- 1College of Public Health, Gansu University of Chinese Medicine, Lanzhou, China
- 2Provincial-level Key Laboratory for Molecular Medicine of Major Diseases and the Prevention and Treatment with Traditional Chinese Medicine Research in Gansu Colleges and University, Gansu University of Chinese Medicine, Lanzhou, China
- 3Key Laboratory of Dunhuang Medicine, Ministry of Education, Lanzhou, China
- 4College of Basic Medicine, Gansu University of Chinese Medicine, Lanzhou, China
Inflammatory bowel disease (IBD) is a chronic and life-treating inflammatory disease that can occur in multiple parts of the human intestine and has become a worldwide problem with a continually increasing incidence. Because of its mild early symptoms, most of them will not attract people’s attention and may cause more serious consequences. There is an urgent need for new therapeutics to prevent disease progression. Natural products have a variety of active ingredients, diverse biological activities, and low toxicity or side effects, which are the new options for preventing and treating the intestinal inflammatory diseases. Because of multiple genetic models, less ethical concerns, conserved signaling pathways with mammals, and low maintenance costs, the fruit fly Drosophila melanogaster has become a suitable model for studying mechanism and treatment strategy of IBD. Here, we review the advantages of fly model as screening platform in drug discovery, describe the conserved molecular pathways as therapetic targets for IBD between mammals and flies, dissect the feasibility of Drosophila model in IBD research, and summarize the natural products for IBD treatment using flies. This review comprehensively elaborates that the benefit of flies as a perfact model to evaluate the therapeutic potential of phytochemicals against IBD.
Introduction
Inflammatory bowel disease (IBD) is a chronic, progressive, life-long disease that leads to bowel damage and disability, including Crohn’s disease (CD) and ulcerative colitis (UC) (Salas et al., 2020). In recent years, the incidence of IBD has generally increased in many countries around the world, and is closely related to genetic susceptibility, environmental factors and dysbiosis, but it also brings great economic and social pressure (Guzzo et al., 2022). To date, IBD is not easy to completely cured, which encourages researchers to investigate more effective therapeutics for this disease (Che et al., 2022; Zilbauer, 2022). At present, some immunosuppressants, 5-aminosalicylic acid, and steroids have been clinically used to alleviate patients’ syndromes. However, they have serious adverse reactions in patients, such as anemia, diarrhea, and glaucoma (Zhang et al., 2021). Therefore, the development of effective and safer drugs for IBD treatment are urgently needed.
Most of research objects on drug screening and evaluation are model organisms, such as cells, C. elegans, Drosophila, zebrafish, mammals (Mccammon and Sive, 2015; Maitra and Ciesla, 2019). Model organisms are essential for investigating the pathogenesis and drug screening for human diseases. Cell culture is often used model for drug screening, but the drug toxicity reactions in the screening process cannot fully reflect the body tissue-specific responses. Although mammal models have provided crucial materials for the study of pathogenesis, pathological process and the mechanisms underlying drug-related behaviors, they are not ideal. This is mainly due to the expensive and long-term experimentations, breeding and ethical implications. Recently, Drosophila has been proved as an excellent model organism for dissecting the mechanism and drug library screening, such as cancer, aging, nociception, neurodegenerative diseases. Until now, Drosophila as a model helps researchers get the Nobel Prize in Physiology or Medicine for six times (Kitani-Morii et al., 2021). The advantages of fly are small size, genetic amenability, low-cost maintenance, and excellent genetic and molecular tools. Meanwhile, fly has a high homology with human at the organ and gene level (Maitra and Ciesla, 2019). These classcial advantages provide great opportunities for researchers to investigate the mechanism of IBD and drug discovery research (Apidianakis and Rahme, 2011; Su, 2019; Madi et al., 2021).
The pathogeny of IBD is very complicated and has not been completely understood. Disruption of intestinal homeostasis is closely related to the occurrence and development of IBD. Many signaling pathways related with IBD such as JAK/STAT, Wnt/Wg, Nrf2/Keap1, TLR4/NF-κB, Notch pathways were identified in flies, and are conserved in humans (Hu et al., 2021; Yang et al., 2022). Various natural products have shown that various natural molecules or herbal extractions are widely applied in the prevention and treatment of IBD in various animal models (Yang et al., 2022). Consistently, the similar function of natural products treating intestinal inflammatory diseases are found in flies and mammals (Pereira et al., 2017). In this article, we discussed the advantages of fly model as screening platforms in drug discovery, and described the conserved modelcular pathways as therapetic targets for IBD in fly and mammal. Nextly, we dissected the feasibility of Drosophila model in IBD research and summarized the natural products for IBD treatment in fly model.
Use of Drosophila model as screening platforms in drug discovery
Screening thousands of drug candidates need to speed various time and money, and often leads to uncertain success. At present, many models are used for screening potential drugs, such as cells, yeast, C. elegans, D. melanogaster and mammals, in which some can accelerate the process of drug discovery, when some are easy to collect valuable data (Figure 1). High-throughput screening of cell cultures is one of the most widely used methods for potential drug screening (Maitra and Ciesla, 2019). However, cell culture belongs to drug administration experiments in vitro, and the drug toxicity reactions in the screening process cannot fully reflect the body tissue-specific responses. Unbiased drug experiments using appropriate model organisms in vivo enable rapid and specific screening of drug candidates with therapeutic potential (Willoughby et al., 2013). Rodents such as rats and mice are the most common models for drug screening, but they often result in economic and ethical pressures, also have low reproductive rates and long lifespan (Bilen and Bonini, 2005). An ideal drug-screening model should be highly manipulable while reflecting human biology (Hergovich et al., 2006). Fruit fly has been universally used by researchers to investigate genetics and human diseases, such as neurodeneration, cancer, and nociception (Hwang and Lu, 2013; Kitani-Morii et al., 2021; Chiang et al., 2022; He et al., 2022). Compared to cell culture model, fruit fly is a complex “whole animal” model with organs and tissue systems functioning synergistically. Fly can be administered in a variety of ways, and its behavioral activity can be easily monitored to analyze the therapeutic and toxic effects of drugs (Shahzad et al., 2021). Compared to rodents, fly is relatively economical and easy to manipulate, and it has short generation time, large collections of transgenetic strains, and less ethical concerns (Hwang and Lu, 2013). Therefore, Drosophila is an ideal model for economical and rapid large-scale screening of therapeutically useful natural products.
The advantages of genetic manipulation
The key reason why fly can serve as a classic biological model is its highly conserved molecular pathways and powerful molecular tools that easily manipulate the expression of specific genes (Senturk and Bellen, 2018). Fly gene sequencing in 2000 shows that many basic physiological and functional characteristics are highly conserved between flies and humans, meanwhile about 75% genes of human-related diseases are homologous in flies (Bier, 2005). Homologues or orthologues of human genes in flies are easily knocked in or knocked out using genetic tools to mimic specific disease-associated phenotype. One of the widely used genetic tools is the GAL4/UAS system. GAL4 as a yeast-derived transcription factor bind to Upstream Activating Sequence (UAS), driving the downstream gene expression (Takano-Shimizu-Kouno and Ohsako, 2018). Many strains that express GAL4 can target diverse tissues, specific cells and given genes. The progeny of crosses between the targeted GAL4 and UAS strains are used to analyze the function. UAS targeted RNA interference (RNAi) or green fluorescent protein (GFP) combines with GAL4 drive to suppress specific gene expression or label fluorescent marker in any tissue or cell, which is beneficial for studying various organ and tissue diseases (Pagliarini and Xu, 2003; Weasner et al., 2017; Xie et al., 2018). This system is widely used to label specific intestinal cells and regulate signaling in intestinal cells in flies. For example, Escargot (Esg) as a specific marker for enteroblasts and intestinal stem cells (ISCs) can generate esg-Gal4; UAS-GFP reporter flies, in which the enteroblasts and ISCs are marked as GFP. External stimulation or infection significantly enhance stem cells proliferation followed by intensity of GFP increasing (Micchelli and Perrimon, 2006; Buchon et al., 2009). Drice is a negative regulator of Imd signaling and is required for intestinal homeostasis. When esg-Gal4 driver flies cross with UAS targeted Drice-RNAi flies, the offspring has decreased Drice expression in ISCs. Developed from this technique is the temporal and regional gene expression targeting (TARGET) system, in which temperature sensitive GAL4-inactivating protein GAL80 could repress GAL4 activity at permissible temperatures, which is beneficial for precise temporal control of transgene expression (Mcguire et al., 2004). The FLP recombinase/FLP recognition target (FLP/FRT) system is also commonly used to regulate gene expression or induce somatic recombination in homologous chromosomes of flies (Theodosiou and Xu, 1998). In addition, other tools such as CRISPR-Cas9 and Cre/LoxP that developed in mammalian system have also been used in flies (Nakazawa et al., 2012; Bier et al., 2018). The availability of these genetic tools makes flies as a favorable model for potential drug screening.
The advantages of phenotype-based research
Most of the drug discovery efforts carried out in flies begin with phenotype-based research, and the related phenotypes are simple and easy to detect, and reliable conclusions can be drawn in a short time (Giacomotto and Segalat, 2010; Maitra et al., 2022). For example, various neurodegenerative diseases exhibit slowness of locomotor ability and loss of a specific subset of neurons. The locomotor ability in flies is monitored by the negative geotaxis climbing test. The specific subset of neurons can be easily marked as fluorescence by using genetic methods, and are monitored by microscopy techniques (Maitra and Ciesla, 2019). Eye degeneration in Alzheimer (AD) and Parkinson (PD) transgenic fly models is used as a tool for pharmacological screening (He et al., 2021). Survival assays in flies are used to determine the role of potential drugs on lifespan, stress resistance and developmental defects (Dai et al., 2020). Simple feeding assays are used to investigate the therapeutic effect of drug candidates (Yang et al., 2022). In addition, survival assays, development and reproduction assays in flies are used to evaluate the toxicity of drug candidates and determine the optimal drug concentrations. Importantly, with easily observable phenotypes associated with gut diseases, flies have significant advantages for discovering drugs that treat IBD disease (Maitra and Ciesla, 2019). For example, the intestinal length is easily measured; integrity of the intestinal epithelial barrier is evaluated by using the “smurfs” experiments; the midgut digestive function is characterized by the gastrointestinal acid-base homeostasis (Sheng Q. et al., 2021).
Thus, using Drosophila model to screen natural drugs will help to overcome the limitiations of cell culture assays regarding toxicity and pharmacological assessment, and will also quickly reduce the scope from huge potential drug candidates. In addition, fly can be widely used to dissect the mechanism of functional compounds on disease pathogenesis.
Conserved molecular pathways as therapetic targets for intestinal inflammatory disease
The intestinal epithelium is the first line of defense in the digestive tract against pathogens entering the body, and maintains the intestinal homeostasis. Intestinal homeostasis in flies is regulated by evolutionarily conserved molecular pathways, such as JAK/STAT, Nrf2/Keap1, TLR4/NF-κB, Wnt/Wg and Notch signling pathways. An imbalance among these types of pathways in epithelium could result in IBD.
