- 1Department of Respiratory Medicine, Taizhou University Affiliated Wenling Hospital, Taizhou University, Taizhou, China
- 2Department of Cardiac Surgery, Lanzhou University Second Hospital, Lanzhou University, Lanzhou, China
- 3Department of Basic Medicine, School of Medicine, Taizhou University, Taizhou, China
Drug delivery systems (DDSs) have recently gained widespread attention for improving drug loading and delivery efficiency in treating many cancers. Elastin-like polypeptides (ELPs) are synthetic peptides derived from a precursor of elastin (tropoelastin), reserving similar structural and physicochemical properties. ELPs have gained a variety of applications in tissue engineering and cancer therapy due to their excellent biocompatibility, complete degradability, temperature-responsive property, controllable sequence and length, and precisely tuned structure and function. ELPs-based drug delivery systems can improve the pharmacokinetics and biodistribution of therapeutic reagents, leading to enhanced antitumor efficacy. In this review, we summarize the recent application of ELPs in cancer treatment, focusing on the delivery of functional peptides, therapeutic proteins, small molecule drugs, and photosensitizers.
1 Introduction
Recent cancer statistics indicate about 19.3 million new cancer cases and 10.0 million deaths caused by cancer worldwide in 2020 (Ferlay et al., 2021). The rising incidence and mortality of cancers promote the urge to develop effective cancer treatments. Surgery, radiotherapy, chemotherapy, targeted therapy, and immunotherapy are common strategies against cancers (Mun et al., 2018). Despite the good outcome of surgery and radiotherapy in treating primary tumors, their applications were greatly limited in treating nonmetastatic tumors (Otake and Goto 2019). Clinical anticancer drugs for chemotherapy, targeted therapy, and immunotherapy have significantly improved the prognosis and survival of cancer patients (Tibau et al., 2018). However, some disadvantages of anticancer drugs, such as poor solubility, inadequate endocytosis, unwanted distribution, and severe side effects, lead to compromised clinical outcomes in some cancers.
Drug delivery systems (DDSs) have shown great potential in improving cancer treatment efficacy through high drug loading capacity, increased cell internalization and tumor distribution, and prolonged circulation time (Dang and Guan 2020; Yang, Wang, and Guan 2022). Many materials, including polymers, lipids, proteins, nucleic acids, and inorganic materials, have been used to construct various delivery platforms to improve the tumor suppression effect (Cheng et al., 2021; Hossen et al., 2019; Patra et al., 2018). Among various DDSs, naturally derived materials-based DDSs attract more and more attention due to their good biocompatibility, excellent biodegradability, and low immunogenicity (Huang and Fu 2010). Elastin, which is widely distributed in mammalian tissues including lung, bladder, blood vessel, skin, and cartilage, helps maintain the elasticity of tissues (Mithieux and Weiss 2005). Elastin-like polypeptides (ELPs), a series of synthetic polymers according to elastin’s amino acid sequence, have shown promising potential in drug delivery for tissue engineering and cancer therapy (Milligan et al., 2022; Shi et al., 2022). This review summarizes the physicochemical properties and the recent applications of ELPs as delivery platforms for cancer treatments.
2 Structural and physicochemical properties of ELPs
ELPs, derived from tropoelastin (a precursor of elastin), are synthetic pentapeptide repeats composed of (VPGXG)n, while X can be any amino acid except for Pro (Figure 1A). (Megeed, Cappello, and Ghandehari 2002). Due to their naturally derived materials, ELPs have excellent biocompatibility, prolonged circulation, and good elasticity in vivo, which make them ideal materials for biomedical application (Suhar et al., 2020). Moreover, precisely adjusting amino acids in the guest residue (X) and repeat numbers (n) make ELPs fine-tuned biomaterials with thermal sensitivity and satisfying mechanical properties for tissue engineering and drug delivery (Ciofani et al., 2014; Gagner, Kim, and Chaikof 2014). In addition, ELPs have a unique temperature-responsive phase-transition property: ELPs undergo from the soluble phase at a lower temperature to ELPs aggregates when the temperature is above the phase transition temperature (Tt), and ELPs aggregates can resolubilize in aqueous solution when the temperature is lower than the Tt (Figure 1B) (Smits et al., 2015). The inverse transition cycling (ITC) property of ELPs has been used to rapidly purify ELPs-fused recombinant proteins with satisfied purity (Floss et al., 2010; Heidari-Japelaghi et al., 2019; Sweet et al., 2021).
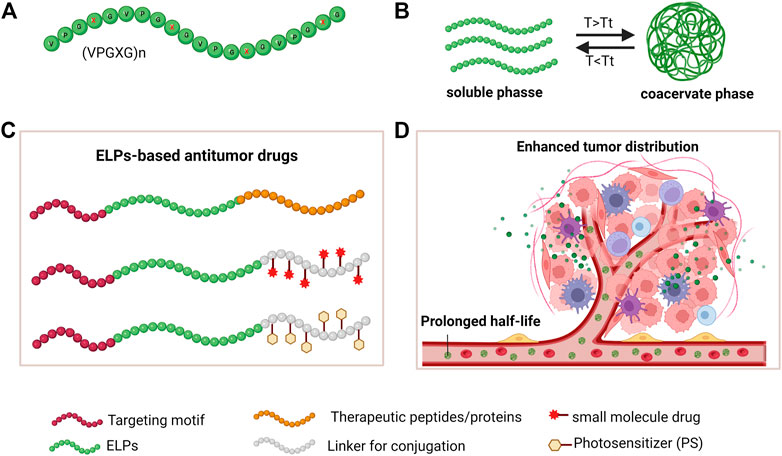
FIGURE 1. The ELPs properties and strategies of developing ELPs-based antitumor drugs. (A) amino acid sequence of ELPs; (B) The inverse transition cycling (ITC) property; (C) Strategies of developing drug-loaded ELPs by fusion expression and drug conjugation; (D) Schematic illustration of ELPs-based drugs in systemically circulating and tumor accumulation.
