Pharmacol. 54, 125–132. doi:10.1016/j.etap.2017.07.003
Kusirisin, P., Chattipakorn, S. C., and Chattipakorn, N. (2020). Contrast-induced Nephropathy and Oxidative Stress: Mechanistic Insights for Better Interventional Approaches. J. Transl Med. 18 (1), 400. doi:10.1186/s12967-020-02574-8
Lan, Z., Bi, K. S., and Chen, X. H. (2014). Ligustrazine Attenuates Elevated Levels of Indoxyl Sulfate, Kidney Injury Molecule-1 and Clusterin in Rats Exposed to Cadmium. Food Chem. Toxicol. 63, 62–68. doi:10.1016/j.fct.2013.10.038
Lewington, A. J., Cerdá, J., and Mehta, R. L. (2013). Raising Awareness of Acute Kidney Injury: a Global Perspective of a Silent Killer. Kidney Int. 84 (3), 457–467. doi:10.1038/ki.2013.153
Li, H. D., Meng, X. M., Huang, C., Zhang, L., Lv, X. W., and Li, J. (2019). Application of Herbal Traditional Chinese Medicine in the Treatment of Acute Kidney Injury. Front. Pharmacol. 10, 376. doi:10.3389/fphar.2019.00376
Li, J., Yu, J., and Liu, Y. (2017). Effects of Ligustrazine on the Expression of MMP-9 and TIMP-1 in Renal Interstitial Fibrosis ratsCNKI:SUN:YXLL. J. Med. Theor. Pract. 25 (3), 3.
Li, Q., Guo, Y., Ou, Q., Cui, C., Wu, W. J., Tan, W., et al. (2009). Gene Transfer of Inducible Nitric Oxide Synthase Affords Cardioprotection by Upregulating Heme Oxygenase-1 via a Nuclear Factor-{kappa}B-dependent Pathway. Circulation 120 (13), 1222–1230. doi:10.1161/CIRCULATIONAHA.108.778688
Linkermann, A., Bräsen, J. H., Darding, M., Jin, M. K., Sanz, A. B., Heller, J. O., et al. (2013). Two Independent Pathways of Regulated Necrosis Mediate Ischemia-Reperfusion Injury. Proc. Natl. Acad. Sci. U S A. 110 (29), 12024–12029. doi:10.1073/pnas.1305538110
Linkermann, A., Chen, G., Dong, G., Kunzendorf, U., Krautwald, S., and Dong, Z. (2014). Regulated Cell Death in AKI. J. Am. Soc. Nephrol. 25 (12), 2689–2701. doi:10.1681/ASN.2014030262
Liston, A., and Masters, S. L. (2017). Homeostasis-altering Molecular Processes as Mechanisms of Inflammasome Activation. Nat. Rev. Immunol. 17 (3), 208–214. doi:10.1038/nri.2016.151
Liu, C. F., Lin, M. H., Lin, C. C., Chang, H. W., and Lin, S. C. (2002). Protective Effect of Tetramethylpyrazine on Absolute Ethanol-Induced Renal Toxicity in Mice. J. Biomed. Sci. 9 (4), 299–302. doi:10.1007/BF02256584
Liu, H., and Baliga, R. (2005). Endoplasmic Reticulum Stress-Associated Caspase 12 Mediates Cisplatin-Induced LLC-PK1 Cell Apoptosis. J. Am. Soc. Nephrol. 16 (7), 1985–1992. doi:10.1681/ASN.2004090768
Liu, M., Ning, X., Li, R., Yang, Z., Yang, X., Sun, S., et al. (2017). Signalling Pathways Involved in Hypoxia-Induced Renal Fibrosis. J. Cel Mol Med 21 (7), 1248–1259. doi:10.1111/jcmm.13060
Liu, X. H., Li, J., Li, Q. X., Ai, Y. X., and Zhang, L. (2008). Protective Effects of Ligustrazine on Cisplatin-Induced Oxidative Stress, Apoptosis and Nephrotoxicity in Rats. Environ. Toxicol. Pharmacol. 26 (1), 49–55. doi:10.1016/j.etap.2008.01.006
Lou, Y., Zhang, H., Xia, C., and Chen, M. (1986). The Pharmacokinetics and In Vivo Fate of Ligustrazine Phosphate in Dogs and Rats. Acta Pharmaceutica Sinica (07), 481–487. doi:10.16438/j.0513-4870.1986.07.001
Lu, Min., Zhou, Juan., Wang, Fei., Liu, Yumin., and Zhang, Yue. (2009). Effects of Ligustrazine on the Expression of Smad7 and SnoN Protein in Renal Interstitial Fibrosis Model Rats. China J. Chin. Materia Med. 34 (01), 84–88. doi:10.3321/j.issn:1001-5302.2009.01.022
Makris, K., and Spanou, L. (2016). Acute Kidney Injury: Definition, Pathophysiology and Clinical Phenotypes. Clin. Biochem. Rev. 37 (2), 85–98.
