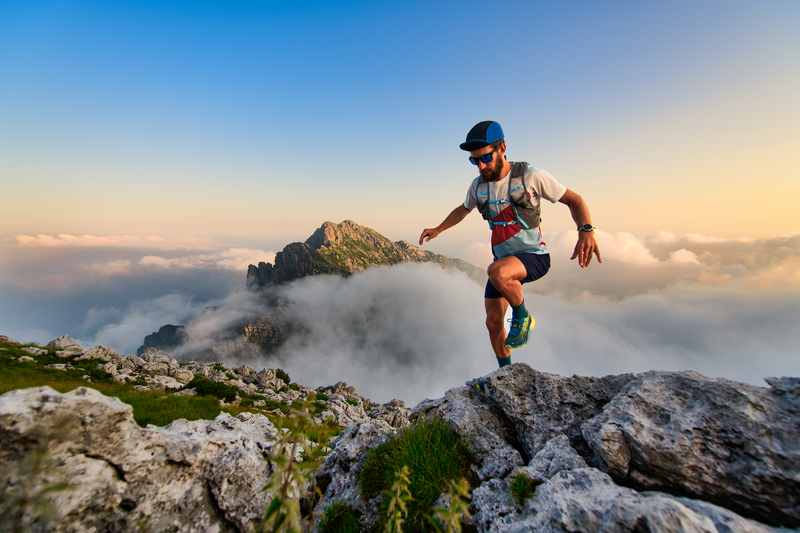
95% of researchers rate our articles as excellent or good
Learn more about the work of our research integrity team to safeguard the quality of each article we publish.
Find out more
ORIGINAL RESEARCH article
Front. Pharmacol. , 14 November 2022
Sec. Gastrointestinal and Hepatic Pharmacology
Volume 13 - 2022 | https://doi.org/10.3389/fphar.2022.1043527
This article is part of the Research Topic Gut Microbiota-Mediated Natural Products Metabolism and Its Role in the Pharmacokinetics and Pharmacodynamics View all 3 articles
Gut microbiota has been reported to be closely associated with Type-II diabetes. Restoration of disordered gut microbiota ecosystem has been developed into a therapeutic strategy and gradually applied on Type-II diabetes treatment with both western drugs and herbal polysaccharides. Although Astragalus membranaceus polysaccharides (AMP) have also been used to treat Type-II diabetes, no study investigated correlations between gut microbiota regulation and its hypoglycemic effect. In the present study, the role of gut microbiota on the hypoglycemic effect of AMP in db/db mice was investigated for the first time. Sixteen days treatment of AMP at the dosage of 600 mg/kg in db/db mice not only alleviated its diabetic symptoms significantly but also restored its gut microbiota community with increased production of fecal short chain fatty acids (SCFA). Our further Pearson correlation analyses revealed that the relative abundance of two intestinal bacteria, Akkermansia and Faecalibaculum, were significantly positively correlated with the hypoglycemic effect of AMP as well as fecal SCFA production. It was also noted that treatment of AMP resulted in increased secretion of glucagon-like peptide-1 (GLP-1) in serum and enhanced intestinal integrity. Further mechanistic study revealed that the increased SCFA after AMP treatment could stimulate GLP-1 secretion and improve intestinal integrity via enhancing the expression of G protein-coupled receptors 41/43 and tight junction proteins (Occudin and ZO-1), respectively, leading to the alleviation of diabetic symptoms in db/db mice.
Dysbiosis of gut microorganism species was found to be associated with metabolic diseases such as diabetes, hyperlipidemia and hypertension (Jose et al., 2012). In recent years, an increasing number of clinical evidence showed that the dysbiosis of gut microbiota is closely related with T2D occurrence (Gurung et al., 2020). It was found that the abundance of producing-butyrate bacteria was decreased while that of reducing-sulphate bacteria was increased in T2D patients in comparison with those from healthy subject (Wang et al., 2012). Another clinical research also reported to observe the compositional and functional alteration of gut microbiota with the increased abundance of Streptococcus and decreased abundance of Clostridiales in T2D patients (Fredrik et al., 2013). Notably, the restoration of disordered gut microbiota ecosystem has been developed into a therapeutic strategy for T2D treatment in clinical practice (Adak and Khan, 2019).
Polysaccharides, as the bioactive macromolecular constituents of many medicinal herbs, have been broadly used in the therapy of T2D (Zheng et al., 2019). A growing number of preclinical studies demonstrated that certain herbal polysaccharides exhibited the antidiabetic effect via regulating the disordered gut microbiota community (Hu et al., 2018; Wu et al., 2019; Ma et al., 2022). Ginseng polysaccharides was observed to decrease the fasting blood glucose significantly and restore the dysbiosis of gut microbiota system by decreasing the abundance of Proteobacteria (Li et al., 2018). Dendrobium polysaccharides were also reported to be able to restore the disordered gut microbiota community by increasing the abundance of bacteria that could produce branched amino acids (Chen et al., 2021).
Recently, Astragalus membranaceus (Chao et al., 2009; Ko et al., 2014) and its polysaccharides fraction (Zheng et al., 2020) have also been reported to possess various kinds of biological activities including hypoglycemic effect. Although a few studies reporting that multiple pharmacological pathways may be involved in the hypoglycemic effect of Astragalus membranaceus polysaccharides (AMP) such as regulating GLUT-4 signal pathway (Liu et al., 2010) and inhibiting the expression of protein tyrosine phosphatase 1B (Mao et al., 2009). To the best of our knowledge, no studies investigated the role of gut microbiota in the hypoglycemic effect of AMP. Since gut microbiota has been reported to be associated with the hypoglycemic effect of herbal polysaccharides, it was hypothesized that gut microbiota might play an important role on the hypoglycemic effect of AMP. The present study is proposed aiming to investigate the role of gut microbiota in the hypoglycemic effect of AMP as well as its underlying mechanisms.
The dry roots of Astragalus membranaceus originated from Gansu, P. R. China was purchased from Fuming Dispensary (Tai Wai, Hong Kong, P. R. China). The voucher specimens of the herb were authenticated by Dr. Lau Tai Wai and kept in the Li Dak Sum Yip Yio Chin R&D Centre for Chinese Medicine at The Chinese University of Hong Kong.
Glucose, rhamnose, mannose, arabinose, galactose, xylose, glucuronic acid, galacturonic acid, 1-phenyl-3-methyl-5-pyrazolone were purchased from Chroma Biotech Co., Ltd. (Chengdu, China). Acetic acid, propanoic acid, butyric acid and DPP4 inhibitor were purchased from Sigma-Aldrich (Saint Louis, MO, United States). Trimethyl acetic acid was purchased from Thermofisher (Waltham, MA, United States). Dextran standards (MW: 1200 Da, 4300 Da, 37600 Da, 121500 Da, 618300 Da, 2556000 Da) were purchased from American Polymer Standards Co., Ltd. (Mentor, OH, United States). Metformin was purchased from Meilun Biotech Co., Ltd. (Dalian, China). The purity of all reagents was more than 99%. Ethanol, chloroform, and methanol were all in HPLC grade and obtained from Merck KGaA (Darmstadt, Germany). Distilled water was supplied by a Millipore water purification system (Milford, MA, United States).
