- 1College of First Clinical Medicine, Shandong University of Traditional Chinese Medicine, Jinan, China
- 2College of Traditional Chinese Medicine, Shandong University of Traditional Chinese Medicine, Jinan, China
- 3College of Traditional Chinese Medicine, Weifang Medical University, Weifang, China
- 4Department of Oncology, Weifang Traditional Chinese Hospital, Weifang, China
- 5Department of Special Medicine, School of Basic Medicine, Qingdao University, Qingdao, China
- 6Faculty of Chinese Medicine, Macau University of Science and Technology, Macau, Macau SAR, China
- 7State Key Laboratory of Quality Research in Chinese Medicine, Macau University of Science and Technology, Macau, Macau SAR, China
- 8Department of Experimental Research Center, China Academy of Chinese Medical Sciences, Beijing, China
Harnessing the broad immunostimulatory capabilities of chemotherapy in combination with immune checkpoint inhibitors has improved immunotherapy outcomes in patients with cancer. Certain chemotherapeutic agents can extensively modify the tumor microenvironment (TME), resulting in the reprogramming of local immune responses. Although chemotherapeutic agents with an enhanced generation of potent anti-tumor immune responses have been tested in preclinical animal models and clinical trials, this strategy has not yet shown substantial therapeutic efficacy in selected difficult-to-treat cancer types. In addition, the efficacy of chemotherapeutic agent-based monotherapy in eliciting a long-term anti-tumor immune response is restricted by the immunosuppressive TME. To enhance the immunomodulatory effect of chemotherapy, researchers have made many attempts, mainly focusing on improving the targeted distribution of chemotherapeutic agents and designing combination therapies. Here, we focused on the mechanisms of the anti-tumor immune response to chemotherapeutic agents and enumerated the attempts to advance the use of chemo-immunotherapy. Furthermore, we have listed the important considerations in designing combinations of these drugs to maximize efficacy and improve treatment response rates in patients with cancer.
Introduction
After years of intensive research to utilize the power of cytotoxic responses to fight cancer, we are witnessing a revolution in cancer therapy that harnesses the tumor recognition and destruction capabilities of the immune system (Principe et al., 2022). Conventional chemotherapy works by blocking the cell cycle and inducing apoptosis, which led to the development of multiple cytotoxic agents with non-specific targets, such as the synthesis of nucleic acids or proteins (Zitvogel et al., 2008). The effects of chemotherapeutic agents on the immune system have been neglected because of the use of cell culture and immune-deficient animal models (Galluzzi et al., 2020a). Recently, an abundance of preclinical literature has demonstrated that the immunomodulatory efficacy of conventional chemotherapeutic agents, including platinum-based drugs, anthracyclines, mitoxantrone, and taxanes, was much higher in immunocompetent mouse models than in their immunodeficient counterparts (Zitvogel et al., 2016). Further studies found that the activation of the immune system by chemotherapeutic agents leads to a two-pronged tumor eradication process: first, chemotherapeutic agents rely on cytotoxicity to directly destroy tumor cells (Casares et al., 2005); and second, the anti-tumor immune response produced by effector lymphocytes, such as cytotoxic T lymphocytes (CTLs), by secreting cytotoxic molecules and expressing ligands (Fas/TRAIL) that can bind to cell death receptors (Minute et al., 2020). This provides a partial understanding of the use of chemotherapeutic agents to potentiate therapeutic responses to immunotherapy and reprogram the tumor immune microenvironment.
The rise of immunotherapy has shifted public attention to a new field of immunomodulatory anti-tumor therapy. The improved clinical benefits brought by chemo-immunotherapy have further changed the long-held belief that chemotherapeutic agents are immunosuppressive (Galluzzi et al., 2020b). Hence, it is vital to identify the biological mechanisms underlying chemotherapy-induced immune stimulation and the key factors that can improve the efficacy of immunotherapy. There is increasing evidence that both dose and treatment interval are indispensable variables for the effective immunomodulatory effects of chemotherapeutic agents (Wu and Waxman, 2018; Fares et al., 2020; Lai et al., 2021). However, further preclinical research on chemotherapeutic agents at appropriate doses and treatment intervals has resulted in moderate anti-tumor immune responses and unsatisfactory results. Most drugs have difficulty producing durable immune efficacy because of their poor stability and off-target distribution in vivo and because of tumor heterogeneity and the persistently immunosuppressive microenvironment. To overcome these restrictive factors and improve overall anti-tumor efficacy, combinatorial regimens of chemotherapeutic agents, including immunotherapy agents, have been tested in clinical trials (Pfirschke et al., 2016). The intersection between chemotherapy and immunotherapy has been under evaluation for a long time, and several FDA-approved chemo-immunotherapy regimens have demonstrated significant advantages in real-world clinical applications. Therapies to obtain selective chemotherapy mediated by nanoformulations (e.g., nab-paclitaxel) or antibody-drug conjugates (ADCs; e.g., TDM-1, brentuximab vedotin) have been recently designed to achieve targeted distribution and local accumulation of anti-tumor drugs, showing significant alterations in the immunogenicity of the tumor microenvironment (TME) (Goldberg, 2015; Gu and Mooney, 2016; Coats et al., 2019).
