- 1School of Health Preservation and Rehabilitation, Chengdu University of Traditional Chinese Medicine, Chengdu, China
- 2State Administration of Traditional Chinese Medicine Key Laboratory of Traditional Chinese Medicine Regimen and Health, Chengdu University of Traditional Chinese Medicine, Chengdu, China
- 3Key Laboratory of Traditional Chinese Medicine Regimen and Health of Sichuan Province, Chengdu University of Traditional Chinese Medicine, Chengdu, China
- 4College of Materials Science and Engineering, Southwest Forestry University, Kunming, Yunnan, China
Initially described as an ancient and highly conserved catabolic biofunction, autophagy plays a significant role in disease pathogenesis and progression. As the bioactive ingredient of Salvia miltiorrhiza, tanshinone has recently shown profound effects in alleviating and treating various diseases by regulating autophagy. However, compared to the remarkable achievements in the known pharmacological effects of this traditional Chinese medicine, there is a lack of a concise and comprehensive review deciphering the mechanism by which tanshinone regulates autophagy for medicinal research. In this context, we concisely review the advances of tanshinone in regulating autophagy for medicinal research, including human cancer, the nervous system, and cardiovascular diseases. The pharmacological effects of tanshinone targeting autophagy involve the regulation of autophagy-related proteins, such as Beclin-1, LC3-II, P62, ULK1, Bax, ATG3, ATG5, ATG7, ATG9, and ATG12; the regulation of the PI3K/Akt/mTOR, MEK/ERK/mTOR, Beclin-1-related, and AMPK-related signaling pathways; the accumulation of reactive oxygen species (ROS); and the activation of AMPK. Notably, we found that tanshinone played a dual role in human cancers in an autophagic manner, which may provide a new avenue for potential clinical application. In brief, these findings on autophagic tanshinone and its derivatives provide a new clue for expediting medicinal research related to tanshinone compounds and autophagy.
Introduction
In the long history of human society, over hundreds of thousands of years, traditional Chinese medicine (TCM) and botanical medicine have played significant roles in protecting human beings from diseases (Yuan et al., 2016; Caesar and Cech, 2019; Atanasov et al., 2021; Scherlach and Hertweck, 2021). Among them, Salvia miltiorrhiza, one of Asia’s most widely used medicinal herbs, demonstrates impressive clinical efficacy against various diseases (Su et al., 2015; Shaito et al., 2020; Ansari et al., 2021), such as cardiovascular and cerebrovascular diseases (Su et al., 2015; Li et al., 2018), liver cirrhosis (Shi et al., 2019), and chronic renal failure (Shao et al., 2021). With modern medicine’s rapid and remarkable development, the scientific community has gradually revealed the magical medicinal effect of Salvia miltiorrhiza. In recent years, multiple reviews have been conducted to decipher the mechanisms and found that anti-inflammation, anti-oxidation, anti-fibrosis, anti-apoptotic, etc., are the main pharmacological effects of this traditional Chinese medicine. Despite the remarkable achievements made above, recent experiments have proven that several tanshinones and their derivatives also play an essential role in regulating the autophagic process to cure different diseases. These potential molecules that regulate autophagy are divided into two types (Figure 1): a) hydrophilic components, which are water-soluble substances such as tanshinone IIA sodium sulfonate (TSN-SS), and b) extensively studied lipophilic components, such as tanshinone I (Tan I), tanshinone IIA (Tan IIA), dihydrotanshinone, and (iso) cryptotanshinone. Tan IIA, a lipophilic diterpene quinone, has positively affected various diseases, such as coronary heart disease, angina pectoris, and Alzheimer’s disease (Gao et al., 2012; Ren et al., 2019; Zhou et al., 2021). Experimental results prove that Tan IIA can regulate cell apoptosis, inhibit cell proliferation and migration, and promote cell viability to treat the diseases mentioned above (Ansari et al., 2021). TSN-SS, a water-soluble compound obtained by sulfonation of Tan IIA, has been approved by the China Food and Drug Administration (CFDA) to treat cardiovascular diseases (Zhou et al., 2019). Moreover, Tan I, another crucial bioactive component of Salvia miltiorrhiza, shows anticancer, neuroprotective, and anti-inflammatory activities (Chen et al., 2014; Li et al., 2018). These advancements provide an opportunity to discover new drugs from Salvia miltiorrhiza.
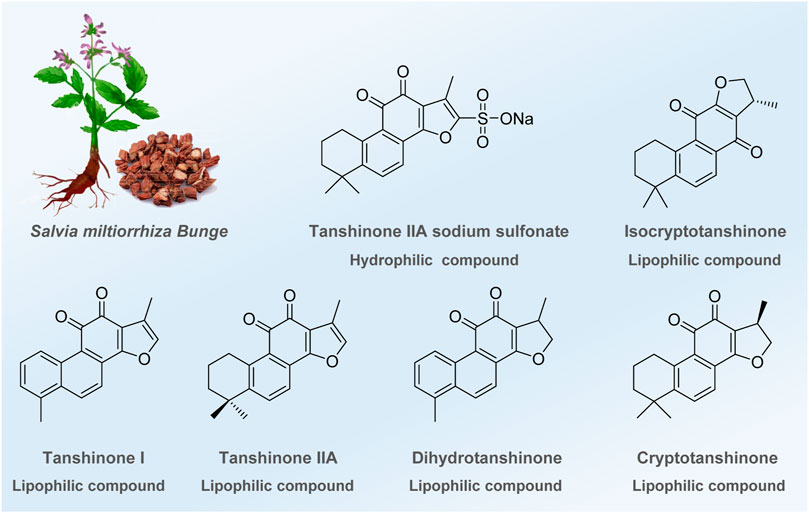
FIGURE 1. Bioactive ingredients identified or modified from Salvia miltiorrhiza in regulating autophagy.
Autophagy, as an evolutionarily ancient and highly conserved catabolic process, is a self-protective mechanism of cells and plays an essential role in regulating cellular homeostasis under physiological and pathophysiological conditions (Mizushima, 2018; Yim and Mizushima, 2020). Generally, the autophagy process involves three steps: the formation of double-membrane vesicles, the formation of autophagosomes by phagocyte proteins and organelles, and subsequent fusion with lysosomes to complete the degradation process (Parzych and Klionsky, 2014). Under normal conditions, autophagy is conducive to maintaining cellular homeostasis, while defects in autophagy function can cause abnormal tissue function and are associated with various diseases. For example, dysregulated autophagy is involved in the pathogenesis of inflammatory bowel disease by producing proinflammatory cytokines and promoting intestinal epithelial cell stress (El-Khider and McDonald, 2016). The absence of autophagy pathways leads to the accumulation of two proteins, tau, and β-amyloid peptide, which are the main pathological changes in Alzheimer’s disease (Li et al., 2017). Recent studies have indicated that the regulation of autophagy plays a non-negligible role in treating diabetes mellitus (Kim and Lee, 2014; Zhang et al., 2018), Parkinson’s disease (Nixon, 2013; Tran and Reddy, 2021), chronic obstructive pulmonary (Racanelli et al., 2018), and malignant tumors (Galluzzi et al., 2017; Li et al., 2020). Of note, Lu et al., 2019 demonstrated that an autophagosome-tethering compound (ATTEC) specifically induced mutant huntingtin protein (mHTT) degradation by targeting pathogenic proteins to autophagosomes through interaction with autophagy-associated protein LC3 and the pathogenic protein mHTT of Huntington’s disease (HD). This result demonstrated the possibility of using autophagosome-coupled compounds to target protein degradation and provided a new opportunity for drug discovery (Li et al., 2019). Moreover, the treatment of hepatocellular carcinoma by artesunate through glucosylceramidase-mediated autophagic degradation has entered preclinical investigation (Chen et al., 2022). Hence, developing relevant drugs targeting autophagy will be a promising strategy for treating diseases that are unavailable with conventional medicinal treatment. Indeed, remarkable progress has been made in discovering small molecules targeting autophagy, such as chloroquine and hydroxychloroquine, in recent years (Limpert et al., 2018; Xiang et al., 2020; Xie et al., 2021). However, current achievements gradually fail to fulfill the vast and complex drug needs in the clinic. Therefore, enriching the toolbox of clinical medicines is becoming a priority for researchers in relevant fields.
Phytochemicals from medicinal herbs and plants have always been a vital source for discovering new drugs, and the successful application of TSN-SS has been confirmed (Newman and Cragg, 2020; Miethke et al., 2021). In recent years, advances in pharmacological research focusing on Salvia miltiorrhiza, mainly concentrating on various cancers (Fang et al., 2021) and cardiovascular system diseases (Li et al., 2018; Ren et al., 2019), have been successively reported and systematically summarized. Nevertheless, compared with these achievements, the progress of tanshinone that regulates autophagy for curing various diseases lacks a concise and systematic review despite scattered literature documented in several reviews (Bao et al., 2020; Subedi and Gaire, 2021). Considering the therapeutic efficacy of tanshinone in the clinic, we concisely and systematically reviewed the advances of tanshinone identified from Salvia miltiorrhiza in treating various diseases by regulating autophagy functions in different cells and animal models. For the convenience of readers, this review is divided into three parts according to the types of autophagy-related diseases, including human cancer, nervous system diseases, and cardiovascular diseases. Additionally, the summary and outlook on this promising field are described at the end of this review. Together, these findings on autophagic tanshinone and its derivatives provide a new clue for expediting medicinal research related to tanshinone and autophagy.
