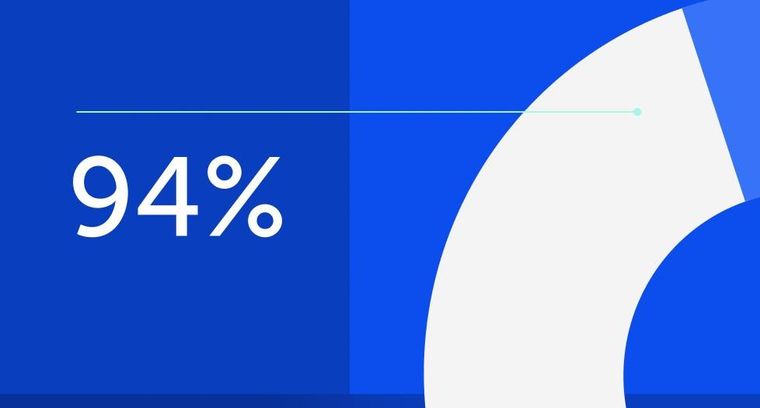
94% of researchers rate our articles as excellent or good
Learn more about the work of our research integrity team to safeguard the quality of each article we publish.
Find out more
MINI REVIEW article
Front. Pharmacol., 24 November 2022
Sec. Pharmacology of Anti-Cancer Drugs
Volume 13 - 2022 | https://doi.org/10.3389/fphar.2022.1062495
A correction has been applied to this article in:
Corrigendum: R-carvedilol, a potential new therapy for Alzheimer’s disease
For decades, the amyloid cascade hypothesis has been the leading hypothesis in studying Alzheimer’s disease (AD) pathology and drug development. However, a growing body of evidence indicates that simply removing amyloid plaques may not significantly affect AD progression. Alternatively, it has been proposed that AD progression is driven by increased neuronal excitability. Consistent with this alternative hypothesis, recent studies showed that pharmacologically limiting ryanodine receptor 2 (RyR2) open time with the R-carvedilol enantiomer prevented and reversed neuronal hyperactivity, memory impairment, and neuron loss in AD mouse models without affecting the accumulation of ß-amyloid (Aβ). These data indicate that R-carvedilol could be a potential new therapy for AD.
As the most common form of dementia, Alzheimer’s disease (AD) is afflicting an increasing number of people around the world. (Rocca et al., 1990; Hynd et al., 2004). In addition to plaguing its patients, AD has also become a heavy burden on patients’ families and society (Prigerson, 2003; Castro et al., 2010). Over the past three decades, scientists across the world have made immense efforts to comprehend the pathogenesis of AD and develop effective AD treatments (Corbett et al., 2012; Elmaleh et al., 2019; Clement et al., 2020). The leading theory of AD pathogenesis is the amyloid cascade hypothesis. Based on this hypothesis, most efforts have been devoted to the development of drugs targeting ß-amyloid (Aβ) (Demattos et al., 2012; Kennedy et al., 2016; Sevigny et al., 2016). Unfortunately, most Aβ-targeted AD clinical trials have failed to yield convincing results (Mehta et al., 2017; Yiannopoulou et al., 2019; Knopman et al., 2021). These disappointing outcomes indicate that our understanding of AD pathogenesis is far from complete, and we urgently need new strategies for AD treatment.
Given the multifactorial nature of AD pathogenesis, multiple pathways besides Aβ metabolism could be targeted for AD treatment. Indeed, recent studies have revealed many new targets and strategies for treating AD. For example, a molecule named leuco-methylthioninium bis(hydromethanesulphonate) (LMTM) that is thought to block the aggregation of tau has been shown to possess some promising anti-AD properties in a phase III clinical trial (Wilcock et al., 2018). Furthermore, recent studies suggested the ε allele of apolipoprotein E (APOE) is another genetic risk factor of AD. By injecting the anti-human APOE antibody into the APOEε4+/+ mouse model, Xiong et al. (2021), showed that this APOE immunotherapy reduced cerebral Aβ plaques and amyloid angiopathy. Besides focusing on certain molecular targets, more and more studies showed that neuronal circuitries could also be a promising target for treating AD. The gamma oscillations, which are important for the storage and maintenance of memory, were impaired in both AD patients and mouse models (Adaikkan and Tsai, 2020; Andrade-Talavera et al., 2020). Andrade-Talavera and colleagues found that small molecule compounds or the molecular chaperone Bri2 BRICHOS reversed the impaired gamma oscillations related to AD (Andrade-Talavera et al., 2020; Andrade-Talavera et al., 2022). In addition, multiple studies form Dr. Li-Hui Tsai’s group have shown that the gamma entrainment using sensory stimuli (GENUS) could reduce the Aβ level, improve learning and memory in multiple AD mouse models (Iaccarino et al., 2016; Adaikkan et al., 2019; Martorell et al., 2019).
In addition to these new trends in AD treatment, substantial evidence indicates that soluble Aβ induces neuronal hyperactivity, which in turn generates more soluble Aβ. These two components form a vicious cycle (Kamenetz et al., 2003; Cirrito et al., 2005; Busche et al., 2012; Yamamoto et al., 2015; Keskin et al., 2017; Zott et al., 2019). This Aβ-hyperactivity cycle is thought to promote Aβ accumulation, increase neuronal excitability, induce circuit dysfunction and exacerbate AD progression (Busche and Konnerth, 2015; Stargardt et al., 2015; Busche and Konnerth, 2016; Zott et al., 2019). Given the failure of many Aβ-targeted AD clinical trials, it seems that simply reducing Aβ expression or removing Aβ plaques is not sufficient to break this vicious cycle. Another way to break this loop is to overcome the neuronal hyperactivity.
Carvedilol is a nonselective ß-adrenergic receptor blocker, widely used for the treatment of congestive heart failure (Feuerstein and Ruffolo, 1995; Frishman, 1998; Dulin and Abraham, 2004). A previous study suggested that ß-blockers such as carvedilol may have anti-inflammatory effects on the AD brain, while it may also improve cognitive function in AD patients by improving cerebral perfusion (Rosenberg et al., 2008). However, since its potent ß-blocking activity that can cause bradycardia and hypotension, the benefits of carvedilol have been shown to be dose-limited (Bristow et al., 1996; Zhang et al., 2015). The clinically used carvedilol is a racemic mixture of ß-blocking S-carvedilol and non-β-blocking R-carvedilol (Bristow et al., 1996; Frishman, 1998; Zhou et al., 2011; Zhang et al., 2015). Recently, it has been shown that R-carvedilol alone prevented and rescued neuronal hyperactivity, memory impairment, and neuron loss without affecting the accumulation of ß-amyloid (Aβ) in mouse models of familial AD (FAD) in vivo and in vitro (Yao et al., 2020; Liu et al., 2021; Sun et al., 2021). This mini-review will discuss the potential application of R-Carvedilol as a new therapeutic strategy to treat AD.