ELPs can be fused with bioactive peptides or proteins as fusion proteins by genetic engineering. Functional peptides or proteins are displayed on the surface of protein assemblies and exert their unique functions (Varanko et al., 2020). Moreover, a linker peptide can be fused at the C-terminus of the ELPs sequence for conjugating cytotoxic drugs (McDaniel et al., 2013). In addition, as a block architecture, ELPs can self-assemble into nanoparticles encapsulating drugs by chemical conjugation or physical absorption (Figure 1C) (Rodríguez-Cabello et al., 2016). ELPs-based platforms have demonstrated many advantages for improved delivery efficiency in cancer treatment (Figure 1D) (Shi et al., 2013).
3 Drug-loaded ELPs for cancer therapy
ELPs-based DDSs have proven to be an effective strategy to improve the pharmacokinetics of a range of drugs (Milligan et al., 2022). ELPs polymers or nanoparticles could significantly decrease the blood clearance of drugs by reticuloendothelial system and extend the half-time of cargo-loaded (Figure 2), leading to the enhanced antitumor efficacy in vivo (Zhaoying et al., 2022).
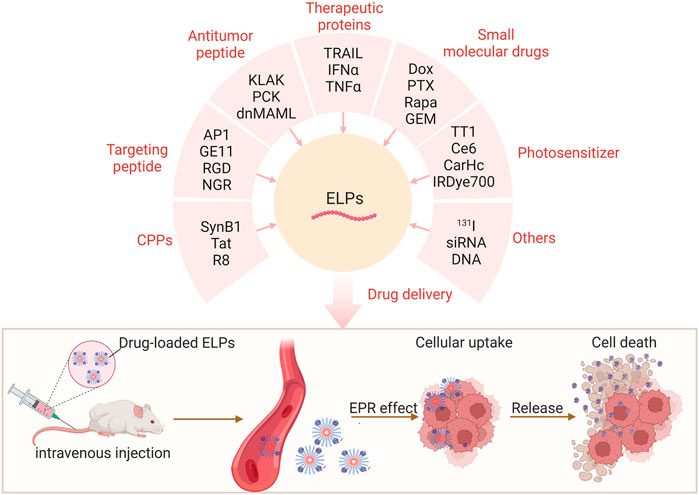
FIGURE 2. ELPs deliver functional peptides, therapeutic proteins, small molecule drugs, and photosensitizers for enhanced cancer therapy.
3.1 ELPs delivering tumor-targeting peptides
To endow the ELPs with tumor-selectively binding properties, researchers have decorated many tumor-targeting peptides in ELPs polymer for improved cancer treatment (Matsumoto et al., 2014). Simnick AJ et al. prepared NGR tripeptide-fused ELPs micelles by genetic engineering for tumor vasculature targeting. The self-assembled NGR-ELP micelles showed selective binding with CD13 and improved tumor vascular retention and extravascular accumulation (Simnick et al., 2011). Sarangthem V incorporated multiple AP1 peptides into the ELP polymer for tumor-targeting delivery. The AP1 peptide presented by ELP polymer showed a 10000-fold increase of interleukin 4 receptor (IL-4R) binding affinity compared to the free AP1 peptide (Bhattacharya et al., 2013). Tumor homing peptide F3, a short peptide derived from high-mobility group protein 2, could specifically bind to nucleolin expressed on tumor endothelial cells and tumor cells. F3-ELP nanocarriers delivering doxorubicin showed a 4.2-fold increase in tumor accumulation and a 3.8-fold decreased tumor size compared with ELP nanoparticles without F3 decoration (Hu et al., 2015). Bld-1 peptide, a bladder tumor targeting peptide, has also been fused with ELP(B5V60) to improve the tumor distribution in bladder tumor xenograft mice (Sarangthem et al., 2018). ELPs fused with gastrin releasing peptide (GRP) could selectively bind with G protein-coupled receptors (GPCRs) and increase the cellular uptake of nanoparticles (Zhang et al., 2016). Cheon SH developed melanoma-targeting ligands-decorated ELPs (M16E108), which accumulated explicitly in cisplatin-resistant B16F10 melanoma cells and had long retention in tumor tissues (Cheon et al., 2020). Many cell-penetrating peptides (CPP) have been incorporated into ELP polymers to improve tumor cells’ internalization (Massodi, Bidwell Iii, and Raucher 2005). SynB1-functionalized ELP combined with hyperthermia enhances the antitumor effects of Taxol in MDA-MB-231 xenograft models (Ryu, Robinson, and Raucher 2019a). Octa-arginine (R8) incorporation enhanced the penetration of ELP nanoparticles into spheroids generated from human glioblastoma U-87 cells (van Oppen et al., 2019). Therefore, in addition to the high selectivity of tumor-homing peptides with targeting receptors, ELPs decorated with targeting peptides have a prolonged half-life in vivo, providing an ideal platform for the efficient delivery of therapeutics agents to the tumors.