McWilliam, S. J., Wright, R. D., Welsh, G. I., Tuffin, J., Budge, K. L., Swan, L., et al. (2021). The Complex Interplay between Kidney Injury and Inflammation. Clin. Kidney J. 14 (3), 780–788. doi:10.1093/ckj/sfaa164
Mehta, R. L., Cerdá, J., Burdmann, E. A., Tonelli, M., García-García, G., Jha, V., et al. (2015). International Society of Nephrology's 0by25 Initiative for Acute Kidney Injury (Zero Preventable Deaths by 2025): a Human Rights Case for Nephrology. Lancet 385 (9987), 2616–2643. doi:10.1016/S0140-6736(15)60126-X
Meng, X. M., Nikolic-Paterson, D. J., and Lan, H. Y. (2016). TGF-β: the Master Regulator of Fibrosis. Nat. Rev. Nephrol. 12 (6), 325–338. doi:10.1038/nrneph.2016.48
Michel, H. E., and Menze, E. T. (2019). Tetramethylpyrazine Guards against Cisplatin-Induced Nephrotoxicity in Rats through Inhibiting HMGB1/TLR4/NF-Κb and Activating Nrf2 and PPAR-γ Signaling Pathways. Eur. J. Pharmacol. 857, 172422. doi:10.1016/j.ejphar.2019.172422
Molema, G., Zijlstra, J. G., van Meurs, M., and Kamps, J. A. A. M. (2021). Renal Microvascular Endothelial Cell Responses in Sepsis-Induced Acute Kidney Injury. Nat. Rev. Nephrol. doi:10.1038/s41581-021-00489-1
Nezu, M., Souma, T., Yu, L., Suzuki, T., Saigusa, D., Ito, S., et al. (2017). Transcription Factor Nrf2 Hyperactivation in Early-phase Renal Ischemia-Reperfusion Injury Prevents Tubular Damage Progression. Kidney Int. 91 (2), 387–401. doi:10.1016/j.kint.2016.08.023
Nielsen, P. M., Laustsen, C., Bertelsen, L. B., Qi, H., Mikkelsen, E., Kristensen, M. L., et al. (2017). In Situ lactate Dehydrogenase Activity: a Novel Renal Cortical Imaging Biomarker of Tubular Injury? Am. J. Physiol. Ren. Physiol 312 (3), F465–F473. doi:10.1152/ajprenal.00561.2015
Noel, S., Arend, L. J., Bandapalle, S., Reddy, S. P., and Rabb, H. (2016). Kidney Epithelium Specific Deletion of Kelch-like ECH-Associated Protein 1 (Keap1) Causes Hydronephrosis in Mice. BMC Nephrol. 17 (1), 110. doi:10.1186/s12882-016-0310-y
Norgren, S., and Gong, X. Z. (2018). Contrast-induced Nephropathy-Time for Western Medicine and Chinese Medicine to Team up. Chin. J. Integr. Med. 24 (1), 3–5. doi:10.1007/s11655-017-2905-y
Pan, J., Zhang, L., Xiong, D., Li, B., and Qu, H. (2021). A HPLC-DAD-MS/MS Method for Simultaneous Determination of Six Active Ingredients of Salviae Miltiorrhizae and Ligustrazine Hydrochloride Injection in Rat Plasma and its Application to Pharmacokinetic Studies. Curr. Drug Metab. 22 (1), 60–69. doi:10.2174/1389200221999200819143230
Peerapornratana, S., Manrique-Caballero, C. L., Gómez, H., and Kellum, J. A. (2019). Acute Kidney Injury from Sepsis: Current Concepts, Epidemiology, Pathophysiology, Prevention and Treatment. Kidney Int. 96 (5), 1083–1099. doi:10.1016/j.kint.2019.05.026
