- 1Faculty of Medicine, Institute of Biochemistry I, Goethe University Frankfurt, Frankfurt, Germany
- 2Frankfurt Cancer Institute, Goethe University Frankfurt, Frankfurt, Germany
- 3German Cancer Consortium (DKTK), Partner Site Frankfurt, Frankfurt, Germany
- 4Fraunhofer Institute for Translational Medicine and Pharmacology ITMP, Frankfurt, Germany
As a lipoxygenase (LOX), arachidonate 15-lipoxygenase type B (ALOX15B) peroxidizes polyenoic fatty acids (PUFAs) including arachidonic acid (AA), eicosapentaenoic acid (EPA), docosahexaenoic acid (DHA), and linoleic acid (LA) to their corresponding fatty acid hydroperoxides. Distinctive to ALOX15B, fatty acid oxygenation occurs with positional specificity, catalyzed by the non-heme iron containing active site, and in addition to free PUFAs, membrane-esterified fatty acids serve as substrates for ALOX15B. Like other LOX enzymes, ALOX15B is linked to the formation of specialized pro-resolving lipid mediators (SPMs), and altered expression is apparent in various inflammatory diseases such as asthma, psoriasis, and atherosclerosis. In primary human macrophages, ALOX15B expression is associated with cellular cholesterol homeostasis and is induced by hypoxia. Like in inflammation, the role of ALOX15B in cancer is inconclusive. In prostate and breast carcinomas, ALOX15B is attributed a tumor-suppressive role, whereas in colorectal cancer, ALOX15B expression is associated with a poorer prognosis. As the biological function of ALOX15B remains an open question, this review aims to provide a comprehensive overview of the current state of research related to ALOX15B.
Introduction
Arachidonate 15-lipoxygenase type B (ALOX15B) is one of two described lipoxygenases that catalyze the peroxidation of arachidonic acid (AA) with positional specificity at carbon atom 15 (C15) (Brash et al., 1997). Lipoxygenases (LOXs) are non-heme iron containing dioxygenases that convert polyenoic fatty acids (PUFAs) to the corresponding short-lived hydroperoxy derivatives (Kühn et al., 2015). These fatty acid hydroperoxides then undergo a subsequent reduction to lipid alcohols or conversion to secondary oxygenation products. As biologically active lipid mediators, the produced fatty acids are involved in a wide range of acute and chronic pathological conditions (Kühn et al., 2015). Depending on the metabolite, its effect and implication can be ambiguous: anti-inflammatory, pro-resolving, pro-inflammatory (Fogh et al., 1988), as well as pro- or anti-tumorigenic (Vaezi et al., 2021). Although initially classified according to their positional specificity of AA oxygenation, the six functional human LOX enzymes ALOX15B, ALOX15, ALOX5, ALOX12, ALOX12B, and ALOXE3 can also oxygenate other PUFA substrates i.e.; linoleic acid (LA), docosahexaenoic acid (DHA), and eicosapentaenoic acid (EPA). With the exception of the ALOX5 gene, which maps to the long arm of chromosome 10 (10q11.21), all other functional LOX genes are located in a common gene cluster on the short arm of chromosome 17 (Kühn et al., 2018). Although differing mainly in their tissue expression and positional specificity of PUFA oxygenation, all mammalian LOX enzymes perform non-redundant biological functions. In particular, the LOX enzymes ALOX5 and ALOX15 are well studied for their singular and collective synthesis of bioactive lipid mediators, such as hydro(pero)xy fatty acids (Kühn 1996), AA-derived leukotrienes (LT) (Savari et al., 2014) and lipoxins as well as EPA- and DHA-derived resolvins (Rv) (Schebb et al., 2022). Nevertheless, ALOX15B has also been implicated in the formation of distinct pro-resolving mediators, including resolvin RvD5 (Perry et al., 2020b).
ALOX15B was first described in 1997 as a previously unrecognized second 15-lipoxygenase with expression in human skin, prostate, lung and cornea (Brash et al., 1997) (for more details on tissue expression see Table 1 and in vitro expression Table 2). Brash et al. (1997) reported regiospecific and S-stereospecific oxygenation of AA to the corresponding fatty acid hydroperoxide 15(S)-hydroperoxyeicosatetraenoic acid (15(S)-HpETE), which was detected as the reduced hydroxide 15-hydroxyeicosatetraenoic acid (15(S)-HETE) (Brash et al., 1997). Shortly after, a mouse homolog structurally close to human ALOX15B was identified as the murine enzyme Alox8 (often also stated as mouse Alox15b or 8-LOX) (Jisaka et al., 1997; Brash et al., 1999). Alox8 shares 78% sequence identity with human ALOX15B but both enzymes differ with respect to their regiospecificity of AA oxygenation. In contrast to ALOX15B, Alox8 introduces molecular oxygen at C8 of AA, therefore catalyzing the formation of 8-HETE (Brash et al., 1999; Jisaka et al., 2000). Intriguingly, the difference in substrate regiospecificity is mediated by the substitution of only two amino acids between Alox8 and ALOX15B, namely exchange of Tyr603/His604 in Alox8 towards Asp602/Val603 in ALOX15B and their substitution converts the positional specificity of AA oxygenation from C8 to C15 and vice versa (Jisaka et al., 2000). Interestingly, inducible expression of both enzymes attenuated cell growth in keratinocytes (Schweiger et al., 2007). Recent data from Alox15b knock-in mice, humanized by mutation of the identified amino acid pair in Alox8, show that the hematopoietic system including erythrocyte number, hematocrit and hemoglobin is impaired in male mice. Also, this is paralleled by a premature growth arrest (Schäfer et al., 2022). These mice provide a rare insight into the biological function of human ALOX15B and mouse Alox8 in vivo, as there are no commercially available Alox8-deficient mice to date. How ALOX15B is implicated in the regulation of erythropoiesis is unknown so far, but apart from this, ALOX15B is linked to a variety of pathological states. With this review, we aim to summarize the existing findings on the enzymatic and biological function of ALOX15B and cast light on its implication in inflammatory diseases and cancer.
Structural organization of ALOX15B
Mammalian LOX enzymes are single polypeptide chain proteins that fold into a two-domain structure with a C-terminal α-helical domain, containing a non-heme iron carrying putative substrate-binding pocket and an N-terminal 'β-barrel’ (Figures 1A,B). The latter functions in the acquisition of the PUFA substrate (Figure 1C) and the calcium (Ca2+)-dependent membrane binding via a polycystin-1-lipoxygenase α-toxin (PLAT) domain (Brinckmann et al., 1998; Kühn et al., 2015). Crystal structure analysis indicated that ALOX15B obtains the typical LOX fold, harboring an amino-terminal PLAT domain with two Ca2+-binding sites and a large α-helical catalytic domain (Kobe et al., 2014) (Figure 1A). Furthermore, the ALOX15B crystal structure revealed an ALOX15B unique hydrophobic hairpin, which apparently helps to position the enzyme at the phospholipid bilayer upon Ca2+-binding, thereby allowing it to function as a membrane anchor and supporting the accessibility to membrane-bound substrates (Kobe et al., 2014). In agreement, further reports of crystal structure analysis and ALOX15B expressing cell lines demonstrated a Ca2+-dependent association of human ALOX15B with the membrane fraction (Kilty et al., 1999; Bender et al., 2016) and described a Ca2+-stabilized putative membrane insertion loop, which was found to project from the amino-terminal membrane binding domain (Bender et al., 2016). Using cytosol-free, recombined fractions from LOX-absent cell lines, the presence of Ca2+ led to translocation of recombinant ALOX15B to the membrane fraction, which renders dependence on a membrane-docking protein unlikely (Kilty et al., 1999). However, Western blotting of membrane and cytosol fractions of ALOX15B-expressing cell lines demonstrated the dominant cytosolic location of ALOX15B in resting cells (Kilty et al., 1999).
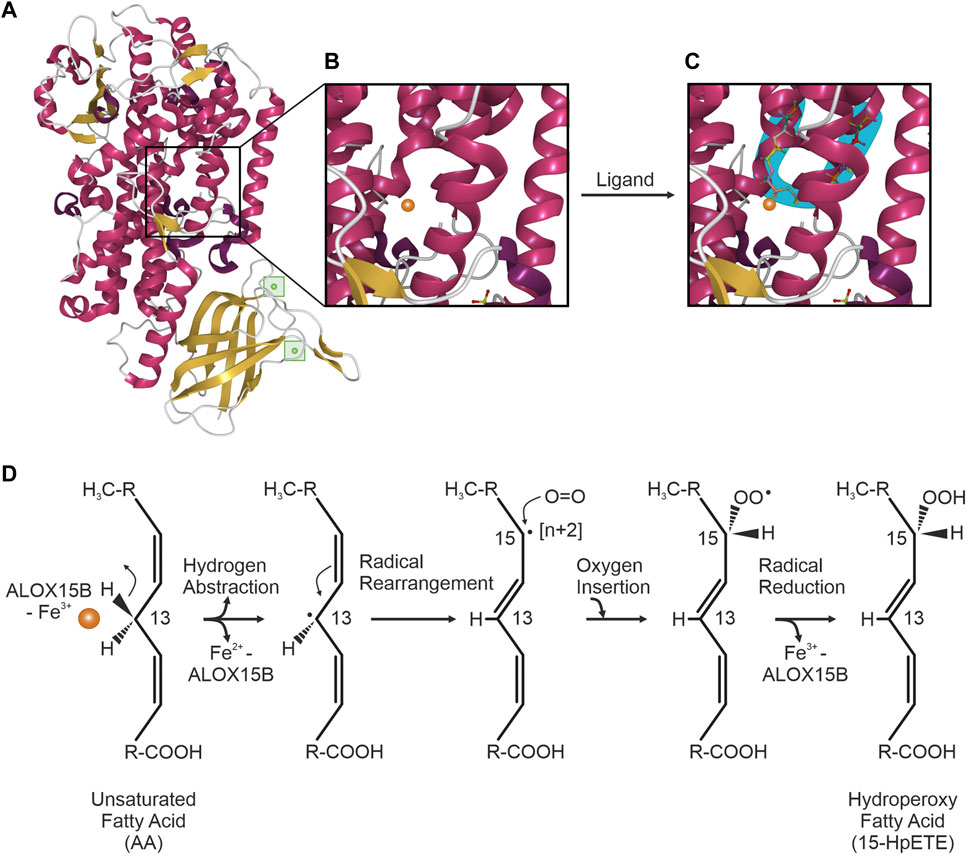
FIGURE 1. ALOX15B crystal structure and mechanism of fatty acid oxygenation. (A) Crystal structure of ALOX15B with C-terminal α-helical domain (red) and β-sheets (yellow). Inside the N-terminal 'β-barrel’, two Ca2+-binding sites (green) allow membrane binding of ALOX15B. (B) Zoom-in into the putative substrate-binding pocket inside the α-helical domain, containing a non-heme iron (orange). (C) Ligand binding to the U-shaped substrate binding channel (blue) places the respective pentadiene close to the reactive iron (orange). Crystal structure was obtained and modified based on the RCSB PDB (rcsb.org) dataset 4NRE (DOI: 10.2210/pdb4NRE/pdb), deposited by Kobe et al. (2014) and visualized with Mol* Viewer (Sehnal et al., 2021). (D) ALOX15B-catalyzed oxygenation of an unsaturated fatty acid [example attack here with arachidonic acid (AA)] is initiated by the hydrogen abstraction of a cis,cis-1,4-pentadiene (at C13 of AA) promoted through the ferric (Fe3+) iron (orange) of the ALOX15B catalytic center, leading to an inactive enzyme (Fe2+). Upon rearrangement of the radical from C13 to C15 [+2], molecular oxygen is inserted antarafacially to the hydrogen abstraction at C15. Reduction of the resulting peroxyl-radical is mediated through electron transfer from Fe2+ and is accompanied with the re-oxidation to Fe3+, thus restoring enzyme activity. Finally, a fatty acid hydroperoxide is formed through the protonation of the resulting peroxy-anion.
Also, in normal human prostate cells (NHP) ALOX15B was found to be predominantly localized in the cytosol, while some protein was detected in the nucleus or associated with cytoskeletal proteins, microsomes, and membranes (Bhatia et al., 2003). Localization in the nucleus may suggest a non-enzymatic function of the protein as proposed for other LOX isoforms (Kreiß et al., 2022). Interestingly, alternative splice variants of ALOX15B have not been described to localize to the nucleus (Bhatia et al., 2003) and demonstrated very little AA metabolizing activity (Tang et al., 2007). Potentially, nuclear ALOX15B enzyme behaves comparable to other nuclear LOX isoforms in producing ligands for nuclear receptors.
In contrary to previous studies, using nanodisc-associated ALOX15B, kinetics of hydrogen/deuterium exchange indicated no structural changes of the PLAT domain upon Ca2+-binding, only upon ligand binding. It was proposed that the hydrophobic PLAT loop rather mediates a transient than a deep and stable insertion into the membrane. The authors proposed that Ca2+-binding to the PLAT domain mediates membrane association more likely through the addition of a positive charge to the PLAT domain, thereby coordinating an association with phosphatidylserine, than changing the structure and dynamics of the protein (Droege et al., 2017). This finding was supported by data of former work with nanodisc-associated ALOX15B, where no “scooting mode” but a “hopping mode” was suggested for membrane-bound substrate accession through ALOX15B. In this suggested mode of action, ALOX15B is thought to hop from one membrane contact point to the next, thereby reaching all available substrates. This mode requires a collision of enzyme and membrane for each turnover (Bender et al., 2016).
LOX-mediated fatty acid peroxidation
LOX-catalyzed fatty acid peroxidation is widely recognized as the free radical mechanism (de Groot et al., 1975; Ludwig et al., 1987; Lehnert and Solomon 2003). The non-heme iron (Fe3+, active) in the catalytic center of the LOX enzyme promotes a stereospecific hydrogen abstraction from the central carbon of a cis,cis-1,4-pentadiene PUFA moiety, generating a carbon-centered fatty acid radical. Subsequently, the disintegration of the abstracted hydrogen atom facilitates a proton-coupled electron transfer of a single electron to the catalytic Fe3+ center, leading to an inactive Fe2+. Followed by the rearrangement of the radical, molecular oxygen is antarafacially inserted into the PUFA backbone and the corresponding mono-peroxyl radical is formed. The catalytic machinery of the enzyme is restored by a re-oxidation of Fe2+ to Fe3+ through the electron transfer from the ferrous iron to the radical, forming a fatty acid peroxy-anion that subsequently is protonated to a hydroperoxide. For 15-LOX orthologs, AA-peroxidation involves C13-hydrogen abstraction and introduction of oxygen [+2] at C15 (Ivanov et al., 2015) (Figure 1D).
Regiospecificity and substrate orientation of ALOX15B-catalyzed oxygenation
Although the regiospecificity of ALOX15B with respect to AA oxygenation was reported simultaneously with its discovery (Brash et al., 1997), its underlying mechanisms and the conditions that regulate substrate orientation have been the content of many further studies. In general, besides the 1) access of molecular oxygen to the reacting pentadiene, two more aspects determine LOX regiospecificity and stereospecificity: 2) the PUFA carbon-chain positioning in the active site and 3) the head-to-tail orientation of the PUFA (Newcomer and Brash 2015).
(to 2) Based on various individual crystal structures of LOX enzymes, several concepts have been proposed over the years to explain the positional specificity of LOX enzymes. Initially, with the “Triad Concept” a structural model explaining the variable regiospecificity of mammalian 15-LOX orthologs was defined on the basis of three clusters of amino acid determinants (Borngräber et al., 1999; Ivanov et al., 2010). This triad of amino acids was proposed to form the bottom of the substrate binding pocket and thus, determine the depth of substrate entry and thereby the positional specificity of 15-LOX orthologs. Although confirmed for all mammalian 15-LOX orthologs (Heydeck et al., 2022), the Triad Concept was not applicable to ALOX15B and the closely related ALOX12B (Vogel et al., 2010). More recently, the existence of a U-shaped substrate binding channel that determines the position of the PUFA-pentadiene attack has been at the center of the currently most conjectured concept (Neau et al., 2009; Kobe et al., 2014). Based on the analysis of the detergent octyltetraethylene glycol ether (C8E4)-bound crystal structure of human ALOX15B, C8E4 was found to be positioned in a U-shaped conformation in the active site of ALOX15B (Figure 1C). The superimposition of AA onto the C8E4 location placed the C13 atom of AA adjacent to the catalytic iron of ALOX15B, thereby generating an appropriate position for hydrogen abstraction and oxygenation at C15 (Kobe et al., 2014). Additionally to the substrate mimic, PUFA catalysis in a boomerang-shaped fatty acid cavity was confirmed with coral 8(R)-LOX binding of AA under anaerobic conditions (Neau et al., 2014).
(to 3) Control of PUFA entry in head or tail orientation is regulated by highly conserved amino acids. Based on the model of a U-shaped substrate binding cavity, these amino acids determine the positioning of the fatty acid with its respective pentadiene opposite to the non-heme iron, making it suitable for the initial stereoselective hydrogen abstraction and the subsequent reaction with molecular oxygen (Newcomer and Brash 2015). The superimposition of AA onto the ligand-bound crystal structure of human ALOX15B indicated that only the entry of the substrate in a “tail first” orientation places the reactive pentadiene close to the catalytic iron of ALOX15B (Kobe et al., 2014). This concept was supported by the analysis of the generated product profile of ALOX15B-catalyzed oxygenation of phosphatidylcholine (PC)-esterified AA (Bender et al., 2016). The exclusive detection of 15(S)-HETE-PC indicated that fatty acids enter the active site of ALOX15B with their carbon tail first since the bulky PC head group does not allow for another orientation (Coffa and Brash 2004).
Moreover, free energy simulations confirmed the “tail first” orientation of substrate entry into the active site of ALOX15B (Suardíaz et al., 2016). Calculations indicated an abstraction of the pro-S H13 hydrogen from C13 of AA, therefore only allowing the “tail first” entry and the exclusive generation of 15-HpETE by ALOX15B. Interestingly, mutation of single amino acids to the corresponding amino acids in mouse Alox8 changed the “tail first” orientation of ALOX15B towards the Alox8 typical “head first” substrate entry and vice versa (Jisaka et al., 2000). Moreover, this amino acid exchange converted the positional specificity from 8(S) to nearly exclusive generation of 15(S) (Bender et al., 2016). Recently, these in vitro mutagenesis studies have been confirmed through the generation of Alox15b knock-in mice, in which the reported amino acids of Alox8 were substituted by those from human ALOX15B. As these humanized Alox15b knock-in mice formed only small amounts of 8-HETE, the substitution from Tyr603/His604 in Alox8 towards Asp602/Val603 in Alox15b confirms that these amino acid pairs are responsible for the different regiospecificity of the two enzymes (Schäfer et al., 2022).
ALOX15B reaction specificity
Mammalian LOX enzymes are best studied for their peroxidation of free PUFA substrates including the biologically most abundant omega-6 (ω-6) PUFAs AA (C20:Δ4) and LA (C18:Δ2) as well as the less abundant ω-3 PUFAs EPA (C20:Δ5) and DHA (C22:Δ6) (Kühn et al., 1993; Kutzner et al., 2017). Although named after their concordant oxygenation of AA at C15 (Kühn et al., 2015), 15-LOX orthologs exhibit variable substrate reaction specificities. In contrast to human ALOX15, which was described to not only catalyze the formation of AA to 15(S)-HpETE but also 12(S)-HpETE (Bryant et al., 1982; Kutzner et al., 2017), ALOX15B exclusively produces 15(S)-HpETE from AA (Brash 1999; Kilty et al., 1999; Jacquot et al., 2008; Kutzner et al., 2017). Using purified recombinant enzyme preparations, singular positional specificity of human ALOX15B was also reported for EPA and DHA oxygenation and in simultaneous incubation of ALOX15B with a mixture of substrate PUFAs the singular positional oxygenation specificity of ALOX15B was maintained (Kutzner et al., 2017) (Figure 2). Moreover, a general preference of 15-LOX orthologs for PUFAs with a higher degree of unsaturation was detected in the used experimental conditions and DHA was indicated as the overall preferred PUFA substrate between AA, EPA and DHA (Kutzner et al., 2017). Product analyses of AA and LA oxygenation showed that AA was preferred over LA (Brash 1999; Kutzner et al., 2017) and LA over γ-linolenic acid (GLA, 18:Δ3) (Kutzner et al., 2017). Contrarily, also using purified enzyme, other groups reported a substrate preference of ALOX15B for LA in direct comparison with AA (Kilty et al., 1999; Wecksler et al., 2008). However, the described substrate preference of the isolated protein is reported to differ from the specificity in intact macrophages. There, 50% less 15-LOX catalyzed DHA oxygenation products were identified than monohydroxylated fatty acids derived from AA and EPA (Ebert et al., 2020). As mentioned by Ebert et al., 2020, the reaction specificity of LOXs in intact cells is potentially affected by the availability of the substrate, the substrate specificity of other lipid-mediator generating enzymes and the presence of fatty acid liberating phospholipases, which make it difficult to reconcile a clear substrate specificity of LOX enzymes in the cellular system with those of isolated enzyme.
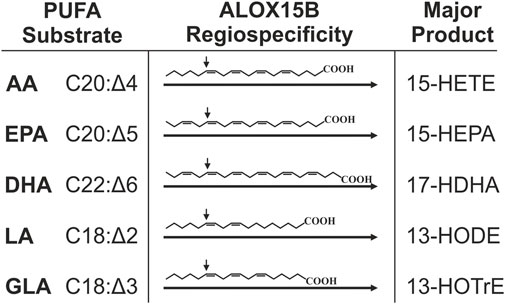
FIGURE 2. Major products derived from PUFA oxygenation through ALOX15B. Singular reaction specificity toward various PUFA substrates was demonstrated for recombinant and purified ALOX15B but also in cellular systems this was apparent. This figure summarizes major hydroperoxides generated by ALOX15B-catalyzed oxygenation of ω-6 PUFAs AA (arachidonic acid), LA (linoleic acid) and GLA (γ-linoleic acid) as well as ω-3 fatty acids EPA (eicosapentaenoic acid) and DHA (docosahexaenoic acid). AA is mainly transformed to 15-hydroxyeicosatetraenoic acid (15-HETE), EPA to 15-hydroxyeicosapentaenoic acid (15-HEPA), DHA to 17-hydroxydocosahexaenoic acid (17-HDHA), LA to 13-hydroxyoctadecadienoic acid (13-HODE), and GLA to 13-hydroxyoctadecatrienoic acid (13-HOTrE). The arrow indicates the site of S-stereospecific oxygen insertion into the respective PUFA mediated by ALOX15B.
