- 1College of Pharmacy, Changchun University of Chinese Medicine, Changchun, China
- 2The First Hospital of Jilin University, Changchun, China
Cancer is one of the most difficult diseases to be treated in the world. Immunotherapy has made great strides in cancer treatment in recent years, and several tumor immunotherapy drugs have been approved by the U.S. Food and Drug Administration. Currently, immunotherapy faces many challenges, such as lacking specificity, cytotoxicity, drug resistance, etc. Nanoparticles have the characteristics of small particle size and stable surface function, playing a miraculous effect in anti-tumor treatment. Nanocarriers such as polymeric micelles, liposomes, nanoemulsions, dendrimers, and inorganic nanoparticles have been widely used to overcome deficits in cancer treatments including toxicity, insufficient specificity, and low bioavailability. Although nanomedicine research is extensive, only a few nanomedicines are approved to be used. Either Bottlenecks or solutions of nanomedicine in immunotherapy need to be further explored to cope with challenges. In this review, a brief overview of several types of cancer immunotherapy approaches and their advantages and disadvantages will be provided. Then, the types of nanomedicines, drug delivery strategies, and the progress of applications are introduced. Finally, the application and prospect of nanomedicines in immunotherapy and Chimeric antigen receptor T-cell therapy (CAR-T) are highlighted and summarized to address the problems of immunotherapy the overall goal of this article is to provide insights into the potential use of nanomedicines and to improve the efficacy and safety of immunotherapy.
1 Introduction
Cancer has become the second fatal disease in the world after cardiovascular diseases, and the number of patients is increasing year by year (Mullard 2020). Current tumor treatments methods mainly include surgery, radiotherapy, chemotherapy, and targeted drug therapy. Conventional surgical resection is difficult to remove the tumor cells completely, so it is easily leading to a postoperative recurrence. Radiotherapy and chemotherapy can kill tumor cells as well as normal cells, causing more harm to patients. Although targeted drug therapy can reduce the side effects of drugs, it is prone to cause tumor resistance and tumor recurrence. With the development of tumor biology, immunotherapy has emerged as a powerful clinical strategy for cancer. Different from conventional treatments, tumor immunotherapy targets mainly immune cells by activating the body’s immune system, achieve the elimination of tumor cells. It has the advantages of good effects, low adverse, and prevention of recurrence (Tan et al., 2020; Zhang and Zhang 2020). Over the past decade, there have been significant developments in the treatment of cancer. The introduction of immunotherapies such as immune checkpoint inhibitors, cellular immunotherapy, antibody therapy, and cytokine therapy help, patients tackle malignancies by boosting immune systems. Unfortunately, as immunotherapy continues to evolve, its side effects have emerged, including off-target effects, whose reason can be the complexity of treatment process, and the viability loss of T cells (Singh and McGuirk 2020; Sterner and Sterner 2021). Although some can be reversed by the use of immunomodulators (such as corticosteroids), high morbidity and mortality still occurs due to other reasons (Kiesgen et al., 2021). Many patients present other symptoms, such as pseudoprogression, hyperprogression, isolated responses, and drug resistance. Despite the rate of pseudoprogression and hyperprogression are not high, related reports are increasing, while immunotherapy is widely used.
Nano drug is a new way cancer immunotherapy with a safer and more controlled manner (Martin et al., 2020), allowing the expansion of therapeutic agents to more patients and reducing toxicity. In particular, nanotechnology can accumulate the efforts of immunotherapy in diseased tissues, desirable tumor and/or immune cells effectively and reduce the negative effects of off-targeting (Qiu et al., 2017). Moreover, the feature of nanomaterials such as temperature-sensitive properties and pH sensitivity can be used to improve therapeutic tolerance while enhancing targeting (Li et al., 2011; Cifuentes-Rius et al., 2021). By optimizing the morphological characteristics and surface properties (Xu et al., 2012), nano drug can have a prolonged half-life in the reticuloendothelial system circulation (Li et al., 2012). Therefore, nanomedicines in immunotherapy are considered as promising strategies for treating and even curing certain types of cancer. Researchers are currently investigate the development of novel nanomedicines for immunotherapy, including nanoparticles, nanocouples, and biomaterials (Shields et al., 2020; Brouillard et al., 2021).
Among cellular immunotherapies chimeric antigen receptor T-cell therapy (CAR-T) has become the focus of clinical immuno-oncology because of its MHC-independence, broad-spectrum replicability and immune memory function (Kiesgen et al., 2021). This therapy targets refractory or relapses B-cell precursor acute lymphoblastic leukemia in a target group of patients mainly under 25 years (Dai et al., 2020; Lu et al., 2020). There are various treatments for B-cell lymphoma, including chemotherapy, small molecule inhibitors of the B-cell receptor pathway, antibody-drug couples, immune checkpoint inhibitors, and RNA interference. Different from these approaches, CAR-T therapies isolate T-cells from a patient’s blood with genetical engineering techniques to express a CAR bindings to B-cell CD19. After in vitro amplification, cells are injected back into the patient, and then continue to proliferate and find target cells in vivo (Wei et al., 2020). To simplify the CAR-T treatment approach, Stephan’s group has developed a low-cost technology based on gene delivery. Polymeric nanoparticles targeting T-cells were prepared by combining leukemia-specific 194-1BBz CAR plasmid DNA with poly-β amino esters. Nanoparticles were covered with anti-CD3e f (ab’) two fragment modified polyglutamic acid by electrostatic interactions. To achieve a rapid nuclear localization of gene drugs (Manchanda et al., 2021), the nanoparticles were further modified with peptides containing nuclear localization signals. In vitro, it was observed that the nanoparticles were rapidly taken up by T-cells within 2 h. After 30 h of incubation, about 4% of T-cells were detected as CAR+, which demonstrates a significant effect (Kapadia et al., 2015). In addition to the complexity of the therapeutic process, CAR-T therapies are also prone to loss of viability of their effector T cells rapidly due to the host’s immunosuppressive microenvironment. Certain cytokines can enhance the viability and function of effector T-cells, however, they can also cause serious side effects. Bai’s group (Bai et al., 2020) found that self-assembled multivalent nucleic acid aptamer nanoparticles with CAR-like properties could increase T-cell proliferation and reverse the inhibitory effect of exogenous B7.1 molecules on Interleukin-2 (IL-2) secretion in vitro. The restriction of melanoma B16 cells growth in mice could be achieved both in vitro and in vivo, demonstrating that T-cells activated by multivalent aptamer nanoparticles could achieve the same function of CAR-T, enhancing the safety of CAR-T cell immunotherapy. Therefore, some shortcomings of pure CAR-T therapy can be overcome with the usage of nanomedicines.
2 Immunotherapy
Tumor immunotherapy is a sort of cancer treatments by activating the immune system (Dai et al., 2020), which has become a new tool for tumor treatment. Compared with conventional treatments, tumor immunotherapy mainly targets immune cells and activate body’s immune system by inhibiting immune negative regulatory factors and enhancing the recognition ability of immune cells to the surface antigens of tumor cells, and achieve the elimination of tumor cells. Up till now, tumor immunotherapy has evolved into various forms, including immune checkpoint inhibitors, antibody therapy, cellular immunotherapy, cytokine therapy, and immune combination therapy (Kumar et al., 2021). Therefore, there are many marketed drugs of immunotherapy (Table 1).
2.1 Checkpoint inhibitor
Immune checkpoints regulate the immune syste, where stimulatory immune checkpoint molecules promote the activation of T-cells and activate the immune response suppress the immune response of the body and prevent the development of autoimmunity (Liu and Xu 2020). Up to now, as many as 70 immune checkpoint inhibitors are in phase III and IV clinical trials; seven immune checkpoint inhibitors have been approved by FDA; and more than ten immune checkpoints have been discovered (Franzin et al., 2020).
Immune checkpoint molecules include CTLA-4, PD-1, PD-L1, which are the most widely researched, and other potentially novel immune checkpoint molecules that has not been used in the clinic, such as lymphocyte activationgene3 (LAG-3), T-cell immunoglobulin and immunoreceptor tyrosine-based inhibitory motif (ITIM) structural domain proteins, T-cell immunoglobulin mucin 3 (TIM-3), and T-cell activation inhibitor immunoglobulin variable region structural domain ISTA) (Mehta et al., 2019; Maruhashi et al., 2020; Fathi et al., 2021; Zhao et al., 2021).
CTLA-4, a transmembrane protein encoded by the CTLA-4 gene, is expressed in activated CD4+ and CD8+ T cells and binds to the ligands CD80 (B7-1) and CD86 (B7-2). It is also a T-cell surface receptor that acts as an immunosuppressive molecule and can participate in immunosuppressive signaling (Wei et al., 2020). CTLA-4 aborts the activated T-cell receptor (TCR) and mediates the suppressive function of regulatory T-cells (Treg). In addition, CTLA-4 mediates the binding of CD80/CD86 by dendritic cells and induces the expression of the tryptophan-degrading enzyme indoleamine 2, 3⁃dioxygenase, leading to the suppression of TCR (Anderson et al., 2016). CTLA-4 antibody reduces the inhibition of Treg and activates the TCR by binding to CTLA-4. In 2011, the FDA approved the first antibody drug working on immune checkpoint CTLA-4, ipilimumab (Kapadia et al., 2015), which is mainly used in treating melanoma and the prolonging of patient survival can reach to 1–2 years (Baas et al., 2021). Due to its early launch, limited monotherapy effect, and more adverse reactions, its combination therapy with PD-1 antibody have some prospects to amplify the tumor-suppressive effec.
In addition to CTLA-4, PD-1 is another common immunosuppressive molecule on the surface of T-cells, which are expressed on the surface of various tumor cells (Bai et al., 2020; Yang et al., 2022). PD-1, with two ligands: Is a member of the CD28 PD-L1 (also known as CD274 or B7⁃H1) and PD-L2 (also known as CD273 or B7⁃DC). PD-L1 is expressed by tumor cells binds to PD-1, blocking T-cell activation and cytokine production. PD-1/PD-L1 antibodies block the pathway by binding to PD-1/PD-L1, restoring immune killing function and enabling tumor immune escape (Zhang and Zheng 2020). Tumor cells express PD-L1 inhibit T-cell activation by binding to PD-1 on the surface of T cells, and PD-1/PD-L1 inhibitors close these immune checkpoints, and enhancing T-cell activity and killing tumor cells (Masarwy et al., 2021). The first PD-1 inhibitor, pembrolizumab, was approved in 2014 for the treatment of melanoma versus lung cancer (Yang et al., 2022). Then in 2016, the first PD-L1 inhibitor, atezolizumab, was approved for the treatment of bladder cancer (Liu and Xu 2020). Currently, clinical studies in solid tumors have found a conclution that overall survival with PD-1 antibodies better than with PD-L1 antibody therapy. However, there was no significant difference in the incidence of overall adverse events and immunotherapy-related adverse events (irAE) between the two groups. Results of head-to-head clinical trials comparing PD-1 and PD-L1 antibodies are not yet available (Duan et al., 2020).
2.2 Cellular immunotherapy
Cellular immunotherapy, also known as pericyte therapy, is also a type of immunotherapy (Reschke et al., 2021). Cellular immunotherapy is used to kill pathogens, cancer cells, and mutated cells in blood and tissues by extracting the body’s immune cells, proliferating them in vitro, increasing the targeting destruction, and infusing them back into the patient (Mizukoshi and Kaneko 2019). With the new research, developed, breakthroughs, and understanding of successive cellular immunotherapies in the global medical community, cellular therapy is gradually pushed to transform a “non-mainstream therapy” to an “adjunct to standard therapy” (Wang and Cao 2020). Chimeric Antigen Receptor T-cell therapy (CAR-T), which extracts T-cells from a patient’s own immune system, is cultured and modified in vitro, and equipping these T-cells with special molecules that enable them to recognize and attack specific cancer cells. The modified T-cells are injected back into the patient’s body and to destroy the immune response of cancer cells (Golubovskaya and Wu 2016). They are not restricted by human leukocyte antigens (HLA) because the antigen recognition sites of CAR-T cells are composed of monoclonal antibodies that specifically recognize tumor surface antigens (Mei et al., 2021). Two products have been approved by the FDA for clinical use (Al Hadidi et al., 2022). Another kind of pericyte therapy is named Engineered T-cell receptor therapy (TCR-T) which genetically modified immune effector cells are directly as anticancer therapy (Levy and Gros 2022). TCR-T therapy is performed by inducing the expression of modified T-cell receptors (TCRs) on the surface of T-cells, redirecting their endogenous cell killing activity to cancer cells. Currently, the TCR-T therapeutic agent CVT-TCR-01, which targets an intracellular cancerous testicular antigen called NY-ESO-1, has made great clinical progress (Zhang and Wang 2019). Tumor-infiltrating lymphocyte (TIL) therapy is a therapy based on tumor-specific T-cells, using infiltrating lymphocytes isolated from resected tumor tissue, expanding and culturing in vitro, and then transfusing back to patients to exert anti-tumor effects. It is a novel anti-tumor effector cell with the advantages of high efficiency, specificity, and low side effects. TIL therapy has been used clinically to treat primary or secondary tumors in the skin, kidney, lung, head and neck, liver, and ovarian sites (Stanton and Disis 2016; El Bairi et al., 2021). Natural killer (NK) cells in natural killer cell therapy are the third group of lymphocytes same as T-cell Band cells (Mao et al., 2021). NK cells in innate immune system and T lymphocytes (cytotoxic T lymphocytes) in postnatal immune system are two main types of cells used to attack malignant tumors. NK cells play an important role in natural hosts to the immune response of viruses and tumors. NK cell therapy requires the isolation from cord blood stored in cord blood banks to be genetic engineered to introduce CARs recognizing the surface targets of cancer cells before transfusion back to patients (Rafei et al., 2021).