Carvedilol is currently used in the form of racemic mixture, containing an equal amount of R-carvedilol (an α-blocker) and S-carvedilol (an α- and β-blocker). In vitro studies have shown that S-carvedilol has approximately 100-fold greater affinity for adrenergic ß-receptors than R-carvedilol. Meanwhile, R-carvedilol and S-carvedilol inhibit adrenergic α-receptors to the same extent (Nichols et al., 1989; Bartsch et al., 1990). Consistent with these studies, a randomized, double-blind, placebo-controlled study performed by Stoschitzky et al. (2001), indicated that only S-carvedilol causes ß-blockade. These findings suggest that the strong ß-blockade caused by S-carvedilol may underlie carvedilol’s adverse effects of bradycardia and hypotension.
Besides blocking the adrenergic receptors, carvedilol has been shown to effectively suppress spontaneous calcium release, also known as store-overload-induced calcium release (SOICR). Single channel recordings revealed that carvedilol can directly shorten the open time of the ryanodine receptor 2 (RyR2) Ca2+ release channel (Zhou et al., 2011). Like the racemic carvedilol, the non-β-blocking R-carvedilol can also directly reduce the open duration of RyR2 and suppress stress-induced ventricular tachyarrhythmia (VT) in mice harboring a RyR2 mutation (RyR2-R4496C+/-) associated with catecholaminergic polymorphic ventricular tachycardia (CPVT). Importantly, RyR2-R4496C+/- mice that received R-carvedilol treatment did not show a significant change in heart rate or blood pressure (Zhou et al., 2011; Zhang et al., 2015).
In addition to its benefits in the heart, carvedilol has also been shown to have neuroprotection property. Studies with cultured neurons suggest that carvedilol protects neuron from cell death induced by cerebral ischemia or stroke (Lysko et al., 1992; Yamagata et al., 2004). Using rat brain homogenate, Yue et al., have shown that compared to other commonly used ß-blockers, carvedilol is a far more potent antioxidant. The antioxidant effect of carvedilol mainly resides in the carbazole moiety, and the substitution of a hydroxyl group at certain positions on the phenyl ring of either carbazole or the ortho-substituted phenoxylethylamine part of carvedilol resulted in an increase in antioxidant activity (Yue et al., 1992). Thus, carvedilol’s actions on scavenging free radicals and inhibiting lipid peroxidation are believed to be the mechanism underlying neuroprotection. More importantly, Wang et al. (2011), reported that chronic oral administration of carvedilol in 2 independent AD mouse models, TgCRND8 transgenic mice and Tg2576 AD transgenic mice, remarkably reduced the expression of oligomeric Aβ and reversed cognitive decline (Arrieta-Cruz et al., 2010). Further studies suggested that carvedilol has the potential to bind to Aβ, thus preventing Aβ aggregation and formation of Aβ oligomeric fibrils (Wang et al., 2011). Therefore, in addition to treating congestive heart failure, carvedilol may potentially be used for treating AD or other neurological diseases. Interestingly, RyR2, a target of carvedilol, is abundantly expressed in both the heart and the brain (Furuichi et al., 1994; Giannini et al., 1995; Murayama and Ogawa, 1996; Bers, 2002). In particular, RyR2 is abundantly expressed in the hippocampus and cortex, which are the regions most vulnerable to damage caused by AD (Yao et al., 2020; Hiess et al., 2022). Increasing evidence suggests that the expression and function of RyR2 are upregulated in animal models of familial AD (FAD) and in human AD patients (Kelliher et al., 1999; Smith et al., 2005; Bruno et al., 2012; Oules et al., 2012; Chakroborty and Stutzmann, 2014; Lacampagne et al., 2017; SanMartin et al., 2017; Stutzmann, 2021). Since R-carvedilol can significantly reduce the open duration of RyR2 and does not have the ß-blocking effect, it represents a promising treatment for AD without the adverse effects often associated with racemic carvedilol, such as bradycardia and hypotension.
Increasing evidence has shown that RyR2-mediated calcium release can regulate membrane excitability of various cells, such as cardiomyocytes, smooth muscles, and neurons (Nelson et al., 1995; Alkon et al., 1998; Bogdanov et al., 2001; Mandikian et al., 2014). Besides causing problems in the heart, enhanced RyR2 function is also involved in AD pathogenesis (Kelliher et al., 1999; Smith et al., 2005; Bruno et al., 2012; Oules et al., 2012; Chakroborty and Stutzmann, 2014; Lacampagne et al., 2017; SanMartin et al., 2017). Consistent with these findings, two-photon calcium imaging of mouse hippocampal slices revealed significantly greater caffeine-induced calcium release in CA1 pyramidal neurons of 5xFAD+/- mouse compared with age-matched wild-type (WT) littermates. Notably, compared to DMSO-treated control group, pre-treatment with R-carvedilol for one-month, markedly reduced caffeine-induced calcium release in CA1 pyramidal neurons from acute 5xFAD+/- mice brain slices (Yao et al., 2020). Therefore, R-carvedilol is able to prevent AD-induced abnormal activation of RyR2-mediated calcium release.
Previous studies with different AD mouse models have shown that hyperactivity of hippocampal CA1 pyramidal neurons is associated with AD pathogenesis (Brown et al., 2011; Kerrigan et al., 2014; Siskova et al., 2014; Scala et al., 2015). By performing in vivo two-photon imaging in mice expressing the Thy-1 promoter-driven GCaMP6f calcium sensor in glutamatergic neurons driven (Chen et al., 2012), Yao et al. (2020), detected increased neuronal activity in glutamatergic pyramidal neurons in the hippocampal CA1 region in anesthetized 5xFAD+/- mice. Consistent with these previous observations (Busche et al., 2008; Busche et al., 2012; Busche and Konnerth, 2015, Busche and Konnerth, 2016; Zott et al., 2019), CA1 glutamatergic pyramidal neurons of 5xFAD+/- mice exhibited a significantly increased proportion of hyperactive neurons and the mean frequency of spontaneous calcium transients, and also a considerably decreased proportion of normal neurons, compared with WT littermates, (Yao et al., 2020). Interestingly, recent studies with mouse brain slices treated with recombinant Aβ1–42 or with brain slices from the novel AppNL−G-F mouse model showed that the gamma oscillation was significantly impaired (Balleza-Tapia et al., 2018; Andrade-Talavera et al., 2021; Arroyo-Garcia et al., 2021). Taken these studies together, it is possible that under the AD condition, the firing rates of excitatory neurons and inhibitory interneurons were altered differently. In other words, the excitatory/inhibitory balance was disrupted. Also, the change of the excitatory/inhibitory balance is dynamic, which means during the development of AD, the overall brain activity could be hyperactive at early stages and hypoactive at late stages (Targa Dias Anastacio et al., 2022).
