- 1Medway School of Pharmacy, University of Kent and University of Greenwich, Chatham Maritime, United Kingdom
- 2Department of Pediatrics, Harbor-UCLA Medical Center, Cedars-Sinai Medical Center, David Geffen School of Medicine at UCLA, Los Angeles, CA, United States
- 3School of Engineering, Arts, Science and Technology, University of Suffolk, Ipswich, United Kingdom
Objective: Numerous pathogenic variants in KCNB1, which encodes the voltage-gated potassium channel, KV2.1, are linked to developmental and epileptic encephalopathies and associated with loss-of-function, -regulation, and -expression of the channel. Here we describe a novel de novo variant (P17T) occurring in the KV2.1 channel that is associated with a gain-of-function (GoF), with altered steady-state inactivation and reduced sensitivity to the selective toxin, guanxitoxin-1E and is clinically associated with neurodevelopmental disorders, without seizures.
Methods: The autosomal dominant variant was identified using whole exome sequencing (WES). The functional effects of the KCNB1 variant on the encoded KV2.1 channel were investigated using whole-cell patch-clamp recordings.
Results: We identified a de novo missense variant in the coding region of the KCNB1 gene, c.49C>A which encodes a p.P17T mutation in the N-terminus of the voltage-gated, KV2.1 potassium channel. Electrophysiological studies measuring the impact of the variant on the functional properties of the channel, identified a gain of current, rightward shifts in the steady-state inactivation curve and reduced sensitivity to the blocker, guanxitoxin-1E.
Interpretation: The clinical evaluation of this KCNB1 mutation describes a novel variant that is associated with global developmental delays, mild hypotonia and joint laxity, but without seizures. Most of the phenotypic features described are reported for other variants of the KCNB1 gene. However, the absence of early-onset epileptic disorders is a much less common occurrence. This lack of seizure activity may be because other variants reported have resulted in loss-of-function of the encoded KV2.1 potassium channel, whereas this variant causes a gain-of-function.
Introduction
KV2.1 (Shab-related subfamily, member 1) is a voltage-gated potassium channel protein which, in humans, is encoded by the KCNB1 gene (Alexander et al., 2021). KV2.1 channels give rise to a predominant delayed rectifier current (IK) that regulates action potential duration, firing frequency and neuronal excitability (Misonou et al., 2005). KV2.1 channels are widely expressed in the brain and are the main determinants of IK currents in several neurons including cortical and hippocampal pyramidal cells and cerebellar granule neurons (Johnson et al., 2019) where their activity contributes to pro-apoptotic events (Shah & Aizenman 2014). KV2.1 channels are also found in the heart, pancreas, pulmonary arteries, auditory outer hair cells and the retina (Johnson et al., 2019).
Each KV2.1 channel is composed of four alpha-subunits, which can either be homomeric or form heteromeric complexes with other KV channels including “silent” subunits (Salinas et al., 1997) such as, for example, with KV9.3 in cerebral arterial myocytes (O’Dwyer et al., 2020). Each alpha-subunit of a KV2.1 channel is comprised of six transmembrane spanning regions (S1 to S6), with a pore (P) loop between S5 and S6, (the S5-S6 linker) which incorporates the selectivity filter of the channel, while the voltage sensor is found on S4 (see Yellen 2002; Figure 1). The long N- and C- termini are located on the cytoplasmic side of the membrane and play a crucial role in the activation and inactivation of the channel (Ju et al., 2003).
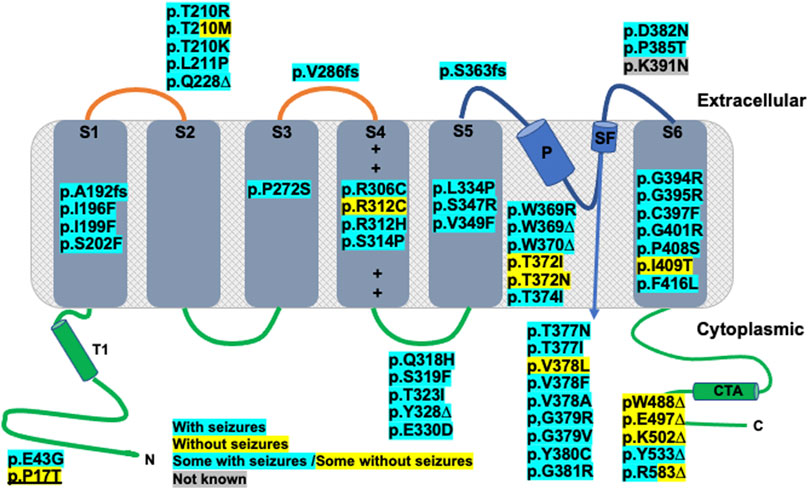
FIGURE 1. Kv2.1 protein α-subunit topology schematic representation, adapted from Bar et al., 2020 showing location of published and novel (underlined) clinical variants. Functional topology of single α-subunit consists of an N-terminal domain (N), residues 1–186, containing a tetramerization domain (T1), six transmembrane domains: S1 (residues 187–208), S2 (residues 229–250), S3 (residues 260–280), S4 (residues 295–316. + corresponds to positively charged amino acids of voltage sensor), S5 (residues 331–351), S6 (residues 392–420), pore helix (P), residues 365–376 and selectivity filter (SF), residues 377–381 and C-terminal domain (C) and tetramerization domain (CTA), residues 421–858. Truncating variants are indicated with an ∆, whilst frameshift mutations are indicated with fs. All other mutations are missense. Any variants highlighted with blue correspond to patients with seizures, those highlighted yellow correspond to patients without seizures and blue/yellow corresponds to patients with and patients without seizure. For the variant highlighted grey, seizure data was not available.
Mutations of KV2.1 channels underlie epileptic encephalopathies, infantile epilepsy, autism and other neurodevelopmental disorders in identified individuals (Torkamani et al., 2014; Saitsu et al., 2015; Thiffault et al., 2015; Calhoun et al., 2017). More recent studies have extended identification of KV2.1 variants to 74 patients with 55 distinct missense or loss of function mutations (de Kovel et al., 2017; Bar et al., 2020; Xiong et al., 2022). 85% of the patients examined (62/73) developed epilepsy and all examined (73/73) had developmental delays, albeit with varying degrees of severity (de Kovel et al., 2017; Bar et al., 2020; Xiong et al., 2022).
In this study, a 5 years old boy with neurodevelopmental delay, speech apraxia, normal growth, normal EKG, and normal EEG with no seizures, was found to have a de novo variant in KCNB1 (p.P17T; c49C>A). Proline 17 is located in the N-terminal region of the KV2.1 channel, proximal to the inactivation domain, which is distinct from all but one of the mutations described above (de Kovel et al., 2017; Bar et al., 2020; Xiong et al., 2022, Figure 1). This is a non-conservative amino acid substitution likely to impact secondary protein structure and, as such, is predicted to be a likely pathogenic variant. Here, we describe the clinical phenotype of this patient and provide a detailed functional characterization of the P17T mutated KV2.1 channel.