2.3 Cytokine therapy
Cytokine therapy, also known as non-specific immunotherapy, is one of the first tools used in tumor immunotherapy. It mainly works through cytokines (e.g., IL-2, INF), immune adjuvants, and short peptides to enhance the body’s immune response. Substances used to regulate immunity are often referred as immune system modulators. The initial cytokine drugs approved by the FDA were IFNα-2a (Roferon-A) and IFNα-2b (Intron-A). Subsequently, high doses of IL-2 (Proleukin) were approved for the clinical treatment of metastatic melanoma and renal cancer (Khan et al., 2021). In addition, the immune-adjuvant class is not antigenic per se but enhances the stimulation of the body’s immune system by antigen. Immune restorer-type modulators can restore the suppressed immune reaction into normal levels. It treats cancer through the overall upregulation of the body’s immune function from different aspects. Immunomodulators cannot identify immune cells from enemy cells, and may “accidentally” harm normal cells and lead to certain adverse effects. Moreover, there appears to be a dose response for IL-2, as it appears that the most effective dose is also associated with significant toxicity. The most important cause of toxicity is vascular leakage syndrome, which manifests as fluid loss into the interstitial space as a result of increased vascular permeability. Other effects include thrombocytopenia, elevated hepatic serum transaminases, hepatocyte necrosis, hypoalbuminemia, tissue and peripheral eosinophilia, and pre-renal azotemia (Mier et al., 1988). It was shown that only very high intravenous doses lead to adequate clinical responses, but administration leads to severe, poorly tolerated, reversible but often life-threatening multisystem toxicity that may cause life-threatening side effects in patients (Rosenberg et al., 1985). IL-2 therapy, although one of the oldest successes of cancer immunotherapy to date, is still not widely implemented due to the high toxicity associated with it. To present, one drug (Ceplene, histamine dichloride) has successfully entered regulatory and clinical thresholds as a drug to reduce IL-2 toxicity and enhance efficacy (Hornyak et al., 2005). Thus, a regulatory pathway has been established for the development of such a drug. Therefore, cytokine therapy is often used in combination with other therapies for treatment or with adjuvants in order to reduce the associated toxicity (Passalacqua et al., 2014).
2.4 Problems in immunotherapy
Tumor immunotherapy mainly relies on regulating or activating the host’s immune system to inhibit or kill tumors, which has the advantages of low toxicity and high efficiency. However, challenges in clinical tumor immunotherapy are still need to be solved. (Routy et al., 2018). First, the similarity between tumor cells and normal cells leads to difficulties for human immune system to distinguish them correctly (Sau et al., 2018). For example, CAR-T therapies can attack tumor cells and attack other normal cells as well, which is also known as “off-target effect” (Song et al., 2015). Secondly, solid tumors have an immunosuppressive microenvironment, preventing some injected immune cells or cytokines from reaching the tumor site successfully, and leading to effective in hematologic tumors in immunotherapy. However, the problem of attacking solid tumors cannot be solved (Jiang et al., 2017). With the development of materials science and nanotechnology, nanomedicines have the following advantages that are expected to provide new ideas for improving the effectiveness of immunotherapy: First, nanomedicines can enhance drug accumulation at the tumor site due to their unique high permeation and long retention (EPR) effect, thus therapeutic effect is improved (Li et al., 2011). Second, some nanomaterials have special properties, including temperature-sensitive properties (Li et al., 2011) and pH-sensitive properties Through nanotechnology, modified drugs can achieve dual targeting actively and passively, reduce drug concentration and improve therapeutic tolerance while enhancing targeting (Li et al., 2011; Zhu et al., 2017). Third, by optimizing the morphological characteristics and surface properties of nanomedicines (Xu et al., 2012), the half-life in the circulation of the reticuloendothelial system can be prolonged to prevent its rapid clearance by immune syst hematological malignancies em (Li et al., 2012). Therefore, the use of nanomedicines can overcome some shortcomings of immunotherapy and enhance the effect of tumor immunotherapy and provide more effective treatments for patients through further development of nano immunotherapy.
3 Nanomedicine
Nanomedicines, as an emerging technology, have great efficiency in tumors, cellular oxidative damage, and many other diseases because of features including small particle size and stable surface function (Golubovskaya and Wu 2016; Wang and Cao 2020). One of the most important applications of nanoparticles is anti-tumor, improving the effectiveness and persistence of immunotherapy. The main methods include enhancing tumor drug enrichment, reducing drug resistance in the form of modified drugs and increasing the half-life of the modified drugs (Figueiras et al., 2022).
3.1 Types and characteristics of nanomedicines
Nanomedicines include polymeric micelles, liposomes, nanoemulsions, dendritic macromolecules and inorganic nanoparticles. Their main characteristics are shown in Table 2. As the main type of nanomedicines, polymeric micelle, has advantages of core-shell structure, high drug-carrying capacity, targeting ability, biodegradability, and stability (Abdel-Hafez et al., 2018). However, polymeric micelles have some shortcomings, such as toxicity and instability in the blood (Wang et al., 2014). Therefore, polymeric micelles should be intervened before being used in such conditions. Intervening methods include polyethylene glycolization and chain length improvement (Mao et al., 2017). As carriers, liposomes has the advantages of high biosafety, strong activity, rapid release, high bioavailability, high efficiency, rapid detection speed and long-term preservation in a circulating environment (Cheng et al., 2018). However, liposomes have disadvantages such as difficult to overcome biological barriers, for example, the blood-brain barrier and the blood-tumor barrier (Satta et al., 2022). Nanoemulsions can improve encapsulation efficiency, improve stability, reduce adverse effects, improve safety and enhance solubility (Peer and Margalit 2004; Cheng et al., 2022). However, its limitations, contain higher costs and short period for storage (Lakshmanan et al., 2021). Dendritic macromolecular nanomaterial has features including reducing dose of the drug, increasing drug effeciency, modulating biodistribution, reducing side effects, improving delivery of targeted drug detecting rapidly by fluorescence test and improving loading capacity (Sabjan et al., 2020; Mohamad et al., 2021). However, it faces issues of equipment and theory shortage, and high expansion, etc (Delyanee et al., 2021). Therefore, at present, the development of dendritic macromolecules mainly focus on generalization and economization. With unique magnetic and optical properties, inorganic nanoparticles can control nano-structural properties for effective transport, overcome biological barriers at the cellular and tissue levels, and replenish the body with essential elements and substances (Bober et al., 2022). In addition, it has the advantages of mucosal penetration, skin penetration and systemic circulation.
Currently, researchers constructed d-α-tocopherol polyethylene glycol 1000 succinate (TPGS)-modified carboxymethyl chitosan rhodopsin (TCR) polymeric micelles (PMs) for the oral administration of paclitaxel (PTX) (Wang et al., 2022). The results showed that TCR PMs with a drug loading of 47.52 ± 1.65% can significantly improve the intestinal absorption and oral bioavailability of paclitaxel. Furthermore, nanomedicines exerted the effects of altering allergic phenotypes, prolonging half-life, overcoming biological barriers at cellular and tissue levels, improving antitumor effects and reducing side effects (Mao et al., 2017), through the development of nanoemulsion vaccines (O’Konek et al., 2020), dendritic macromolecular nanoparticles (NP-TPGS-SFB) (Li Z et al., 2020), inorganic nanoparticles (Xie et al., 2019), cisplatin (CDDP) and curcumin (CUR) co-loaded liposomes (CDDP and CUR-Lip) (Cheng et al., 2018) and novel bee toxin nanoliposomes.
Based on the properties of each material, it is known that polymeric micelles can be used for oncology treatments, drugs with high targeting requirements, etc.; nanoliposomes are recommended for transporting small polar drugs such as paclitaxel and artemisinin; nanoemulsions can be used for drugs with poor solubility and encapsulating capacity, like ophthalmic drugs, oral agents and injectables; dendritic macromolecules can be used when there is a need for large volumes and rapid detection; apheresis nanoparticles can be used when metal trace elements are shortening and biological barriers cannot be overcome.
3.2 Drug delivery strategies
Drug delivery of nanoparticles mainly contains three ways: cellular uptake, cytosolic delivery by endosome escape and direct intracellular delivery, as detailed in Figure 1A–C.
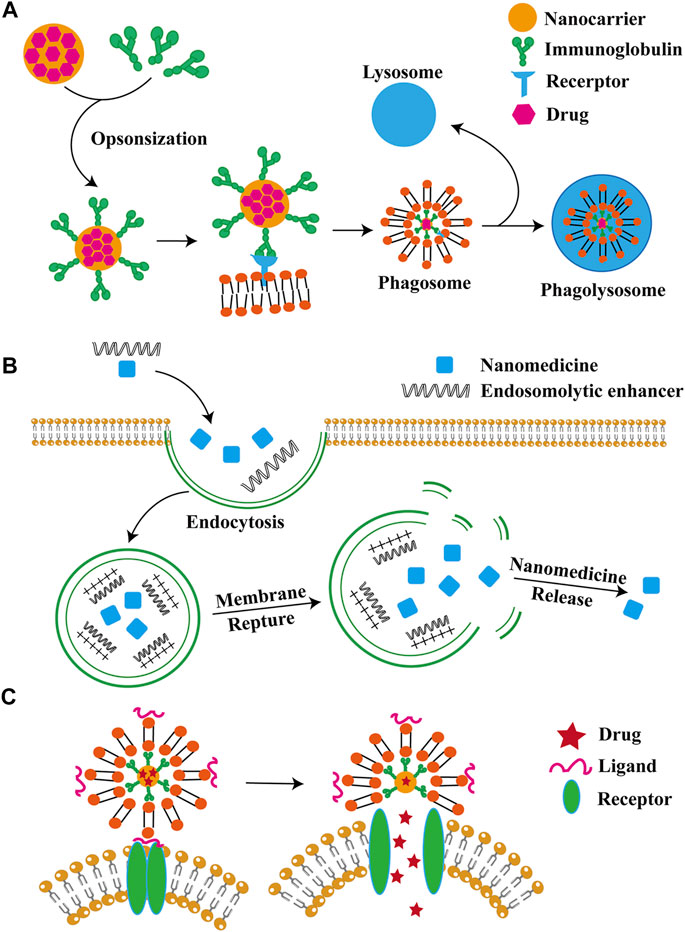
FIGURE 1. Major nanoparticle delivery strategies. Drug delivery of nanoparticles is mainly through cellular uptake, intracellular solute delivery and intracellular direct delivery, as shown in (A–C), respectively.
3.2.1 Cellular absorbing mechanisms
Nanoparticles (NPs) can enter into cells by endocytosis. In thisprocess, cell membrane engulfs NPs and forms a separate vesicle in the cell. The mechanisms of endocytosis include phagocytosis, latticin- and niche protein-mediated endocytosis, and latticin- and niche protein-independent pathways (Lee et al., 2021). Phagocytosis occurs in immune cells, such as dendritic cells, T cells, B cells, neutrophils and macrophages, which work as means of removal of foreign bodies (Gordon 2016).