Yao et al. (2020), also tested whether R-carvedilol treatment can prevent or reverse the hyperactivity of CA1 neurons in the 5xFAD+/- mice. 5xFAD+/- mice 2–3 months old (i.e., before the onset of AD symptoms) and 3–4 months old (i.e., after the onset of AD symptoms) (Oakley et al., 2006) were pre-treated with R-carvedilol or DMSO for one month. Compared to the DMSO pre-treated mice at both ages, the CA1 pyramidal neurons in 5xFAD+/- mice receiving R-carvedilol treatment showed a significant decrease in the proportion of hyperactive neurons with an apparent reduction of the mean frequency of spontaneous calcium transients. These observations suggested that R-carvedilol pre-treatment not only prevented but also reversed neuronal hyperactivity of 5xFAD+/- hippocampal CA1 neurons in vivo (Yao et al., 2020). Similar results were also found from ex vivo recordings with 5xFAD+/- hippocampal slices (Sun et al., 2021). Thus, both in vivo and ex vivo recordings showed that R-carvedilol treatment before the onset of AD pathologies can prevent neuronal hyperactivity, while R-carvedilol treatment after the onset of AD pathologies can rescue neuronal hyperactivity in 5xFAD CA1 neurons.
As a disease characterized primarily by cognitive impairment, a fundamental readout for any intervention is the measure of learning and memory. To test whether R-carvedilol treatment can protect animals from AD-related learning and memory deficits, Yao et al., performed the Morris water maze (MWM) test and novel object recognition (NOR) test, together with measurement of hippocampal CA3-CA1 long-term potentiation (LTP). Similar to the results of calcium imaging, pretreatment with R-carvedilol in 2–3 months old 5xFAD+/- mice (before AD symptoms) prevented memory loss and reversed LTP deficit, while in 3–4 months old 5xFAD+/- mice (after AD symptoms), pretreatment with R-carvedilol also reversed these deficits. The drug was also tested on 6–7 and 10–12-month-old 5xFAD+/- mice to further assess whether R-carvedilol pretreatment remains effective in advanced AD stages. Results from behavioral tests and LTP recordings also suggested that pretreatment with R-carvedilol could still reverse memory deficits even in 6–7 months and 10–12 months old 5xFAD+/- mice (i.e., late AD). (Yao et al., 2020).
As mentioned above, previous studies suggested that the racemic carvedilol may reduce the expression of oligomeric Aβ and prevent plaque formation and cognitive decline in multiple mouse models of AD (Arrieta-Cruz et al., 2010; Wang et al., 2011). However, Yao et al. (2020), showed that racemic carvedilol did not prevent cognitive decline in 3–4 months old 5xFAD+/- mice (Yao et al., 2020). This new finding may help us to explain the failure of a recent AD clinical trial with the racemic mixture of carvedilol (https://www.clinicaltrials.gov/ct2/show/study/NCT01354444). The exact reason for the ineffectiveness of the carvedilol racemic mixture in inhibiting AD progression is unknown. One possibility is that the strong ß-blocking effect of S-carvedilol in the racemic mixtures, especially at high doses, may adversely affect neuronal activity and cognitive function, thus counteracting the beneficial effects of R-carvedilol. Furthermore, the beneficial effect of R-carvedilol on learning and memory is dose-dependent. R-carvedilol at a dose of 3.2 mg/kg/day or 1.6 mg/kg/day, but not 0.8 mg/kg/day, rescued cognitive decline in 5xFAD mice.
In the study of Yao et al. (2020), they employed the 5xFAD mouse as the animal model of AD. Based on the original study of 5xFAD mice, this model contains 5 human FAD mutations. Thus, in addition to reproducing the prominent symptoms found in AD patients, it significantly shortens the latency to onset of AD-related pathological features (Oakley et al., 2006), significantly reducing the time period of experimental studies. However, this fast onset of AD pathology in 5xFAD mice is very different from that in human AD (Lee and Han, 2013; Jankowsky and Zheng, 2017). To determine whether R-carvedilol can also prevent and rescue cognitive decline in a mouse model whose AD progression is relatively slower than the 5xFAD mouse, Liu et al. (2021), employed the well-known 3xTG AD mouse model, which is also widely used in AD studies, but the onset of AD symptoms is relatively late (Oddo et al., 2003; Jankowsky and Zheng, 2017). 3xTG+/- mice (12–15 months old) were treated with R-carvedilol or DMSO for one month and behavioral tests and LTP measurements were conducted. Similar to the studies with 5xFAD mice, Liu et al. (2021) showed that in the training session of the MWM test, compared to the 3xTG+/- mice pretreated with DMSO, 3xTG+/- mice with R-carvedilol pre-treatment spent significantly less time to find the target platform. While in the probe trials, 3xTG+/- mice that received R-carvedilol pre-treatment spent significantly more time in the area where the platform used to be. Interestingly, mice received R-carvedilol pre-treatment also showed increased speed of swimming compared to the DMSO control group. Similarly, results from the NOR test suggested R-carvedilol pre-treatment increased the discrimination index and the walking velocity in the 3xTG+/- mice. LTP recordings also suggested that R-carvedilol pre-treatment significantly improved the neuronal circuit function in 3xTG+/- mice (Liu et al., 2021). In light of these observations, it would also be of interest to assess whether R-carvedilol could prevent cognitive decline in other AD mouse models, such as the AppNL−G-F mouse model, the AppNL/NL and AppNL−F/NL−F mouse models, and the APOEε4+/+ mouse model (Saito et al., 2014; Andrade-Talavera and Rodriguez-Moreno, 2021; Arroyo-Garcia et al., 2021; Xiong et al., 2021).