Materials and methods
Exome sequencing and variant analysis
Exome sequencing was carried out by GeneDx. Using genomic DNA from the proband and parents, the exonic regions and flanking splice junctions of the genome were captured using the SureSelect Human All Exon V4 (50 Mb), the Clinical Research Exome kit (Agilent Technologies, Santa Clara, CA) or the IDT xGen Exome Research Panel v1.0 (Integrated DNA Technologies, Coralville, IA). Massively parallel (NextGen) sequencing was done on an Illumina system with 100 bp or greater paired-end reads. Reads were aligned to human genome build GRCh37/UCSC hg19 and analyzed for sequence variants using a custom-developed analysis tool. A prediction tool, PROVEAN, (https://www.jcvi.org/research/provean), was used to assist in interpretation. Additional sequencing technology and the variant interpretation protocol has been previously described (Retterer et al., 2016). The general assertion criteria for variant classification are publicly available on the GeneDx ClinVar submission page (http://www.ncbi.nlm.nih.gov/clinvar/submitters/26957/).
Mammalian expression plasmids and mutagenesis
Human full-length wild-type (WT) voltage-gated channel, Shab-related subfamily, member 1, KV2.1 (KCNB1, Genbank™ NM_004975 cDNA incorporated into pCMV6-XL4 vector (Cambridge Bioscience, Cambridge, United Kingdom) and human voltage-gated channel modifier subfamily S member 3 (KCNS3, KV9.3), Genbank™ NM_002252 incorporated into pCMV6-XL5 (OriGene Techologies, Inc. United States) was utilised. The reporter plasmid, Green Fluorescent Protein (GFP) incorporated in the pcDNA3.1 vector, was a kind gift of Helen Meadows, GlaxoSmithKline, United Kingdom. The clinically identified KV2.1 mutation, proline (P) to threonine (T) at position 17 (p.P17T; c49C>A, KV2.1_P17T) was introduced using the QuikChange kit (Agilent, CA, United States) as previously described (Veale et al., 2014a). All constructs were fully sequenced by DNA Sequencing and Services, MRC/PPU, University of Dundee, Scotland.
Cell culture and transfection
All individual expression plasmids were transiently co-expressed along with the green fluorescent reporter gene GFP at a concentration of 0.5 µg per well (0.25 µg per well for the toxin experiments to allow for adequate voltage control), using a modified calcium-phosphate protocol, as previously described (Cunningham et al., 2019; Mathie et al., 2021) in a modified human embryonic kidney 293 cell line, tsA201 (European Collection of Authenticated Cell Cultures, Sigma-Aldrich, United Kingdom) prepared and maintained as previously described (Veale et al., 2014a; Cunningham et al., 2019; Mathie et al., 2021). For experiments where KV2.1 or KV2.1_P17T was co-expressed with the KV9.3 subunit a ratio of 0.5:0.5 µg was transfected.
Electrophysiology solutions and compounds
Electrophysiological experiments were conducted using an extracellular solution comprising (in mM): 145 NaCl, 2.5 KCl, 2 MgCl2, 1 CaCl2 and 10 4-(2- hydroxyethyl)-1-piperazineethanesulfonic acid (HEPES) and an intracellular solution comprising 150 KCl, 3 MgCl2, 5 EGTA and 10 HEPES, adjusted to pH 7.4 for both solutions. For extracellular solutions, containing 10 mM tetraethylammonium (TEA) an equimolar amount of NaCl was substituted. Guangxitoxin 1E (GxTx-1E) was purchased from Tocris (Bio-Techne Ltd., Abingdon, United Kingdom) and prepared in distilled water to a concentration of 10 µM and stored at −20°C until required. GxTX-1E was diluted directly into the extracellular solution prior to experimentation at a concentration of either 10 or 100 nM and cells were incubated in toxin for 20 min prior to recording.
Electrophysiological recordings in tsA201 cells
Currents passing through WT or mutant channels transiently expressed in tsA201 cells were identified by their green fluorescence (excitation 395–440 nm, emission 470–600 nm) and were recorded using whole-cell patch clamp electrophysiology using three different protocols (A–C). Protocol A (see Figure 2A) consisted of cells being held at a holding potential of -60 mV followed by a step to −80 mV for 500-millisecond (ms) then cells were stepped from −80 mV to +60 mV in 10 mV steps, over 500 m, followed by a 500 m step to −80 mV before returning to the holding potential of −60 mV. Protocol B (see Figure 3A) consisted of cells being held at a holding potential of −60 mV followed by a step to −80 mV for 500 m then cells were stepped from +50 mV to −90 mV in 10 mV steps, over 500 m, followed by a 500 m step to −80 mV before returning to the holding potential of −60 mV. For both these protocols, individual sweeps were applied once every 15 s. For Protocol C, (see Figure 5A), cells were held at −80 mV, stepped to prepulse potentials from −100 mV to +10 mV in 10 mV increments for 30 s then to a test potential of +50 mV for 500 m. Cells were stepped back to 80 mV. For protocol C, sweeps were applied once every 70 s. Currents were recorded at room temperature (20–23oC) using an Axopatch 1D patch clamp amplifier (molecular Devices), low-pass filtered at 5 kHz before sampling (2–10 kHz).
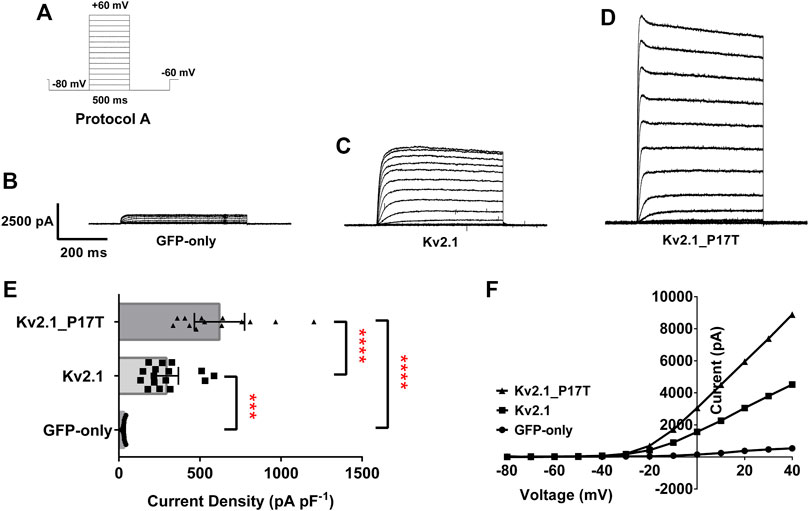
FIGURE 2. KV2.1 mutation, P17T is a gain of function mutation. (A) Schematic representation of voltage protocol A. tsA201 cells were held at −60 mV, stepped down to −80 mV for 500 m and then from −80 to +60 mV in 10 mV steps for 500 m, followed by a step back down to −80 mV for 500 m, then back to −60 mV. One sweep was recorded every 15 s, for 15 sweeps. (B) Average whole-cell current trace recorded using protocol A when expressing GFP-only.(C) Average whole-cell current trace recorded using protocol A when expressing KV2.1-WT. (D) Average whole-cell current trace recorded using protocol A when expressing KV2.1-P17T. (E) Histogram of current density (pA pF-1) measured at +50 mV from individual cells, transiently expressing 500 ng of GFP-only (n = 13), KV2.1-WT (n = 16) or KV2.1_P17T (n = 13). Error bars represent the 95% confidence intervals (CI). Statistical significance was tested using a one-way ANOVA with a post-hoc Dunnett’s multiple comparisons test, p < .01 for *** and p < 0.001 for ****. (F) Representative current voltage relationships for cells expressing GFP-only, KV2.1-WT or KV2.1-P17T.