The dry roots of Astragalus membranaceus (1.2 kg) were cut into slices and soaked into 95% ethanol (3 L) for 3 days to remove the fat followed by air-drying and extracting with water (18 L) for 3 h under 100°C in round bottom flask connected with a reflux condenser. The extraction was repeated for three times and the resulted extraction mixture was combined and centrifuged at 4500 rpm for 20 min under room temperature. The obtained supernatant was concentrated to 2.5 L under reduced pressure followed by addition of 10 L ethanol. The mixture solution was stood still at 4°C overnight and centrifuged (4500 rpm, 20 min) to obtain the pellet, which was re-dissolved into water (3.5 L) and dialyzed in the dialysis bag (cutting-off molecular weight: 3500 Da) against running water for 2 days. The resulted solution was lyophilized to obtain Astragalus membranaceus polysaccharides (AMP) by a Freezone freeze dryer (Labconco, MO, United States)
The total sugar content was measured by anthrone-sulfuric acid method with glucose was selected as standard to plot the calibration curve according to the previous report (Leyva et al., 2008). In addition, the total protein residue content of the prepared AMP was measured by Bradford method with bovine serum albumin as standard as described before (Fu et al., 2019).
The monosaccharide composition of AMP was analyzed after 1-phenyl-3-methyl-5-pyrazolone (PMP) derivatization by HPLC method with modifications (Kuang et al., 2020). Briefly, 2 mg AMP sample was hydrolyzed with 1 ml trifluoroacetic acid (TFA, 2 mol/L) in a sealed tube at 100°C for 6 h. The mixture solution was evaporated to dryness and the hydrolysate was re-dissolved in 2 ml of distilled water for subsequent derivatization reaction with PMP. Briefly, about 100 μL hydrolysate solution (1 mg/ml) or standard monosaccharide solution (1 mg/ml) was mixed with 100 μL NaOH solution (0.6 mol/L) and 200 μL PMP solution (0.5 mol/L in methanol), followed by reacting at 70°C for 2 h and subsequent neutralization via addition of 100 μL HCl solution (0.6 mol/L) and 1 ml distilled water. After extracting the resulting mixture with 1.5 ml chloroform for five times, the aqueous layers were combined and filtered through 0.22 μm filter prior to HPLC analyses. The chromatographic analysis was carried out with an Agilent 1260 HPLC system (Santa Clara, United States) equipped with a diode array detector set at wavelength of 250 nm. The chromatographic separation was achieved with a BPS HYPERSIL C18 column (5 μm, 250 × 4.6 mm, Thermofisher) and a mobile phase consisting of acetonitrile and 0.1 mol/L phosphate buffer (pH = 6.85) (15.5:84.5, v/v).
The AMP sample or dextran standards with different molecular weight (MW: 1200 Da, 4300 Da, 37600 Da, 121500 Da, 618300 Da and 2556000 Da) was dissolved in the deionized water to prepare an aqueous solution of 10 mg/ml followed by filtering through 0.45 μm filter. The filtrate was injected into a thermofisher U-3000 HPLC system equipped with a charged aerosol detector (Thermofisher, MA, United States). The molecular weight distribution was analyzed with a TSK GMPWXL gel permeation column (7.8 × 300 mm, Tosoh Bio-science, Tokyo, Japan) and a mobile phase of 20 mM ammonium acetate at the flow rate of 0.6 ml/min as described previously (Xu et al., 2014).
About 2 mg of AMP sample was mixed with potassium bromide (150 mg) completely in the dry environment followed by detection with a Fourier transform infrared (FT-IR) spectrometer at a scanning range of 4000–550 cm−1 according to the previous method (Kuang et al., 2020).
Male db/db mice (BKS.Cg-Dock7m+/+Lepdb/J, n = 24, 6-week-old) and wild type C57BL/6J mice (6-week-old, n = 8) were purchased from Laboratory Animal Services Center of The Chinese University of Hong Kong. Animals were housed in the cages with free access to food and distilled water under the controlled environment (23°C–27°C; 45–55% humidity; 12 h light-dark cycle). The animal experimental protocol was approved by Animal Ethics Committee of The Chinese University of Hong Kong (Ref. No: 22/008/MIS).
After an acclimatization for 1 week, animals were randomly divided into four groups (n = 8 for each group) including wild type normal group (WT), diabetic control group (db/db), metformin group (MET) and Astragalus membranaceus polysaccharides group (AMP). Mice from WT group and db/db group were treated with 0.2 ml distilled water. Mice from MET group and AMP group received 250 mg/kg metformin and 600 mg/kg AMP, respectively. All the mice were treated by oral gavage once daily for consecutive 16 days with daily body weight monitoring.
On Day 13, after 4 h fasting, the insulin tolerance test (iTT) was conducted according to previously reported method (Ángela and Herminia, 2015). All mice were intraperitoneally injected with human insulin at the dose of 0.75 IU/kg and the blood drop was collected from tail vein at different timepoints (0, 30, 60 and 90 min) for measuring blood glucose level by a glucometer (Bayer, Germany). After an overnight fasting on Day 14, the blood was collected via tail vein into a tube without anticoagulants on Day 15 for fasting serum insulin level measurement by a commercial mouse insulin kit (Millipore, Darmstadt, Germany). Subsequently, intraperitoneal glucose tolerance test (ipGTT) was conducted by injecting glucose (0.3 g/kg) with analyzing the blood glucose level before and 30, 60, 90, 120 min post injection by a glucometer (Bayer, Germany) as reported before (Ángela and Herminia, 2015). On Day 16, fresh feces from all the mice were collected into sterile tubes before dosing and stored at -80°C till analysis. On Day 17, after fasting for 4 h, all the animals were orally administered with glucose (1.5 g/kg) and anesthetized at 30 min post administration, followed by withdrawing the whole blood into a tube (including 5 mM DPP4 inhibitor but without anticoagulants) for GLP-1 and cytokine measurement and being sacrificed to harvest the small intestine and colon to store at -80°C till further analysis.
The levels of TNF-α, IL-6, IL-1β, IFN-γ, and GLP-1 in the serum were measured with the corresponding commercial ELISA kits (Invitrogen, MA, United States) according to the manufacturer’s protocol.
The collected small intestine and colon tissues (about 0.1 g) were homogenized in RIPA lysis buffer solution (Bio-Rad Lab, Hercules, CA, United States). After centrifugation for 20 min at 15000 rpm, the obtained supernatant was mixed with Laemmli sample loading buffer, followed by loading on 7.5–12% SDS-PAGE gels for electrophoresis. Then the proteins in the gel were transferred into PVDF membrane (Bio-Rad Lab, Hercules, CA, United States). After blocking with 5% bovine serum albumin, the PVDF membranes were incubated with anti-Occludin antibody (1:1000, Thermofisher, Cat. No: 711500), anti-ZO-1 antibody (1:500, Thermofisher, Cat. No: PA585256), anti-GPCR41 antibody (1:500, Thermofisher, Cat. No: PA575521), anti-GPCR43 antibody (1:300, Thermofisher, Cat. No: PA5111780), with the GAPDH as the internal control (1:10000, Abcam, Cat. No: ab181602) overnight at 4°C. The protein bands were detected by incubating with HRP-conjugated secondary antibody (1:1000, Abcam, Cat. No: ab97051) at room temperature for 1 h and quantified by ImageJ software.