In this review, we first summarized the immunomodulatory effects of chemotherapeutic agents, with a particular emphasis on immunogenicity and immuno-adjuvant effects. Additionally, we described the main factors affecting the immunomodulatory effects of chemotherapeutic agents and how the drugs can be used in new combinations to maximize treatment outcomes and improve response rates. Finally, we outlined some key considerations in the design of combinatorial regimens of chemotherapeutic agents to increase the application of chemo-immunotherapy in the future.
Multiple immunoregulatory mechanisms of chemotherapeutic agents
The ability of chemotherapeutic agents to drive adaptive immunity depends on three main parameters: immunogenicity, adjuvanticity, and microenvironmental conditions, all of which dramatically influence neoplastic cells to develop potentially immunogenic mutations or immune susceptibility, ultimately blocking both the priming and effector phases of the immunological response (Figure 1).
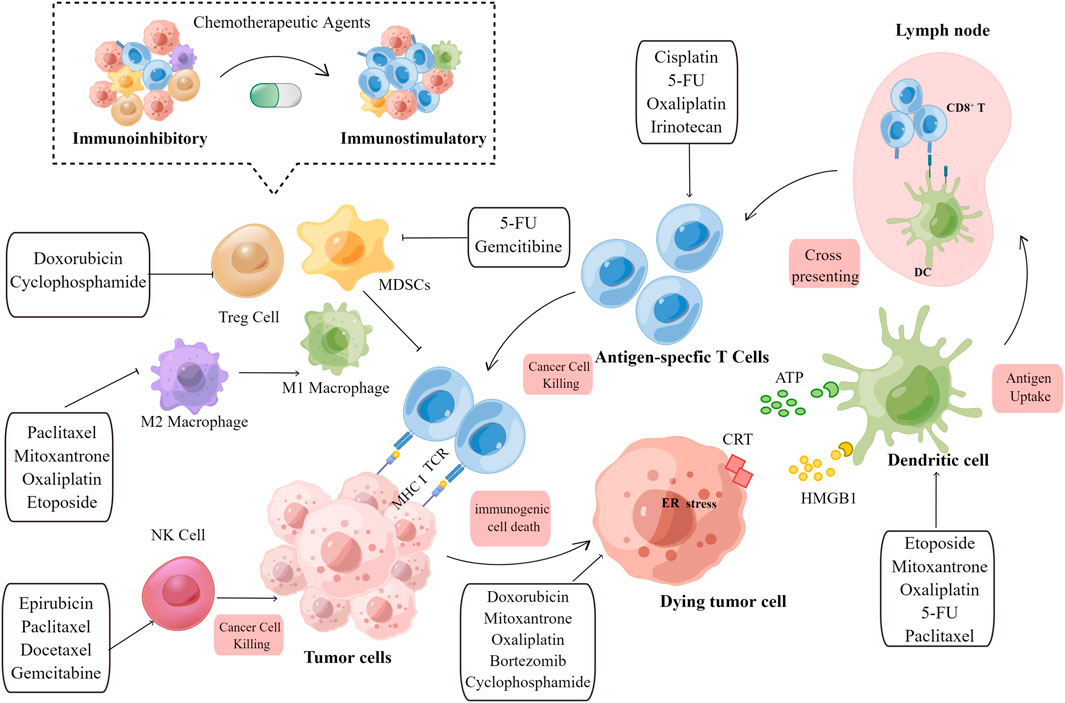
FIGURE 1. Overview of the immunostimulatory properties of chemotherapeutic agents. Immunogenic effects: When tumor cells are exposed to chemotherapeutic agents, TAA, TSA, and DAMPs released by dying tumor cells are engulfed by immature DCs, which promote APC maturation. Archived antigen-bearing APCs then migrate to the tumor-draining lymph node, where they cross-prime to T cells. Subsequently, antigen-specific T cells undergo clonal expansion. Activated T cells then recognize tumor cells and mediate the cytotoxic killing of tumor cells. Immuno-adjuvant effects: Chemotherapeutic agents can activate immune effector cells, including natural killer (NK) cells and dendritic cells (DCs), and promote the infiltration of cytotoxic T cells. The immunoinhibitory microenvironment is then transformed into an immunostimulatory microenvironment upon the depletion of immunosuppressive cells, including Treg cells, M2 macrophages, and MDSCs. Chemotherapeutic agents and their corresponding immunomodulatory effects are shown in the text boxes with arrows. TAA, tumor-associated antigen; TSA, tumor specific antigen; DAMP, damage-associated molecular pattern; DC, dendritic cell; APC, antigen-presenting cell; NK, natural killer cell; MDSC, myeloid-derived suppressor cells; Treg, regulatory T cell; MHC, major histocompatibility complex; TCR, T cell receptor; CRT,calreticulin; HMGB1, high-mobility group box 1.