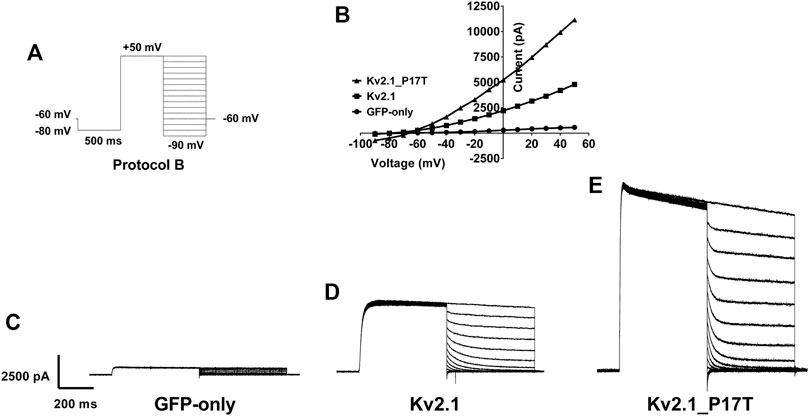
FIGURE 3. Reversal potential of currents through WT and KV2.1-P17T channels is unaltered. (A) Schematic representation of voltage protocol B. tsA201 cells were held at −60 mV, stepped down to −80 mV for 500 m then to +50 mV for 500 m. 10 mV steps from +50 to −90 mV for 500 milliseconds were then applied where the current was measured, then cells were stepped back to −60 mV, with one sweep every 15 s, for 15 sweeps.(B) Representative peak currents measured during the hyperpolarising current steps following current activation for cells expressing GFP-only, KV2.1-WT or KV2.1-P17T. Note that although the currents differ greatly in amplitude, they reverse direction at the same voltage. (C) Average whole-cell current trace recorded using protocol B when expressing GFP-only. (D) Average whole-cell current trace recorded using protocol B when expressing KV2.1-WT. (E) Average whole-cell current trace recorded using protocol B when expressing KV2.1-P17T.
Electrophysiology data analysis and statistics
A measurement of current (picoampere, pA) for WT or the mutant channel was taken at the +50 mV step following the −80 mV prepulse of Protocol B and normalized to the cells membrane capacitance (picofarad, pF). Data acquisition was performed using pClamp 10.2 software (Axon Instruments). Data acquired was analysed using GraphPad Prism six software and Microsoft Excel and expressed as the mean ±95% Confidence Intervals (CI) and n represents the number of individual cells for each experimental condition. Where current amplitudes were used for comparisons, control currents (e.g., absence of toxin) were recorded on the same experimental days. Statistical comparisons were carried out using a one-way analysis of variance (ANOVA) with a post-hoc Dunnett’s multiple comparisons test. Data was considered statistically different p < .05 (*), p < .01 (**), p < 0.001 (***) and p < 0.0001 (****).
Results
Description of clinical phenotype
This patient was the full-term product of a normal pregnancy, labor and delivery born to a 30- year-old mother and a 39-year-old father. At 18.5 months old, he presented to our clinic due to global developmental delays. He had no intelligible speech, pulled to stand, took a few steps with support, army crawled, and scooted on his bottom. At 5 years 2 months, his height was 115 cm (85th percentile), with weight 18.5 kg (45th percentile), and head circumference 52.5 cm (81st percentile). His facial features were normal with no dysmorphic features. His speech articulation was compromised by a flaccid tongue that did not articulate speech sounds well. He had mild pectus excavatum, prominent fetal finger tip pads on his middle 3 fingers, and malalignment of his toes with incurving of his fourth and fifth toes, as well as incurving of his hallux and first toe. He had everted flat feet with a wide-based gait, mild joint laxity, good strength, and mild hypotonia. He had good social skills, a very short attention span, and a high level of activity. Developmental evaluation at age 34 months showed receptive language skills at 27–30 months, expressive language skills at 15–18 months, play skills at 30–33 months, interaction/attachment at 15–18 months, and social-emotional skills at 31 months. EKG and EEG were normal, and he has never had any seizures.
Exome sequencing revealed a de novo variant (p.Pro17Thr (CCG>ACG): c.49 C>A) in exon 1 in the KCNB1 gene of this patient (Gene Dx #1859197). This individual’s mother (GeneDx #1859680) and father (GeneDx #1860837) do not harbour this P17T variant and sequence analysis and deletion testing of the mitochondrial genome in this patient was negative.
The P17T variant in the KCNB1 gene has not been reported previously. This variant is a non-conservative amino acid substitution, which is likely to impact secondary protein structure as these residues differ in polarity, charge, size and/or other properties. In-silico analyses (see Methods), suggested that P17T is a likely pathogenic variant, consistent with the global developmental delay and hypotonia seen in this individual. These findings led us to investigate the functional properties of the P17T variant directly in this study.
The P17T mutation enhances current through KV2.1 channels
Currents through homozygous human KV2.1-WT channels transiently transfected into human tsA-201 cells, were elicited by 10 mV steps in voltage from −80 to +60 mV (Protocol A, Figure 2A). This resulted in large voltage-dependent potassium currents, with characteristic outward rectification (Figures 2C, F). The average whole-cell current (pA) measured at +50 mV and normalised to cell capacitance (picofarads, pF) was 294 pA pF−1 (n = 16) 95% CI = 221 to 367 (Figure 2E). This was significantly different [p < 0.001, (95% CI = 111 to 412)] to cells expressing 500 ng GFP alone, (Figures 2B, E), which, when stimulated with the same voltage-protocol (Figures 2B, F), displayed small currents of 33 pA pF−1 (n = 13) 95% CI = 28 to 37 (Figure 2E). Untransfected (or GFP alone transfected) tsA201 cells naturally express a small background KV component (see Figure 2B), which can be blocked by TEA (10 mM), significantly reducing (p < 0.0001, 95% CI: −34 to −17) the endogenous current to 7.4 pA pF−1 (n = 4) 95% CI = 0.4 to 14 (data not shown, see also Mathie et al., 2021).
Similar experiments with homozygous human KV2.1-P17T gave outward currents of 621 pA pF−1 at +50 mV, (n = 13) 95% CI = 466 to 775 (Figures 2D–F) that were significantly larger (p < 0.0001, 95% CI = −746 to −430, GFP-only and 95% CI = −477 to −176, KV2.1-WT) than both background and KV2.1-WT currents, respectively.
To investigate the effects of the mutant channel in a heterozygous background, we co-expressed KV2.1-P17T with KV2.1-WT in a 1:1 ratio. Co-expression of KV2.1-WT with KV2.1-P17T resulted in large outward currents of 707 pA pF−1 (n = 3) 95% CI = 76 to 1,338 that were not significantly different [p > .05, using a one-way ANOVA, Dunnett’s multiple comparisons test (95% CI = −494 to 377)] from the homozygous mutant channel (KV2.1-P17T) but were significantly different [p < 0.05, (95% CI = −750 to −8)] from homozygous KV2.1-WT (see also Supplementary Figure S1). Thus, in these co-expression experiments, KV2.1-P17T appears to be acting as a dominant-positive mutant channel subunit to increase the current through heteromers with KV2.1-WT channel subunits.