Targeting autophagy for human cancers
Autophagy plays a dual role in the initiation and progression of human cancers (Li et al., 2020; Yun et al., 2021). On the one hand, by preventing the accumulation of damaged proteins and organelles, autophagy limits oncogenic signaling, oxidative stress, and chronic tissue damage to suppress cancer initiation (White et al., 2015). On the other hand, cancer cells can utilize autophagy-mediated recycling to meet the elevated metabolic demand of growth and proliferation (White et al., 2015). This section introduces the potential of tanshinone to target autophagy for various human cancers, which are classified by signaling pathways (Amaravadi et al., 2019).
PI3K/Akt/mTOR signaling pathway
The PI3K/Akt/mTOR signaling pathway is a classical signaling pathway that regulates autophagy and mainly includes phosphatidylinositol-3-kinase (PI3K), the serine/threonine kinase Akt, known as protein kinase B (PKB), and mammalian target of rapamycin (mTOR) (Jung et al., 2010). In recent years, studies have revealed that inhibiting the PI3K/Akt/mTOR signaling pathway can induce autophagic cell death by up-regulating the level of autophagy, which is an effective means to end or inhibit the progression of cancer (Popova and Juecker, 2021).
Glioma seriously affects patients’ quality of life as a malignant intracranial tumor. Ding et al. (2017) demonstrated that Tan IIA could inhibit glioma cell proliferation, promote cell autophagy and apoptosis, and play an antitumor role by suppressing the PI3K/Akt/mTOR pathway and increasing the expression of the proteins MAP1LC3 (LC3B), Beclin-1, and Bcl2-associated X (Bax) in U251 cells. This result might provide a new effective targeted therapeutic strategy for glioma. As a highly malignant tumor, melanoma lacks specific drugs except for early surgical treatment. Liu’s group proposed a model in which Tan IIA reduced A375 cell proliferation by activating autophagy by blocking the PI3K/Akt/mTOR/p70S6K1 signaling pathway and increasing autophagy-related genes such as Beclin-1 and LC3-II (Li et al., 2017). Meanwhile, the author confirmed that Tan IIA could reduce melanoma A375-induced tumor volume and weight in the mouse model. This result may inspire the search for new anti-melanoma drugs derived from Tan IIA. As the dominant form of liver cancer, hepatocellular carcinoma (HCC) is a fatal disease with no effective treatment or positive prognosis. Yuan’s work demonstrated that cryptotanshinone (CPT) could simultaneously induce apoptosis and autophagy in Huh7 and MHCC97-H cells by regulating the PI3K/Akt/mTOR signaling pathway, leading to up-regulation of related proteins such as poly ADP-ribose polymerase (PARP), Bax, caspase-3, LC3-II, Beclin-1, and ATG5. In addition, CPT was found to inhibit the growth of Huh7 xenograft tumors, and is expected to be a potential candidate therapeutic agent for HCC treatment (Luo et al., 2020). Lung cancer is one of the most common and worst cancers worldwide, with a 5-year survival rate of 10%–20% (Neal et al., 2019). A recent report demonstrated that isocryptotanshinone (ICPT) could induce pro-death autophagy by down-regulating the expression of p-Akt and p-mTOR in A549 cells, thereby inhibiting cell proliferation and exerting anti-lung cancer effects. (Guo et al., 2016). Breast cancer has overtaken lung cancer as the most newly diagnosed cancer globally. While exploring the antitumor mechanism of Tan IIA, Zhang and coworkers found that it could induce cell cycle arrest and autophagy by inhibiting the activation of the PI3K/Akt/mTOR pathway in MCF-7 breast cancer cells (Lv et al., 2018). In addition, another active component, Tan I, also showed inhibitory effects in ovarian cancer. In 2020, a research report provided the first evidence that Tan I promoted autophagy by inhibiting the PI3K/AKT/mTOR pathway and up-regulating the autophagy-associated proteins Beclin-1, ATG7, and LC3-II in A2780 and ID-8 ovarian cancer cells, leading to the inhibition of tumor growth. These reports provided new insight into the anticancer mechanism of tanshinone (Zhou et al., 2020).
Beclin-1-related signaling pathway
The Beclin-1 gene was the first mammalian autophagy gene found by yeast two-hybrid screening in 1998 by Beth Levine’s group (Liang Xiao et al., 1998). Beclin-1 is extremely important for autophagic vesicle enucleation at the beginning of autophagosome formation as a critical regulator of autophagy. Several studies have revealed that Tan IIA displays an antitumor effect via the Beclin-1-dependent pathway. Hu’s group explored the antitumor effect of Tan IIA on human osteosarcoma MG-63 cells and found that concentrations of Tan IIA (5 and 10 mg/L) induced autophagic cell death and eventually contributed to apoptosis by the production of excessive ROS (Ma et al., 2016). Oral squamous cell carcinoma is one of the most malignant and harmful tumors. Ji’s group found that Tan IIA induced cell death in an autophagy-dependent manner in SCC-9 cells in a multipronged manner by inducing the Beclin-1/Atg7/Atg12-Atg5 signaling pathway and suppressing the PI3K/Akt/mTOR pathway (Qiu et al., 2018). Additionally, the author found that Tan IIA inhibited the growth of solid tumors in severe combined immune deficiency (SCID) mice in a Beclin-1-dependent manner. In addition, Tan IIA can also be used as an adjuvant drug for oral cell carcinoma. Wang’s group reported that Tan IIA exerted a strong radiosensitizing effect on SCC090 cells compared with the simple drug or single radiation treatment due to enhanced autophagy with increased protein levels of Beclin-1, Atg5, and LC3-II, the three critical proteins involved in autophagy (Ding et al., 2016). The drug combination is becoming an effective strategy for cancer treatment, and He’s group demonstrated that Tan I could induce pro-survival autophagy in vitro and in vivo by increasing the expression of the Beclin-1/Vps34 complex after treatment with Tan I by inhibiting B-cell lymphoma-2 (Bcl-2) expression in gastric cancer. The report also showed that the combination of the autophagy inhibitor chloroquine and Tan I could inhibit tumor growth more efficiently than monotherapy, which might be considered an effective strategy for treating gastric cancer (Jing et al., 2016).
Reactive oxygen species
ROS is a general term for a class of active oxygen-containing compounds produced by aerobic metabolism organisms, including superoxide anions, free radicals, and peroxides (Rahal et al., 2014). Autophagy is one of the essential mechanisms for coping with ROS-mediated oxidative stress (Filomeni et al., 2015; Wu et al., 2022a). On the one hand, autophagy can be triggered to remove oxidized proteins or damaged organelles, thus maintaining cell homeostasis. On the other hand, when ROS accumulation is excessive or prolonged, autophagic death is subsequently induced. Therefore, the regulation of ROS and autophagy is significant for maintaining body homeostasis.
Cell death is an essential physiological process and regulating the autophagy of tumor cells is a good strategy for cancer therapy. Li and coworkers demonstrated that Tan IIA induced apoptosis and autophagy by accumulating ROS in PC-3 cells, thus inhibiting cancer cell growth and inducing cell death (Li et al., 2016). Notably, compared with the cells treated with Tan IIA, the combination of Tan IIA with 3-methyladenine (3-MA) increased the apoptotic ratio, implying that Tan IIA-induced apoptosis was mediated through autophagy in PC-3 prostate cancer cells. In addition, it has been reported that estrogen (ER) stress is closely related to cancer cell death and is an important downstream target of ROS. Guo’s group evaluated the anticancer potential of Tan I against glioblastoma and demonstrated that Tan I inhibited the proliferation of U87MG cells via the induction of apoptosis and G2/M cell cycle arrest (Jian et al., 2020). Mechanistic experiments indicated that Tan I triggered ER oxidative stress and Akt-mediated apoptosis by inducing the accumulation of intracellular ROS. Interestingly, protective autophagy was also triggered by the mechanism described above, supported by pretreatment with 3-MA, which effectively enhanced the Tan I-induced inhibition of U87 MG cells. These findings indicated that Tan I could be a potential anticancer drug candidate for glioblastoma treatment. For the multidrug-resistant colon cancer cell line SW620 Ad300, another report demonstrated that CPT induced autophagic cell death via the ROS-p38 MAPK-NF-κB signaling pathway. Importantly, their results also demonstrated a complementary relationship between CPT-induced apoptosis and autophagic cell death. This study indicated that CPT might be a potential chemotherapeutic agent for cancer treatment (Xu et al., 2017). In addition to the monomeric component, the mixed extract of total tanshinone (TDT) also plays a vital role in cancer treatment. Chen’s group found that TDT inhibited the proliferation of cancer cells by inducing apoptosis and protective autophagy by increasing the formation of intracellular ROS in 95D lung cancer cells and showed better cytotoxic effects than Tan IIA (Gao et al., 2015).
AMPK-related signaling pathway
Adenosine 5′-monophosphate (AMP)-activated protein kinase (AMPK), a trimer composed of α, β, and γ subunits, is a critical kinase regulating bioenergy metabolism (Yuan et al., 2020). It is mainly affected by the adenosine monophosphate/adenosine triphosphate (AMP/ATP) ratio and maintains metabolic balance by regulating the activity of downstream signals (Faubert et al., 2015). Moreover, AMPK plays a positive role in autophagy. Under starvation conditions, the AMP/ATP ratio is increased, and phosphorylation of AMPKα activates AMPK, which phosphorylates the tuberous sclerosis complex (TSC) and regulatory-associated protein of the mammalian target of rapamycin (RAPTOR), and then inhibits the mTORC1 for the regulation of autophagy (Wu et al., 2015). In addition, the activation of AMPK also phosphorylates unc-51-like kinase 1 (ULK1), promoting its activity and activating the autophagy process (Fan et al., 2015). Li’s group explored the effect and mechanism of Tan I on breast cancer and found that Tan I could induce autophagy by up-regulating the phosphorylation of AMPKα and its downstream ULK1, thus effectively inhibiting the proliferation of the breast cancer cell line MDA-MB-231 (Zheng et al., 2020). Furthermore, the conduction of APMK upstream signaling molecules can also affect the regulation of autophagy. Su’s group demonstrated that Tan IIA-induced recruitment of the sestrin 2 (SESN2) promoter through the hematopoietic progenitor kinase-germinal cell kinase-like kinase/stress-activated protein kinase/c-Jun N-terminal kinase (HGK/SAPK/JNK-Jun) signaling axis led to SESN2/AMPK-α activation, thereby inducing autophagy and inhibiting osteosarcoma growth (Yen et al., 2018). In addition, Park et al. reported that CPT robustly activated the AMPK signaling pathway, including liver kinase B1 (LKB1), p53, and TSC2, suppressing mTORC1 in HepG2 cells and inducing autophagic cell death. These data suggest that CPT deserves further investigation as a novel specific AMPK activator in cancer treatment (Park et al., 2014).