Immunogenic regulation of chemotherapeutic agents
Eliciting immunogenic cell death
Various cytotoxic chemotherapy agents, such as anthracyclines, platinum-based drugs, mitoxantrone, oxaliplatin, and taxanes, that block cell cycle progression and induce apoptosis have been demonstrated to potentiate immunogenic cell death (ICD) (Casares et al., 2005; Kopecka et al., 2018; Li C et al., 2020; Tesniere et al., 2010; Fucikova et al., 2022). As a form of regulated cell death, ICD is amenable to activating the cellular stress response that promotes the spatiotemporally coordinated production and localization of damage-associated molecular patterns (DAMPs) from dying cells in immunocompetent hosts (Kroemer et al., 2022). DAMPs can be recognized by inherent pattern recognition receptors, such as Toll-like receptors and Nod-like receptors, on dendritic cells (DCs), which attract these cells to the tumor cells and ultimately promote tumor-associated antigen (TAA) presentation. Subsequently, antigen-presenting cells (APCs) represented by DCs, could migrate to the draining lymph nodes and present antigens to CTLs, killing tumor cells presenting the major histocompatibility complex (MHC). This event then triggers immune memory in a favorable environment with high levels of co-stimulatory signals and cytokines.
Howener, the release of DAMPs from dying cancer cells occurs during a failed intracellular stress response, and the kinetics and intensity of DAMP release can be determined through the cellular response driven by the initiating stressor (Clement et al., 2021). An increasing amount of research has confirmed that cells succumbing to intracellular stress can initiate an adaptive immune response associated with immunological memory. In contrast to chemotherapeutic agents that do not elicit ICD, most ICD inducers efficiently stimulate integrated stress responses (ISRs) (Costa-Mattioli and Walter, 2020). As a result, defects in various mechanisms associated with maintaining cellular homeostasis could influence the strength of the immune response.
ISR, a multi-pronged molecular mechanism for maintaining cellular homeostasis, is an adaptive signaling pathway activated by various forms of cellular stress, such as endoplasmic reticulum (ER) stress and the unfolded protein response (UPR) (Hetz et al., 2020; Kohli et al., 2021). One of the core molecules modulating ISR is eukaryotic translation initiation factor 2 alpha (eIF2α), whose phosphorylation is regulated by eIF2α kinase (EIF2AK1-4) (Bezu et al., 2018). Cisplatin was previously defined as incapable of triggering ICD because it does not promote the kinase-dependent phosphorylation of eIF2α or exposure of ER chaperones on the cell surface (Tesniere et al., 2010). This can be corrected by administering drugs, such as digoxin, tetracycline, that stimulate the ER stress response (Michaud et al., 2014; Xiang et al., 2020). In addition, non-phosphorylated eIF2α (eIF2αS51A) has been shown to inhibit the activation of anthracycline-driven autophagy, further demonstrating the central role of ISR in chemotherapy-induced ICD (Martins et al., 2011). This implies that one of the mechanisms underlying chemotherapeutic agent-induced ICD is ISR associated with cellular stress.