Neuronal cell death is one of the clinicopathological features observed from human AD patients (Shimohama, 2000; Niikura et al., 2006; Goel et al., 2022), but neuron loss in AD transgenic mouse models is controversial (Wirths and Bayer, 2010; Wirths and Zampar, 2020). Consistent with previous reports (Oakley et al., 2006; Jawhar et al., 2012), Yao et al. (2020) showed that in the subiculum region of the hippocampus, the number of pyramidal neurons was significantly reduced in both aged 5xFAD+/- and 3xTG+/- brain slices (Liu et al., 2021). Notably, R-carvedilol pretreated 5xFAD+/- and 3xTG+/- mice showed a significantly higher density of neurons in the subiculum area compared to the DMSO-pretreated mice. These results suggested that, like carvedilol, R-carvedilol may also have the ability to protect neurons from neuronal cell death. Besides neuronal hyperactivity, learning and memory impairment, and neuronal cell death, accumulation of aggregated Aβ in the brain is believed to be one of the major hallmarks of AD (Bharadwaj et al., 2009; Murphy and LeVine, 2010; Chen et al., 2017). R-carvedilol treatment seems to be able to rescue major deficits in AD mouse models. Can it also reduce Aβ accumulation? Surprisingly, immunohistochemical staining and immunoblotting analyses of brain samples from 5xFAD+/- mice or 3xTG+/- mice indicated that R-carvedilol pre-treatment did not affect Aβ accumulation (Yao et al., 2020; Liu et al., 2021). Therefore, shortening RyR2 open time with R-carvedilol may represent a novel strategy for treating AD without targeting Aβ.
Carvedilol has been used for treating cardiovascular diseases for decades. New indications for carvedilol are emerging. In addition to its beneficial effects on the heart, carvedilol has been shown to have potential for combatting cancer, neurological disorders, and other diseases (Lysko et al., 1992; Yamagata et al., 2004; Arrieta-Cruz et al., 2010; Wang et al., 2011; Huang et al., 2017; Chen et al., 2020; Liang et al., 2021; Abdullah Shamim et al., 2022). The list of new applications of carvedilol is expected to increase with better understanding of the mechanisms of action of carvedilol and its enantiomer, R-carvedilol. Recent studies have shown that pharmacologically shortening the open time of RyR2 with R-carvedilol prevented and reversed neuronal hyperactivity, memory impairment, and neuronal cell death in different AD mouse models without affecting the accumulation of Aβ (Yao et al., 2020; Liu et al., 2021; Sun et al., 2021). The exact mechanism(s) of these beneficial effects of R-carvedilol has not been determined, but it is likely complex and multifactorial. Nevertheless, increasing evidence suggests that in addition to targeting Aβ, R-carvedilol enantiomer brings us a novel hyperactivity-directed anti-AD therapeutic that warrants further preclinical studies and clinical trials.
JY and SC wrote the paper.
This work was supported by research grants from the Canadian Institutes of Health Research (PJT-152914) and the Heart and Stroke Foundation Chair in Cardiovascular Research (END611955) to SC. We also gratefully acknowledge the generous support from the Krembil Foundation.
The authors declare that the research was conducted in the absence of any commercial or financial relationships that could be construed as a potential conflict of interest.
All claims expressed in this article are solely those of the authors and do not necessarily represent those of their affiliated organizations, or those of the publisher, the editors and the reviewers. Any product that may be evaluated in this article, or claim that may be made by its manufacturer, is not guaranteed or endorsed by the publisher.
Abdullah Shamim, M., Yeung, S., Shahid, A., Chen, M., Wang, J., Desai, P., et al. (2022). Topical carvedilol delivery prevents UV-induced skin cancer with negligible systemic absorption. Int. J. Pharm. 611, 121302. doi:10.1016/j.ijpharm.2021.121302
Adaikkan, C., Middleton, S. J., Marco, A., Pao, P. C., Mathys, H., Kim, D. N., et al. (2019). Gamma entrainment binds higher-order brain regions and offers neuroprotection. Neuron 102 (5), 929–943. doi:10.1016/j.neuron.2019.04.011
Adaikkan, C., and Tsai, L. H. (2020). Gamma entrainment: Impact on neurocircuits, glia, and therapeutic opportunities. Trends Neurosci. 43 (1), 24–41. doi:10.1016/j.tins.2019.11.001
Alkon, D. L., Nelson, T. J., Zhao, W., and Cavallaro, S. (1998). Time domains of neuronal Ca2+ signaling and associative memory: Steps through a calexcitin, ryanodine receptor, K+ channel cascade. Trends Neurosci. 21 (12), 529–537. doi:10.1016/s0166-2236(98)01277-6
Andrade-Talavera, Y., Arroyo-Garcia, L. E., Chen, G., Johansson, J., and Fisahn, A. (2020). Modulation of Kv3.1/Kv3.2 promotes gamma oscillations by rescuing Aβ-induced desynchronization of fast-spiking interneuron firing in an AD mouse model in vitro. J. Physiol. 598 (17), 3711–3725. doi:10.1113/JP279718
Andrade-Talavera, Y., Chen, G., Kurudenkandy, F. R., Johansson, J., and Fisahn, A. (2021). Bri2 BRICHOS chaperone rescues impaired fast-spiking interneuron behavior and neuronal network dynamics in an AD mouse model in vitro. Neurobiol. Dis. 159, 105514. doi:10.1016/j.nbd.2021.105514
Andrade-Talavera, Y., Chen, G., Pansieri, J., Arroyo-Garcia, L. E., Toleikis, Z., Smirnovas, V., et al. (2022). S100A9 amyloid growth and S100A9 fibril-induced impairment of gamma oscillations in area CA3 of mouse hippocampus ex vivo is prevented by Bri2 BRICHOS. Prog. Neurobiol. 2022, 102366. doi:10.1016/j.pneurobio.2022.102366