3.2.2 Endosomal escape
Nanoparticles with enhanced endosomal escape ability are the best choice for bio-delivery. Commonly used nanoparticle that are commonly used for endocytosis and biological agents’ delivery includes liposomal and lipid-based NPs, polymer micelles and polymer NPs, lipid-polymer hybrid NPs, nanocrystals, and inorganic NPs such as gold NPs, magnetic NPs, graphene, quantum dots, and silicon NPs. The mechanism of intranuclear body escape involves instability of the intranuclear body membrane, which can be solved by increasing the interaction between the intranuclear body membrane and intranuclear solvents such as pH-sensitive solvation compounds or polymers (Wang et al., 2016). Currently, the main strategies for endosome escape include endosomes lysis with intranuclear enhancers; endosome membrane pore formation; effect of high pH buffers mediating the proton sponge; fusion agents interference of forming membrane fusion; and the application of photosensitizers causing photochemical destruction of endosome membranes (Lorenz et al., 2014).
3.2.3 Direct intracellular delivery
Various methods have been developed to enhance intranuclear body escape, including delivering cytoplasmic directly bypassing intranuclear body wrapping and delivering organisms to the cytoplasm directly. The solutions contain fossa-mediated pathways, contact release, and intermembrane transfer, membrane fusion, direct intracellular translocation, membrane rupture, and microinjection (Liu et al., 2014).
Although nanocarriers is developing rapidly, many challenges are still to be addressed. The intracellular drug delivery and efficacy of nanocarriers are mainly controlled by the mode of internalization and intracellular transport. Endocytosis of NPs is a major pathway, but intranuclear endosomal encapsulation leads to a lower possibility of cytoplasmic delivery. Materials for inducing intranuclear endosome escape can be limited by toxicity (Zhang et al., 2018). To solve this problem, choosing alternative drug delivery or reducing the potential toxicity of nanoparticles. Improving poor intracytoplasmic drug delivery can be achieved as well. Direct intracytoplasmic delivery is a promising intracellular delivery for biologics because its rate of delivering into cytoplasm can almost achieve 100%, However, more safety issues needed to be addressed (Schmid et al., 2017). Safe and scalable NPs is still a focus of translational biology.
3.3 Nanoparticles and coupling substances
Nanoparticles and coupling compounds are widely used in the following six areas.
3.3.1 Vascular receptor-mediated drug in immune cells
Immune cells play a crucial role in the immune response process. However, the continuous activation of the immune system may disrupt immune homeostasis and lead to inflammatory diseases. Targeted immunomodulators may be useful in the treatment of such diseases and their complications. However, the disadvantages of immunomodulators include high immune-mediated toxicity, low solubility, and) loss of biological activity after long-term circulation (Ko et al., 2022). At this stage, nanocarriers have become a very promising tool to overcome these barriers due to their unique properties such as continuous circulation, ideal biodistribution, and preferred pharmacokinetic and pharmacodynamic properties. It has become one of the irreplaceable materials in the delivery of immunomodulatory drugs and vaccine adjuvants.
3.3.2 Nanoparticles for mRNA cancer vaccines
Among the many cancer vaccines, mRNA is becoming more prevalent for the following three reasons (Miao et al., 2021). First, mRNA can encode multiple antigens simultaneously, or contain whole proteins with MHCI and MHCII binding epitopes, which can promote humoral and cellular adaptive immune responses and enhance anti-tumor immunity. Second, compared with DNA vaccines, mRNA vaccines has features of non-integrated highly degraded, and lack of insertional mutagenic potential. In contrast to protein or cell-mediated vaccines, it does not contain cellular and pathogenic viral components, meaning no potential for infection. Most mRNA vaccines tested in ongoing clinical trials are generally well tolerated and cases show a reactions at injected part. Finally, production of mRNA cancer vaccines is rapid and scalable. In addition, nanoparticles in mRNA vaccines has the following advantages: retaining activity of the encapsulated adjuvant and significantly improving the efficiency of mRNA transfection. It induces an effective adaptive immune response by increasing the expansion and the infiltration into the lesion in vivo of specific T cells (Islam et al., 2021). Effective delivery of the mRNA vaccine will be critical to success clinical application. Studies have shown that lipid nanoparticles can be synthesized easily in a scalable manner to protect mRNA from degradation, facilitate endosome release, modify the surface of ligands to achieve desired cell types targeting, and co-deliver adjuvants as needed (Miao et al., 2021).
3.3.3 Ionizable lipid nanoparticles for in vivo mRNA delivery
Gene therapy, including mRNA delivery in vivo, has great potential for treating almost any disease, lipid nanoparticles are increasingly well-known as an excellent lipophilic gene therapy delivery vehicle. The study used bounded mRNA lipid nanoparticles as a carrier for targeted delivery because of two inspirations: the principle of absorption of apolipoprotein E (ApoE) to the surface of lipid nanoparticles, and the principle of achieving specific hepatocyte targeting. Studies have shown that lipid nanoparticle particles have better stability, biodistribution, and targeting capacity, making this technique suitable to be developed, having the potential of an extrahepatic targeting to develop a novel gene therapy (Francia et al., 2020). Libraries of ionizable lipid nanoparticles are designed to encapsulate mRNA to prevent its degradation, and mediate intracellular delivery. However, these ionizable lipid nanoparticles are typically characterized and screened in vitro environments, which may not fully replicate the biological barriers encountered. To address this problem, Guimaraes et al. designed an in vivo platform for accelerating mRNA delivery screening. This platform includes an engineered LNP library with functions of encapsulating and custom-designing barcoded mRNA (b-mRNA). These b-mRNAs are structurally and functionally similar to conventional mRNAs and contain barcodes that allow them to be quantified by deep sequencing (Guimaraes et al., 2019). The platform enables direct barcoding and subsequent quantification of functional mRNA, which can accelerate the in vivo screening and designing of ionizable lipid nanoparticles for mRNA therapeutic applications, such as CRISPR/Cas9 gene editing, mRNA vaccination and other mRNA-based regenerative medicine and protein replacement therapies. Hald et al. suggested that lipid nanoparticles play an important role in the mRNA vaccine against COVID-19 (Hald Albertsen et al., 2022). A growing number of data confirms that the scope of lipid nanoparticle-based gene therapy and vaccines treatment will be determined by the kind of lipid components are added and combined when used as carriers.
3.3.4 Biologically inspired molecular coupled subunit vaccines
The development of effective vaccines is essential to prevent potential epidemics. However, there is a standard for rapid design and development of effective vaccines. Inspired by the natural budding process of invading a host cell to replicate, and fracturing cell membrane, a similar strategy was applied to achieve virus-mimicking nano vacuoles. This is achieved by molecularly coupled subunit vaccines, a strategy that loading genetically engineered viral antigens onto mammalian cell membranes to produce antigen-loaded vesicles, and then being optimized for size and stability by surfactants (Mi et al., 2016).
3.3.5 Matrix-binding checkpoint inhibitor couples
CD40 is an immune co-stimulatory receptor expressed by antigen-presenting cells. Agonistic anti-CD40 antibodies have shown significant antitumor effects but may also cause serious adverse events such as hepatotoxicity. Ishihara et al. designed a variant to enhance the effect of partial administration of anti-CD40 through extracellular matrix of a super affinity peptide derived from placental growth factor-2. It proves the significant therapeutic advantage of cancer immunotherapy, from engineering matrix-binding structural domains to agonistic anti-CD40 antibodies injections (Ishihara et al., 2018).
3.4 Current situation and problems
As an emerging drug delivery system, nanomaterials have advantages and disadvantages. At this stage, the current status and problems of common nanomaterials (targeted ligand nanomaterials, nanomedicine delivery systems, size and surface modification of nanomedicine formulations, etc.) are shown below (Table 3).
4 Application of nanomedicine in antitumor immunotherapy
4.1 Nanomedicines in immune checkpoint blockade (ICB) therapy
The development of immune antibodies has revolutionized cancer immunotherapy. Studies have demonstrated that nanomedicines can be targeted and retained at tumor sites with less adverse effects (Kulkarni et al., 2016). Nanomedicines derived from anti-CTLA-4 and anti-PD-L1 antibodies can inhibit tumor growth and reduce adverse effects (Kulkarni et al., 2016). Targeted delivery of ICB antibodies to residual tumor sites with platelet nanomedicines can inhibit tumor recurrence and treat tumor recurrence and treats metastasis with high clinical relevance (Lamla and Erdmann 2004; Lee et al., 2012). Wilson’s team deliver intracellularly interferon gene stimulator (STING) agonists by damaging polymers of endosomes, which is a natural form and difficult to cross the cell membrane. Treatment with these polymeric vesicles significantly improves the efficacy of anticancer immunity and checkpoint blocking therapies (Li et al., 2016).
4.2 Application of nanomedicines in modulate immunosuppressive cytokines modulation
Soluble cytokines is one of the essential method for tumor immunotherapy. The cytokine TGF-β can decrease the number and activity of NK cells and tumor-infiltrating T lymphocytes (CTL), also affect the number of regulatory T-cells. Irvine’s group investigated the immunosuppressive effects of small molecule inhibitor TGF-β by T-cell-targeted delivery a (SB525334) (Derynck, Turley et al.). Fahmy and others co-loaded the nanoparticles with TGF-β inhibitors and interleukin 2, attenuating immunosuppression and enhancing T-cell proliferation. Huang et al. (Park et al., 2012) developed a nano-formulation TGF-β siRNA that achieves approximately 50% knockdown of TGF-β expression in tumors and synergizes with cancer vaccination. For example, it shows more on cytotoxic T-cell infiltration and significantly inhibits tumor growth (Xu et al., 2014). In addition, liposomes that modified with ligands targeting T-cell internalized receptor (CD90) or non-internalized receptor (CD45), are tested their performance, revealing that both two liposome agents can induce high expression of CTL in tumors. Furthermore, lower tumor suppression was observed with anti-CD45 liposomes than with anti-CD90 liposomes. This observation was attributed to the fact that the receptor CD45 was normally expressed by nuclear hematopoietic cells (Simpson et al., 2015). It was also shown that peripheral blood B cells, dendritic cells and macrophages did not ideally internalize anti-CD45 liposomes (Lu et al., 2016).
5 Chimeric antigen receptor T-cell immunotherapy and applications
5.1 Chimeric antigen receptor T-cell immunotherapy
Since its introduction, chimeric antigen receptor T-cell immunotherapy (CAR-T) has shown greater clinical advantages and potential. CAR-T therapy is worked by collecting peripheral blood from patients and isolating lymphocytes, introducing CAR genes into T-cells through genetic engineering techniques to express specific receptors to bind specific tumor-associated antigens. After being a amplified in vitro, they are transfused back to patients specifically binding of CAR-T cells to tumor-associated antigens in vivo, activating T-cells through signal transduction, promoting T-cell proliferation and releasing cytokines to exert anti-tumor effects (Gauthier and Yakoub-Agha 2017). Typically, a CAR is a fusion protein consisting of a combination of an extracellular antibody variable region used to recognize a tumor antigen site and an intracellular signal transduction module from a T-cell. Single-chain antibody light and heavy chain variable regions scFv fragments in the extracellular are responsible for recognizing and binding to tumor antigen targets. Hinges in the transmembrane region provide flexibility in antibody recognition. CD28 and CD3ζ in the intracellular signal transduction region are responsible for delivering activation and proliferation signals to the cell (Kosti et al., 2018). After being activated, CAR-T cells secrete a large number of cytokines activating themselves as well as other immune cells rapidly and induce apoptosis of target cells (Figure 2).