3.2 ELPs delivering therapeutic peptides or proteins
During the past two decades, therapeutic peptides have attracted considerable attention due to their high selectivity in treating many solid tumors (Fosgerau and Hoffmann 2015; Marqus, Pirogova, and Piva 2017). However, the existence of peptidases and protease in vivo significantly limits the application of therapeutic peptides or proteins in cancer treatment (Strohl 2015). A cell cycle inhibitory peptide (p21)-fused with ELPs (p21-ELP1-Bac) demonstrated enhanced cytotoxicity on pancreatic cancer cell lines through cell cycle arrest. ELPs polymers showed synergistic antitumor effects in S2013 xenograft models combined with gemcitabine (Ryu and Raucher 2014). Sarangthem V designed a proapoptotic peptide (KLAKLAK)2-loaded ELPs delivery system for treating breast cancer. AP1-ELP-KLAK could significantly enhance tumor localization and suppress tumor growth in breast cancer and melanoma xenografts (Sarangthem et al., 2016). Liu N et al. designed a fluorescent ELPs nanocarrier for targeted delivery of PCK 3145 peptide to epidermal growth factor receptor (EGFR)-overexpressed tumors. The multifunctional ELPs nanoparticles triggered the apoptosis of tumor cells and enhanced antitumor efficacy in the CT26 tumor xenograft model (Liu et al., 2022). A Notch inhibitory peptide (dnMAML) conjugated ELP polymer was developed, showing an enhanced antitumor effect on glioblastoma cells. SynB1-modified complexes inhibited the growth of D54 and U251 cells by inducing apoptosis and cell cycle arrest (Ryu, Robinson, and Raucher 2019b).
As for therapeutic protein delivery, Huang K and his coworkers fabricated tumor-targeting ELPs delivering Tumor necrosis factor-related apoptosis-inducing ligand (TRAIL) protein to colon cancers. ELP-RGD-TRAIL demonstrated a 3-fold increase of apoptosis than without ELP fusion. Single intraperitoneal injection of the RGD-targeted ELPs nanomedicine could inhibit tumor regression in the COLO-205 tumor xenograft model (Huang et al., 2017). ELPs were used to deliver interferon alpha (IFN-α) for cancer treatment. The ELP fused IFN-α showed much higher activity retention than PEGylated IFN-α and IFN-HAS. ELP fusion also significantly prolonged the half-life of IFN-α and excellent antitumor efficacy in ovarian carcinoma xenografts (Hu et al., 2015). Liang P fused IFN-α with ELPs to form a deport injected into the GBM resection cavity. IFN-ELP(V) in the depot dramatically improved pharmacokinetics and biodistribution, and significantly inhibited GBM recurrence, especially combined with temozolomide (TMZ) (Liang et al., 2021). In summary, combining their unique therapeutic mechanisms, ELPs fusion prolonged the half-time of therapeutic peptides or proteins and enhanced their tumor distribution, contributing to enhanced tumor suppression in vivo.
3.3 ELPs delivering small molecule chemotherapeutics
Due to the prolonged half-time ability, ELPs have been used to improve the delivery efficiency of small-molecule chemotherapeutics for cancer therapy (Bidwell and Gene, 2021). Bhattacharyya J et al. prepared recombinant ELPs containing eight cysteine residues for paclitaxel (PTX) conjugation. PTX-conjugated polypeptides could spontaneously self-assemble into monodisperse nanoparticles, which had twofold increased cell uptake than Abraxane. PTX-loaded nanoparticles near-completely inhibit tumor growth after a single dose in a murine cancer model of human triple-negative breast and prostate cancer (Bhattacharyya et al., 2015). Mie M and his coworkers designed a DNA aptamer conjugated ELPs through a poly-aspartic acid for PTX delivery. PTX-loaded nanoparticles with DNA aptamer showed increased cytotoxicity with MCF-7 breast cancer cells (Mie et al., 2019). Ryu JS conjugated doxorubicin (Dox) to the CPP-ELP polymer using a matrix metalloproteinase (MMP) sensitive linker. CPP-complexed Dox showed significantly increased cell penetration and cell death, even in doxorubicin-resistant breast cancer cells (Ryu, Kratz, and Raucher 2021). Vallejo R prepared docetaxel (DTX) encapsulated, RGD peptide-decorated ELP nanoparticles, suggesting higher cytotoxicity on breast cancer than endothelial cells (Vallejo et al., 2021). Peddi S fused the FKBP12 receptor with ELPs for selective delivery of Rapamycin (Rapa). The Rapa-loaded formulation was internalized by MDA-MB-468 breast cancer cells through macropinocytosis (Peddi, Pan, and MacKay 2018). Avila H prepared an ELPs-based nanocarrier containing rapamycin-binding domains for targeting glucose-regulated protein (csGRP78). The targeted carriers significantly enhanced cellular uptake and reduced mTOR activity by 3-fold compared to free rapamycin (Avila et al., 2022). Ramamurthi D also prepared gemcitabine-conjugated ELPs for ovarian cancer therapy (Ramamurthi et al., 2022). In summary, ELPs delivering chemotherapeutics greatly enhanced their cellular uptake and increased the cytotoxicity against tumors in vitro and in vivo, suggesting the excellent antitumor effect of the ELPs-based delivery platform.