JAK/STAT pathway
The Janus kinase/signal transducer and activator of transcription (JAK/STAT) signaling pathway is a transport hub that transduces cues from extracellular cytokines into transcriptional changes in the nucleus, which participates in many cellular processes, such as cell growth, differentiation and migration of immune cells (O'Shea et al., 2013). The inappropriate activation or delection of JAK/STAT pathway is associated with inflammatory and autoimmune diseases, including IBD, Parkinson’s disease (PD) and psoriasis (Xin et al., 2020). Inhibition of this pathway can suppress multiple cytokine pathways in the treatment of IBD. JAK is a key intracellular signaling mediator in IBD, which transduces signals from cytokine receptors on the cell surface to the nucleues, and its dysregulation leads to the pathological process of IBD (Dudek et al., 2021). Presently, several JAK inhibitors are used to treat IBD patients (Wang L. et al., 2021). Tofacitinib as a JAK inhibitor is clinically used for UC patients, and various other inhibitors such as filgotinib, TD-1473 and upadacitinib are currently being investigated in preclinical and clinical trials (Salas et al., 2020; Harris and Cummings, 2021). In addition, STAT is the final effector of JAK-STAT signaling pathway (Moon et al., 2021). Some STAT inhibitors have also been studied in treating IBD, although no clinical trials have been conducted in patients with IBD (Kasembeli et al., 2018) Various plant-derived natural compounds such as curcumin, ellagic acid and paeonol have been proved to alleviate IBD by affecting the JAK-STAT pathway in IBD animal models (Marin et al., 2013; Yang et al., 2013; Moon et al., 2021). Thus, therapeutic intervention of the JAK-STAT pathway can efficiently regulate the complex inflammation driven by diverse inflammatory cytokines in IBD.
The JAK/STAT pathway in flies has the same essential signaling components as in mammals (Herrera and Bach, 2019). When enterocytes (ECs) in fly midgut are subjected to stress signaling mediated by apoptosis, chemical injury, or pathogen infection, pro-inflammatory ligands (Upd, Upd2, Upd3) are rapidly produced and released. These ligands activate one receptor Domeless (Dome), leading to the activation of one JAK and one STAT transcription factor, termed Hopscotch (Hop) and Stat 92E, respectively. The pathway activity is downregulated by Socs36E in a negative-feedback loop. Socs36E is a suppressor of cytokine signaling protein. Core components of the JAK-STAT pathway in flies are homologous to interleukin 6 (IL-6), the JAK2 and STAT5 in mammals (Myllymaki and Ramet, 2014). The JAK/STAT pathway plays an important role in fly midgut homeostasis and tissue regeneration following various challenges, such as bacterial infection, directed cell ablation or stress signaling (Buchon et al., 2009). Under normal conditions, JAK/STAT pathway facilitates the rapid proliferation and differentiation of ISCs to drive epithelial regeneration (Jiang et al., 2009). The over-activation of JAK/STAT pathway causes excessive proliferation of ISCs and abnormal differentiation of EC cells, which disrupts the balance of intestinal homeostasis, and promotes the deterioration of intestinal epithelium (Herrera and Bach, 2019).
Nrf2/Keap1 pathway
Nuclear factor-erythroid-derived 2-related factor 2 (Nrf2), a member of the basic-region leucine zipper (bZIP) transcription factor, is one of the most important regulators of the cell defense system against oxidative stress and inflammatory damage (Mou et al., 2020). Nrf2 regulates the transcription of more than 200 genes, including antioxidant proteases and inflammatory regulators, by binding antioxidant response elements (AREs) in the promoter region (Raghunath et al., 2018). The activity of Nrf2 is negatively mediated by Kelch-like ECH-associated protein 1 (Keap1) that is a protein rich in cysteine (Sekhar et al., 2010). Various studies have shown that activation of the Keap1-Nrf2-ARE signaling pathway can provide protection against various stress and inflammation-related diseases, including IBD (Cuadrado et al., 2018; Piotrowska et al., 2021). Previous studies found that DSS-induced Nrf2 knockout mice had higher expression of colonic inflammatory markers and cytokines, and more severe colonic injury compared to control colitis mice (Cheung et al., 2014). Administration of Nrf2 activator dimethyl fumarate (DMF) alleviated DSS-induced experimental colitis in mice (Li et al., 2020). The activator of Nrf2, 5-aminosalicylic acid, has been used clinically in the treatment of IBD (Kang et al., 2017; El-Baz et al., 2020). Meanwhile, various plant-derived natural compounds have been demonstrated to alleviate IBD by affecting the Keap1-Nrf2-ARE pathway in animal model systems of IBD, such as luteoline (Li et al., 2016), curcumin (Lin et al., 2019) and Flos puerariae extract (Yang et al., 2022) Therefore, the Nrf2 activator is considered as a potential drug for the treatment of IBD.
Nrf2 is highly homologous to CncC in Drosophila (Loboda et al., 2016). There are three isoforms of Cnc: CncA, CncB and CncC, of which CncC plays an important role in the oxidative stress process in flies (Pomatto et al., 2017). The mechanism of the oxidative stress response in flies is similar to that in mammals. Under non-stress conditions, CncC activity is restricted by dKeap1 (Sykiotis and Bohmann, 2008). When flies are under oxidative stress and intestinal damage, electrophile and ROS interrupt the interaction between CncC and Keap1. CncC forms a heterodimer with Maf-S in the nucleus, binds to the ARE and activates transcription of the target gene (Misra et al., 2013). Nrf2 can promote intestinal homeostasis by specifically controlling the proliferation activity of ISCs. Loss of Nrf2 in ISCs led to accumulation of ROS and accelerated degeneration of the intestinal epithelium (Hochmuth et al., 2011).
TLR4/NF-κB pathway
TLR4/NF-κB is an important inflammatory signaling transduction pathway, which closely participates in cell differentiation and proliferation, apoptosis, and pro-inflammatory response (Yu et al., 2022). Toll-like receptors (TLRs) play an important role in recognizing invading microbial pathogens and leading to innate immune response for the host defense, and also involved in the pathogenesis of IBD (Frantz et al., 2018; Lu et al., 2018). As one class of TLRs, TLR4 is the first characterized TLR in the mammalian, and mainly regulates the intestinal inflammation. The expression of TLR4 significantly increases in the intestinal epithelium of patients with active UC (Toiyama et al., 2006). Nuclear factor kappa B (NF-κB) is the final transcription factor of the TLR4 pathway. Upon activation, NF-κB dimers translocate to the nucleus, and promotes the transcription and translation of inflammatory mediators, which results in the development of intestinal diseases in mammals (Chen et al., 2018). Many components of natural products such as apigenin, luteolin and hesperidin have been proven to ameliorate intestinal inflammation by inhibiting the TLR4 receptor activation and blocking the nuclear translocation of NF-κB in mammals (Guazelli et al., 2021; Zuo et al., 2021; Begum et al., 2022; Zhang et al., 2022). Thus, downregulation of the TLR4/NF-κB pathway is a potential therapeutic strategy against IBD.
Toll signaling pathway is first identified in Drosophila (Lye and Chtarbanova, 2018). The first identification of TLRs in 1988 and then subsequent recognition of its one homolog called TLR4 in humans in 1997 (Hashimoto et al., 1988; Medzhitov et al., 1997). Activation of Toll in flies results in the formation of a signaling complex containing the adaptor proteins MyD88, Tube and the kinase Pelle via a homotypic TIR interaction (Tauszig-Delamasure et al., 2002). This complex indirectly promotes the NF-κB-like transcription factors Dif and Dorsal to the nucleus, leading to the expression of cytokines and antimicrobial peptides (AMPs) (Lamiable et al., 2016; He et al., 2022). Intestinal epithelial cells have the evolutionarily conserved TLR pathway in flies and mammals (Ferguson and Foley, 2022). Toll signaling in flies plays a role in the maintance of gut homeostasis via regulating the balance between microbe-induced epithelial cell damage and stem cell repair (Buchon et al., 2009).
Wnt/Wg pathway
Wnt signaling pathway is an important pathway for the maintenance of stem cells, which controls cell proliferation, impacts the cell cycle and regulates the self-renewal of some tissues in mammals (Nusse and Clevers, 2017; Liu et al., 2022). Wnt signaling pathway regulates the stem cell proliferation, differentiation and migration in the intestinal epithelium, and participates in the pathogenesis of IBD (Flanagan et al., 2018). Decreased the Paneth cell alpha-defensin is one of the factors in IBD pathogenesis (Khoramjoo et al., 2022). Diminished the Wnt pathway transcription factor (Tcf-4) expression could weaken enteric antimicrobial defense by reducing the Paneth cell alpha-defensin (Pu et al., 2021; Khoramjoo et al., 2022). In addition, studies have shown that in Tcf-4 knockout mice, reduced level of Paneth cell alpha-defensin in intestine permitted bacteria to invade the epithelium and result in colitis (Wehkamp et al., 2007). Inhibition of Wnt signaling pathway could disrupt the intestinal-stem-cell homeostasis, consequently leading to intestinal diseases in mammals (Kuhnert et al., 2004; Perochon et al., 2018). Various natural molecules, such as Astragaloside IV and procyanidin, have been reported to promote mucosal healing and alleviate colitis symptoms by activating the Wnt pathway in mice (Pu et al., 2021). Thus, it is worthwhile to increase the window of opportunities for IBD treatment by activating Wnt pathway.
Fly and mammalian guts not only have similar morphology, but also share the same Wnt signaling pathway. The Drosophila genome encodes seven Wnt genes including Wingless (Wg), Wnt2, Wnt4, Wnt5, Wnt6, Wnt10, and WntD (Tian et al., 2018). Only Wg and Wnt4 are expressed in the fly midgut (Perochon et al., 2018). Wnt pathway plays an important role in the self-renewal of the fly gut. When flies are exposed to damage from chemical toxins, bacterial infection and mechanical stress, the expression level of Wg protein increases in EBs of the midgut epithelium, leading to compensatory ISC proliferation and differentiation to re-establish homeostasis (Jiang et al., 2016; Liu et al., 2017). Meanwhile, the inhibition of Wnt signaling in the intestinal epithelium abolishes gut regeneration (Jiang et al., 2016; Liu et al., 2017).
Notch pathway
Notch signaling is a highly conserved cell-cell communication pathway. It regulates the development and differentiation of cells, tissue function, organs formation, and maintains the homeostasis of the body through interactions between adjacent cells (Artavanis-Tsakonas et al., 1999; Herbert and Stainier, 2011). Notch signaling pathway is critically linked to the pathogenesis of several diseases such as IBD, cancer, and autoimmune diseases (Okamoto et al., 2009). In the intestine, Notch pathway regulates the secretory of intestinal cells such as Paneth cells and goblet cells. Increased Notch pathway leads to a deficiency of Paneth cells, and ultimately induces a collapse of the intestinal barrier in patients with IBD (Gersemann et al., 2011). Activated γ-secretase promotes the generation of Notch intracellular domain (NICD) (Kumar et al., 2016). Aberrant expression of NICD leads to decrease in the quantity of goblet cells in patients with UC (Zheng et al., 2011). Consequently, studies have shown that the γ-secretase inhibitor dibenzoazepine alleviates IBD by suppressing the Notch pathway (Shinoda et al., 2010). L. acidophilus could regenerate goblet cells by inhibiting Notch transcriptional program factors to alleviate Salmonella-induced-colitis in mice (Wu et al., 2018). Thus, inhibiting Notch pathway is considered to be an effective strategy in the treatment of IBD.
The Notch gene is first named in flies in the 1910s (Stubbs et al., 1990). Most essential components of the Notch signaling pathway are conserved between flies and humans (Yang et al., 2022). Notch pathway participates in regulating the self-renewal and differentiation of ISCs. In adult Drosophila intestines, the Notch ligand Delta is specifically expressed in ISCs (Ohlstein and Spradling, 2007). Upon division of the ISC, Delta promotes the expression of Notch target genes by activating the Notch receptor in its sister cell (Fre et al., 2011). Notch pathway is closely associated with gut homeostasis. Under normal conditions, Notch pathway promotes ISCs to replenish the loss of EE and EC to maintain intestinal homeostasis (Fre et al., 2011). Ingestion of chemicals or pathogenic bacteria could disrupt stem cell differentiation and midgut homeostasis by activating Notch pathway in the Drosophila intestine (Kux and Pitsouli, 2014).