The collected fecal sample (100 mg) was homogenized in methanol (1 ml) by vigorous vortex for 3 min and sonicated for 10 min. The mixture was then centrifuged at 4°C (14000 rpm, 5 min) to obtain the supernatant, which was further diluted by 5-folds with methanol. The diluted supernatant (0.5 ml) was mixed with trimethyl acetic acid (internal standard, 5 μg/ml) solution (0.5 ml) and followed by injecting the mixture (1 μL) into Shimadzu QP2010 GC-MS system (Tokyo, Japan) for analysis. The analysis was carried out on an Agilent J & W fused silica capillary column (0.25 μm, 30 m) and the instrument parameters were set according to the reported method with modifications (Tsang et al., 2018). Briefly, the transfer line temperature and ion source temperature were 230°C and 200°C, respectively. High purity helium served as the carrier gas. The ionization mode was electron impact, with the electron energy of -70 eV and scanning time of 0.5 s. Mass fragments (m/z) in the range of 10–650 were monitored and identified in NIST Mass Spectral Library.
Bacterial genomic DNA was extracted from the collected fecal samples (180–220 g feces/each sample, stored at -80°C) by using the QIAamp® PowerFecal® Pro DNA Kit (QIAGEN, Hilden, Germany). 16S ribosomal RNA (rRNA) amplicon sequencing method was employed to amplify and analyze the gut microbiota composition in collected fecal samples. The V3-V4 regions were amplified by polymerase chain reaction (PCR) with the primers 341F (CCTAYGGGRBGCASCAG) and 806R (GGACTACNNGGGTATCTAAT) as we described before (Lyu et al., 2021). Sequencing libraries were generated on an Illumina platform. Paired-end sequencing were merged and filtered on Fastp software to obtain the effective tags. The effective tags were analyzed and conducted denoise with DADA2 method in QIIME2 software. The sequences with less than 5 units were filtered out to obtain the final Amplicon Sequence Variables (ASV) and the ASV dataset was analyzed in QIIME2 software to obtain species annotation and calculate the bacteria diversity.
The insulin resistance was evaluated by the homeostatic model assessment of insulin resistance (HOMA-IR) index, which was calculated as follows:
Statistical analyses were performed using GraphPad Prism 5.01 software (GraphPad Software, Inc., CA, United States). One-way analysis of variance (ANOVA) with Turkey’s test was employed for multiple group comparisons. In addition, Pearson correlation analyses between relative abundance of gut microbiota and hypoglycemic effect index (ipGTT test result, HOMA-IR index, and fasting glucose level) or total SCFA in mice from WT, db/db and AMP groups were conducted with R software package (Version 4.1.1). Data was present as mean ± standard error of mean.
The lyophilized AMP was a light-yellow powder with the extraction yield of 2.05% (w/w). The total sugar content and protein content of AMP were 71.82 ± 3.94% and 1.97 ± 0.43% (w/w, n = 3), respectively, indicating that carbohydrates were the main composition of the prepared AMP sample.
The monosaccharide composition analysis of our prepared AMP (Figure 1A) indicated that it was comprised of mannose, rhamnose, galacturonic acid, glucose, galactose, and arabinose, at their molar ratio of 0.12:0.16:1.00:1.06:0.73: 2.33. The HPGPC chromatogram of AMP (Figure 1B) showed four peaks, suggesting the average molecular weight of AMP were 3.7 kDa, 59.3 kDa, 274.5 kDa, and 900.7 kDa, respectively. FT-IR spectrum of AMP shown in Figure 1C suggested the following signal assignment: the signal at 3422.93 cm−1 was assigned to the stretching vibration bond of γ-hydroxyl group (Haxaire et al., 2003); the signal at 2932.72 cm−1 was assigned to the stretching vibration bond of γ-methylene group (Haxaire et al., 2003); the signal at 1741.48 cm−1 indicated the presence of carbonyl group (Zhou et al., 2018); the signal at 1636.51 cm−1 was the characteristic peak of water; the signal at 1419.25 cm−1 and 1384.31 cm−1 were assigned to the bending vibration bond of δ-methylene group (Liu et al., 2019); the signal at 1240.61 cm−1 was the stretching vibration bond of γ-C-O-C (Haxaire et al., 2003); the multiple signals around 1101.73 cm−1 indicated the presence of C-O (Haxaire et al., 2003) and the signal at 1021.28 cm−1 was the characteristic peak of pyranose ring (Liu et al., 2019).
FIGURE 1. Characterizations of AMP with monosaccharide composition analysis (A), molecular weight distribution (B) and FT-IR spectrometry (C).
As shown in Figure 2A, compared with the WT group (4.9 ± 0.1 mmol/L), mice from db/db group exhibited significantly higher fasting blood glucose level (18.9 ± 0.7 mmol/L), which indicated the obvious diabetic symptom. Compared among the db/db mice received different treatment, it was noticed that both AMP group (11.0 ± 0.9 mmol/L) and MET group (10.8 ± 1.0 mmol/L) exhibited significantly lower fasting blood glucose level. Similar trend was observed in the intraperitoneal glucose tolerance test as well (Figure 2B), db/db group (2915.1 ± 105.1 mmol/L*min) exhibited significantly higher blood glucose level compared with that of WT group (745.3 ± 16.9 mmol/L*min), after treatment with AMP or MET, the ipGTT result of db/db mice from AMP group (2039.0 ± 121.5 mmol/L*min) and MET group (2221.7 ± 88.0 mmol/L*min) were observed to decline significantly compared with that of db/db group. In the insulin tolerance test (Figure 2C), db/db group (2110.2 ± 105.1 mmol/L*min) exhibited significantly higher blood glucose level compared with that of WT group (480.0 ± 16.9 mmol/L*min). However, MET group (1698.8 ± 88.0 mmol/L*min) and AMP group (1396.7 ± 121.5 mmol/L*min) showed significantly lower level compared with that of db/db group. The fasting insulin measurement (Figure 2D) indicated that db/db group (130.40 ± 12.54 mIU/L) exhibited significantly higher insulin level compared with that of WT group (14.76 ± 1.74 mIU/L). After treatment with MET or AMP, the fasting insulin level of db/db mice from MET group (72.50 ± 6.04 mIU/L) and AMP group (89.18 ± 6.84 mIU/L) both significantly decreased compared with that from db/db group.
FIGURE 2. Evaluation of the hypoglycemic effect of AMP via monitoring of Fasting blood glucose level (A), iTT test (B), ipGTT test (C), fasting insulin level (D), and HOMA-IR index (E) in diabetic mice under different treatment (n = 8); #: p <0.05, ##: p <0.01, ###: p < 0.001 compared with WT group; *: p <0.05, **: p <0.01, ***: p <0.001 compared with db/db group.
The calculated HOMA-IR index was the golden standard to evaluate the degree of insulin resistance. As shown in Figure 2E, it was observed that AMP group (43.4 ± 4.9) exhibited significantly lower HOMA-IR index compared with db/db group (99.03 ± 9.1), suggesting that AMP could significantly ameliorate the insulin resistance of db/db mice.
As shown in Figure 3A, the Glucagon-like peptide-1 (GLP-1) level in the serum from WT group was significantly higher than that from db/db group. After treatment with AMP or MET, both AMP group and MET group exhibited significantly higher GLP-1 level compared with db/db group. In addition, G protein-coupled receptor 41/43 (GPCR 41/43), as the signal proteins which may be involved in the GLP-1 secretion, whose expression in the colon tissue was evaluated as well. As expected, the GPCR 41/43 expression in the WT group were significantly higher than that of db/db group. GPCR 41/43 in the AMP and MET group both exhibited significantly higher expression compared with db/db group. Notably, the GPCR 43 expression in AMP group was significantly higher than that of MET group, but no significant difference was observed on GPCR 41 expression between the two groups.