Among all LOX enzymes, oxygenation of ester lipid-bound PUFAs (Schewe et al., 1975; Kühn et al., 1990; Coffa and Brash 2004) and lipoproteins (Belkner et al., 1993; Kühn et al., 1994; Belkner et al., 1998) is a peculiar characteristic specific to 15-LOX orthologs. In line with its reported singular reaction specificity of free substrate PUFAs, recombinant ALOX15B was found to oxygenate phospholipid (PL)-esterified AA exclusively to 15-HETE (Coffa and Brash 2004; Bender et al., 2016). Recalling the suggested Ca2+-dependent membrane association, membrane-bound activity of ALOX15B has been shown by incubation of exogenous ALOX15B with PUFA-enriched HEK293 lysates. Addition of exogenous phospholipase A2 (PLA2) to heat-inactivated ALOX15B massively liberated 15-HETE, pointing toward an ALOX15B-generated membrane PL-esterified product, generated from the esterified substrate. However, data from cellular systems are lacking as no conclusive discrimination between free and esterified product could be made from experiments using HEK293 cells expressing ALOX15B (Bender et al., 2016).
Inhibition of ALOX15B
The only inhibitors known to date that specifically inhibit ALOX15B with micromolar potency were synthesized based on the concept of a U-shaped substrate binding cavity (Jameson et al., 2014; Tsai et al., 2021a). The respective inhibitors (MLS000327069, MLS000327186, and MLS000327206) are described to have a 50-fold higher selectivity for ALOX15B versus human ALOX5, ALOX12, ALOX15, cyclooxygenase (COX)-1 and COX-2, without affecting redox activity (Tsai et al., 2021a). Besides recombinant enzyme preparations, inhibition of ALOX15B was studied in a cell assay that used ALOX15B-overexpressing HEK293T cells. The cells were collected, diluted, incubated with the respective inhibitor for 20 min at 37°C, following stimulation with CaCl2 (100 µM), calcium-Ionophore A23487 (5 µM) and AA (1 µM). Proof of ALOX15B inhibition was assessed by measurement of 15-HETE levels. In addition to these ALOX15B-specific inhibitors, other compounds have been ascribed an inhibitory function for ALOX15B. Apart from the universal LOX and COX inhibitor nordihydroguaiaretic acid (NDGA) (Vasquez-Martinez et al., 2007; Kakularam et al., 2022), PD146176 (Abrial et al., 2015) and distinct flavonoids (Vasquez-Martinez et al., 2007; Mascayano et al., 2013) have been described to inhibit ALOX15B activity.
Product-regulation of ALOX15B enzyme function
In normal human prostate epithelial cells (NHP), which basally express ALOX15B, Ca2+-induced membrane association of ALOX15B massively increased enzyme activity, possibly due to improved enzyme stabilization through membrane or substrate binding (Kilty et al., 1999). However, regulation of enzyme activity and substrate specificity of LOX enzymes has been shown to occur not only through substrate binding but also through their own hydro(pero)xide products. Incubation with 15-HETE (Kilty et al., 1999) and AA-derived hydroperoxides 15-HpETE and 12-HpETE as well as LA-derived 13-hydroperoxyoctadecadienoic acid (13-HpODE) (Wecksler et al., 2008) have been described to activate ALOX15 and ALOX15B. Mechanistically, it was suggested that the hydroperoxides increase Fe2+ oxidation, therefore enabling a faster transition towards the active enzyme status after substrate oxygenation (Jones et al., 1996; Solomon et al., 1997; Wecksler et al., 2008). Moreover, addition of 13-HpODE changed the substrate specificity of purified recombinant ALOX15B and ALOX15, whereas incubation with 12-HpETE solely affected ALOX15 and 15-HpETE mediated only little effects on substrate specificity of both enzymes. Since the reduced products 13-hydroxyoctadecadienoic acid (13-HODE) and 12-HETE also altered the substrate specificity, it was excluded that the change in iron oxidation mediates substrate specificity as it was proposed for enzyme activity (Wecksler et al., 2008). Based on this highly selective effect of LOX products, a role in allosteric regulation of ALOX15 and ALOX15B with direct effects on substrate specificity were proposed for 13-HpODE and 12-HpETE (Wecksler et al., 2008; Offenbacher and Holman 2020). Further research showed that binding of 13-HODE eliminated the kinetic dependency on the hydrogen bonding rearrangement in ALOX15B. Binding to the allosteric site, which was suggested to be at histidine H627, induced a conformational change in ALOX15B and thereby an increase in substrate specificity, likely by adjusting the enzyme to a catalytically competent state (Wecksler et al., 2009). It was concluded that very small amounts of 13-HODE shift the enzyme’s substrate specificity from AA towards LA and thereby promote a positive feedback loop of 13-HODE generation (Wecksler et al., 2009).
Further on, a pH-dependence and the implication of the PLAT domain in substrate specificity was reported for ALOX15B (Joshi et al., 2013). For example, ALOX15B was found to favor GLA at physiologically relevant pH but AA at slightly elevated pH values. Since the addition of 13-HODE induced the reaction of ALOX15B with AA but inhibited the reaction with GLA, an increased preference towards AA for both pH conditions was concluded (Joshi et al., 2013), which was supported by the findings of Kutzner et al. (2017). Of note, previous reports also demonstrated a controversial suicidal self-inactivation of LOXs through their own products. For example, 15-HpETE and 13-HpODE have been shown to mediate the inactivation of mammalian 15-LOXs (Rapoport et al., 1986; Gan et al., 1995). In this context, oxidation of LOX active site residues by radical intermediates from the catalytic fatty acid peroxidation was proposed as a potential mechanism (Hoobler et al., 2013). With regard to rabbit Alox15 it was shown that 15-HpETE induces a covalent modification of rabbit Alox15, leading to an inactive enzyme (Wiesner et al., 2003), which was confirmed later on by separation of proteolytic cleavage peptides (Kühn et al., 2005). As the molecular basis for suicidal enzyme inactivation has not been proven conclusively, the process of hydroperoxide-induced auto-inactivation remains unclear, especially for ALOX15B, where no suicidal self-inactivation has been reported so far.
Apart from the concept of allosteric regulation through LOX products, the allosteric regulation by enzyme monomers was proposed. In this concept, LOXs function as dimeric proteins with one monomer acting as an allosteric regulator of the other monomer (Ivanov et al., 2021). It was described that 13-HODE induces transient dimerization of rabbit Alox15 in aqueous solution (Ivanov et al., 2012). However, in contrast to rabbit Alox15, purified recombinant ALOX15B was mainly found in two monomeric conformers in aqueous solution but not as a dimer (Ivanov et al., 2021). So far, evidence is lacking whether ALOX15B monomers can act as allosteric regulators of the partner monomer.
Transcriptional regulation of ALOX15B
Transcriptional regulation of ALOX15B has been reported to occur through a TATA less promoter (Tang et al., 2007). However, binding sites of zinc finger transcription factors specificity protein 1 and 3 were also detected within the ALOX15B promoter, and have been shown to positively and negatively regulate transcription of ALOX15B (Figure 3A) (Tang et al., 2004).
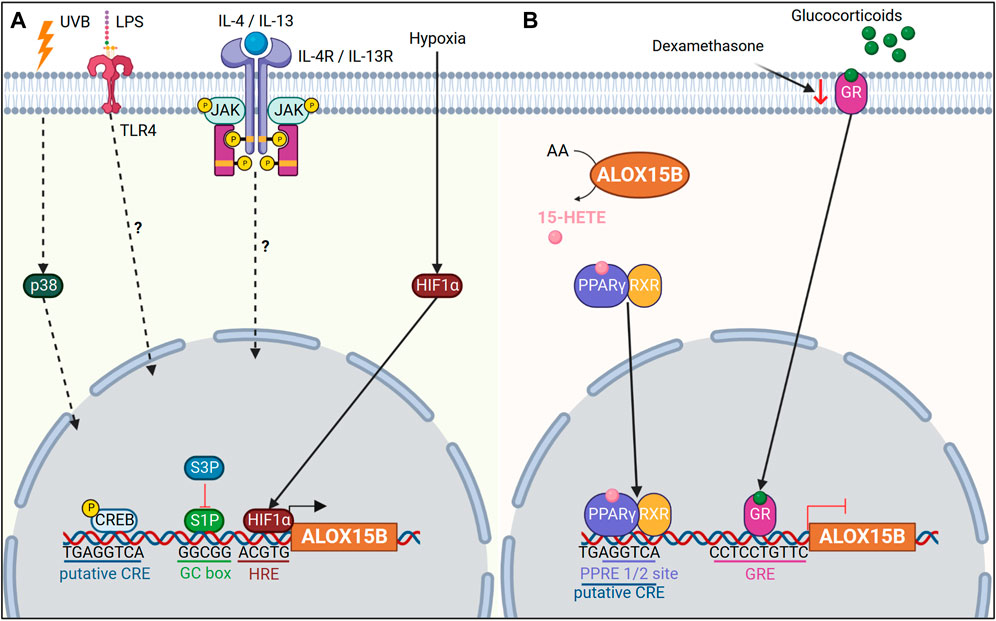
FIGURE 3. Transcriptional regulation of ALOX15B. (A) Ultraviolet B (UVB) radiation upregulates ALOX15B expression via mitogen-activated protein kinase 11 (p38). Lipopolysaccharide (LPS) and interleukin (IL)-4/IL-13 upregulate ALOX15B expression in macrophages via an unknown mechanism. Hypoxia inducible factor 1 subunit alpha (HIF1α) increases ALOX15B expression under hypoxic conditions. The ALOX15B promoter contains a putative cAMP response element (CRE) binding site, in addition to GC box binding sites for specificity protein 1 (S1P). S1P positively regulates ALOX15B transcription, while S3P regulates negatively. (B) ALOX15B activates peroxisome proliferator activated receptor gamma (PPARγ) via 15-HETE, which in turn negatively regulates ALOX15B transcription. A peroxisome proliferator response element (PPRE) half-site in the ALOX15B promoter overlaps with the putative CRE site. Binding of glucocorticoids to their receptor initiates binding to the glucocorticoid response element (GRE) located within the ALOX15B promoter, thus inhibiting expression of ALOX15B. Dexamethasone, a glucocorticoid, reduces expression of the glucocorticoid receptor (GR) and upregulates ALOX15B expression.
In addition, interleukin (IL)-4/IL-13 cytokines or lipopolysaccharide (LPS) stimulation induced ALOX15B expression in macrophages (Wuest et al., 2012; Snodgrass et al., 2018). Yet, the exact mechanism of how IL-4/IL-13 or LPS induce ALOX15B remains to be uncovered. One should also keep in mind that the cytokine concentration used for enzyme induction in vitro differ significantly from those found under physiological conditions (Kühn et al., 2016). One potential molecular mechanism for enzyme induction seems to be through cAMP response element binding protein (CREB) signaling, mediated via toll like receptor 4 (TLR4) (Wen et al., 2010). In line, Subbarayan et al. (2006) reported a putative cAMP response element (CRE) in the promoter of ALOX15B, and treatment of NHP cells with dibutryryl cAMP increased ALOX15B expression. However, it should be noted that direct binding of phosphorylated CREB to the promoter of ALOX15B has not been confirmed and other nuclear receptor proteins, which are associated with IL-4/IL-13 or LPS signaling cannot be ruled out.
In human macrophages hypoxia-induced ALOX15B expression has also been reported in addition to a positive correlation of ALOX15B expression with hypoxia inducible factor 1 subunit alpha (HIF1α) in carotid plaques (Rydberg et al., 2004; Hultén et al., 2010). Furthermore, dimethyloxalylglycine, which mimics hypoxic conditions through stabilization of HIF1α, induced ALOX15B expression (Hultén et al., 2010). Likewise, ALOX15B expression was also increased under hypoxic conditions in cardiac fibroblasts (Sandstedt et al., 2018), pulmonary artery smooth muscle and endothelial cells (Liu et al., 2009), as well as lung cancer cell line A549 (Yang et al., 2018). However, in human retinal microvascular endothelial cells a reduced ALOX15B expression was observed when cells were exposed to hypoxia (Bajpai et al., 2007). In silico analysis of the ALOX15B promoter indicated three potential binding sites for HIF1α (Hultén et al., 2010) and computational analysis revealed the location of a hypoxia response element “ACGTG” immediately upstream of the transcription start site in the ALOX15B promoter. Yet conclusive evidence needs to be performed to see if direct binding of HIF1α to the ALOX15B promoter occurs or if an indirect mechanism induces ALOX15B expression under hypoxia.
As reported previously, ALOX15B products have also been shown to regulate enzyme activity (Rapoport et al., 1986; Gan et al., 1995; Kilty et al., 1999). In particular, AA-derived 15-HETE has been associated with a negative feedback loop in the regulation of the nuclear receptor peroxisome proliferator activated receptor gamma (PPARγ) (Subbarayan et al., 2006). 15-HETE, a ligand for PPARγ, was found to initiate translocation of PPARγ to the nucleus and transcription of PPARγ target genes (Sun et al., 2015). In accordance, a peroxisome proliferator response element half-site was described to be located -560 to -596 base pairs upstream of the ALOX15B transcription start site, overlapping with a putative CRE (Subbarayan et al., 2006). Subbarayan et al. (2006) speculated that either binding of PPARγ to the peroxisome proliferator response element half-site may therefore inhibit CREB binding to the ALOX15B promoter, thus inhibiting transcription of ALOX15B or cooperative interaction of these transcription factors may occur. Alternatively, nuclear factor kappa B (NFκB)-mediated transcription has been shown to be inhibited through PPARs (Wang et al., 2007). Although human ALOX15B has not been previously linked with NFκB, upregulation of murine Alox8 has been reported for IκBα (inhibitor of NFκB subunit alpha) deficient mice (Schneider et al., 2004).
Expression of ALOX15B has been found in the epithelium of steroidogenic tissues such as prostate (Tang et al., 2007), breast (Wang et al., 2017; Vasiliou et al., 2022), endometrium (Russell et al., 2011) and skin (Brash et al., 1997) (Table 1). Given that dysregulation in steroidogenesis is associated with cancer and other diseases, distinct and cell specific alterations in ALOX15B expression have been linked to various pathologies. Whereas treatment of breast cancer cell lines with dihydroxytestosterone (DHT) upregulated ALOX15B expression (Vasiliou et al., 2022), treatment of prostate cancer with DHT did not induce ALOX15B expression, although a partially matched androgen response element (ARE) was described to be located within ∼1.4 kb upstream of the ALOX15B transcription start site (Tang et al., 2004). These data correlate with the high androgen receptor activity and reduced expression of ALOX15B found in prostate cancer (Tang et al., 2007). Indeed, a glucocorticoid response element (GRE) was found within the promoter region of ALOX15B (Feng et al., 2010), and overexpression of the glucocorticoid receptor (GR) decreased ALOX15B transcription (Figure 3B). Moreover, inhibition of GR through siRNA, RU486 or dexamethasone, lowered GR expression in prostate cancer cells and restored ALOX15B expression (Feng et al., 2010). Also, expression of ALOX15B positively correlated with estrogen receptor (ER) expression in unilateral breast cancer tumors, however transcription of ALOX15B was significantly higher in ER negative breast tissue from the contralateral normal breast tissue (Wang et al., 2017). Interestingly, γ-tocotrienol, a member of the vitamin E family, has also been shown to induce ALOX15B expression (Campbell et al., 2011), whereas vitamin E treatment inhibited ALOX5 (Pein et al., 2018) and ALOX15 (Hinman et al., 2018).
ALOX15B in inflammation and disease
Pro-resolving lipid mediators
Regulation of inflammation is precisely controlled through diverse messengers including bioactive lipid mediators derived from LOX- or COX-mediated PUFA oxygenation (Serhan et al., 2000; Buckley et al., 2014; Schebb et al., 2022). Apart from 15-LOX generated monohydroxy fatty acids, which have been described to exhibit biological activity (Ivanov et al., 2015), 15-LOX orthologs have been implicated in the synthesis of anti-inflammatory and pro-resolving lipid mediators such as AA-derived lipoxins (lipoxygenase interaction products) (Adel et al., 2016). Lipoxins (LXs) constitute, together with EPA-derived E-series Rv, and DHA-derived D-series Rvs, protectins, and maresins the class of specialized pro-resolving lipid mediators (SPMs) (Serhan et al., 2002; Bannenberg et al., 2005; Serhan et al., 2009; Spite et al., 2009; Buckley et al., 2014; Serhan and Levy 2018). The biosynthesis of SPMs is thought to occur as a transcellular process that involves a first and a second oxygenation of the fatty acid substrate involving several LOX isoforms such as ALOX5, ALOX12, ALOX15 and ALOX15B (Schebb et al., 2022) as well as the 5-lipoxygenase activating protein (FLAP) (Lehmann et al., 2015).
The initial peroxidation of AA to 5-HpETE by ALOX5 or to 15-HpETE by ALOX15/ALOX15B forms the early precursors of LX synthesis. Using isolated and purified enzyme preparations, ALOX15B has been shown to oxygenate 5-HpETE and 5-HETE to the LX intermediate 5,15-dihydroperoxyeicosatetraenoic acid (5,15-diHpETE), which can also be generated through the ALOX5-mediated oxygenation of 15-HpETE (Green et al., 2016). Remarkably, biosynthetic kcat/KM flux analysis revealed that ALOX15B-catalyzed conversion of 5-H(p)ETE to 5,15-HpETE happened with a 2-fold greater flux than through ALOX15 (Perry et al., 2020a). However, as ALOX15B did not further react with the LX precursor 5,15-diHpETE, no final LX generation was documented for ALOX15B (Green et al., 2016).
Similar to its implication in LX synthesis, ALOX15B was found to oxygenate 7-HDHA and 7-HpDHA almost exclusively at C17, forming RvD5 (7,17-diHDHA), again with faster kinetics than ALOX15 (Perry et al., 2020b) (Figure 4). In contrast to ALOX15B, peroxidation of 7-HpDHA by ALOX15 lead primarily (80%) to the product 7,14-diHDHA, underscoring the potential of ALOX15B in RvD5 formation. Based on these findings, Perry et al. (2020b) raised the question whether ALOX15B might play a larger role in SPM biosynthesis than previously thought. Although conclusive in experiments with isolated enzyme preparations or in cellular systems, the implication of ALOX15B in SPM formation is debated as well as compliance with methodological standards in the detection of SPMs (O'Donnell et al., 2021).
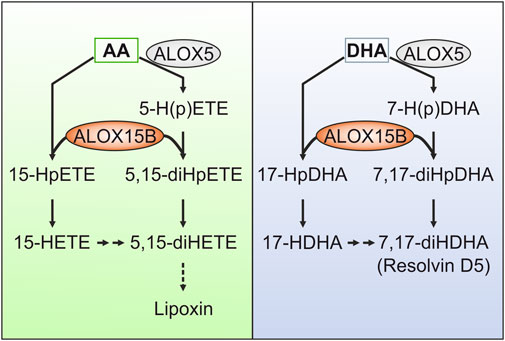
FIGURE 4. Contribution of ALOX15B in the formation of specialized pro-resolving lipid mediators (SPMs). Although debated, ALOX15B has been described to contribute to the formation of SPMs by generating mono- or dihydroxy fatty acid products that either directly perform pro-resolving functions or serve as intermediates of SPM synthesis. For example, lipoxins (LX) derive from arachidonic acid (AA), whereas docosahexaenoic acid (DHA) gives rise to resolvins (Rv). ALOX15B has been implicated in the oxygenation of ALOX5-derived AA metabolites 5-hydroperoxyeicosatetraenoic acid (HpETE) and 5-hydroxyeicosatetraenoic acid (HETE) to the LX intermediate 5,15-dihydroperoxyeicosatetraenoic acid (diHpETE). As ALOX15B did not further react with the LX precursor 5,15-diHpETE, no ultimate LX generation was attributed to ALOX15B. With respect to DHA-derived Rvs, ALOX15B was reported to catalyze the formation of the SPM precursor 17-hydroxyl-DHA (HDHA). Furthermore, ALOX15B-mediated oxygenation of ALOX5-derived 7-hydroperoxy-DHA (HpDHA) and 7-HDHA may stimulate the formation of RvD5 (7,17-diHDHA).
Polarization of macrophages to the anti-inflammatory M2 phenotype depends on the presence of the T-helper type 2 (Th2) cytokine IL-4 (Gordon and Martinez, 2010). As discussed earlier, in vitro stimulation of macrophages with IL-4 increased the expression of ALOX15 (Conrad et al., 1992) and ALOX15B as well as their products, 15-HETE and 13-HODE (Snodgrass et al., 2018). However, only silencing of ALOX15, but not ALOX15B, decreased the SPM precursors 15-HETE and 13-HODE to basal levels, hinting toward a small potential of ALOX15B in contribution to SPM synthesis from these hydroperoxides in primary human macrophages. Also, stimulation of macrophages with inflammatory stimuli lead to only minor detection of 15-LOX-derived lipid metabolites (Wuest et al., 2012; Russell et al., 2019; Werner et al., 2019). Given that ALOX15B is constitutively expressed in primary human macrophages and its expression is increased upon bacterial LPS treatment (Wuest et al., 2012; Snodgrass et al., 2018; Russell et al., 2019), the implication of ALOX15B in formation of SPM intermediates was questioned (Snodgrass and Brüne 2019). On the contrary, the stimulation of previously polarized M2-type macrophages with LPS induced a much higher release of 17-HDHA than of 14-HDHA (Ebert et al., 2020). For this, one has to keep in mind that it has been shown that ALOX15 generates equal amounts of 17-HDHA and 14-HDHA but ALOX15B forms almost exclusively 17-HDHA (Kutzner et al., 2017; Tsai et al., 2021b). Therefore, based on this high 17-HDHA/14-HDHA ratio in LPS-treated M2 macrophages the SPM precursor 17-HDHA is potentially primarily derived from ALOX15B (Ebert et al., 2020). Interestingly, only TLR stimulation of M2-polarized macrophages but neither of naïve nor pro-inflammatory M1-polarized macrophages led to a concerted increase of ALOX15B mRNA and protein above its basal level. In these cells, in which ALOX15 was expressed at a similar level as in control cells, the SPM intermediate 5,15-diHETE as well as RvD5 have been most abundant compared with all other conditions (Ebert et al., 2020). This is in accordance with the data of isolated ALOX15B protein that demonstrated a function of ALOX15B in the formation of the LX precursor 5,15-diHETE as well as RvD5 (Green et al., 2016; Perry et al., 2020a; Perry et al., 2020b). These data link ALOX15B to a contributing role in SPM synthesis, with a focus on the anti-inflammatory M2-type macrophages that helps to support resolution of inflammation after undergoing a priming pro-inflammatory phase. Data from tissues of children that suffer from the inflammatory lung disease cystic fibrosis suggest that decreased expression of ALOX15B is associated with low LXA4 levels (Ringholz et al., 2014) and that the activity of TLR4, which binds LPS, is crucial to maintain tissue homeostasis and to promote resolution of inflammation (Yang et al., 2012). Nevertheless, future studies are necessary to evaluate the role of ALOX15B-catalyzed SPM synthesis during inflammation and its resolution in man.