Andrade-Talavera, Y., and Rodriguez-Moreno, A. (2021). Synaptic plasticity and oscillations in Alzheimer's disease: A complex picture of a multifaceted disease. Front. Mol. Neurosci. 14, 696476. doi:10.3389/fnmol.2021.696476
Arrieta-Cruz, I., Wang, J., Pavlides, C., and Pasinetti, G. M. (2010). Carvedilol reestablishes long-term potentiation in a mouse model of Alzheimer's disease. J. Alzheimers Dis. 21 (2), 649–654. doi:10.3233/JAD-2010-100225
Arroyo-Garcia, L. E., Isla, A. G., Andrade-Talavera, Y., Balleza-Tapia, H., Loera-Valencia, R., Alvarez-Jimenez, L., et al. (2021). Impaired spike-gamma coupling of area CA3 fast-spiking interneurons as the earliest functional impairment in the App(NL-G-F) mouse model of Alzheimer's disease. Mol. Psychiatry 26 (10), 5557–5567. doi:10.1038/s41380-021-01257-0
Balleza-Tapia, H., Crux, S., Andrade-Talavera, Y., Dolz-Gaiton, P., Papadia, D., Chen, G., et al. (2018). TrpV1 receptor activation rescues neuronal function and network gamma oscillations from Aβ-induced impairment in mouse hippocampus in vitro. Elife 7, e37703. doi:10.7554/eLife.37703
Bartsch, W., Sponer, G., Strein, K., Muller-Beckmann, B., Kling, L., Bohm, E., et al. (1990). Pharmacological characteristics of the stereoisomers of carvedilol. Eur. J. Clin. Pharmacol. 38, S104–S107. doi:10.1007/BF01409475
Bers, D. M. (2002). Cardiac excitation-contraction coupling. Nature 415 (6868), 198–205. doi:10.1038/415198a
Bharadwaj, P. R., Dubey, A. K., Masters, C. L., Martins, R. N., and Macreadie, I. G. (2009). Abeta aggregation and possible implications in Alzheimer's disease pathogenesis. J. Cell. Mol. Med. 13 (3), 412–421. doi:10.1111/j.1582-4934.2009.00609.x
Bogdanov, K. Y., Vinogradova, T. M., and Lakatta, E. G. (2001). Sinoatrial nodal cell ryanodine receptor and Na(+)-Ca(2+) exchanger: Molecular partners in pacemaker regulation. Circ. Res. 88 (12), 1254–1258. doi:10.1161/hh1201.092095
Bristow, M. R., Gilbert, E. M., Abraham, W. T., Adams, K. F., Fowler, M. B., Hershberger, R. E., et al. (1996). Carvedilol produces dose-related improvements in left ventricular function and survival in subjects with chronic heart failure. MOCHA Investigators. Circulation 94 (11), 2807–2816. doi:10.1161/01.cir.94.11.2807
Brown, J. T., Chin, J., Leiser, S. C., Pangalos, M. N., and Randall, A. D. (2011). Altered intrinsic neuronal excitability and reduced Na+ currents in a mouse model of Alzheimer's disease. Neurobiol. Aging 32 (11), e1–e14. doi:10.1016/j.neurobiolaging.2011.05.025
Bruno, A. M., Huang, J. Y., Bennett, D. A., Marr, R. A., Hastings, M. L., and Stutzmann, G. E. (2012). Altered ryanodine receptor expression in mild cognitive impairment and Alzheimer's disease. Neurobiol. Aging 33 (5), 1001. doi:10.1016/j.neurobiolaging.2011.03.011
Busche, M. A., Chen, X., Henning, H. A., Reichwald, J., Staufenbiel, M., Sakmann, B., et al. (2012). Critical role of soluble amyloid-beta for early hippocampal hyperactivity in a mouse model of Alzheimer's disease. Proc. Natl. Acad. Sci. U. S. A. 109 (22), 8740–8745. doi:10.1073/pnas.1206171109
Busche, M. A., Eichhoff, G., Adelsberger, H., Abramowski, D., Wiederhold, K. H., Haass, C., et al. (2008). Clusters of hyperactive neurons near amyloid plaques in a mouse model of Alzheimer's disease. Science 321 (5896), 1686–1689. doi:10.1126/science.1162844
Busche, M. A., and Konnerth, A. (2016). Impairments of neural circuit function in Alzheimer's disease. Philos. Trans. R. Soc. Lond. B Biol. Sci. 371, 20150429. doi:10.1098/rstb.2015.0429
Busche, M. A., and Konnerth, A. (2015). Neuronal hyperactivity--A key defect in Alzheimer's disease? Bioessays 37 (6), 624–632. doi:10.1002/bies.201500004
Castro, D. M., Dillon, C., Machnicki, G., and Allegri, R. F. (2010). The economic cost of Alzheimer's disease: Family or public health burden? Dement. Neuropsychol. 4 (4), 262–267. doi:10.1590/S1980-57642010DN40400003
Chakroborty, S., and Stutzmann, G. E. (2014). Calcium channelopathies and Alzheimer's disease: Insight into therapeutic success and failures. Eur. J. Pharmacol. 739, 83–95. doi:10.1016/j.ejphar.2013.11.012
Chen, G. F., Xu, T. H., Yan, Y., Zhou, Y. R., Jiang, Y., Melcher, K., et al. (2017). Amyloid beta: Structure, biology and structure-based therapeutic development. Acta Pharmacol. Sin. 38 (9), 1205–1235. doi:10.1038/aps.2017.28
Chen, M., Shamim, M. A., Shahid, A., Yeung, S., Andresen, B. T., Wang, J., et al. (2020). Topical delivery of carvedilol loaded nano-transfersomes for skin cancer chemoprevention. Pharmaceutics 12 (12), E1151. doi:10.3390/pharmaceutics12121151
Chen, Q., Cichon, J., Wang, W., Qiu, L., Lee, S. J., Campbell, N. R., et al. (2012). Imaging neural activity using Thy1-GCaMP transgenic mice. Neuron 76 (2), 297–308. doi:10.1016/j.neuron.2012.07.011
Cirrito, J. R., Yamada, K. A., Finn, M. B., Sloviter, R. S., Bales, K. R., May, P. C., et al. (2005). Synaptic activity regulates interstitial fluid amyloid-beta levels in vivo. Neuron 48 (6), 913–922. doi:10.1016/j.neuron.2005.10.028
Clement, A., Wiborg, O., and Asuni, A. A. (2020). Steps towards developing effective treatments for neuropsychiatric disturbances in Alzheimer's disease: Insights from preclinical models, clinical data, and future directions. Front. Aging Neurosci. 12, 56. doi:10.3389/fnagi.2020.00056
Corbett, A., Smith, J., and Ballard, C. (2012). New and emerging treatments for Alzheimer's disease. Expert Rev. Neurother. 12 (5), 535–543. doi:10.1586/ern.12.43