The P17T mutation does not alter the ion selectivity of KV2.1 channels
A second voltage protocol was employed to determine the reversal potential of the KV2.1 WT and mutated channels. In this protocol (protocol B, Figure 3A), current was activated by a depolarising step to +50 mV before hyerpolarising steps in 10 mV increments were employed following the channel activation, in order to measure the currents’ reversal potential. From this protocol, it is clear that there is no difference in the reversal potential of currents evoked in untransfected cells (Figures 3B, C), cells transfected with KV2.1 (Figures 3B, D) or cells transfected with KV2.1-P17T (Figures 3B, E) showing that the mutated channel retains the K selectivity of the WT channel. This is an important observation, because certain mutations of KV2.1 (S347R, T374I, V378A, and G379R) do cause changes in reversal potential, indicative of effects on ion selectivity with a loss of selectivity for K+ over Na+ seen in these mutated channels (Torkamani et al., 2014; Thiffault et al., 2015). Furthermore, ion selectivity in other potassium channels in influenced by the N terminus. For example, in TREK-1 K2P channels (Veale et al., 2014b), alternative translation initiation produces a shorter N terminus truncated form of the channel with an altered ion selectivity.
The P17T mutation also enhances current through KV2.1/KV9.3 heteromeric channels
We then investigated the effect of co-expressing the KV2.1 mutant channel with an electrically silent KV channel, KV9.3, that has been shown, in vitro, to form heterotetramers with KV2.1 to modulate its electrophysiological and pharmacological properties (e.g., Patel et al., 1997; Kerschensteiner and Stocker, 1999). On their own, KV9.3 channels cannot get to the membrane and remain in the ER (Bocksteins 2016) and so do not evoke measurable functional currents (Patel et al., 1997).
Co-expression of KV9.3 (500 ng) with KV2.1-WT (500 ng) gave a current density of 95 pA pF−1 (n = 8) 95% CI = −3 to 193 (see Figures 4A, B, D). The current density of KV2.1-P17T when co-expressed with KV9.3 was 473 pA pF−1 (n = 6) 95% CI = 365 to 581 (see Figures 4A, C, D). Interestingly, KV2.1-P17T/KV9.3 current density is significantly larger than KV2.1-WT/KV9.3 [p < 0.0001 (95% CI = −665 to −90), see Figure 4A]. This suggests that KV2.1-P17T also acts as a dominant positive in the presence of Kv9.3 channels. Using protocol B, it is clear that there is no difference in the reversal potential of currents evoked in cells transfected with KV2.1-WT/KV9.3 (Figures 4E, G) or cells transfected with KV2.1-P17T/KV9.3 (Figures 4F, G) showing that the mutated channel retains the K selectivity of the WT channel in heteromultimers with KV9.3 subunits.
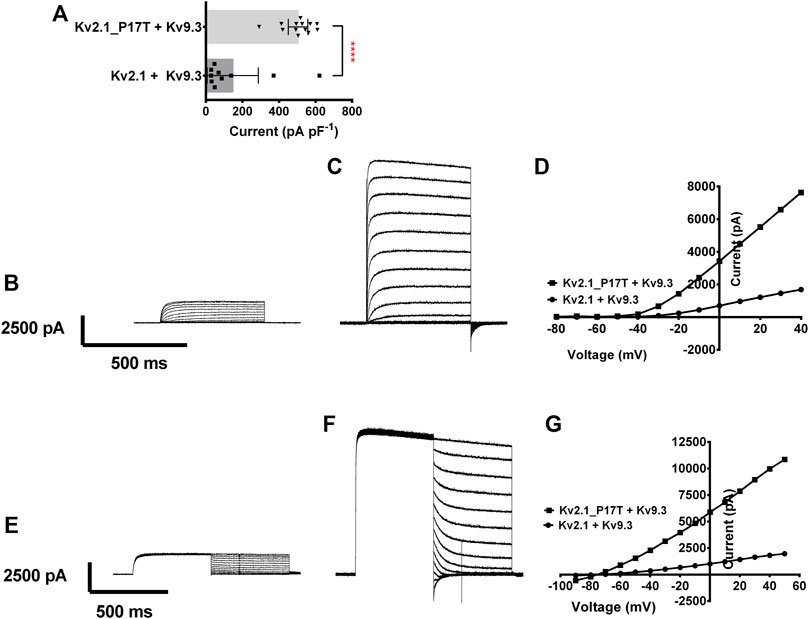
FIGURE 4. Coexpression of KV2.1-WT and KV2.1-P17T with KV9.3 shows gain of function for heteromeric channels. (A) Histogram of current density (pA pF−1) measured at +50 mV from individual cells, transiently expressing 500 ng of KV2.1-WT + KV9.3 (1:1, n = 8), or KV2.1-P17T + KV9.3 (1:1, n = 6). Error bars represent 95% CI. Statistical significance was tested using a Student’s t test, p < 0.001 for ****. (B) Average whole-cell current trace recorded using protocol A when expressing 500 ng KV2.1 + KV9.3 (1:1). (C) Average whole-cell current trace recorded using protocol A when expressing KV2.1-P17T + KV9.3 (1:1). (D) Representative current voltage relationships for cells expressing KV2.1-WT + KV9.3 (1:1), or KV2.1-P17T + KV9.3 (1:1). (E) Average whole-cell current trace recorded using protocol B when expressing 500 ng Kv2.1 + KV9.3 (1:1). (F) Average whole-cell current trace recorded using protocol B when expressing KV2.1-P17T + KV9.3 (1:1). (G) Representative peak currents measured during the hyperpolarising current steps following current activation with protocol B for cells expressing KV2.1-WT + KV9.3 (1:1), or KV2.1-P17T + KV9.3 (1:1).
The P17T mutation alters the steady state inactivation of both KV2.1 and KV2.1/KV9.3 channels, such that more channels are available for activation at depolarised voltages.
Specific N-terminal interactions between KV2.1 and modulatory alpha-subunits such as KV9.3, promote the assembly of heterotetrameric channels (Post et al., 1996; Kramer et al., 1998; Stocker et al., 1999). However, these interactions can also alter the functional properties of the resulting channels when compared to WT KV2.1 channels. For example, the steady-state inactivation of KV2.1 channels is considerably altered in the presence of KV9.3 channel subunits (Kerschensteiner and Stocker, 1999; Kerschensteiner et al., 2003). Furthermore, a number of studies have suggested that amino acids in the N terminus of both KV2.1 (Lvov et al., 2008) and other KV channels such as KV3.1 and KV1.5 (Klemic et al., 2001; Kurata et al., 2002; Kurata et al., 2005) are determinants of the slow “U-type” steady-state inactivation from closed states seen for these channels (Klemic et al., 1998), although other regions of these channels have also been shown to be important (Kurata & Fedida 2006; Coonen et al., 2020).
To determine any effect of the P17T mutation on steady-state inactivation of both KV2.1 homomeric channels and KV2.1/KV9.3 heteromeric channels, we utilised a protocol (protocol C, Figure 5A) with a long conditioning prepulse (30 s) to various voltages before the test pulse (to +50 mV), in order to reach steady-state inactivation at each voltage before the same test pulse was applied (Kerschensteiner and Stocker, 1999).