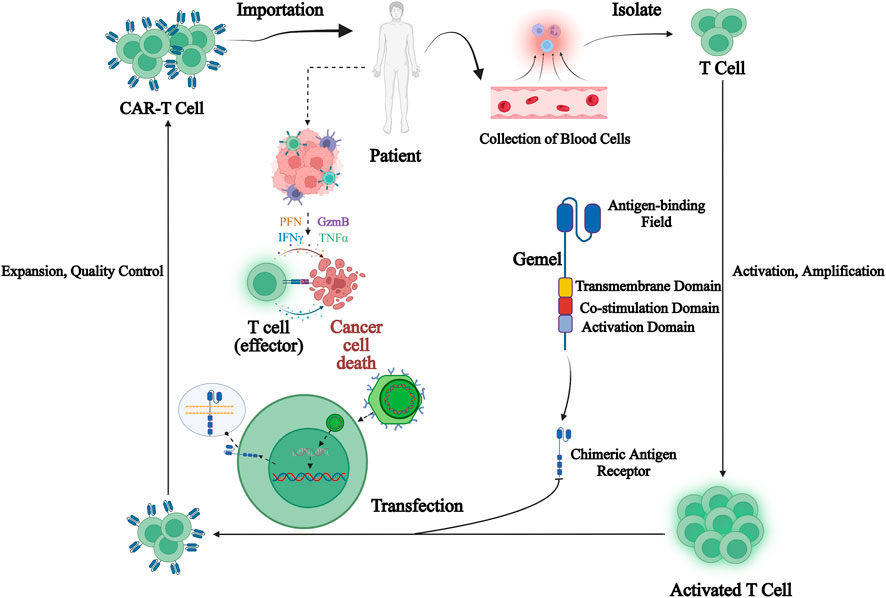
FIGURE 2. Chimeric antigen receptor T (CAR-T) cell immunotherapy process. CAR-T therapy is performed by collecting peripheral blood from patients and isolating lymphocytes from it, introducing CAR genes into T-cells through genetic engineering techniques to express specific receptors to bind specific tumor-associated antigens, amplifying them in vitro and then transfusing them back to patients, and after specific binding of CAR-T-cells to tumor-associated antigens in vivo, activating T-cells through signal transduction, promoting T-cell proliferation and releasing cytokines to exert anti-tumor effects.
CARs are classified into five generations based on intracellular structural domains: The first generation has a single activating structural domain, mainly CD3ζ, and some research studied on the gamma chain of the Fc receptor. The second generation added a co-stimulatory structural domain obtaining from co-stimulatory molecules such as 4-1BB or CD28 attaching to activate structural domain (CD3ζ/γ chain of the Fc receptor) to enhance the cell proliferation and cytotoxic capacity of CAR-T cells (Acuto and Michel 2003). The third generation is similar to the second generation but has multiple structural domains co-stimulated with CD3ζ, such as 4-1BB and CD28, CD134 and CD137 (Fang et al., 2014). The fourth-generation CARs, known as T-cells retargeted cytokine-mediated killings (TRUCKs), were designed to release the transgenic cytokine IL-12 during CAR signaling in tumor tissue to overcome TME immunosuppression and achieve robust therapeutic outcomes (Li W et al., 2020). IL-12 is responsible for the induction of IFN-γ, perforin and granzyme in T-cells and inhibits Treg proliferation (Yu and De Geest 2020). Other cytokines mentioned in the fourth generation are IL-15 and IL-18 (Xue et al., 2019). IL-15is γ-chain segment and it has important properties of expansion and survival for T-cells (Cao et al., 2009). In addition, IL-18 CAR T-cell treatment of large pancreatic and lung tumors showed changes in the immune cell landscape; significant increases in macrophages (CD 206 M1) and NKs (NKG2D+) were observed Apart from this, a decrease in Tregs such as M2 macrophage-suppressive CD 103 + DCs, indicates the ability of “IL-18 TRUCKs” to sensitize large tumor lesions for effective immune destruction (Hurton et al., 2016). Fifth-generation CAR-T therapies, based on the second-generation are being explored. It contains a truncated cytoplasmic receptor (IL-12), a β-chain structural domain (IL-2Rβ truncated intracellular interleukin two β-chain receptor) as well as the transcription factor STAT3/5 binding motif (Klebanoff et al., 2004) (Figure 3).
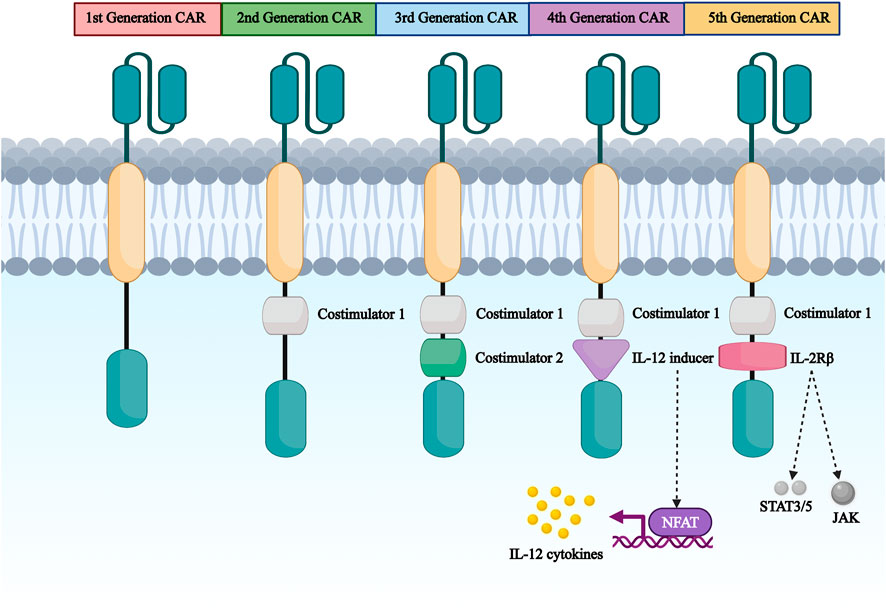
FIGURE 3. The first generation to the fifth generation CAR structure diagram. CAR are divided into five generations according to their intracellular domains. The first generation has a single activating structural domain, mainly the cytoplasmic structural domain of CD3ζ.The second generation has CD3ζ plus a co-stimulatory structural domain obtained from co-stimulatory molecules such as 4-1BB or CD28 attached to the activating structural domain. The third to fifth generation are based on the first or second generation of design.
5.2 Application of nanomedicines in CAR-T therapy
Traditional cancer treatments often cause damage to normal cells in the body, or residual malignant tumor cells flow into the bloodstream through the wounded area, making poor prognosis. Moreover, single immunotherapy modalities have limitations in their efficacy when treating solid tumors. Based on this, the use of combined nanoparticles for cancer immunotherapy can achieve synergistic effects. The current limitations of CAR-T immunotherapy in clinical tumor immunotherapy include the following: stimulating T-cell expansion and persistence; improving T-cell trafficking; stimulating intrinsic T-cell activity; resetting immunosuppressive cells and vascular microenvironment; methods to monitor the therapeutic efficacy of CAR-T cell therapies, etc.
5.2.1 Nanomedicines stimulates T-cell expansion and persistence
The complex preparation procedures and severe toxicities are two major obstacles facing the wide use of chimeric antigen receptor-modified T (CAR-T) cells in clinical cancer immunotherapy. The nanotechnology-based T-cell temporary CAR modification may be a potential approach to solve these problems and make the CAR-T cell-based tumor therapy feasible and broadly applicable. To solve this problem, Judith Guasch (Guasch et al., 2018) propose to activate primary human T-cells using a combination of nanostructured surfaces functionalized with the stimulating anti-CD3 antibody and the peptidic sequence arginine-glycine-aspartic acid, as well as costimulatory agents (anti-CD28 antibody and a cocktail of phorbol 12-myristate 13-acetate, ionomycin, and protein transport inhibitors). This method that combines nanotechnology with cell biology procedures to efficiently produce T-cells in the laboratory, challenging the current state-of-the-art expansion methodologies. Qianru Yu’s study (Yu et al., 2020) showed that a series of plasmid DNA-loaded self-assembled nanoparticles (pDNA@SNPsx/y) prepared from adamantane-grafted polyamidoamine (Ad-PAMAM) dendrimers of different generations (G1 or G5) and cyclodextrin-grafted branched polyethylenimine (CD-PEI) of different molecular weights (800, 2000, or 25,000 Da) were characterized and evaluated. The detailed physicochemical properties, cellular interaction, and cytotoxicity of selected pDNA@SNPG1/800 were systematically investigated. Finally, the response of EGFRvIII CAR-positive Jurkat T-cell to target tumor cell was evaluated. The results indicated that pEGFRvIII-CAR@SNPG1/800 can effectively achieve T-cell transient CAR modification, thereby demonstrating considerable potential in CAR-T cancer therapy. Subsequently, scientists have developed hydrogels (Guasch et al., 2017, Pérez Del Río et al., 2020), 3D hydrogels (Santos et al., 2022), nanocapsules (Thiramanas et al., 2020), nanoparticles (Yu, Zhang et al., 2020), and other nanoformulations to reduce cytotoxicity and achieve the goal of stimulating T-cell expansion and persistence.
5.2.2 Nanomedicines enhance the effectiveness of CAR-T cells
Chimeric antigen receptor (CAR)-modified T-cell therapy has the potential to improve the overall survival of patients with malignancies by enhancing the effectiveness of CAR T-cells. Precisely predicting the effectiveness of various CAR T-cells represents one of today’s key unsolved problems in immunotherapy. Qin Liu (Liu et al., 2020) encapsulated interferon-α2b (IFN-α2b) into microporous hydrogels as an enhancement factor and utilized low-dose irradiation (LDI) as a tumoral attractor of T-cells. The results show that hydrogels kept the activity of IFN-α2b and stably release of IFN-α2b to stimulate T-cells for a long time. At the same time, low-dose radiation recruits T-cells into tumors. This innovative integration mode of IFN-α2b-loaded hydrogels and radiotherapy offers a potent strategy to improve the therapeutic outcome of T-cell therapy. Xiong W and colleagues (Xiong et al., 2018) predict the effectiveness of CAR-modified cells by evaluating the quality of the CAR-mediated immunological synapse (IS) by quantitation of F-actin, clustering of tumor antigen, polarization of lytic granules (LGs), and distribution of key signaling molecules within the IS. Long-term killing capability, but not secretion of conventional cytokines or standard 4-h cytotoxicity, correlates positively with the quality of the IS in two different CAR T-cells that share identical antigen specificity. Xenograft model data confirm that the quality of the IS in vitro correlates positively with performance of CAR-modified immune cells in vivo. Therefore, they propose that the quality of the IS predicts the effectiveness of CAR-modified immune cells, which provides a novel strategy to guide CAR therapy. Yingmei Luo et al. (Luo et al., 2022) develop IL-12 nanostimulant-engineered CAR T-cell (INS-CAR T) biohybrids for boosting antitumor immunity of CAR T cells via immunofeedback. IL-12 is responsively released from INS-CAR T biohybrids in presence of the increased thiol groups on cell-surface triggered by tumor antigens. In return, released IL-12 obviously promotes the secretion of CCL5, CCL2, and CXCL10, which further selectively recruits and expands CD8+ CAR T-cells in tumors. Ultimately, the immune-enhancing effects of IL-12 nanochaperone significantly boost CAR T-cell antitumor capabilities, dramatically eliminated solid tumor and minimized unwanted side effects.
5.2.3 Nanomedicines reduce immunosuppression in the tumor microenvironment
The efficacy of Adoptive Cell Therapy (ACT) for solid tumor is still mediocre. This is mainly because tumor cells can hijack ACT T-cells’ immune checkpoint pathways to exert immunosuppression in the tumor microenvironment. Immune Checkpoint Inhibitors such as anti-PD-1 (aPD1) can counter the immunosuppression, but the synergizing effects of aPD1 to ACT was still not satisfactory. (Chen et al., 2022) demonstrate an approach to safely anchor aPD1-formed nanogels onto T-cell surface via bio-orthogonal click chemistry before adoptive transfer. The spatial-temporal co-existence of aPD1 with ACT T-cells and the responsive drug release significantly improved the treatment outcome of ACT in murine solid tumor model. The average tumor weight of the group treated by cell-surface anchored aPD1 was only 18% of the group treated by equivalent dose of free aPD1 and T-cells. The result of this research indicates that technology can be broadly applicable in ACTs employing natural or Chimeric Antigen Receptor (CAR) T-cells. In addition, the exploitation of embedded protein-targeted nanoliposomes (Xie et al., 2021) and γδ-T cell therapies (Man et al., 2019) would be beneficial to reduce immunosuppression of the tumor microenvironment, non-invasive cell tracking approaches and increased targeting to tumor sites.
5.2.4 Nanomedicines improve T-cell transport
Laura Wayteck et al. (Wayteck et al., 2016) conclude that many barriers need to be overcome in route to the tumor by developing a nanoparticle that can couple to the surface of CD8 (+) T-cells. Verified that liposomes reversibly attach to the surface of cytotoxic T lymphocytes via reduction-sensitive coupling. The activation state of the T-cell and liposome composition was shown to strongly influence the up-sampling efficiency. Loading cells with liposomes did not impair the activation status of the T-cells and the liposome composition are shown to strongly influence the loading efficiency. Loading the cells with liposomes does not compromise T-cell functionalities like proliferation and cytolytic function.