Privratsky, J. R., Zhang, J., Lu, X., Rudemiller, N., Wei, Q., Yu, Y. R., et al. (2018). Interleukin 1 Receptor (IL-1R1) Activation Exacerbates Toxin-Induced Acute Kidney Injury. Am. J. Physiol. Ren. Physiol 315 (3), F682–F691. doi:10.1152/ajprenal.00104.2018
Rabb, H., Griffin, M. D., McKay, D. B., Swaminathan, S., Pickkers, P., Rosner, M. H., et al. (2016). Inflammation in AKI: Current Understanding, Key Questions, and Knowledge Gaps. J. Am. Soc. Nephrol. 27 (2), 371–379. doi:10.1681/ASN.2015030261
Ramesh, G., and Reeves, W. B. (2004). Inflammatory Cytokines in Acute Renal Failure. Kidney Int. Suppl. Suppl (91), S56–S61. doi:10.1111/j.1523-1755.2004.09109.x
Reichard, J. F., Sartor, M. A., and Puga, A. (2016). BACH1 Is a Specific Repressor of HMOX1 that Is Inactivated by Arsenite. J. Biol. Chem. 283 (33), 22363–22370. doi:10.1074/jbc.M801784200
Ronco, C., Bellomo, R., and Kellum, J. A. (2019). Acute Kidney Injury. Lancet 394 (10212), 1949–1964. doi:10.1016/S0140-6736(19)32563-2
Saito, H. (2013). Toxico-pharmacological Perspective of the Nrf2-Keap1 Defense System against Oxidative Stress in Kidney Diseases. Biochem. Pharmacol. 85 (7), 865–872. doi:10.1016/j.bcp.2013.01.006
Sancho-Martínez, S. M., López-Novoa, J. M., and López-Hernández, F. J. (2015). Pathophysiological Role of Different Tubular Epithelial Cell Death Modes in Acute Kidney Injury. Clin. Kidney J. 8 (5), 548–559. doi:10.1093/ckj/sfv069
Scherz-Shouval, R., and Elazar, Z. (2007). ROS, Mitochondria and the Regulation of Autophagy. Trends Cel Biol 17 (9), 422–427. doi:10.1016/j.tcb.2007.07.009
See, E. J., See, K., Glassford, N., Bailey, M., Johnson, D. W., Polkinghorne, K. R., et al. (2019). Long-term Risk of Adverse Outcomes after Acute Kidney Injury: a Systematic Review and Meta-Analysis of Cohort Studies Using Consensus Definitions of Exposure. Kidney Int. 95 (1), 160–172. doi:10.1016/j.kint.2018.08.036
Shen, J., Wang, L., Jiang, N., Mou, S., Zhang, M., Gu, L., et al. (2016). NLRP3 Inflammasome Mediates Contrast media-induced Acute Kidney Injury by Regulating Cell Apoptosis. Sci. Rep. 6, 34682. doi:10.1038/srep34682
Shi, J., Gao, W., and Shao, F. (2017). Pyroptosis: Gasdermin-Mediated Programmed Necrotic Cell Death. Trends Biochem. Sci. 42 (4), 245–254. doi:10.1016/j.tibs.2016.10.004
Sies, H., Berndt, C., and Jones, D. P. (2017). Oxidative Stress. Annu. Rev. Biochem. 86, 715–748. doi:10.1146/annurev-biochem-061516-045037
Sies, H. (1997). Oxidative Stress: Oxidants and Antioxidants. Exp. Physiol. 82 (2), 291–295. doi:10.1113/expphysiol.1997.sp004024
Simonetto, D. A., Gines, P., and Kamath, P. S. (2020). Hepatorenal Syndrome: Pathophysiology, Diagnosis, and Management. BMJ 370, m2687. doi:10.1136/bmj.m2687
Singh, R., Letai, A., and Sarosiek, K. (2019). Regulation of Apoptosis in Health and Disease: the Balancing Act of BCL-2 Family Proteins. Nat. Rev. Mol. Cel Biol 20 (3), 175–193. doi:10.1038/s41580-018-0089-8