The feasibility of Drosophila model in IBD research
The Drosophila fly has been demonstrated to be an excellent model for dissecting the mechanisms of intestinal disease, due to its similar anatomical features with mammal intestine, and its genetic and functional conservation with mammals (Lin and Hackam, 2011; Medina et al., 2022). A suitable method for investigating the pathogenesis of human IBD and screening candidate drugs from natural products is to produce animal model of IBD, including fly, zebrafish, and rodents.
Conserved structure of midgut between fly and mammalian
The gastrointestinal (GI) tract is a first layer of defense against the various microbes. The fly GI tract is the tissue of digestion and absorption, and shares many properties with the mammalian counterparts, including the stomach, small intestine, and colon (Figure 2) (Liu et al., 2017). Fly midgut has emerged as an attractive system to investigate the intestinal inflammatory disease due to not only the cell lineage of this tissue is simple and well-defined, but also it shows similarites to the mammalian intestine (Jiang and Edgar, 2012). The flies intestine is composed of three main regions: foregut, midgut and hindgut (Lemaitre and Miguel-Aliaga, 2013). The foregut encompasses pharynx, oesophagus and crop, which is an organ involved in food storage. The midgut extends from the cardia to the junction with the hindgut, while the Malpighian tubules connect to the gut. The hindgut fulfills the excretory functions of the fly gastrointestinal system, which is similar with the mammal large intestine (Miguel-Aliaga et al., 2018). The copper cell region (CCR) is located approximately in the middle of the midgut and is acidic similar to the mammalian stomach (Strand and Micchelli, 2013). The posterior midgut is the most metabolically active and immune responsive region of the fly gut and is similar with the mammal small intestine, where the hindgut corresponds to the mammal colon (Micchelli and Perrimon, 2006; Capo et al., 2019).
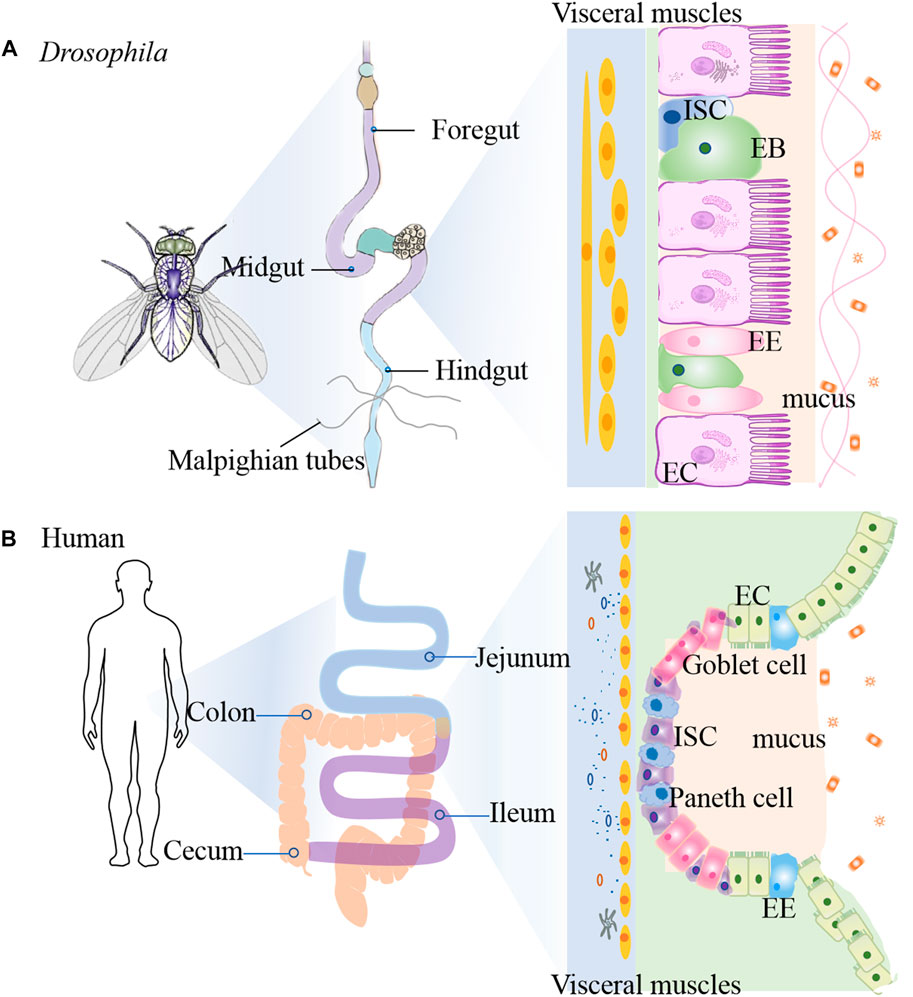
FIGURE 2. Comparison of intestinal tract anatomy between adult Drosophila melanogaster and human. The adult Drosophila (A) and human (B) intestinal tracts share structural and functional homology.
The epithelium of the fly midgut and mammal gut contains uniform ISCs that undergo division and asymmetric cell fate decision (Liu et al., 2017). The adult Drosophila gut is composed of an epithelial monolayer consisting of 4 cell types: intestinal stem cells (ISCs), absorptive enterocytes (ECs), enteroblasts (EBs) and secretory enteroendocrine (EE) cells (Medina et al., 2022). Each ISC divides symmetrically into two ISCs or asymmetrically into an renewed ISC and EB. EBs differentiate into diploid EEs or polyploid ECs (Chen et al., 2018). Similarly, ISCs self-renew and differentiate into the transit amplifying cells in mammals, and then proliferate and differentiate into secretory cells and ECs, and dedicate Paneth cell progenitors (Liu et al., 2017). ISCs are characterized by expression of high levels of cytoplasmic Delta-rich vesicles, triggering Notch signaling in neighboring EBs (Ohlstein and Spradling, 2007). Su(H)Gbe-lacZ as a transcriptional reporter of Notch signaling is used as EB cell marker (Micchelli and Perrimon, 2006). The enhancer trap fly snail family gene escargot (esg) targets both ISC and EB (Micchelli and Perrimon, 2006). Brush border Myosin (MyolA) marks the ECs and Prospero (Pros) marks the EEs (Jiang et al., 2009). Chemicals such as dextran sulfate sodium (DSS) and sodium dodecyl sulfate (SDS), or bacterial infection can damage the midgut, and also stimulate ISC proliferation (Amcheslavsky et al., 2009). Compared to mammalian stem cells, the flies possess a much simpler lineage in intestinal epithelium. However, the cellular functions and molecular principles that dictate ISC proliferation and differentiation are well conserved from flies to mammals (Medina et al., 2022). For example, JAK-STAT pathway (Xu et al., 2011), Wg/Wnt pathway (Liu et al., 2017), Hippo pathway (Ren et al., 2010) and EGFR pathway (Jiang et al., 2011). All these pathways have been implicated in human IBD. Thus, investating the role of ISC proliferation in flies will help us to find the way for human IBD mechanism.
Intestinal inflammatory model in flies
Many preclinical models of IBD are currently estabolished to investigate the pathogenesis and therapy. In rodents, DSS, SDS and 2,4,6-trinitrobenzene sulfonic acid (TNBS) have been frequently employed (Yu et al., 2022; Zhang et al., 2022). Because of the high conservation with mammals, flies are also used to induce intestinal inflammation model via feeding DSS or SDS (Figure 3) (Lee et al., 2021; Wei et al., 2022; Yang et al., 2022). Briefly, newly ecolosed (3–5 day old) female or male flies were maintained on control diet or natural products diet for 7 days. Then flies was transferred into the empty vial containg 1% agar to starve for 2 h, flies were moved into vials containing filter papers soaked with 5% sucrose solution with or without DSS (3% or 4%) or SDS (0.5% or 0.6%), respectively. Filter papers were replaced every 2 days. For survival studies, adult flies were fed with DSS or SDS until all flies died, and number of dead flies was recorded twice per day. For intestinal morphology analysis, flies treated with DSS or SDS for 72 h were dissected in the cold PBS and immediately observed under a microscope. After flies were fed with DSS or SDS for 60 h, the intestine integrity and gastrointestinal acid-base homeostasis were investigated. Smurf assay was widely used to detect the intestine integrity, in which flies were fed with food containing a blue dye (2.5% w/v) for 12 h, fly was remarked as a Smurf when the dye coloration could be observed outside the digestive tract. The bromophenol blue assay was used to measure gastrointestinal acid-base homeostasis, in which flies were fed with 2% Brmophenol blue sodium (Sigma, B5525) for 12 h, images were captured after dissection. For observation of midgut epithelial cells, flies were fed with DSS or SDS for 72 h, then the nucleus and microvilli of midgut epithelial cells were observed by using transmission electron microscope. For dead intestinal cells detection, the dissected guts of flies fed with DSS or SDS for 72 h were stained with 7-amino-actinomycin D (7-AAD) for 30 min. The imaged were observed under confocal microscope. For reactive oxygen species assay, flies were exposed to SDS or DSS for 48 h, the intestines were dissected in cold PBS, incubated in 5 μM H2DCFDA or 5 μM dihydroethidium (DHE) for 10 min in dark environment, then washed in PBST, and immediately observed under a confocal microscope. For detecting proliferation and differentiation of ISCs, the guts of esg-GAl4; UAS-GFP flies or Dl-GAl4; UAS-GFP flies were dissected, and observed under a confocal microscope. The number of progenitor cells or ISCs was performed by counting the number of GFP positive cells per unit area.
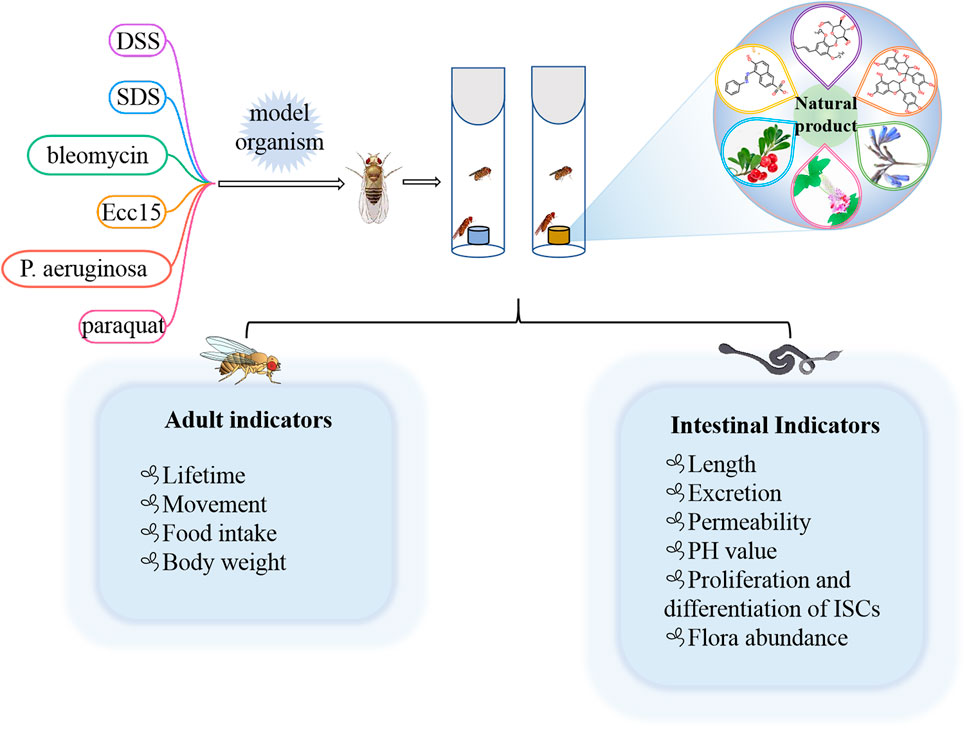
FIGURE 3. Different chemical inducers were used to construct IBD models in Drosophila, and effective natural products were screened by detecting corresponding indicators in adults and intestines.