5.2.5 Stimulation of intrinsic T-cell activity
Karlo Perica’s team (Perica et al., 2014) manufactured Artificial antigen presenting cells (aAPC) based on two types of nanoscale particle platforms: biocompatible iron-dextran paramagnetic particles (50–100 nm in diameter) and avidin-coated quantum dot nanocrystals (∼30 nm). Nanoscale aAPC induced antigen-specific T-cell proliferation from mouse splenocytes and human peripheral blood T-cells. When injected in vivo, both iron-dextran particles and quantum dot nanocrystals enhanced tumor rejection in a subcutaneous mouse melanoma model. This is the first description of nanoscale aAPC that induce antigen-specific T-cell proliferation in vitro and lead to effective T-cell stimulation and inhibition of tumor growth in vivo. This team of investigators have manufactured two types of nanoscale particle platform-based aAPCs and demonstrates that both iron-dextran particles and quantum dot nanocrystals enhance tumor rejection in a melanoma model, providing the first description of nanoscale aAPCs that lead to effective T-cell stimulation and inhibition of tumor growth.
5.2.6 Ways to monitor the therapeutic effectiveness of CAR-T cell therapy
Adoptive cell transfer of targeted chimeric antigen receptor (CAR) T-cells has emerged as a highly promising cancer therapy. The pharmacodynamic action or CAR T-cells is closely related to their pharmacokinetic profile; because of this as well as the risk of non-specific action, it is important to monitor their biodistribution and fate following infusion. Stefan Harmsen (Harmsen et al., 2021) developed a dual-modal PET/near infrared fluorescent (NIRF) nanoparticle-based imaging agent for non-genomic labeling of human CAR T cells. Since the PET/NIRF nanoparticles did not affect cell viability or cytotoxic functionality and enabled long-term whole-body CAR T-cell tracking using PET and NIRF in an ovarian peritoneal carcinomatosis model, this platform is a viable imaging technology to be applied in other cancer models.
Combination with nanotechnology, CAR-T cells can construct a lentiviral expression vector and complete the culture of a complete CAR-T cell expansion system. Clinical studies (Hockemeyer et al., 2011) have shown that combining nanotechnology with CAR-T can provide better remission and treatment for highly recurrent pediatric acute lymphoblastic leukemia. Meanwhile, patients with poor prognosis B-cell malignancies can be effectively treated by CAR-T cell redirected immunization (Ren et al., 2017). Due to the frequency of chemotherapy resistance and relapse, Leukemia can lead to certain adverse effects, such as severe acute tissue necrosis and other symptoms (Eyquem et al., 2017). Combining with nanotechnology and CAR-T can precisely screen the best target antigens, significantly reduce CAR- T-cell resistance, and significantly improve the remission rate of leukemia patients. For treating acute lymphoblastic leukemia, abandoning traditional chemotherapy and opting for nanotechnology and CAR-T can fundamentally address the problem of overload tumor in drug-resistant patients. Therefore, nanotechnology combining with CAR-T has a broad application prospect in the treatment of malignant tumors.
6 Conclusion and outlook
In recent years, the rapid development of nanomedicine provides new insights to cancer immunotherapy. The most obvious advantage of nanoparticles is tunability. They can be designed in various sizes and shapes. Being modified with targeting molecules or loading various drugs shows the ability of targeted delivery and therapeutic delivery simultaneously. Nanomedicines can also be targeted for immunotherapy applications based on different needs because of different materials properties, such as high targeting requirements, transporting small polarities, poor solubility, not easily encapsulated, and unable to overcome biological barrier situations. Finally, compared to low molecular weight immunomodulators, nanoscale immunomodulators control pharmacokinetic behavior and have the potential to enhance immune activation through synergistic effects due to their unique size effect and co-loading capacity. Indeed, the presence of multiple functional domainsovercome barriers to effective immunotherapy in solid tumors. This paper introduces the characteristics and advantages of immunotherapy, nanomedicines and their current research status in immunotherapeutic approaches, focusing on reviewing the application and prospects of various types of nanoparticles in CAR-T therapy for tumor treatment, learning about the advantages of nanomedicines in immunotherapy and preparing for the development of more nanomedicines in CAR-T therapies for oncology.
Currently, CAR-T therapies mainly work in hematologic tumors and lymphomas. It has much less effect in solid tumors with toxicity. This variation is caused by various factors, For example, multiple cells and molecules with different immunosuppressive effects are appeared in solid tumors; the level of antigen expression in solid tumor cells varies between tumor types and different stages; and antigens in solid tumors are generally expressed in small amounts in other sites, such as heart, lung, and liver, accompanying by off-target effects. Therefore, nanoparticle immunotherapy can be used in combination therapies to address the limitations solid tumors treatments, including chemotherapy, radiotherapy, and photodynamic therapy. For example, compared to immunotherapy, radiotherapy combing with immunotherapy significantly improves the efficiency of patient treatment in clinical trials, which overturning the traditional view that radiotherapy kills immune cells and has an immunosuppressive effect. This suggests a synergistic effect of radiotherapy and immunotherapy (Ai et al., 2020).
Partial radiotherapy activates the immune system, inducing immune cells to attack tumor cells outside the radiotherapy area. It is rare for clinical radiotherapy but immunotherapy can enhance the immune-inducing effects of radiotherapy and increase the incidence of distant effects (Wang 2020). Although the synergistic effects of combination regimens have been confirmed through various phase III clinical studies, clinicians still have many questions about their application, especially some controversial issues needing more valid information. With a better understanding of cancer immunology and nanomedicine, efforts are still needed to optimize the properties of nanoparticles to assess their potential risks before translating them into clinical practice. More effective nano-types and nanoparticles will transform cancer immunotherapy in the near future.
Author contributions
LjW and HX these authors contributed equally to this work and should be considered co-first authors. LlW and JS supervised the research. CX and YJ designed and co-wrote the manuscript, and supervised the research.
Funding
This work was supported by the Science and Technology Project of Jilin Provincial Department of Science and Technology [YDZJ202201ZYTS636].
Conflict of interest
The authors declare that the research was conducted in the absence of any commercial or financial relationships that could be construed as a potential conflict of interest.
The handling editor declared a shared parent affiliation with the author HX at the time of review.
Publisher’s note
All claims expressed in this article are solely those of the authors and do not necessarily represent those of their affiliated organizations, or those of the publisher, the editors and the reviewers. Any product that may be evaluated in this article, or claim that may be made by its manufacturer, is not guaranteed or endorsed by the publisher.
References
Abdel-Hafez, S. M., Hathout, R. M., and Sammour, O. A. (2018). Tracking the transdermal penetration pathways of optimized curcumin-loaded chitosan nanoparticles via confocal laser scanning microscopy. Int. J. Biol. Macromol. 108, 753–764. doi:10.1016/j.ijbiomac.2017.10.170
Acuto, O., and Michel, F. (2003). CD28-mediated co-stimulation: A quantitative support for TCR signalling. Nat. Rev. Immunol. 3 (12), 939–951. doi:10.1038/nri1248
Ai, H., Yin, Q. S., Wang, Q., Fu, Y. W., Wei, X. D., and Song, Y. P. (2020). Safety and efficacy of patients with refractory B-lymphoblastic leukemia treated with anti-CD19 CAR-T cell bridging to allogeneic hematopoietic stem cell transplantation. Zhonghua Xue Ye Xue Za Zhi 41 (3), 239–244. doi:10.3760/cma.j.issn.0253-2727.2020.03.010
Al Hadidi, S., Schinke, C., Thanendrarajan, S., Zangari, M., and van Rhee, F. (2022). Enrollment of Black Participants in pivotal clinical trials supporting US Food and drug administration approval of chimeric antigen receptor-T cell therapy for hematological malignant Neoplasms. JAMA Netw. Open 5 (4), e228161. doi:10.1001/jamanetworkopen.2022.8161
Anderson, A. C., Joller, N., and Kuchroo, V. K. (2016). Lag-3, tim-3, and TIGIT: Co-Inhibitory receptors with specialized functions in immune regulation. Immunity 44 (5), 989–1004. doi:10.1016/j.immuni.2016.05.001
Baas, P., Scherpereel, A., Nowak, A. K., Fujimoto, N., Peters, S., Tsao, A. S., et al. (2021). First-line nivolumab plus ipilimumab in unresectable malignant pleural mesothelioma (CheckMate 743): A multicentre, randomised, open-label, phase 3 trial. Lancet 397 (10272), 375–386. doi:10.1016/S0140-6736(20)32714-8
Bai, C., Gao, S., Hu, S., Liu, X., Li, H., Dong, J., et al. (2020). Self-assembled multivalent aptamer nanoparticles with potential CAR-like characteristics could activate T cells and inhibit melanoma growth. Mol. Ther. Oncolytics 17, 9–20. doi:10.1016/j.omto.2020.03.002
Bober, Z., Bartusik-Aebisher, D., and Aebisher, D. (2022). Application of dendrimers in anticancer diagnostics and therapy. Molecules 27 (10), 3237. doi:10.3390/molecules27103237
Brouillard, A., Deshpande, N., and Kulkarni, A. A. (2021). Engineered multifunctional nano- and biological materials for cancer immunotherapy. Adv. Healthc. Mat. 10 (6), 2001680. doi:10.1002/adhm.202001680
Buchbinder, E. I., and Desai, A. (2016). CTLA-4 and PD-1 pathways: Similarities, differences, and implications of their inhibition. Am. J. Clin. Oncol. 39 (1), 98–106. doi:10.1097/COC.0000000000000239
Cao, X., Leonard, K., Collins, L. I., Cai, S. F., Mayer, J. C., Payton, J. E., et al. (2009). Interleukin 12 stimulates IFN-γ–Mediated inhibition of tumor-induced regulatory T-cell proliferation and enhances tumor clearance. Cancer Res. 69 (22), 8700–8709. doi:10.1158/0008-5472.CAN-09-1145
Chen, X., Gao, M., An, S., Zhao, L., Han, W., Wan, W., et al. (2022). Enhancing adoptive T cell therapy for solid tumor with cell-surface anchored immune checkpoint inhibitor nanogels. Nanomedicine 45, 102591. doi:10.1016/j.nano.2022.102591
Cheng, X., Yan, H., Pang, S., Ya, M., Qiu, F., Qin, P., et al. (2022). Liposomes as multifunctional nano-carriers for medicinal natural products. Front. Chem. 10, 963004. doi:10.3389/fchem.2022.963004
Cheng, Y., Zhao, P., Wu, S., Yang, T., Chen, Y., Zhang, X., et al. (2018). Cisplatin and curcumin co-loaded nano-liposomes for the treatment of hepatocellular carcinoma. Int. J. Pharm. 545 (1-2), 261–273. doi:10.1016/j.ijpharm.2018.05.007
Cifuentes-Rius, A., Desai, A., Yuen, D., Johnston, A. P. R., and Voelcker, N. H. (2021). Inducing immune tolerance with dendritic cell-targeting nanomedicines. Nat. Nanotechnol. 16 (1), 37–46. doi:10.1038/s41565-020-00810-2