3.4 ELPs delivering photosensitizer
Photodynamic therapy (PDT) has attracted considerable attention in cancer therapy due to precise and non-invasive treatment (Zhang et al., 2020). Photosensitizers (PS) can absorb light to generate cytotoxic reactive oxygen species (ROS) for tumor killing (Shen et al., 2022). PS-loaded ELPs increased tissue permeation and prolonged half-life without loss of ROS production ability (Shi et al., 2022). Pille J prepared llama heavy-chain antibody fragments (VHHs)–decorated ELPs micelles to deliver photosensitizer (IRDye700DX) to EGFR-overexpressed cancers. The PS-loaded micelles demonstrated an EGFR-targeted light-induced tumor cell killing (Pille et al., 2017). Sun M et al. designed a near-infrared absorbing polymer (polypyrrole, PPy)-conjugated PPy-ELP-F3 nanoparticles to treat melanoma. F3-decorated nanoparticles demonstrated higher cellular uptake than nanoparticles without tumor-homing functions in human high-mobility group protein 2 (HMGN2)-overexpressed cancer cells, and they also completely abolish tumors through the combination of photothermal and chemical therapy (Sun et al., 2018). Ibrahimova V and his coworkers conjugated photosensitizer TT1 (a peripherally substituted carboxy-Zn(II)- phthalocyanine derivative) to ELP polymer (M1V3-40), which could produce singlet oxygen (1O2) for photodynamic therapy (PDT) (Ibrahimova et al., 2021). Thus, the ELPs platform improved the biocompatibility and tumor accumulation of PS without decreasing their ability to generate ROS for tumor killing, showing a good candidate for the safe and efficient delivery of PS to tumors.
3.5 ELPs delivering other drugs
Liu X et al. developed a 131I –labeled ELPs for radiotherapy and explored their antitumor effect in rabbits with VX2 liver tumors (Liu et al., 2016). ELPs delivering 131I could improve liver function and inhibit tumor growth. Rang-Woon Park group designed ELP nanocarriers containing TAT and AP1 peptides for nucleic acids delivery. TAT-targeted ELPs nanocarriers could selectively deliver siRNA into tumors and significantly downregulate the Luciferase gene expression in the murine breast carcinoma model (Yi et al., 2020). In addition to siRNA, the same nanocarriers could efficiently introduce EGFP plasmids into IL-4R-expressed tumor cells and enhance EGFP expression with low toxicity (Yi et al., 2022).
4 Conclusion and perspectives
ELPs have shown great potential in delivering various anticancer drugs for enhanced cancer therapy due to their excellent biocompatibility, complete degradability, temperature-responsive property, controllable sequence and length, and precisely tuned function (Zhaoying et al., 2022). However, many problems remain to be solved for further application of ELPs for cancer therapy. For example, the reported ELPs for drug delivery are prepared using the Escherichia coli expression system, which lacks post-translational modification ability. Thus, ELPs may not be suitable for delivering the functional proteins which need sophisticated protein modification. Moreover, the concrete guest residue and peptide length of ELPs need to be optimized for drug delivery to different tumor types. Sarangthem V indicated that increased molecular weight of AP1-ELPs contributes to better tumor penetration and retention in 4T1 tumor-bearing mice (Sarangthem et al., 2020). Kuna M also demonstrated that the increased molecular weight of ELPs had longer plasma half-lives and higher total renal accumulation (Kuna et al., 2018). Due to the high heterogeneity of tumors, the best guest residue and molecular weight of ELPs in delivering antitumor drugs for specific cancer should be investigated in detail. In addition, drug-loaded ELPs nanoparticles hold great promise for next-generation advancement in improving the efficacy of cancer treatment. Therapeutic peptides or proteins could be genetically fused with ELPs for fusion expression, and small molecule drugs or PS could be attached to the backbone or linker peptide of ELPs polymers through post-translational modifications (Milligan et al., 2022). Because of the numerous potential reaction groups on the protein surface, the precise conjugation of antitumor drugs to ELPs remains a significant challenge. Costa SA attached Dox into a nanobody-targeted ELPs nanoparticle by incorporating an unnatural amino acid (p-acetylphenylalanine), providing a promising strategy for targeted conjugation with ELPs polymers (Costa et al., 2018). The biorthogonal drug attachment strategy could be used to develop more and more ELPs-drug conjugates with improved efficacy in the future (Sisila et al., 2022).
In conclusion, ELPs, as flexible and tunable biomaterials, are good candidates for constructing efficient drug delivery platforms for cancer treatment. By improving the pharmacokinetics and tumor distribution, ELPs have enhanced the cytotoxicity of loaded drugs and significantly inhibited tumor growth in many solid tumor xenografts. Despite no approved ELPs-based formulation for cancer therapy, their clinical testing in treating diabetes and heart failure may accelerate the transformation process. The ELPs-based delivery platform will likely bring approved drugs for cancer therapy in the future.
Author contributions
AJ and XG wrote the original manuscript. LH designed the figures. XG reviewed the manuscript and funded the publication. All authors have read and agreed to the published version of the manuscript.