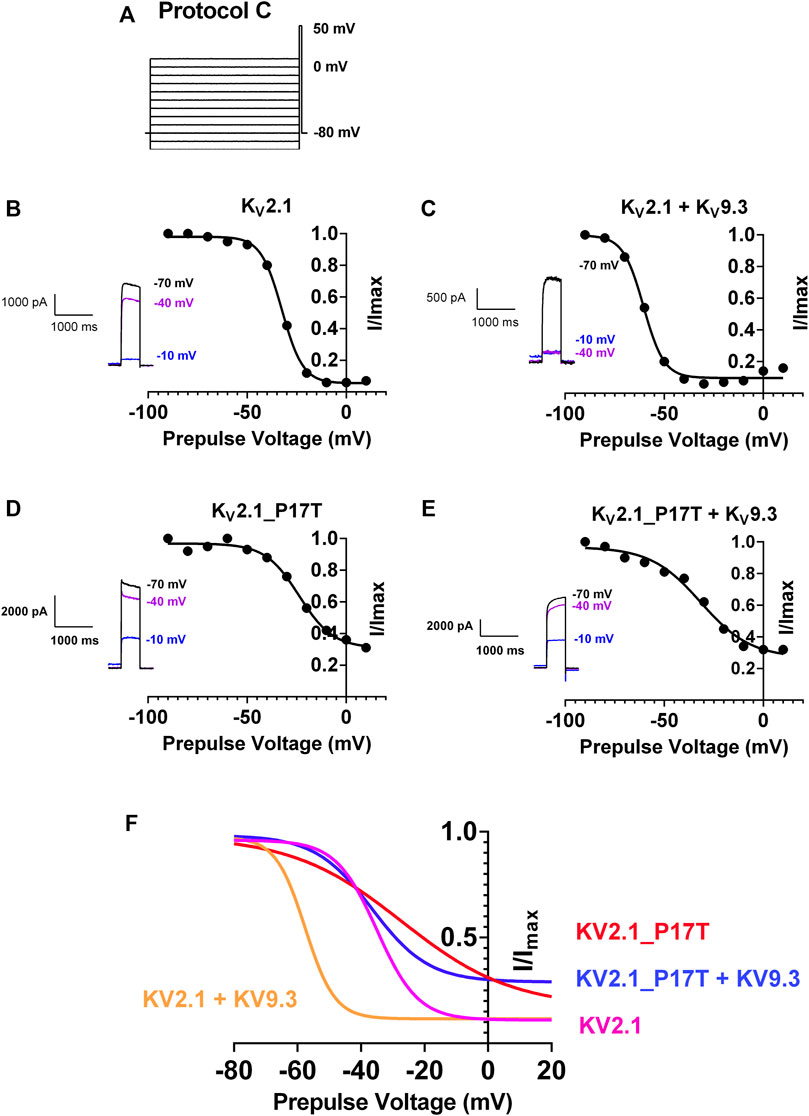
FIGURE 5. P17T mutation alters steady-state inactivation of both KV2.1 and KV2.1/KV9.3 channels. (A) Schematic representation of voltage protocol C. tsA201 cells were held at −80 mV, stepped to prepulse potentials from −100 mV to +10 mV in 10 mV increments for 30 s then to a test potential of +50 mV for 500 m. Cells were stepped back to 80 mV (B) graph of current recorded at +50 mV following a prepulse to the voltage indicated, normalised to the current seen when the prepulse was −90 mV, for KV2.1 channels. Solid line represents an unconstrained inverse Boltzmann fit to the data. Inset shows current steps at +50 mV following prepulses of −70, −40, and −10 mV (C–E) as for (B) for KV2.1/KV9.3 channels (C), KV2.1_P17T channels (D) and KV2.1_P17T/KV9.3 channels (E) respectively. (F) Superimposition of Boltzmann curves from panels (B–E).
For KV2.1 alone, unconstrained inverse Boltzmann fits to the data show that the V50 (50% steady state inactivation) occurs at −32.5 mV (95% CI = −33.5 to −31.5 mV, Figures 5B, F). This means that at a holding potential of −40 mV, say, much of the current is still available for activation (see Figure 5B inset). Co-expression with KV9.3 channels shifted the steady-state inactivation curve to the left, with a corresponding shift in V50 to −60.4 mV (95% CI = −62.7 to −58.3 mV, Figures 5C, F), so that virtually all of the current is now inactivated at −40 mV (see Figure 5C inset).
The P17T mutation shifts the steady state inactivation curve for KV2.1 to the right, with a corresponding shift in V50 to −23.7 mV (95% CI = −27.1 to −19.7 mV, Figures 5D, F) so that now some channels are available for activation even at −10 mV (see Figure 5D inset). The steady state inactivation for the P17T mutated channels co-expressed with KV9.3 is also shifted to the right, with a corresponding shift in V50 to −31.6 mV (95% CI = −37.7 to −24.4 mV, Figures 5E, F) so that again, some channels are available for activation even at −10 mV (see Figure 5E inset). This represents even more of a shift to the right in V50, for the KV2.1/KV9.3 co-expressed channels (28.8 mV) compared to KV2.1 channels alone (8.8 mV).
These shifts in the steady state inactivation curves can explain the increased currents seen for the mutated channels, when steps are made from a voltage close to the resting membrane potential (Figures 2, 3), since more mutated channels are available for activation at this voltage. Indeed, even at the highest voltage prepulse tested (+10 mV) there is still significant current available for activation in the P17T mutant KV2.1 channels whether alone or co-expressed with KV9.3.
P17T mutation alters the effectiveness of the KV2.1 channel blocking toxin, guanxitoxin-1E
The tarantula toxin, guanxitoxin-1E, is a known blocking agent of KV2.1 channels (Gupta et al., 2015), whose activity is influenced by the gating state of the KV2.1 channel (Navarro et al., 2019), being less effective at more depolarised voltages (Tilley et al., 2019). Given that the effect of this toxin is influenced by channel gating and that this is altered in the P17T mutated channels, we investigated whether the effectiveness of this toxin was also altered by the point mutation. Figure 6 shows that at a relatively low concentration (10 nM), the small but significant effect of the toxin on WT KV2.1 channels was not seen for the mutated channels. Guanxitoxin-1E (10 nM) reduced KV2.1-WT current from 199 ± 30 pA/pF (n = 12) to 122 ± 39 pA/pF (n = 6), (p < .05) but had no effect on KV2.1-P17T current (348 ± 63 pA/pF, n = 10, compared to 334 ± 40 pA/pF, n = 7 in the toxin). However, the toxin was effective on both WT and mutated KV2.1 channels at a 10-fold higher concentration (100 nM) showing that whilst the toxin has a reduced potency against the mutated channels, it can still block these channels at higher concentrations.