ALOX15B in atherosclerosis
Atherosclerosis is a multifactorial inflammatory disease, which progressively is driven by lipid retention and inflammation. In the arterial wall, macrophages accumulate oxidized low-density lipoproteins (oxLDL) and cholesterol esters, resulting in macrophage foam cells. Due to the growing atherosclerotic plaque size and thickening of the vessel wall, impaired diffusion gives rise to hypoxic areas (<1% O2) and the recruitment of various immune cells supports chronic inflammation (Koelwyn et al., 2018). Culture of primary human macrophages under hypoxic conditions promoted LDL oxidation and increased ALOX15B expression and activity (Rydberg et al., 2004). In line, ALOX15B staining was enhanced in atherosclerotic plaque tissue (Rydberg et al., 2004; Gertow et al., 2011) and correlated well with the staining of macrophage-rich areas and HIF1α (Hultén et al., 2010) (Figure 5A). Interestingly, ALOX15B mRNA levels were described to be higher in symptomatic plaques with attributable cerebrovascular symptoms (amaurosis fugax, transient ischemic attack, or stroke) than in asymptomatic plaques (Gertow et al., 2011). Supporting the data of its hypoxic regulation, stabilization of HIF1α provoked ALOX15B expression and the knockdown of HIF1α reduced ALOX15B activity (Hultén et al., 2010). Since transcriptional activity of HIF1α is associated with an inflammatory plaque phenotype (Vink et al., 2007), release of pro-inflammatory signal molecules was assessed. Indeed, macrophages overexpressing ALOX15B increased the secretion of chemokine (C-X-C motif) ligand 10 (CXCL10) and C-C motif chemokine ligand 2 (CCL2) as well as activation and migration of T-cells (Danielsson et al., 2008). Accordingly, knockdown of ALOX15B decreased macrophage lipid accumulation as well as pro-inflammatory cytokine secretion (Magnusson et al., 2012). This was confirmed by silencing of mouse Alox8, which decreased plaque lipid content, plaque localized T-cell number and inflammation markers in LDL-receptor deficient mice, therefore linking ALOX15B to a pro-atherogenic role by impacting foam cell formation, immune cell recruitment and local inflammation (Magnusson et al., 2012). In line, data of a case-control study indicated the association of distinct ALOX15B gene polymorphisms with coronary artery disease (Wuest et al., 2014) and patients diagnosed with stroke were found to have high expression of ALOX15B (Vijil et al., 2014). Also, ALOX15B expression in human carotid plaques was associated with thrombus formation along with in vitro increased platelet aggregation and thrombus formation mediated by ALOX15B products 15-HpETE and 15-HETE (Vijil et al., 2014). Concurringly, knockdown of ALOX15B in macrophages reduced 15-HETE formation and decreased platelet aggregation and thrombin generation. Interestingly, and in contrast to 15-HETE, metabolites 7,14-diHDHA and RvD5 share potent anti-aggregation properties and therefore were proposed to directly regulate clot resolution (Perry et al., 2020b) and also 17-HpDHA demonstrated anti-aggregation properties against isolated human platelets (Tsai et al., 2021b). As all three bioactive lipid mediators were generated by ALOX15B, an ambiguous role for ALOX15B in clot formation can be assumed.
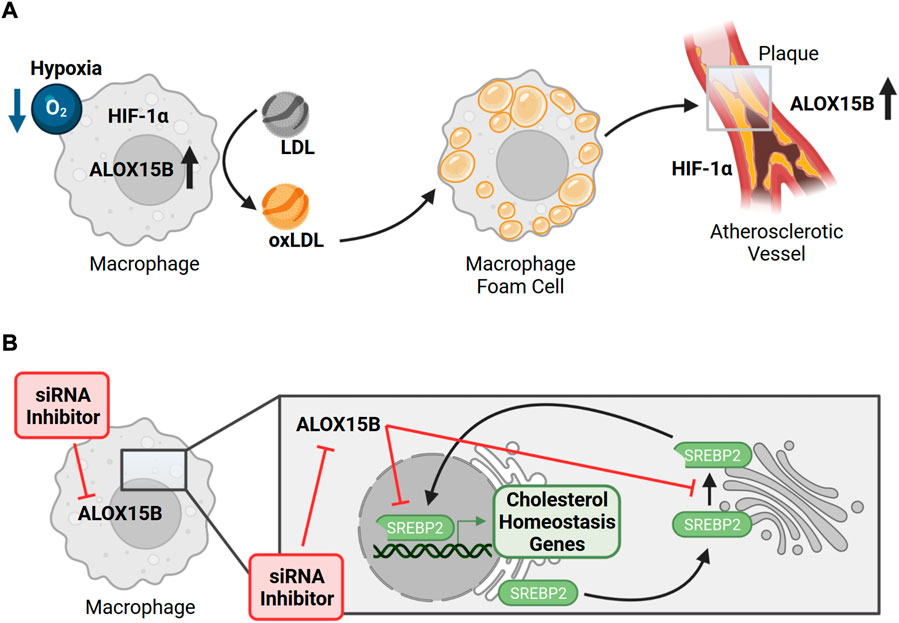
FIGURE 5. ALOX15B in atherosclerosis and its implication on macrophage cholesterol homeostasis. (A) Hypoxic conditions of primary human macrophages promote oxidation of low-density lipoprotein (LDL → oxLDL) and an increase of ALOX15B expression and activity. In the arterial wall, macrophages accumulate oxLDL as cholesterol esters, provoking the formation of macrophage foam cells, which can assemble into an atherosclerotic plaque. Due to the thickening of the vessel wall, reduced diffusion gives rise to hypoxic areas. Atherosclerotic plaque tissue (grey box) is associated with higher expression of ALOX15B, which correlates with the staining of macrophage-rich areas and hypoxia inducible factor 1 subunit alpha (HIF1α). While stabilization of HIF1α increases ALOX15B expression, overexpression of ALOX15B induces the secretion of chemokines and migration of T-cells, which play important roles in atherogenesis. (B) In primary human macrophages inhibition as well as silencing of ALOX15B impairs cellular cholesterol homeostasis by limiting maturation of the major transcription factor sterol regulatory element binding protein 2 (SREBP2) as well as its binding to target gene promotors. Therefore, suppressing ALOX15B in macrophages reduces expression of genes implicated in endogenous cholesterol biosynthesis and cholesterol uptake, which is confirmed by attenuated levels of cholesterol biosynthetic intermediates.
Rupture of the atherosclerotic plaque, which can clot the vessel and thereby lead to thrombosis, stroke or heart attack, is promoted by a high blood pressure. Besides its atherogenic role, ALOX15B was suggested as a driver of pulmonary hypertension (Shen et al., 2013) and has been described to induce over-proliferation of pulmonary artery endothelial cells (PAECs) (Zhang et al., 2018). In PAECs, hypoxia-induced ubiquitination of ALOX15B was proposed to initiate the sorting of ALOX15B into exosomes. Upon subsequent secretion, they were thought to trigger signal transducer and activator of transcription 3 (STAT3)-dependent proliferation of PAECs, promoting a dysfunctional and abnormal remodeling of the pulmonary vascular homeostasis. In line, hypoxia-induced expression of ALOX15B and production of its metabolite 15-HETE promoted proliferation of rabbit pulmonary artery smooth muscle cells, likely through the mitogen-activated protein kinase 3/1 (ERK1/2) mitogen-activated protein kinase (MAPK) pathway (Shan et al., 2012). Again, this sheds light on ALOX15B as a potential participant in hypoxia-induced pulmonary hypertension. In line, expression of ALOX15B was detected in fibroblasts isolated from hearts of healthy donors or donors suffering from chronic heart failure and its expression was increased upon hypoxia (Sandstedt et al., 2018). Collectively, these data demonstrate a role of ALOX15B in cardiovascular inflammation and pathophysiology. More research of ALOX15B as a driver of cardiovascular events is warranted.
ALOX15B in cellular cholesterol homeostasis
In the pathophysiological context of atherosclerosis macrophages are confronted with the task to restore cellular cholesterol homeostasis. As phagocytes, macrophages eliminate pathogens and pathogen-infected mammalian cells and contribute to the maintenance of tissue homeostasis by eliminating apoptotic cells, named efferocytosis (Arandjelovic and Ravichandran, 2015). During cell clearance, macrophages take up immense levels of surplus lipids. To achieve cellular cholesterol homeostasis as quickly as possible, excess sterol intermediates simultaneously activate liver X receptor (LXR) target genes, which induce cholesterol export, and suppress sterol regulatory element binding protein 2 (SREBP2)-dependent genes to inhibit endogenous cholesterol biosynthesis (Spann et al., 2012; Snodgrass et al., 2021). Regarding macrophage cholesterol homeostasis, we recently reported that silencing ALOX15B in primary human macrophages reduces maturation of SREBP2 to an active transcription factor, binding of SREBP2 to sterol regulatory elements, and SREBP2-dependent gene expression (Snodgrass et al., 2018) (Figure 5B). In accordance, suppression of ALOX15B lowered cellular cholesterol, sterol intermediates lanosterol, 24,25-dihydrolanosterol and desmosterol as well as 27-hydroxycholesterol. During IL-4 treatment of macrophages, knockdown of ALOX15B and, to a lesser extent ALOX15, lowered the production of CCL17 in a SREBP2-dependent manner, thereby reducing the migration of CCR4 (CCL17 receptor)-expressing T-cells (Snodgrass et al., 2018). Analysis of datasets from human bronchoalveolar lavage cells isolated from control and asthma patients revealed high expression of ALOX15B, CCL17, and SREBP2-dependent genes in severe asthmatics compared with healthy donors and those with moderate asthma, implicating ALOX15B and SREBP2 in a pathophysiological relevant context.
Based on these findings, we continued to study the role of ALOX15B in macrophage cholesterol homeostasis. Based on whole transcriptome analyses and verification at both mRNA and protein levels, we confirmed our previous findings of reduced SREBP2 target gene expression in ALOX15B-silenced macrophages. In addition, suppression of ALOX15B disrupts intracellular cholesterol distribution in primary human macrophages. Thus, our data will add to the limited information available on the biological function of ALOX15B in primary human macrophages through modulating intracellular cholesterol homeostasis. These data may help to clarify the impact of ALOX15B expression in cholesterol-related diseases such as atherosclerosis.
ALOX15B in inflammatory lung diseases
Recently the role of ALOX15 in inflammatory airway diseases was reviewed (Xu et al., 2021). The authors state that ALOX15 plays a greater importance than ALOX15B in the respiratory system. Indeed, a Th2 cytokine response and thus ALOX15 expression, as shown for A549 cells (Han et al., 2014), is present in the pathology of asthma. Nevertheless, Gras et al. (2012) report significantly higher ALOX15B expression in 3D cultures of airway epithelial cells grown at the air liquid interphase from severe asthma patients when compared to mild asthmatics, whereas ALOX15 revealed a non-significant small increase. Furthermore, 15-HETE was found to be secreted from the basolateral side of air liquid interphase experiments (Jakiela et al., 2013), which may be indicative of a function in reducing immune cell infiltration. In horses, an increase in Alox15b expression in inflammatory airway disease has also been reported (Ramery et al., 2015). In contrast, Planagumà et al. (2008) describe the reduction of ALOX15B expression in bronchoalveolar lavage cells, endobronchial lung biopsies and peripheral blood from patients with severe asthma, compared to non-severe asthma. However, significantly higher 15-HETE was secreted and present in the cellular plasma membrane of asthmatic patients (Profita et al., 2000).
In addition to type II pneumocytes, resident lung macrophages express ALOX15B (Gonzalez et al., 2004). Indeed, a correlation of 15-HETE and macrophages in sputum samples has been observed (Profita et al., 2000). Additionally, 15-LOX PUFA metabolites may also play a role in lung inflammation, as extracellular 13-HODE increased in asthmatic airways (Mabalirajan et al., 2013). Moreover, supplementation of the LA metabolite 13-HODE induced mitochondria dysfunction in bronchial epithelial Beas-2B cells (Mabalirajan et al., 2013). However, it should be noted that high concentrations (25 μM) of 13-HODE were added to these cells.
In contrast, cystic fibrosis patients showed a significant decrease in ALOX15B in macrophages and neutrophils of bronchoalveolar lavage (Ringholz et al., 2014). This reduction was associated with a lower ratio of LXA4:LTB4 as well as LXA4:IL-8, which the authors linked to impaired resolution of inflammation. Moreover, the authors suggested that reduced ALOX15B expression may be due to the cystic fibrosis gene CF transmembrane conductance regulator (CFTR), impairing macrophage function. Indeed, Shum et al. (2022) also report reduced ALOX15B expression in cystic fibrosis patients, although no significant reduction in 15-HETE was observed, lower LXA4 was apparent. As LXA4 was in much greater abundance than 15-HETE in these samples, it may be indicative of a predominate role of ALOX15B in metabolizing 5-HETE in these nasal polyp and mucosa samples. Furthermore, cells with F508del CFTR [a phenylalanine deletion common in cystic fibrosis patients (Nieddu et al., 2013)] demonstrated a further reduction of ALOX15B expression when compared to the WT CFTR expression in CFBE41o− bronchial epithelial cells (Shum et al., 2022). In addition, DHA supplementation in cystic fibrosis patients lowered 15-HETE in addition to LTB4 levels, along with decreasing the 15-HETE:17-HDHA ratio (Teopompi et al., 2019).
The contrasting ALOX15B expression levels between asthma and cystic fibrosis may be explained by the vastly different pathologies of these diseases. Indeed, both diseases have dysregulated airway epithelium, however, the increased Th2 cytokines in asthma could have potentially upregulated ALOX15B in both macrophages and airway epithelial cells. Furthermore, asthma symptoms involve shortness of breath resulting from a narrowing of the airway, whereas cystic fibrosis implicates impaired mucosal clearance (Fahy and Dickey 2010; Carlier et al., 2021). Interestingly, Teopompi et al. (2019) reported reduced levels of 15-HETE in chronic obstructive pulmonary disease (COPD) when compared to cystic fibrosis, which may be indicative of reduced 15-LOX enzyme levels. However, very few investigations into chronic inflammatory airway diseases have been conducted and further research is needed to fully understand the role of ALOX15B in the respiratory system.
ALOX15B in inflammatory skin diseases
The importance of lipids in cutaneous biology is underscored by the function in the permeability barrier, in which PUFAs are integral components (Kendall et al., 2017; Simard et al., 2019). Indeed, ALOX12B and ALOXE3 have been shown to be crucial to corneocyte lipid envelope through incorporation of ω-hydroxy-ceramides (Zheng et al., 2011). In addition, lipids have been described to modulate cellular signaling, differentiation and inflammation (Krieg and Fürstenberger 2014). The mechanism of ALOX15B regulation in skin inflammation remains unclear, however, there are some suggestions pointing to a pro-resolution function.
Transcriptome analysis comparing normal human and psoriasis skin biopsies revealed lower ALOX15B expression in the disease state (Li et al., 2014), furthermore an additional study also detected a decrease in ALOX15B expression in both lesional and non-lesional psoriasis samples (Gudjonsson et al., 2009). However, AA and LA metabolites 15-HETE and 13-HODE were increased in both total and free form in lesional psoriasis (Camp et al., 1983; Fogh et al., 1987; Fogh et al., 1989b; Sorokin et al., 2018; Takeichi et al., 2019; Tyrrell et al., 2021). Additionally, 15-HETE was elevated in a rat imiquimod psoriasis model, which was reversed by dexamethasone (Pipper et al., 2019). In contrast, Grøn et al. (1993) reported a significant reduction of 15-HETE and 13-HODE, but it should be noted that this only taken from 0.2 mm psoriatic scales. Moreover, intralesional injection of 15-HETE both improved psoriasis and upregulated 15-HETE production (Fogh et al., 1988).
Fogh and Kragballe (2000) speculated on potential mechanisms how 15-LOX products may play a role in the resolution of psoriasis. Firstly, the incorporation of 15-HETE or 13-HODE into phospholipids may suggest a role in cellular signaling. Protein kinase C-β inhibition has been previously shown by 13-HODE incorporation into diacylglycerol (DAG) (Cho and Ziboh 1994a; Cho and Ziboh 1994b; Cho and Ziboh 1994c). Secondly, the increased levels of 15-HETE found in psoriatic plaques and lesions may inhibit the formation of LTB4, initiating a switch from pro-to anti-inflammatory lipid mediators (Fogh and Kragballe 2000).
Interestingly, 15-HETE was found to be increased in the dermis of skin equivalent formed from psoriatic keratinocytes when compared to skin equivalents from healthy patients. Furthermore, the elevated 15-HETE was reduced by the addition of α-linolenic acid (ALA) along with reducing epidermal thickness (Simard et al., 2022). In addition to the epidermis, production of 15-HETE was reported to be lower from the dermis of uninvolved psoriatic skin compared to normal (Kragballe et al., 1986). Indeed, ALOX15 has been reported to be absent from both the epidermal and dermal compartments of normal human skin and a low level of ALOX15B was reported to be present in the dermis. Human skin equivalents (Simard-Bisson et al., 2018) and cultured human dermal fibroblasts have also been shown to express ALOX15 (Kubat et al., 2015). Which cells or 15-LOX enzyme contribute to the formation of 15-HETE in the dermis in situ remains unclear and upregulation of 15-LOX enzymes may occur from in vitro culture. Nevertheless, 15-HETE is associated with a positive correlation of collagen, laminin and fibronectin, and 13-HODE with collagen and laminin in human skin equivalents (Simard et al., 2022).
Although psoriasis is predominately a T-cell mediated disease, infiltration of monocytes/macrophages has been well characterized (Vestergaard et al., 2004; Kamata and Tada 2022). A significant increase in 15-HETE was detected in monocytes from patients with psoriatic arthritis (Wójcik et al., 2019). Epidermal expression of ALOX15B has been detected in normal human skin, as well as keratinocytes grown in culture (Shappell et al., 2001a; Setsu et al., 2006). Treatment of normal human epidermal keratinocytes and the keratinocyte cell line HaCaT with interferon-γ (IFNγ) (Setsu et al., 2006) or tumor necrosis factor-α (TNFα) in normal human epidermal keratinocytes (Banno et al., 2004) induced ALOX15B expression, indicating immune cell infiltration and inflammation may both induce epidermal ALOX15B in inflammatory skin diseases along with macrophage ALOX15B expression aiding in the production of oxylipins.
Alox8 expression is absent from normal murine skin, but can be induced by treatment with phorbol esters (Krieg et al., 1998; Heidt et al., 2000; Kim et al., 2005). Muga et al. (2000) generated skin specific Alox8 transgenic mice using a loricrin expression vector and these mice exhibited an increased keratin 1 (K1) expression. Although an increase in proliferation was observed, epidermal thinning was also reported. In line, 8(S)-HETE treatment induced K1 expression in WT keratinocytes, which was shown to be mediated by PPARα (Muga et al., 2000). Thuillier et al. (2002) demonstrated that both 8- and 15-HETE induce K1 expression in murine keratinocytes, in addition to activation of PPARγ and δ. Inhibition of LOXs with NDGA was shown to block PPRE and decrease PPARγ levels. Likewise, 13-HODE treatment in murine keratinocytes induced K1 expression, which was shown to be in a NFκB dependent mechanism (Ogawa et al., 2011). Where concentrations of 30 nM 13-HODE were enough to induce K1 and NFκB activity, this did not show significant differences in PPARγ activity (Ogawa et al., 2011). Furthermore, inhibition of NFκB with SN50 inhibited 13-HODE mediated K1 expression. As PPARγ is known to inhibit NFκB activity, it is unclear if 15-HETE induced K1 expression is mediated through a mechanism independent of NFκB, or if the PPARγ activation was insufficient to suppress NFκB activity.
In contrast, inducible in vitro overexpression in murine keratinocytes of both humanized ALOX15B and murine Alox8 reduced proliferation (Schweiger et al., 2007). This reduction was unrelated to apoptosis, but dependent on p38 kinase. Likewise, addition of the AA metabolites 8-HETE and 15-HETE both reduced bromodeoxyuridine (BrdU) incorporation, however no change with the addition of LA metabolites 9-HODE or 13-HODE have been found (Schweiger et al., 2007). The conflicting proliferation in these findings is likely to be underscored through differences in experimental design (in vivo vs. in vitro) and BrdU incorporation time points (30 min vs. 4 h). However, as differentiation is associated with reduced proliferation it could be plausible that increased keratinocyte differentiation occurs with overexpression of ALOX15B in vitro, yet this was not investigated. As both ALOX15B and Alox8 produced similar results Schweiger et al. (2007) hypothesized that both enzymes work in a similar manner, nevertheless this needs to be confirmed in a human setting to be more conclusive.
In addition to psoriasis, ALOX15B expression has been reported to increase with ultraviolet (UV) B radiation (Yoo et al., 2008). Furthermore, treatment of HaCaT cells with 13-HODE and 15-HETE reduced ALOX12 expression and proliferation, along with increasing apoptosis. Therefore, the authors hypothesized that UV therapy to induce ALOX15B expression can aid psoriasis through inhibiting hyperproliferation and production of pro-inflammatory 12-HETE. Moreover, ω-3 PUFAs have been shown to promote wound healing of sunburn in mice following UVB radiation (Meng et al., 2021), it is therefore plausible to speculate that induction of ALOX15B by UVB radiation may play a role in the protection or resolution of sunburn.
The information on the role of ALOX15B in other inflammatory skin diseases is scarce, however, increased 15-HETE levels were detected in lesional and peri-lesional atopic dermatitis skin (Fogh et al., 1989a), in addition to increases in 13-HODE in atopic dermatitis compared with healthy skin (Giménez-Arnau et al., 1997). Furthermore, the 15-LOX pathway (increased formation of 15-HETE and 15-HEPA) was upregulated in epidermal equivalents derived from atopic dermatitis patients with filaggrin mutations (Blunder et al., 2017). Interestingly, the skin science foundation bioinformatics hub indicates that increased ALOX15B expression is detected in lesional rosacea samples (Skin science foundation bioinformatics hub), yet so far no links to 15-LOXs or metabolites have been investigated.
Hellmann et al. (2018) investigated the role of Rvs in wound healing, here the authors demonstrated that topical application of Rvs to murine wound models resulted in enhanced re-epithelization. Furthermore, in cultured human keratinocytes ALOX15B expression was enhanced by calcium-mediated differentiation, which correlated with 17-HDHA production, proving that 15-LOXs can generate the Rv precursor 17-HDHA from DHA in keratinocytes. Proliferation was not altered, however, RvD2 promoted keratinocyte migration in a human wound model, which was shown to be mediated by the PI3K-AKT pathway. In addition to the promotion of wound healing, RvD2 decreased expression of the pro-inflammatory cytokine IL1β in the murine wound modal, demonstrating a role of ALOX15B in cutaneous wound healing and resolution of inflammation.