Funding
This study was supported by Zhejiang Provincial Natural Science Foundation of China (LY23C100001), Start-up Research Grant at Taizhou University (T20220101026), Science and Technology Program of Taizhou City (22gya03), Science and Technology Program of The First People’s Hospital of Wenling (YXHZ005), Taizhou Anti-Cancer Association special research project (TACA-C06).
Conflict of interest
The authors declare that the research was conducted in the absence of any commercial or financial relationships that could be construed as a potential conflict of interest.
Publisher’s note
All claims expressed in this article are solely those of the authors and do not necessarily represent those of their affiliated organizations, or those of the publisher, the editors and the reviewers. Any product that may be evaluated in this article, or claim that may be made by its manufacturer, is not guaranteed or endorsed by the publisher.
References
Avila, H., Yu, J., Boddu, G., Phan, A., Truong, A., Peddi, S., et al. (2022). Hydra-Elastin-like polypeptides increase rapamycin potency when targeting cell surface GRP78. Biomacromolecules 23 (8), 3116–3129. doi:10.1021/acs.biomac.2c00048
Bhattacharyya, J., Bellucci, J. J., Weitzhandler, I., McDaniel, J. R., Spasojevic, I., Li, X., et al. (2015). A paclitaxel-loaded recombinant polypeptide nanoparticle outperforms Abraxane in multiple murine cancer models. Nat. Commun. 6, 7939. doi:10.1038/ncomms8939
Bidwell, G. L. (2021). Novel protein therapeutics created using the elastin-like polypeptide platform. Physiology 36 (6), 367–381. doi:10.1152/physiol.00026.2021
Cheng, Z., Li, M., Dey, R., and Chen, Y. (2021). Nanomaterials for cancer therapy: Current progress and perspectives. J. Hematol. Oncol. 14 (1), 85. doi:10.1186/s13045-021-01096-0
Cheon, S. H., Seo, B. Y., Lee, Y. J., Sim, D., Lee, S. B., Guruprasath, P., et al. (2020). Targeting of cisplatin-resistant melanoma using a multivalent ligand presenting an elastin-like polypeptide. ACS Biomater. Sci. Eng. 6 (9), 5024–5031. doi:10.1021/acsbiomaterials.0c00599
Ciofani, G., Genchi, G. G., Mattoli, V., Mazzolai, B., and Bandiera, A. (2014). The potential of recombinant human elastin-like polypeptides for drug delivery. Expert Opin. Drug del. 11 (10), 1507–1512. doi:10.1517/17425247.2014.926885
Costa, S. A., Mozhdehi, D., Dzuricky, M. J., Isaacs, F. J., Brustad, E. M., and Chilkoti, A. (2018). Active targeting of cancer cells by nanobody decorated polypeptide micelle with bio-orthogonally conjugated drug. Nano Lett. 19 (1), 247–254. doi:10.1021/acs.nanolett.8b03837
Dang, Y., and Guan, J. (2020). Nanoparticle-based drug delivery systems for cancer therapy. Smart Mat. Med. 1 (1), 10–19. 10–19. doi:10.1016/j.smaim.2020.04.001
Ferlay, J., Colombet, M., Soerjomataram, I., Parkin, D. M., Piñeros, M., Znaor, A., et al. (2021). Cancer statistics for the year 2020: An overview. Int. J. Cancer 149 (4), 778–789. doi:10.1002/ijc.33588
Floss, D. M., Schallau, K., Rose-John, S., Conrad, U., and Scheller, J. (2010). Elastin-like polypeptides revolutionize recombinant protein expression and their biomedical application. Trends Biotechnol. 28 (1), 37–45. doi:10.1016/j.tibtech.2009.10.004
Fosgerau, K., and Hoffmann, T. (2015). Peptide therapeutics: Current status and future directions. Drug Discov. Today 20 (1), 122–128. doi:10.1016/j.drudis.2014.10.003
Gagner, J. E., Kim, W., and Chaikof, E. L. (2014). Designing protein-based biomaterials for medical applications. Acta Biomater. 10 (4), 1542–1557. doi:10.1016/j.actbio.2013.10.001
Heidari-Japelaghi, R., Haddad, R., Valizadeh, M., Dorani-Uliaie, E., and Jalali-Javaran, M. (2019). Elastin-like polypeptide fusions for high-level expression and purification of human IFN-gamma in Escherichia coli. Anal. Biochem. 585, 113401. doi:10.1016/j.ab.2019.113401
Hossen, S., Hossain, M. K., Basher, M. K., Mia, M. N. H., Rahman, M. T., and Uddin, M. J. (2019). Smart nanocarrier-based drug delivery systems for cancer therapy and toxicity studies: A review. J. Adv. Res. 15, 1–18. doi:10.1016/j.jare.2018.06.005
Hu, J., Wang, G., Liu, X., and Gao, W. (2015). Enhancing pharmacokinetics, tumor accumulation, and antitumor efficacy by elastin-like polypeptide fusion of interferon alpha. Adv. Mat. 27 (45), 7320–7324. doi:10.1002/adma.201503440