Enhancing the antigenicity of cancer cells
While there is ample evidence that chemotherapy increases the immunogenicity of cancer cells via ICD, little is known about the chemotherapy-induced enhancement of antigenicity. In contrast to ICD, the phenotypic changes in tumor cells upon exposure to non-lethal/sublethal doses of chemotherapeutic agents are mainly manifested into enhanced antigenicity, making tumors more sensitive to CTL-mediated killing (Hodge et al., 2013). The chemotherapy-induced enhancement of tumor antigenicity is mainly associated with the upregulation of major histocompatibility complex (MHC) expression and the emergence of tumor neoantigens or TAA. Many commonly used cytotoxic agents, such as topotecan, mitoxantrone, gemcitabine, and cisplatin, have been found to upregulate the expression of antigen-presenting machinery (Grabosch et al., 2019; Li et al., 2021; Zhou et al., 2021). In addition, some chemotherapy drugs, such as docetaxel, cisplatin and 5-fluorouracil, can also promote the expression of TAA and tumor-specific antigen (TSA) and enhance antigenicity (Coral et al., 2002; Correale et al., 2003; Spehner et al., 2020). Furthermore, immune responses of CD4+ T cells against tumor-associated antigens were observed following oxaliplatin treatment in chemotherapy-naïve patients with mCRC (colorectal cancer) (Galaine et al., 2019). Therefore, anti-tumor T-cell responses stand out as key elements for the long-term efficacy of chemotherapy upon treatment discontinuation.
Immuno-adjuvant effects of chemotherapeutic agents
The immune microenvironment in which cancer cells reside is a major determinant of their ability to trigger adaptive immune responses, even in the presence of sufficient antigenicity and immunogenicity (Belli et al., 2018). The immuno-adjuvant effects of the chemotherapeutic agents discussed in this review may principally rely on the recruitment and activation of immunologic effector cells to reverse the effect of an immunosuppressive microenvironment.
Promoting dendritic cell-mediated antigen presentation
DCs have become the core initiator and regulator cells associated with anti-cancer immunity, owing to their highly complex antigen presentation mechanism. However, the abnormal evolution of tumors interferes with the mechanism of DC maturation and antigen processing in tumors, resulting in immunosuppressive effects (Murphy and Murphy, 2022). An increasing amount of evidence supports the role of chemotherapeutic agents in modulating DCs at low doses, particularly in promoting their maturation (Pfannenstiel et al., 2010; Wanderley et al., 2018) and antigen presentation capability (Shurin et al., 2009; Zhang et al., 2021). Antigen cross-presentation is a prerequisite for the induction of an effective anti-tumor immune response, and its immunomodulatory potency can be improved by apoptosis-inducing chemotherapy. McDonnell AM et al. demonstrates that gemcitabine-induced tumor cell apoptosis can increase the incidence of nuclear antigen cross-presentation in vivo, which is associated with an increased proportion of CTLs (McDonnell et al., 2015a; McDonnell et al., 2015b). Other drugs such as cyclophosphamide (CTX) act by altering DC biology, especially by changing DC subsets (Nakahara et al., 2010; Fumet et al., 2020).
Trafficking and infiltration of immune effector cells
In addition, one potentially important biological response to chemotherapy is the ability to initiate T cell influx into the TME. Activated immune cells could enter the bloodstream and initiate T cell influx into the TME. In vivo treatment of tumor-bearing mice showed that doxorubicin, paclitaxel significantly increased the number of CD4+ and CD8+ T cells, which may be related to the expression of IFN-γ and granase B (Tsuda et al., 2007; Alizadeh et al., 2014; Heeren et al., 2019). Recent studies have shown that cisplatin can promote the production of the chemokine CCL20 and the pro-inflammatory cytokine IL-1β in tumor sites, leading to the synthesis and activation of ILC3 (Bruchard et al., 2022). ILC3 promotes the production of chemokine CXCL10 in tumors, which is associated with the generation of CD4+ and CD8+ T lymphocytes (Goc et al., 2021). It has been observed that partial chemotherapeutic agents activate NK cell-mediated anti-tumor immune responses, depending on the release of cytokines (Markasz et al., 2007; Garofalo et al., 2021). Chemotherapeutic agents also affect the composition of tumor-infiltrating lymphocytes (TILs), and it has been proven that the composition ratio between TILs and infiltrating cells is related to the efficacy of immunotherapy (Farhood et al., 2019; Saleh and Elkord, 2019). In metastatic colon cancer, multi-drug chemotherapy regimens, including FOLFOX (5-FU, folinic acid, and oxaliplatin) and FOLFIRI (5-FU, folinic acid, and irinotecan), significantly altered the composition ratio of peripheral blood lymphocytes (Maeda et al., 2011; Che et al., 2021). This change is mainly manifested by an increase in the CD8/Foxp3 TIL ratio, which can effectively predict and improve the recurrence-free and overall survival of patients. These data suggest that the immune adjuvant effect of chemotherapeutic agents runs through all stages of the cancer immune cycle and plays a role in continuous anti-tumor immune regulation.