FIGURE 3. Increase of GLP-1 secretion and inhibition of serum inflammatory level by AMP via promoting the GPCR 41/43 expression in colon (A) and expressions of Occludin and ZO-1 in small intestine (B), respectively. #: p <0.05, ##: p <0.01, ###: p <0.001 compared with WT group; *: p <0.05, **: p <0.01, ***: p <0.001 compared with db/db group.
As shown in Figure 3B, both AMP and MET can significantly decrease the IL-6 level compared with db/db group. Furthermore, AMP also could significantly increase the IFN-ϒ level compared with db/db group. Notably, both AMP group and MET group exhibited no significant difference on the IL-1β and TNF-α expression compared with db/db group. In addition, the expression of some tight junction proteins in the small intestine was evaluated as well. It was observed that db/db group exhibited significantly lower level on both ZO-1 and Occludin expression compared with WT group. However, after treatment with AMP or MET, both AMP group and MET group can significantly increase the ZO-1 and Occludin expression, which meant AMP and MET might improve the intestinal integrity and decrease the inflammation level of db/db mice.
In the present study, trimethylacetic acid was selected as the internal standard for GC-MS analyses of SCFA due to its similar physiochemical properties and ideal retention time (Figure 4A). As shown in Figures 4B–D, it was observed that WT group exhibited significantly higher level on acetic acid and butyric acid, but with no significant difference on propanoic acid level, compared with db/db group. Furthermore, both AMP group and MET group exhibited significantly higher value on all the three SCFA level compared with the db/db group. Notably, AMP group exhibited significantly higher value (p <0.001) on acetic acid level compared with WT group or MET group. Besides acetic acid level, AMP group also exhibited significantly higher value (p <0.05) on butyric acid level compared with WT group or MET group. Collectively, AMP can significantly stimulate the generation of SFCA in the feces from db/db mice.
FIGURE 4. Comparison of fecal SCFA from db/db mice with different treatment (n = 8) as shown in the GC-MS chromatogram of SCFA extracted from feces (A), acetic acid level (B); propionic acid level (C) and butyric acid level (D). #: p <0.05, ##: p <0.01, ###: p <0.001 compared with WT group; *: p <0.05, **: p <0.01, ***: p <0.001 compared with db/db group.
The richness and diversity of gut microbiota community in different groups were evaluated by biodiversity analysis as shown in Figures 5A–D Db/db group showed significant decline on the richness and diversity compared with WT group. After treatment with AMP or MET, Simpson index and Shannon index in both MET and AMP group were significantly higher than that of db/db group. Meanwhile, Chao1 index, Observed OTUs in MET and AMP group had no significant differences compared with db/db group, which meant MET and AMP could significantly improve the diversity of gut microbiota community in db/db mice but with no significant difference on the richness. Secondly, the relative abundance of intestinal bacteria at the phylum level (Figure 6A) was analyzed and it indicated that the Bacteroidota abundance in db/db group was lower than that in WT group. The heatmap of intestinal bacteria at the genus level (Figure 6B) also exhibited that the bacterial composition in each group were different. After treatment with AMP, the bacterial distribution tendency in db/db mice was reversed and became more like that of normal mice in some degree. In addition, the Venn diagram (Figure 7A) indicated that db/db group exhibited the greatest difference on ASV clustering compared with WT group, including 1484 exclusive ASV. However, AMP group showed the least difference on ASV clustering, with only 640 exclusive ASV, suggesting that AMP might decrease the difference of intestinal microorganisms between normal mice and diabetic mice. PCA analysis (Figure 7B) also exhibited that the points of db/db group were completely separated from those of WT group, whereas the points of AMP group were much closer with those of WT group, suggesting that the gut microbiota composition of AMP group was more similar to that of WT group.
FIGURE 5. Comparison of biodiversity of gut microbiota community from C57BL/6J mice and db/db mice received different treatment (n = 8) via the Chao1 index (A), Simpson index (B), Shannon index (C) and Observed-OTUs (D). #: p <0.05, ##: p <0.01, ###: p <0.001 compared with WT group; *: p <0.05, **: p <0.01, ***: p <0.001 compared with db/db group.
FIGURE 6. Comparison of relative abundance of gut microbiota community from C57BL/6J mice and db/db mice received different treatment as shown in relative bacterial abundance of top 10 at phylum level (A) and heatmap of top 35 at genus level (B).
FIGURE 7. Difference in gut microbiota composition from C57BL/6J mice and db/db mice received different treatment (n = 8) as demonstrated in their Venn Diagrams of overlapping and exclusive ASVs clustering (A) and Principal Component Analysis (B).
Pearson correlation analyses between hypoglycemic effect index (fasting glucose level, ipGTT and HOMA-IR index) and the relative abundance of top 35 intestinal bacteria species were conducted as shown in Figures 8A–C. It was noted that the relative abundance of Allobaculum, Faecalibaculum, Akkermansia, Bifidobacterium, and Dubosiella were negatively correlated (p<0.05) with the hypoglycemic effect index with the correlation coefficients for Bifidobacterium and Dubosiella greater than 0.6. In addition, the relative abundance of 23 intestinal bacteria species exhibited positively correlation with the hypoglycemic effect index with correlation coefficients for Odoribacter, Lachnospiraceae__A2, and Lachnoclostridium greater than 0.6. Furthermore, the correlation analysis between total SCFA level and the relative abundance of top 35 intestinal bacteria species (Figure 8D) revealed that the level of total SCFA was positively correlated (p<0.05) with the relative abundance of Akkermansia, Faecalibaculum, Romboutsia, Prevotellaceae_UCG−001, and Oscillospiraceae_UCG−005 with correlation coefficients all greater than 0.4. Meanwhile, the level of total SCFA was negatively correlated (p<0.05) with the relative abundance of Odoribacter, Lachnoclostridium, Lachnospiraceae_UCG-006, Lachnospiraceae_A2, and Bacteroides with correlation coefficients all greater than 0.4.
FIGURE 8. Correlation analyses between relative abundance of gut microbiota in mice from WT, db/db and AMP groups with their ipGTT test result (A), HOMA-IR index (B), Fasting blood glucose level (C), Total SCFA level (D).
Since increasing evidence demonstrated that the dysbiosis of gut microbiota was closely associated with T2D occurrence (Gentile and Weir, 2018), many therapeutic strategies designed based on alterations of gut microbiota have been gradually applied on the T2D treatment such as healthy feces transplantation, probiotics treatment and herbal polysaccharides treatment (Fan and Pedersen, 2021). Although AMP, as important chemical constituents of Astragalus membranaceus, have been reported to exhibit impressive potential on T2D therapy (Zou et al., 2009; Ye et al., 2014), our current study for the first time demonstrated its gut microbiota mediated hyperglycemic effect and disclosed the related underlying mechanisms. Our prepared AMP consisted of multiple monosaccharide compositions of galacturonic acid, glucose, galactose, and arabinose, which is similar to those reported in previous studies (Liu et al., 2019; Yang et al., 2021). The signal at 1741.48 cm−1 in the FT-IR spectrum also indicated the presence of carbonyl group, which was possibly due to the existence of galacturonic acid in our prepared AMP. It was noted that the molar ratio of galactose (13.5%) and arabinose (43.1%) in our prepared AMP was obviously higher than that in the previous study (Liu et al., 2019). Since our sample was extracted from planted herbs, but the reported sample was extracted from the herbal residue provided by commercial supplier. Therefore, it was speculated that the difference on monosaccharide composition might be resulted from different herb sources.