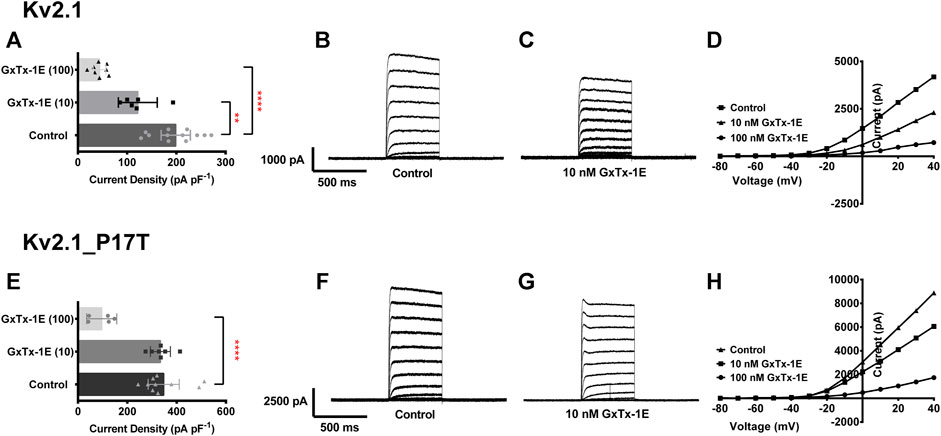
FIGURE 6. P17T mutation alters the effectiveness of the KV2.1 channel blocking toxin, guanxitoxin-1E (GxTx-1E). (A) Histogram of current density (pA pF-1) measured at +50 mV from individual cells, transiently expressing 500 ng of KV2.1-WT in control solution (n = 12), 10 nM GxTx-1E (n = 6) and 100 nM GxTx-1E (n = 8). Error bars represent 95% CI. Statistical significance was tested using a one-way ANOVA with a post-hoc Dunnett’s multiple comparisons test, p < .05 for ** and p < 0.001 for ****. (B,C) Average whole-cell current trace recorded using protocol A when expressing KV2.1-WT in the absence (B) and presence (C) of 10 nM GxTx-1E. (D) Representative current voltage relationships for cells expressing KV2.1-WT in control solution, 10 nM GxTx-1E and 100 nM GxTx-1E. (E) Histogram of current density (pA pF−1) measured at +50 mV from individual cells, transiently expressing 500 ng of KV2.1_P17T in control solution (n = 10), 10 nM GxTx-1E (n = 7) and 100 nM GxTx-1E (n = 5). Error bars represent 95% CI. Statistical significance was tested using a one-way ANOVA with a post-hoc Dunnett’s multiple comparisons test, p < 0.001 for ****. (F,G) Average whole-cell current trace recorded using protocol A when expressing KV2.1_P17T in the absence (F) and presence (G) of 10 nM GxTx-1E. (H) Representative current voltage relationships for cells expressing KV2.1_P17T in control solution, 10 nM GxTx-1E and 100 nM GxTx-1E.
Discussion
A patient has been identified with a novel mutation of KCNB1 (p.P17T; c49C>A) resulting in neurodevelopmental delay, speech apraxia, normal growth, normal EKG, and normal EEG. However, unlike 85% of patients identified with mutations in KCNB1, the patient has not developed seizures (Calhoun et al., 2017; de Kovel et al., 2017). As such, this mutation does not fit into the category of mutations known as Developmental and Epileptic Encephalopathies (DEE), whereby epileptic activity contributes to the observed developmental impairment (Scheffer et al., 2017). Other features of the phenotype, such as developmental delays and speech apraxia, however, are consistent with previous descriptions of patients with mutations of KCNB1 (Bar et al., 2020).
Of the 55 distinct missense or loss of function mutations previously described (de Kovel et al., 2017; Bar et al., 2020; Xiong et al., 2022), 49 of them were located in the S1-S6 transmembrane segments of the protein, 5 were in the C terminus and just 1 of the mutations (a missense mutation, E43G) was located in the N terminus of the channel similar to P17T. Whilst the patient with this E43G mutation did suffer from epileptic seizures they also had a predicted damaging mutation in the GABAA receptor GABRA5 which may underlie many if not all of the clinical symptoms observed (Bar et al., 2020).
Characterization of the functional properties of the KV2.1 channel, showed that the P17T mutation enhances current through these homomeric channels without changing ion selectivity of the channel. The P17T mutation is even more effective at enhancing current through KV2.1/KV9.3 channel heteromeric channels. At least part of this effect is due to a change in the steady-state inactivation curves for these channels in the presence of the P17T mutation, so that more channels are available for activation at depolarised voltages, including the normal physiological resting potential.
In addition, the inactivation of P17T channels differs from that of WT (see Figure 2C, D), in that it exhibits two distinct phases of inactivation. As such, P17T channels may have a propensity to reside in a state from where channel opening is facilitated which would also contribute to the increased current seen. In addition, differences in state transitions between P17T and WT channels may contribute to the differential effects seen for guanxitoxin (see Figure 6 and below).
KV2.1 channels are highly expressed in the brain and are involved in regulating neuronal excitability. They have been demonstrated to act as homeostatic suppressors of heightened neuronal activity (Speca et al., 2014). The gain-of-function mutation described here, increases current, either when expressed as a homotetramer or when heteromerized with KV2.1-WT or silently inactive KV channels, such as KV9.3. The GoF of these channels will act to put a brake on neuronal excitability by stabilising the resting membrane potential of the neurons where they are expressed. However, and paradoxically, if an excitatory stimulus is large enough to overcome this tonic reduced excitability, then the increased KV current present will repolarise neuronal cells more quickly following an action potential, making them more readily available to fire repetitively should the stimulation be large enough.
This functional difference in channel properties, when contrasted with the known properties of other KV2.1 channel mutations, may explain why this patient does not suffer from epileptic seizures. Other de novo variants in KV2.1 act as dominant-negative influences to reduce WT currents. These include the mutations I199F which reduces channel availability (Calhoun et al., 2017), G401R which is a dominant negative mutation (Saitsu et al., 2015) and S347R, T374I, V378A, and G379R which all cause a loss of ion selectivity and reduced current density (Torkamani et al., 2014; Thiffault et al., 2015). These will result in a depolarised membrane potential and impaired membrane repolarisation leading to increased cellular excitability. In a high-throughput study of 17 variants (Kang et al., 2019), the majority of these (14/17) were shown to result in reduced channel function though a variety of molecular mechanisms, such as lower cell surface expression and/or alterations in the voltage dependence of channel activation and inactivation, all leading to a reduction in measured current. None of these mutations showed a gain of function phenotype (Kang et al., 2019) as described here. Similarly, deletion of KV2.1 channels in mice leads to neuronal and behavioural excitability (Speca et al., 2014).
It is perhaps surprising that although the novel GoF mutation described here does not lead to epileptic seizures it does lead to several symptoms such as developmental delay and speech apraxia. This may suggest that alteration in channel function in either direction away from the baseline activity seen in the absence of mutations can lead to the same clinical outcome, as seen for the K2P potassium channel TASK-3 in KCNK9 imprinting syndrome (Cousin et al., 2022). A similar phenomenon has also been seen for the potassium channel BK (KCNMA1) where mutations of the channel lead to paroxysmal non-kinesigenic dyskinesia (PNKD3) with a broad spectrum of developmental and neurological phenotypes and seizures seen in a proportion of the patients (Liang et al., 2019). Some of these mutations are gain of function and others loss of function, albeit that gain of function mutations appear to have higher pathogenic potential (Park et al., 2022).
Alternatively, it may not the level of channel activity per se that leads to at least some of the common phenotypes observed across all mutations whether GOF or LOF, but rather, some other property of the mutated KV2.1 channels. In this respect it is of interest that KV2.1 channels have a non-conducting role in forming stable junctions between endoplasmic reticulum and plasma membranes and that this function produces effects that are independent of the channels roles in regulating membrane excitability (Maverick et al., 2021).
Interestingly, we have shown that the KV2.1 channel toxin, GxTx-1, is less effective at blocking P17T mutated channels compared to KV2.1-WT channels (Gupta et al., 2015), because the altered functional properties of the mutated channels interfere with the blocking action of the toxin (Navarro et al., 2019; Tilley et al., 2019). Other existing compounds which block KV2.1 channels may also have a different potency when used to block mutated channels, compared to WT channels, because of the altered functional properties of the channel. This has implications for any therapeutic approaches designed to reduce channel activity to resting levels, where it will be important that these approaches are tested against the mutated KV2.1 channels and not solely KV2.1-WT channels.
Data availability statement
The original contributions presented in the study are included in the article/Supplementary Materials, further inquiries can be directed to the corresponding author.