Depletion of immunosuppressive cells
Immunosuppressive cells, represented by myeloid-derived suppressor cells (MDSCs), regulatory T cells (Tregs), and tumor-associated macrophages (TAMs), are key influencing factors of immune escape and immunotherapy resistance and play a major role in the anti-tumor immune response (Tie et al., 2022). Interestingly, some chemotherapeutic agents have been shown to deplete immunosuppressive cell populations, thus remodeling the immune cell landscape to mount an efficient anti-tumor immune response.
The absolute or relative depletion of Treg cells, especially during tumor infiltration, is associated with the (re)induction of protective anti-cancer immunity, indicating a shift from silent or ineffective immune responses to open or effective ones (Ikegawa and Matsuoka, 2021; Nishikawa and Koyama, 2021). Previous studies on CTX-induced Treg cell depletion have obtained favorable results, confirming that CTX can mediate multiple mechanisms to selectively deplete Treg cells and further improve the effectiveness of immunotherapy by increasing the activation of autoreactive T cells (Laheurte et al., 2020). Cytokines such as IFN, IL-6, and CXCL10 play a role in CTX-mediated restoration of Treg homeostasis. CTX-induced Treg depletion and the expression of related cytokines have been shown to be directly or indirectly regulated by IFN regulatory factor (IRF)-1 (Buccione et al., 2018). In addition to CTX, many other chemotherapeutic agents selectively target Tregs. For example, patients with NSCLC who received four cycles of docetaxel chemotherapy presented fewer peripheral Tregs than at baseline, similar to that observed with cisplatin and vinorelbine (Roselli et al., 2013; Li et al., 2014).
Recent studies have shown that several aspects of macrophage biology are affected by chemotherapy. Some chemotherapeutic agents, such as CTX and doxorubicin, can activate macrophages by enhancing the secretion of GM-CSF to generate anti-tumor immune responses in mouse models of breast cancer or further potentiating Th1 responses that can enhance the tumoricidal effects of macrophages (Machiels et al., 2001; Li T et al., 2020). In addition to promoting macrophage activation, chemotherapeutic drugs can also induce macrophage polarization. For example, paclitaxel promoted the repolarization of TAMs from an M2-like to an M1-like phenotype (Wanderley et al., 2018). However, studies have similarly shown that a taxane-based chemotherapy regimen can enhance the recruitment of Tie2-expressing macrophages (TEMs) in breast cancer, promote tumor cell entry into circulation, and lead to metastasis (Karagiannis et al., 2017). Thus, the mechanism underlying the impact of chemotherapeutic agents on macrophage biology is still unclear but is most likely dependent on microenvironmental factors.
The same phenomenon can be observed with cytotoxic agents against MDSCs since many chemotherapeutic agents can selectively inhibit MDSC differentiation. Cisplatin has been shown to inhibit the conversion of monocyte precursors to inhibitory M-MDSCs through the regulation of the STAT3-COX-2 signaling axis, overcoming M-MDSC-mediated immunosuppression and improving the overall response rate to cancer immunotherapy (Van Wigcheren et al., 2021). Oxaliplatin is also known to selectively deplete MDSCs, especially Mo-MDSCs, by decreasing the expression of the immunosuppressive functional mediators argininase 1 (ARG1) and NADPH oxidase 2 (NOX2) (Kim and Kim, 2019). However, it appears that different drug combinations affect the immunomodulatory effects of chemotherapeutic drugs. Both 5-FU and gemcitabine are anti-metabolic chemotherapeutic agents that inhibit the proliferation of MDSCs by inhibiting thymidylate synthase and cytidine deaminase (Vincent et al., 2010). Interestingly, this effect was only maintained with oxaliplatin alone, and the blocking effect of MDSCs was discontinued when 5-FU was combined with irinotecan (Kanterman et al., 2014; Li et al., 2022). Given the complex regulatory effects of chemotherapeutic agents on MDSCs, this is an important area worthy of further research.