Dai, H., Wu, Z., Jia, H., Tong, C., Guo, Y., Ti, D., et al. (2020). Bispecific CAR-T cells targeting both CD19 and CD22 for therapy of adults with relapsed or refractory B cell acute lymphoblastic leukemia. J. Hematol. Oncol. 13 (1), 30. doi:10.1186/s13045-020-00856-8
Delyanee, M., Akbari, S., and Solouk, A. (2021). Amine-terminated dendritic polymers as promising nanoplatform for diagnostic and therapeutic agents' modification: A review. Eur. J. Med. Chem. 221, 113572. doi:10.1016/j.ejmech.2021.113572
Dong, H., Strome, S. E., Salomao, D. R., Tamura, H., Hirano, F., Flies, D. B., et al. (2002). Tumor-associated B7-H1 promotes T-cell apoptosis: A potential mechanism of immune evasion. Nat. Med. 8 (8), 793–800. doi:10.1038/nm730
Duan, J., Cui, L., Zhao, X., Bai, H., Cai, S., Wang, G., et al. (2020). Use of immunotherapy with programmed cell Death 1 vs programmed cell Death ligand 1 inhibitors in patients with cancer: A systematic review and meta-analysis. JAMA Oncol. 6 (3), 375–384. doi:10.1001/jamaoncol.2019.5367
El Bairi, K., Haynes, H. R., Blackley, E., Fineberg, S., Shear, J., Turner, S., et al. (2021). The tale of TILs in breast cancer: A report from the international immuno-oncology biomarker working group. NPJ Breast Cancer 7 (1), 150. doi:10.1038/s41523-021-00346-1
Eyquem, J., Mansilla-Soto, J., Giavridis, T., van der Stegen, S. J. C., Hamieh, M., Cunanan, K. M., et al. (2017). Targeting a CAR to the TRAC locus with CRISPR/Cas9 enhances tumour rejection. Nature 543 (7643), 113–117. doi:10.1038/nature21405
Fang, R. H., Hu, C.-M. J., Luk, B. T., Gao, W., Copp, J. A., Tai, Y., et al. (2014). Cancer cell membrane-coated nanoparticles for anticancer vaccination and drug delivery. Nano Lett. 14 (4), 2181–2188. doi:10.1021/nl500618u
Fathi, M., Pustokhina, I., Kuznetsov, S. V., Khayrullin, M., Hojjat-Farsangi, M., Karpisheh, V., et al. (2021). T-cell immunoglobulin and ITIM domain, as a potential immune checkpoint target for immunotherapy of colorectal cancer. IUBMB Life 73 (5), 726–738. doi:10.1002/iub.2461
Figueiras, A., Domingues, C., Jarak, I., Santos, A. I., Parra, A., Pais, A., et al. (2022). New advances in biomedical application of polymeric micelles. Pharmaceutics 14 (8), 1700. doi:10.3390/pharmaceutics14081700
Francia, V., Schiffelers, R. M., Cullis, P. R., and Witzigmann, D. (2020). The biomolecular Corona of lipid nanoparticles for gene therapy. Bioconjug. Chem. 31 (9), 2046–2059. doi:10.1021/acs.bioconjchem.0c00366
Franzin, R., Netti, G. S., Spadaccino, F., Porta, C., Gesualdo, L., Stallone, G., et al. (2020). The use of immune checkpoint inhibitors in oncology and the occurrence of AKI: Where do we Stand? Front. Immunol. 11, 574271. doi:10.3389/fimmu.2020.574271
Gauthier, J., and Yakoub-Agha, I. (2017). Chimeric antigen-receptor T-cell therapy for hematological malignancies and solid tumors: Clinical data to date, current limitations and perspectives. Curr. Res. Transl. Med. 65 (3), 93–102. doi:10.1016/j.retram.2017.08.003
Golubovskaya, V., and Wu, L. (2016). Different subsets of T cells, memory, effector functions, and CAR-T immunotherapy. Cancers (Basel) 8 (3), 36. doi:10.3390/cancers8030036
Gordon, S. (2016). Phagocytosis: An immunobiologic process. Immunity 44 (3), 463–475. doi:10.1016/j.immuni.2016.02.026
Guasch, J., Hoffmann, M., Diemer, J., Riahinezhad, H., Neubauer, S., Kessler, H., et al. (2018). Combining adhesive nanostructured surfaces and costimulatory signals to increase T cell activation. Nano Lett. 18 (9), 5899–5904. doi:10.1021/acs.nanolett.8b02588
Guasch, J., Muth, C. A., Diemer, J., Riahinezhad, H., and Spatz, J. P. (2017). Integrin-assisted T-cell activation on nanostructured hydrogels. 17 (10), 6110–6116. doi:10.1021/acs.nanolett.7b02636
Guimaraes, P. P. G., Zhang, R., Spektor, R., Tan, M., Chung, A., Billingsley, M. M., et al. (2019). Ionizable lipid nanoparticles encapsulating barcoded mRNA for accelerated in vivo delivery screening. J. Control. Release 316, 404–417. doi:10.1016/j.jconrel.2019.10.028
Hald Albertsen, C., Kulkarni, J. A., Witzigmann, D., Lind, M., Petersson, K., and Simonsen, J. B. (2022). The role of lipid components in lipid nanoparticles for vaccines and gene therapy. Adv. Drug Deliv. Rev. 188, 114416. doi:10.1016/j.addr.2022.114416
Harmsen, S., Medine, E. I., Moroz, M., Nurili, F., Lobo, J., Dong, Y., et al. (2021). A dual-modal PET/near infrared fluorescent nanotag for long-term immune cell tracking. Biomaterials 269, 120630. doi:10.1016/j.biomaterials.2020.120630
Hockemeyer, D., Wang, H., Kiani, S., Lai, C. S., Gao, Q., Cassady, J. P., et al. (2011). Genetic engineering of human pluripotent cells using TALE nucleases. Nat. Biotechnol. 29 (8), 731–734. doi:10.1038/nbt.1927
Hornyak, S. C., Orentas, D. M., Karavodin, L. M., and Gehlsen, K. R. (2005). Histamine improves survival and protects against interleukin-2-induced pulmonary vascular leak syndrome in mice. Vasc. Pharmacol. 42 (4), 187–193. doi:10.1016/j.vph.2005.02.011
Hurton, L. V., Singh, H., Najjar, A. M., Switzer, K. C., Mi, T., Maiti, S., et al. (2016). Tethered IL-15 augments antitumor activity and promotes a stem-cell memory subset in tumor-specific T cells. Proc. Natl. Acad. Sci. U. S. A. 113 (48), E7788–E7797. doi:10.1073/pnas.1610544113
Ishihara, J., Ishihara, A., Potin, L., Hosseinchi, P., Fukunaga, K., Damo, M., et al. (2018). Improving efficacy and safety of agonistic anti-CD40 antibody through extracellular matrix affinity. Mol. Cancer Ther. 17 (11), 2399–2411. doi:10.1158/1535-7163.MCT-18-0091
Islam, M. A., Rice, J., Reesor, E., Zope, H., Tao, W., Lim, M., et al. (2021). Adjuvant-pulsed mRNA vaccine nanoparticle for immunoprophylactic and therapeutic tumor suppression in mice. Biomaterials 266, 120431. doi:10.1016/j.biomaterials.2020.120431
Jaunalksne, I., Brokāne, L., Petroška, D., Rasa, A., and Alberts, P. (2020). ECHO-7 oncolytic virus Rigvir® in an adjuvant setting for stage I uveal melanoma; A retrospective case report. Am. J. Ophthalmol. Case Rep. 17, 100615. doi:10.1016/j.ajoc.2020.100615
Jiang, W., Yuan, H., Chan, C. K., von Roemeling, C. A., Yan, Z., Weissman, I. L., et al. (2017). Lessons from immuno-oncology: A new era for cancer nanomedicine? Nat. Rev. Drug Discov. 16 (6), 369–370. doi:10.1038/nrd.2017.34
Kapadia, C. H., Perry, J. L., Tian, S., Luft, J. C., and DeSimone, J. M. (2015). Nanoparticulate immunotherapy for cancer. J. Control. Release 219, 167–180. doi:10.1016/j.jconrel.2015.09.062
Khan, M., Maker, A. V., and Jain, S. (2021). Role of NKT cells during viral infection and the development of NKT cell-based nanovaccines. Vaccines (Basel) 9 (6), 949. doi:10.3390/vaccines9090949
Kiesgen, S., Messinger, J. C., Chintala, N. K., Tano, Z., and Adusumilli, P. S. (2021). Comparative analysis of assays to measure CAR T-cell-mediated cytotoxicity. Nat. Protoc. 16 (3), 1331–1342. doi:10.1038/s41596-020-00467-0
Klebanoff, C. A., Finkelstein, S. E., Surman, D. R., Lichtman, M. K., Gattinoni, L., Theoret, M. R., et al. (2004). IL-15 enhances the in vivo antitumor activity of tumor-reactive CD8+ T Cells. Proc. Natl. Acad. Sci. U. S. A. 101 (7), 1969–1974. doi:10.1073/pnas.0307298101
Ko, C.-N., Zang, S., Zhou, Y., Zhong, Z., and Yang, C. (2022). Nanocarriers for effective delivery: Modulation of innate immunity for the management of infections and the associated complications. J. Nanobiotechnology 20 (1), 380. doi:10.1186/s12951-022-01582-8
Korman, A. J., Peggs, K. S., and Allison, J. P. (2006). Checkpoint blockade in cancer immunotherapy. Adv. Immunol. 90, 297–339. doi:10.1016/S0065-2776(06)90008-X
Kosti, P., Maher, J., and Arnold, J. N. (2018). Perspectives on chimeric antigen receptor T-cell immunotherapy for solid tumors. Front. Immunol. 9, 1104. doi:10.3389/fimmu.2018.01104
Kulkarni, A., Pandey, P., Rao, P., Mahmoud, A., Goldman, A., Sabbisetti, V., et al. (2016). Algorithm for designing nanoscale supramolecular therapeutics with increased anticancer efficacy. ACS Nano 10 (9), 8154–8168. doi:10.1021/acsnano.6b00241
Kumar, A. R., Devan, A. R., Nair, B., Vinod, B. S., and Nath, L. R. (2021). Harnessing the immune system against cancer: Current immunotherapy approaches and therapeutic targets. Mol. Biol. Rep. 48 (12), 8075–8095. doi:10.1007/s11033-021-06752-9
Lakshmanan, V. K., Jindal, S., Packirisamy, G., Ojha, S., Lian, S., Kaushik, A., et al. (2021). Nanomedicine-based cancer immunotherapy: Recent trends and future perspectives. Cancer Gene Ther. 28 (9), 911–923. doi:10.1038/s41417-021-00299-4
Lamla, T., and Erdmann, V. A. (2004). The Nano-tag, a streptavidin-binding peptide for the purification and detection of recombinant proteins. Protein Expr. Purif. 33 (1), 39–47. doi:10.1016/j.pep.2003.08.014
Lee, I.-H., Kwon, H.-K., An, S., Kim, D., Kim, S., Yu, M. K., et al. (2012). Imageable antigen-presenting gold nanoparticle vaccines for effective cancer immunotherapy in vivo. Angew. Chem. Int. Ed. Engl. 51 (35), 8800–8805. doi:10.1002/anie.201203193
Lee, J., Sands, I., Zhang, W., Zhou, L., and Chen, Y. (2021). DNA-inspired nanomaterials for enhanced endosomal escape. Proc. Natl. Acad. Sci. U. S. A. 118 (19), e2104511118. doi:10.1073/pnas.2104511118
Levy, P. L., and Gros, A. (2022). Fast track to personalized TCR T cell therapies. Cancer Cell 40 (5), 447–449. doi:10.1016/j.ccell.2022.04.013
Li, J., Yang, W., Cui, R., Wang, D., Chang, Y., Gu, W., et al. (2016). Metabolizerin vivo of fullerenes and metallofullerenes by positron emission tomography. Nanotechnology 27 (15), 155101. doi:10.1088/0957-4484/27/15/155101
Li, W., Li, J., Gao, J., Li, B., Xia, Y., Meng, Y., et al. (2011). The fine-tuning of thermosensitive and degradable polymer micelles for enhancing intracellular uptake and drug release in tumors. Biomaterials 32 (15), 3832–3844. doi:10.1016/j.biomaterials.2011.01.075
Li, W., Nakayama, M., Akimoto, J., and Okano, T. (2011). Effect of block compositions of amphiphilic block copolymers on the physicochemical properties of polymeric micelles. Polymer 52 (17), 3783–3790. doi:10.1016/j.polymer.2011.06.026
Li, W., Peng, A., Wu, H., Quan, Y., Li, Y., Lu, L., et al. (2020). Anti-cancer nanomedicines: A revolution of tumor immunotherapy. Front. Immunol. 11, 601497. doi:10.3389/fimmu.2020.601497
Li, W., Zhao, H., Qian, W., Li, H., Zhang, L., Ye, Z., et al. (2012). Chemotherapy for gastric cancer by finely tailoring anti-Her2 anchored dual targeting immunomicelles. Biomaterials 33 (21), 5349–5362. doi:10.1016/j.biomaterials.2012.04.016
Li, Z., Ye, L., Liu, J., Lian, D., and Li, X. (2020). Sorafenib-loaded nanoparticles based on biodegradable dendritic polymers for enhanced therapy of hepatocellular carcinoma. Int. J. Nanomedicine 15, 1469–1480. doi:10.2147/IJN.S237335
Liu, J., Chen, Z., Li, Y., Zhao, W., Wu, J., and Zhang, Z. (2021a). PD-1/PD-L1 checkpoint inhibitors in tumor immunotherapy. Front. Pharmacol. 12. doi:10.3389/fphar.2021.731798
Liu, J., Liu, K., Zhang, L., Zhong, M., Hong, T., Zhang, R., et al. (2021b). Heat/pH-boosted release of 5-fluorouracil and albumin-bound paclitaxel from Cu-doped layered double hydroxide nanomedicine for synergistical chemo-photo-therapy of breast cancer. J. Control. Release 335, 49–58. doi:10.1016/j.jconrel.2021.05.011
Liu, J., and Xu, J. (2020). Regulation of cancer immune checkpoints molecular and cellular mechanisms and therapy introduction. Regulation of cancer immune checkpoints: Molecular and cellular mechanisms and therapy. J. Xu. 1248, 1–6.