SCFA are the gut microbiota-producing metabolites derived from plant fibers or polysaccharides. Previous study reported that diabetic mice exhibited significant decline on SCFA level compared with normal mice (Han et al., 2021). In our current study, the level of all the three SCFA in the feces from db/db mice were increased significantly after treatment with AMP, suggesting that AMP could promote the production of SCFA, which were also observed in other antidiabetic herbal polysaccharides (Qi et al., 2022) including Dendrobium polysaccharides (Fang et al., 2022), Polygonatum polysaccharides (Zheng et al., 2020) and Ophiopogon polysaccharides (Wang et al., 2019). A growing number of evidence illustrated that SCFA were deeply involved in various physiological process like glycemic control and immunity modulation (Ley et al., 2005). GPCR 41 and GPCR 43, the two receptors mainly locating in colon, which can be activated selectively by SCFA (Brown et al., 2003). Although the function of GPCR 41/43 was still not illustrated very clearly, increasing studies indicated that they were the important signal proteins involving in glucose metabolism (Xiong et al., 2004; Bindels et al., 2013). GLP-1 is an intestinal hormone secreted by intestinal L cells and its physiological function is to stimulate insulin secretion and inhibit the appetite (Lewis et al., 2020). Previous studies reported that the increasing expression of GPCR41/43 proteins in colon could trigger the secretion of GLP-1. (Izumi et al., 2011; Gwen et al., 2012). Furthermore, an in vitro study demonstrated that SCFA might trigger the secretion of GLP-1 by enhancing the expression of GPCR 41/43 in the intestinal L cells (Kimura et al., 2013). In the present study, treatment of AMP in db/db mice not only lead to the increased level of three kinds of SCFA in fecal sample but also enhanced the GPCR 41/43 expression in the colon tissue as well as more GLP-1 was secreted, it indicated that the increased GLP-1 secretion stimulated by elevated GPCR 41/43 expression after AMP treatment might contribute to its hypoglycemic effect.
Some clinical studies found that the gut microbiota system of T2D patients contained higher concentration of detrimental intestinal metabolites such as indole, hydrogen sulfide, which may damage the intestinal integrity and result in the high level of inflammation factors in vivo (Zhao et al., 2018). Increasing evidence reported that some herbal polysaccharides were able to reshape the disordered gut microbiota community and maintain the intestinal health environment in diabetic animal model (Yan et al., 2017; Zhou et al., 2020). As the major metabolites derived from herbal polysaccharides, SCFA have been reported to exhibit impressive capability on improving intestinal microenvironment, enhancing intestinal integrity, and decreasing inflammation level (Greenblum et al., 2012). In this study, besides increased SCFA production level, treatment with AMP in db/db mice for the first time demonstrated to be able to improve the intestinal integrity by increasing the expression of tight junction proteins (Occludin and ZO-1) in small intestine and decrease the serum level of proinflammatory factor, like IL-6, TNF-α and IL-1β. Previous studies also found that increased intestinal integrity could improve the hyperglycemic symptoms by inhibiting the glucose and inflammatory factors influx in small intestine (Thaiss, C., et al., 2018; Pearce et al., 2013). Consequently, our study implied that the increased expressions of tight junction proteins (Occludin and ZO-1) might also contribute to the hypoglycemic effect of AMP.
To evaluate the impact of AMP on gut microbiota composition from db/db mice, a comprehensive analysis on gut microbiota composition as well as their function was conducted. It was found that the diversity of gut microbiota community was increased after treatment with AMP, but the richness exhibited no significant difference, which suggested gut microbiota composition became more diverse and complex under the treatment of AMP although the total number of bacteria did not increase. Diversity and richness were the two critical ecological index to evaluate the health of gut microbiota community (Cotillard et al., 2013). Previous research also found that the balanced and diverse gut microbiota system was beneficial in the prevention and therapy on chronic disease and metabolic dysfunction (Gentile and Weir, 2018), which was also supported by the current study. To investigate which intestinal bacteria was regulated by AMP, relative abundance analysis of gut microbiota was conducted. In the phylum level. It was observed that the ratio of Bacteroidota/Firmicutes in db/db mice (1.16 ± 0.07) was decreased compared with that in normal mice (1.76 ± 0.17). Meanwhile, the ratio of Bacteroidota/Firmicutes (1.52 ± 0.14) in db/db mice after treatment with AMP was increased. Although the ratio of Bacteroidota/Firmicutes was reported to correlate with the obesity and other metabolic disease, some contradictory conclusions also were observed when treated with different medicine (Patterson et al., 2016; Li et al., 2020). It was also evidenced by our findings that MET group (1.09 ± 0.10) and AMP group (1.52 ± 0.14) exhibited the contrary impact on the ratio, which implied that the regulatory effect of AMP and MET on the disordered bacteria community were different. The relative abundance analysis on the bacteria species in the genus level also demonstrated that the microbiota composition in different treatment groups was lack of similarity. Notably, the relative abundance of gut microbiota from mice in healthy state and diabetic state was obviously different, the similar phenomenon was also observed in T2D patients and healthy donors (Wang et al., 2012). The microbiota composition of AMP group was partially overlapped with that of WT group, which suggested AMP could partially restore the dysbiosis of gut microbiota community and reestablish the healthy intestinal microenvironment.
Our Pearson correlation analyses between hypoglycemic effect index and the relative abundance of gut microbiota revealed that Allobaculum, Faecalibaculum, Akkermansia, Bifidobacterium, and Dubosiella exhibited positively association with hypoglycemic effect significantly, while Odoribacter, Lachnospiraceae__A2, and Lachnoclostridium were found to be negatively correlated with the hypoglycemic effect significantly. Among these identified bacteria, Bifidobacterium is the most reported genus with the potential to protect gastrointestinal tract (Le et al., 2015) and improve glucose tolerance (Moya-Pérez et al., 2015; Aoki et al., 2017), Faecalibaculum were also found in lower abundance in T2D patients (Candela et al., 2016). Akkermansia were found to be beneficial in maintaining the intestinal integrity (Simpson et al., 2021), which was supported by our findings that treatment with AMP in db/db mice not only enriched the abundance of Akkermansia, also increased the expression of tight junction proteins. On the other hand, Odoribacter was also reported to exhibit higher abundance in diabetic mice and cause some health problems such as abdominal inflammation (Geurts et al., 2011). Our further correlation analyses between total SCFA level and the relative abundance of gut microbiota indicated that 18 intestinal bacteria including Akkermansia, Faecalibaculum, Romboutsia, Prevotellaceae_UCG−001, and Oscillospiraceae_UCG−005 were positive correlated with SCFA level significantly. Faecalibaculum was found to be one kind of intestinal bacteria closely associated with the production of SCFA (Zagato et al., 2020). Akkermansia was the bacteria species with the function of utilizing non-digestible herbal polysaccharides and producing SCFA (Louis et al., 2014). In our study, treatment with AMP group in db/db mice also resulted in higher abundance of Akkermansia, Faecalibaculum, and Romboutsia significantly, which suggested that AMP might restore the disordered gut microbiota community by increasing the relative abundance of SCFA-producing bacteria. In summary, our current study served as the first attempt to investigate the role of gut microbiota on the hypoglycemic effect of AMP as evidenced by the positive correlation between the abundance of Akkermansia, Faecalibaculum and hypoglycemic effect as well as SCFA production, which provided an insight on the gut microbiota mediated hypoglycemic mechanism of AMP.