Author contributions
Electrophysiological experiments were conceived and designed by AM and EV. Patient phenotyping and review was performed by JG and KG. The mutation was prepared by EV and electrophysiological functional assays were performed by EV and AG. Data analysis was performed by EV and AM. The manuscript was prepared by AM, EV, and JG. All authors reviewed the manuscript.
Funding
EV and AM were supported by a LifeArc Centre for Therapeutics Discovery Award.
Acknowledgments
The authors would like to thank the patient and their family for their cooperation in this study.
Conflict of interest
The authors declare that the research was conducted in the absence of any commercial or financial relationships that could be construed as a potential conflict of interest.
Publisher’s note
All claims expressed in this article are solely those of the authors and do not necessarily represent those of their affiliated organizations, or those of the publisher, the editors and the reviewers. Any product that may be evaluated in this article, or claim that may be made by its manufacturer, is not guaranteed or endorsed by the publisher.
Supplementary material
The Supplementary Material for this article can be found online at: https://www.frontiersin.org/articles/10.3389/fphar.2022.1093313/full#supplementary-material
References
Alexander, S. P. H., Mathie, A., Peters, J., Veale, E. L., Striessnig, J., and Kelly, E. (2021). The concise guide to pharmacology 2021/2022: Ion channels. Br. J. Pharmacol. 178, S157–S245. doi:10.1038/sj.bjp.0706581
Bar, C., Barcia, G., Jennesson, M., Le Guyader, G., Schneider, A., Mignot, C., et al. (2020). Expanding the genetic and phenotypic relevance of KCNB1 variants in developmental and epileptic encephalopathies: 27 new patients and overview of the literature. Hum. Mutat. 41, 69–80. doi:10.1002/humu.23915
Bocksteins, E. (2016). Kv5, Kv6, Kv8, and Kv9 subunits: No simple silent bystanders. J. Gen. Physiol. 145, 105–125. doi:10.1085/jgp.201511507
Calhoun, J. D., Vanoye, C. G., Kok, F., George, A. L., and Kearney, J. A. (2017). Characterization of a KCNB1 variant associated with autism, intellectual disability, and epilepsy. Neurol. Genet. 3, e198. doi:10.1212/NXG.0000000000000198
Coonen, L., Mayeur, E., De Neuter, N., Snyders, D. J., Cuello, L. G., and Labro, A. J. (2020). The selectivity filter is involved in the U-type inactivation process of Kv2.1 and Kv3.1 channels. Biophys. J. 118, 2612–2620. doi:10.1016/j.bpj.2020.03.032
Cousin, M. A., Veale, E. L., Dsouza, N. R., Tripathi, S., Holden, R. G., Arelin, M., et al. (2022). Gain and loss of TASK3 channel function and its regulation by novel variation cause KCNK9 imprinting syndrome. Genome Med. 14 (1), 62. doi:10.1186/s13073-022-01064-4
Cunningham, K. P., Holden, R. G., Escribano-Subias, P. M., Cogolludo, A., Veale, E. L., and Mathie, A. (2019). Characterization and regulation of wild-type and mutant TASK-1 two pore domain potassium channels indicated in pulmonary arterial hypertension. J. Physiol. 597 (4), 1087–1101. doi:10.1113/JP277275
de Kovel, C. G. F., Syrbe, S., Brilstra, E. H., Verbeek, N., Kerr, B., Dubbs, H., et al. (2017). Neurodevelopmental disorders caused by de novo variants in KCNB1 genotypes and phenotypes. JAMA Neurol. 74, 1228–1236. doi:10.1001/jamaneurol.2017.1714
Gupta, K., Zamanian, M., Bae, C., Milescu, M., Krepkiy, D., Tilley, D. C., et al. (2015). Tarantula toxins use common surfaces for interacting with Kv and ASIC ion channels. eLife 4, e06774. doi:10.7554/eLife.06774
Johnson, B., Leek, A. N., and Tamkun, M. M. (2019). Kv2 channels create endoplasmic reticulum/plasma membrane junctions: A brief history of Kv2 channel subcellular localization. Channels 13, 88–101. doi:10.1080/19336950.2019.1568824
Ju, M., Stevens, L., Leadbitter, E., and Wray, D. (2003). The roles of N- and C- terminal determinants in the activation of the Kv2.1 potassium channel. J. Biol. Chem. 278, 12769–12778. doi:10.1074/jbc.M212973200
Kang, S. K., Vanoye, C. G., Misra, S. N., Echevarria, D. M., Calhoun, J. D., O'Connor, J. B., et al. (2019). Spectrum of Kv2.1 dysfunction in KCNB1-associated neurodevelopmental disorders. Ann. Neurol. 86, 899–912. doi:10.1002/ana.25607
Kerschensteiner, D., Monje, F., and Stocker, M. (2003). Structural determinants of the regulation of the voltage-gated potassium channel Kv2.1 by the modulatory α-subunit Kv9.3. J. Biol. Chem. 278, 18154–18161. doi:10.1074/jbc.M213117200
Kerschensteiner, D., and Stocker, M. (1999). Heteromeric assembly of Kv2.1 with Kv9.3: Effect on the state dependence of inactivation. Biophys. J. 77, 248–257. doi:10.1016/S0006-3495(99)76886-4
Klemic, K. G., Kirsch, G. E., and Jones, S. W. (2001). U-type inactivation of Kv3.1 and shaker potassium channels. Biophys. J. 81, 814–826. doi:10.1016/S0006-3495(01)75743-8
Klemic, K. G., Shieh, C-C., Kirsch, G. E., and Jones, S. W. (1998). Inactivation of Kv2.1 potassium channels. Biophys. J. 74, 1779–1789. doi:10.1016/S0006-3495(98)77888-9
Kramer, J. W., Post, M. A., Brown, A. M., and Kirsch, G. E. (1998). Modulation of potassium channel gating by coexpression of Kv2.1 with regulatory Kv5.1 or Kv6.1 α-subunits. Am. J. Physiol. 274, C1501–C1510. doi:10.1152/ajpcell.1998.274.6.C1501
Kurata, H. T., Doerksen, K. W., Eldstrom, J. R., Rezazadeh, S., and Fedida, D. (2005). Separation of P/C- and U-type inactivation pathways in Kv1.5 potassium channels. J. Physiol. 568, 31–46. doi:10.1113/jphysiol.2005.087148
Kurata, H. T., and Fedida, D. (2006). A structural interpretation of voltage-gated potassium channel inactivation. Prog. Biophys. Mol. Biol. 92, 185–208. doi:10.1016/j.pbiomolbio.2005.10.001
Kurata, H. T., Soon, G. S., Eldstrom, J. R., Lu, G. W. K., Steele, D. F., and Fedida, D. (2002). Amino-terminal determinants of U-type inactivation of voltage-gated K+ channels. J. Biol. Chem. 277, 29045–29053. doi:10.1074/jbc.M111470200
Liang, L., Li, X., Moutton, S., Schrier Vergano, S. A., Cogne, B., Saint-Martin, A., et al. (2019). De novo loss-of-function KCNMA1 variants are associated with a new multiple malformation syndrome and a broad spectrum of developmental and neurological phenotypes. Hum. Mol. Gen. 28, 2937–2951. doi:10.1093/hmg/ddz117