Some of the less common agents such as bleomycin, P. aeruginosa, Erwinia carotovora 15 (Ecc15), and paraquat could also lead to gut injury in Drosophila (Amcheslavsky et al., 2009; Apidianakis et al., 2009; Lei et al., 2022). For example, bleomycin leaded to enterocyte-specific damage and cell loss in gut of flies, which in turn caused ISC to divide faster and facilitated enteroblast differentiation into new enterocytes (Amcheslavsky et al., 2009). Oral administration of P. aeruginosa and Ecc15 strains could increase the number of intestinal progenitors and induced apoptosis of mature cells to establish an intestinal injury model (Apidianakis et al., 2009; Lei et al., 2022). Overall, the current methods used to estabolish the IBD model in flies are easy and simple to operate. It is beneficial for researchers exploring the occurrence and development mechanism of IBD in human and screening the potential drugs from nature products.
Natural products screening for IBD treatment in flies
Currently, the clinical drugs for IBD treatment are mainly synthetic compounds such as aminosalicylic acids, corticosteroids, immunosuppressants, biological agents, etc., which have many side effects (Neurath, 2017). Natural products as secondary metabolite have a wide range of biological activities and a high degree of bio-availability. Their multi-component and multi-target action characteristics have unique advantages in the prevention and treatment of IBD. Until now, various natural molecules and herbal extractions have been found to treat IBD (Duan et al., 2021). Therefore, it is very important to further screen natural products that have therapeutic effects on IBD using different animial model (Ding et al., 2017; Shao et al., 2019). Here, we summarized the natural molecules and herbal extractions that have significantly protective and therapeutic effects on intestinal inflammation in flies (Figure 4).
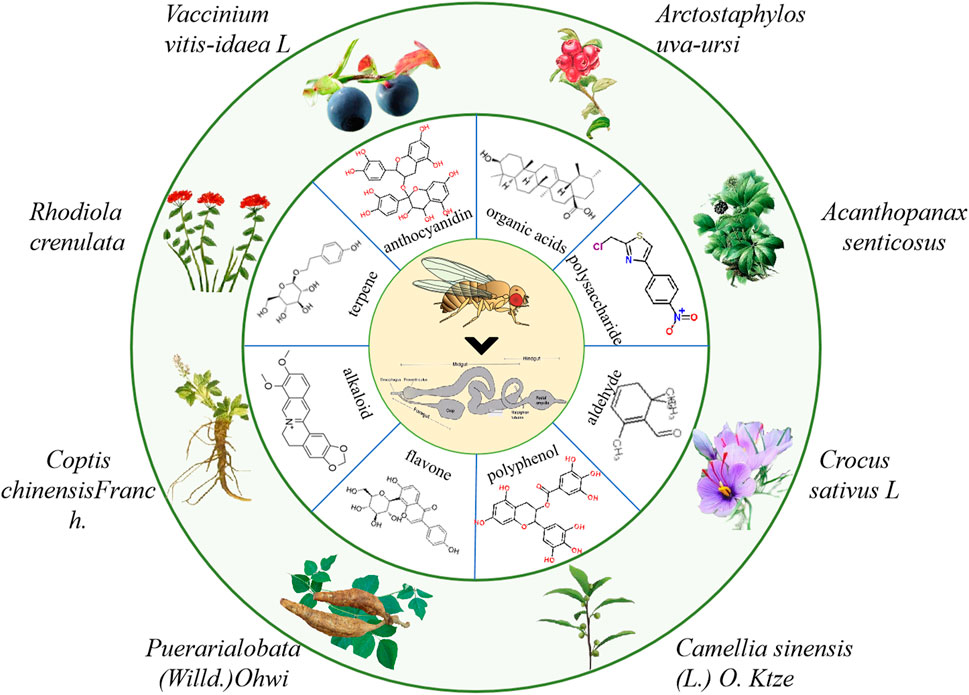
FIGURE 4. The information of partial natural products that play a crucial role in the treatment of IBD in flies.
Natural molecules for protecting intestinal injury
Natural molecules have exhibited efficiency in protecting intestinal injury and improving symptoms in flies. For example, Acanthopanax senticosus polysaccharides (ASPS) supplementation could improve the disrupted intestinal homeostasis in flies under SDS stimulation, in which reduced the intestinal epithelial cell death, decreased ROS accumulation and antimicrobial peptide (AMP) expression (Zhang et al., 2020). Administration of ASPS also reduced the excessive ISCs proliferation and differentiation mainly by epidermal growth factor receptor (EGFR), JNK and Notch signaling pathway when flies were exposed to DSS (Lei et al., 2022). Consistently, ASPS supplementation in mice could also ameliorate LPS-induced intestinal injury, including decreased intestinal morphological deterioration, elevated the mucosal barrier and enhanced intestinal tight junction proteins expression, which mainly through inhibiting TLR4/NF-κB signaling pathway (Yasueda et al., 2020). Safranal as one of the main components of saffron significantly alleviated the DSS or Ecc15 induced intestinal epithelial cell death and excessive proliferation of ISCs to protect intestinal integrity in flies (Lei et al., 2022). This protective process was regulated through inhibition of the JAK/STAT signaling, EGFR signaling, and JNK signaling pathways in flies (Huang et al., 2022). The protective function of safranal were also reported in vitro and mice (Lertnimitphun et al., 2019), in which safranal supplementation decreased NO production, COX-2 and iNOS in LPS-stimulated RAW264.7 cells, and also alleviated severity of inflammation and crypt damage in the DSS-induced colitis mice. These studies elucidate that safranal may be a candidate for IBD therapy. Agar oligosaccharides (AOS) are marine prebiotics with significant anti-inflammatory effects (Ma et al., 2019). AOS supplementation alleviated the injuries of microvilli and mitochondria of gut, ameliorated the intestinal inflammation by modulating the microbiota and the gene expression of AMPs, mTOR and AMPK pathways that related with immune and cell autophagy in SDS-induced inflammatory model of flies (Ma et al., 2021). Ursolic acid (UA) is an anti-inflammatory natural triterpenoid widely distributed in various vegetables and fruits (Checker et al., 2012). UA could remarkably prevent intestine injury in SDS-stimuated flies by inhibiting ISCs hyperproliferation, decreasing excessive activation of JNK/JAK/STAT signaling pathway (Wei et al., 2022). Meanwhile, UA was found to alleviate the DSS-induced intestinal damage by reducing the upregulation of NF-κB in mice (Liu et al., 2016; Ma et al., 2021). Caffeic acid (CA) is a widespread natural phenolic small molecule, which also inhibited the dysregulation of ISCs, ameliorated the gut hyperplasia defect, and reduced aging induced mortality in flies (Sheng et al., 2021). CA could significantly attenuate the DSS-induced murine UC mainly via ameliorating the disease severity, loss of eptithelium and crypts, mucosal ulcerations, and secretion of inflammatory cytokines (Xiang et al., 2021). Polysaccharide from Premna microphylla turcz (PPMT) have anti-inflammatory functions in vitro (Li et al., 2021). In SDS-induced inflammatory flies, PPMT significantly prolonged the lifespan, reduced the rupture of microvilli and restored the nuclear structure in the midgut, and improved gene expression levels of immune-related AMP pathway, mTOR pathway and Imd pathway (Ma et al., 2021).
Herbal extractions for protecting intestinal injury
Some herbal extractions have also been validated to have great protective function in fly model of IBD. For example, bilberry anthocyanins extracts (BANCs) have a wide range of biological activities and can be used to prevent or treat inflammation-related diseases (Farzaei et al., 2015). In DSS-induced inflammatory flies, BANCs remarkably enhanced the survival rate, restored the intestinal morphology and integrity, which mainly by modulating Nrf2 signaling pathway (Zhang et al., 2022). Consistently, BANCs could reduce intestinal inflammation in acute and chronic DSS-colitis with decreased histological scores and cytokine secretion in DSS-induced Balb/c mice (Piberger et al., 2011). Our previous studies found that Flos Puerariae extract (FPE) ameliorated the intestinal inflammation via modulating intestinal integrity and various signaling pathways in SDS-inflamed flies, in which FPE enhanced the survival rate, maintained intestinal morphological integrity, reduced the ISCs proliferation, and also rescued the altered expression levels of gene and protein in JAK-STAT signaling, Nrf2/Keap1 signaling and Wnt signaling pathways in the gut (Yang et al., 2022). Larvae of the Allomyrina dichotoma (ADL) as a high nutritional food are widely used to treat gut-related disease in China and Korea. In DSS-fed flies, oral administration of ADL extract remarkably increased the survival rate, reduced intestinal cell apoptosis and gut permeability. Meanwhile, ADL extract supplementation promoted the E-cadherin gene expression and restored the original membrane localization of DSS-disrupted E-cadherin contiguous with the armadillo (Lee et al., 2021). Rhodiola crenulata is widely used in phytotherapy in Asian countries and Eastern European (Chen et al., 2020). R crenulata extracts supplementation could prevent inflammatory diseases of the intestine in flies, in which protected against shorten intestinal length and epithelial cell death, decreased ROS levels, and increased the expression of antimicrobial peptide genes under bacterial and SDS stimulation (Zhu et al., 2014). Furthermore, the protective function of R crenulata extracts were also reported in mice. Ingestion of R crenulata extracts alleviated damage of inflammation, maintained intestinal barrier function, inhibited cell apoptosis and regulated gut microbiome in DSS-induced colitis mice (Wang et al., 2021). Extracts of Crocus sativus L. supplementation protected against SDS-induced intestinal damage in flies mainly via decreasing epithelial cell death and ROS levels in the gut (Liu et al., 2016). However, because of multiple compounds in these herbal extractions, the active ingredients and mechanisms have not been determined clearly, which need to be further explored in various animal model of IBD in future.
As mentioned above, the pharmacological function of many natural molecules and herbal extractions in treating IBD is conserved in Drosophila and rodents. Thus, Drosophila can be used as an excellent model for screening natural products for treating IBD that can be subsequently validated in a mammal system (Table 1).
Concluding remarks and future directions
The fruit fly has been proved as an excellent model organism for investigating the mechanism and drug library screening of cancer (Gonzalez, 2013), nociception (He et al., 2022), and neurodegenerative diseases (Sneddon, 2018); Here, we have emphasized that Drosophila is widely used to study the molecular mechanisms of IBD and is a perfect model for high-throughput drug screening from natural products. The major advantages of flies are its sophisticated genetics, low cost, high fecundity, and short generation time. The fly genome contains about 14,000 genes and many are well-conserved in mammals (Bier, 2005). Furthermore, it would avoid the ethical controversy if using fruit flies as human disease model. In addition, fly as a fast-track model could be used for screening novel compounds from the large chemical libraies, which will shorten the time from experimental setup to clinical use.
Despite the conservation of important basic cell processes in flies and mammals, there are still some differences between flies and mammals. Flies have some limitations in the study of intestinal inflammatory disease. First of all, due to differences in physiology and development, it is difficult to directly apply the results of Drosophila intestinal microbiota to mammals. Second, there are the extensive anatomical differences in gut between flies and mammals. Flies lack specific vertebrate cell types such as goblet cells, tuft cells, paneth cells, and M cells. Third, immune systems are different between mammalian and fly. Drosophila does not have the acquired immune system found in mammals, and solely depends on general mechanisms of innate immunity for its immune defenses (Lemaitre and Hoffmann, 2007). However, flies have high conserved features for innate immunity with mammals, such as immune cascades, signal transduction pathways, and transcriptional regulators (Arch et al., 2022).
In general, flies offer value as parallel alternatives to mammal models in use for screening drugs that treat IBD. Its potential as intestinal inflammatory disease research model is important for discovering mechanisms of intestinal disease and potential therapeutics.