Hu, J., Xie, L., Zhao, W., Sun, M., Liu, X., and Gao, W. (2015). Design of tumor-homing and pH-responsive polypeptide-doxorubicin nanoparticles with enhanced anticancer efficacy and reduced side effects. Chem. Commun. (Camb) 51 (57), 11405–11408. doi:10.1039/c5cc04035c
Huang, K., Duan, N., Zhang, C., Mo, R., and Hua, Z. (2017). Improved antitumor activity of TRAIL fusion protein via formation of self-assembling nanoparticle. Sci. Rep. 7, 41904. doi:10.1038/srep41904
Huang, S., and Fu, X. (2010). Naturally derived materials-based cell and drug delivery systems in skin regeneration. J. Control. Release 142 (2), 149–159. doi:10.1016/j.jconrel.2009.10.018
Ibrahimova, V., González-Delgado, J. A., Levêque, M., Torres, T., Garanger, E., and Lecommandoux, S. (2021). Photooxidation responsive elastin-like polypeptide conjugates for photodynamic therapy application. Bioconjugate Chem. 32 (8), 1719–1728. doi:10.1021/acs.bioconjchem.1c00251
Kuna, M., Mahdi, F., Chade, A. R., and Bidwell, G. L. (2018). Molecular size modulates pharmacokinetics, biodistribution, and renal deposition of the drug delivery biopolymer elastin-like polypeptide. Sci. Rep. 8, 7923. doi:10.1038/s41598-018-24897-9
Liang, P., Wang, G., Liu, X., Wang, Z., Wang, J., and Gao, W. (2021). Spatiotemporal combination of thermosensitive polypeptide fused interferon and temozolomide for post-surgical glioblastoma immunochemotherapy. Biomaterials 264, 120447. doi:10.1016/j.biomaterials.2020.120447
Liu, N., Cui, M., Hu, N., Yang, F., Mu, Y., Guo, C., et al. (2022). An engineered three-in-one hybrid nanosystem from elastin-like polypeptides for enhanced cancer suppression. Colloids Surfaces B 220, 112976. doi:10.1016/j.colsurfb.2022.112976
Liu, X., Shen, Y., Zhang, X., Lin, R., Jia, Q., Chang, Y., et al. (2016). Brachytherapy using elastin-like polypeptides with 131I inhibit tumor growth in rabbits with VX2 liver tumor. Dig. Dis. Sci. 61 (10), 2921–2927. doi:10.1007/s10620-016-4212-4
Marqus, S., Elena, P., and Piva, J. T. (2017). Evaluation of the use of therapeutic peptides for cancer treatment. J. Biomed. Sci. 24 (1), 21–15. doi:10.1186/s12929-017-0328-x
Massodi, I., Bidwell, G. L., and Raucher, D. (2005). Evaluation of cell penetrating peptides fused to elastin-like polypeptide for drug delivery. J. Control. Release 108 (2–3), 396–408. doi:10.1016/j.jconrel.2005.08.007
Matsumoto, R., Hara, R., Andou, T., Mie, M., and Kobatake, E. (2014). Targeting of EGF-displayed protein nanoparticles with anticancer drugs. J. Biomed. Mat. Res. B 102 (8), 1792–1798. doi:10.1002/jbm.b.33162
McDaniel, J. R., Bhattacharyya, J., Vargo, K. B., Hassouneh, W., Hammer, D. A., and Chilkoti, A. (2013). Self-assembly of thermally responsive nanoparticles of a genetically encoded peptide polymer by drug conjugation. Angew. Chem. Int. Ed. 52 (6), 1683–1687. doi:10.1002/anie.201200899
Megeed, Z, Cappello, J., and Ghandehari, H. (2002). Genetically engineered silk-elastinlike protein polymers for controlled drug delivery. Adv. Drug Deliv. Rev. 54 (8), 1075–1091. doi:10.1016/S0169-409X(02)00063-7
Mie, M., Matsumoto, R., Mashimo, Y., Cass, A. E. G., and Kobatake, E. (2019). Development of drug-loaded protein nanoparticles displaying enzymatically-conjugated DNA aptamers for cancer cell targeting. Mol. Biol. Rep. 46 (1), 261–269. doi:10.1007/s11033-018-4467-2
Milligan, J. J., Saha, S., Jenkins, I. C., and Chilkoti, A. (2022). Genetically encoded elastin-like polypeptide nanoparticles for drug delivery. Curr. Opin. Biotechnol. 74, 146–153. doi:10.1016/j.copbio.2021.11.006
Mithieux, S. M., and Weiss, A. S. (2005). Elastin. Adv. Protein Chem. 70, 437–461. doi:10.1016/S0065-3233(05)70013-9
Mun, E. J., Babiker, H. M., Weinberg, U., EilonKirson, D., and Von Hoff, D. D. (2018). Tumor-treating fields: A fourth modality in cancer treatment. Clin. Cancer Res. 24 (2), 266–275. doi:10.1158/1078-0432.CCR-17-1117
Otake, S., and Goto, T. (2019). Stereotactic radiotherapy for oligometastasis. Cancers 11 (2), 133. doi:10.3390/cancers11020133
Patra, J. K., Das, G., Fraceto, L. F., Campos, E. V. R., Rodriguez-Torres, M. D. P., Acosta-Torres, L. S., et al. (2018). Nano based drug delivery systems: Recent developments and future prospects. J. Nanobiotechnol. 16 (1), 71–33. doi:10.1186/s12951-018-0392-8