Challenges with immunoregulatory chemotherapeutic agents to be as successful as monotherapies
As mentioned above, various chemotherapeutic agents can alter the crosstalk between cancer and the immune system at multiple levels. This alteration may inhibit or kill cancer cells in an immunogenic or immuno-adjuvant-modulated manner, affect different leukocyte populations, or affect systemic physiological responses. However, it is often difficult to overcome the complexity and compensatory evolution of tumors, resulting in limited anti-tumor immune effects. Through a review of relevant studies, we have listed several key factors affecting the clinical application of chemotherapy in the following sections (Figure 2).
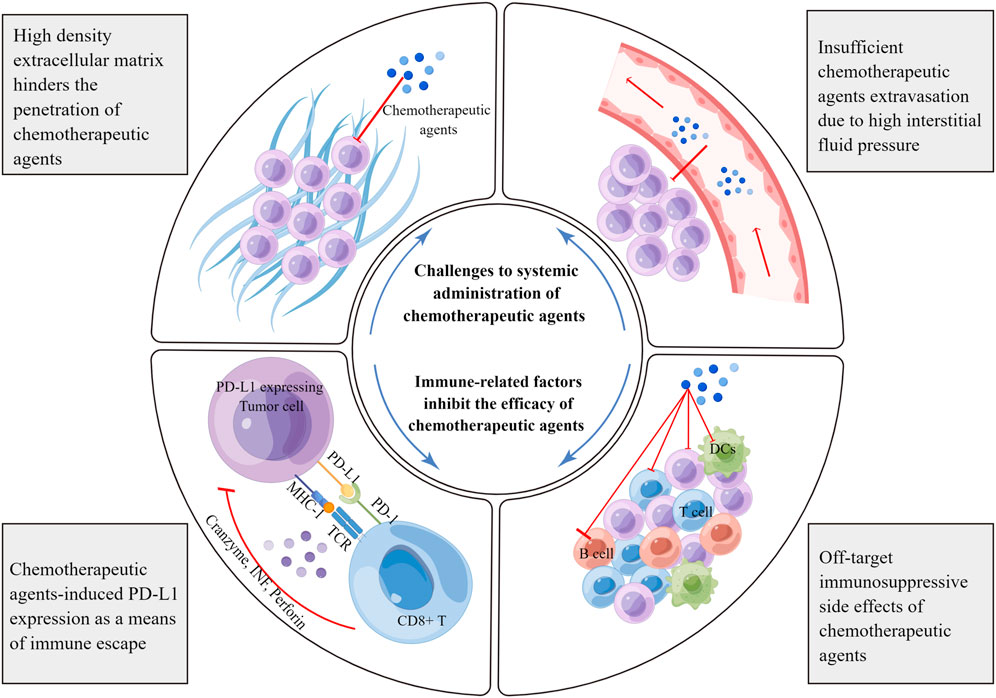
FIGURE 2. Limiting factors affecting the immunoregulatory effects of chemotherapeutic agents. Chemotherapeutic agents can induce the expression of negative immune checkpoints, especially PD-L1. PD-L1 associates with the PD-1 receptor on effector T cells, blunting anti-tumor immune responses and facilitating immune escape. Furthermore, chemotherapeutic agents target rapidly proliferating immune cells, resulting in off-target side effects. The dense network of the extracellular matrix hinders the spread of chemotherapeutic agents in tumors. In addition, there is high interstitial fluid pressure in tumor tissues, which prevents the extravasation of chemotherapeutic agents from the blood vessels. MHC, major histocompatibility complex; TCR, T cell receptor; TNF, tumor necrosis factor; DC, dendritic cell.
Substantial barriers that hinder the penetration of chemotherapeutic agents
Emerging evidence suggests that the TME imposes biological barriers that hinder effective cancer therapy (Binnewies et al., 2018). The structures of these biological barriers are highly irregular and are mainly characterized by a highly disordered vascular network, the absence of a lymphatic network, and high interstitial fluid pressure (Heldin et al., 2004; Jain et al., 2007). In addition, the abundant expression of extracellular matrix proteins and the proliferation of interstitial tissue in the TME constitute the mechanical barrier in cancer, resulting in insufficient tumor infiltration and obstructed blood circulation after the intravenous injection of chemotherapeutic agents (Casazza et al., 2014). Previous experiments have shown that gaps between vascular endothelial cells rarely occur in tumors in mouse models and in tumors from patients with cancer; this phenomenon is always associated with the insufficient extravasation of chemotherapeutic agents (Golombek et al., 2018). This observation suggests that trans-endothelial pathways play an integral role in the precise infiltration of chemotherapeutic agents into tumors (Sindhwani et al., 2020). To promote more efficient cancer chemotherapy in the clinic, various nanoscale drug delivery systems have been explored to achieve tumor-targeted delivery of chemotherapeutic agents. These drug delivery systems can be used to enhance the tissue-specific distribution of chemotherapeutic agents, activate immune cells, and amplify anti-tumor immune responses (Saeed et al., 2019; Wang W. et al., 2020). However, the improvement effect of traditional nanomedicine is still limited owing to the metabolic environment in tumor tissue, such as hypoxia and inflammation, which negatively affect the intratumoral immune activation effect of chemotherapeutic agents.