Author contributions
JH and MX contributed to conception and design of the study. MX, YW, DY, XZ, YD and BL wrote the paper. JH, YL and XL criticaly revised the manuscript. All authors read and approved the final manuscript.
Funding
This work was supported by the National Natural Science Foundation of China (Nos. 82004228, 82104562), Gansu Lngyuan Youth Talent Innovation and Entrepreneurship Project, Talent Introduction Project in Gansu University of Chinese Medicine (No. 2018YJRC-11), The Project from the Key Laboratory of Dunhuang Medicine, Ministry of Education (Nos. DHYX19-04, DHYX19-05) (Nos. 2022CX33, 2021CX34).
Acknowledgments
We would like to acknowledge the contribitions of members of He laboratory for useful comments on the manuscript.
Conflict of interest
The authors declare that the research was conducted in the absence of any commercial or financial relationships that could be construed as a potential conflict of interest.
Publisher’s note
All claims expressed in this article are solely those of the authors and do not necessarily represent those of their affiliated organizations, or those of the publisher, the editors and the reviewers. Any product that may be evaluated in this article, or claim that may be made by its manufacturer, is not guaranteed or endorsed by the publisher.
References
Amcheslavsky, A., Jiang, J., and Ip, Y. T. (2009). Tissue damage-induced intestinal stem cell division in Drosophila. Cell Stem Cell 4 (1), 49–61. doi:10.1016/j.stem.2008.10.016
Apidianakis, Y., Pitsouli, C., Perrimon, N., and Rahme, L. (2009). Synergy between bacterial infection and genetic predisposition in intestinal dysplasia. Proc. Natl. Acad. Sci. U. S. A. 106 (49), 20883–20888. doi:10.1073/pnas.0911797106
Apidianakis, Y., and Rahme, L. G. (2011). Drosophila melanogaster as a model for human intestinal infection and pathology. Dis. Model. Mech. 4 (1), 21–30. doi:10.1242/dmm.003970
Arch, M., Vidal, M., Koiffman, R., Melkie, S. T., and Cardona, P. J. (2022). Drosophila melanogaster as a model to study innate immune memory. Front. Microbiol. 13, 991678. doi:10.3389/fmicb.2022.991678
Artavanis-Tsakonas, S., Rand, M. D., and Lake, R. J. (1999). Notch signaling: Cell fate control and signal integration in development. Science 284 (5415), 770–776. doi:10.1126/science.284.5415.770
Begum, N., Rajendra, P. N., Kanimozhi, G., and Agilan, B. (2022). Apigenin prevents gamma radiation-induced gastrointestinal damages by modulating inflammatory and apoptotic signalling mediators. Nat. Prod. Res. 36 (6), 1631–1635. doi:10.1080/14786419.2021.1893316
Bier, E. (2005). Drosophila, the golden bug, emerges as a tool for human genetics. Nat. Rev. Genet. 6 (1), 9–23. doi:10.1038/nrg1503
Bier, E., Harrison, M. M., O'Connor-Giles, K. M., and Wildonger, J. (2018). Advances in engineering the fly genome with the CRISPR-cas system. Genetics 208 (1), 1–18. doi:10.1534/genetics.117.1113
Bilen, J., and Bonini, N. M. (2005). Drosophila as a model for human neurodegenerative disease. Annu. Rev. Genet. 39, 153–171. doi:10.1146/annurev.genet.39.110304.095804
Buchon, N., Broderick, N. A., Poidevin, M., Pradervand, S., and Lemaitre, B. (2009). Drosophila intestinal response to bacterial infection: Activation of host defense and stem cell proliferation. Cell Host Microbe 5 (2), 200–211. doi:10.1016/j.chom.2009.01.003
Capo, F., Wilson, A., and Di Cara, F. (2019). The intestine of Drosophila melanogaster: An emerging versatile model system to study intestinal epithelial homeostasis and host-microbial interactions in humans. Microorganisms 7 (9), 336. doi:10.3390/microorganisms7090336
Che, Z., Ye, Z., Zhang, X., Lin, B., Yang, W., Liang, Y., et al. (2022). Mesenchymal stem/stromal cells in the pathogenesis and regenerative therapy of inflammatory bowel diseases. Front. Immunol. 13, 952071. doi:10.3389/fimmu.2022.952071
Checker, R., Sandur, S. K., Sharma, D., Patwardhan, R. S., Jayakumar, S., Kohli, V., et al. (2012). Potent anti-inflammatory activity of ursolic acid, a triterpenoid antioxidant, is mediated through suppression of NF-κB, AP-1 and NF-AT. PLoS One 7 (2), e31318. doi:10.1371/journal.pone.0031318
Chen, C. Y., Kao, C. L., and Liu, C. M. (2018a). The cancer prevention, anti-inflammatory and anti-oxidation of bioactive phytochemicals targeting the TLR4 signaling pathway. Int. J. Mol. Sci. 19 (9), 2729. doi:10.3390/ijms19092729
Chen, H. I., Ou, H. C., Chen, C. Y., Yu, S. H., Cheng, S. M., Wu, X. B., et al. (2020). Neuroprotective effect of rhodiola crenulata in D-galactose-induced aging model. Am. J. Chin. Med. 48 (2), 373–390. doi:10.1142/S0192415X20500196
Chen, J., Xu, N., Wang, C., Huang, P., Huang, H., Jin, Z., et al. (2018b). Transient Scute activation via a self-stimulatory loop directs enteroendocrine cell pair specification from self-renewing intestinal stem cells. Nat. Cell Biol. 20 (2), 152–161. doi:10.1038/s41556-017-0020-0
Chen, Y., Miao, Z., Sheng, X., Li, X., Ma, J., Xu, X., et al. (2022). Sesquiterpene lactones-rich fraction from Aucklandia lappa Decne. alleviates dextran sulfate sodium induced ulcerative colitis through co-regulating MAPK and Nrf2/Hmox-1 signaling pathway. J. Ethnopharmacol. 295, 115401. doi:10.1016/j.jep.2022.115401
Cheung, K. L., Lee, J. H., Khor, T. O., Wu, T. Y., Li, G. X., Chan, J., et al. (2014). Nrf2 knockout enhances intestinal tumorigenesis in Apc(min/+) mice due to attenuation of anti-oxidative stress pathway while potentiates inflammation. Mol. Carcinog. 53 (1), 77–84. doi:10.1002/mc.21950
Chiang, M. H., Ho, S. M., Wu, H. Y., Lin, Y. C., Tsai, W. H., Wu, T., et al. (2022). Drosophila model for studying gut microbiota in behaviors and neurodegenerative diseases. Biomedicines 10 (3), 596. doi:10.3390/biomedicines10030596
Choi, K. C., Cho, S. W., Kook, S. H., Chun, S. R., Bhattarai, G., Poudel, S. B., et al. (2016). Intestinal anti-inflammatory activity of the seeds of Raphanus sativus L. in experimental ulcerative colitis models. J. Ethnopharmacol. 179, 55–65. doi:10.1016/j.jep.2015.12.045
Cuadrado, A., Manda, G., Hassan, A., Alcaraz, M. J., Barbas, C., Daiber, A., et al. (2018). Transcription factor NRF2 as a therapeutic target for chronic diseases: A systems medicine approach. Pharmacol. Rev. 70 (2), 348–383. doi:10.1124/pr.117.014753
Dai, Z., Li, D., Du, X., Ge, Y., Hursh, D. A., and Bi, X. (2020). Drosophila Caliban preserves intestinal homeostasis and lifespan through regulating mitochondrial dynamics and redox state in enterocytes. PLoS Genet. 16 (10), e1009140. doi:10.1371/journal.pgen.1009140
Ding, A. J., Zheng, S. Q., Huang, X. B., Xing, T. K., Wu, G. S., Sun, H. Y., et al. (2017). Current perspective in the discovery of anti-aging agents from natural products. Nat. Product. Bioprospecting. 7 (5), 335–404. doi:10.1007/s13659-017-0135-9
Duan, L., Cheng, S., Li, L., Liu, Y., Wang, D., and Liu, G. (2021). Natural anti-inflammatory compounds as drug candidates for inflammatory bowel disease. Front. Pharmacol. 12, 684486. doi:10.3389/fphar.2021.684486
Dudek, P., Fabisiak, A., Zatorski, H., Malecka-Wojciesko, E., and Talar-Wojnarowska, R. (2021). Efficacy, safety and future perspectives of JAK inhibitors in the IBD treatment. J. Clin. Med. 10 (23), 5660. doi:10.3390/jcm10235660
El-Baz, A. M., Khodir, A. E., Adel, E. M., and Shata, A. (2020). The protective effect of Lactobacillus versus 5-aminosalicylic acid in ulcerative colitis model by modulation of gut microbiota and Nrf2/Ho-1 pathway. Life Sci. 256, 117927. doi:10.1016/j.lfs.2020.117927
Farzaei, M. H., Rahimi, R., and Abdollahi, M. (2015). The role of dietary polyphenols in the management of inflammatory bowel disease. Curr. Pharm. Biotechnol. 16 (3), 196–210. doi:10.2174/1389201016666150118131704
Feng, J., Set, B. K., Hee, J. L., Su, M. S., Soon, S. L., and Hong, W. S. (2021). Effects of Salvia miltiorrhiza Bunge extract and its single components on monosodium urate-induced pain in vivo and lipopolysaccharide-induced inflammation in vitro. J. Tradit. Chin. Med. 41 (2), 219–226. doi:10.19852/j.cnki.jtcm.20210224.002
Ferguson, M., and Foley, E. (2022). Microbial recognition regulates intestinal epithelial growth in homeostasis and disease. FEBS J. 289 (13), 3666–3691. doi:10.1111/febs.15910
Flanagan, D. J., Austin, C. R., Vincan, E., and Phesse, T. J. (2018). Wnt signalling in gastrointestinal epithelial stem cells. Genes 9 (4), 178. doi:10.3390/genes9040178
Frantz, S., Falcao-Pires, I., Balligand, J. L., Bauersachs, J., Brutsaert, D., Ciccarelli, M., et al. (2018). The innate immune system in chronic cardiomyopathy: A European society of cardiology (ESC) scientific statement from the working group on myocardial function of the ESC. Eur. J. Heart Fail. 20 (3), 445–459. doi:10.1002/ejhf.1138
Fre, S., Bardin, A., Robine, S., and Louvard, D. (2011). Notch signaling in intestinal homeostasis across species: The cases of Drosophila, zebrafish and the mouse. Exp. Cell Res. 317 (19), 2740–2747. doi:10.1016/j.yexcr.2011.06.012
Gersemann, M., Stange, E. F., and Wehkamp, J. (2011). From intestinal stem cells to inflammatory bowel diseases. World J. Gastroenterol. 17 (27), 3198–3203. doi:10.3748/wjg.v17.i27.3198
Giacomotto, J., and Segalat, L. (2010). High-throughput screening and small animal models, where are we? Br. J. Pharmacol. 160 (2), 204–216. doi:10.1111/j.1476-5381.2010.00725.x
Gonzalez, C. (2013). Drosophila melanogaster: A model and a tool to investigate malignancy and identify new therapeutics. Nat. Rev. Cancer. 13 (3), 172–183. doi:10.1038/nrc3461