Sue, Y. M., Cheng, C. F., Chang, C. C., Chou, Y., Chen, C. H., and Juan, S. H. (2009). Antioxidation and Anti-inflammation by Haem Oxygenase-1 Contribute to protection by Tetramethylpyrazine against Gentamicin-Induced Apoptosis in Murine Renal Tubular Cells. Nephrol. Dial. Transpl. 24 (3), 769–777. doi:10.1093/ndt/gfn545
Suen, D. F., Norris, K. L., and Youle, R. J. (2008). Mitochondrial Dynamics and Apoptosis. Genes Dev. 22 (12), 1577–1590. doi:10.1101/gad.1658508
Sun, L., Li, Y., Shi, J., Wang, X., and Wang, X. (2002). Protective Effects of Ligustrazine on Ischemia-Reperfusion Injury in Rat Kidneys. Microsurgery 22 (8), 343–346. doi:10.1002/micr.10058
Sun, W., Li, A., Wang, Z., Sun, X., Dong, M., Qi, F., et al. (2020). Tetramethylpyrazine Alleviates Acute Kidney Injury by Inhibiting NLRP3/HIF-1α and A-poptosis. Mol. Med. Rep. 22 (4), 2655–2664. doi:10.3892/mmr.2020.11378
Sureshbabu, A., Ryter, S. W., and Choi, M. E. (2015). Oxidative Stress and Autophagy: Crucial Modulators of Kidney Injury. Redox Biol. 4, 208–214. doi:10.1016/j.redox.2015.01.001
Susantitaphong, P., Cruz, D. N., Cerda, J., Abulfaraj, M., Alqahtani, F., Koulouridis, I., et al. (2013). World Incidence of AKI: a Meta-Analysis. Clin. J. Am. Soc. Nephrol. 8 (9), 1482–1493. doi:10.2215/cjn.00710113
Suzuki, T., and Yamamoto, M. (2015). Molecular Basis of the Keap1-Nrf2 System. Free Radic. Biol. Med. 88 (Pt B), 93–100. doi:10.1016/j.freeradbiomed.2015.06.006
Tan, R. J., Chartoumpekis, D. V., Rush, B. M., Zhou, D., Fu, H., Kensler, T. W., et al. (2016). Keap1 Hypomorphism Protects against Ischemic and Obstructive Kidney Disease. Sci. Rep. 6, 36185. doi:10.1038/srep36185
Tracz, M. J., Juncos, J. P., Croatt, A. J., Ackerman, A. W., Grande, J. P., Knutson, K. L., et al. (2007). Deficiency of Heme Oxygenase-1 Impairs Renal Hemodynamics and Exaggerates Systemic Inflammatory Responses to Renal Ischemia. Kidney Int. 72 (9), 1073–1080. doi:10.1038/sj.ki.5002471
Tucker, P. S., Scanlan, A. T., and Dalbo, V. J. (20152015). Chronic Kidney Disease Influences Multiple Systems: Describing the Relationship between Oxidative Stress, Inflammation, Kidney Damage, and Concomitant Disease. Oxid Med. Cel Longev 2015, 806358. doi:10.1155/2015/806358
Vernon, M. A., Mylonas, K. J., and Hughes, J. (2010). Macrophages and Renal Fibrosis. Semin. Nephrol. 30 (3), 302–317. doi:10.1016/j.semnephrol.2010.03.004
Wang, B., Ni, Q., Wang, X., and Lin, L. (2012). Meta-analysis of the Clinical Effect of Ligustrazine on Diabetic Nephropathy. Am. J. Chin. Med. 40 (1), 25–37. doi:10.1142/S0192415X12500036
Wang, H., Zhang, J., and Huang, J. (2006). The Effect of Aristolochic Acid on Rat Renal Function and Histopathology and the Intervention of Ligustrazine and Benazepril. Chin. J. Integrated Traditional Chin. West. Med. Nephropathy (06), 328–331+374. doi:10.3969/j.issn.1009-587X.2006.06.006