Our current study demonstrated that AMP exhibited the hypoglycemic effect and could restore the disordered gut microbiota community in db/db mice. Two intestinal bacteria, Akkermansia and Faecalibaculum, were disclosed to positively correlate with hypoglycemic effect and fecal SCFA production significantly. Mechanistic study found that the increased SCFA level in AMP treated db/db mice might stimulate GLP-1 secretion and improve intestinal integrity by enhancing expressions of GPCR41/43 and tight junction proteins (Occludin and ZO-1), respectively, which might be involved in the alleviating diabetic symptoms of db/db mice.
The datasets presented in this study can be found in online repositories. The names of the repository/repositories and accession number(s) can be found below: https://www.ncbi.nlm.nih.gov/bioproject/PRJNA880037.
The animal study was reviewed and approved by Animal Experimentation Ethics Committee of The Chinese University of Hong Kong.
ZZ conceived, coordinated, and supervised the study and wrote the manuscript. SQB conducted experiments, performed data analyses, and wrote the manuscript. CSW conducted experiments and performed data analyses. LD, CHY, and LYZ conducted the experiments. HQB, WHY, and SPC coordinated the study.
The study was partially supported by the Li Dak Sum Yip Yio Chin R & D center for Chinese Medicine at The Chinese University of Hong Kong.
The authors declare that the research was conducted in the absence of any commercial or financial relationships that could be construed as a potential conflict of interest.
All claims expressed in this article are solely those of the authors and do not necessarily represent those of their affiliated organizations, or those of the publisher, the editors and the reviewers. Any product that may be evaluated in this article, or claim that may be made by its manufacturer, is not guaranteed or endorsed by the publisher.
Adak, A., and Khan, M. R. (2019). An insight into gut microbiota and its functionalities. Cell. Mol. Life Sci. 76, 473–493. doi:10.1007/s00018-018-2943-4
Ángela, V., and Herminia, G. (2015). Glucose and insulin tolerance tests in the mouse. Methods Mol. Biol. 1339, 247–254. doi:10.1007/978-1-4939-2929-0_17
Aoki, R., Kamikado, K., Suda, W., Takii, H., Mikami, Y., Suganuma, N., et al. (2017). A proliferative probiotic Bifidobacterium strain in the gut ameliorates progression of metabolic disorders via microbiota modulation and acetate elevation. Sci. Rep. 7, 43522–43610. doi:10.1038/srep43522
Bindels, L. B., Dewulf, E. M., and Delzenne, N. M. (2013). GPR43/FFA2: Physiopathological relevance and therapeutic prospects. Trends Pharmacol. Sci. 34, 226–232. doi:10.1016/j.tips.2013.02.002
Brown, A. J., Goldsworthy, S. M., Barnes, A. A., Eilert, M. M., Tcheang, L., Daniels, D., et al. (2003). The orphan G protein-coupled receptors GPR41 and GPR43 are activated by propionate and other short chain carboxylic acids. J. Biol. Chem. 278, 11312–11319. doi:10.1074/jbc.M211609200
Candela, M., Biagi, E., Soverini, M., Consolandi, C., Quercia, S., Severgnini, M., et al. (2016). Modulation of gut microbiota dysbioses in type 2 diabetic patients by macrobiotic Ma-Pi 2 diet. Br. J. Nutr. 116, 80–93. doi:10.1017/S0007114516001045
Chao, M. L., Zou, D. J., Zhang, Y. F., Chen, Y. H., Wang, M., Wu, H., et al. (2009). Improving insulin resistance with traditional Chinese medicine in type 2 diabetic patients. Endocrine 36, 268–274. doi:10.1007/s12020-009-9222-y
Chen, H., Nie, Q., Hu, J., Huang, X., Yin, J., and Nie, S. (2021). Multiomics approach to explore the amelioration mechanisms of glucomannans on the metabolic disorder of type 2 diabetic rats. J. Agric. Food Chem. 69, 2632–2645. doi:10.1021/acs.jafc.0c07871
Cotillard, A., Kennedy, S. P., Kong, L. C., Prifti, E., Pons, N., Le Chatelier, E., et al. (2013). Dietary intervention impact on gut microbial gene richness. Nature 500, 585–588. doi:10.1038/nature12480
Fan, Y., and Pedersen, O. (2021). Gut microbiota in human metabolic health and disease. Nat. Rev. Microbiol. 19, 55–71. doi:10.1038/s41579-020-0433-9
Fang, J., Lin, Y., Xie, H., Farag, M. A., Feng, S., Li, J., et al. (2022). Dendrobium officinale leaf polysaccharides ameliorated hyperglycemia and promoted gut bacterial associated SCFAs to alleviate type 2 diabetes in adult mice. Food Chem. X 13, 100207. doi:10.1016/j.fochx.2022.100207
Fredrik, H. K., Valentina, T., Intawat, N., Göran, B., Carl, J. B., Björn, F., et al. (2013). Gut metagenome in European women with normal, impaired and diabetic glucose control. Nature 498, 99–103. doi:10.1038/nature12198
Fu, Z., Xia, L., De, J., Zhu, M., Li, H., Lu, Y., et al. (2019). Beneficial effects on H1N1-induced acute lung injury and structure characterization of anti-complementary acidic polysaccharides from Juniperus pingii var. wilsonii. Int. J. Biol. Macromol. 129, 246–253. doi:10.1016/j.ijbiomac.2019.01.163
Gentile, C. L., and Weir, T. L. (2018). The gut microbiota at the intersection of diet and human health. Science 362, 776–780. doi:10.1126/science.aau5812
Geurts, L., Lazarevic, F., Derrien, M., Everard, A., Roye, M. V., Knauf, C., et al. (2011). Altered gut microbiota and endocannabinoid system tone in obese and diabetic leptin-resistant mice: Impact on apelin regulation in adipose tissue. Front. Microbiol. 2, 149–217. doi:10.3389/fmicb.2011.00149
Greenblum, S., Turnbaugh, P. J., and Borenstein, E. (2012). Metagenomic systems biology of the human gut microbiome reveals topological shifts associated with obesity and inflammatory bowel disease. Proc. Natl. Acad. Sci. U. S. A. 109, 594–599. doi:10.1073/pnas.1116053109
Gurung, M., Li, Z., You, H., Rodrigues, R., Jump, D. B., Morgun, A., et al. (2020). Role of gut microbiota in type 2 diabetes pathophysiology. EBioMedicine 51, 102590. doi:10.1016/j.ebiom.2019.11.051
Gwen, T., Heffron, H., Lam, Y. S., Parkers, H., Habib, A., Cameron, J., et al. (2012). Short-chain fatty acids stimulate glucagon-like peptide-1 secretion via the G-Protein–Coupled Receptor FFAR2. Diabetes 61, 364–371. doi:10.2337/db11-1019