Peddi, S., Pan, X., and MacKay, J. A. (2018). Intracellular delivery of Rapamycin from FKBP elastin-like polypeptides is consistent with macropinocytosis. Front. Pharmacol. 9, 1184. doi:10.3389/fphar.2018.01184
Pille, J., Van Lith, S. A., van Hest, J. C., and Leenders, W. P. (2017). Self-Assembling VHH-Elastin-Like peptides for photodynamic nanomedicine. Biomacromolecules 18 (4), 1302–1310. doi:10.1021/acs.biomac.7b00064
Ramamurthi, D., Selvaraj, J., Vasanth Raj, P., Tallapaneni, V., and Nanjan Chandrasekar, M. J. N. (2022). Downregulation of NT5C3 gene expressions by elastin-like polypeptide gemcitabine conjugate for ovarian cancer therapy. J. Drug Deliv. Sci. Tec. 76, 103821. doi:10.1016/j.jddst.2022.103821
Rodríguez-Cabello, J. C., Arias, F. J., Rodrigo, M. A., and Girotti, A. (2016). Elastin-like polypeptides in drug delivery. Adv. Drug Deliv. Rev. 97, 85–100. doi:10.1016/j.addr.2015.12.007
Ryu, J. S., Robinson, L., and Raucher, D. (2019). Elastin-like polypeptide delivers a Notch inhibitory peptide to inhibit tumor growth in combination with paclitaxel. J. Chemother. 31 (1), 23–29. doi:10.1080/1120009X.2018.1537554
Ryu, J. S., Kratz, F., and Raucher, D. (2021). Cell-penetrating doxorubicin released from elastin-like polypeptide kills doxorubicin-resistant cancer cells in in vitro study. Int. J. Mol. Sci. 22 (3), 1126. doi:10.3390/ijms22031126
Ryu, J. S., and Raucher, D. (2014). Antitumor efficacy of a therapeutic peptide based on thermo-responsive elastin-like polypeptide in combination with gemcitabine. Cancer Lett. 348 (1-2), 177–184. doi:10.1016/j.canlet.2014.03.021
Ryu, Jung Su, Robinson, Leslie, and Raucher, Drazen (2019b). Elastin-like polypeptide delivers a Notch inhibitory peptide to inhibit tumor growth in combination with paclitaxel. J. Chemother. 31 (1), 23–29. doi:10.1080/1120009X.2018.1537554
Sarangthem, V., Cho, E. A., Bae, S. M., Singh, T. D., Kim, S. J., Kim, S., et al. (2013). Construction and application of elastin like polypeptide containing IL-4 receptor targeting peptide. PLoS One 8 (12), e81891. doi:10.1371/journal.pone.0081891
Sarangthem, V., Cho, E. A., Yi, A., Kim, S. K., Lee, B. H., and Park, R. W. (2018). Application of bld-1-embedded elastin-like polypeptides in tumor targeting. Sci. Rep. 8, 3892. doi:10.1038/s41598-018-21910-z
Sarangthem, V., Seo, B. Y., Yi, A., Lee, Y. J., Cheon, S. H., Kim, S. K., et al. (2020). Effects of molecular weight and structural conformation of multivalent-based elastin-like polypeptides on tumor accumulation and tissue biodistribution. Nanotheranostics 4 (2), 57–70. doi:10.7150/ntno.39804
Sarangthem, V., Kim, Y., Singh, T. D., Seo, B. Y., Cheon, S. H., Lee, Y. J., et al. (2016). Multivalent targeting based delivery of therapeutic peptide using AP1-ELP carrier for effective cancer therapy. Theranostics 6 (12), 2235–2249. doi:10.7150/thno.16425
Shen, W., Hu, T., Liu, X., Zha, J., Meng, F., Wu, Z., et al. (2022). Defect engineering of layered double hydroxide nanosheets as inorganic photosensitizers for NIR-III photodynamic cancer therapy. Nat. Commun. 13 (1), 3384. doi:10.1038/s41467-022-31106-9
Shi, P., Aluri, S., Lin, Y. A., Shah, M., Edman, M., Dhandhukia, J., et al. (2013). Elastin-based protein polymer nanoparticles carrying drug at both corona and core suppress tumor growth in vivo. J. Control. Release 171 (3), 330–338. doi:10.1016/j.jconrel.2013.05.013
Shi, X., Chen, D., Liu, G., Zhang, H., Wang, X., Wu, Z., et al. (2022). Application of elastin-like polypeptide in tumor therapy. Cancers (Basel) 14 (15), 3683. doi:10.3390/cancers14153683
Simnick, A. J., Amiram, M., Liu, W., Hanna, G., Dewhirst, M. W., Kontos, C. D., et al. (2011). In vivo tumor targeting by a NGR-decorated micelle of a recombinant diblock copolypeptide. J. Control. Release 155 (2), 144–151. doi:10.1016/j.jconrel.2011.06.044
Sisila, V., Indhu, M., Radhakrishnan, J., and Ayyadurai, N. (2022). Building biomaterials through genetic code expansion. Trends Biotechnol. doi:10.1016/j.tibtech.2022.07.003
Smits, F. C., Buddingh, B. C., van Eldijk, M. B., and van Hest, J. C. (2015). Elastin-like polypeptide based nanoparticles: Design rationale toward nanomedicine. Macromol. Biosci. 15 (1), 36–51. doi:10.1002/mabi.201400419
Strohl, W. R. (2015). Fusion proteins for half-life extension of biologics as a strategy to make biobetters. BioDrugs 29 (4), 215–239. doi:10.1007/s40259-015-0133-6