Immune resistance to chemotherapeutic agents
During tumor-host co-evolution, robust immunosuppressive circuits established in the TME hamper the ability of chemotherapeutics to drive anti-tumor immunomodulatory effects. Consequently, it is crucial to maintain the function of chemotherapeutic agents within the immunosuppressive TME. Although cisplatin, paclitaxel, and 5-fluorouracil promoted the clonal expansion of tumor-specific CD8+ T cells, an inadequate magnitude of an immune response was eventually manifested, owing to the increased expression of microenvironment-related IDO (Munn and Mellor, 2016). Additionally, oxaliplatin has been shown to suppress T-cell responses by promoting PD-L1 expression on DCs and reducing the expression of the co-stimulatory molecules CD80/CD86 (Tel et al., 2012). Subsequent trials have also revealed that the combination of 5-FU and oxaliplatin decreased the expression of immune checkpoints PD-L1 and PD-L2 on the surface of DCs, promoting DC maturation in tumor-bearing mice (Hong et al., 2018). Notably, the perturbation of multiple factors in the immune system drives the expression of these negative regulatory pathways, making chemotherapy monotherapy insufficient to reverse the effects of an immunosuppressive microenvironment. In addition to the influence of immunosuppressive microenvironment-related factors, the activation of unknown immune-related signaling pathways during treatment is also a key factor that inhibits the anti-tumor efficacy of chemotherapeutic drugs. Recent studies have shown that cessation of immunogenic chemotherapy may provoke the restoration of the immunosuppressive microenvironment and the immune escape of tumor cells, which could accelerate the malignant progression of tumors. Gemcitabine, a standard chemotherapy regimen for advanced pancreatic ductal carcinoma, induces the infiltration of pro-inflammatory macrophages into the liver and activates cytotoxic T cells. However, Bellomo et al. experimentally observed that after cessation of the standard chemotherapy regimen, tumor cells recruited growth- and arrest-specific 6 (Gas6)-expressing neutrophils to the liver through the secretion of CXCL1 and CXCL2, resulting in liver metastases (Bellomo et al., 2022).
Off-target immunosuppressive side effects of chemotherapeutics
Many chemotherapeutic agents have notable immunosuppressive side effects. These effects occur either directly by inhibiting or killing effector cells, characterized by rapid proliferation, or indirectly by inducing anergy or immune paralysis (Zitvogel et al., 2008). The off-target effects of chemotherapeutic agents on the immune system are extensive and could affect their therapeutic efficacy (Medzhitov and Janeway, 2002). This applies to CTX, which primarily impairs the proliferation of peripheral T cells and hinders their effector functions. In addition, post-transplant CTX alters immune signatures and leads to impaired T cell reconstitution in allogeneic hematopoietic stem cell transplant by increasing Treg while reducing naïve T cells (Zhao et al., 2022). Current research suggests that the number and function of immune-infiltrating effector cells are closely related to the efficacy and prognosis (Denkert et al., 2018; Paijens et al., 2021); similarly, the depletion of non-targeted immune cells affects the anti-tumor effect of chemotherapy. Clinically, owing to myelosuppression and its related immunosuppressive adverse effects, the maximum tolerated dose of chemotherapeutic agents is rarely used for the routine treatment of patients with cancer. However, it has also been suggested that the recovery phase from chemotherapy-induced lymphopenia can serve as an important window to enhance the anti-tumor immune response, providing a reasonable and feasible theoretical support for the application of chemotherapy followed by immunotherapy (Williams et al., 2007; Moschella et al., 2011). Hence, the role of transient immunosuppression in long-term immune responses and immune memory needs to be further demonstrated.