Han, X., Wang, Y., Zhang, P., Zhu, M., Li, L., Mao, X., et al. (2021). Kazak faecal microbiota transplantation induces short-chain fatty acids that promote glucagon-like peptide-1 secretion by regulating gut microbiota in db/db mice. Pharm. Biol. 59, 1077–1087. doi:10.1080/13880209.2021.1954667
Haxaire, K., Maréchal, Y., Milas, M., and Rinaudo, M. (2003). Hydration of polysaccharide hyaluronan observed by IR spectrometry. I. Preliminary experiments and band Assignments. Biopolymers 72, 10–20. doi:10.1002/bip.10245
Hu, J. L., Nie, S. P., and Xie, M. Y. (2018). Antidiabetic mechanism of dietary polysaccharides based on their gastrointestinal functions. J. Agric. Food Chem. 66, 4781–4786. doi:10.1021/acs.jafc.7b05410
Izumi, K., Shinichiro, K., Ryo, T., and Atsukazu, K. (2011). Density distribution of free fatty acid receptor 2 (FFA2) expressing and GLP-1-producing enteroendocrine L cells in human and rat lower intestine, and increased cell numbers after ingestion of fructo-oligosaccharide. J. Mol. Histol. 42, 27–38. doi:10.1007/s10735-010-9304-4
Jose, C., Luke, K. U., Laura, W. P., and Rob, K. (2012). The impact of the gut microbiota on human health: An integrative view. Cell 148, 1258–1270. doi:10.1016/j.cell.2012.01.035
Kimura, I., Ozawa, K., Inoue, D., Imamura, T., Kimura, K., Maeda, T., et al. (2013). The gut microbiota suppresses insulin-mediated fat accumulation via the short-chain fatty acid receptor GPR43. Nat. Commun. 4, 1829–1914. doi:10.1038/ncomms2852
Ko, C. H., Yi, S., Ozaki, R., Chung, H., Cochrane, H. D., Lau, W. N., et al. (2014). Healing effect of a two-herb recipe (NF3) on foot ulcers in Chinese patients with diabetes: A randomized double-blind placebo-controlled study. J. Diabetes 6, 323–334. doi:10.1111/1753-0407.12117
Kuang, M. T., Li, J. Y., Yang, X. B., Yang, L., Xu, J. Y., Yan, S., et al. (2020). Structural characterization and hypoglycemic effect via stimulating glucagon-like peptide-1 secretion of two polysaccharides from Dendrobium officinale. Carbohydr. Polym. 241, 116326. doi:10.1016/j.carbpol.2020.116326
Le, T. K. C., Hosaka, T., Nguyen, T. T., Kassu, A., Dang, T. O., Tran, H. B., et al. (2015). Bifidobacterium species lower serum glucose, increase expressions of insulin signaling proteins, and improve adipokine profile in diabetic mice. Biomed. Res. 36, 63–70. doi:10.2220/biomedres.36.63
Lewis, J. E., Miedzybrodzka, E. L., Foreman, R. E., Woodward, O. R. M., Kay, R. G., Goldspink, D. A., et al. (2020). Selective stimulation of colonic L cells improves metabolic outcomes in mice. Diabetologia 63, 1396–1407. doi:10.1007/s00125-020-05149-w
Ley, R. E., Sonnenburg, J. L., Peterson, D. A., and Gordon, J. I. (2005). Host-bacterial mutualism in the human intestine. Lancet 216, 1302–1303. doi:10.1016/S0140-6736(00)90267-8
Leyva, A., Quintana, A., Sánchez, M., Rodríguez, E. N., Cremata, J., and Sánchez, J. C. (2008). Rapid and sensitive anthrone-sulfuric acid assay in microplate format to quantify carbohydrate in biopharmaceutical products: Method development and validation. Biologicals 36, 134–141. doi:10.1016/j.biologicals.2007.09.001
Li, H., Fang, Q., Nie, Q., Hu, J., Yang, C., Huang, T., et al. (2020). Hypoglycemic and hypolipidemic mechanism of tea polysaccharides on type 2 diabetic rats via gut microbiota and metabolism alteration. J. Agric. Food Chem. 68, 10015–10028. doi:10.1021/acs.jafc.0c01968
Li, J., Li, R., Li, N., Zheng, F., Dai, Y., Ge, Y., et al. (2018). Mechanism of antidiabetic and synergistic effects of ginseng polysaccharide and ginsenoside Rb1 on diabetic rat model. J. Pharm. Biomed. Anal. 158, 451–460. doi:10.1016/j.jpba.2018.06.024
Liu, M., Wu, K., Mao, X., Wu, Y., and Ouyang, J. (2010). Astragalus polysaccharide improves insulin sensitivity in KKAy mice: Regulation of PKB/GLUT4 signaling in skeletal muscle. J. Ethnopharmacol. 127, 32–37. doi:10.1016/j.jep.2009.09.055
Liu, Y., Liu, W., Li, J., Tang, S., Wang, M., Huang, W., et al. (2019). A polysaccharide extracted from Astragalus membranaceus residue improves cognitive dysfunction by altering gut microbiota in diabetic mice. Carbohydr. Polym. 205, 500–512. doi:10.1016/j.carbpol.2018.10.041
Louis, P., Hold, G. L., and Flint, H. J. (2014). The gut microbiota, bacterial metabolites and colorectal cancer. Nat. Rev. Microbiol. 12, 661–672. doi:10.1038/nrmicro3344
Lyu, Y., Lin, L., Xie, Y., Li, D., Xiao, M., Zhang, Y., et al. (2021). Blood-glucose-lowering effect of coptidis rhizoma extracts from different origins via gut microbiota modulation in db/db mice. Front. Pharmacol. 12, 684358–684415. doi:10.3389/fphar.2021.684358
Ma, Q. Y., Zhai, R. H., Xie, X. Q., Chen, T., Zhang, Z. Q., Liu, H. C., et al. (2022). Hypoglycemic effects of lycium barbarum polysaccharide in type 2 diabetes mellitus mice via modulating gut microbiota. Front. Nutr. 9, 916271. doi:10.3389/fnut.2022.916271
Mao, X. Q., Yu, F., Wang, N., Wu, Y., Zou, F., Wu, K., et al. (2009). Hypoglycemic effect of polysaccharide enriched extract of Astragalus membranaceus in diet induced insulin resistant C57BL/6J mice and its potential mechanism. Phytomedicine 16, 416–425. doi:10.1016/j.phymed.2008.12.011
Moya-Pérez, A., Neef, A., and Sanz, Y. (2015). Bifidobacterium pseudocatenulatum CECT 7765 reduces obesity-associated inflammation by restoring the lymphocyte-macrophage balance and gut microbiota structure in high-fat diet-fed mice. Plos One 10, 01269766–e127028. doi:10.1371/journal.pone.0126976
Patterson, E. E., Ryan, P. M., Cryan, J. F., Dinan, T. G., Paul Ross, R., Fitzgerald, G. F., et al. (2016). Gut microbiota, obesity and diabetes. Postgrad. Med. J. 92, 286–300. doi:10.1136/postgradmedj-2015-133285
Pearce, S., Mani, V., Boddicker, R., Johnson, J., Weber, T., Ross, J., et al. (2013). Heat stress reduces intestinal barrier integrity and favors intestinal glucose transport in growing pigs. Plos One 8, e70215. doi:10.1371/journal.pone.0070215
Qi, B., Ren, D., Li, T., Niu, P., Zhang, X., Yang, X., et al. (2022). Fu brick tea manages HFD/STZ-Induced type 2 diabetes by regulating the gut microbiota and activating the IRS1/PI3K/akt signaling pathway. J. Agric. Food Chem. 70, 8274–8287. doi:10.1021/acs.jafc.2c02400