Miscellaneous signaling pathway
Apart from the pathways described above, components of Salvia miltiorrhiza can also regulate autophagy through other pathways to exert antitumor activity, such as the caspase-dependent mitogen-activated protein kinase/extracellular regulated protein kinase/mammalian target of rapamycin (MEK/ERK/mTOR) pathway (Qian et al., 2020), and p53/damage-regulated autophagy modulator (p53/DRAM)-mediated autophagy (Liu and Liu, 2020). Colon cancer is a common malignancy of the digestive tract. Wu’s group reported that dihydrotanshinone I (DHTS) promoted cancer cell apoptosis by mediating caspase-dependent autophagy through mitochondria in the human colon cancer HCT116 cell line (Wang et al., 2015). Later, Huo’s group found that Tan IIA stimulated autophagy in the colon cancer cell lines SW480 and HT29 by activating the MEK/ERK/mTOR pathway, thus inhibiting the growth of colon cancer cells (Qian et al., 2020). In addition, a report proposed by Chen indicated that another bioactive ingredient, CPT, induced apoptosis of HCT116 cells through ER stress-mediated autophagy, suggesting that CPT could be a promising therapeutic candidate for colorectal cancer treatment (Fu et al., 2021). P62/sequestosome1 (P62/SQSTM1) is a multifunctional protein that plays an essential role in autophagy. Kim’s group reported that Tan I exhibited antitumor activity in mesothelioma cells, and the activation of JNK and inositol-requiring enzyme 1 (IRE1) was critically involved in Tan I-induced p62/SQSTM1-dependent autophagy (Lee et al., 2017). As a critical tumor suppressor gene, p53 can also participate in the regulation of autophagy. Liu’s group investigated Tan I-induced cell apoptosis by repressing p53/DRAM-mediated autophagy in human hepatocellular carcinoma HepG2 and Huh7 cells (Liu and Liu, 2020). These results suggested that the bioactive ingredients of Salvia miltiorrhiza could be promising lead compounds for curing cancers through autophagy function.
Notably, Tan IIA is also used in combination therapy for cancer. Su’s group reported that Tan IIA potentiated the efficacy of fluorouracil (5-FU) in colon cancer in SCID mouse model by down-regulating LC3-II protein expression. This outcome suggested that Tan IIA may be a promising strategy for adjuvant chemotherapy for colon cancer (Su, 2012). In addition, Shen’s group first revealed that cotreatment with doxorubicin and Tan IIA activated autophagic cell death in drug-resistant gastric cancer cells, indicating that Tan IIA was a valuable agent with the potential to treat drug-resistant gastric cancer in combination therapy (Xu et al., 2018).
Targeting autophagy for nervous system disease
Recent studies have disclosed that Tan IIA and its derivatives show potential in treating Alzheimer’s disease, age-related macular degeneration (AMD), and cerebral ischemic stroke in an autophagy-dependent manner (Ansari et al., 2021; Subedi and Gaire, 2021). Alzheimer’s disease (AD), caused by the accumulation of β-amyloid protein (Aβ), is a neurodegenerative disease that seriously affects the function of neurons (Yan and Vassar, 2014; Fan et al., 2020; Ju and Tam, 2022). Neurons rely heavily on autophagy to degrade dysfunctional cytoplasmic components, protein aggregates, and damaged organelles within cells to maintain the homeostasis of protein metabolism (Scrivo et al., 2018). Hence, eliminating the neurotoxicity caused by Aβ is considered a potential strategy for treating AD. Wan’s group investigated the possible neuroprotective mechanism of Tan IIA on Aβ25–35-induced spatial memory impairment in mice (Zhu et al., 2017). The results indicated that Tan IIA inhibited autophagy in the hippocampus by up-regulating RACK1 to suppress Beclin1 protein expression and down-regulate the LC3-II/I ratio, thereby attenuating Aβ25–35-induced spatial memory impairment in mice. As a progressive and devastating neurodegenerative malady, age-related macular degeneration (AMD) contributes to blindness among elderly individuals globally (Mitchell et al., 2018; Fleckenstein et al., 2021). Oxidative stress injury in the retinal pigment epithelium (RPE) is the main factor in the occurrence of AMD (Kauppinen et al., 2016; Kaarniranta et al., 2020), which triggers the degradation of RPE in an autophagic manner. Huang’s group investigated the protective effect and mechanism of TSN-SS on ARPE-19 cells under oxidative stress (Han et al., 2018). Their report showed that TSN-SS activated the PI3K/AKT/mTOR pathway to inhibit autophagy and diminished the expression of the autophagic proteins Beclin-1, ATG3, ATG7, and ATG9 in ARPE-19 cells under oxidative stress.
Meanwhile, oxidative stress has been shown to severely impact the nervous system during a cerebral ischemic stroke by generating ROS that leads to mitochondrial dysfunction, DNA integrity loss, and protein misfolding (Shi et al., 2019), followed by autophagic degradation to remove these dysfunctional substances. Han’s group established a cerebral ischemia model in vivo with hippocampal neurons cultured by hypoxia-glucose deprivation-reperfusion (OGD/R) (Zhu et al., 2017). The author found that Tan IIA activated the PI3K/Akt/mTOR signaling pathway by increasing the expression of p-p85, p-Akt, and p-mTOR and repressing LC3-II expression, and subsequently inhibited autophagy level. As a result, Tan IIA reduced cell death and protected hippocampal neuronal cells from reactive oxygen species. However, it is unknown whether Tan IIA inhibits OGD/R-induced autophagy by inhibiting the PI3K/Akt/mTOR pathway in neural cells. Compared with Tan IIA promoting autophagy for down-regulating the PI3K/Akt/mTOR signaling pathway in glioma cells (Ding et al., 2017), this exciting phenomenon firmly pointed out the possibility that Tan IIA may undergo neuroprotective activities through other pathways. Supportive experiments need to be performed for further investigation. Subsequently, researchers observed that TSN-SS inhibited middle cerebral artery occlusion (MCAO)-induced autophagy by down-regulating associated proteins, such as LC3-II, Beclin-1, and Sirt 6, and significantly reducing the infarct volume and brain water content (Wang et al., 2020). These reports offer valuable information for preventing and treating nervous system disease by using Tan IIA or TSN-SS in regulating autophagy function.
Targeting autophagy for cardiovascular disease
Cardiovascular diseases, also known as circulatory diseases, are mainly caused by the blockage of the heart and blood vessels, featuring high prevalence, high disability, and high mortality (Sun et al., 2020; Goswami et al., 2021). Several pharmacological studies have proven that Tan IIA and its derivatives can treat various cardiovascular diseases by regulating autophagy via AMPK, miR-375/KLF4, MAPK, and other signaling pathways. Atherosclerosis (AS) is one of the leading causes of many cardiovascular diseases, such as coronary heart disease (Libby, 2021). Recent research has revealed that macrophages, another type of autophagy, play a pivotal role in destabilizing atherosclerotic plaques through the lysosome system (Tabas and Bornfeldt, 2016). Jia’s group elucidated that Tan IIA enhanced autophagy by inhibiting the miR-375/KLF4 mediated pathway, resulting in M2 polarization of macrophages and attenuating atherosclerosis. Moreover, increasing evidence indicates that the abnormal proliferation of vascular smooth muscle cells (VSMCs) is involved in the pathogenesis of AS and other cardiovascular diseases (Basatemur et al., 2019). Wang’s group demonstrated for the first time that Tan IIA could inhibit Ang II-induced proliferation and autophagy of VSMCs by down-regulating the p38/MAPK signaling pathway, suggesting that Tan IIA may be a potential novel medication for the treatment of cardiovascular disease that is associated with VSMC dysfunction (Lu et al., 2019).
As the most common complication of myocardial infarction (Musher et al., 2019), heart failure (HF) is highly associated with cardiomyocyte apoptosis (Bravo-San Pedro et al., 2017). Recent studies reveal that autophagy plays a protective role in disposing defective proteins in heart failure (HF) initiated by proteotoxicity. Wang’s group investigated the protective mechanism of Tan IIA on HF and found that Tan IIA increased autophagy and inhibited cardiomyocyte apoptosis by activating the AMPK/mTOR-dependent signaling pathway and up-regulating the expression of LC3 and Beclin-1. As a result, Tan IIA led to the protection of cardiomyocytes and improved cardiac function (Zhang et al., 2019). In addition, maladaptive myocardial remodeling consistently contributes to the poor prognosis of patients following myocardial infarction (MI). Hinek’s group demonstrated that TSN-SS could enhance autophagy through multiple mechanisms, including the up-regulation of Bcl-2, increased ratio of LC3 lipidation (LC3-II/LC3-I), and decreased level of autophagy substrate p62 (Mao et al., 2019). Meanwhile, they found that inhibiting the mTOR/P70S6K and TGFβ1/Smad3 signaling pathways, triggered by AMPK and Sirt-1, significantly reduced pathologic cardiac remodeling. These studies provide insight into the pharmacological mechanism of Tan IIA and offer a potential novel therapeutic strategy for managing MI. Myocardial dysfunction is organ damage caused partly by sepsis (Hsieh et al., 2020), which then initiates the autophagy program to remove dysfunctional or harmful substances. High mobility group box 1 (HMGB1), a late mediator of lethal systemic inflammation, can trigger sepsis when it leaks into the bloodstream (Deng et al., 2022). Wang’s group found that TSN-SS effectively facilitated the internalization of exogenous HMGB1 via active transport through clathrin- and caveolin-dependent endocytosis to LC3-positive cytoplasmic vesicles, such as amphisomes, for subsequent degradation via lysosome-dependent autophagy (Zhang et al., 2012). This discovery suggested that facilitating the return of endocytic HMGB1 to phagocytes may hold potential for the treatment of inflammatory-induced myocardial dysfunction. Myocardial injury is also considered an essential pathological phenomenon caused by sepsis. Mao and coworkers demonstrated that TSN-SS enhanced autophagy via the AMPK/mTOR signaling pathway and decreased NLRP3 inflammasome activation by regulating proinflammatory cytokine secretion Table 1 (Chen et al., 2021).