Lvov, A., Chikvashvili, D., Michaelevski, I., and Lota, I. (2008). VAMP2 interacts directly with the N terminus of Kv2.1 to enhance channel inactivation. Pflugers Arch. 456, 1121–1136. doi:10.1007/s00424-008-0468-7
Mathie, A., Veale, E. L., and Holden, R. G. (2021). Heterologous expression of ion channels in mammalian cell lines. Methods Mol. Biol. 2188, 51–65. doi:10.1007/978-1-0716-0818-0_3
Maverick, E. E., Leek, A. N., and Tamkun, M. M. (2021). Kv2-channel-AMIGOβ-subunit assembly modulates both channel function and cell adhesion molecule surface trafficking. J. Cell Sci. 134 (12), jcs256339. doi:10.1242/jcs.256339
Misonou, H., Mohapatra, D. P., and Trimmer, J. S. (2005). Kv2.1: A voltage-gated K+ channel critical to dynamic control of neuronal excitability. Neurotoxicol 26, 743–752. doi:10.1016/j.neuro.2005.02.003
Navarro, M. A., Milescu, L. S., and Milescu, M. (2019). Unlocking the gating mechanism of Kv2.1 using guangxitoxin. J. Gem. Physiol. 151, 275–278. doi:10.1085/jgp.201812254
O’Dwyer, S. C., Palacio, S., Matsumoto, C., Guarina, L., Klug, N. R., Tajada, S., et al. (2020). Kv2.1 channels play opposing roles in regulating membrane potential, Ca2+ channel function, and myogenic tone in arterial smooth muscle. PNAS U. S. A. 117, 3858–3866. doi:10.1073/pnas.1917879117
Park, S. M., Roache, C. E., Iffland, P. H., Moldenhauer, H. J., Matychak, K. K., Plante, A. E., et al. (2022). BK channel properties correlate with neurobehavioral severity in three KCNMA1-linkedchannelopathy mouse models. Elife 11, e77953. doi:10.7554/eLife.77953
Patel, A. J., Lazdunski, M., and Honoré, E. (1997). Kv2.1/Kv9.3, a novel ATP-dependent delayed-rectifier K+ channel in oxygen-sensitive pulmonary artery myocytes. EMBO J. 16, 6615–6625. doi:10.1093/emboj/16.22.6615
Post, M. A., Kirsch, G. E., and Brown, A. M. (1996). Kv2.1 and electrically silent Kv6.1 potassium channel subunits combine and express a novel current. FEBS Lett. 399, 177–182. doi:10.1016/s0014-5793(96)01316-6
Retterer, K., Juusola, J., Megan, T., Vitazka, P., Millan, F., Gibellini, F., et al. (2016). Clinical application of whole-exome sequencing across clinical indications. Genet. Med. 18, 696–704. doi:10.1038/gim.2015.148
Saitsu, H., Akita, T., Tohyama, J., Goldberg-Stern, H., Kobayashi, Y., Cohen, R., et al. (2015). De novo KCNB1 mutations in infantile epilepsy inhibit repetitive neuronal firing. Sci. Rep. 5, 15199. doi:10.1038/srep15199
Salinas, M., Duprat, F., Heurteaux, C., Hugnot, J. P., and Lazdunski, M. (1997). New modulatory alpha subunits for mammalian Shab K+ channels. J. Biol. Chem. 272, 24371–24379. doi:10.1074/jbc.272.39.24371
Scheffer, I. E., Berkovic, S., Capovilla, G., Connolly, M. B., French, J., Guilhoto, L., et al. (2017). ILAE classification of the epilepsies: Position paper of the ILAE commission for classification and terminology. Epilepsia 58, 512–521. doi:10.1111/epi.13709
Shah, N. H., and Aizenman, E. (2014). Voltage-gated potassium channels at the crossroads of neuronal function, ischemic tolerance, and neurodegeneration. Transl. Stroke Res. 5, 38–58. doi:10.1007/s12975-013-0297-7
Speca, D. J., Ogata, G., Mandikian, D., Bishop, H. I., Wiler, S. W., Eum, K., et al. (2014). Deletion of the Kv2.1 delayed rectifier potassium channel leads to neuronal and behavioral hyperexcitability. Genes Brain Behav. 13, 394–408. doi:10.1111/gbb.12120
Stocker, M., Hellwig, M., and Kerschensteiner, D. (1999). Subunit assembly and domain analysis of electrically silent K+ channel alpha-subunits of the rat Kv9 subfamily. J. Neurochem. 72, 1725–1734. doi:10.1046/j.1471-4159.1999.721725.x
Thiffault, I., Speca, D. J., Austin, D. C., Cobb, M. M., Eum, K. S., Safina, N. P., et al. (2015). A novel epileptic encephalopathy mutation in KCNB1 disrupts Kv2.1 ion selectivity, expression, and localization. J. Gen. Physiol. 146, 399–410. doi:10.1085/jgp.201511444
Tilley, D. C., Angueyra, J. M., Eum, K. S., Kim, H., Chao, L. H., Peng, A. W., et al. (2019). The tarantula toxin GxTx detains K+ channel gating charges in their resting conformation. J. Gen. Physiol. 151, 292–315. doi:10.1085/jgp.201812213
Torkamani, A., Bersell, K., Jorge, B. S., Bjork, R. L., Friedman, J. R., Bloss, C. S., et al. (2014). De novo KCNB1 mutations in epileptic encephalopathy. Ann. Neurol. 76, 529–540. doi:10.1002/ana.24263
Veale, E. L., Al Moubarak, E., Bajaria, N., Omoto, K., Cao, L., Tucker, S. J., et al. (2014b). Influence of the N-terminus on the biophysical properties and pharmacology of TREK-1 potassium channels. Mol. Pharmacol. 85, 671–681. doi:10.1124/mol.113.091199
Veale, E. L., Hassan, M., Walsh, Y., Al-Moubarak, E., and Mathie, A. (2014a). Recovery of current through mutated TASK3 potassium channels underlying birk barel syndrome. Mol. Pharmacol. 85, 397–407. doi:10.1124/mol.113.090530
Xiong, J., Liu, Z., Chen, S., Kessi, M., Chen, B., Duan, H., et al. (2022). Correlation analyses of clinical manifestations and variant effects in KCNB1-related neurodevelopmental disorder. Front. Pediatr. 9, 755344. doi:10.3389/fped.2021.755344
Keywords: Kv2.1 channel, KCNB1, pathogenic variant, developmental delay, gain of function, guanxitoxin-1E
Citation: Veale EL, Golluscio A, Grand K, Graham JM and Mathie A (2022) A KCNB1 gain of function variant causes developmental delay and speech apraxia but not seizures. Front. Pharmacol. 13:1093313. doi: 10.3389/fphar.2022.1093313
Received: 08 November 2022; Accepted: 09 December 2022;
Published: 21 December 2022.
Edited by:
Roope Mannikko, University College London, United KingdomReviewed by:
Geza Berecki, University of Melbourne, AustraliaBernhard E. Flucher, Innsbruck Medical University, Austria
Copyright © 2022 Veale, Golluscio, Grand, Graham and Mathie. This is an open-access article distributed under the terms of the Creative Commons Attribution License (CC BY). The use, distribution or reproduction in other forums is permitted, provided the original author(s) and the copyright owner(s) are credited and that the original publication in this journal is cited, in accordance with accepted academic practice. No use, distribution or reproduction is permitted which does not comply with these terms.
*Correspondence: Alistair Mathie, YS5hLm1hdGhpZUBrZW50LmFjLnVr; John M. Graham Jr., Sm9obi5HcmFoYW1AY3Nocy5vcmc=