Guazelli, C., Fattori, V., Ferraz, C. R., Borghi, S. M., Casagrande, R., Baracat, M. M., et al. (2021). Antioxidant and anti-inflammatory effects of hesperidin methyl chalcone in experimental ulcerative colitis. Chem. Biol. Interact. 333, 109315. doi:10.1016/j.cbi.2020.109315
Guzzo, G. L., Andrews, J. M., and Weyrich, L. S. (2022). The neglected gut microbiome: Fungi, Protozoa, and bacteriophages in inflammatory bowel disease. Inflamm. Bowel Dis. 28 (7), 1112–1122. doi:10.1093/ibd/izab343
Han, J., Liu, L., Yu, N., Chen, J., Liu, B., Yang, D., et al. (2016). Polysaccharides from Acanthopanax senticosus enhances intestinal integrity through inhibiting TLR4/NF-κB signaling pathways in lipopolysaccharide-challenged mice. Anim. Sci. J. 87 (8), 1011–1018. doi:10.1111/asj.12528
Harris, C., and Cummings, J. (2021). JAK1 inhibition and inflammatory bowel disease. RHEUMATOLOGY 60 (2), ii45–ii51. doi:10.1093/rheumatology/keaa896
Hashimoto, C., Hudson, K. L., and Anderson, K. V. (1988). The Toll gene of Drosophila, required for dorsal-ventral embryonic polarity, appears to encode a transmembrane protein. Cell 52 (2), 269–279. doi:10.1016/0092-8674(88)90516-8
He, J., Li, B., Han, S., Zhang, Y., Liu, K., Yi, S., et al. (2022). Drosophila as a model to study the mechanism of nociception. Front. Physiol. 13, 854124. doi:10.3389/fphys.2022.854124
He, J., Li, X., Yang, S., Li, Y., Lin, X., Xiu, M., et al. (2021). Gastrodin extends the lifespan and protects against neurodegeneration in the Drosophila PINK1 model of Parkinson's disease. Food Funct. 12 (17), 7816–7824. doi:10.1039/d1fo00847a
He, X., Wei, Z., Wang, J., Kou, J., Liu, W., Fu, Y., et al. (2016). Alpinetin attenuates inflammatory responses by suppressing TLR4 and NLRP3 signaling pathways in DSS-induced acute colitis. Sci. Rep. 6, 28370. doi:10.1038/srep28370
Herbert, S. P., and Stainier, D. Y. (2011). Molecular control of endothelial cell behaviour during blood vessel morphogenesis. Nat. Rev. Mol. Cell Biol. 12 (9), 551–564. doi:10.1038/nrm3176
Hergovich, A., Stegert, M. R., Schmitz, D., and Hemmings, B. A. (2006). NDR kinases regulate essential cell processes from yeast to humans. Nat. Rev. Mol. Cell Biol. 7 (4), 253–264. doi:10.1038/nrm1891
Herrera, S. C., and Bach, E. A. (2019). JAK/STAT signaling in stem cells and regeneration: From Drosophila to vertebrates. Development 146 (2), dev167643. doi:10.1242/dev.167643
Hochmuth, C. E., Biteau, B., Bohmann, D., and Jasper, H. (2011). Redox regulation by Keap1 and Nrf2 controls intestinal stem cell proliferation in Drosophila. Cell Stem Cell 8 (2), 188–199. doi:10.1016/j.stem.2010.12.006
Hu, L. H., Liu, J. Y., and Yin, J. B. (2021). Eriodictyol attenuates TNBS-induced ulcerative colitis through repressing TLR4/NF-kB signaling pathway in rats. Kaohsiung J. Med. Sci. 37 (9), 812–818. doi:10.1002/kjm2.12400
Huang, J., Sheng, X., Zhuo, Z., Xiao, D., Wu, K., Wan, G., et al. (2022). ClC-c regulates the proliferation of intestinal stem cells via the EGFR signalling pathway in Drosophila. Cell Prolif. 55 (1), e13173. doi:10.1111/cpr.13173
Hwang, H., and Lu, H. (2013). Microfluidic tools for developmental studies of small model organisms--nematodes, fruit flies, and zebrafish. Biotechnol. J. 8 (2), 192–205. doi:10.1002/biot.201200129
Jiang, H., and Edgar, B. A. (2012). Intestinal stem cell function in Drosophila and mice. Curr. Opin. Genet. Dev. 22 (4), 354–360. doi:10.1016/j.gde.2012.04.002
Jiang, H., Grenley, M. O., Bravo, M. J., Blumhagen, R. Z., and Edgar, B. A. (2011). EGFR/Ras/MAPK signaling mediates adult midgut epithelial homeostasis and regeneration in Drosophila. Cell Stem Cell 8 (1), 84–95. doi:10.1016/j.stem.2010.11.026
Jiang, H., Patel, P. H., Kohlmaier, A., Grenley, M. O., Mcewen, D. G., and Edgar, B. A. (2009). Cytokine/Jak/Stat signaling mediates regeneration and homeostasis in the Drosophila midgut. Cell 137 (7), 1343–1355. doi:10.1016/j.cell.2009.05.014
Jiang, H., Tian, A., and Jiang, J. (2016). Intestinal stem cell response to injury: Lessons from Drosophila. Cell. Mol. Life Sci. 73 (17), 3337–3349. doi:10.1007/s00018-016-2235-9
Jo, A. R., Han, H. S., Seo, S., Shin, J. S., Lee, J. Y., Kim, H. J., et al. (2017). Inhibitory effect of moschamine isolated from Carthamus tinctorius on LPS-induced inflammatory mediators via AP-1 and STAT1/3 inactivation in RAW 264.7 macrophages. Bioorg. Med. Chem. Lett. 27 (23), 5245–5251. doi:10.1016/j.bmcl.2017.10.035
Kang, S., Kim, W., Jeong, S., Lee, Y., Nam, J., Lee, S., et al. (2017). Oxidized 5-aminosalicylic acid activates Nrf2-HO-1 pathway by covalently binding to Keap1: Implication in anti-inflammatory actions of 5-aminosalicylic acid. Free Radic. Biol. Med. 108, 715–724. doi:10.1016/j.freeradbiomed.2017.04.366
Kasembeli, M. M., Bharadwaj, U., Robinson, P., and Tweardy, D. J. (2018). Contribution of STAT3 to inflammatory and fibrotic diseases and prospects for its targeting for treatment. Int. J. Mol. Sci. 19 (8), 2299. doi:10.3390/ijms19082299
Khoramjoo, S. M., Kazemifard, N., Baradaran, G. S., Farmani, M., Shahrokh, S., Asadzadeh, A. H., et al. (2022). Overview of three proliferation pathways (Wnt, notch, and Hippo) in intestine and immune system and their role in inflammatory bowel diseases (IBDs). Front. Med. 9, 865131. doi:10.3389/fmed.2022.865131
Kitani-Morii, F., Friedland, R. P., Yoshida, H., and Mizuno, T. (2021). Drosophila as a model for microbiota studies of neurodegeneration. J. Alzheimers Dis. 84 (2), 479–490. doi:10.3233/JAD-215031
Kuhnert, F., Davis, C. R., Wang, H. T., Chu, P., Lee, M., Yuan, J., et al. (2004). Essential requirement for Wnt signaling in proliferation of adult small intestine and colon revealed by adenoviral expression of Dickkopf-1. Proc. Natl. Acad. Sci. U. S. A. 101 (1), 266–271. doi:10.1073/pnas.2536800100
Kumar, R., Juillerat-Jeanneret, L., and Golshayan, D. (2016). Notch antagonists: Potential modulators of cancer and inflammatory diseases. J. Med. Chem. 59 (17), 7719–7737. doi:10.1021/acs.jmedchem.5b01516
Kux, K., and Pitsouli, C. (2014). Tissue communication in regenerative inflammatory signaling: Lessons from the fly gut. Front. Cell. Infect. Microbiol. 4, 49. doi:10.3389/fcimb.2014.00049
Lamiable, O., Meignin, C., and Imler, J. L. (2016). WntD and Diedel: Two immunomodulatory cytokines in Drosophila immunity. Fly 10 (4), 187–194. doi:10.1080/19336934.2016.1202387
Lee, S. H., Goo, T. W., and Yun, E. Y. (2021). Allomyrina dichotoma larval extract has protective effects against gut permeability of dextran sulfate sodium-fed Drosophila by E-cadherin and armadillo. J. Ethnopharmacol. 279, 113786. doi:10.1016/j.jep.2021.113786
Lei, X., Zhou, Z., Wang, S., and Jin, L. H. (2022). The protective effect of safranal against intestinal tissue damage in Drosophila. Toxicol. Appl. Pharmacol. 439, 115939. doi:10.1016/j.taap.2022.115939
Lemaitre, B., and Hoffmann, J. (2007). The host defense of Drosophila melanogaster. Annu. Rev. Immunol. 25, 697–743. doi:10.1146/annurev.immunol.25.022106.141615
Lemaitre, B., and Miguel-Aliaga, I. (2013). The digestive tract of Drosophila melanogaster. Annu. Rev. Genet. 47, 377–404. doi:10.1146/annurev-genet-111212-133343
Lertnimitphun, P., Jiang, Y., Kim, N., Fu, W., Zheng, C., Tan, H., et al. (2019). Safranal alleviates dextran sulfate sodium-induced colitis and suppresses macrophage-mediated inflammation. Front. Pharmacol. 10, 1281. doi:10.3389/fphar.2019.01281
Li, S., Takasu, C., Lau, H., Robles, L., Vo, K., Farzaneh, T., et al. (2020). Dimethyl fumarate alleviates dextran sulfate sodium-induced colitis, through the activation of nrf2-mediated antioxidant and anti-inflammatory pathways. Antioxidants 9 (4), 354. doi:10.3390/antiox9040354
Li, X., Wei, Z., Wang, X., Duan, F., Xiong, L., Li, J., et al. (2021). Premna microphylla Turcz leaf pectin exhibited antioxidant and anti-inflammatory activities in LPS-stimulated RAW 264.7 macrophages. Food Chem. 349, 129164. doi:10.1016/j.foodchem.2021.129164
Li, Y., Shen, L., and Luo, H. (2016). Luteolin ameliorates dextran sulfate sodium-induced colitis in mice possibly through activation of the Nrf2 signaling pathway. Int. Immunopharmacol. 40, 24–31. doi:10.1016/j.intimp.2016.08.020
Lim, J. S., Lee, S. H., Lee, S. R., Lim, H. J., Roh, Y. S., Won, E. J., et al. (2020). Inhibitory effects of aucklandia lappa decne. Extract on inflammatory and oxidative responses in LPS-treated macrophages. Molecules 25 (6), 1336. doi:10.3390/molecules25061336
Lin, J., and Hackam, D. J. (2011). Worms, flies and four-legged friends: The applicability of biological models to the understanding of intestinal inflammatory diseases. Dis. Model. Mech. 4 (4), 447–456. doi:10.1242/dmm.007252
Lin, X., Bai, D., Wei, Z., Zhang, Y., Huang, Y., Deng, H., et al. (2019). Curcumin attenuates oxidative stress in RAW264.7 cells by increasing the activity of antioxidant enzymes and activating the Nrf2-Keap1 pathway. PLoS One 14 (5), e0216711. doi:10.1371/journal.pone.0216711
Liu, B., Piao, X., Guo, L., Liu, S., Chai, F., and Gao, L. (2016a). Ursolic acid protects against ulcerative colitis via anti-inflammatory and antioxidant effects in mice. Mol. Med. Rep. 13 (6), 4779–4785. doi:10.3892/mmr.2016.5094
Liu, J., Xiao, Q., Xiao, J., Niu, C., Li, Y., Zhang, X., et al. (2022). Wnt/β-catenin signalling: Function, biological mechanisms, and therapeutic opportunities. Signal Transduct. Target. Ther. 7 (1), 3. doi:10.1038/s41392-021-00762-6
Liu, J., Yue, S., Yang, Z., Feng, W., Meng, X., Wang, A., et al. (2018). Oral hydroxysafflor yellow A reduces obesity in mice by modulating the gut microbiota and serum metabolism. Pharmacol. Res. 134, 40–50. doi:10.1016/j.phrs.2018.05.012
Liu, X., Hodgson, J. J., and Buchon, N. (2017). Drosophila as a model for homeostatic, antibacterial, and antiviral mechanisms in the gut. PLoS Pathog. 13 (5), e1006277. doi:10.1371/journal.ppat.1006277