Suhar, R. A., Marquardt, L. M., Shang, S., Buabbas, H., Doulames, V. M., Johansson, P. K., et al. (2020). Elastin-like proteins to support peripheral nerve regeneration in guidance conduits. ACS Biomater. Sci. Eng. 7 (9), 4209–4220. doi:10.1021/acsbiomaterials.0c01053
Sun, M., Guo, J., Hanjun, H., Tong, T., Wang, K., and Gao, W. (2018). Tumour-homing chimeric polypeptide-conjugated polypyrrole nanoparticles for imaging-guided synergistic photothermal and chemical therapy of cancer. Theranostics 8 (10), 2634–2645. doi:10.7150/thno.24705
Sweet, C., Aayush, A., Logan, R., Solomon, K. V., and Thompson, D. H. (2021). Development of a fast organic extraction–precipitation method for improved purification of elastin-like polypeptides that is independent of sequence and molecular weight. Biomacromolecules 22 (5), 1990–1998. doi:10.1021/acs.biomac.1c00096
Tibau, A., Molto, C., Alberto, O., Templeton, A. J., Del Carpio, P. L., Paggio, J. C., et al. (2018). Magnitude of clinical benefit of cancer drugs approved by the US Food and Drug Administration. J. Natl. Cancer I. 110 (5), 486–492. doi:10.1093/jnci/djx232
Vallejo, R., Gonzalez-Valdivieso, J., Santos, M., Rodriguez-Rojo, S., and Arias, F. J. (2021). Production of elastin-like recombinamer-based nanoparticles for docetaxel encapsulation and use as smart drug-delivery systems using a supercritical anti-solvent process. J. Ind. Eng. Chem. 93, 361–374. doi:10.1016/j.jiec.2020.10.013
van Oppen, L. M., Pille, J., Stuut, C., van Stevendaal, M., van der Vorm, L. N., Smeitink, J. A., et al. (2019). Octa-arginine boosts the penetration of elastin-like polypeptide nanoparticles in 3D cancer models. Eur. J. Med. Chem. 137, 175–184. doi:10.1016/j.ejpb.2019.02.010
Varanko, A. K., Su, J. C., and Chilkoti, A. (2020). Elastin-like polypeptides for biomedical applications. Annu. Rev. Biomed. Eng. 22, 343–369. doi:10.1146/annurev-bioeng-092419-061127
Yang, F., Wang, M., and Guan, X. (2022). Exosomes and mimics as novel delivery platform for cancer therapy. Front. Pharmacol. 13, 1001417. doi:10.3389/fphar.2022.1001417
Yi, A., Sim, D., Lee, S. B., Sarangthem, V., and Park, R. W. (2022). Application of bioengineered elastin-like polypeptide-based system for targeted gene delivery in tumor cells. Biomater. Biosyst. 6, 100050. doi:10.1016/j.bbiosy.2022.100050
Yi, A., Sim, D., Lee, Y. J., Sarangthem, V., and Park, R. W. (2020). Development of elastin-like polypeptide for targeted specific gene delivery in vivo. J. Nanobiotechnol. 18 (1), 15–14. doi:10.1186/s12951-020-0574-z
Zhang, W., Garg, S., Eldi, P., Zhou, F. H., Johnson, I. R., Brooks, D. A., et al. (2016). Targeting prostate cancer cells with genetically engineered polypeptide-based micelles displaying gastrin-releasing peptide. Int. J. Pharm. 513 (1-2), 270–279. doi:10.1016/j.ijpharm.2016.09.039
Zhang, Y., Wang, B., Zhao, R., Zhang, Q., and Kong, X. (2020). Multifunctional nanoparticles as photosensitizer delivery carriers for enhanced photodynamic cancer therapy. Mat. Sci. Eng. C-Mater. 115, 111099. doi:10.1016/j.msec.2020.111099
Keywords: elastin-like polypeptide, drug delivery, nanoparticle, inverse transition cycling, cancer therapy
Citation: Jiang A, Guan X, He L and Guan X (2023) Engineered elastin-like polypeptides: An efficient platform for enhanced cancer treatment. Front. Pharmacol. 13:1113079. doi: 10.3389/fphar.2022.1113079
Received: 01 December 2022; Accepted: 21 December 2022;
Published: 09 January 2023.
Edited by:
Yuxia Tang, First Affiliated Hospital, Nanjing Medical University, ChinaReviewed by:
Xuefang Hao, Inner Mongolia University for Nationalities, ChinaCopyright © 2023 Jiang, Guan, He and Guan. This is an open-access article distributed under the terms of the Creative Commons Attribution License (CC BY). The use, distribution or reproduction in other forums is permitted, provided the original author(s) and the copyright owner(s) are credited and that the original publication in this journal is cited, in accordance with accepted academic practice. No use, distribution or reproduction is permitted which does not comply with these terms.
*Correspondence: Xingang Guan, Z3VhbnhnQGNpYWMuYWMuY24=
†These authors have contributed equally to this work