ALOX15B in inflammatory bowel disease
Elevated levels of 15-HETE were noticed in inflamed human colon tissue (Zijlstra et al., 1992). Yet, Pochard et al. (2016) reported a slightly lower ALOX15B expression in Crohn’s disease patients and lower 15-HETE levels. ALOX15B expression was also significantly lower in colonic mucosa of ulcerative colitis patients (Mangino et al., 2006), while the DGLA (dihomo-γ-linolenic acid) metabolite 15(S)-hydroxyeicosatrienoic acid (HETrE) was significantly upregulated in the mucosa of remission colitis patients, compared to healthy controls (Diab et al., 2019). Moreover, inhibition of 15-LOXs with PD146176 lowered the body weight in a murine ulcerative colitis model, suggesting that 15-LOX metabolites play a role in the resolution of ulcerative colitis. Furthermore, 15-HETE induced expression of the tight junction protein 1 in human colorectal adenocarcinoma cells (Caco-2), along with reducing permeability and increasing transepithelial electrical resistance (Pochard et al., 2016). Indeed, 15-HETE induced ERK phosphorylation, and the 15-HETE mediated reduced permeability was facilitated through AMP-activated protein kinase (AMPK) induced tight junction protein 1 expression. Along these lines, either shRNA for tight junction protein 1 or the AMPK activator AICAR reversed 15-HETE effects on permeability. As inflammatory bowel disease is characterized by an impaired permeability barrier (Michielan and D'Incà 2015), therapies targeting ALOX15B expression may help in the resolution.
ALOX15B in arthritis
Mao et al. (2020) detected increased Alox15b expression in a rat rheumatoid arthritis model and lipoprotein associated 15-HETE and 13-HODE were increased in the plasma of human rheumatoid arthritis patients (Charles-Schoeman et al., 2018). Treatment of synovial fibroblasts acquired from patients with rheumatoid arthritis with 15-HETE showed nuclear translocation of p68, an increase in phosphoinositide 3-kinase (PI3K)-mediated AKT phosphorylation and a reduced IκBα (inhibitor of NFκB subunit alpha) expression (Wu et al., 2012). 15-HETE mediated PI3K-signaling increased matrix metalloproteinase (MMP)-2, a protein facilitating degradation of joints. However, the authors linked this to ALOX15 expression, while ALOX15B was not investigated. Therefore, ALOX15B expression in human macrophages or tissues associated with rheumatoid arthritis still needs to be determined. In contrast, 13-HODE and 15-HETE decreased MMP-1 and MMP-3 expression in chondrocytes derived from patients with osteoarthritis (Chabane et al., 2009). The decrease in MMP was mediated by PPARγ, however, a 10-fold increase in oxylipin concentrations were used to achieve this. These observations were from different cell types and expression of both 15-LOX enzymes was reported in chondrocytes (Chabane et al., 2009), however physiologically relevant oxylipin concentrations need to be determined.
ALOX15B in reproductive diseases
ALOX15B expression along with increased 15-HETE levels were noticed in the placenta and umbilical cord artery from pre-eclampsia patients (Wang et al., 2012; Yuan et al., 2014), which was associated with an increase in HIF1α (Yuan et al., 2014). Furthermore, ALOX15B expression was induced in human umbilical vein endothelial cells derived from patients with pre-eclampsia or when exposed to a hypoxic environment. Treatment of human umbilical vein endothelial cells with 15-HETE increased cell viability, proliferation, and cell cycle progression, whereas this effect was reduced by inhibition of LOXs with NDGA. Moreover, 15-HETE induced migration and tube formation, and therefore the authors suggested that the increase in 15-HETE/15-LOXs is involved as a compensatory mechanism of reduced angiogenesis in pre-eclampsia (Yuan et al., 2014).
Moreover, Alox15b expression was increased in the labyrinth zone (“site of maternal-fetal exchange”) of the placenta from rats fed a high ω-3 PUFA diet (Jones et al., 2013). Additionally, elevated 13-HODE levels were found in poly cystic ovarian syndrome (Dong et al., 2015). These results suggest potential implications of ALOX15B in additional reproductive disorders, however more evidence on expression and metabolite levels are needed for definitive conclusion.
Recently, a role for prostaglandin E2 (PGE2) in the induction of ALOX15B expression in amnion fibroblasts was reported (Zhang et al., 2022). Inhibition of protein kinase A or antagonism of the PGE2 receptor prevented PGE2-mediated ALOX15B expression. Moreover, 15(S)-HETE activated the NF-κB pathway through phosphorylation of p65, leading to an increased expression of COX-2 and PGE2 synthesis. Interestingly, increased 15-HETE and PGE2 levels were detected in the amnion of termed birth deliveries in which labor occurred than in births without labor. Furthermore, 15(S)-HETE increases with gestational age in the murine fetal membrane and placenta. Subcutaneous injection of 15(S)-HETE resulted in 50% of murine births to be premature. The authors hypothesize that interruption of the positive feedback loop between COX-2 and 15-LOXs could provide a therapeutic target in preventing premature births.
ALOX15B in obesity
ALOX15B expression was significantly increased in both obese and lean critically ill patients in comparison to healthy controls, which correlated with increased PPARγ expression along with cellular differentiation and proliferation. However, 15(S)-HETE levels remained unchanged (Goossens et al., 2017). ALOX15B was predominantly expressed in the CD34−leukocyte population of adipose tissue. Although no ALOX15B expression was detected in the adipocyte fraction, a low level of ALOX15B was found in the CD34+ population, which contained pre-adipocytes. However, protein expression of ALOX15B was not examined in this study (Dobrian et al., 2010). In contrast, 15-HETE has been positively correlated with body mass index (BMI) and waist circumference (Pickens et al., 2017). Furthermore, Song et al. (2016) suggested 15(S)-HETE to be a positive regulator of proliferation and PPARγ activation in the murine pre-adipocyte cell line 3T3-L1; however, the focus here was on Alox15.
ALOX15B in multiple sclerosis
ALOX15B was found to be the 3rd highest differentially expressed gene in slowly expanding lesions from progressive multiple sclerosis (Jäckle et al., 2020). Validation by immunohistochemistry revealed ALOX15B expression in macrophages/microglia at the lesion edge, along with significantly higher expression in slowly expanding lesions than in chronic inactive lesions. Safizadeh et al. (2018) also detected significantly higher ALOX15B expression, 15-HETE and 13-HODE in peripheral blood mononuclear cells (PBMCs) from multiple sclerosis patients. Interestingly, higher expression of 15-LOXs/13-HODE/15-HETE correlated with higher circulating cholesterol levels.
The role of ALOX15B in cancer
Investigations of ALOX15B in cancer have predominantly focused on a role as a tumor suppressor; however, conflicting evidence suggests that tissue-dependent regulation and functions are apparent. Whereas in prostate (Shappell et al., 1999; Bhatia et al., 2003; Tang et al., 2004; Suraneni et al., 2010) and breast carcinoma (Kort et al., 1992; Jiang et al., 2006; Vaezi et al., 2021; Wu et al., 2021) ALOX15B is downregulated, expression of ALOX15B in colorectal cancer is associated with a poorer prognosis (Yuan et al., 2020). Table 3 provides further insight into expression alterations of ALOX15B or its products 15-HETE and 13-HODE in different types of cancers. Moreover, research towards ALOX15B silencing or overexpression in cancer cells lines has been performed, providing further insight. The data suggest a role of ALOX15B in cellular signaling pathways (PPAR, MAPK/ERK, AKT), which are linked to cellular survival, proliferation, and apoptosis (Figure 6). Therefore, the following paragraphs will summarize the information on prostate, breast, and lung carcinomas.
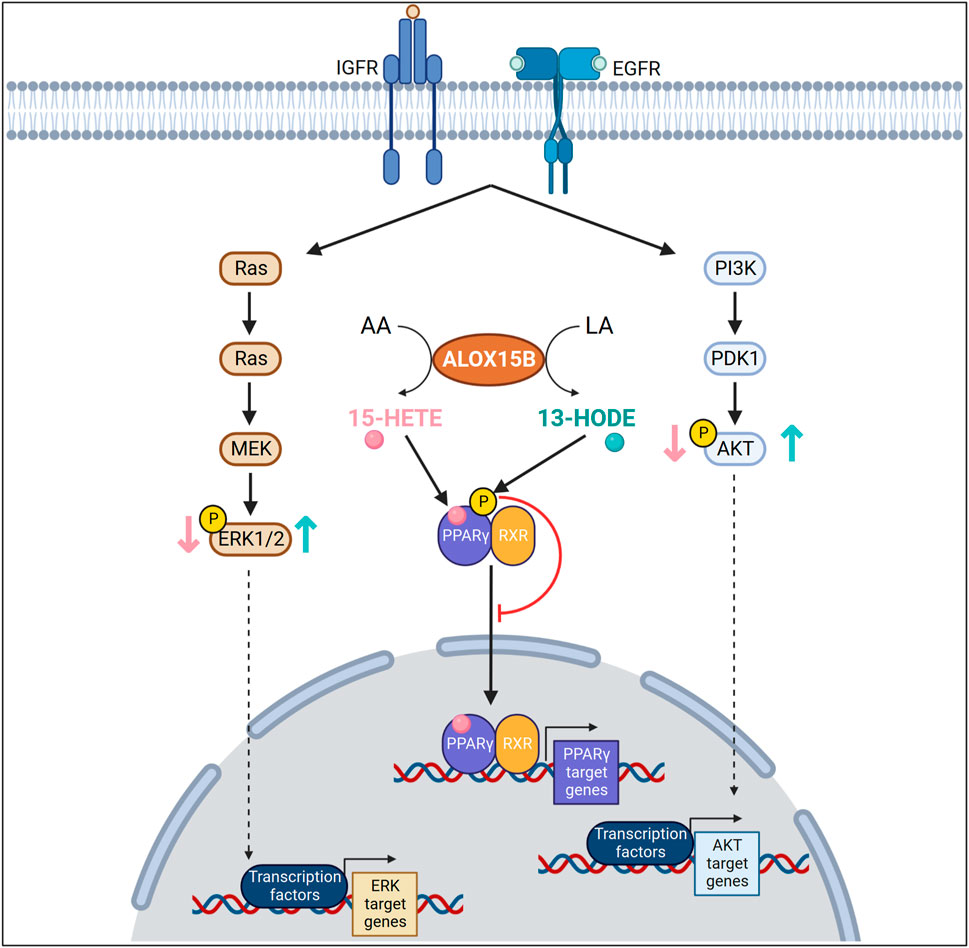
FIGURE 6. Arachidonic (AA) and linoleic acid (LA) metabolites in cellular signaling in cancer cells. ALOX15B converts AA or LA into 15-HETE or 13-HODE, respectively. In an insulin like growth factor (IGF) or epidermal growth factor (EGF) dependent manner, 15-HETE decreases mitogen-activated protein kinase 3 (ERK1), mitogen-activated protein kinase 1 (ERK2) or AKT phosphorylation, whereas 13-HODE increases their phosphorylation. 15-HETE, a known ligand for peroxisome proliferator activated receptor gamma (PPARγ) activates and increases transcriptional activity of PPARγ. In contrast, 13-HODE increases the phosphorylation of PPARγ, thereby reducing transcription of PPARγ target genes.
While normal prostate epithelium expresses ALOX15B but not ALOX15 in the luminal compartment (Subbarayan et al., 2006), expression of ALOX15B was lost in prostate cancer (Shappell et al., 1999; Bhatia et al., 2005) as well as associated cell lines (Tang et al., 2002), and also 15-HETE production was found to be reduced (Shappell et al., 2001b). Recently Vaezi et al. (2021) reviewed the role of 15-LOXs in breast cancer, summarizing the contrasting roles of ALOX15B and ALOX15, being negative and positive respectively, towards tumor formation. This notion is reflected in prostate cancer, where ALOX15’s substrate preference to LA is reported along with differential effects of 15-HETE and 13-HODE (Hsi et al., 2002). Similar to prostate cancer, a lower expression of ALOX15B has been detected in breast cancer in comparison to normal breast tissue (Nony et al., 2005; Jiang et al., 2006; Wu et al., 2021), in addition to 15-HETE being reduced (Subbarayan et al., 2005; Vaezi et al., 2021).
While the information on prostate and breast carcinoma is relatively consistent, investigations in lung cancer have shown both increased (Gonzalez et al., 2004; Sausville et al., 2017) and decreased (Li et al., 2019) ALOX15B expression. In normal human lungs, ALOX15B is expressed in both the nucleus and cytoplasm of alveolar type II cells (Gonzalez et al., 2004). Although Gonzalez et al. (2004) reported an increase in ALOX15B expression in lung cancer, an inverse correlation with ki67 expression was observed. Furthermore, tumor grade was inversely correlated with ALOX15B (Gonzalez et al., 2004). Interestingly, ALOX15B expression was lost in neuroendocrine tumors, whereas Li et al. (2019) reported a significant reduction of ALOX15B expression in lung carcinomas when compared to non-tumor tissue. However, a normal level of ALOX15B expression was reported for the majority of adenocarcinoma samples. Differences in the reported increase or decrease in ALOX15B expression may be explained through arbitrary qualitative analysis of immunohistochemical staining.
Interestingly, significantly lower expression of ALOX15B has been reported in metastatic breast cancer (Jiang et al., 2006). Furthermore, ALOX15B was downregulated in the pre-cancerous high grade prostatic intraepithelial neoplasia (HGPIN) and had a negative correlation with Gleason score (prostate histopathological scoring guide) (Jack et al., 2000). Also, ALOX15B expression was negatively correlated with breast tumor stage and a low ALOX15:ALOX15B ratio was associated with a poorer prognosis (Jiang et al., 2006), yet amplification of ALOX15B had a negative effect on survival (Wang et al., 2017). A negative correlation was reported between 15-HETE and tumor diameter by Kort et al. (1992), in addition to a higher level of 15-HETE reported in malignant than benign breast tumors. As it cannot be ruled out that infiltrating macrophages or ALOX15 expressing tumor cells are a source of 15-HETE and the above-mentioned study does not directly link ALOX15B to levels of 15-HETE this could be an explanation to the increased 15-HETE levels measured. Tian et al. (2016) suggest ALOX15B may play an important role in early stage non-small cell lung carcinoma and single nucleotide polymorphisms in ALOX15B have been associated with increased risk for lung cancer (Shen et al., 2009), along with rare single nucleotide polymorphisms associated with a poorer prognosis in women with non-small cell lung carcinoma (Sausville et al., 2017). When comparing lung carcinomas with mutated tumor suppressors, ALOX15B expression was downregulated (Kim et al., 2021), whereas in adenocarcinoma both ALOX15B and 15-HETE were induced (Sausville et al., 2017; Yang et al., 2018).
In prostate carcinoma cell lines, the decrease in ALOX15B expression was neither affected by inhibition of global histone deacetylation nor by inhibition of DNA methyltransferase (Tang et al., 2002). Suraneni et al. (2014) hypothesized that overexpression of the MYC proto-oncogene in prostate cancer may account for lower ALOX15B expression. The anti-inflammatory glucocorticoid dexamethasone increased ALOX15B expression (Torosyan et al., 2006). Although dexamethasone is a ligand for the GR, treatment of prostate cancer results in the compensatory downregulation of the GR (which is overexpressed in prostate cancer). Indeed, a glucocorticoid response element is located within -157 to -33 upstream of the ALOX15B transcription start site, a region which was found to be critical for promoter activity (Feng et al., 2010). Therefore, GR signaling is a likely mechanism for loss of ALOX15B in prostate cancer (for more details see section “Transcriptional Regulation of ALOX15B″).
Contrastingly, DHT treatment of breast carcinoma BT474 cells increased ALOX15B expression (Vasiliou et al., 2022), whereas in prostate carcinoma LNCaP cells, transfection with a plasmid containing the ALOX15B promoter remained unresponsive to androgen treatment (Tang et al., 2004). Although Tang et al. (2004) treated LNCaP cells with 100 nM DHT, 10 nM was sufficient to induce ALOX15B expression in BT474 cells, therefore differences in concentration are unlikely to be an explanation as to the differences in DHT responses reported. As both BT474 and LNCaP cell lines were androgen responsive and expressed the androgen receptor, an indirect mechanism of DHT induction of ALOX15B in breast cancer cells is plausible. Intriguingly, ER expression inversely correlated with ALOX15B expression in normal breast tissue, however ER positive breast tumors had a higher ALOX15B expression than ER negative tumor (Wang et al., 2017). As stable ER overexpression in MCF10A cells did not significantly alter ALOX15B expression, it is likely that ER expression alone does not play a role in the transcriptional regulation of ALOX15B in breast cancer. BT474 cells express both ER and AR, whereas LNCaP cells do not express ER (Lafront et al., 2020), and higher androgen receptor expression was detected in ER+ breast tumors (Kensler et al., 2019). Therefore, co-expression and crosstalk of both nuclear receptors may be required for DHT to induce ALOX15B expression and explain differences in the correlation between ALOX15B and ER between normal and cancerous breast tissue.
Studies in carcinoma cell lines reveal more insight into the role of ALOX15B as a tumor suppressor in cancer through cellular signaling pathways (Figure 6). As discussed earlier, ALOX15B products may work as PPARγ ligands and 15-HETE increased transcriptional activity of PPARγ and caused expression of the fatty acid binding protein (FABP) in PC3 cells (Shappell. et al., 2001c). 15-HETE treatment of PC3 cells reduced phosphorylation of PPARγ in the presence of insulin like growth factor-1 or epidermal growth factor, therefore implying a MAPK dependent pathway (Hsi et al., 2002). Although, 13-HODE has also been reported as a PPARγ ligand (Nagy et al., 1998), 13-HODE was described to induce PPARγ phosphorylation (Hsi et al., 2002) thus, suppressing transcriptional activity (Burns and Vanden Heuvel 2007). Furthermore, overexpression of PPARγ lowered ALOX15B expression (Subbarayan et al., 2004), whilst PPARγ activation has been shown by overexpression of ALOX15B (Li et al., 2015). Likewise with prostate cancer an inverse correlation between PPARγ and ALOX15B expression has been shown in breast cancer (Subbarayan et al., 2005). Elevated levels of PPARγ in breast cancer tumors may be responsible for low ALOX15B expression. Addition of white tea extract (immature, unopened buds of Camellia sinensis) to lung carcinoma A549 cells upregulated ALOX15B expression along with the production of 15-HETE, which led to an upregulation of PPARγ (Mao et al., 2010). This increase in PPARγ was suppressed by lipoxygenase inhibition (via NGDA or caffeic acid). Moreover, inhibition of LOXs or PPARγ resulted in a reduction in white tea extract induced caspase activity, suggesting that ALOX15B induces apoptosis in A549 cells via 15-HETE mediated activation of PPARγ (Mao et al., 2010).
Activation of p38 MAPK was observed through overexpressing ALOX15B in MDA-MB-435 cells (Nony et al., 2005). Whereas addition of 15-HETE or AA to PC3 cells reduced both ERK1/2 and AKT phosphorylation, treatment with 13-HODE or LA induced their phosphorylation in an epidermal growth factor or insulin like growth factor dependent manner (Hsi et al., 2002). However, since siRNA for ALOX15 and not ALOX15B inhibited DHA induced phosphorylation of AKT or phosphoinositide-dependent kinase-1 (PDK1), Hu et al. (2013) reported that PDK1/AKT activation in PC3 cells is ALOX15 but not ALOX15B dependent, which express low levels of both enzymes. As there is a lower level of ALOX15B in PC3 cells than NHP, further reduction by siRNA may be redundant. Repetition of these experiments in NHP or ALOX15B overexpressing cells would be more accurate in understanding whether ALOX15B alters the AKT pathway.
Indeed, earlier studies in prostate cells suggested that ALOX15 favored LA to produce 13-HODE in comparison to ALOX15B predominantly metabolizing AA to 15-HETE, which stimulated the assumption that the effects of exogenous 15-HETE treatment to prostate cells correlates with ALOX15B. However, as there is an upregulation of ALOX15 in prostate carcinoma (Kelavkar et al., 2006) and both enzymes are capable of metabolizing AA and LA it is difficult to distinguish function between the two enzymes. There is a lack of investigations involving DHA treatment in an ALOX15B context and very few studies have investigated other PUFAs. Collectively these alterations in signaling pathways suggests a role for 15-HETE produced by ALOX15B as a tumor suppressor through reducing cellular proliferation, promoting apoptosis and enhancing cytokine secretion. Furthermore, this is reflected by investigations summarized in the following paragraphs.
Prostate carcinoma cells grown in vitro retain a low ALOX15B phenotype and do not produce 15-HETE (Shappell et al., 2001c; Bhatia et al., 2005). ALOX15B expression and hence, generation of its metabolite 15-HETE, negatively affected cellular proliferation in prostate cells. Furthermore, overexpression of ALOX15B in UMSCC1 cells (Squamous cell carcinoma derived from the oral cavity) also reduced cellular proliferation (Wang et al., 2006). Addition of 15-HETE to NHP cells reduced proliferation (Tang et al., 2002) and increased the number of cells in the G0/G1 phase (Shappell et al., 2001c). In addition, overexpression of a GFP-ALOX15B in prostate cancer cell lines DUP145 and PC3 increased the number of cells in G0/G1 and reduced those in S phase, although no significant change in the number of ki67+ cells were detected (Tang et al., 2009). Moreover, Tang et al. (2002) reported that overexpression of ALOX15B reduced the number of BrdU+ cells. Serum starving induced ALOX15B expression and decreased BrdU incorporation, which was reversed by the re-addition of serum (Tang et al., 2002). Bhatia et al. (2005) observed that overexpression of either a fully active ALOX15B, or a non-functioning splice variant decreased proliferation. Moreover, attenuated cell proliferation with ALOX15B being overexpressed correlated with increases in cellular senescence. Furthermore, both ALOX15B and 15-HETE increased with cellular passage in NHP cells (Bhatia et al., 2005). Controversially, slower proliferating NHPrE1 cells (spontaneously immortalized NHP cells) expressed lower levels of ALOX15B than the faster proliferating BHPrE1 cells (spontaneously immortalized benign human prostate cells) (Jiang et al., 2010). Suggestively, reduced proliferation is more likely to be associated with cellular senescence than ALOX15B expression itself. Future studies should investigate whether inhibition or attenuation of ALOX15B expression in NHP cells impedes the cell’s ability to senesce with increasing passage number. The ALOX15B-generated DHA metabolites 17-HDHA, 17-HpDHA along with double peroxidized products 10(S),17(S)-diHDHA (protectin DX) and RvD5, inhibited proliferation at much lower concentrations than 15-HETE in PC3, DU145 and LNCaP cells (O'Flaherty et al., 2012).