Liu, Z., Chen, Y., Zhang, H., and Jin, L. H. (2016b). Crocus sativus L. protects against SDSinduced intestinal damage and extends lifespan in Drosophila melanogaster. Mol. Med. Rep. 14 (6), 5601–5606. doi:10.3892/mmr.2016.5944
Loboda, A., Damulewicz, M., Pyza, E., Jozkowicz, A., and Dulak, J. (2016). Role of Nrf2/HO-1 system in development, oxidative stress response and diseases: An evolutionarily conserved mechanism. Cell. Mol. Life Sci. 73 (17), 3221–3247. doi:10.1007/s00018-016-2223-0
Lu, Y., Li, X., Liu, S., Zhang, Y., and Zhang, D. (2018). Toll-like receptors and inflammatory bowel disease. Front. Immunol. 9, 72. doi:10.3389/fimmu.2018.00072
Lye, S. H., and Chtarbanova, S. (2018). Drosophila as a model to study brain innate immunity in health and disease. Int. J. Mol. Sci. 19 (12), 3922. doi:10.3390/ijms19123922
Ma, C., Wang, Y., Zhang, G., and Dai, X. (2021). Agar oligosaccharides ameliorate the intestinal inflammation of male Drosophila melanogaster via modulating the microbiota, and immune and cell autophagy. Food Sci. Nutr. 9 (2), 1202–1212. doi:10.1002/fsn3.2108
Ma, C., Yang, K., Wang, Y., and Dai, X. (2019). Anti-aging effect of agar oligosaccharide on male Drosophila melanogaster and its preliminary mechanism. Mar. Drugs. 17 (11), 632. doi:10.3390/md17110632
Madi, J. R., Outa, A. A., Ghannam, M., Hussein, H. M., Shehab, M., Hasan, Z., et al. (2021). Drosophila melanogaster as a model system to assess the effect of epstein-barr virus DNA on inflammatory gut diseases. Front. Immunol. 12, 586930. doi:10.3389/fimmu.2021.586930
Maitra, U., and Ciesla, L. (2019). Using Drosophila as a platform for drug discovery from natural products in Parkinson's disease. MedChemComm 10 (6), 867–879. doi:10.1039/c9md00099b
Maitra, U., Stephen, C., and Ciesla, L. M. (2022). Drug discovery from natural products - old problems and novel solutions for the treatment of neurodegenerative diseases. J. Pharm. Biomed. Anal. 210, 114553. doi:10.1016/j.jpba.2021.114553
Marin, M., Maria, G. R., Rios, J. L., and Recio, M. C. (2013). Intestinal anti-inflammatory activity of ellagic acid in the acute and chronic dextrane sulfate sodium models of mice colitis. J. Ethnopharmacol. 150 (3), 925–934. doi:10.1016/j.jep.2013.09.030
Mccammon, J. M., and Sive, H. (2015). Addressing the genetics of human mental health disorders in model organisms. Annu. Rev. Genomics Hum. Genet. 16, 173–197. doi:10.1146/annurev-genom-090314-050048
Mcguire, S. E., Mao, Z., and Davis, R. L. (2004). Spatiotemporal gene expression targeting with the TARGET and gene-switch systems in Drosophila. Sci. STKE 2004 (220), pl6. doi:10.1126/stke.2202004pl6
Medina, A., Bellec, K., Polcownuk, S., and Cordero, J. B. (2022). Investigating local and systemic intestinal signalling in health and disease with Drosophila. Dis. Model. Mech. 15 (3), dmm049332. doi:10.1242/dmm.049332
Medzhitov, R., Preston-Hurlburt, P., and Janeway, C. J. (1997). A human homologue of the Drosophila Toll protein signals activation of adaptive immunity. Nature 388 (6640), 394–397. doi:10.1038/41131
Micchelli, C. A., and Perrimon, N. (2006). Evidence that stem cells reside in the adult Drosophila midgut epithelium. Nature 439 (7075), 475–479. doi:10.1038/nature04371
Miguel-Aliaga, I., Jasper, H., and Lemaitre, B. (2018). Anatomy and physiology of the digestive tract of Drosophila melanogaster. Genetics 210 (2), 357–396. doi:10.1534/genetics.118.300224
Misra, J. R., Lam, G., and Thummel, C. S. (2013). Constitutive activation of the Nrf2/Keap1 pathway in insecticide-resistant strains of Drosophila. Insect biochem. Mol. Biol. 43 (12), 1116–1124. doi:10.1016/j.ibmb.2013.09.005
Moon, S. Y., Kim, K. D., Yoo, J., Lee, J. H., and Hwangbo, C. (2021). Phytochemicals targeting JAK-STAT pathways in inflammatory bowel disease: Insights from animal models. Molecules 26 (9), 2824. doi:10.3390/molecules26092824
Mou, Y., Wen, S., Li, Y. X., Gao, X. X., Zhang, X., and Jiang, Z. Y. (2020). Recent progress in Keap1-Nrf2 protein-protein interaction inhibitors. Eur. J. Med. Chem. 202, 112532. doi:10.1016/j.ejmech.2020.112532
Myllymaki, H., and Ramet, M. (2014). JAK/STAT pathway in Drosophila immunity. Scand. J. Immunol. 79 (6), 377–385. doi:10.1111/sji.12170
Nakazawa, N., Taniguchi, K., Okumura, T., Maeda, R., and Matsuno, K. (2012). A novel Cre/loxP system for mosaic gene expression in the Drosophila embryo. Dev. Dyn. 241 (5), 965–974. doi:10.1002/dvdy.23784
Neurath, M. F. (2017). Current and emerging therapeutic targets for IBD. Nat. Rev. Gastroenterol. Hepatol. 14 (5), 269–278. doi:10.1038/nrgastro.2016.208
Nusse, R., and Clevers, H. (2017). Wnt/β-Catenin signaling, disease, and emerging therapeutic modalities. Cell 169 (6), 985–999. doi:10.1016/j.cell.2017.05.016
O'Shea, J. J., Holland, S. M., and Staudt, L. M. (2013). JAKs and STATs in immunity, immunodeficiency, and cancer. N. Engl. J. Med. 368 (2), 161–170. doi:10.1056/NEJMra1202117
Ohlstein, B., and Spradling, A. (2007). Multipotent Drosophila intestinal stem cells specify daughter cell fates by differential notch signaling. Science 315 (5814), 988–992. doi:10.1126/science.1136606
Okamoto, R., Tsuchiya, K., Nemoto, Y., Akiyama, J., Nakamura, T., Kanai, T., et al. (2009). Requirement of Notch activation during regeneration of the intestinal epithelia. Am. J. Physiol. Gastrointest. Liver Physiol. 296 (1), G23–G35. doi:10.1152/ajpgi.90225.2008
Pagliarini, R. A., and Xu, T. (2003). A genetic screen in Drosophila for metastatic behavior. Science 302 (5648), 1227–1231. doi:10.1126/science.1088474
Peng, K. Y., Gu, J. F., Su, S. L., Zhu, Y., Guo, J. M., Qian, D. W., et al. (2021). Salvia miltiorrhiza stems and leaves total phenolic acids combination with tanshinone protect against DSS-induced ulcerative colitis through inhibiting TLR4/PI3K/AKT/mTOR signaling pathway in mice. J. Ethnopharmacol. 264, 113052. doi:10.1016/j.jep.2020.113052
Pereira, S. R., Pereira, R., Figueiredo, I., Freitas, V., Dinis, T. C., and Almeida, L. M. (2017). Comparison of anti-inflammatory activities of an anthocyanin-rich fraction from Portuguese blueberries (Vaccinium corymbosum L.) and 5-aminosalicylic acid in a TNBS-induced colitis rat model. PLoS One 12 (3), e0174116. doi:10.1371/journal.pone.0174116
Perochon, J., Carroll, L. R., and Cordero, J. B. (2018). Wnt signalling in intestinal stem cells: Lessons from mice and flies. Genes 9 (3), 138. doi:10.3390/genes9030138
Piberger, H., Oehme, A., Hofmann, C., Dreiseitel, A., Sand, P. G., Obermeier, F., et al. (2011). Bilberries and their anthocyanins ameliorate experimental colitis. Mol. Nutr. Food Res. 55 (11), 1724–1729. doi:10.1002/mnfr.201100380
Piotrowska, M., Swierczynski, M., Fichna, J., and Piechota-Polanczyk, A. (2021). The Nrf2 in the pathophysiology of the intestine: Molecular mechanisms and therapeutic implications for inflammatory bowel diseases. Pharmacol. Res. 163, 105243. doi:10.1016/j.phrs.2020.105243
Pomatto, L., Wong, S., Carney, C., Shen, B., Tower, J., and Davies, K. (2017). The age- and sex-specific decline of the 20s proteasome and the Nrf2/CncC signal transduction pathway in adaption and resistance to oxidative stress in Drosophila melanogaster. Aging (Albany NY) 9 (4), 1153–1185. doi:10.18632/aging.101218
Pu, Z., Yang, F., Wang, L., Diao, Y., and Chen, D. (2021). Advancements of compounds targeting Wnt and Notch signalling pathways in the treatment of inflammatory bowel disease and colon cancer. J. Drug Target. 29 (5), 507–519. doi:10.1080/1061186X.2020.1864741
Raghunath, A., Sundarraj, K., Nagarajan, R., Arfuso, F., Bian, J., Kumar, A. P., et al. (2018). Antioxidant response elements: Discovery, classes, regulation and potential applications. Redox Biol. 17, 297–314. doi:10.1016/j.redox.2018.05.002
Ren, F., Wang, B., Yue, T., Yun, E. Y., Ip, Y. T., and Jiang, J. (2010). Hippo signaling regulates Drosophila intestine stem cell proliferation through multiple pathways. Proc. Natl. Acad. Sci. U. S. A. 107 (49), 21064–21069. doi:10.1073/pnas.1012759107
Salas, A., Hernandez-Rocha, C., Duijvestein, M., Faubion, W., Mcgovern, D., Vermeire, S., et al. (2020). JAK-STAT pathway targeting for the treatment of inflammatory bowel disease. Nat. Rev. Gastroenterol. Hepatol. 17 (6), 323–337. doi:10.1038/s41575-020-0273-0
Sekhar, K. R., Rachakonda, G., and Freeman, M. L. (2010). Cysteine-based regulation of the CUL3 adaptor protein Keap1. Toxicol. Appl. Pharmacol. 244 (1), 21–26. doi:10.1016/j.taap.2009.06.016
Senturk, M., and Bellen, H. J. (2018). Genetic strategies to tackle neurological diseases in fruit flies. Curr. Opin. Neurobiol. 50, 24–32. doi:10.1016/j.conb.2017.10.017
Shahzad, U., Taccone, M. S., Kumar, S. A., Okura, H., Krumholtz, S., Ishida, J., et al. (2021). Modeling human brain tumors in flies, worms, and zebrafish: From proof of principle to novel therapeutic targets. Neuro. Oncol. 23 (5), 718–731. doi:10.1093/neuonc/noaa306
Shao, M. J., Yan, Y. X., Qi, Q., Tang, W., and Zuo, J. P. (2019). Application of active components from traditional Chinese medicine in treatment of inflammatory bowel disease. Zhongguo Zhong Yao Za Zhi 44 (3), 415–421. doi:10.19540/j.cnki.cjcmm.20180907.001
Sheng, Q., Li, F., Chen, G., Li, J., Li, J., Wang, Y., et al. (2021a). Ursolic acid regulates intestinal microbiota and inflammatory cell infiltration to prevent ulcerative colitis. J. Immunol. Res. 2021, 6679316. doi:10.1155/2021/6679316
Sheng, X., Zhu, Y., Zhou, J., Yan, Du G., Liu, Z., Chen, H., et al. (2021b). Antioxidant effects of caffeic acid lead to protection of Drosophila intestinal stem cell aging. Front. Cell Dev. Biol. 9, 735483. doi:10.3389/fcell.2021.735483