Wang, J., Hong, G., Li, G., Wang, W., and Liu, T. (2019). Novel Homo-Bivalent and Polyvalent Compounds Based on Ligustrazine and Heterocyclic Ring as Anticancer Agents. Molecules 24 (24), 4505. doi:10.3390/molecules24244505
Wu, H., and Huang, J. (2018). Drug-Induced Nephrotoxicity: Pathogenic Mechanisms, Biomarkers and Prevention Strategies. Curr. Drug Metab. 19 (7), 559–567. doi:10.2174/1389200218666171108154419
Wu, X. J., Sun, X. H., Wang, S. W., Chen, J. L., Bi, Y. H., and Jiang, D. X. (2018). Mifepristone Alleviates Cerebral Ischemia-Reperfusion Injury in Rats by Stimulating PPAR γ. Eur. Rev. Med. Pharmacol. Sci. 22 (17), 5688–5696. doi:10.26355/eurrev_201809_15836
Xue, Y., Enosi Tuipulotu, D., Tan, W. H., Kay, C., and Man, S. M. (2019). Emerging Activators and Regulators of Inflammasomes and Pyroptosis. Trends Immunol. 40 (11), 1035–1052. doi:10.1016/j.it.2019.09.005
Yang, Y., Song, M., Liu, Y., Liu, H., Sun, L., Peng, Y., et al. (2016). Renoprotective Approaches and Strategies in Acute Kidney Injury. Pharmacol. Ther. 163, 58–73. doi:10.1016/j.pharmthera.2016.03.015
Yatim, K. M., and Oberbarnscheidt, M. H. (2015). Endotoxin and AKI: Macrophages Protect after Preconditioning. J. Am. Soc. Nephrol. 26 (6), 1231–1232. doi:10.1681/ASN.2014101042
Ying, J., Wu, J., Zhang, Y., Han, Y., Qian, X., Yang, Q., et al. (2020). Ligustrazine Suppresses Renal NMDAR1 and Caspase-3 Expressions in a Mouse Model of Sepsis-Associated Acute Kidney Injury. Mol. Cel Biochem 464 (1-2), 73–81. doi:10.1007/s11010-019-03650-4
Youle, R. J., and Narendra, D. P. (2011). Mechanisms of Mitophagy. Nat. Rev. Mol. Cel Biol 12 (1), 9–14. doi:10.1038/nrm3028
Youle, R. J., and Strasser, A. (2008). The BCL-2 Protein Family: Opposing Activities that Mediate Cell Death. Nat. Rev. Mol. Cel Biol 9 (1), 47–59. doi:10.1038/nrm2308
Yuan, X. P., Liu, L. S., Fu, Q., and Wang, C. X. (2012). Effects of Ligustrazine on Ureteral Obstruction-Induced Renal Tubulointerstitial Fibrosis. Phytother Res. 26 (5), 697–703. doi:10.1002/ptr.3630
Zhang, H., Bosch-Marce, M., Shimoda, L. A., Tan, Y. S., Baek, J. H., Wesley, J. B., et al. (2008). Mitochondrial Autophagy Is an HIF-1-dependent Adaptive Metabolic Response to Hypoxia. J. Biol. Chem. 283 (16), 10892–10903. doi:10.1074/jbc.M800102200
Zhang, J. X., Dang, S. C., Qu, J. G., and Wang, X. Q. (2006). Ligustrazine Alleviates Acute Renal Injury in a Rat Model of Acute Necrotizing Pancreatitis. World J. Gastroenterol. 12 (47), 7705–7709. doi:10.3748/wjg.v12.i47.7705
Zhao, Y., Liu, Y., and Chen, K. (20162016). Mechanisms and Clinical Application of Tetramethylpyrazine (An Interesting Natural Compound Isolated from Ligusticum Wallichii): Current Status and Perspective. Oxid Med. Cel Longev 2016, 2124638. doi:10.1155/2016/2124638