In line with these data Li et al. (2015) reported reduced proliferation and increased caspase 3 and 9 activation with 15-HETE and 13-HODE treatment in lung adenocarcinoma, NCI-H23, and large cell cancer, NCI-H460, cells. Moreover, overexpression of both 15-lipoyxgenating enzymes also induced the cleavage of caspase 3 and 9 during simultaneous treatment with both LA and AA (Li et al., 2015). Furthermore, siRNA mediated knockdown of ALOX15B reduced grape seed procyanidin extract induced cell death of A549 and EAS-2B-CSC 1198 cells (Mao et al., 2016). The authors note that although there was no increase in ALOX15 or ALOX15B expression, there was an increase in 15-HETE production, which likely resulted from a shunting of AA metabolism towards 15-LOX enzymes as grape seed extract inhibits COX-2. In a previous study Mao et al. (2010) had observed increased ALOX15 and ALOX15B expression from green and white tea extracts in A549 cells. The white tea extract induced 15-HETE secretion and caspase 3 activity. Li et al. (2019) reported that smoking carcinogen 4-methylnitrosamino-l-3-pyridyl-butanone and air pollutants drive carcinogenesis though inhibition of ALOX15B expression. It was suggested that microRNAs 17, 106, 590-3p, 20a, 20b, 93 may be the likely cause of the reduced ALOX15B expression in lung cell lines Bet1A and NCI-H23 exposed to air pollution or smoking carcinogen (Li et al., 2019).
Yet in lung adenocarcinoma A549 cells, ALOX15B expression and 15-HETE production has been induced under hypoxia (Yang et al., 2018). Contrastingly, addition of 15-HETE under both normoxia and hypoxia induced proliferation and migration of A549 cells. It should be noted that this study treated the cells with 1 µM 15-HETE for 24 h, whereas Li et al. (2015) saw reductions in proliferation with 10–70 µM 15-HETE over periods of 24, 48, and 72 h, which may explain the differences observed. Additionally, 15-HETE induced STAT3 phosphorylation and inhibition of STAT3 activation prevented 15-HETE mediated proliferation and migration (Yang et al., 2018). Moreover, ubiquitination of ALOX15B and expression in exosomes activated STAT3 in hypoxic pulmonary artery endothelial cells (Zhang et al., 2018). Tumor hypoxia is extremely common and is negatively correlated with survival. Evidence in A549 cells suggested that ALOX15B promotes proliferation through 15-HETE in lung adenocarcinomas. The underlying mechanism between ALOX15B and STAT3 activation remains unknown. Future research should question if pharmaceutical inhibition of ALOX15B can be targeted in treatments for lung cancers with high ALOX15B expression.
While the ALOX15B corresponding murine ortholog Alox8 is not expressed in murine prostate glands (Shappell et al., 2003), humanized ALOX15B overexpression studies have been performed in vivo. Prostate carcinoma PC3 cells (which do not express ALOX15B) were injected into the mouse prostate to develop tumors and stable ALOX15B overexpression resulted in significantly smaller tumors (Bhatia et al., 2003). An additional study using GFP tagged ALOX15B overexpressing prostate carcinoma cells (DU145 and PC3) that were injected into the right flank of nude mice also resulted in a lower tumor volume (Tang et al., 2009). Furthermore, these tumors showed more cleaved caspase 3 and high numbers of apoptotic cells even though there also was a small increase in ki67+ proliferating cells (Tang et al., 2009). Tang et al. (2009) also observed a downregulation of vascular endothelial growth factor (VEGF), suggesting that ALOX15B expression may reduce angiogenesis in prostate carcinoma. Likewise Suraneni et al. (2010) reported a similar phenotype as prostate specific transgenic expression of ALOX15B caused hyperplasia, increased ki67+ cells and higher numbers of both luminal and basal cells, along with an increase of p27, a senescence marker (Suraneni et al., 2010). ALOX15B expression was associated with the age of prostate tissue (Bhatia et al., 2005). As benign prostatic hyperplasia is correlated with age, there may be an associated link of ALOX15B and hyperplasia in the human prostate. Using an enzymatically inactive splice variant of ALOX15B revealed that the PUFA-metabolizing activity of ALOX15B was integral to the tumor suppressor function in prostate carcinoma. Suraneni et al. (2014) demonstrated that although hyperplasia was noticed in ALOX15B transgenic mice, when crossed with tumor suppressor p53−/− or p53−/+ mice, no prostate cancer occurred. Furthermore, MYC induced carcinoma was inhibited by ALOX15B expression, which is likely through induction of cellular senescence as demonstrated by p27 and β-galactosidase staining (Suraneni et al., 2014).
Treatment with both AA and 15-HETE without ALOX15B overexpression increased cell adhesion of MDA-MB-435 cells to collagen (Nony et al., 2005). The 13-HODE/15-HETE ratio inversely correlated with the level of E-cadherin expression in breast cancer MCF-7 cells as well as in colon cancer HRT-18 cells (Pasqualini et al., 2003). However, it should be noted that MDA-MB-435 cell line has been re-described as a melanoma cell line (Prasad and Gopalan, 2015). Therefore, the assumption that 15-HETE prevents migration and metastases should be applied as a general cancer principle rather than being specific for breast cancer.
As reviewed previously by Weigert et al. (2018) tumor associated macrophages (TAMs) may play a major role in the expression of ALOX15B and lipid mediation of the tumor microenvironment. Reinartz et al. (2016) observed a slight but non-significant higher level of ALOX15B expression in TAMs, when compared to ovarian carcinoma tumors. Indeed Roffeis et al. (2007) revealed an increase of ALOX15B expression in ovarian tumors in comparison to normal ovarian tissue, however the precise cell type that expresses ALOX15B was not determined. It is therefore possible that an increase in ALOX15B expression occurs in both in carcinoma cells derived from ovarian tissue along with TAMs.
Extremely low level of ALOX15B expression has been found in normal kidney tissue. However, expression and 15-HETE levels were increased in carcinoma tissue and TAMs. Inhibition of LOXs through NDGA in TAMs obtained from renal cell carcinoma reduced the expression of CCL2 and IL-10 (Daurkin et al., 2011). Furthermore, inhibition of 15-LOXs via ML351 or PD146176 in lung macrophages derived from normal lung tissue of patients undergoing surgery for carcinoma reduced cytokine and chemokine release (Abrial et al., 2015). Both IL-4/IL-13-induced release of cytokines CCL13, 18 and 22 along with LPS-induced release of TNFα, CCL2, 3 and 4, and CXCL1, 8 and 10 were reduced by 15-LOX inhibition.
Collectively, these findings suggest a role of ALOX15B acting as a tumor suppressor in breast and prostate cancer. Whereas in vitro experiments pointed to reduced cellular proliferation, migration and cell cycle progression along with increased apoptosis, hyperplasia in vivo is accompanied by attenuated tumor formation, and both in vitro and in vivo experiments link ALOX15B expression with senescence. Inhibition of MAPK and ERK signaling by ALOX15B derived 15-HETE implies an anti-tumorigenic effect. Yet, there have been no follow-up studies on the role of ALOX15B in prostate cancer since 2014. Indeed, in recent years, treatments of prostate cancer vastly improved towards androgen receptor targeted approaches. It would therefore be interesting to explore whether current therapies result in the re-expression of ALOX15B in the prostate.
Conclusion
As a member of the heterogeneous family of lipoxygenases, ALOX15B oxygenates poly-unsaturated fatty acids with positional specificity to the corresponding hydroperoxides, which can serve in the formation of SPMs. Present in a variety of different tissues, ALOX15B expression and activity has the ability to affect many cellular signaling pathways, involved with proliferation, survival, and cell cycle. For instance, in primary human macrophages, a role of ALOX15B in regulating cholesterol homeostasis was proposed. Moreover, attenuation or inhibition of ALOX15B has been shown to reduce cytokine and chemokine production and ALOX15B expression was linked to atherosclerosis. A clear role of ALOX15B as a tumor suppressor in prostate and breast cancer is apparent, yet there remains diversity in other carcinomas. Future investigations into standardizing physiologically relevant concentrations of lipids in vitro may help elucidate discrepancies. Moreover, the use of cells naturally expressing ALOX15B could provide a useful tool in detecting PUFA favorability and metabolite synthesis.
Author contributions
This manuscript was conceptualized by YB, MAP, and BB, written by YB and MAP, and edited by YB, MAP, and BB.
Funding
This work was supported by Deutsche Forschungsgemeinschaft (SFB 1039, TP B04, and Br999/25-1).
Acknowledgments
Figures 3, 5, 6 were created with BioRender.com.
Conflict of interest
The authors declare that the research was conducted in the absence of any commercial or financial relationships that could be construed as a potential conflict of interest.
Publisher’s note
All claims expressed in this article are solely those of the authors and do not necessarily represent those of their affiliated organizations, or those of the publisher, the editors and the reviewers. Any product that may be evaluated in this article, or claim that may be made by its manufacturer, is not guaranteed or endorsed by the publisher.
References
Abrial, C., Grassin-Delyle, S., Salvator, H., Brollo, M., Naline, E., and Devillier, P. (2015). 15-Lipoxygenases regulate the production of chemokines in human lung macrophages. Br. J. Pharmacol. 172 (17), 4319–4330. doi:10.1111/bph.13210
Adel, S., Karst, F., González-Lafont, À., Pekárová, M., Saura, P., Masgrau, L., et al. (2016). Evolutionary alteration of ALOX15 specificity optimizes the biosynthesis of antiinflammatory and proresolving lipoxins. Proc. Natl. Acad. Sci. U. S. A. 113 (30), E4266–E4275. doi:10.1073/pnas.1604029113
Arandjelovic, S., and Ravichandran, K. S. (2015). Phagocytosis of apoptotic cells in homeostasis. Nat. Immunol. 16 (9), 907–917. doi:10.1038/ni.3253
Bajpai, A. K., Blaskova, E., Pakala, S. B., Zhao, T., Glasgow, W. C., Penn, J. S., et al. (2007). 15(S)-HETE production in human retinal microvascular endothelial cells by hypoxia: Novel role for MEK1 in 15(S)-HETE–Induced angiogenesis. Invest. Ophthalmol. Vis. Sci. 48 (11), 4930–4938. doi:10.1167/iovs.07-0617
Bannenberg, G. L., Chiang, N., Ariel, A., Arita, M., Tjonahen, E., Gotlinger, K. H., et al. (2005). Molecular circuits of resolution: formation and actions of resolvins and protectins. J. Immunol. 174 (7), 4345–4355. doi:10.4049/jimmunol.174.7.4345
Banno, T., Gazel, A., and Blumenberg, M. (2004). Effects of tumor necrosis factor-alpha (TNF alpha) in epidermal keratinocytes revealed using global transcriptional profiling. J. Biol. Chem. 279 (31), 32633–32642. doi:10.1074/jbc.M400642200
Barooni, A. B., Ghorbani, M., Salimi, V., Alimohammadi, A., Khamseh, M. E., Akbari, H., et al. (2019). Up-regulation of 15-lipoxygenase enzymes and products in functional and non-functional pituitary adenomas. Lipids Health Dis. 18 (1), 152. doi:10.1186/s12944-019-1089-1
Belkner, J., Stender, H., and Kühn, H. (1998). The rabbit 15-lipoxygenase preferentially oxygenates LDL cholesterol esters, and this reaction does not require vitamin E. J. Biol. Chem. 273 (36), 23225–23232. doi:10.1074/jbc.273.36.23225
Belkner, J., Wiesner, R., Rathman, J., Barnett, J., Sigal, E., and Kühn, H. (1993). Oxygenation of lipoproteins by mammalian lipoxygenases. Eur. J. Biochem. 213 (1), 251–261. doi:10.1111/j.1432-1033.1993.tb17755.x
Bender, G., Schexnaydre, E. E., Murphy, R. C., Uhlson, C., and Newcomer, M. E. (2016). Membrane-dependent activities of human 15-LOX-2 and its murine counterpart: Implications for murine models of atherosclerosis. J. Biol. Chem. 291 (37), 19413–19424. doi:10.1074/jbc.M116.741454
Bhatia, B., Maldonado, C. J., Tang, S., Chandra, D., Klein, R. D., Chopra, D., et al. (2003). Subcellular localization and tumor-suppressive functions of 15-lipoxygenase 2 (15-LOX2) and its splice variants. J. Biol. Chem. 278 (27), 25091–25100. doi:10.1074/jbc.M301920200
Bhatia, B., Tang, S., Yang, P., Doll, A., Aumüeller, G., Newman, R. A., et al. (2005). Cell-autonomous induction of functional tumor suppressor 15-lipoxygenase 2 (15-LOX2) contributes to replicative senescence of human prostate progenitor cells. Oncogene 24 (22), 3583–3595. doi:10.1038/sj.onc.1208406
Blunder, S., Rühl, R., Moosbrugger-Martinz, V., Krimmel, C., Geisler, A., Zhu, H., et al. (2017). Alterations in epidermal eicosanoid metabolism contribute to inflammation and impaired late differentiation in FLG-mutated atopic dermatitis. J. Invest. Dermatol. 137 (3), 706–715. doi:10.1016/j.jid.2016.09.034
Bodnarczuk, T., Deskur, A., Dolegowska, K., Dolegowska, B., Starzynska, T., and Blogowski, W. (2018). Hydroxyeicosatetraenoic acids in patients with pancreatic cancer: A preliminary report. Am. J. Cancer Res. 8 (9), 1865–1872. Available online at https://pubmed.ncbi.nlm.nih.gov/30323978 (Accessed June 16, 2022).
Borngräber, S., Browner, M., Gillmor, S., Gerth, C., Anton, M., Fletterick, R., et al. (1999). Shape and specificity in mammalian 15-lipoxygenase active site The functional interplay of sequence determinants for the reaction specificity. J. Biol. Chem. 274 (52), 37345–37350. doi:10.1074/jbc.274.52.37345
Brash, A. R., Boeglin, W. E., and Chang, M. S. (1997). Discovery of a second 15S-lipoxygenase in humans. Proc. Natl. Acad. Sci. U. S. A. 94 (12), 6148–6152. doi:10.1073/pnas.94.12.6148
Brash, A. R., Jisaka, M., Boeglin, W. E., and Chang, M. S. (1999). Molecular cloning of a second human 15S-lipoxygenase and its murine homologue, an 8S-lipoxygenase Their relationship to other mammalian lipoxygenases. Adv. Exp. Med. Biol. 447, 29–36. doi:10.1007/978-1-4615-4861-4_3
Brash, A. R. (1999). Lipoxygenases: Occurrence, functions, catalysis, and acquisition of substrate. J. Biol. Chem. 274 (34), 23679–23682. doi:10.1074/jbc.274.34.23679
Brinckmann, R., Schnurr, K., Heydeck, D., Rosenbach, T., Kolde, G., and Kühn, H. (1998). Membrane translocation of 15-lipoxygenase in hematopoietic cells is calcium-dependent and activates the oxygenase activity of the enzyme. Blood 91 (1), 64–74. doi:10.1182/blood.v91.1.64
Bryant, R. W., Bailey, J. M., Schewe, T., and Rapoport, S. M. (1982). Positional specificity of a reticulocyte lipoxygenase Conversion of arachidonic acid to 15-S-hydroperoxy-eicosatetraenoic acid. J. Biol. Chem. 257 (11), 6050–6055. doi:10.1016/s0021-9258(20)65103-1
Buckley, C. D., Gilroy, D. W., and Serhan, C. N. (2014). Proresolving lipid mediators and mechanisms in the resolution of acute inflammation. Immunity 40 (3), 315–327. doi:10.1016/j.immuni.2014.02.009
Burns, K. A., and Vanden Heuvel, J. P. (2007). Modulation of PPAR activity via phosphorylation. Biochim. Biophys. Acta 1771 (8), 952–960. doi:10.1016/j.bbalip.2007.04.018
Camp, R. D. R., Mallet, A. I., Woollard, P. M., Brain, S. D., Kobza Black, A., and Greaves, M. W. (1983). The identification of hydroxy fatty acids in psoriatic skin. Prostaglandins 26 (3), 431–447. doi:10.1016/0090-6980(83)90178-8
Campbell, S. E., Rudder, B., Phillips, R. B., Whaley, S. G., Stimmel, J. B., Leesnitzer, L. M., et al. (2011). γ-Tocotrienol induces growth arrest through a novel pathway with TGFβ2 in prostate cancer. Free Radic. Biol. Med. 50 (10), 1344–1354. doi:10.1016/j.freeradbiomed.2011.02.007
Carlier, F. M., de Fays, C., and Pilette, C. (2021). Epithelial barrier dysfunction in chronic respiratory diseases. Front. Physiol. 12, 691227. Available online at: https://www.frontiersin.org/articles/10.3389/fphys.2021.691227 (Accessed June 16, 2022). doi:10.3389/fphys.2021.691227
Chabane, N., Zayed, N., Benderdour, M., Martel-Pelletier, J., Pelletier, J. P., Duval, N., et al. (2009). Human articular chondrocytes express 15-lipoxygenase-1 and -2: Potential role in osteoarthritis. Arthritis Res. Ther. 11 (2), R44. doi:10.1186/ar2652
Charles-Schoeman, C., Meriwether, D., Lee, Y. Y., Shahbazian, A., and Reddy, S. T. (2018). High levels of oxidized fatty acids in HDL are associated with impaired HDL function in patients with active rheumatoid arthritis. Clin. Rheumatol. 37 (3), 615–622. doi:10.1007/s10067-017-3896-y
Cho, Y., and Ziboh, V. A. (1994a). Expression of protein kinase C isozymes in Guinea pig epidermis: Selective inhibition of PKC-beta activity by 13-hydroxyoctadecadienoic acid-containing diacylglycerol. J. Lipid Res. 35 (5), 913–921. doi:10.1016/S0022-2275(20)39185-9
Cho, Y., and Ziboh, V. A. (1994b). Incorporation of 13-hydroxyoctadecadienoic acid (13-HODE) into epidermal ceramides and phospholipids: Phospholipase C-catalyzed release of novel 13-HODE-containing diacylglycerol. J. Lipid Res. 35 (2), 255–262. doi:10.1016/S0022-2275(20)41214-3
Cho, Y., and Ziboh, V. A. (1994c). 13-Hydroxyoctadecadienoic acid reverses epidermal hyperproliferation via selective inhibition of protein kinase C-beta activity. Biochem. Biophys. Res. Commun. 201 (1), 257–265. doi:10.1006/bbrc.1994.1697
Coffa, G., and Brash, A. R. (2004). A single active site residue directs oxygenation stereospecificity in lipoxygenases: Stereocontrol is linked to the position of oxygenation. Proc. Natl. Acad. Sci. U. S. A. 101 (44), 15579–15584. doi:10.1073/pnas.0406727101
Conrad, D. J., Kühn, H., Mulkins, M., Highland, E., and Sigal, E. (1992). Specific inflammatory cytokines regulate the expression of human monocyte 15-lipoxygenase. Proc. Natl. Acad. Sci. U. S. A. 89 (1), 217–221. doi:10.1073/pnas.89.1.217
Danielsson, K. N., Rydberg, E. K., Ingelsten, M., Akyürek, L. M., Jirholt, P., Ullström, C., et al. (2008). 15-Lipoxygenase-2 expression in human macrophages induces chemokine secretion and T cell migration. Atherosclerosis 199 (1), 34–40. doi:10.1016/j.atherosclerosis.2007.10.027
Daurkin, I., Eruslanov, E., Stoffs, T., Perrin, G. Q., Algood, C., Gilbert, S. M., et al. (2011). Tumor-associated macrophages mediate immunosuppression in the renal cancer microenvironment by activating the 15-lipoxygenase-2 pathway. Cancer Res. 71 (20), 6400–6409. doi:10.1158/0008-5472.CAN-11-1261
de Groot, J. J., Veldink, G. A., Vliegenthart, J. F. G., Boldingh, J., Wever, R., and van Gelder, B. F. (1975). Demonstration by EPR spectroscopy of the functional role of iron in soybean lipoxygenase-1. Biochim. Biophys. Acta 377 (1), 71–79. doi:10.1016/0005-2744(75)90287-9
Diab, J., Al-Mahdi, R., Gouveia-Figueira, S., Hansen, T., Jensen, E., Goll, R., et al. (2019). A quantitative analysis of colonic mucosal oxylipins and endocannabinoids in treatment-naïve and deep remission ulcerative colitis patients and the potential link with cytokine gene expression. Inflamm. Bowel Dis. 25 (3), 490–497. doi:10.1093/ibd/izy349
Dobrian, A. D., Lieb, D. C., Ma, Q., Lindsay, J. W., Cole, B. K., Ma, K., et al. (2010). Differential expression and localization of 12/15 lipoxygenases in adipose tissue in human obese subjects. Biochem. Biophys. Res. Commun. 403 (3-4), 485–490. doi:10.1016/j.bbrc.2010.11.065
Dong, F., Deng, D., Chen, H., Cheng, W., Li, Q., Luo, R., et al. (2015). Serum metabolomics study of polycystic ovary syndrome based on UPLC-QTOF-MS coupled with a pattern recognition approach. Anal. Bioanal. Chem. 407 (16), 4683–4695. doi:10.1007/s00216-015-8670-x
Droege, K. D., Keithly, M. E., Sanders, C. R., Armstrong, R. N., and Thompson, M. K. (2017). Structural dynamics of 15-lipoxygenase-2 via hydrogen-deuterium exchange. Biochemistry 56 (38), 5065–5074. doi:10.1021/acs.biochem.7b00559
Ebert, R., Cumbana, R., Lehmann, C., Kutzner, L., Toewe, A., Ferreirós, N., et al. (2020). Long-term stimulation of toll-like receptor-2 and -4 upregulates 5-LO and 15-LO-2 expression thereby inducing a lipid mediator shift in human monocyte-derived macrophages. Biochim. Biophys. Acta. Mol. Cell. Biol. Lipids 1865 (9), 158702. doi:10.1016/j.bbalip.2020.158702
Fahy, J. V., and Dickey, B. F. (2010). Airway mucus function and dysfunction. N. Engl. J. Med. 363 (23), 2233–2247. doi:10.1056/nejmra0910061
Feng, Y., Bai, X., Yang, Q., Wu, H., and Wang, D. (2010). Downregulation of 15-lipoxygenase 2 by glucocorticoid receptor in prostate cancer cells. Int. J. Oncol. 36 (6), 1541–1549. doi:10.3892/ijo_00000641
Fogh, K., and Kragballe, K. (2000). Eicosanoids in inflammatory skin diseases. Prostagl. Other Lipid Mediat. 63 (1), 43–54. doi:10.1016/S0090-6980(00)00096-4
Fogh, K., Søgaard, H., Herlin, T., and Kragballe, K. (1988). Improvement of psoriasis vulgaris after intralesional injections of 15-hydroxyeicosatetraenoic acid (15-HETE). J. Am. Acad. Dermatol. 18, 279–285. doi:10.1016/S0190-9622(88)70040-7