Liu, Q., Zhang, D., Qian, H., Chu, Y., Yang, Y., Shao, J., et al. (2020). Superior antitumor efficacy of IFN-α2b-Incorporated photo-cross-linked hydrogels combined with T cell transfer and low-dose irradiation against gastric cancer. Int. J. Nanomedicine 15, 3669–3680. doi:10.2147/IJN.S249174
Liu, Y., Xiao, L., Joo, K.-I., Hu, B., Fang, J., and Wang, P. (2014). In situ modulation of dendritic cells by injectable thermosensitive hydrogels for cancer vaccines in mice. Biomacromolecules 15 (10), 3836–3845. doi:10.1021/bm501166j
Lorenz, C., Fotin-Mleczek, M., Roth, G., Becker, C., Dam, T. C., Verdurmen, W. P. R., et al. (2014). Protein expression from exogenous mRNA: Uptake by receptor-mediated endocytosis and trafficking via the lysosomal pathway. RNA Biol. 8 (4), 627–636. doi:10.4161/rna.8.4.15394
Lu, K., He, C., Guo, N., Chan, C., Ni, K., Weichselbaum, R. R., et al. (2016). Chlorin-based nanoscale metal–organic Framework systemically Rejects colorectal cancers via synergistic photodynamic therapy and checkpoint blockade immunotherapy. J. Am. Chem. Soc. 138 (38), 12502–12510. doi:10.1021/jacs.6b06663
Lu, P., He, T., Zhou, X., Liu, H., Wang, H., Wu, Y., et al. (2020). Efficacy and safety of anti-CD19 CAR T-cell therapy in 110 patients with B-cell acute lymphoblastic leukemia with high-risk features. Blood Adv. 4 (10), 2325–2338. doi:10.1182/bloodadvances.2020001466
Luo, Y., Chen, Z., Sun, M., Li, B., Pan, F., Ma, A., et al. (2022). IL-12 nanochaperone-engineered CAR T cell for robust tumor-immunotherapy. Biomaterials 281, 121341. doi:10.1016/j.biomaterials.2021.121341
Man, F., Lim, L., Volpe, A., Gabizon, A., Shmeeda, H., Draper, B., et al. (2019). In Vivo PET tracking of 89Zr-labeled Vγ9Vδ2 T cells to mouse xenograft breast tumors activated with liposomal alendronate. Mol. Ther. 27 (1), 219–229. doi:10.1016/j.ymthe.2018.10.006
Manchanda, R., Fernandez-Fernandez, A., Paluri, S. L. A., and Smith, B. R. (2021). Nanomaterials to target immunity. Adv. Pharmacol. 91, 293–335. doi:10.1016/bs.apha.2021.03.003
Mao, J., Liu, S., Ai, M., Wang, Z., Wang, D., Li, X., et al. (2017). A novel melittin nano-liposome exerted excellent anti-hepatocellular carcinoma efficacy with better biological safety. J. Hematol. Oncol. 10 (1), 71. doi:10.1186/s13045-017-0442-y
Mao, J., Yin, H., Wang, L., Wu, J. Z., Xia, Y., Zhu, H. Y., et al. (2021). Prognostic value of 25-hydroxy vitamin D in extranodal NK/T cell lymphoma. Ann. Hematol. 100 (2), 445–453. doi:10.1007/s00277-020-04320-y
Martin, J. D., Cabral, H., Stylianopoulos, T., and Jain, R. K. (2020). Improving cancer immunotherapy using nanomedicines: Progress, opportunities and challenges. Nat. Rev. Clin. Oncol. 17 (4), 251–266. doi:10.1038/s41571-019-0308-z
Maruhashi, T., Sugiura, D., Okazaki, I. M., and Okazaki, T. (2020). LAG-3: From molecular functions to clinical applications. J. Immunother. Cancer 8 (2), e001014. doi:10.1136/jitc-2020-001014
Masarwy, R., Kampel, L., Horowitz, G., Gutfeld, O., and Muhanna, N. (2021). Neoadjuvant PD-1/PD-L1 inhibitors for resectable head and neck cancer: A systematic review and meta-analysis. JAMA Otolaryngol. Head. Neck Surg. 147 (10), 871–878. doi:10.1001/jamaoto.2021.2191
Mehta, N., Maddineni, S., Mathews, , Andres Parra Sperberg, R., Huang, P. S., and Cochran, J. R. (2019). Structure and functional binding epitope of V-domain Ig suppressor of T cell activation. Cell Rep. 28 (10), 2509–2516. doi:10.1016/j.celrep.2019.07.073
Mei, H., Li, C., Jiang, H., Zhao, X., Huang, Z., Jin, D., et al. (2021). A bispecific CAR-T cell therapy targeting BCMA and CD38 in relapsed or refractory multiple myeloma. J. Hematol. Oncol. 14 (1), 161. doi:10.1186/s13045-021-01170-7
Mi, P., Zhang, P., and Liu, G. (2016). Bio-inspired virus-like nanovesicle for effective vaccination. Hum. Vaccin. Immunother. 12 (8), 2090–2091. doi:10.1080/21645515.2016.1157244
Miao, L., Zhang, Y., and Huang, L. (2021). mRNA vaccine for cancer immunotherapy. Mol. Cancer 20 (1), 41. doi:10.1186/s12943-021-01335-5
Mier, J. W., Aronson, F. R., Numerof, R. P., Vachino, G., and Atkins, M. B. (1988). Toxicity of immunotherapy with interleukin-2 and lymphokine-activated killer cells. Pathol. Immunopathol. Res. 7 (6), 459–476. doi:10.1159/000157075
Mizukoshi, E., and Kaneko, S. (2019). Immune cell therapy for hepatocellular carcinoma. J. Hematol. Oncol. 12 (1), 52. doi:10.1186/s13045-019-0742-5
Mohamad, S. A., Safwat, M. A., Elrehany, M., Maher, S. A., Badawi, A. M., and Mansour, H. F. (2021). A novel nasal co-loaded loratadine and sulpiride nanoemulsion with improved downregulation of TNF-α, TGF-β and IL-1 in rabbit models of ovalbumin-induced allergic rhinitis. Drug Deliv. 28 (1), 229–239. doi:10.1080/10717544.2021.1872741
Mullard, A. (2020). Addressing cancer's grand challenges. Nat. Rev. Drug Discov. 19 (12), 825–826. doi:10.1038/d41573-020-00202-0
Ning, Y. M., Suzman, D., Maher, V. E., Zhang, L., Tang, S., Ricks, T., et al. (2017). FDA approval summary: Atezolizumab for the treatment of patients with progressive advanced urothelial carcinoma after platinum-containing chemotherapy. Oncologist 22 (6), 743–749. doi:10.1634/theoncologist.2017-0087
O'Konek, J. J., Landers, J. J., Janczak, K. W., Lindsey, H. K., Mondrusov, A. M., Totten, T. D., et al. (2020). Intranasal nanoemulsion vaccine confers long-lasting immunomodulation and sustained unresponsiveness in a murine model of milk allergy. Allergy 75 (4), 872–881. doi:10.1111/all.14064
Overchuk, M., Harmatys, K. M., Sindhwani, S., Rajora, M. A., Koebel, A., Charron, D. M., et al. (2020). Subtherapeutic photodynamic treatment facilitates tumor nanomedicine delivery and overcomes Desmoplasia. Nano Lett. 21 (1), 344–352. doi:10.1021/acs.nanolett.0c03731
Park, J., Wrzesinski, S. H., Stern, E., Look, M., Criscione, J., Ragheb, R., et al. (2012). Combination delivery of TGF-β inhibitor and IL-2 by nanoscale liposomal polymeric gels enhances tumour immunotherapy. Nat. Mat. 11 (10), 895–905. doi:10.1038/nmat3355
Passalacqua, R., Caminiti, C., Buti, S., Porta, C., Camisa, R., Braglia, L., et al. (2014). POLAR-01 trial InvestigatorsAdjuvant low-dose interleukin-2 (IL-2) plus interferon-α (IFN-α) in operable renal cell carcinoma (RCC): A phase III, randomized, multicentre trial of the Italian oncology group for clinical research (GOIRC). J. Immunother. 37 (9), 440–447. doi:10.1097/CJI.0000000000000055
Peer, D., and Margalit, R. (2004). Loading mitomycin C inside long circulating hyaluronan targeted nano-liposomes increases its antitumor activity in three mice tumor models. Int. J. Cancer 108 (5), 780–789. doi:10.1002/ijc.11615
Pérez Del Río, E., Santos, F., Rodriguez Rodriguez, X., Martínez-Miguel, M., Roca-Pinilla, R., Arís, A., et al. (2020). CCL21-loaded 3D hydrogels for T cell expansion and differentiation. Biomaterials 259, 120313. doi:10.1016/j.biomaterials.2020.120313
Perica, K., De León Medero, A., Durai, M., Chiu, Y. L., Bieler, J. G., Sibener, L., et al. (2014). Nanoscale artificial antigen presenting cells for T cell immunotherapy. Nanomedicine 10 (1), 119–129. doi:10.1016/j.nano.2013.06.015
Qiu, H., Min, Y., Rodgers, Z., Zhang, L., and Wang, A. Z. (2017). Nanomedicine approaches to improve cancer immunotherapy. WIREs Nanomed. Nanobiotechnol. 9 (5). doi:10.1002/wnan.1456
Rafei, H., Daher, M., and Rezvani, K. (2021). Chimeric antigen receptor (CAR) natural killer (NK)-cell therapy: Leveraging the power of innate immunity. Br. J. Haematol. 193 (2), 216–230. doi:10.1111/bjh.17186
Ramelyte, E., Tastanova, A., Balázs, Z., Ignatova, D., Turko, P., Menzel, U., et al. (2021). Oncolytic virotherapy-mediated anti-tumor response: A single-cell perspective. Cancer Cell 39 (3), 394–406. e4. doi:10.1016/j.ccell.2020.12.022
Ren, J., Liu, X., Fang, C., Jiang, S., June, C. H., and Zhao, Y. (2017). Multiplex Genome editing to generate universal CAR T cells resistant to PD1 inhibition. Clin. Cancer Res. 23 (9), 2255–2266. doi:10.1158/1078-0432.CCR-16-1300
Reschke, R., Yu, J., Flood, B., Higgs, E. F., Hatogai, K., and Gajewski, T. F. (2021). Immune cell and tumor cell-derived CXCL10 is indicative of immunotherapy response in metastatic melanoma. J. Immunother. Cancer 9 (9), e003521. doi:10.1136/jitc-2021-003521
Rosenberg, S. A., Lotze, M. T., Muul, L. M., Leitman, S., Chang, A. E., Ettinghausen, S. E., et al. (1985). Observations on the systemic administration of autologous lymphokine-activated killer cells and recombinant interleukin-2 to patients with metastatic cancer. N. Engl. J. Med. 313 (23), 1485–1492. doi:10.1056/NEJM198512053132327
Routy, B., Le Chatelier, E., Derosa, L., Duong, C. P. M., Alou, M. T., Daillère, R., et al. (2018). Gut microbiome influences efficacy of PD-1-based immunotherapy against epithelial tumors. Science 359 (6371), 91–97. doi:10.1126/science.aan3706
Rowshanravan, B., Halliday, N., and Sansom, D. M. (2018). CTLA-4: A moving target in immunotherapy. Blood 131 (1), 58–67. doi:10.1182/blood-2017-06-741033
Sabjan, K. B., Munawar, S. M., Rajendiran, D., Vinoji, S. K., and Kasinathan, K. (2020). Nanoemulsion as oral drug delivery - a review. Curr. Drug Res. Rev. 12 (1), 4–15. doi:10.2174/2589977511666191024173508
Santoni, M., Piva, F., Conti, A., Santoni, A., Cimadamore, A., Scarpelli, M., et al. (2018). Re: Gut microbiome influences efficacy of PD-1-based immunotherapy against epithelial tumors. Eur. Urol. 74 (4), 521–522. doi:10.1016/j.eururo.2018.05.033
Santos, F., Valderas-Gutiérrez, J., Pérez Del Río, E., Castellote-Borrell, M., Rodriguez, X. R., Veciana, J., et al. (2022). Enhanced human T cell expansion with inverse opal hydrogels. Biomater. Sci. 10 (14), 3730–3738. doi:10.1039/d2bm00486k