Demattos, R. B., Lu, J., Tang, Y., Racke, M. M., Delong, C. A., Tzaferis, J. A., et al. (2012). A plaque-specific antibody clears existing beta-amyloid plaques in Alzheimer's disease mice. Neuron 76 (5), 908–920. doi:10.1016/j.neuron.2012.10.029
Dulin, B., and Abraham, W. T. (2004). Pharmacology of carvedilol. Am. J. Cardiol. 93 (9A), 3B–6B. doi:10.1016/j.amjcard.2004.01.003
Elmaleh, D. R., Farlow, M. R., Conti, P. S., Tompkins, R. G., Kundakovic, L., and Tanzi, R. E. (2019). Developing effective Alzheimer's disease therapies: Clinical experience and future directions. J. Alzheimers Dis. 71 (3), 715–732. doi:10.3233/JAD-190507
Feuerstein, G. Z., and Ruffolo, R. R. (1995). Carvedilol, a novel multiple action antihypertensive agent with antioxidant activity and the potential for myocardial and vascular protection. Eur. Heart J. 16, 38–42. doi:10.1093/eurheartj/16.suppl_f.38
Frishman, W. H. (1998). Carvedilol. N. Engl. J. Med. 339 (24), 1759–1765. doi:10.1056/NEJM199812103392407
Furuichi, T., Furutama, D., Hakamata, Y., Nakai, J., Takeshima, H., and Mikoshiba, K. (1994). Multiple types of ryanodine receptor/Ca2+ release channels are differentially expressed in rabbit brain. J. Neurosci. 14 (8), 4794–4805. doi:10.1523/jneurosci.14-08-04794.1994
Giannini, G., Conti, A., Mammarella, S., Scrobogna, M., and Sorrentino, V. (1995). The ryanodine receptor/calcium channel genes are widely and differentially expressed in murine brain and peripheral tissues. J. Cell Biol. 128 (5), 893–904. doi:10.1083/jcb.128.5.893
Goel, P., Chakrabarti, S., Goel, K., Bhutani, K., Chopra, T., and Bali, S. (2022). Neuronal cell death mechanisms in Alzheimer's disease: An insight. Front. Mol. Neurosci. 15, 937133. doi:10.3389/fnmol.2022.937133
Hiess, F., Yao, J., Song, Z., Sun, B., Zhang, Z., Huang, J., et al. (2022). Subcellular localization of hippocampal ryanodine receptor 2 and its role in neuronal excitability and memory. Commun. Biol. 5 (1), 183. doi:10.1038/s42003-022-03124-2
Huang, K. M., Liang, S., Yeung, S., Oiyemhonlan, E., Cleveland, K. H., Parsa, C., et al. (2017). Topically applied carvedilol attenuates solar ultraviolet radiation induced skin carcinogenesis. Cancer Prev. Res. 10 (10), 598–606. doi:10.1158/1940-6207.CAPR-17-0132
Hynd, M. R., Scott, H. L., and Dodd, P. R. (2004). Glutamate-mediated excitotoxicity and neurodegeneration in Alzheimer's disease. Neurochem. Int. 45 (5), 583–595. doi:10.1016/j.neuint.2004.03.007
Iaccarino, H. F., Singer, A. C., Martorell, A. J., Rudenko, A., Gao, F., Gillingham, T. Z., et al. (2016). Gamma frequency entrainment attenuates amyloid load and modifies microglia. Nature 540 (7632), 230–235. doi:10.1038/nature20587
Jankowsky, J. L., and Zheng, H. (2017). Practical considerations for choosing a mouse model of Alzheimer's disease. Mol. Neurodegener. 12 (1), 89. doi:10.1186/s13024-017-0231-7
Jawhar, S., Trawicka, A., Jenneckens, C., Bayer, T. A., and Wirths, O. (2012). Motor deficits, neuron loss, and reduced anxiety coinciding with axonal degeneration and intraneuronal Aβ aggregation in the 5XFAD mouse model of Alzheimer's disease. Neurobiol. Aging 33 (1), e29. doi:10.1016/j.neurobiolaging.2010.05.027
Kamenetz, F., Tomita, T., Hsieh, H., Seabrook, G., Borchelt, D., Iwatsubo, T., et al. (2003). APP processing and synaptic function. Neuron 37 (6), 925–937. doi:10.1016/s0896-6273(03)00124-7
Kelliher, M., Fastbom, J., Cowburn, R. F., Bonkale, W., Ohm, T. G., Ravid, R., et al. (1999). Alterations in the ryanodine receptor calcium release channel correlate with Alzheimer's disease neurofibrillary and beta-amyloid pathologies. Neuroscience 92 (2), 499–513. doi:10.1016/s0306-4522(99)00042-1
Kennedy, M. E., Stamford, A. W., Chen, X., Cox, K., Cumming, J. N., Dockendorf, M. F., et al. (2016). The BACE1 inhibitor verubecestat (MK-8931) reduces CNS beta-amyloid in animal models and in Alzheimer's disease patients. Sci. Transl. Med. 8 (363), 363ra150. 363ra150. doi:10.1126/scitranslmed.aad9704
Kerrigan, T. L., Brown, J. T., and Randall, A. D. (2014). Characterization of altered intrinsic excitability in hippocampal CA1 pyramidal cells of the Aβ-overproducing PDAPP mouse. Neuropharmacology 79, 515–524. doi:10.1016/j.neuropharm.2013.09.004
Keskin, A. D., Kekus, M., Adelsberger, H., Neumann, U., Shimshek, D. R., Song, B., et al. (2017). BACE inhibition-dependent repair of Alzheimer's pathophysiology. Proc. Natl. Acad. Sci. U. S. A. 114 (32), 8631–8636. doi:10.1073/pnas.1708106114
Knopman, D. S., Jones, D. T., and Greicius, M. D. (2021). Failure to demonstrate efficacy of aducanumab: An analysis of the EMERGE and ENGAGE trials as reported by Biogen, December 2019. Alzheimers Dement. 17 (4), 696–701. doi:10.1002/alz.12213
Lacampagne, A., Liu, X., Reiken, S., Bussiere, R., Meli, A. C., Lauritzen, I., et al. (2017). Post-translational remodeling of ryanodine receptor induces calcium leak leading to Alzheimer's disease-like pathologies and cognitive deficits. Acta Neuropathol. 134 (5), 749–767. doi:10.1007/s00401-017-1733-7
Lee, J. E., and Han, P. L. (2013). An update of animal models of Alzheimer disease with a reevaluation of plaque depositions. Exp. Neurobiol. 22 (2), 84–95. doi:10.5607/en.2013.22.2.84
Liang, S., Shamim, M. A., Shahid, A., Chen, M., Cleveland, K. H., Parsa, C., et al. (2021). Prevention of skin carcinogenesis by the non-beta-blocking R-carvedilol enantiomer. Cancer Prev. Res. 14 (5), 527–540. doi:10.1158/1940-6207.CAPR-20-0609
Liu, Y., Yao, J., Song, Z., Guo, W., Sun, B., Wei, J., et al. (2021). Limiting RyR2 open time prevents Alzheimer's disease-related deficits in the 3xTG-AD mouse model. J. Neurosci. Res. 99 (11), 2906–2921. doi:10.1002/jnr.24936