Fogh, K., Herlin, T., and Kragballe, K. (1989a). Eicosanoids in acute and chronic psoriatic lesions: Leukotriene B4, but not 12-hydroxy-eicosatetraenoic acid, is present in biologically active amounts in acute guttate lesions. J. Invest. Dermatol. 92, 837–841. doi:10.1111/1523-1747.ep12696858
Fogh, K., Herlin, T., and Kragballe, K. (1989b). Eicosanoids in skin of patients with atopic dermatitis: Prostaglandin E2 and leukotriene B4 are present in biologically active concentrations. J. Allergy Clin. Immunol. 83 (2), 450–455. doi:10.1016/0091-6749(89)90132-2
Fogh, K., Kiil, J., Herlin, T., Ternowitz, Th., and Kragballe, K. (1987). Heterogeneous distribution of lipoxygenase products in psoriatic skin lesions. Arch. Dermatol. Res. 279 (8), 504–511. doi:10.1007/BF00413280
Gan, Q. F., Witkop, G. L., Sloane, D. L., Straub, K. M., and Sigal, E. (1995). Identification of a specific methionine in mammalian 15-lipoxygenase which is oxygenated by the enzyme product 13-HPODE: Dissociation of sulfoxide formation from self-inactivation. Biochemistry 34 (21), 7069–7079. doi:10.1021/bi00021a019
Gertow, K., Nobili, E., Folkersen, L., Newman, J. W., Pedersen, T. L., Ekstrand, J., et al. (2011). 12- and 15-lipoxygenases in human carotid atherosclerotic lesions: Associations with cerebrovascular symptoms. Atherosclerosis 215 (2), 411–416. doi:10.1016/j.atherosclerosis.2011.01.015
Giménez-Arnau, A., Barranco, C., Alberola, M., Wale, C., Serrano, S., Buchanan, M. R., et al. (1997). Effects of linoleic acid supplements on atopic dermatitis. Adv. Exp. Med. Biol. 433, 285–289. doi:10.1007/978-1-4899-1810-9_60
Gonzalez, A. L., Roberts, R. L., Massion, P. P., Olson, S. J., Shyr, Y., and Shappell, S. B. (2004). 15-lipoxygenase-2 expression in benign and neoplastic lung: An immunohistochemical study and correlation with tumor grade and proliferation. Hum. Pathol. 35 (7), 840–849. doi:10.1016/j.humpath.2004.04.001
Goossens, C., Vander Perre, S., van den Berghe, G., and Langouche, L. (2017). Proliferation and differentiation of adipose tissue in prolonged lean and obese critically ill patients. Intensive Care Med. Exp. 5 (1), 16. doi:10.1186/s40635-017-0128-3
Gordon, S., and Martinez, F. O. (2010). Alternative activation of macrophages: Mechanism and functions. Immunity 32 (5), 593–604. doi:10.1016/j.immuni.2010.05.007
Gras, D., Bourdin, A., Vachier, I., de Senneville, L., Bonnans, C., and Chanez, P. (2012). An ex vivo model of severe asthma using reconstituted human bronchial epithelium. J. Allergy Clin. Immunol. 129 (5), 1259–1266. e1. doi:10.1016/j.jaci.2012.01.073
Green, A. R., Barbour, S., Horn, T., Carlos, J., Raskatov, J. A., and Holman, T. R. (2016). Strict regiospecificity of human epithelial 15-lipoxygenase-2 delineates its transcellular synthesis potential. Biochemistry 55 (20), 2832–2840. doi:10.1021/acs.biochem.5b01339
Grøn, B., Iversen, L., Ziboh, V., and Kragballe, K. (1993). Monohydroxy fatty acids esterified to phospholipids are decreased in lesional psoriatic skin. Arch. Dermatol. Res. 285 (8), 449–454. doi:10.1007/BF00376816
Gudjonsson, J. E., Ding, J., Li, X., Nair, R. P., Tejasvi, T., Qin, Z. S., et al. (2009). Global gene expression analysis reveals evidence for decreased lipid biosynthesis and increased innate immunity in uninvolved psoriatic skin. J. Invest. Dermatol. 129 (12), 2795–2804. doi:10.1038/jid.2009.173
Han, H., Xu, D., Liu, C., Claesson, H. E., Björkholm, M., and Sjöberg, J. (2014). Interleukin-4-mediated 15-lipoxygenase-1 trans-activation requires UTX recruitment and H3K27me3 demethylation at the promoter in A549 cells. PloS one 9 (1), e85085. doi:10.1371/journal.pone.0085085
Heidt, M., Fürstenberger, G., Vogel, S., Marks, F., and Krieg, P. (2000). Diversity of mouse lipoxygenases: Identification of a subfamily of epidermal isozymes exhibiting a differentiation-dependent mRNA expression pattern. Lipids 35 (7), 701–707. doi:10.1007/s11745-000-0576-0
Hellmann, J., Sansbury, B. E., Wong, B., Li, X., Singh, M., Nuutila, K., et al. (2018). Biosynthesis of D-series resolvins in skin provides insights into their role in tissue repair. J. Invest. Dermatol. 138 (9), 2051–2060. doi:10.1016/j.jid.2018.03.1498
Heydeck, D., Reisch, F., Schäfer, M., Kakularam, K. R., Roigas, S. A., Stehling, S., et al. (2022). The reaction specificity of mammalian ALOX15 orthologs is changed during late primate evolution and these alterations might offer evolutionary advantages for hominidae. Front. Cell. Dev. Biol. 10, 871585. doi:10.3389/fcell.2022.871585
Hinman, A., Holst, C. R., Latham, J. C., Bruegger, J. J., Ulas, G., McCusker, K. P., et al. (2018). Vitamin E hydroquinone is an endogenous regulator of ferroptosis via redox control of 15-lipoxygenase. PLoS One 13 (8), e0201369. doi:10.1371/journal.pone.0201369
Hoobler, E. K., Holz, C., and Holman, T. R. (2013). Pseudoperoxidase investigations of hydroperoxides and inhibitors with human lipoxygenases. Bioorg. Med. Chem. 21 (13), 3894–3899. doi:10.1016/j.bmc.2013.04.016
Hsi, L. C., Wilson, L. C., and Eling, T. E. (2002). Opposing effects of 15-lipoxygenase-1 and -2 metabolites on MAPK signaling in prostate: Alteration in peroxisome proliferator-activated receptor γ. J. Biol. Chem. 277 (43), 40549–40556. doi:10.1074/jbc.M203522200
Hu, Y., Sun, H., O'Flaherty, J. T., and Edwards, I. J. (2013). 15-Lipoxygenase-1-mediated metabolism of docosahexaenoic acid is required for syndecan-1 signaling and apoptosis in prostate cancer cells. Carcinogenesis 34 (1), 176–182. doi:10.1093/carcin/bgs324
Hultén, L. M., Olson, F. J., Åberg, H., Carlsson, J., Karlström, L., Borén, J., et al. (2010). 15-Lipoxygenase-2 is expressed in macrophages in human carotid plaques and regulated by hypoxia-inducible factor-1alpha. Eur. J. Clin. Invest. 40 (1), 11–17. doi:10.1111/j.1365-2362.2009.02223.x
Ivanov, I., Cruz, A., Zhuravlev, A., Di Venere, A., Nicolai, E., Stehling, S., et al. (2021). Conformational heterogeneity and cooperative effects of mammalian ALOX15. Int. J. Mol. Sci. 22 (6), 3285. doi:10.3390/ijms22063285
Ivanov, I., Heydeck, D., Hofheinz, K., Roffeis, J., O'Donnell, V. B., Kühn, H., et al. (2010). Molecular enzymology of lipoxygenases. Arch. Biochem. Biophys. 503 (2), 161–174. doi:10.1016/j.abb.2010.08.016
Ivanov, I., Kühn, H., and Heydeck, D. (2015). Structural and functional biology of arachidonic acid 15-lipoxygenase-1 (ALOX15). Gene 573 (1), 1–32. doi:10.1016/j.gene.2015.07.073
Ivanov, I., Shang, W., Toledo, L., Masgrau, L., Svergun, D. I., Stehling, S., et al. (2012). Ligand-induced formation of transient dimers of mammalian 12/15-lipoxygenase: A key to allosteric behavior of this class of enzymes? Proteins 80 (3), 703–712. doi:10.1002/prot.23227
Jack, G. S., Brash, A. R., Olson, S. J., Manning, S., Coffey, C. S., Smith, J. A., et al. (2000). Reduced 15-lipoxygenase-2 immunostaining in prostate adenocarcinoma: Correlation with grade and expression in high-grade prostatic intraepithelial neoplasia. Hum. Pathol. 31 (9), 1146–1154. doi:10.1053/hupa.2000.16670
Jäckle, K., Zeis, T., Schaeren-Wiemers, N., Junker, A., van der Meer, F., Kramann, N., et al. (2020). Molecular signature of slowly expanding lesions in progressive multiple sclerosis. Brain 143 (7), 2073–2088. doi:10.1093/brain/awaa158
Jacquot, C., Wecksler, A. T., McGinley, C. M., Segraves, E. N., Holman, T. R., and van der Donk, W. A. (2008). Isotope sensitive branching and kinetic isotope effects in the reaction of deuterated arachidonic acids with human 12- and 15-lipoxygenases. Biochemistry 47 (27), 7295–7303. doi:10.1021/bi800308q
Jakiela, B., Gielicz, A., Plutecka, H., Hubalewska, M., Mastalerz, L., Bochenek, G., et al. (2013). Eicosanoid biosynthesis during mucociliary and mucous metaplastic differentiation of bronchial epithelial cells. Prostagl. Other Lipid Mediat. 106, 116–123. doi:10.1016/j.prostaglandins.2013.05.001
Jameson, J. B., Kantz, A., Schultz, L., Kalyanaraman, C., Jacobson, M. P., Maloney, D. J., et al. (2014). A high throughput screen identifies potent and selective inhibitors to human epithelial 15-lipoxygenase-2. PloS one 9 (8), e104094. doi:10.1371/journal.pone.0104094
Jiang, M., Strand, D. W., Fernandez, S., He, Y., Yi, Y., Birbach, A., et al. (2010). Functional remodeling of benign human prostatic tissues in vivo by spontaneously immortalized progenitor and intermediate cells. Stem Cells 28 (2), 344–356. doi:10.1002/stem.284
Jiang, W. G., Watkins, G., Douglas-Jones, A., and Mansel, R. E. (2006). Reduction of isoforms of 15-lipoxygenase (15-LOX)-1 and 15-LOX-2 in human breast cancer. Prostagl. Leukot. Essent. Fat. Acids 74 (4), 235–245. doi:10.1016/j.plefa.2006.01.009
Jisaka, M., Kim, R. B., Boeglin, W. E., and Brash, A. R. (2000). Identification of amino acid determinants of the positional specificity of mouse 8S-lipoxygenase and human 15S-lipoxygenase-2. J. Biol. Chem. 275 (2), 1287–1293. doi:10.1074/jbc.275.2.1287
Jisaka, M., Kim, R. B., Boeglin, W. E., Nanney, L. B., and Brash, A. R. (1997). Molecular cloning and functional expression of a phorbol ester-inducible 8S-lipoxygenase from mouse skin. J. Biol. Chem. 272 (39), 24410–24416. doi:10.1074/jbc.272.39.24410
Jones, G. D., Russell, L., Darley-Usmar, V. M., Stone, D., and Wilson, M. T. (1996). Role of lipid hydroperoxides in the activation of 15-lipoxygenase. Biochemistry 35 (22), 7197–7203. doi:10.1021/bi952425h
Jones, M. L., Mark, P. J., Keelan, J. A., Barden, A., Mas, E., Mori, T. A., et al. (2013). Maternal dietary omega-3 fatty acid intake increases resolvin and protectin levels in the rat placenta. J. Lipid Res. 54 (8), 2247–2254. doi:10.1194/jlr.M039842
Joshi, N., Hoobler, E. K., Perry, S., Diaz, G., Fox, B., and Holman, T. R. (2013). Kinetic and structural investigations into the allosteric and pH effect on the substrate specificity of human epithelial 15-lipoxygenase-2. Biochemistry 52 (45), 8026–8035. doi:10.1021/bi4010649
Kakularam, K. R., Karst, F., Polamarasetty, A., Ivanov, I., Heydeck, D., and Kühn, H. (2022). Paralog- and ortholog-specificity of inhibitors of human and mouse lipoxygenase-isoforms. Biomed. Pharmacother. 145, 112434. doi:10.1016/j.biopha.2021.112434
Kamata, M., and Tada, Y. (2022). Dendritic cells and macrophages in the pathogenesis of psoriasis. Front. Immunol. 13, 941071. doi:10.3389/fimmu.2022.941071
Kelavkar, U., Lin, Y., Landsittel, D., Chandran, U., and Dhir, R. (2006). The yin and yang of 15-lipoxygenase-1 and delta-desaturases: Dietary omega-6 linoleic acid metabolic pathway in prostate. J. Carcinog. 5, 9. doi:10.1186/1477-3163-5-9
Kendall, A. C., Kiezel-Tsugunova, M., Brownbridge, L. C., Harwood, J. L., and Nicolaou, A. (2017). Lipid functions in skin: Differential effects of n-3 polyunsaturated fatty acids on cutaneous ceramides, in a human skin organ culture model. Biochim. Biophys. Acta. Biomembr. 1859, 1679–1689. doi:10.1016/j.bbamem.2017.03.016
Kensler, K. H., Regan, M. M., Heng, Y. J., Baker, G. M., Pyle, M. E., Schnitt, S. J., et al. (2019). Prognostic and predictive value of androgen receptor expression in postmenopausal women with estrogen receptor-positive breast cancer: Results from the breast international group trial 1–98. Breast Cancer Res. 21 (1), 30. doi:10.1186/s13058-019-1118-z
Kilty, I., Logan, A., and Vickers, P. J. (1999). Differential characteristics of human 15-lipoxygenase isozymes and a novel splice variant of 15S-lipoxygenase. Eur. J. Biochem. 266 (1), 83–93. doi:10.1046/j.1432-1327.1999.00818.x
Kim, A., Lim, S. M., Kim, J. H., and Seo, J. S. (2021). Integrative genomic and transcriptomic analyses of tumor suppressor genes and their role on tumor microenvironment and immunity in lung squamous cell carcinoma. Front. Immunol. 12, 598671. doi:10.3389/fimmu.2021.598671
Kim, E., Rundhaug, J. E., Benavides, F., Yang, P., Newman, R. A., and Fischer, S. M. (2005). An antitumorigenic role for murine 8S-lipoxygenase in skin carcinogenesis. Oncogene 24 (7), 1174–1187. doi:10.1038/sj.onc.1208269
Kobe, M. J., Neau, D. B., Mitchell, C. E., Bartlett, S. G., and Newcomer, M. E. (2014). The structure of human 15-lipoxygenase-2 with a substrate mimic. J. Biol. Chem. 289 (12), 8562–8569. doi:10.1074/jbc.M113.543777
Koelwyn, G. J., Corr, E. M., Erbay, E., and Moore, K. J. (2018). Regulation of macrophage immunometabolism in atherosclerosis. Nat. Immunol. 19 (6), 526–537. doi:10.1038/s41590-018-0113-3
Kort, W. J., Bijma, A. M., van Dam, J. J., van der Ham, A. C., Hekking, J. M., van der Ingh, ., et al. (1992). Eicosanoids in breast cancer patients before and after mastectomy. Prostagl. Leukot. Essent. Fat. Acids 45 (4), 319–327. doi:10.1016/0952-3278(92)90090-6
Kragballe, K., Duell, E. A., and Voorhees, J. J. (1986). Selective decrease of 15-hydroxyeicosatetraenoic acid (15-HETE) formation in uninvolved psoriatic dermis. Arch. Dermatol. 122 (8), 877–880. doi:10.1001/archderm.1986.01660200049012
Kreiß, M., Oberlis, J. H., Seuter, S., Bischoff-Kont, I., Sürün, D., Thomas, D., et al. (2022). Human 5-lipoxygenase regulates transcription by association to euchromatin. Biochem. Pharmacol. 203, 115187. doi:10.1016/j.bcp.2022.115187
Krieg, P., and Fürstenberger, G. (2014). The role of lipoxygenases in epidermis. Biochim. Biophys. Acta 1841 (3), 390–400. doi:10.1016/j.bbalip.2013.08.005
Krieg, P., Kinzig, A., Heidt, M., Marks, F., and Fürstenberger, G. (1998). cDNA cloning of a 8-lipoxygenase and a novel epidermis-type lipoxygenase from phorbol ester-treated mouse skin. Biochim. Biophys. Acta 1391 (1), 7–12. doi:10.1016/s0005-2760(97)00214-2
Kubat, N. J., Moffett, J., and Fray, L. M. (2015). Effect of pulsed electromagnetic field treatment on programmed resolution of inflammation pathway markers in human cells in culture. J. Inflamm. Res. 8, 59–69. doi:10.2147/JIR.S78631
Kühn, H., Banthiya, S., and van Leyen, K. (2015). Mammalian lipoxygenases and their biological relevance. Biochim. Biophys. Acta 1851 (4), 308–330. doi:10.1016/j.bbalip.2014.10.002
Kühn, H., Barnett, J., Grunberger, D., Baecker, P., Chow, J., Nguyen, B., et al. (1993). Overexpression, purification and characterization of human recombinant 15-lipoxygenase. Biochim. Biophys. Acta 1169 (1), 80–89. doi:10.1016/0005-2760(93)90085-N
Kühn, H. (1996). Biosynthesis, metabolization and biological importance of the primary 15-lipoxygenase metabolites 15-hydro(pero)xy-5Z, 8Z, 11Z, 13E-eicosatetraenoic acid and 13-hydro(pero)xy-9Z, 11E-octadecadienoic acid. Prog. Lipid Res. 35 (3), 203–226. doi:10.1016/S0163-7827(96)00008-2
Kühn, H., Gehring, T., Schroter, A., and Heydeck, D. (2016). Cytokine-dependent expression regulation of ALOX15. J. Cytokine Biol. 01 (02). doi:10.4172/2576-3881.1000106
Kühn, H., Humeniuk, L., Kozlov, N., Roigas, S., Adel, S., and Heydeck, D. (2018). The evolutionary hypothesis of reaction specificity of mammalian ALOX15 orthologs. Prog. Lipid Res. 72, 55–74. doi:10.1016/j.plipres.2018.09.002
Kühn, H., Saam, J., Eibach, S., Holzhütter, H. G., Ivanov, I., and Walther, M. (2005). Structural biology of mammalian lipoxygenases: Enzymatic consequences of targeted alterations of the protein structure. Biochem. Biophys. Res. Commun. 338 (1), 93–101. doi:10.1016/j.bbrc.2005.08.238
Kühn, H., Belkner, J., Suzuki, H., and Yamamoto, S. (1994). Oxidative modification of human lipoproteins by lipoxygenases of different positional specificities. J. Lipid Res. 35 (10), 1749–1759. doi:10.1016/s0022-2275(20)39770-4
Kühn, H., Belkner, J., Wiesner, R., and Brash, A. R. (1990). Oxygenation of biological membranes by the pure reticulocyte lipoxygenase. J. Biol. Chem. 265 (30), 18351–18361. doi:10.1016/s0021-9258(17)44759-4
Kutzner, L., Goloshchapova, K., Heydeck, D., Stehling, S., Kühn, H., and Schebb, N. H. (2017). Mammalian ALOX15 orthologs exhibit pronounced dual positional specificity with docosahexaenoic acid. Biochim. Biophys. Acta. Mol. Cell. Biol. Lipids 1862 (7), 666–675. doi:10.1016/j.bbalip.2017.04.001
Lafront, C., Germain, L., Weidmann, C., and Audet-Walsh, É. (2020). A systematic study of the impact of estrogens and selective estrogen receptor modulators on prostate cancer cell proliferation. Sci. Rep. 10 (1), 4024. doi:10.1038/s41598-020-60844-3
Lehmann, C., Homann, J., Ball, A. K., Blöcher, R., Kleinschmidt, T. K., Basavarajappa, D., et al. (2015). Lipoxin and resolvin biosynthesis is dependent on 5-lipoxygenase activating protein. FASEB J. 29 (12), 5029–5043. doi:10.1096/fj.15-275487
Lehnert, N., and Solomon, E. I. (2003). Density-functional investigation on the mechanism of H-atom abstraction by lipoxygenase. J. Biol. Inorg. Chem. 8 (3), 294–305. doi:10.1007/s00775-002-0415-6
Li, B., Tsoi, L. C., Swindell, W. R., Gudjonsson, J. E., Tejasvi, T., Johnston, A., et al. (2014). Transcriptome analysis of psoriasis in a large case–control sample: RNA-seq provides insights into disease mechanisms. J. Invest. Dermatol. 134 (7), 1828–1838. doi:10.1038/jid.2014.28
Li, M. Y., Liu, L. Z., Li, W., Ng, C. S. H., Liu, Yi, Kong, A. W. Y., et al. (2019). Ambient fine particulate matter inhibits 15-lipoxygenases to promote lung carcinogenesis. J. Exp. Clin. Cancer Res. 38 (1), 359. doi:10.1186/s13046-019-1380-z
Li, M. Y., Yuan, H. L., Ko, F. W. S., Wu, B., Long, X., Du, J., et al. (2015). Antineoplastic effects of 15(S)-hydroxyeicosatetraenoic acid and 13-S-hydroxyoctadecadienoic acid in non–small cell lung cancer. Cancer 121 (S17), 3130–3145. doi:10.1002/cncr.29547
Lin, L., Yu, W., Zhang, W., Li, S., Hu, S., Jiang, B., et al. (2021). Expression profile of lipoxygenases in gingival tissues of human periodontitis. Oral Dis. 27 (3), 567–576. doi:10.1111/odi.13558
Liu, Y., Tang, X., Lu, C., Han, W., Guo, S., and Zhu, D. (2009). Expression of 15-lipoxygenases in pulmonary arteries after hypoxia. Pathology 41 (5), 476–483. doi:10.1080/00313020903040970
Ludwig, P., Holzhütter, H. G., Colosimo, A., Silvestrini, M. C., Schewe, T., and Rapoport, S. M. (1987). A kinetic model for lipoxygenases based on experimental data with the lipoxygenase of reticulocytes. Eur. J. Biochem. 168 (2), 325–337. doi:10.1111/j.1432-1033.1987.tb13424.x
Mabalirajan, U., Rehman, R., Ahmad, T., Kumar, S., Singh, S., Leishangthem, G. D., et al. (2013). Linoleic acid metabolite drives severe asthma by causing airway epithelial injury. Sci. Rep. 3, 1349. doi:10.1038/srep01349
Magnusson, L. U., Lundqvist, A., Asp, J., Synnergren, J., Johansson, C. T., Palmqvist, L., et al. (2012). High expression of arachidonate 15-lipoxygenase and proinflammatory markers in human ischemic heart tissue. Biochem. Biophys. Res. Commun. 424 (2), 327–330. doi:10.1016/j.bbrc.2012.06.117
Mangino, M. J., Brounts, L., Harms, B., and Heise, C. (2006). Lipoxin biosynthesis in inflammatory bowel disease. Prostagl. Other Lipid Mediat. 79 (1-2), 84–92. doi:10.1016/j.prostaglandins.2005.10.004
Mao, J. T., Nie, W. X., Tsu, I. H., Jin, Y. S., Rao, J. Y., Lu, Q. Y., et al. (2010). White tea extract induces apoptosis in non-small cell lung cancer cells: The role of peroxisome proliferator-activated receptor-{gamma} and 15-lipoxygenases. Cancer Prev. Res. 3 (9), 1132–1140. doi:10.1158/1940-6207.CAPR-09-0264
Mao, J. T., Smoake, J., Park, H. K., Lu, Q. Y., and Xue, B. (2016). Grape seed procyanidin extract mediates antineoplastic effects against lung cancer via modulations of prostacyclin and 15-HETE eicosanoid pathways. Cancer Prev. Res. 9 (12), 925–932. doi:10.1158/1940-6207.CAPR-16-0122
Mao, X., Li, W., Chen, W., Li, Y., Wang, Q., Wang, X., et al. (2020). Exploring and characterizing a novel combination of paeoniflorin and talatizidine for the treatment of rheumatoid arthritis. Pharmacol. Res. 153, 104658. doi:10.1016/j.phrs.2020.104658
Mascayano, C., Espinosa, V., Sepúlveda-Boza, S., Hoobler, E. K., and Perry, S. (2013). In vitro study of isoflavones and isoflavans as potent inhibitors of human 12- and 15-lipoxygenases. Chem. Biol. Drug Des. 82 (3), 317–325. doi:10.1111/cbdd.12157
Meng, F., Qiu, J., Chen, H., Shi, X., Yin, M., Zhu, M., et al. (2021). Dietary supplementation with N-3 polyunsaturated fatty acid-enriched fish oil promotes wound healing after ultraviolet B-induced sunburn in mice. Food Sci. Nutr. 9 (7), 3693–3700. doi:10.1002/fsn3.2330
Michielan, A., and D'Incà, R. (2015). Intestinal permeability in inflammatory bowel disease: Pathogenesis, clinical evaluation, and therapy of leaky gut. Mediat. Inflamm. 2015, 628157. doi:10.1155/2015/628157
Muga, S. J., Thuillier, P., Pavone, A., Rundhaug, J. E., Boeglin, W. E., Jisaka, M., et al. (2000). 8S-lipoxygenase products activate peroxisome proliferator-activated receptor alpha and induce differentiation in murine keratinocytes. Cell. Growth Differ. 11 (8), 447–454.