Shinoda, M., Shin-Ya, M., Naito, Y., Kishida, T., Ito, R., Suzuki, N., et al. (2010). Early-stage blocking of Notch signaling inhibits the depletion of goblet cells in dextran sodium sulfate-induced colitis in mice. J. Gastroenterol. 45 (6), 608–617. doi:10.1007/s00535-010-0210-z
Sneddon, L. U. (2018). Comparative physiology of nociception and pain. Physiology 33 (1), 63–73. doi:10.1152/physiol.00022.2017
Song, G., Chen, F., Chen, S., and Ye, S. (2021). Polysaccharides from Premna microphylla turcz ameliorate inflammation via the enhancement of intestinal resistance in host. J. Ethnopharmacol. 276, 114208. doi:10.1016/j.jep.2021.114208
Strand, M., and Micchelli, C. A. (2013). Regional control of Drosophila gut stem cell proliferation: EGF establishes GSSC proliferative set point & controls emergence from quiescence. PLoS One 8 (11), e80608. doi:10.1371/journal.pone.0080608
Stubbs, J. D., Lekutis, C., Singer, K. L., Bui, A., Yuzuki, D., Srinivasan, U., et al. (1990). cDNA cloning of a mouse mammary epithelial cell surface protein reveals the existence of epidermal growth factor-like domains linked to factor VIII-like sequences. Proc. Natl. Acad. Sci. U. S. A. 87 (21), 8417–8421. doi:10.1073/pnas.87.21.8417
Su, T. T. (2019). Drug screening in Drosophila; why, when, and when not? Wiley Interdiscip. Rev. Dev. Biol. 8 (6), e346. doi:10.1002/wdev.346
Sykiotis, G. P., and Bohmann, D. (2008). Keap1/Nrf2 signaling regulates oxidative stress tolerance and lifespan in Drosophila. Dev. Cell. 14 (1), 76–85. doi:10.1016/j.devcel.2007.12.002
Takano-Shimizu-Kouno, T., and Ohsako, T. (2018). Humanized flies and resources for cross-species study. Adv. Exp. Med. Biol. 1076, 277–288. doi:10.1007/978-981-13-0529-0_15
Tauszig-Delamasure, S., Bilak, H., Capovilla, M., Hoffmann, J. A., and Imler, J. L. (2002). Drosophila MyD88 is required for the response to fungal and Gram-positive bacterial infections. Nat. Immunol. 3 (1), 91–97. doi:10.1038/ni747
Theodosiou, N. A., and Xu, T. (1998). Use of FLP/FRT system to study Drosophila development. Methods 14 (4), 355–365. doi:10.1006/meth.1998.0591
Tian, A., Benchabane, H., and Ahmed, Y. (2018). Wingless/wnt signaling in intestinal development, homeostasis, regeneration and tumorigenesis: A Drosophila perspective. J. Dev. Biol. 6 (2), 8. doi:10.3390/jdb6020008
Toiyama, Y., Araki, T., Yoshiyama, S., Hiro, J., Miki, C., and Kusunoki, M. (2006). The expression patterns of Toll-like receptors in the ileal pouch mucosa of postoperative ulcerative colitis patients. Surg. Today. 36 (3), 287–290. doi:10.1007/s00595-005-3144-y
Wan, F., Zhong, R., Wang, M., Zhou, Y., Chen, Y., Yi, B., et al. (2021). Caffeic acid supplement alleviates colonic inflammation and oxidative stress potentially through improved gut microbiota community in mice. Front. Microbiol. 12, 784211. doi:10.3389/fmicb.2021.784211
Wang, L., Hu, Y., Song, B., Xiong, Y., Wang, J., and Chen, D. (2021a). Targeting JAK/STAT signaling pathways in treatment of inflammatory bowel disease. Inflamm. Res. 70 (7), 753–764. doi:10.1007/s00011-021-01482-x
Wang, Y., Tao, H., Huang, H., Xiao, Y., Wu, X., Li, M., et al. (2021b). The dietary supplement Rhodiola crenulata extract alleviates dextran sulfate sodium-induced colitis in mice through anti-inflammation, mediating gut barrier integrity and reshaping the gut microbiome. Food Funct. 12 (7), 3142–3158. doi:10.1039/d0fo03061a
Weasner, B. M., Zhu, J., and Kumar, J. P. (2017). FLPing genes on and off in Drosophila. Methods Mol. Biol. 1642, 195–209. doi:10.1007/978-1-4939-7169-5_13
Wehkamp, J., Wang, G., Kubler, I., Nuding, S., Gregorieff, A., Schnabel, A., et al. (2007). The Paneth cell alpha-defensin deficiency of ileal Crohn's disease is linked to Wnt/Tcf-4. J. Immunol. 179 (5), 3109–3118. doi:10.4049/jimmunol.179.5.3109
Wei, T., Wu, L., Ji, X., Gao, Y., and Xiao, G. (2022). Ursolic acid protects sodium dodecyl sulfate-induced Drosophila ulcerative colitis model by inhibiting the JNK signaling. Antioxidants 11 (2), 426. doi:10.3390/antiox11020426
Willoughby, L. F., Schlosser, T., Manning, S. A., Parisot, J. P., Street, I. P., Richardson, H. E., et al. (2013). An in vivo large-scale chemical screening platform using Drosophila for anti-cancer drug discovery. Dis. Model. Mech. 6 (2), 521–529. doi:10.1242/dmm.009985
Wu, H., Ye, L., Lu, X., Xie, S., Yang, Q., and Yu, Q. (2018). Lactobacillus acidophilus alleviated salmonella-induced goblet cells loss and colitis by notch pathway. Mol. Nutr. Food Res. 62 (22), e1800552. doi:10.1002/mnfr.201800552
Xiang, C., Liu, M., Lu, Q., Fan, C., Lu, H., Feng, C., et al. (2021). Blockade of TLRs-triggered macrophage activation by caffeic acid exerted protective effects on experimental ulcerative colitis. Cell. Immunol. 365, 104364. doi:10.1016/j.cellimm.2021.104364
Xie, T., Ho, M., Liu, Q., Horiuchi, W., Lin, C. C., Task, D., et al. (2018). A genetic toolkit for dissecting dopamine circuit function in Drosophila. Cell Rep. 23 (2), 652–665. doi:10.1016/j.celrep.2018.03.068
Xin, P., Xu, X., Deng, C., Liu, S., Wang, Y., Zhou, X., et al. (2020). The role of JAK/STAT signaling pathway and its inhibitors in diseases. Int. Immunopharmacol. 80, 106210. doi:10.1016/j.intimp.2020.106210
Xu, N., Wang, S. Q., Tan, D., Gao, Y., Lin, G., and Xi, R. (2011). EGFR, Wingless and JAK/STAT signaling cooperatively maintain Drosophila intestinal stem cells. Dev. Biol. 354 (1), 31–43. doi:10.1016/j.ydbio.2011.03.018
Yang, J. Y., Zhong, X., Yum, H. W., Lee, H. J., Kundu, J. K., Na, H. K., et al. (2013). Curcumin inhibits STAT3 signaling in the colon of dextran sulfate sodium-treated mice. J. Cancer Prev. 18 (2), 186–191. doi:10.15430/jcp.2013.18.2.186
Yang, S., Li, X., Xiu, M., Dai, Y., Wan, S., Shi, Y., et al. (2022). Flos puerariae ameliorates the intestinal inflammation of Drosophila via modulating the Nrf2/Keap1, JAK-STAT and Wnt signaling. Front. Pharmacol. 13, 893758. doi:10.3389/fphar.2022.893758
Yasueda, A., Kayama, H., Murohashi, M., Nishimura, J., Wakame, K., Komatsu, K. I., et al. (2020). Sanguisorba officinalis L. derived from herbal medicine prevents intestinal inflammation by inducing autophagy in macrophages. Sci. Rep. 10 (1), 9972. doi:10.1038/s41598-020-65306-4
Yu, C., Wang, D., Yang, Z., and Wang, T. (2022). Pharmacological effects of polyphenol phytochemicals on the intestinal inflammation via targeting TLR4/NF-κB signaling pathway. Int. J. Mol. Sci. 23 (13), 6939. doi:10.3390/ijms23136939
Zhang, G., Gu, Y., and Dai, X. (2022). Protective effect of bilberry anthocyanin extracts on dextran sulfate sodium-induced intestinal damage in Drosophila melanogaster. Nutrients 14 (14), 2875. doi:10.3390/nu14142875
Zhang, H., Wang, S., and Jin, L. H. (2020). Acanthopanax senticosus polysaccharide regulates the intestinal homeostasis disruption induced by toxic chemicals in Drosophila. Phytother. Res. 34 (1), 193–200. doi:10.1002/ptr.6522
Zhang, W., Michalowski, C. B., and Beloqui, A. (2021). Oral delivery of biologics in inflammatory bowel disease treatment. Front. Bioeng. Biotechnol. 9, 675194. doi:10.3389/fbioe.2021.675194
Zheng, X., Tsuchiya, K., Okamoto, R., Iwasaki, M., Kano, Y., Sakamoto, N., et al. (2011). Suppression of hath1 gene expression directly regulated by hes1 via notch signaling is associated with goblet cell depletion in ulcerative colitis. Inflamm. Bowel Dis. 17 (11), 2251–2260. doi:10.1002/ibd.21611
Zhou, Y., Liu, Z., Chen, Y., and Jin, L. H. (2016). Identification of the protective effects of traditional medicinal plants against SDS-induced Drosophila gut damage. Exp. Ther. Med. 12 (4), 2671–2680. doi:10.3892/etm.2016.3641
Zhu, C., Guan, F., Wang, C., and Jin, L. H. (2014). The protective effects of Rhodiola crenulata extracts on Drosophila melanogaster gut immunity induced by bacteria and SDS toxicity. Phytother. Res. 28 (12), 1861–1866. doi:10.1002/ptr.5215
Zielinska, D., Zielinski, H., Laparra-Llopis, J. M., Szawara-Nowak, D., Honke, J., and Gimenez-Bastida, J. A. (2021). Caffeic acid modulates processes associated with intestinal inflammation. Nutrients 13 (2), 554. doi:10.3390/nu13020554
Zilbauer, M. (2022). Epigenetics in IBD: A conceptual framework for disease pathogenesis. Frontline Gastroenterol. 13 (1), e22–e27. doi:10.1136/flgastro-2022-102120
Keywords: inflammatory bowel disease, Drosophila melanogaster, natural products, drug discovery, molecular pathways
Citation: Xiu M, Wang Y, Yang D, Zhang X, Dai Y, Liu Y, Lin X, Li B and He J (2022) Using Drosophila melanogaster as a suitable platform for drug discovery from natural products in inflammatory bowel disease. Front. Pharmacol. 13:1072715. doi: 10.3389/fphar.2022.1072715
Received: 17 October 2022; Accepted: 24 November 2022;
Published: 05 December 2022.
Edited by:
Andresa Heemann Betti, Feevale University, BrazilReviewed by:
Jay V. Patankar, University of Erlangen Nuremberg, GermanySaeideh Momtaz, Academic Center for Education, Culture and Research, Iran
Copyright © 2022 Xiu, Wang, Yang, Zhang, Dai, Liu, Lin, Li and He. This is an open-access article distributed under the terms of the Creative Commons Attribution License (CC BY). The use, distribution or reproduction in other forums is permitted, provided the original author(s) and the copyright owner(s) are credited and that the original publication in this journal is cited, in accordance with accepted academic practice. No use, distribution or reproduction is permitted which does not comply with these terms.
*Correspondence: Jianzheng He, aGVqaWFuemhlbmcxMDA2QDE2My5jb20=
†These authors have contributed equally to this work