Summary and outlook
As an ancient and conserved cellular self-regulating biological function, autophagy plays a significant role in disease pathogenesis and progression. As the bioactive ingredient of Salvia miltiorrhiza, one of Asia’s most widely used traditional Chinese medicines, tanshinone has shown a profound effect in alleviating and treating various diseases by regulating autophagy. Table 2 summarizes that tanshinone demonstrates therapeutic effects on human cancer, the nervous system, and cardiovascular diseases. The mechanisms by which tanshinone participates in treating various diseases are diverse and complex. The multiple pharmacological effects of tanshinone mentioned above mainly involve the expression of autophagy-related proteins, such as Beclin-1, LC3-II, P62, ULK1, Bax, ATG3, ATG5, ATG7, ATG9, and ATG12; the regulation of the PI3K/Akt/mTOR, MEK/ERK/mTOR, Beclin-1-related, and AMPK-related signaling pathways; the accumulation of ROS and the activation of AMPK (Figure 2). As a promising drug candidate in cancer treatment, tanshinone exerts corresponding curative effects by promoting tumor cell death and inhibiting tumor cell proliferation. At the same time, it can also be used as a combination or adjuvant drug in cancer treatment, such as enhancing the efficacy of 5-FU and radiosensitization (Su, 2012). Moreover, Tan IIA and TSN-SS mainly exert neuroprotective activities by regulating the PI3K/AKT/mTOR signaling pathway and reducing the expression of autophagy-related proteins to inhibit autophagy functions. Additionally, Tan IIA and TSN-SS show potential in treating cardiovascular diseases by regulating autophagy, inhibiting the proliferation of VSMCs, reducing myocardial pathological remodeling, improving cardiac function, etc.
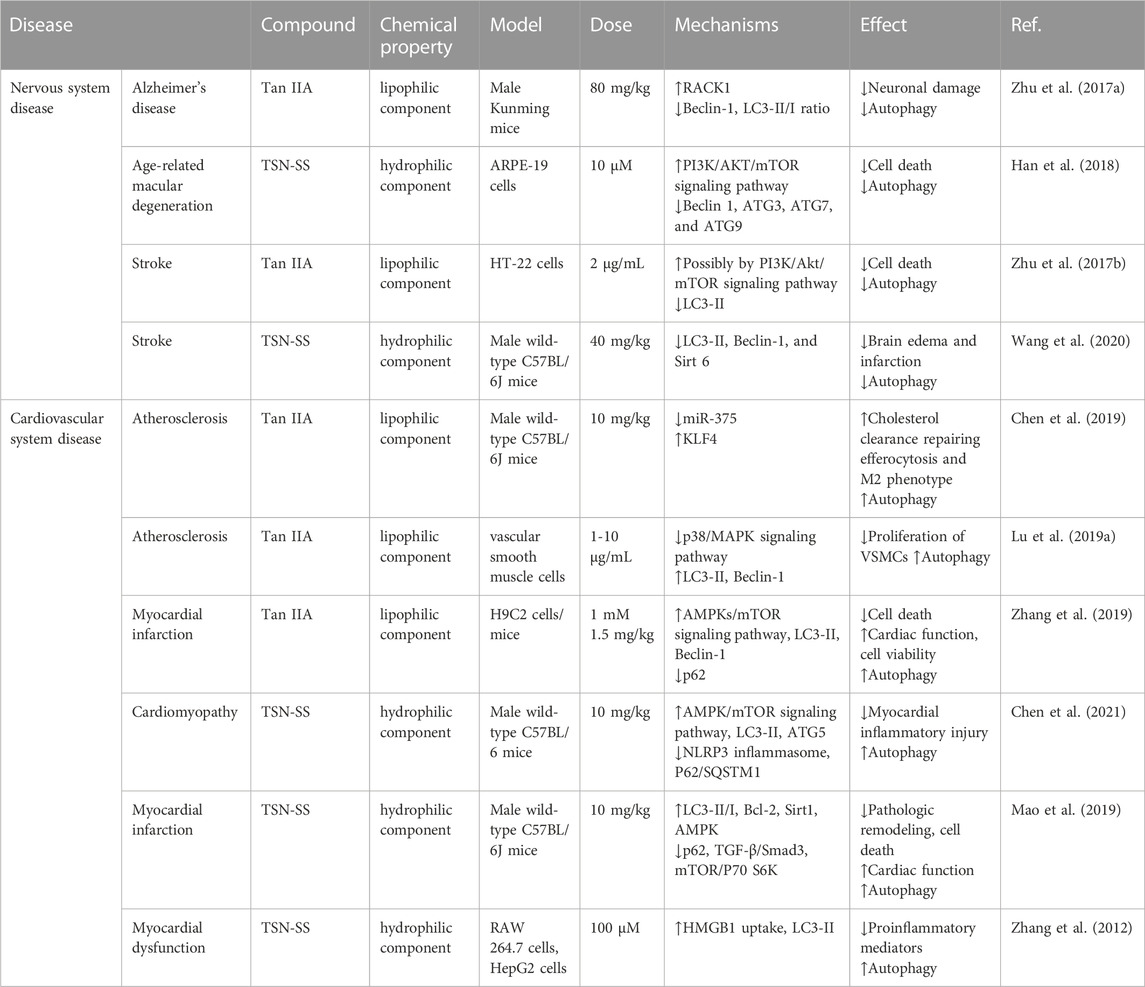
TABLE 2. Detailed information on tanshinone in regulating autophagy in the nervous system and cardiovascular diseases.
According to the literature documented in this review, we are pleased to find that tanshinone has shown pharmacological effects on autophagy-related diseases. Current results have proven that using tanshinone in autophagy as a potential anticancer drug, especially Tan IIA, can be a potential strategy in the clinic. Continuous investments in tanshinone, including sufficient in vivo experiments and further pharmacological research, can benefit the discovery of novel drugs from Salvia miltiorrhiza. In addition, Salvia miltiorrhiza has been used for treating cardiovascular diseases throughout history, and remarkable curative effects concerning its bioactive ingredients have been investigated. Nevertheless, only a few reports have focused on the relationship between cardiovascular diseases and autophagy, suggesting great value and opportunity exist in this promising direction. Notably, we found that autophagy plays a dule role in cancer treatment. On the one hand, the regulation of autophagy by utilizing tanshinone can promote the inhibition of cancer cell growth and death by synergistic effects with apoptosis and cycle arrest. On the other hand, tanshinone promotes cancer cell survival by inducing protective autophagy that antagonizes the effects of apoptosis and other mechanisms. (Gao et al., 2015; Jing et al., 2016; Li et al., 2016; Luo et al., 2020; Jian et al., 2020). Therefore, disrupting the protective autophagy of tanshinone to enhance the anticancer effect is a possible therapeutic strategy, such as using autophagy inhibitors. Interestingly, compared with the monomeric component, total tanshinone shows better efficacy in alleviating cancers, suggesting that a synergistic effect of multiple tanshinone components may play a significant but unknown role in these biological processes (Gao et al., 2015). Sufficient experiments are needed to elucidate its potential mechanism, which is probably related to autophagy.
Moreover, some PI3K and mTOR inhibitors are currently used in antitumor research. Studies have shown that PI3K is widely overactivated in cancer and immune dysregulation and is closely associated with tumorigenesis and progression (Elmenier et al., 2019; Castel et al., 2021). Several PI3K inhibitors, such as buparlisib, copanlisib, alpelisib, duvelisib, and idelalisib, have been shown to produce clinically meaningful benefits in solid tumors and hematologic malignancies, and additional indications and combinations are being investigated (Furman et al., 2014; Baselga et al., 2017; Dreyling et al., 2017; Flinn et al., 2018; Juric et al., 2018; Juric et al., 2019). However, undesirable adverse drug reactions such as rash, hyperglycemia, diarrhea, and nerve damage have negated the clinical value of numerous candidate agents (Geuna et al., 2015; Lampson and Brown, 2017; André et al., 2019). In addition, mTOR is involved in regulating energy and metabolism (Hua et al., 2019). Inhibiting the mTOR pathway and thus curbing tumor growth is one of the classical antitumor strategies (Teng et al., 2019; Zou et al., 2020). However, the negative feedback mechanism of S6 kinase β-1 in this pathway makes the inhibitor-resistant (Zou et al., 2020). Recent strategies have focused on developing dual PI3K and mTOR inhibitors, which nicely circumvent the feedback loop (Wu et al., 2022b). As a potent PI3K/mTOR inhibitor, the excessively rapid metabolism of PI-103 in vivo has hindered its further clinical development. Although these shortcomings have been overcome to some extent, the consequent decrease in efficacy is another hurdle (Luo et al., 2020). Therefore, oncology therapeutic options targeting PI3K and mTOR are still in urgent need of development. Small molecule compounds of natural origin with low toxicity and high-efficiency properties have been a vital source for developing anticancer drugs (Dutta et al., 2019; Talib et al., 2021). This review describes that tanshinone has shown promising efficacy in various cancers through the multifaceted modulation of PI3K and mTOR targets. Notably, little literature has been reported on tanshinone having significant toxic side effects. Therefore, tanshinone is expected to be a powerful addition to the field of developing novel small-molecule inhibitors for the treatment of cancer.