Satta, S., Shahabipour, F., Gao, W., Lentz, S. R., Perlman, S., Ashammakhi, N., et al. (2022). Engineering viral genomics and nano-liposomes in microfluidic platforms for patient-specific analysis of SARS-CoV-2 variants. Theranostics 12 (10), 4779–4790. doi:10.7150/thno.72339
Sau, S., Alsaab, H. O., Bhise, K., Alzhrani, R., Nabil, G., and Iyer, A. K. (2018). Multifunctional nanoparticles for cancer immunotherapy: A groundbreaking approach for reprogramming malfunctioned tumor environment. J. Control. Release 274, 24–34. doi:10.1016/j.jconrel.2018.01.028
Schmid, D., Park, C. G., Hartl, C. A., Subedi, N., Cartwright, A. N., Puerto, R. B., et al. (2017). T cell-targeting nanoparticles focus delivery of immunotherapy to improve antitumor immunity. Nat. Commun. 8 (1), 1747. doi:10.1038/s41467-017-01830-8
Shields, C. W. t., Wang, L. L., Evans, M. A., and Mitragotri, S. (2020). Materials for immunotherapy. Adv. Mat. 32 (13)–e1901633. doi:10.1002/adma.201901633
Simpson, J. T., Hunter, S. R., and Aytug, T. (2015). Superhydrophobic materials and coatings: A review. Rep. Prog. Phys. 78 (8), 086501. doi:10.1088/0034-4885/78/8/086501
Singh, A. K., and McGuirk, J. P. (2020). CAR T cells: Continuation in a revolution of immunotherapy. Lancet. Oncol. 21 (3), e168–e178. doi:10.1016/S1470-2045(19)30823-X
Song, H., Su, X., Yang, K., Niu, F., Li, J., Song, J., et al. (2015). CD20 antibody-conjugated Immunoliposomes for targeted chemotherapy of melanoma cancer initiating cells. J. Biomed. Nanotechnol. 11 (11), 1927–1946. doi:10.1166/jbn.2015.2129
Stanton, S. E., and Disis, M. L. (2016). Clinical significance of tumor-infiltrating lymphocytes in breast cancer. J. Immunother. Cancer 4, 59. doi:10.1186/s40425-016-0165-6
Sterner, R. C., and Sterner, R. M. (2021). CAR-T cell therapy: Current limitations and potential strategies. Blood Cancer J. 11 (4), 69. doi:10.1038/s41408-021-00459-7
Sul, J., Blumenthal, G. M., Jiang, X., He, K., Keegan, P., and Pazdur, R. (2016). FDA approval summary: Pembrolizumab for the treatment of patients with metastatic non-small cell lung cancer whose tumors express programmed death-ligand 1. Oncologist 21 (5), 643–650. doi:10.1634/theoncologist.2015-0498
Tan, S., Li, D., and Zhu, X. (2020). Cancer immunotherapy: Pros, cons and beyond. Biomed. Pharmacother. 124, 109821. doi:10.1016/j.biopha.2020.109821
Thiramanas, R., Jiang, S., Simon, J., Landfester, K., and Mailänder, V. (2020). Silica nanocapsules with different sizes and physicochemical properties as suitable nanocarriers for uptake in T-cells. Int. J. Nanomedicine 15, 6069–6084. doi:10.2147/IJN.S246322
Wang, C., Ye, Y., Hochu, G. M., Sadeghifar, H., and Gu, Z. (2016). Enhanced cancer immunotherapy by Microneedle patch-assisted delivery of anti-PD1 antibody. Nano Lett. 16 (4), 2334–2340. doi:10.1021/acs.nanolett.5b05030
Wang, G., Kang, X., Chen, K. S., Jehng, T., Jones, L., Chen, J., et al. (2020). An engineered oncolytic virus expressing PD-L1 inhibitors activates tumor neoantigen-specific T cell responses. Nat. Commun. 11 (1), 1395. doi:10.1038/s41467-020-15229-5
Wang, J. S. (2020). Progress and trend of CAR-T and PD-1 blockade in the treatment of acute myeloid leukemia-review. Zhongguo Shi Yan Xue Ye Xue Za Zhi 28 (3), 1069–1074. doi:10.19746/j.cnki.issn.1009-2137.2020.03.059
Wang, X., Zheng, Y., Qiu, L., Ouyang, H., Xu, X., Xu, W., et al. (2022). Evaluation and antitumor mechanism of functionalized chitosan-based polymeric micelles for oral delivery of paclitaxel. Int. J. Pharm. 625, 122138. doi:10.1016/j.ijpharm.2022.122138
Wang, Y., Liu, D., Zheng, Q., Zhao, Q., Zhang, H., Ma, Y., et al. (2014). Disulfide bond bridge insertion turns hydrophobic anticancer prodrugs into self-assembled nanomedicines. Nano Lett. 14 (10), 5577–5583. doi:10.1021/nl502044x
Wang, Z., and Cao, Y. J. (2020). Adoptive cell therapy targeting neoantigens: A frontier for cancer research. Front. Immunol. 11, 176. doi:10.3389/fimmu.2020.00176
Wayteck, L., Dewitte, H., De Backer, L., Breckpot, K., Demeester, J., De Smedt, S. C., et al. (2016). Hitchhiking nanoparticles: Reversible coupling of lipid-based nanoparticles to cytotoxic T lymphocytes. Biomaterials 77, 243–254. doi:10.1016/j.biomaterials.2015.11.016
Wei, J., Guo, Y., Wang, Y., Wu, Z., Bo, J., Zhang, B., et al. (2020). Clinical development of CAR T cell therapy in China: 2020 update. Cell. Mol. Immunol. 18 (4), 792–804. doi:10.1038/s41423-020-00555-x
Xie, J., Mei, L., Huang, K., Sun, Y., Iris, A., Ma, B., et al. (2019). A photo-inducible protein-inorganic nanoparticle assembly for active targeted tumour theranostics. Nanoscale 11 (13), 6136–6144. doi:10.1039/c9nr01120j
Xie, S., Hou, X., Yang, W., Shi, W., Yang, X., Duan, S., et al. (2021). Endoglin-aptamer-functionalized liposome-equipped PD-1-silenced T cells enhance antitumoral immunotherapeutic effects. Int. J. Nanomedicine. 16, 6017–6034. doi:10.2147/IJN.S317220
Xiong, W., Chen, Y., Kang, X., Chen, Z., Zheng, P., Hsu, Y. H., et al. (2018). Immunological synapse predicts effectiveness of chimeric antigen receptor cells. Mol. Ther. 26 (4), 963–975. doi:10.1016/j.ymthe.2018.01.020
Xu, F., Inci, F., Mullick, O., Gurkan, U. A., Sung, Y., Kavaz, D., et al. (2012). Release of magnetic nanoparticles from cell-encapsulating biodegradable nanobiomaterials. ACS Nano 6 (8), 6640–6649. doi:10.1021/nn300902w
Xu, Z., Wang, Y., Zhang, L., and Huang, L. (2014). Nanoparticle-delivered transforming growth factor-β siRNA enhances vaccination against advanced melanoma by modifying tumor microenvironment. ACS Nano 8 (4), 3636–3645. doi:10.1021/nn500216y
Xue, D., Liu, P., Chen, W., Zhang, C., and Zhang, L. (2019). An anti-CD103 antibody-drug conjugate prolongs the survival of pancreatic islet allografts in mice. Cell Death Dis. 10 (10), 735. doi:10.1038/s41419-019-1980-8
Yang, L., Ning, Q., Tang, S. S., and Cui, D. (2022). Recent advances and Next breakthrough in immunotherapy for cancer treatment. J. Immunol. Res. 2022, 8052212–8052219. doi:10.1155/2022/8052212
Yu, H. J., and De Geest, B. G. (2020). Nanomedicine and cancer immunotherapy. Acta Pharmacol. Sin. 41 (7), 879–880. doi:10.1038/s41401-020-0426-2
Yu, Q., Zhang, M., Chen, Y., Chen, X., Shi, S., Sun, K., et al. (2020). Self-assembled nanoparticles prepared from low-molecular-weight PEI and low-generation PAMAM for EGFRvIII-chimeric antigen receptor gene loading and T-cell transient modification. Int. J. Nanomedicine. 15, 483–495. doi:10.2147/IJN.S229858
Zeng, J., Li, X., Sander, M., Zhang, H., Yan, G., and Lin, Y. (2021). Oncolytic viro-immunotherapy: An emerging option in the treatment of Gliomas. Front. Immunol. 12, 721830. doi:10.3389/fimmu.2021.721830
Zhang, J., and Wang, L. (2019). The emerging world of TCR-T cell trials against cancer: A systematic review. Technol. Cancer Res. Treat. 18, 1533033819831068. doi:10.1177/1533033819831068
Zhang, Y., Li, N., Suh, H., and Irvine, D. J. (2018). Nanoparticle anchoring targets immune agonists to tumors enabling anti-cancer immunity without systemic toxicity. Nat. Commun. 9 (1), 6. doi:10.1038/s41467-017-02251-3
Zhang, Y., Liu, X., Wang, X., He, P., Xiao, C., Yu, H., et al. (2021). Nanoparticles composed of PEGylated alternating copolymer-combretastatin A4 conjugate for cancer therapy. Macromol. Biosci. 21 (8), 2100077. doi:10.1002/mabi.202100077
Zhang, Y., and Zhang, Z. (2020). The history and advances in cancer immunotherapy: Understanding the characteristics of tumor-infiltrating immune cells and their therapeutic implications. Cell. Mol. Immunol. 17 (8), 807–821. doi:10.1038/s41423-020-0488-6
Zhang, Y., and Zheng, J. (2020). Functions of immune checkpoint molecules beyond immune evasion. Adv. Exp. Med. Biol. 1248, 201–226. doi:10.1007/978-981-15-3266-5_9
Zhao, L., Cheng, S., Fan, L., Zhang, B., and Xu, S. (2021). TIM-3: An update on immunotherapy. Int. Immunopharmacol. 99, 107933. doi:10.1016/j.intimp.2021.107933
Zhu, X., Sun, Y., Chen, D., Li, J., Dong, X., Wang, J., et al. (2017). Mastocarcinoma therapy synergistically promoted by lysosome dependent apoptosis specifically evoked by 5-Fu@nanogel system with passive targeting and pH activatable dual function. J. Control. Release 254, 107–118. doi:10.1016/j.jconrel.2017.03.038
Keywords: immunotherapy, the CAR-T therapy, nanoparticles, drug delivery, nanoparticles combined with CAR-T therapy
Citation: Wang L, Xu H, Weng L, Sun J, Jin Y and Xiao C (2022) Activation of cancer immunotherapy by nanomedicine. Front. Pharmacol. 13:1041073. doi: 10.3389/fphar.2022.1041073
Received: 10 September 2022; Accepted: 05 December 2022;
Published: 22 December 2022.
Edited by:
Lesheng Teng, Jilin University, ChinaReviewed by:
Shahryar Khoshtinat Nikkhoi, Rutgers, The State University of New Jersey, United StatesChenhui Wang, Chongqing University, China
Yanan Shi, Yantai University, China
Copyright © 2022 Wang, Xu, Weng, Sun, Jin and Xiao. This is an open-access article distributed under the terms of the Creative Commons Attribution License (CC BY). The use, distribution or reproduction in other forums is permitted, provided the original author(s) and the copyright owner(s) are credited and that the original publication in this journal is cited, in accordance with accepted academic practice. No use, distribution or reproduction is permitted which does not comply with these terms.
*Correspondence: Ye Jin, anlfY2N1Y21AMTYzLmNvbQ==; Chunping Xiao, YnR4bndAMTYzLmNvbQ==
†These authors have contributed equally to this work and share first authorship