Zou, J., Gao, P., Hao, X., Xu, H., Zhan, P., and Liu, X. (2018). Recent Progress in the Structural Modification and Pharmacological Activities of Ligustrazine Derivatives. Eur. J. Med. Chem. 147 (147), 150–162. doi:10.1016/j.ejmech.2018.01.097
Glossary
ALP alkaline phosphatase
ANP acute necrotizing pancreatitis
ARS2 Arsenic response protein 2
BUN blood urea nitrogen
CBS cystathionine-beta-synthase
CIN contrast-induced nephropathy
COX-2 cyclooxygenase-2
ET-1 endothelin-1
FoxO1 Fork-head box O1 transcriptional factor
GR glutathione reductase
GSH glutathione
GSSG glutathione disulfide
GST Glutathione-S-transferase
HAX-1 HS-1-associated protein
HMGB1 high mobility group box 1
HIF-1 hypoxia inducible factor-1α
HO-1 Heme oxygenase-1
ICAM-1 intercellular cell adhesion molecule-1
IL interleukin
I/R Ischemia-reperfusion
kim-1 kidney injury molecule-1
LDH lactate dehydrogenase
LPO lipid peroxidation
MAPK Mitogen-activated protein kinase
MATs methionine adenosyltransferases
MCP-1 monocyte chemoattractant protein
MDA malondialdehyde
MICA major histocompatability complex class I chain-related antigen A
NAG N-acetyl-b-D-glucosaminidase
NF-κB Nuclear factor-κB
NLRP3 nucleotide-oligomerization domain-like receptor 3
NMDARs N-methyl-d-aspartate receptors
NO Nitric oxide
NOD2 Nucleotide-binding oligomerization domain-containing 2
NOS nitric oxide synthase
NQO1 NAD (P) H: quinone oxidoreductase 1
Nrf2 Nuclear factor erythroid derived-2
OGD oxygen-glucose deprivation
PGI2 prostaglandin I2
PPAR-γ peroxisome proliferator-activated receptor-gamma
ROS reactive oxygen species
SAM S-adenosylmethionine
SOD superoxide dismutase
SrCr serum creatinine
TBSA total body surface area
TLR4 toll-like receptor 4
TNF-α tumor necrosis factor
TOX total antioxidant activity
TXA2 thromboxane A2
4-HNE 4-hydroxynonenal
Keywords: tetramethylpyrazine, acute kidney injury, renal fibrosis, Chinese medicine, mechanism
Citation: Li J and Gong X (2022) Tetramethylpyrazine: An Active Ingredient of Chinese Herbal Medicine With Therapeutic Potential in Acute Kidney Injury and Renal Fibrosis. Front. Pharmacol. 13:820071. doi: 10.3389/fphar.2022.820071
Received: 22 November 2021; Accepted: 10 January 2022;
Published: 25 January 2022.
Edited by:
Dan-Qian Chen, Northwest University, ChinaReviewed by:
Quan Hong, Chinese PLA General Hospital, ChinaYing Liu, Central South University, China
Copyright © 2022 Li and Gong. This is an open-access article distributed under the terms of the Creative Commons Attribution License (CC BY). The use, distribution or reproduction in other forums is permitted, provided the original author(s) and the copyright owner(s) are credited and that the original publication in this journal is cited, in accordance with accepted academic practice. No use, distribution or reproduction is permitted which does not comply with these terms.
*Correspondence: Xuezhong Gong, shnanshan@yeah.net