Druggability has always been an inevitable topic in drug discovery (Zhang and Tang, 2018). Tan IIA and other lipid-soluble components have poor water solubility and bioavailability, significantly limiting their utilization as ideal drug candidates. One promising direction is to improve their bioavailability using chemical modification and nano-delivery systems (Aminu et al., 2020). Toxicity is also a critical problem that restricts the clinical application of tanshinone. Although the toxic effects of tanshinone and its derivatives on normal tissues have rarely been reported, sufficient experimental data are needed to evaluate its safety. Moreover, many side effects, such as fever, pain, and anaphylactic shock, occurred after TSN-SS injection, but the detailed mechanism was far behind its clinical application. The relationship between the above symptoms and autophagy may provide an answer (Zhou et al., 2019). Subsequently, conducting extensive and in-depth toxicological research on tanshinone and its derivatives is urgent and necessary to maximize clinical efficacy and avoid safety problems. In brief, we hope this review will shed light on the pharmacological mechanism by which tanshinone regulates autophagy and provide an experimental basis and inspiration for future research on tanshinone and autophagy.
Author contributions
SW and LQ contributed to the conception and design of the study. JW, KN, KZ, and NL organized the database and performed the statistical analysis and figures (by Figdraw). SW wrote the first draft of the manuscript. LQ and LX contributed to the manuscript revision. All authors read and approved the submitted version.
Funding
This work was financially supported by the National Natural Science Foundation of China (81774387 and 82205240).
Conflict of interest
The authors declare that the research was conducted in the absence of any commercial or financial relationships that could be construed as a potential conflict of interest.
Publisher’s note
All claims expressed in this article are solely those of the authors and do not necessarily represent those of their affiliated organizations, or those of the publisher, the editors and the reviewers. Any product that may be evaluated in this article, or claim that may be made by its manufacturer, is not guaranteed or endorsed by the publisher.
References
Amaravadi, R. K., Kimmelman, A. C., and Debnath, J. (2019). Targeting autophagy in cancer: Recent advances and future directions. Cancer Discov. 9 (9), 1167–1181. doi:10.1158/2159-8290.Cd-19-0292
Aminu, N., Bello, I., Umar, N. M., Tanko, N., Aminu, A., and Audu, M. M. (2020). The influence of nanoparticulate drug delivery systems in drug therapy. J. Drug Deliv. Sci. Technol. 60, 101961. doi:10.1016/j.jddst.2020.101961
André, F., Ciruelos, E., Rubovszky, G., Campone, M., Loibl, S., Rugo, H. S., et al. (2019). Alpelisib for PIK3CA-mutated, hormone receptor–positive advanced breast cancer. N. Engl. J. Med. 380 (20), 1929–1940. doi:10.1056/NEJMoa1813904
Ansari, M. A., Khan, F. B., Safdari, H. A., Almatroudi, A., Alzohairy, M. A., Safdari, M., et al. (2021). Prospective therapeutic potential of Tanshinone IIA: An updated overview. Pharmacol. Res. 164, 105364. doi:10.1016/j.phrs.2020.105364
Atanasov, A. G., Zotchev, S. B., Dirsch, V. M., Supuran, C. T., and Int Nat Prod Sci, T. (2021). Natural products in drug discovery: Advances and opportunities. Nat. Rev. Drug Discov. 20 (3), 200–216. doi:10.1038/s41573-020-00114-z
Bao, Y. R., Chen, J. W., Jiang, Y., Wang, L. H., Xue, R., Qian, J. X., et al. (2020). Sodium tanshinone II sulfonate A ameliorates hypoxia-induced pulmonary hypertension. Front. Pharmacol. 11, 687. doi:10.3389/fphar.2020.00687
Basatemur, G. L., Jorgensen, H. F., Clarke, M. C. H., Bennett, M. R., and Mallat, Z. (2019). Vascular smooth muscle cells in atherosclerosis. Nat. Rev. Cardiol. 16 (12), 727–744. doi:10.1038/s41569-019-0227-9
Baselga, J., Im, S. A., Iwata, H., Cortés, J., De Laurentiis, M., Jiang, Z., et al. (2017). Buparlisib plus fulvestrant versus placebo plus fulvestrant in postmenopausal, hormone receptor-positive, HER2-negative, advanced breast cancer (BELLE-2): A randomised, double-blind, placebo-controlled, phase 3 trial. Lancet Oncol. 18 (7), 904–916. doi:10.1016/S1470-2045(17)30376-5
Bravo-San Pedro, J. M., Kroemer, G., and Galluzzi, L. (2017). Autophagy and mitophagy in cardiovascular disease. Circ. Res. 120 (11), 1812–1824. doi:10.1161/circresaha.117.311082
Caesar, L. K., and Cech, N. B. (2019). Synergy and antagonism in natural product extracts: When 1+1 does not equal 2. Nat. Prod. Rep. 36 (6), 869–888. doi:10.1039/c9np00011a
Castel, P., Toska, E., Engelman, J. A., and Scaltriti, M. (2021). The present and future of PI3K inhibitors for cancer therapy. Nat. Cancer 2 (6), 587–597. doi:10.1038/s43018-021-00218-4
Chen, P., An, Q., Huang, Y., Zhang, M., and Mao, S. (2021). Prevention of endotoxin-induced cardiomyopathy using sodium tanshinone IIA sulfonate: Involvement of augmented autophagy and NLRP3 inflammasome suppression. Eur. J. Pharmacol. 909, 174438. doi:10.1016/j.ejphar.2021.174438
Chen, W., Li, X., Guo, S., Song, N., Wang, J., Jia, L., et al. (2019). Tanshinone IIA harmonizes the crosstalk of autophagy and polarization in macrophages via miR-375/KLF4 pathway to attenuate atherosclerosis. Int. Immunopharmacol. 70, 486–497. doi:10.1016/j.intimp.2019.02.054
Chen, W., Ma, Z., Yu, L., Mao, X., Ma, N., Guo, X., et al. (2022). Preclinical investigation of artesunate as a therapeutic agent for hepatocellular carcinoma via impairment of glucosylceramidase-mediated autophagic degradation. Exp. Mol. Med. 54, 1536–1548. doi:10.1038/s12276-022-00780-6
Chen, X., Guo, J., Bao, J., Lu, J., and Wang, Y. (2014). The anticancer properties of Salvia miltiorrhiza bunge (danshen): A systematic review. Med. Res. Rev. 34 (4), 768–794. doi:10.1002/med.21304
Deng, C., Zhao, L., Yang, Z., Shang, J. J., Wang, C. Y., Shen, M. Z., et al. (2022). Targeting HMGB1 for the treatment of sepsis and sepsis-induced organ injury. Acta Pharmacol. Sin. 43 (3), 520–528. doi:10.1038/s41401-021-00676-7
Ding, L., Wang, S., Qu, X., and Wang, J. (2016). Tanshinone IIA sensitizes oral squamous cell carcinoma to radiation due to an enhanced autophagy. Environ. Toxicol. Pharmacol. 46, 264–269. doi:10.1016/j.etap.2016.07.021
Ding, L., Wang, S., Wang, W., Lv, P., Zhao, D., Chen, F., et al. (2017). Tanshinone IIA affects autophagy and apoptosis of glioma cells by inhibiting phosphatidylinositol 3-kinase/akt/mammalian target of rapamycin signaling pathway. Pharmacology 99 (3-4), 188–195. doi:10.1159/000452340
Dreyling, M., Santoro, A., Mollica, L., Leppä, S., Follows, G. A., Lenz, G., et al. (2017). Phosphatidylinositol 3-kinase inhibition by copanlisib in relapsed or refractory indolent lymphoma. J. Clin. Oncol. 35 (35), 3898–3905. doi:10.1200/JCO.2017.75.4648
Dutta, S., Mahalanobish, S., Saha, S., Ghosh, S., and Sil, P. C. (2019). Natural products: An upcoming therapeutic approach to cancer. Food Chem. Toxicol. 128, 240–255. doi:10.1016/j.fct.2019.04.012
El-Khider, F., and McDonald, C. (2016). Links of autophagy dysfunction to inflammatory bowel disease onset. Dig. Dis. 34 (1-2), 27–34. doi:10.1159/000442921
Elmenier, F. M., Lasheen, D. S., and Abouzid, K. A. M. (2019). Phosphatidylinositol 3 kinase (PI3K) inhibitors as new weapon to combat cancer. Eur. J. Med. Chem. 183, 111718. doi:10.1016/j.ejmech.2019.111718
Fan, L., Mao, C., Hu, X., Zhang, S., Yang, Z., Hu, Z., et al. (2020). New insights into the pathogenesis of Alzheimer's disease. Front. Neurol. 10, 1312. doi:10.3389/fneur.2019.01312