Lysko, P. G., Lysko, K. A., Yue, T. L., Webb, C. L., Gu, J. L., and Feuerstein, G. (1992). Neuroprotective effects of carvedilol, a new antihypertensive agent, in cultured rat cerebellar neurons and in gerbil global brain ischemia. Stroke 23 (11), 1630–1635. doi:10.1161/01.str.23.11.1630
Mandikian, D., Bocksteins, E., Parajuli, L. K., Bishop, H. I., Cerda, O., Shigemoto, R., et al. (2014). Cell type-specific spatial and functional coupling between mammalian brain Kv2.1 K+ channels and ryanodine receptors. J. Comp. Neurol. 522 (15), 3555–3574. doi:10.1002/cne.23641
Martorell, A. J., Paulson, A. L., Suk, H. J., Abdurrob, F., Drummond, G. T., Guan, W., et al. (2019). Multi-sensory gamma stimulation ameliorates alzheimer's-associated pathology and improves cognition. Cell 177 (2), 256–271. doi:10.1016/j.cell.2019.02.014
Mehta, D., Jackson, R., Paul, G., Shi, J., and Sabbagh, M. (2017). Why do trials for Alzheimer's disease drugs keep failing? A discontinued drug perspective for 2010-2015. Expert Opin. Investig. Drugs 26 (6), 735–739. doi:10.1080/13543784.2017.1323868
Murayama, T., and Ogawa, Y. (1996). Properties of Ryr3 ryanodine receptor isoform in mammalian brain. J. Biol. Chem. 271 (9), 5079–5084. doi:10.1074/jbc.271.9.5079
Murphy, M. P., and LeVine, H. (2010). Alzheimer's disease and the amyloid-beta peptide. J. Alzheimers Dis. 19 (1), 311–323. doi:10.3233/JAD-2010-1221
Nelson, M. T., Cheng, H., Rubart, M., Santana, L. F., Bonev, A. D., Knot, H. J., et al. (1995). Relaxation of arterial smooth muscle by calcium sparks. Science 270 (5236), 633–637. doi:10.1126/science.270.5236.633
Nichols, A. J., Sulpizio, A. C., Ashton, D. J., Hieble, J. P., and Ruffolo, R. R. (1989). The interaction of the enantiomers of carvedilol with alpha 1- and beta 1-adrenoceptors. Chirality 1 (4), 265–270. doi:10.1002/chir.530010404
Niikura, T., Tajima, H., and Kita, Y. (2006). Neuronal cell death in Alzheimer's disease and a neuroprotective factor, humanin. Curr. Neuropharmacol. 4 (2), 139–147. doi:10.2174/157015906776359577
Oakley, H., Cole, S. L., Logan, S., Maus, E., Shao, P., Craft, J., et al. (2006). Intraneuronal beta-amyloid aggregates, neurodegeneration, and neuron loss in transgenic mice with five familial Alzheimer's disease mutations: Potential factors in amyloid plaque formation. J. Neurosci. 26 (40), 10129–10140. doi:10.1523/JNEUROSCI.1202-06.2006
Oddo, S., Caccamo, A., Shepherd, J. D., Murphy, M. P., Golde, T. E., Kayed, R., et al. (2003). Triple-transgenic model of Alzheimer's disease with plaques and tangles: Intracellular abeta and synaptic dysfunction. Neuron 39 (3), 409–421. doi:10.1016/s0896-6273(03)00434-3
Oules, B., Del Prete, D., Greco, B., Zhang, X., Lauritzen, I., Sevalle, J., et al. (2012). Ryanodine receptor blockade reduces amyloid-beta load and memory impairments in Tg2576 mouse model of Alzheimer disease. J. Neurosci. 32 (34), 11820–11834. doi:10.1523/JNEUROSCI.0875-12.2012
Prigerson, H. G. (2003). Costs to society of family caregiving for patients with end-stage Alzheimer's disease. N. Engl. J. Med. 349 (20), 1891–1892. doi:10.1056/NEJMp038157
Rocca, W. A., Bonaiuto, S., Lippi, A., Luciani, P., Turtu, F., Cavarzeran, F., et al. (1990). Prevalence of clinically diagnosed Alzheimer's disease and other dementing disorders: A door-to-door survey in appignano, macerata province, Italy. Neurology 40 (4), 626–631. doi:10.1212/wnl.40.4.626
Rosenberg, P. B., Mielke, M. M., Tschanz, J., Cook, L., Corcoran, C., Hayden, K. M., et al. (2008). Effects of cardiovascular medications on rate of functional decline in Alzheimer disease. Am. J. Geriatr. Psychiatry 16 (11), 883–892. doi:10.1097/JGP.0b013e318181276a
Saito, T., Matsuba, Y., Mihira, N., Takano, J., Nilsson, P., Itohara, S., et al. (2014). Single App knock-in mouse models of Alzheimer's disease. Nat. Neurosci. 17 (5), 661–663. doi:10.1038/nn.3697
SanMartin, C. D., Veloso, P., Adasme, T., Lobos, P., Bruna, B., Galaz, J., et al. (2017). RyR2-Mediated Ca(2+) release and mitochondrial ROS generation partake in the synaptic dysfunction caused by amyloid beta peptide oligomers. Front. Mol. Neurosci. 10, 115. doi:10.3389/fnmol.2017.00115
Scala, F., Fusco, S., Ripoli, C., Piacentini, R., Li Puma, D. D., Spinelli, M., et al. (2015). Intraneuronal Aβ accumulation induces hippocampal neuron hyperexcitability through A-type K(+) current inhibition mediated by activation of caspases and GSK-3. Neurobiol. Aging 36 (2), 886–900. doi:10.1016/j.neurobiolaging.2014.10.034
Sevigny, J., Chiao, P., Bussiere, T., Weinreb, P. H., Williams, L., Maier, M., et al. (2016). The antibody aducanumab reduces Aβ plaques in Alzheimer's disease. Nature 537 (7618), 50–56. doi:10.1038/nature19323
Shimohama, S. (2000). Apoptosis in Alzheimer's disease--an update. Apoptosis 5 (1), 9–16. doi:10.1023/a:1009625323388
Siskova, Z., Justus, D., Kaneko, H., Friedrichs, D., Henneberg, N., Beutel, T., et al. (2014). Dendritic structural degeneration is functionally linked to cellular hyperexcitability in a mouse model of Alzheimer's disease. Neuron 84 (5), 1023–1033. doi:10.1016/j.neuron.2014.10.024
Smith, I. F., Hitt, B., Green, K. N., Oddo, S., and LaFerla, F. M. (2005). Enhanced caffeine-induced Ca2+ release in the 3xTg-AD mouse model of Alzheimer's disease. J. Neurochem. 94 (6), 1711–1718. doi:10.1111/j.1471-4159.2005.03332.x
Stargardt, A., Swaab, D. F., and Bossers, K. (2015). Storm before the quiet: Neuronal hyperactivity and aβ in the presymptomatic stages of Alzheimer's disease. Neurobiol. Aging 36 (1), 1–11. doi:10.1016/j.neurobiolaging.2014.08.014