Nagy, L., Tontonoz, P., Alvarez, J. G. A., Chen, H., and Evans, R. M. (1998). Oxidized LDL regulates macrophage gene expression through ligand activation of PPARgamma. Cell. 93 (2), 229–240. doi:10.1016/S0092-8674(00)81574-3
Neau, D. B., Bender, G., Boeglin, W. E., Bartlett, S. G., Brash, A. R., and Newcomer, M. E. (2014). Crystal structure of a lipoxygenase in complex with substrate: The arachidonic acid-binding site of 8R-lipoxygenase. J. Biol. Chem. 289 (46), 31905–31913. doi:10.1074/jbc.M114.599662
Neau, D. B., Gilbert, N. C., Bartlett, S. G., Boeglin, W., Brash, A. R., and Newcomer, M. E. (2009). The 185 A structure of an 8R-lipoxygenase suggests a general model for lipoxygenase product specificity. Biochemistry 48 (33), 7906–7915. doi:10.1021/bi900084m
Newcomer, M. E., and Brash, A. R. (2015). The structural basis for specificity in lipoxygenase catalysis. Protein Sci. 24 (3), 298–309. doi:10.1002/pro.2626
Nieddu, E., Pollarolo, B., Merello, L., Schenone, S., and Mazzei, M. (2013). F508del-CFTR rescue: A matter of cell stress response. Curr. Pharm. Des. 19 (19), 3476–3496. doi:10.2174/13816128113199990317
Nony, P. A., Kennett, S. B., Glasgow, W. C., Olden, K., and Roberts, J. D. (2005). 15(S)-Lipoxygenase-2 mediates arachidonic acid-stimulated adhesion of human breast carcinoma cells through the activation of TAK1, MKK6, and p38 MAPK. J. Biol. Chem. 280 (36), 31413–31419. doi:10.1074/jbc.M500418200
O'Donnell, V., Schebb, N. H., Milne, G. L., Murphy, M. P., Thomas, C. P., Dieter, S., et al. (2021). Failure to apply standard limit-of-detection or limit-of-quantitation criteria to specialized pro-resolving mediator analysis incorrectly characterizes their presence in biological samples. Zenodo 1, 1. Preprint. doi:10.5281/ZENODO.5766267
O'Flaherty, J. T., Hu, Y., Wooten, R. E., Horita, D. A., Samuel, M. P., Thomas, M. J., et al. (2012). 15-lipoxygenase metabolites of docosahexaenoic acid inhibit prostate cancer cell proliferation and survival. PloS one 7 (9), e45480. doi:10.1371/journal.pone.0045480
Offenbacher, A. R., and Holman, T. R. (2020). Fatty acid allosteric regulation of C-H activation in plant and animal lipoxygenases. Mol. (Basel, Switz. 25 (15), E3374. doi:10.3390/molecules25153374
Ogawa, E., Owada, Y., Ikawa, S., Adachi, Y., Egawa, T., Nemoto, K., et al. (2011). Epidermal FABP (FABP5) regulates keratinocyte differentiation by 13(S)-HODE-mediated activation of the NF-κB signaling pathway. J. Invest. Dermatol. 131 (3), 604–612. doi:10.1038/jid.2010.342
Pasqualini, M. E., Heyd, V. L., Manzo, P., and Eynard, A. R. (2003). Association between E-cadherin expression by human colon, bladder and breast cancer cells and the 13-HODE:15-HETE ratio A possible role of their metastatic potential. Prostagl. Leukot. Essent. Fat. Acids 68 (1), 9–16. doi:10.1016/S0952-3278(02)00230-2
Pein, H., Ville, A., Pace, S., Temml, V., Garscha, U., Raasch, M., et al. (2018). Endogenous metabolites of vitamin E limit inflammation by targeting 5-lipoxygenase. Nat. Commun. 9 (1), 3834. doi:10.1038/s41467-018-06158-5
Perry, S. C., Horn, T., Tourdot, B. E., Yamaguchi, A., Kalyanaraman, C., Conrad, W. S., et al. (2020a). Role of human 15-lipoxygenase-2 in the biosynthesis of the lipoxin intermediate, 5S, 15S-diHpETE, implicated with the altered positional specificity of human 15-lipoxygenase-1. Biochemistry 59 (42), 4118–4130. doi:10.1021/acs.biochem.0c00622
Perry, S. C., Kalyanaraman, C., Tourdot, B. E., Conrad, W. S., Akinkugbe, O., Freedman, J. C., et al. (2020b). 15-Lipoxygenase-1 biosynthesis of 7S, 14S-diHDHA implicates 15-lipoxygenase-2 in biosynthesis of resolvin D5. J. Lipid Res. 61 (7), 1087–1103. doi:10.1194/jlr.RA120000777
Pickens, C. A., Sordillo, L. M., Zhang, C., and Fenton, J. I. (2017). Obesity is positively associated with arachidonic acid-derived 5- and 11-hydroxyeicosatetraenoic acid (HETE). Metabolism. 70, 177–191. doi:10.1016/j.metabol.2017.01.034
Pipper, C., Bordag, N., Reiter, B., Economides, K., Florian, P., Birngruber, T., et al. (2019). LC/MS/MS analyses of open-flow microperfusion samples quantify eicosanoids in a rat model of skin inflammation. J. Lipid Res. 60 (4), 758–766. doi:10.1194/jlr.M087221
Planagumà, A., Kazani, S., Marigowda, G., Haworth, O., Mariani, T. J., Israel, E., et al. (2008). Airway lipoxin A4 generation and lipoxin A4 receptor expression are decreased in severe asthma. Am. J. Respir. Crit. Care Med. 178 (6), 574–582. doi:10.1164/rccm.200801-061OC
Pochard, C., Coquenlorge, S., Jaulin, J., Cenac, N., Vergnolle, N., Meurette, G., et al. (2016). Defects in 15-HETE production and control of epithelial permeability by human enteric glial cells from patients with crohn's disease. Gastroenterology 150 (1), 168–180. doi:10.1053/j.gastro.2015.09.038
Prasad, V. V. T. S., and Gopalan, R. O. G. (2015). Continued use of MDA-MB-435, a melanoma cell line, as a model for human breast cancer, even in year, 2014. npj Breast Cancer 1 (1), 15002. doi:10.1038/npjbcancer.2015.2
Profita, M., Sala, A., Riccobono, L., Paternò, A., Mirabella, A., Bonanno, A., et al. (2000). 15-Lipoxygenase expression and 15(S)-hydroxyeicoisatetraenoic acid release and reincorporation in induced sputum of asthmatic subjects. J. Allergy Clin. Immunol. 105 (4), 711–716. doi:10.1067/mai.2000.105122
Ramery, E., Fraipont, A., Richard, E. A., Art, T., Pirottin, D., van Delm, W., et al. (2015). Expression microarray as a tool to identify differentially expressed genes in horses suffering from inflammatory airway disease. Vet. Clin. Pathol. 44 (1), 37–46. doi:10.1111/vcp.12216
Rapoport, S., Härtel, B., Schewe, T., and Kühn, H. (1986). Hydroperoxyfatty acids inactivate the reticulocyte lipoxygenase independently of a hydroperoxidase reaction. FEBS Lett. 202 (2), 202–206. doi:10.1016/0014-5793(86)80687-1
Reinartz, S., Finkernagel, F., Adhikary, T., Rohnalter, V., Schumann, T., Schober, Y., et al. (2016). A transcriptome-based global map of signaling pathways in the ovarian cancer microenvironment associated with clinical outcome. Genome Biol. 17 (1), 108. doi:10.1186/s13059-016-0956-6
Ringholz, F. C., Buchanan, P. J., Clarke, D. T., Millar, R. G., McDermott, M., Linnane, B., et al. (2014). Reduced 15-lipoxygenase 2 and lipoxin A4/leukotriene B4 ratio in children with cystic fibrosis. Eur. Respir. J. 44 (2), 394–404. doi:10.1183/09031936.00106013
Roffeis, J., Hornung, D., Kühn, H., and Walther, M. (2007). 15-Lipoxygenase-2 is differentially expressed in normal and neoplastic ovary. Eur. J. Cancer Prev. 16 (6), 568–575. doi:10.1097/CEJ.0b013e32801023c4
Russell, D. G., Huang, L., and Vander Ven, B. C. (2019). Immunometabolism at the interface between macrophages and pathogens. Nat. Rev. Immunol. 19 (5), 291–304. doi:10.1038/s41577-019-0124-9
Russell, R., Gori, I., Pellegrini, C., Kumar, R., Achtari, C., and Canny, G. O. (2011). Lipoxin A4 is a novel estrogen receptor modulator. FASEB J. 25 (12), 4326–4337. doi:10.1096/fj.11-187658
Rydberg, E. K., Krettek, A., Ullström, C., Ekström, K., Svensson, P. A., Carlsson, L. M. S., et al. (2004). Hypoxia increases LDL oxidation and expression of 15-lipoxygenase-2 in human macrophages. Arterioscler. Thromb. Vasc. Biol. 24 (11), 2040–2045. doi:10.1161/01.ATV.0000144951.08072.0b
Safizadeh, B., Hoshyar, R., Mehrpour, M., Eftekhar, M., Salimi, V., Yazdani, S., et al. (2018). The role of expression and activity of 15-Lipoxygenase isoforms and related cytokines in patients with Multiple Sclerosis and healthy controls. J. Neuroimmunol. 325, 32–42. doi:10.1016/j.jneuroim.2018.10.009
Sandstedt, M., Rotter Sopasakis, V., Lundqvist, A., Vukusic, K., Oldfors, A., Dellgren, G., et al. (2018). Hypoxic cardiac fibroblasts from failing human hearts decrease cardiomyocyte beating frequency in an ALOX15 dependent manner. PLoS One 13 (8), e0202693. doi:10.1371/journal.pone.0202693
Sausville, L. N., Jones, C. C., Aldrich, M. C., Blot, W. J., Pozzi, A., and Williams, S. M. (2017). Genetic variation in the eicosanoid pathway is associated with non-small-cell lung cancer (NSCLC) survival. PLoS One 12 (7), e0180471. doi:10.1371/journal.pone.0180471
Savari, S., Vinnakota, K., Zhang, Y., and Sjölander, A. (2014). Cysteinyl leukotrienes and their receptors: Bridging inflammation and colorectal cancer. World J. Gastroenterol. 20 (4), 968–977. doi:10.3748/wjg.v20.i4.968
Schäfer, M., Kakularam, K. R., Reisch, F., Rothe, M., Stehling, S., Heydeck, D., et al. (2022). Male knock-in mice expressing an arachidonic acid lipoxygenase 15B (Alox15B) with humanized reaction specificity are prematurely growth arrested when aging. Biomedicines 10 (6), 1379. doi:10.3390/biomedicines10061379
Schebb, N. H., Kühn, H., Kahnt, A. S., Rund, K. M., O'Donnell, V. B., Flamand, N., et al. (2022). Formation, signaling and occurrence of specialized pro-resolving lipid mediators-what is the evidence so far? Front. Pharmacol. 13, 838782. doi:10.3389/fphar.2022.838782
Schewe, T., Halangk, W., Hiebsch, Ch., and Rapoport, S. M. (1975). A lipoxygenase in rabbit reticulocytes which attacks phospholipids and intact mitochondria. FEBS Lett. 60 (1), 149–152. doi:10.1016/0014-5793(75)80439-X
Schneider, C., Strayhorn, W. D., Brantley, D. M., Nanney, L. B., Yull, F. E., and Brash, A. R. (2004). Upregulation of 8-lipoxygenase in the dermatitis of IkappaB-alpha-deficient mice. J. Invest. Dermatol. 122 (3), 691–698. doi:10.1111/j.0022-202X.2004.22329.x
Schweiger, D., Fürstenberger, G., and Krieg, P. (2007). Inducible expression of 15-lipoxygenase-2 and 8-lipoxygenase inhibits cell growth via common signaling pathways. J. Lipid Res. 48 (3), 553–564. doi:10.1194/jlr.M600311-JLR200
Sehnal, D., Bittrich, S., Deshpande, M., Svobodová, R., Berka, K., Bazgier, V., et al. (2021). Mol* viewer: Modern web app for 3D visualization and analysis of large biomolecular structures. Nucleic Acids Res. 49 (W1), W431–W437. doi:10.1093/nar/gkab314
Serhan, C. N., Clish, C. B., Brannon, J., Colgan, S. P., Chiang, N., and Gronert, K. (2000). Novel functional sets of lipid-derived mediators with antiinflammatory actions generated from omega-3 fatty acids via cyclooxygenase 2-nonsteroidal antiinflammatory drugs and transcellular processing. J. Exp. Med. 192 (8), 1197–1204. doi:10.1084/jem.192.8.1197
Serhan, C. N., Hong, S., Gronert, K., Colgan, S. P., Devchand, P. R., Mirick, G., et al. (2002). Resolvins: A family of bioactive products of omega-3 fatty acid transformation circuits initiated by aspirin treatment that counter proinflammation signals. J. Exp. Med. 196 (8), 1025–1037. doi:10.1084/jem.20020760
Serhan, C. N., and Levy, B. D. (2018). Resolvins in inflammation: Emergence of the pro-resolving superfamily of mediators. J. Clin. Invest. 128 (7), 2657–2669. doi:10.1172/JCI97943
Serhan, C. N., Yang, R., Martinod, K., Kasuga, K., Pillai, P. S., Porter, T. F., et al. (2009). Maresins: Novel macrophage mediators with potent antiinflammatory and proresolving actions. J. Exp. Med. 206 (1), 15–23. doi:10.1084/jem.20081880
Setsu, N., Matsuura, H., Hirakawa, S., Arata, J., and Iwatsuki, K. (2006). Interferon-gamma-induced 15-lipoxygenase-2 expression in normal human epidermal keratinocytes and a pathogenic link to psoriasis vulgaris. Eur. J. Dermatol. 16 (2), 141–145.
Shan, R., Chen, L., Li, X., Wu, H., Liang, Q., and Tang, X. (2012). Hypoxia promotes rabbit pulmonary artery smooth muscle cells proliferation through a 15-LOX-2 product 15(S)-hydroxyeicosatetraenoic acid. Prostagl. Leukot. Essent. Fat. Acids 86 (1-2), 85–90. doi:10.1016/j.plefa.2011.10.001
Shappell, S. B., Boeglin, W. E., Olson, S. J., Kasper, S., and Brash, A. R. (1999). 15-lipoxygenase-2 (15-LOX-2) is expressed in benign prostatic epithelium and reduced in prostate adenocarcinoma. Am. J. Pathol. 155 (1), 235–245. doi:10.1016/S0002-9440(10)65117-6
Shappell, S. B., Gupta, R. A., Manning, S., Whitehead, R., Boeglin, W. E., Schneider, C., et al. (2001c). 15S-Hydroxyeicosatetraenoic acid activates peroxisome proliferator-activated receptor gamma and inhibits proliferation in PC3 prostate carcinoma cells. Cancer Res. 61 (2), 497–503.
Shappell, S. B., Keeney, D. S., Zhang, J., Page, R., Olson, S. J., and Brash, A. R. (2001a). 15-Lipoxygenase-2 expression in benign and neoplastic sebaceous glands and other cutaneous adnexa. J. Invest. Dermatol. 117 (1), 36–43. doi:10.1046/j.1523-1747.2001.01378.x
Shappell, S. B., Manning, S., Boeglin, W. E., Guan, Y. F., Roberts, R. L., Davis, L., et al. (2001b). Alterations in lipoxygenase and cyclooxygenase-2 catalytic activity and mRNA expression in prostate carcinoma. Neoplasia 3 (4), 287–303. doi:10.1038/sj.neo.7900166
Shappell, S. B., Olson, S. J., Hannah, S. E., Manning, S., Roberts, R. L., Masumori, N., et al. (2003). Elevated expression of 12/15-lipoxygenase and cyclooxygenase-2 in a transgenic mouse model of prostate carcinoma. Cancer Res. 63 (9), 2256–2267.