Fan, X. Y., Tian, C., Wang, H., Xu, Y., Ren, K., Zhang, B. Y., et al. (2015). Activation of the AMPK-ULK1 pathway plays an important role in autophagy during prion infection. Sci. Rep. 5, 14728. doi:10.1038/srep14728
Fang, Z. Y., Zhang, M., Liu, J. N., Zhao, X., Zhang, Y. Q., and Fang, L. (2021). Tanshinone IIA: A review of its anticancer effects. Front. Pharmacol. 11, 611087. doi:10.3389/fphar.2020.611087
Faubert, B., Vincent, E. E., Poffenberger, M. C., and Jones, R. G. (2015). The AMP-activated protein kinase (AMPK) and cancer: Many faces of a metabolic regulator. Cancer Lett. 356 (2), 165–170. doi:10.1016/j.canlet.2014.01.018
Filomeni, G., De Zio, D., and Cecconi, F. (2015). Oxidative stress and autophagy: The clash between damage and metabolic needs. Cell. Death Differ. 22 (3), 377–388. doi:10.1038/cdd.2014.150
Fleckenstein, M., Keenan, T. D. L., Guymer, R. H., Chakravarthy, U., Schmitz-Valckenberg, S., Klaver, C. C., et al. (2021). Age-related macular degeneration. Nat. Rev. Dis. Prim. 7 (1), 31. doi:10.1038/s41572-021-00265-2
Flinn, I. W., Hillmen, P., Montillo, M., Nagy, Z., Illés, Á., Etienne, G., et al. (2018). The phase 3 DUO trial: Duvelisib vs ofatumumab in relapsed and refractory CLL/SLL. Blood 132 (23), 2446–2455. doi:10.1182/blood-2018-05-850461
Fu, X., Zhao, W., Li, K., Zhou, J., and Chen, X. (2021). Cryptotanshinone inhibits the growth of HCT116 colorectal cancer cells through endoplasmic reticulum stress-mediated autophagy. Front. Pharmacol. 12, 653232. doi:10.3389/fphar.2021.653232
Furman, R. R., Sharman, J. P., Coutre, S. E., Cheson, B. D., Pagel, J. M., Hillmen, P., et al. (2014). Idelalisib and rituximab in relapsed chronic lymphocytic leukemia. N. Engl. J. Med. 370 (11), 997–1007. doi:10.1056/NEJMoa1315226
Galluzzi, L., Bravo-San Pedro, J. M., Demaria, S., Formenti, S. C., and Kroemer, G. (2017). Activating autophagy to potentiate immunogenic chemotherapy and radiation therapy. Nat. Rev. Clin. Oncol. 14 (4), 247–258. doi:10.1038/nrclinonc.2016.183
Gao, H., Sun, W., Zhao, W., Hao, W., Leung, C. H., Lu, J., et al. (2015). Total tanshinones-induced apoptosis and autophagy via reactive oxygen species in lung cancer 95D cells. Am. J. Chin. Med. 43 (6), 1265–1279. doi:10.1142/s0192415x1550072x
Gao, S., Liu, Z., Li, H., Little, P. J., Liu, P., and Xu, S. (2012). Cardiovascular actions and therapeutic potential of tanshinone IIA. Atherosclerosis 220 (1), 3–10. doi:10.1016/j.atherosclerosis.2011.06.041
Geuna, E., Milani, A., Martinello, R., Aversa, C., Valabrega, G., Scaltriti, M., et al. (2015). Buparlisib, an oral pan-PI3K inhibitor for the treatment of breast cancer. Expert Opin. Invest. Drugs 24 (3), 421–431. doi:10.1517/13543784.2015.1008132
Goswami, S. K., Ranjan, P., Dutta, R. K., and Verma, S. K. (2021). Management of inflammation in cardiovascular diseases. Pharmacol. Res. 173, 105912. doi:10.1016/j.phrs.2021.105912
Guo, S., Luo, W., Liu, L., Pang, X., Zhu, H., Liu, A., et al. (2016). Isocryptotanshinone, a STAT3 inhibitor, induces apoptosis and pro-death autophagy in A549 lung cancer cells. J. Drug Target. 24 (10), 934–942. doi:10.3109/1061186x.2016.1157882
Han, D., Wu, X., Liu, L., Shu, W., and Huang, Z. (2018). Sodium tanshinone IIA sulfonate protects ARPE-19 cells against oxidative stress by inhibiting autophagy and apoptosis. Sci. Rep. 8, 15137. doi:10.1038/s41598-018-33552-2
Hsieh, Y. C., Tsou, P. Y., Wang, Y. H., Chao, C. C. T., Lee, W. C., Lee, M. T. G., et al. (2020). Risk factors for myocardial infarction and stroke among sepsis survivors: A competing risks analysis. J. Intensive Care Med. 35 (1), 34–41. doi:10.1177/0885066619844936
Hua, H., Kong, Q., Zhang, H., Wang, J., Luo, T., and Jiang, Y. (2019). Targeting mTOR for cancer therapy. J. Hematol. Oncol. 12 (1), 71. doi:10.1186/s13045-019-0754-1
Jian, S., Liang, C., Lian, M., Che, H., Tang, R., Fan, X., et al. (2020). Tanshinone I induces apoptosis and protective autophagy in human glioblastoma cells via a reactive oxygen species-dependent pathway. Int. J. Mol. Med. 45 (4), 983–992. doi:10.3892/ijmm.2020.4499
Jing, X., Xu, Y., Cheng, W., Guo, S., Zou, Y., and He, L. (2016). Tanshinone I induces apoptosis and pro-survival autophagy in gastric cancers. Cancer Chemother. Pharmacol. 77 (6), 1171–1181. doi:10.1007/s00280-016-3034-6
Ju, Y., and Tam, K. Y. (2022). Pathological mechanisms and therapeutic strategies for Alzheimer's disease. Neural Regen. Res. 17 (3), 543–549. doi:10.4103/1673-5374.320970
Jung, C. H., Ro, S. H., Cao, J., Otto, N. M., and Kim, D. H. (2010). mTOR regulation of autophagy. FEBS Lett. 584 (7), 1287–1295. doi:10.1016/j.febslet.2010.01.017
Juric, D., Janku, F., Rodón, J., Burris, H. A., Mayer, I. A., Schuler, M., et al. (2019). Alpelisib plus fulvestrant in PIK3CA-altered and PIK3CA-wild-type estrogen receptor–positive advanced breast cancer: A phase 1b clinical trial. JAMA Oncol. 5 (2), e184475. doi:10.1001/jamaoncol.2018.4475
Juric, D., Rodon, J., Tabernero, J., Janku, F., Burris, H. A., Schellens, J. H. M., et al. (2018). Phosphatidylinositol 3-kinase α–selective inhibition with alpelisib (BYL719) in PIK3CA-altered solid tumors: Results from the first-in-human study. J. Clin. Oncol. 36 (13), 1291–1299. doi:10.1200/JCO.2017.72.7107
Kaarniranta, K., Uusitalo, H., Blasiak, J., Felszeghy, S., Kannan, R., Kauppinen, A., et al. (2020). Mechanisms of mitochondrial dysfunction and their impact on age-related macular degeneration. Prog. Retin. Eye Res. 79, 100858. doi:10.1016/j.preteyeres.2020.100858
Kauppinen, A., Paterno, J. J., Blasiak, J., Salminen, A., and Kaarniranta, K. (2016). Inflammation and its role in age-related macular degeneration. Cell. Mol. Life Sci. 73 (9), 1765–1786. doi:10.1007/s00018-016-2147-8
Kim, K. H., and Lee, M. S. (2014). Autophagy-a key player in cellular and body metabolism. Nat. Rev. Endocrinol. 10 (6), 322–337. doi:10.1038/nrendo.2014.35
Lampson, B. L., and Brown, J. R. (2017). PI3Kδ-selective and PI3Kα/δ-combinatorial inhibitors in clinical development for B-cell non-Hodgkin lymphoma. Expert Opin. Invest. Drugs 26 (11), 1267–1279. doi:10.1080/13543784.2017.1384815
Lee, J., Sohn, E. J., Yoon, S., Won, G., Kim, C. G., Jung, J. H., et al. (2017). Activation of JNK and IRE1 is critically involved in tanshinone I-induced p62 dependent autophagy in malignant pleural mesothelioma cells: Implication of p62 UBA domain. Oncotarget 8 (15), 25032–25045. doi:10.18632/oncotarget.15336
Li, C., Han, X., Zhang, H., Wu, J., and Li, B. (2016). The interplay between autophagy and apoptosis induced by tanshinone IIA in prostate cancer cells. Tumor Biol. 37 (6), 7667–7674. doi:10.1007/s13277-015-4602-9
Li, Q., Liu, Y., and Sun, M. (2017a). Autophagy and Alzheimer's disease. Cell. Mol. Neurobiol. 37 (3), 377–388. doi:10.1007/s10571-016-0386-8
Li, X., He, S., and Ma, B. (2020). Autophagy and autophagy-related proteins in cancer. Mol. Cancer 19 (1), 12. doi:10.1186/s12943-020-1138-4
Li, X., Li, Z., Li, X., Liu, B., and Liu, Z. (2017b). Mechanisms of Tanshinone II a inhibits malignant melanoma development through blocking autophagy signal transduction in A375 cell. BMC Cancer 17, 357. doi:10.1186/s12885-017-3329-y
Li, Z. M., Xu, S. W., and Liu, P. Q. (2018). Salvia miltiorrhiza burge (danshen): A golden herbal medicine in cardiovascular therapeutics. Acta Pharmacol. Sin. 39 (5), 802–824. doi:10.1038/aps.2017.193
Li, Z., Wang, C., Wang, Z., Zhu, C., Li, J., Sha, T., et al. (2019). Allele-selective lowering of mutant HTT protein by HTT-LC3 linker compounds. Nature 575 (7781), 203–209. doi:10.1038/s41586-019-1722-1