Stoschitzky, K., Koshucharova, G., Lercher, P., Maier, R., Sakotnik, A., Klein, W., et al. (2001). Stereoselective effects of (R)- and (S)-carvedilol in humans. Chirality 13 (7), 342–346. doi:10.1002/chir.1042
Stutzmann, G. E. (2021). RyR2 calcium channels in the spotlight-I'm ready for my close up, Dr. Alzheimer. Cell Calcium 94, 102342. doi:10.1016/j.ceca.2020.102342
Sun, B., Yao, J., Chen, A. W., Estillore, J. P., Wang, R., Back, T. G., et al. (2021). Genetically and pharmacologically limiting RyR2 open time prevents neuronal hyperactivity of hippocampal CA1 neurons in brain slices of 5xFAD mice. Neurosci. Lett. 758, 136011. doi:10.1016/j.neulet.2021.136011
Targa Dias Anastacio, H., Matosin, N., and Ooi, L. (2022). Neuronal hyperexcitability in Alzheimer's disease: What are the drivers behind this aberrant phenotype? Transl. Psychiatry 12 (1), 257. doi:10.1038/s41398-022-02024-7
Wang, J., Ono, K., Dickstein, D. L., Arrieta-Cruz, I., Zhao, W., Qian, X., et al. (2011). Carvedilol as a potential novel agent for the treatment of Alzheimer's disease. Neurobiol. Aging 32 (12), 2321. doi:10.1016/j.neurobiolaging.2010.05.004
Wilcock, G. K., Gauthier, S., Frisoni, G. B., Jia, J., Hardlund, J. H., Moebius, H. J., et al. (2018). Potential of low dose leuco-methylthioninium bis(hydromethanesulphonate) (LMTM) monotherapy for treatment of mild Alzheimer's disease: Cohort analysis as modified primary outcome in a phase III clinical trial. J. Alzheimers Dis. 61 (1), 435–457. doi:10.3233/JAD-170560
Wirths, O., and Bayer, T. A. (2010). Neuron loss in transgenic mouse models of Alzheimer's disease. Int. J. Alzheimers Dis. 2010, 723782. doi:10.4061/2010/723782
Wirths, O., and Zampar, S. (2020). Neuron loss in Alzheimer's disease: Translation in transgenic mouse models. Int. J. Mol. Sci. 21 (21), E8144. doi:10.3390/ijms21218144
Xiong, M., Jiang, H., Serrano, J. R., Gonzales, E. R., Wang, C., Gratuze, M., et al. (2021). APOE immunotherapy reduces cerebral amyloid angiopathy and amyloid plaques while improving cerebrovascular function. Sci. Transl. Med. 13 (581), eabd7522. doi:10.1126/scitranslmed.abd7522
Yamagata, K., Ichinose, S., and Tagami, M. (2004). Amlodipine and carvedilol prevent cytotoxicity in cortical neurons isolated from stroke-prone spontaneously hypertensive rats. Hypertens. Res. 27 (4), 271–282. doi:10.1291/hypres.27.271
Yamamoto, K., Tanei, Z. I., Hashimoto, T., Wakabayashi, T., Okuno, H., Naka, Y., et al. (2015). Chronic optogenetic activation augments aβ pathology in a mouse model of Alzheimer disease. Cell Rep. 11 (6), 859–865. doi:10.1016/j.celrep.2015.04.017
Yao, J., Sun, B., Institoris, A., Zhan, X., Guo, W., Song, Z., et al. (2020). Limiting RyR2 open time prevents Alzheimer's disease-related neuronal hyperactivity and memory loss but not beta-amyloid accumulation. Cell Rep. 32 (12), 108169. doi:10.1016/j.celrep.2020.108169
Yiannopoulou, K. G., Anastasiou, A. I., Zachariou, V., and Pelidou, S. H. (2019). Reasons for failed trials of disease-modifying treatments for alzheimer disease and their contribution in recent research. Biomedicines 7 (4), E97. doi:10.3390/biomedicines7040097
Yue, T. L., Cheng, H. Y., Lysko, P. G., McKenna, P. J., Feuerstein, R., Gu, J. L., et al. (1992). Carvedilol, a new vasodilator and beta adrenoceptor antagonist, is an antioxidant and free radical scavenger. J. Pharmacol. Exp. Ther. 263 (1), 92–98.
Zhang, J., Zhou, Q., Smith, C. D., Chen, H., Tan, Z., Chen, B., et al. (2015). Non-beta-blocking R-carvedilol enantiomer suppresses Ca2+ waves and stress-induced ventricular tachyarrhythmia without lowering heart rate or blood pressure. Biochem. J. 470 (2), 233–242. doi:10.1042/BJ20150548
Zhou, Q., Xiao, J., Jiang, D., Wang, R., Vembaiyan, K., Wang, A., et al. (2011). Carvedilol and its new analogs suppress arrhythmogenic store overload-induced Ca2+ release. Nat. Med. 17 (8), 1003–1009. doi:10.1038/nm.2406
Keywords: carvedilol, R-carvedilol, Alzheimer’s disease, RyR2, neuronal hyperactivity
Citation: Yao J and Chen SRW (2022) R-carvedilol, a potential new therapy for Alzheimer’s disease. Front. Pharmacol. 13:1062495. doi: 10.3389/fphar.2022.1062495
Received: 06 October 2022; Accepted: 15 November 2022;
Published: 24 November 2022.
Edited by:
Jianqiang Xu, Dalian University of Technology, ChinaReviewed by:
Yuniesky Andrade Talavera, Universidad Pablo de Olavide, SpainCopyright © 2022 Yao and Chen. This is an open-access article distributed under the terms of the Creative Commons Attribution License (CC BY). The use, distribution or reproduction in other forums is permitted, provided the original author(s) and the copyright owner(s) are credited and that the original publication in this journal is cited, in accordance with accepted academic practice. No use, distribution or reproduction is permitted which does not comply with these terms.
*Correspondence: Jinjing Yao, amluamluZy55YW9AdWNhbGdhcnkuY2E=; S. R. Wayne Chen, c3djaGVuQHVjYWxnYXJ5LmNh
Disclaimer: All claims expressed in this article are solely those of the authors and do not necessarily represent those of their affiliated organizations, or those of the publisher, the editors and the reviewers. Any product that may be evaluated in this article or claim that may be made by its manufacturer is not guaranteed or endorsed by the publisher.
Research integrity at Frontiers
Learn more about the work of our research integrity team to safeguard the quality of each article we publish.