Shen, M., Vermeulen, R., Rajaraman, P., Menashe, I., He, X., Chapman, R. S., et al. (2009). Polymorphisms in innate immunity genes and lung cancer risk in Xuanwei, China. Environ. Mol. Mutagen. 50 (4), 285–290. doi:10.1002/em.20452
Shen, T., Ma, J., Zhang, L., Yu, X., Liu, M., Hou, Y., et al. (2013). Positive feedback-loop of telomerase reverse transcriptase and 15-lipoxygenase-2 promotes pulmonary hypertension. PloS one 8 (12), e83132. doi:10.1371/journal.pone.0083132
Shum, M., London, C. M., Briottet, M., Sy, K. A., Baillif, V., Philippe, R., et al. (2022). CF patients' airway epithelium and sex contribute to biosynthesis defects of pro-resolving lipids. Front. Immunol. 13, 915261. doi:10.3389/fimmu.2022.915261
Shureiqi, I., Chen, D., Day, R. S., Zuo, X., Hochman, F. L., Ross, W. A., et al. (2010). Profiling lipoxygenase metabolism in specific steps of colorectal tumorigenesis. Cancer Prev. Res. 3 (7), 829–838. doi:10.1158/1940-6207.CAPR-09-0110
Simard, M., Grenier, A., Rioux, G., Tremblay, A., Blais, I., Flamand, N., et al. (2022). Remodeling of the dermal extracellular matrix in a tissue-engineered psoriatic skin model by n-3 polyunsaturated fatty acids. Biomedicines 10 (5), 1078. doi:10.3390/biomedicines10051078
Simard, M., Julien, P., Fradette, J., and Pouliot, R. (2019). Modulation of the lipid profile of reconstructed skin substitutes after essential fatty acid supplementation affects testosterone permeability. Cells 8 (10), E1142. doi:10.3390/cells8101142
Simard-Bisson, C., Parent, L. A., Moulin, V. J., and de Laclos, B. F. (2018). Characterization of epidermal lipoxygenase expression in normal human skin and tissue-engineered skin substitutes. J. Histochem. Cytochem. 66 (11), 813–824. Skin science foundation bioinformatics hub, checked on 8/18/2022. doi:10.1369/0022155418788117
Snodgrass, R. G., Benatzy, Y., Schmid, T., Namgaladze, D., Mainka, M., Schebb, N. H., et al. (2021). Efferocytosis potentiates the expression of arachidonate 15-lipoxygenase (ALOX15) in alternatively activated human macrophages through LXR activation. Cell. Death Differ. 28 (4), 1301–1316. doi:10.1038/s41418-020-00652-4
Snodgrass, R. G., and Brüne, B. (2019). Regulation and functions of 15-lipoxygenases in human macrophages. Front. Pharmacol. 10, 719. doi:10.3389/fphar.2019.00719
Snodgrass, R. G., Zezina, E., Namgaladze, D., Gupta, S., Angioni, C., Geisslinger, G., et al. (2018). A novel function for 15-lipoxygenases in cholesterol homeostasis and CCL17 production in human macrophages. Front. Immunol. 9, 1906. doi:10.3389/fimmu.2018.01906
Solomon, E. I., Zhou, J., Neese, F., and Pavel, E. G. (1997). New insights from spectroscopy into the structure/function relationships of lipoxygenases. Chem. Biol. 4 (11), 795–808. doi:10.1016/s1074-5521(97)90113-7
Song, Y. S., Lee, D. H., Yu, J. H., Oh, D. K., Hong, J. T., and Yoon, D. Y. (2016). Promotion of adipogenesis by 15-(S)-hydroxyeicosatetraenoic acid. Prostagl. Other Lipid Mediat. 123, 1–8. doi:10.1016/j.prostaglandins.2016.02.001
Soon, P. S. H., Libe, R., Benn, D. E., Gill, A., Shaw, J., Sywak, M. S., et al. (2008). Loss of heterozygosity of 17p13, with possible involvement of ACADVL and ALOX15B, in the pathogenesis of adrenocortical tumors. Ann. Surg. 247 (1), 157–164. Available online at: https://journals.lww.com/annalsofsurgery/Fulltext/2008/01000/Loss_of_Heterozygosity_of_17p13, _With_Possible.23.aspx (Accessed June 16, 2022). doi:10.1097/SLA.0b013e318153ff55
Sorokin, A. V., Domenichiello, A. F., Dey, A. K., Yuan, Z. X., Goyal, A., Rose, S. M., et al. (2018). Bioactive lipid mediator profiles in human psoriasis skin and blood. J. Invest. Dermatol. 138 (7), 1518–1528. doi:10.1016/j.jid.2018.02.003
Spann, N. J., Garmire, L. X., McDonald, J. G., Myers, D. S., Milne, S. B., Shibata, N., et al. (2012). Regulated accumulation of desmosterol integrates macrophage lipid metabolism and inflammatory responses. Cell. 151 (1), 138–152. doi:10.1016/j.cell.2012.06.054
Spite, M., Norling, L. V., Summers, L., Yang, R., Cooper, D., Petasis, N. A., et al. (2009). Resolvin D2 is a potent regulator of leukocytes and controls microbial sepsis. Nature 461 (7268), 1287–1291. doi:10.1038/nature08541
Suardíaz, R., Jambrina, P. G., Masgrau, L., González-Lafont, À., Rosta, E., and Lluch, J. M. (2016). Understanding the mechanism of the hydrogen abstraction from arachidonic acid catalyzed by the human enzyme 15-lipoxygenase-2 A quantum mechanics/molecular mechanics free energy simulation. J. Chem. Theory Comput. 12 (4), 2079–2090. doi:10.1021/acs.jctc.5b01236
Subbarayan, V., Sabichi, A. L., Kim, J., Llansa, N., Logothetis, C. J., Lippman, S. M., et al. (2004). Differential peroxisome proliferator-activated receptor-gamma isoform expression and agonist effects in normal and malignant prostate cells. Cancer Epidemiol. Biomarkers Prev. 13, 1710–1716. doi:10.1158/1055-9965.1710.13.11
Subbarayan, V., Xu, X. C., Kim, J., Yang, P., Hoque, A., Sabichi, A. L., et al. (2005). Inverse relationship between 15-lipoxygenase-2 and PPAR-gamma gene expression in normal epithelia compared with tumor epithelia. Neoplasia 7 (3), 280–293. doi:10.1593/neo.04457
Subbarayan, V., Krieg, P., Hsi, L. C., Kim, J., Yang, P., Sabichi, A. L., et al. (2006). 15-Lipoxygenase-2 gene regulation by its product 15-(S)-hydroxyeicosatetraenoic acid through a negative feedback mechanism that involves peroxisome proliferator-activated receptor gamma. Oncogene 25 (44), 6015–6025. doi:10.1038/sj.onc.1209617
Sun, L., Xu, Y. W., Han, J., Liang, H., Wang, N., and Cheng, Y. (2015). 12/15-Lipoxygenase metabolites of arachidonic acid activate PPARγ: A possible neuroprotective effect in ischemic brain. J. Lipid Res. 56 (3), 502–514. doi:10.1194/jlr.M053058
Suraneni, M. V., Moore, J. R., Zhang, D., Badeaux, M., Macaluso, M. D., DiGiovanni, J., et al. (2014). Tumor-suppressive functions of 15-Lipoxygenase-2 and RB1CC1 in prostate cancer. Cell. cycleGeorget. Tex.) 13 (11), 1798–1810. doi:10.4161/cc.28757
Suraneni, M. V., Schneider-Broussard, R., Moore, J. R., Davis, T. C., Maldonado, C. J., Li, H., et al. (2010). Transgenic expression of 15-lipoxygenase 2 (15-LOX2) in mouse prostate leads to hyperplasia and cell senescence. Oncogene 29 (30), 4261–4275. doi:10.1038/onc.2010.197
Takeichi, T., Kinoshita, F., Tanaka, H., Fujita, S., Kobayashi, Y., Nakatochi, M., et al. (2019). The lipoxygenase-hepoxilin pathway is activated in cutaneous plaque lesions of psoriasis. J. Cutan. Immunol. Allergy 2 (1), 15–24. doi:10.1002/cia2.12039
Tang, D. G., Bhatia, B., Tang, S., and Robin, S. B. (2007). 15-Lipoxygenase 2 (15-LOX2) is a functional tumor suppressor that regulates human prostate epithelial cell differentiation, senescence, and growth (size). Prostagl. Other Lipid Mediat. 82 (1), 135–146. doi:10.1016/j.prostaglandins.2006.05.022
Tang, S., Bhatia, B., Maldonado, C. J., Yang, P., Newman, R. A., Liu, J., et al. (2002). Evidence that arachidonate 15-lipoxygenase 2 is a negative cell cycle regulator in normal prostate epithelial cells. J. Biol. Chem. 277 (18), 16189–16201. doi:10.1074/jbc.M111936200
Tang, S., Bhatia, B., Zhou, J., Maldonado, C. J., Chandra, D., Kim, E., et al. (2004). Evidence that Sp1 positively and Sp3 negatively regulate and androgen does not directly regulate functional tumor suppressor 15-lipoxygenase 2 (15-LOX2) gene expression in normal human prostate epithelial cells. Oncogene 23 (41), 6942–6953. doi:10.1038/sj.onc.1207913
Tang, Y., Wang, M. T., Chen, Y., Yang, D., Che, M., Honn, K. V., et al. (2009). Downregulation of vascular endothelial growth factor and induction of tumor dormancy by 15-lipoxygenase-2 in prostate cancer. Int. J. Cancer 124 (7), 1545–1551. doi:10.1002/ijc.24118
Teopompi, E., Risé, P., Pisi, R., Buccellati, C., Aiello, M., Pisi, G., et al. (2019). Arachidonic acid and docosahexaenoic acid metabolites in the airways of adults with cystic fibrosis: Effect of docosahexaenoic acid supplementation. Front. Pharmacol. 10, 938. doi:10.3389/fphar.2019.00938
Thuillier, P., Brash, A. R., Kehrer, J. P., Stimmel, J. B., Leesnitzer, L. M., Yang, P., et al. (2002). Inhibition of peroxisome proliferator-activated receptor (PPAR)-mediated keratinocyte differentiation by lipoxygenase inhibitors. Biochem. J. 366, 901–910. doi:10.1042/BJ20020377
Tian, W., Liu, J., Pei, B., Wang, X., Guo, Y., and Yuan, L. (2016). Identification of miRNAs and differentially expressed genes in early phase non-small cell lung cancer. Oncol. Rep. 35 (4), 2171–2176. doi:10.3892/or.2016.4561
Torosyan, Y., Dobi, A., Naga, S., Mezhevaya, K., Glasman, M., Norris, C., et al. (2006). Distinct effects of annexin A7 and p53 on arachidonate lipoxygenation in prostate cancer cells involve 5-lipoxygenase transcription. Cancer Res. 66 (19), 9609–9616. doi:10.1158/0008-5472.CAN-06-1574
Tsai, W. C., Gilbert, N. C., Ohler, A., Armstrong, M., Perry, S., Kalyanaraman, C., et al. (2021a). Kinetic and structural investigations of novel inhibitors of human epithelial 15-lipoxygenase-2. Bioorg. Med. Chem. 46, 116349. doi:10.1016/j.bmc.2021.116349
Tsai, W. C., Kalyanaraman, C., Yamaguchi, A., Holinstat, M., Jacobson, M. P., and Holman, T. R. (2021b). In vitro biosynthetic pathway investigations of neuroprotectin D1 (NPD1) and protectin DX (PDX) by human 12-lipoxygenase, 15-lipoxygenase-1, and 15-lipoxygenase-2. Biochemistry 60 (22), 1741–1754. doi:10.1021/acs.biochem.0c00931
Tyrrell, V. J., Ali, F., Boeglin, W. E., Andrews, R., Burston, J., Birchall, J. C., et al. (2021). Lipidomic and transcriptional analysis of the linoleoyl-omega-hydroxyceramide biosynthetic pathway in human psoriatic lesions. J. Lipid Res. 62, 100094. doi:10.1016/j.jlr.2021.100094
Vaezi, M. A., Safizadeh, B., Eghtedari, A. R., Ghorbanhosseini, S. S., Rastegar, M., Salimi, V., et al. (2021). 15-Lipoxygenase and its metabolites in the pathogenesis of breast cancer: A double-edged sword. Lipids Health Dis. 20 (1), 169. doi:10.1186/s12944-021-01599-2
Vasiliou, S. K., Filippou, P. S., Clotet-Freixas, S., Soosaipillai, A., Batruch, I., Foivos, V. T., et al. (2022). Transcriptome profiling and proteomic validation reveals targets of the androgen receptor signaling in the BT-474 breast cancer cell line. Clin. Proteomics 19 (1), 14. doi:10.1186/s12014-022-09352-2
Vasquez-Martinez, Y., Ohri, R. V., Kenyon, V., Holman, T. R., and Sepúlveda-Boza, S. (2007). Structure-activity relationship studies of flavonoids as potent inhibitors of human platelet 12-hLO, reticulocyte 15-hLO-1, and prostate epithelial 15-hLO-2. Bioorg. Med. Chem. 15 (23), 7408–7425. doi:10.1016/j.bmc.2007.07.036
Vestergaard, C., Just, H., Jane, B. N., Thestrup-Pedersen, K., and Deleuran, M. (2004). Expression of CCR2 on monocytes and macrophages in chronically inflamed skin in atopic dermatitis and psoriasis. Acta Derm. Venereol. 84 (5), 353–358. doi:10.1080/00015550410034444
Vijil, C., Hermansson, C., Jeppsson, A., Bergström, G., and Hultén, L. M. (2014). Arachidonate 15-lipoxygenase enzyme products increase platelet aggregation and thrombin generation. PloS one 9 (2), e88546. doi:10.1371/journal.pone.0088546
Vink, A., Schoneveld, A. H., Lamers, D., Houben, A. J. S., van der Groep, P., van Diest, P. J., et al. (2007). van der Groep, Petra; van Diest, Paul J; Pasterkamp, GerardHIF-1 alpha expression is associated with an atheromatous inflammatory plaque phenotype and upregulated in activated macrophages. Atherosclerosis 195 (2), e69–e75. doi:10.1016/j.atherosclerosis.2007.05.026
Vogel, R., Jansen, C., Roffeis, J., Reddanna, P., Forsell, P., Claesson, H-E., et al. (2010). Applicability of the triad concept for the positional specificity of mammalian lipoxygenases. J. Biol. Chem. 285 (8), 5369–5376. doi:10.1074/jbc.M109.057802
Wang, D., Chen, S., Feng, Y., Yang, Q., Campbell, B. H., Tang, X., et al. (2006). Reduced expression of 15-lipoxygenase 2 in human head and neck carcinomas. Tumour Biol. 27 (5), 261–273. doi:10.1159/000094761
Wang, J., Shidfar, A., Ivancic, D., Ranjan, M., Liu, L., Choi, M. R., et al. (2017). Overexpression of lipid metabolism genes and PBX1 in the contralateral breasts of women with estrogen receptor-negative breast cancer. Int. J. Cancer 140 (11), 2484–2497. doi:10.1002/ijc.30680
Wang, L. H., Yang, X. Y., Zhang, X., and Farrar, W. L. (2007). Inhibition of adhesive interaction between multiple myeloma and bone marrow stromal cells by PPARgamma cross talk with NF-kappaB and C/EBP. Blood 110 (13), 4373–4384. doi:10.1182/blood-2006-07-038026
Wang, Y., Zhu, D., An, Y., Sun, J., Cai, L., and Zheng, J. (2012). Preeclampsia activates 15-lipoxygenase and its metabolite 15-hydroxyeicosatetraenoic acid enhances constriction in umbilical arteries. Prostagl. Leukot. Essent. Fat. Acids 86 (1-2), 79–84. doi:10.1016/j.plefa.2011.10.006
Wecksler, A. T., Kenyon, V., Deschamps, J. D., and Holman, T. R. (2008). Substrate specificity changes for human reticulocyte and epithelial 15-lipoxygenases reveal allosteric product regulation. Biochemistry 47 (28), 7364–7375. doi:10.1021/bi800550n
Wecksler, A. T., Kenyon, V., Garcia, N. K., Deschamps, J. D., van der Donk, , Wilfred, A., et al. (2009). Kinetic and structural investigations of the allosteric site in human epithelial 15-lipoxygenase-2. Biochemistry 48 (36), 8721–8730. doi:10.1021/bi9009242
Weigert, A., Strack, E., Snodgrass, R. G., and Brüne, B. (2018). mPGES-1 and ALOX5/-15 in tumor-associated macrophages. Cancer Metastasis Rev. 37 (2-3), 317–334. doi:10.1007/s10555-018-9731-3
Wen, A. Y., Sakamoto, K. M., and Miller, L. S. (2010). The role of the transcription factor CREB in immune function. J. Immunol. 185 (11), 6413–6419. doi:10.4049/jimmunol.1001829
Werner, M., Jordan, P. M., Romp, E., Czapka, A., Rao, Z., Kretzer, C., et al. (2019). Targeting biosynthetic networks of the proinflammatory and proresolving lipid metabolome. FASEB J. 33 (5), 6140–6153. doi:10.1096/fj.201802509R
Wiesner, R., Suzuki, H., Walther, M., Yamamoto, S., and Kühn, H. (2003). Suicidal inactivation of the rabbit 15-lipoxygenase by 15S-HpETE is paralleled by covalent modification of active site peptides. Free Radic. Biol. Med. 34 (3), 304–315. doi:10.1016/S0891-5849(02)01244-3
Wójcik, P., Biernacki, M., Wroński, A., Łuczaj, W., Waeg, G., Žarković, N., et al. (2019). Altered lipid metabolism in blood mononuclear cells of psoriatic patients indicates differential changes in psoriasis vulgaris and psoriatic arthritis. Int. J. Mol. Sci. 20 (17), E4249. doi:10.3390/ijms20174249
Wu, M. Y., Lin, T. H., Chiu, Y. C., Liou, H. C., Yang, R. S., and Fu, W. M. (2012). Involvement of 15-lipoxygenase in the inflammatory arthritis. J. Cell. Biochem. 113 (7), 2279–2289. doi:10.1002/jcb.24098
Wu, Z. H., Tang, Y., Yu, H., and Li, H. D. (2021). The role of ferroptosis in breast cancer patients: A comprehensive analysis. Cell. Death Discov. 7 (1), 93. doi:10.1038/s41420-021-00473-5
Wuest, S. J. A., Crucet, M., Gemperle, C., Loretz, C., and Hersberger, M. (2012). Expression and regulation of 12/15-lipoxygenases in human primary macrophages. Atherosclerosis 225 (1), 121–127. doi:10.1016/j.atherosclerosis.2012.07.022
Wuest, S. J. A., Horn, T., Marti-Jaun, J., Kühn, H., and Hersberger, M. (2014). Association of polymorphisms in the ALOX15B gene with coronary artery disease. Clin. Biochem. 47 (6), 349–355. doi:10.1016/j.clinbiochem.2013.12.013
Xu, X., Li, J., Zhang, Y., and Zhang, L. (2021). Arachidonic acid 15-lipoxygenase: Effects of its expression, metabolites, and genetic and epigenetic variations on airway inflammation. Allergy Asthma Immunol. Res. 13 (5), 684–696. doi:10.4168/aair.2021.13.5.684
Xu, X. C., Shappell, S. B., Liang, Z., Song, S., Menter, D., Subbarayan, V., et al. (2003). Reduced 15S-lipoxygenase-2 expression in esophageal cancer specimens and cells and upregulation in vitro by the cyclooxygenase-2 inhibitor, NS398. Neoplasia 5 (2), 121–127. doi:10.1016/s1476-5586(03)80003-9
Yang, H. Z., Wang, J. P., Mi, S., Liu, H. Z., Cui, B., Yan, H. M., et al. (2012). TLR4 activity is required in the resolution of pulmonary inflammation and fibrosis after acute and chronic lung injury. Am. J. Pathol. 180 (1), 275–292. doi:10.1016/j.ajpath.2011.09.019
Yang, L., Ma, C., Zhang, L., Zhang, M., Li, F., Zhang, C., et al. (2018). 15-Lipoxygenase-2/15(S)-hydroxyeicosatetraenoic acid regulates cell proliferation and metastasis via the STAT3 pathway in lung adenocarcinoma. Prostagl. Other Lipid Mediat. 138, 31–40. doi:10.1016/j.prostaglandins.2018.07.003
Yang, Q., Feng, Y., Schultz, C. J., Li, X. A., Wu, H., and Wang, D. (2008). Synergistic effect of 15-lipoxygenase 2 and radiation in killing head-and-neck cancer. Cancer Gene Ther. 15 (5), 323–330. doi:10.1038/cgt.2008.9
Yang, S., Gong, Z., Liu, Z., Wei, M., Xue, L., Vlantis, A. C., et al. (2021). Differential effects of estrogen receptor alpha and beta on endogenous ligands of peroxisome proliferator-activated receptor gamma in papillary thyroid cancer. Front. Endocrinol. 12, 708248. doi:10.3389/fendo.2021.708248
Yoo, H., Jeon, B., Jeon, M. S., Lee, H., and Kim, T. Y. (2008). Reciprocal regulation of 12- and 15-lipoxygenases by UV-irradiation in human keratinocytes. FEBS Lett. 582 (21-22), 3249–3253. doi:10.1016/j.febslet.2008.08.017
Yuan, D., Ran, Y., Liu, Q., Zhang, Y., Li, H., Li, P., et al. (2014). Enhancement of the HIF-1α/15-LO/15-HETE axis promotes hypoxia-induced endothelial proliferation in preeclamptic pregnancy. PloS one 9 (5), e96510. doi:10.1371/journal.pone.0096510
Yuan, H., Li, M. Y., Ma, L. T., Hsin, M. K. Y., Mok, T. S. K., Underwood, M. J., et al. (2010). 15-Lipoxygenases and its metabolites 15(S)-HETE and 13(S)-HODE in the development of non-small cell lung cancer. Thorax 65 (4), 321–326. doi:10.1136/thx.2009.122747
Yuan, Y., Chen, J., Wang, J., Xu, M., Zhang, Y., Sun, P., et al. (2020). Development and clinical validation of a novel 4-gene prognostic signature predicting survival in colorectal cancer. Front. Oncol. 10, 595. doi:10.3389/fonc.2020.00595
Zhang, F., Sun, K., and Wang, W. S. (2022). Identification of a feed-forward loop between 15(S)-HETE and PGE2 in human amnion at parturition. J. lipid Res., 100294. In Press, Journal Pre-proof. doi:10.1016/j.jlr.2022.100294
Zhang, M., Xin, W., Ma, C., Zhang, H., Mao, M., Liu, Y., et al. (2018). Exosomal 15-LO2 mediates hypoxia-induced pulmonary artery hypertension in vivo and in vitro. Cell. Death Dis. 9 (10), 1022. doi:10.1038/s41419-018-1073-0
Zheng, Y., Yin, H., Boeglin, W. E., Elias, P. M., Crumrine, D., Beier, D. R., et al. (2011). Lipoxygenases mediate the effect of essential fatty acid in skin barrier formation: A proposed role in releasing omega-hydroxyceramide for construction of the corneocyte lipid envelope. J. Biol. Chem. 286 (27), 24046–24056. doi:10.1074/jbc.M111.251496
Zijlstra, F. J., van Dijk, A. P., Garrelds, I. M., Ouwendijk, R. J., and Wilson, J. H. (1992). Species differences in the pattern of eicosanoids produced by inflamed and non-inflamed tissue. Agents Actions. Spec No, C73-5. doi:10.1007/bf01996099
Glossary
AA arachidonic acid
ALOX arachidonate lipoxygenase
Alox8 arachidonate 8-lipoxygenase (murine)
AR androgen receptor
BrdU bromodeoxyuridine
CCL C-C motif chemokine ligand
CFTR CF transmembrane conductance regulator
COX cyclooxygenase
CRE(B) cAMP response element (binding protein)
DHA docosahexaenoic acid
DHT dihydroxytestosterone
diHpETE dihydroperoxyeicosatetraenoic acid
EPA eicosapentaenoic acid
ER estrogen receptor
ERK1/2 mitogen-activated protein kinase 3/1
GLA gamma-linolenic acid
GR glucocorticoid receptor
HDHA hydroxydocosahexaenoic acid
HETE hydroxyeicosatetraenoic acid
HIF1α hypoxia inducible factor 1 subunit alpha
HODE hydroxyoctadecadienoic acid
HpETE hydroperoxyeicosatetraenoic acid
HpODE hydroperoxyoctadecadienoic acid
IL interleukin
K1 keratin 1
LA linoleic acid
LOX lipoxygenase
LPS lipopolysaccharide
LT leukotrienes
LX lipoxin
MAPK mitogen-activated protein kinase
MMP matrix metalloproteinase
NDGA nordihydroguaiaretic acid
NFκB nuclear factor kappa B
NHP normal human prostate cells
PAEC pulmonary artery endothelial cells
PLAT polycystin-1-lipoxygenase α-toxin
PPAR peroxisome proliferator activated receptor
PUFA polyenoic fatty acid
Rv resolvin
SPM specialized pro-resolving lipid mediator
SREBP2 sterol regulatory element binding protein 2
STAT signal transducer and activator of transcription
TAM tumor associated macrophage
UV ultraviolet
(ox)LDL (oxidized) low-density lipoprotein
Keywords: ALOX15B, lipoxygenase, PUFA, 15-HETE, lipid mediator, cancer, immunity, inflammation
Citation: Benatzy Y, Palmer MA and Brüne B (2022) Arachidonate 15-lipoxygenase type B: Regulation, function, and its role in pathophysiology. Front. Pharmacol. 13:1042420. doi: 10.3389/fphar.2022.1042420
Received: 12 September 2022; Accepted: 26 October 2022;
Published: 09 November 2022.
Edited by:
Meike Julia Saul, Darmstadt University of Technology, GermanyReviewed by:
Antonio Recchiuti, University of Studies G. d’Annunzio Chieti and Pescara, ItalyUlrike Garscha, University of Greifswald, Germany
Copyright © 2022 Benatzy, Palmer and Brüne. This is an open-access article distributed under the terms of the Creative Commons Attribution License (CC BY). The use, distribution or reproduction in other forums is permitted, provided the original author(s) and the copyright owner(s) are credited and that the original publication in this journal is cited, in accordance with accepted academic practice. No use, distribution or reproduction is permitted which does not comply with these terms.
*Correspondence: Megan A. Palmer, cGFsbWVyQGJpb2NoZW0udW5pLWZyYW5rZnVydC5kZQ==
†These authors share senior authorship