Liang Xiao, H., Kleeman Linda, K., Jiang Hui, H., Gordon, G., Goldman James, E., Berry, G., et al. (1998). Protection against fatal sindbis virus encephalitis by Beclin, a novel bcl-2-interacting protein. J. Virol. 72 (11), 8586–8596. doi:10.1128/JVI.72.11.8586-8596.1998
Libby, P. (2021). The changing landscape of atherosclerosis. Nature 592 (7855), 524–533. doi:10.1038/s41586-021-03392-8
Limpert, A. S., Lambert, L. J., Bakas, N. A., Bata, N., Brun, S. N., Shaw, R. J., et al. (2018). Autophagy in cancer: Regulation by small molecules. Trends Pharmacol. Sci. 39 (12), 1021–1032. doi:10.1016/j.tips.2018.10.004
Liu, X., and Liu, J. (2020). Tanshinone I induces cell apoptosis by reactive oxygen species-mediated endoplasmic reticulum stress and by suppressing p53/DRAM-mediated autophagy in human hepatocellular carcinoma. Artif. Cells, Nanomed., Biotechnol. 48 (1), 488–497. doi:10.1080/21691401.2019.1709862
Lu, J., Shan, J., Liu, N., Ding, Y., and Wang, P. (2019a). Tanshinone IIA can inhibit angiotensin II-induced proliferation and autophagy of vascular smooth muscle cells via regulating the MAPK signaling pathway. Biol. Pharm. Bull. 42 (11), 1783–1788. doi:10.1248/bpb.b19-00053
Lu, L., Hu, W., Tian, Z., Yuan, D., Yi, G., Zhou, Y., et al. (2019b). Developing natural products as potential anti-biofilm agents. Chin. Med. 14, 11. doi:10.1186/s13020-019-0232-2
Luo, L., Zhong, Q., Guo, S., Zhang, C., Zhang, Q., Zheng, S., et al. (2020a). Development of a bioavailable boron-containing PI-103 Bioisostere, PI-103BE. Bioorg. Med. Chem. Lett. 30 (14), 127258. doi:10.1016/j.bmcl.2020.127258
Luo, Y., Song, L., Wang, X., Huang, Y., Liu, Y., Wang, Q., et al. (2020b). Uncovering the mechanisms of cryptotanshinone as a therapeutic agent against hepatocellular carcinoma. Front. Pharmacol. 11, 1264. doi:10.3389/fphar.2020.01264
Lv, C., Zeng, H. W., Wang, J. X., Yuan, X., Zhang, C., Fang, T., et al. (2018). The antitumor natural product tanshinone IIA inhibits protein kinase C and acts synergistically with 17-AAG. Cell. Death Dis. 9, 165. doi:10.1038/s41419-017-0247-5
Ma, K., Zhang, C., Huang, M. Y., Guo, Y. X., and Hu, G. Q. (2016). Crosstalk between Beclin-1-dependent autophagy and caspase-dependent apoptosis induced by tanshinone IIA in human osteosarcoma MG-63 cells. Oncol. Rep. 36 (4), 1807–1818. doi:10.3892/or.2016.5003
Mao, S., Vincent, M., Chen, M., Zhang, M., and Hinek, A. (2019). Exploration of multiple signaling pathways through which sodium tanshinone IIA sulfonate attenuates pathologic remodeling experimental infarction. Front. Pharmacol. 10, 779. doi:10.3389/fphar.2019.00779
Miethke, M., Pieroni, M., Weber, T., Bronstrup, M., Hammann, P., Halby, L., et al. (2021). Towards the sustainable discovery and development of new antibiotics. Nat. Rev. Chem. 5 (10), 726–749. doi:10.1038/s41570-021-00313-1
Mitchell, P., Liew, G., Gopinath, B., and Wong, T. Y. (2018). Age-related macular degeneration. Lancet 392 (10153), 1147–1159. doi:10.1016/s0140-6736(18)31550-2
Mizushima, N. (2018). A brief history of autophagy from cell biology to physiology and disease. Nat. Cell. Biol. 20 (5), 521–527. doi:10.1038/s41556-018-0092-5
Musher, D. M., Abers, M. S., and Corrales-Medina, V. F. (2019). Acute infection and myocardial infarction. N. Engl. J. Med. 380 (2), 171–176. doi:10.1056/NEJMra1808137
Neal, R. D., Sun, F., Emery, J. D., and Callister, M. E. (2019). Lung cancer. BMJ-British Med. J. 365, l1725. doi:10.1136/bmj.l1725
Newman, D. J., and Cragg, G. M. (2020). Natural products as sources of new drugs over the nearly four decades from 01/1981 to 09/2019. J. Nat. Prod. 83 (3), 770–803. doi:10.1021/acs.jnatprod.9b01285
Nixon, R. A. (2013). The role of autophagy in neurodegenerative disease. Nat. Med. 19 (8), 983–997. doi:10.1038/nm.3232
Park, I. J., Yang, W. K., Nam, S. H., Hong, J., Yang, K. R., Kim, J., et al. (2014). Cryptotanshinone induces G1 cell cycle arrest and autophagic cell death by activating the AMP-activated protein kinase signal pathway in HepG2 hepatoma. Apoptosis 19 (4), 615–628. doi:10.1007/s10495-013-0929-0
Parzych, K. R., and Klionsky, D. J. (2014). An overview of autophagy: Morphology, mechanism, and regulation. Antioxid. Redox Signal. 20 (3), 460–473. doi:10.1089/ars.2013.5371
Popova, N. V., and Juecker, M. (2021). The role of mTOR signaling as a therapeutic target in cancer. Int. J. Mol. Sci. 22 (4), 1743. doi:10.3390/ijms22041743
Qian, J., Cao, Y., Zhang, J., Li, L., Wu, J., Wei, G., et al. (2020). Tanshinone IIA induces autophagy in colon cancer cells through MEK/ERK/mTOR pathway. Transl. Cancer Res. 9 (11), 6919–6928. doi:10.21037/tcr-20-1963
Qiu, Y., Li, C., Wang, Q., Zeng, X., and Ji, P. (2018). Tanshinone IIA induces cell death via Beclin-1-dependent autophagy in oral squamous cell carcinoma SCC-9 cell line. Cancer Med. 7 (2), 397–407. doi:10.1002/cam4.1281
Racanelli, A. C., Kikkers, S. A., Choi, A. M. K., and Cloonan, S. M. (2018). Autophagy and inflammation in chronic respiratory disease. Autophagy 14 (2), 221–232. doi:10.1080/15548627.2017.1389823
Rahal, A., Kumar, A., Singh, V., Yadav, B., Tiwari, R., Chakraborty, S., et al. (2014). Oxidative stress, prooxidants, and antioxidants: The interplay. Biomed. Res. Int. 2014, 761264. doi:10.1155/2014/761264
Ren, J., Fu, L., Nile, S. H., Zhang, J., and Kai, G. (2019). Salvia miltiorrhiza in treating cardiovascular diseases: A review on its pharmacological and clinical applications. Front. Pharmacol. 10, 753. doi:10.3389/fphar.2019.00753
Scherlach, K., and Hertweck, C. (2021). Mining and unearthing hidden biosynthetic potential. Nat. Commun. 12 (1), 3864. doi:10.1038/s41467-021-24133-5
Scrivo, A., Bourdenx, M., Pampliega, O., and Cuervo, A. M. (2018). Selective autophagy as a potential therapeutic target for neurodegenerative disorders. Lancet Neurol. 17 (9), 802–815. doi:10.1016/s1474-4422(18)30238-2
Shaito, A., Duong Thi Bich, T., Hoa Thi, P., Thi Hieu Dung, N., Hasan, H., Halabi, S., et al. (2020). Herbal medicine for cardiovascular diseases: Efficacy, mechanisms, and safety. Front. Pharmacol. 11, 422. doi:10.3389/fphar.2020.00422
Shao, M., Ye, C., Bayliss, G., and Zhuang, S. (2021). New insights into the effects of individual Chinese herbal medicines on chronic kidney disease. Front. Pharmacol. 12, 774414. doi:10.3389/fphar.2021.774414
Shi, K., Tian, D. C., Li, Z. G., Ducruet, A. F., Lawton, M. T., and Shi, F. D. (2019a). Global brain inflammation in stroke. Lancet Neurol. 18 (11), 1058–1066. doi:10.1016/s1474-4422(19)30078-x
Shi, M. J., Dong, B. S., Yang, W. N., Su, S. B., and Zhang, H. (2019b). Preventive and therapeutic role of Tanshinone ⅡA in hepatology. Biomed. Pharmacother. 112, 108676. doi:10.1016/j.biopha.2019.108676
Su, C. C. (2012). Tanshinone IIA potentiates the efficacy of 5-FU in Colo205 colon cancer cells in vivo through downregulation of P-gp and LC3-II. Exp. Ther. Med. 3 (3), 555–559. doi:10.3892/etm.2011.441
Su, C. Y., Ming, Q. L., Rahman, K., Han, T., and Qin, L. P. (2015). Salvia miltiorrhiza: Traditional medicinal uses, chemistry, and pharmacology. Chin. J. Nat. Med. 13 (3), 163–182. doi:10.1016/s1875-5364(15)30002-9
Subedi, L., and Gaire, B. P. (2021). Tanshinone IIA: A phytochemical as a promising drug candidate for neurodegenerative diseases. Pharmacol. Res. 169, 105661. doi:10.1016/j.phrs.2021.105661
Sun, Y., Wu, Y., Tang, S., Liu, H., and Jiang, Y. (2020). Sestrin proteins in cardiovascular disease. Clin. Chim. Acta 508, 43–46. doi:10.1016/j.cca.2020.05.013
Tabas, I., and Bornfeldt, K. E. (2016). Macrophage phenotype and function in different stages of atherosclerosis. Circ. Res. 118 (4), 653–667. doi:10.1161/circresaha.115.306256
Talib, W. H., Alsayed, A. R., Barakat, M., Abu-Taha, M. I., and Mahmod, A. I. (2021). Targeting drug chemo-resistance in cancer using natural products. Biomedicines 9 (10), 1353. doi:10.3390/biomedicines9101353