Simpson, C. A., Diaz-Arteche, C., Eliby, D., Schwartz, O. S., Simmons, J. G., and Cowan, C. S. M. (2021). The gut microbiota in anxiety and depression–A systematic review. Clin. Psychol. Rev. 83, 101943. doi:10.1016/j.cpr.2020.101943
Thaiss, C., Levy, M., Grosheva, I., Zheng, D. P., Soffer, E., Blacher, E., et al. (2018). Hyperglycemia drives intestinal barrier dysfunction and risk for enteric infection. Science 359, 1376–1383. doi:10.1126/science.aar3318
Tsang, M. S. M., Cheng, S. W., Zhu, J., Atli, K., Chan, B. C. L., Liu, D., et al. (2018). Anti-inflammatory activities of pentaherbs formula and its influence on gut microbiota in allergic asthma. Molecules 23, E2776. doi:10.3390/molecules23112776
Wang, H. Y., Guo, L. X., Hu, W. H., Peng, Z. T., Wang, C., Chen, Z. C., et al. (2019). Polysaccharide from tuberous roots of Ophiopogon japonicus regulates gut microbiota and its metabolites during alleviation of high-fat diet-induced type-2 diabetes in mice. J. Funct. Foods 63, 103593. doi:10.1016/j.jff.2019.103593
Wang, J., Qin, J., Li, Y., Cai, Z., Li, S., Zhu, J., et al. (2012). A metagenome-wide association study of gut microbiota in type 2 diabetes. Nature 490, 55–60. doi:10.1038/nature11450
Wu, T. R., Lin, C. S., Chang, C. J., Lin, T. L., Martel, J., Ko, Y. F., et al. (2019). Gut commensal Parabacteroides goldsteinii plays a predominant role in the anti-obesity effects of polysaccharides isolated from Hirsutella sinensis. Gut 68, 248–262. doi:10.1136/gutjnl-2017-315458
Xiong, Y., Miyamoto, N., Shibata, K., Valasek, M. A., Motoike, T., Kedzierski, R. M., et al. (2004). Short-chain fatty acids stimulate leptin production in adipocytes through the G protein-coupled receptor GPR41. Proc. Natl. Acad. Sci. U. S. A. 101, 1045–1050. doi:10.1073/pnas.2637002100
Xu, J., Li, S. L., Yue, R. Q., Ko, C. H., Hu, J. M., Liu, J., et al. (2014). A novel and rapid HPGPC-based strategy for quality control of saccharide-dominant herbal materials: Dendrobium officinale, a case study. Anal. Bioanal. Chem. 406, 6409–6417. doi:10.1007/s00216-014-8060-9
Yan, H. L., Lu, J. M., Wang, Y. F., Gu, W., and Yu, J. (2017). Intake of total saponins and polysaccharides from Polygonatum kingianum affects the gut microbiota in diabetic rats. Phytomedicine 26, 45–54. doi:10.1016/j.phymed.2017.01.007
Yang, Z. M., Wang, Y., and Chen, S. Y. (2021). Astragalus polysaccharide alleviates type 2 diabetic rats by reversing the glucose transporters and sweet taste receptors/GLP-1/GLP-1 receptor signaling pathways in the intestine-pancreatic axis. J. Funct. Foods 76, 104310. doi:10.1016/j.jff.2020.104310
Ye, Y., Deng, T., Wan, X. Y., Ouyang, J. P., Liu, M., and Mao, X. Q. (2014). The role of quantitative changes in the epxression of insulin receptor substrate-1 and nuclear ubiquitin in abnormal glycometabolism in the livers of KKay mice and the relative therapeutic mechanisms of Astragalus polysaccharide. Int. J. Mol. Med. 33, 341–350. doi:10.3892/ijmm.2013.1580
Zagato, E., Pozzi, C., Bertocchi, A., Schioppa, T., Saccheri, F., Guglietta, S., et al. (2020). Endogenous murine microbiota member Faecalibaculum rodentium and its human homologue protect from intestinal tumour growth. Nat. Microbiol. 5, 511–524. doi:10.1038/s41564-019-0649-5
Zhao, L., Zhang, F., Ding, X., Wu, G., Lam, Y. Y., Wang, X., et al. (2018). Gut bacteria selectively promoted by dietary fibers alleviate type 2 diabetes. Science 359, 1151–1156. doi:10.1126/science.aao5774
Zheng, Y., Bai, L., Zhou, Y., Tong, R., Zeng, M., Li, X., et al. (2019). Polysaccharides from Chinese herbal medicine for anti-diabetes recent advances. Int. J. Biol. Macromol. 121, 1240–1253. doi:10.1016/j.ijbiomac.2018.10.072
Zheng, Y. J., Ren, W., Zhang, L., Zhang, Y., Liu, D., and Liu, Y. (2020). A review of the pharmacological action of Astragalus polysaccharide. Front. Pharmacol. 11, 349–415. doi:10.3389/fphar.2020.00349
Zhou, L. S., Liao, W. F., Chen, X., Yue, H., Li, S. J., and Ding, K. (2018). An arabinogalactan from fruits of Lycium barbarum L. inhibits production and aggregation of Aβ42. Carbohydr. Polym. 175, 643–651. doi:10.1016/j.carbpol.2018.05.022
Zhou, W. T., Chen, G. J., Chen, D., Ye, H., and Zeng, X. X. (2020). The antidiabetic effect and potential mechanisms of natural polysaccharides based on the regulation of gut microbiota. J. Funct. Foods 75, 104222. doi:10.1016/j.jff.2020.104222
Keywords: Astragalus membranaceus polysaccharides, diabetes, gut microbiota, hypoglycemic mechanism, short chain fatty acids
Citation: Song Q, Cheng SW, Li D, Cheng H, Lai YS, Han Q, Wu HY, Shaw PC and Zuo Z (2022) Gut microbiota mediated hypoglycemic effect of Astragalus membranaceus polysaccharides in db/db mice. Front. Pharmacol. 13:1043527. doi: 10.3389/fphar.2022.1043527
Received: 13 September 2022; Accepted: 19 October 2022;
Published: 14 November 2022.
Edited by:
Xuan Zeng, Sun Yat-sen University, ChinaReviewed by:
Eugenia Bezirtzoglou, Democritus University of Thrace, GreeceCopyright © 2022 Song, Cheng, Li, Cheng, Lai, Han, Wu, Shaw and Zuo. This is an open-access article distributed under the terms of the Creative Commons Attribution License (CC BY). The use, distribution or reproduction in other forums is permitted, provided the original author(s) and the copyright owner(s) are credited and that the original publication in this journal is cited, in accordance with accepted academic practice. No use, distribution or reproduction is permitted which does not comply with these terms.
*Correspondence: Zhong Zuo, am9hbnp1b0BjdWhrLmVkdS5oaw==
Disclaimer: All claims expressed in this article are solely those of the authors and do not necessarily represent those of their affiliated organizations, or those of the publisher, the editors and the reviewers. Any product that may be evaluated in this article or claim that may be made by its manufacturer is not guaranteed or endorsed by the publisher.
Research integrity at Frontiers
Learn more about the work of our research integrity team to safeguard the quality of each article we publish.