Teng, Q. X., Ashar, Y. V., Gupta, P., Gadee, E., Fan, Y. F., Reznik, S. E., et al. (2019). Revisiting mTOR inhibitors as anticancer agents. Drug Discov. Today 24 (10), 2086–2095. doi:10.1016/j.drudis.2019.05.030
Tran, M., and Reddy, P. H. (2021). Defective autophagy and mitophagy in aging and Alzheimer's disease. Front. Neurosci. 14, 612757. doi:10.3389/fnins.2020.612757
Wang, L., Hu, T., Shen, J., Zhang, L., Chan, R. L. Y., Lu, L., et al. (2015). Dihydrotanshinone I induced apoptosis and autophagy through caspase dependent pathway in colon cancer. Phytomedicine 22 (12), 1079–1087. doi:10.1016/j.phymed.2015.08.009
Wang, L., Xiong, X., Zhang, X., Ye, Y., Jian, Z., Gao, W., et al. (2020). Sodium tanshinone IIA sulfonate protects against cerebral ischemia-reperfusion injury by inhibiting autophagy and inflammation. Neuroscience 441, 46–57. doi:10.1016/j.neuroscience.2020.05.054
White, E., Mehnert, J. M., and Chan, C. S. (2015). Autophagy, metabolism, and cancer. Clin. Cancer Res. 21 (22), 5037–5046. doi:10.1158/1078-0432.Ccr-15-0490
Wu, T., Wang, M. C., Jing, L., Liu, Z. Y., Guo, H., Liu, Y., et al. (2015). Autophagy facilitates lung adenocarcinoma resistance to cisplatin treatment by activation of AMPK/mTOR signaling pathway. Drug Des. devel. Ther. 9, 6421–6431. doi:10.2147/DDDT.S95606
Wu, X., Liu, L., Zheng, Q., Ye, H., Yang, H., Hao, H., et al. (2022a). Dihydrotanshinone I preconditions myocardium against ischemic injury via PKM2 glutathionylation sensitive to ROS. Acta Pharm. Sin. B 5, 0318–0333. doi:10.1016/j.apsb.2022.07.006
Wu, X., Xu, Y., Liang, Q., Yang, X., Huang, J., Wang, J., et al. (2022b). Recent advances in dual PI3K/mTOR inhibitors for tumour treatment. Front. Pharmacol. 13, 875372. doi:10.3389/fphar.2022.875372
Xiang, H., Zhang, J., Lin, C., Zhang, L., Liu, B., and Ouyang, L. (2020). Targeting autophagy-related protein kinases for potential therapeutic purpose. Acta Pharm. Sin. B 10 (4), 569–581. doi:10.1016/j.apsb.2019.10.003
Xie, Q., Chen, Y., Tan, H., Liu, B., Zheng, L. L., and Mu, Y. (2021). Targeting autophagy with natural compounds in cancer: A renewed perspective from molecular mechanisms to targeted therapy. Front. Pharmacol. 12, 748149. doi:10.3389/fphar.2021.748149
Xu, Z., Chen, L., Xiao, Z., Zhu, Y., Jiang, H., Jin, Y., et al. (2018). Potentiation of the anticancer effect of doxorubicinin drug-resistant gastric cancer cells by tanshinone IIA. Phytomedicine 51, 58–67. doi:10.1016/j.phymed.2018.05.012
Xu, Z., Jiang, H., Zhu, Y., Wang, H., Jiang, J., Chen, L., et al. (2017). Cryptotanshinone induces ROS-dependent autophagy in multidrug-resistant colon cancer cells. Chem. Biol. Interact. 273, 48–55. doi:10.1016/j.cbi.2017.06.003
Yan, R., and Vassar, R. (2014). Targeting the beta secretase BACE1 for Alzheimer's disease therapy. Lancet Neurol. 13 (3), 319–329. doi:10.1016/s1474-4422(13)70276-x
Yen, J. H., Huang, S. T., Huang, H. S., Fong, Y. C., Wu, Y. Y., Chiang, J. H., et al. (2018). HGK-sestrin 2 signaling-mediated autophagy contributes to antitumor efficacy of Tanshinone IIA in human osteosarcoma cells. Cell. Death Dis. 9, 1003. doi:10.1038/s41419-018-1016-9
Yim, W. W. Y., and Mizushima, N. (2020). Lysosome biology in autophagy. Cell. Discov. 6 (1), 6. doi:10.1038/s41421-020-0141-7
Yuan, H., Ma, Q., Ye, L., and Piao, G. (2016). The traditional medicine and modern medicine from natural products. Molecules 21 (5), 559. doi:10.3390/molecules21050559
Yuan, J., Dong, X., Yap, J., and Hu, J. (2020). The MAPK and AMPK signalings: Interplay and implication in targeted cancer therapy. J. Hematol. Oncol. 13 (1), 113. doi:10.1186/s13045-020-00949-4
Yun, C. W., Jeon, J., Go, G., Lee, J. H., and Lee, S. H. (2021). The dual role of autophagy in cancer development and a therapeutic strategy for cancer by targeting autophagy. Int. J. Mol. Sci. 22 (1), 179. doi:10.3390/ijms22010179
Zhang, X., Wang, Q., Wang, X., Chen, X., Shao, M., Zhang, Q., et al. (2019). Tanshinone IIA protects against heart failure post-myocardial infarction via AMPKs/mTOR-dependent autophagy pathway. Biomed. Pharmacother. 112, 108599. doi:10.1016/j.biopha.2019.108599
Zhang, Y., Li, W., Zhu, S., Jundoria, A., Li, J., Yang, H., et al. (2012). Tanshinone IIA sodium sulfonate facilitates endocytic HMGB1 uptake. Biochem. Pharmacol. 84 (11), 1492–1500. doi:10.1016/j.bcp.2012.09.015
Zhang, Y., Sowers, J. R., and Ren, J. (2018). Targeting autophagy in obesity: From pathophysiology to management. Nat. Rev. Endocrinol. 14 (6), 356–376. doi:10.1038/s41574-018-0009-1
Zhang, Z., and Tang, W. (2018). Drug metabolism in drug discovery and development. Acta Pharm. Sin. B 8 (5), 721–732. doi:10.1016/j.apsb.2018.04.003
Zheng, L., Zhang, Y., Liu, G., Cheng, S., Zhang, G., An, C., et al. (2020). Tanshinone I regulates autophagic signaling via the activation of AMP-activated protein kinase in cancer cells. Anti-Cancer Drugs 31 (6), 601–608. doi:10.1097/cad.0000000000000908
Zhou, H., Zhao, Y., Peng, W., Han, W., Wang, Z., Ren, X., et al. (2021). Effect of sodium tanshinone IIA sulfonate injection on blood lipid in patients with coronary heart disease: A systematic review and meta-analysis of randomized clinical trials. Front. Cardiovasc. Med. 8, 770746. doi:10.3389/fcvm.2021.770746
Zhou, J., Jiang, Y. Y., Chen, H., Wu, Y. C., and Zhang, L. (2020). Tanshinone I attenuates the malignant biological properties of ovarian cancer by inducing apoptosis and autophagy via the inactivation of PI3K/AKT/mTOR pathway. Cell. Prolif. 53 (2), e12739. doi:10.1111/cpr.12739
Zhou, Z. Y., Zhao, W. R., Zhang, J., Chen, X. L., and Tang, J. Y. (2019). Sodium tanshinone IIA sulfonate: A review of pharmacological activity and pharmacokinetics. Biomed. Pharmacother. 118, 109362. doi:10.1016/j.biopha.2019.109362
Zhu, J., Liao, S., Zhou, L., and Wan, L. (2017a). Tanshinone IIA attenuates A(25-35)-induced spatial memory impairment via upregulating receptors for activated C kinase1 and inhibiting autophagy in hippocampus. J. Pharm. Pharmacol. 69 (2), 191–201. doi:10.1111/jphp.12650
Zhu, Y., Tang, Q., Wang, G., and Han, R. (2017b). Tanshinone IIA protects hippocampal neuronal cells from reactive oxygen species through changes in autophagy and activation of phosphatidylinositol 3-kinase, protein kinas B, and mechanistic target of rapamycin pathways. Curr. Neurovasc. Res. 14 (2), 132–140. doi:10.2174/1567202614666170306105315
Keywords: tanshinone, Salvia miltiorrhiza, autophagy, human cancers, traditional Chinese medicine, medicinal research
Citation: Wu S, Zhao K, Wang J, Liu N, Nie K, Qi L and Xia L (2023) Recent advances of tanshinone in regulating autophagy for medicinal research. Front. Pharmacol. 13:1059360. doi: 10.3389/fphar.2022.1059360
Received: 01 October 2022; Accepted: 19 December 2022;
Published: 12 January 2023.
Edited by:
Yu Chiang Hung, Kaohsiung Chang Gung Memorial Hospital, TaiwanReviewed by:
King-Ho Cheung, Hong Kong Baptist University, Hong Kong SAR, ChinaBing Han, Zhejiang Chinese Medical University, China
Copyright © 2023 Wu, Zhao, Wang, Liu, Nie, Qi and Xia. This is an open-access article distributed under the terms of the Creative Commons Attribution License (CC BY). The use, distribution or reproduction in other forums is permitted, provided the original author(s) and the copyright owner(s) are credited and that the original publication in this journal is cited, in accordance with accepted academic practice. No use, distribution or reproduction is permitted which does not comply with these terms.
*Correspondence: Luming Qi, bG1xaV90Y21AMTI2LmNvbQ==; Lina Xia, eGlhbGluYUBjZHV0Y20uZWR1LmNu