To generate a strong and durable anti-tumor immune response, it is necessary to modify the chemotherapeutic agents themselves or design a more efficient combination regimen. This can be done by changing the distribution of the chemotherapeutic drug in the body or using a combination of drugs that can better target the tumors of interest, activating the anti-tumor immune response, improving the immune response rate, and reducing the incidence of non-target immune-related side effects.
Attempts to improve the immunomodulatory effect of chemotherapeutic agents
Antibody-drug conjugates
ADCs were originally designed to exploit the exquisite specificity of antibodies to deliver targeted and potent chemotherapeutics. ADCs undergo a complex sequence of drug internalization procedures that often require intracellular processing and eventual payload release (Drago et al., 2021). Emerging evidence suggests that the payloads act as the workhorse of ADCs, exerting tumor-killing effects through complex interactions between ADCs and various components of the tumor immune microenvironment. A variety of cytotoxic drugs and their derivatives were used in the design of ADC drugs, which are mainly divided into the following categories: microtubule inhibitors/stability disruptors, such as auristatin derivatives (monomethyl auristatin F [MMAF] or monomethyl auristatin E [MMAE]); Mayden derivatives (DM1/DM4); and calicheamicin and its derivatives that act on DNA grooves or breaks in the double helix. Some ADC payloads, such as MMAF/MMAE, auristatins, pyrrolobenzodiazepines, and anthracycline T-PNU, have been identified as potent substances that could promote DC maturation and activate antigenic responses (Muller et al., 2014a; D'Amico et al., 2019). Additional studies have shown that cytotoxic chemotherapeutics, such as dolastatin, MMAE, and maytansinoid, are commonly used as ADC payloads to stimulate CD8+ effector cell migration to experimental tumors grown in mice (Muller et al., 2014b).
Several experiments have confirmed the immunomodulatory effects of the payload-based ADCs. For example, brentuximab vedotin (SGN-35) comprises an anti-CD30 antibody conjugated to vcMMAE via a protease-cleavable linker. Immunohistochemical analysis of Hodgkin’s lymphoma tumor samples from patients treated with SGN-35 revealed significant changes in the number of intra-tumoral CD8+ effector T cells (Theurich et al., 2013). Furthermore, when used alone or in combination with an anti-PD-L1 antibody, ADCs that target EphA2 molecules in combination with a tubulin payload could induce ICD, showing a strong ability to induce CD8+ T-cell infiltration (Muller et al., 2015; Agostinetto et al., 2022). These studies provide evidence that ADC-destabilizing drugs stimulate the cancer immunity cycle. Recently, the FDA-approved drug belantamab mafodotin (GSK2857916), an ADC that targets B-cell maturation antigen (BCMA) in multiple myeloma cells using MMAF as a payload, induced ICD in BCMA-expressing cancer cells and promoted DC activation in vivo (Yu et al., 2020). In a syngeneic mouse model, GSK2857916 was shown to induce ICD, promoting the intratumoral enrichment of TILs and T cell-dependent anti-tumor activity (Montes de Oca et al., 2021). To further optimize the targeted immunomodulatory effects of ADCs, researchers have screened potential targets of ADCs that are not just limited to malignant cells. Based on this, a CD25-targeted pyrrolobenzodiazepine dimer-based ADC was investigated for its ability to deplete Tregs and eradicate established tumors (Zammarchi et al., 2020).
In addition to ADC monotherapy, numerous ongoing or completed clinical trials have demonstrated the clinical efficacy of ADC combined with chemotherapy (Table 1), which showed good efficacy and safety in hematologic tumors and breast cancer. However, there are many challenges in the development of this type of drug. For example, the selection of potential targets, improvement of potential toxicity caused by off-target effects, and development of cytotoxic drugs with other mechanisms of action require numerous experiments to verify.
Nanosized drug delivery systems based on the tumor microenvironment
Owing to the negative role of the TME in compromising the therapeutic response of various cancer therapies, therapeutic modalities targeting the TME appear to enhance the overall response rate of patients to cancer treatment (Tang et al., 2021). Nano-drug delivery systems, which provide a prerequisite for the TME-targeted delivery of chemotherapeutic agents, are a promising strategy to address these challenges, because of their adequate circulation time, increased intratumoral accumulation and retention, efficient uptake by tumor cells, and precise release at tumor tissues (Weber and Mule, 2015; Abdou et al., 2020). Depending on the particular metabolic characteristics of the TME, such as hypoxia, acidic pH, overexpressed enzymes (e.g., matrix metalloproteinases 2 and 9 [MMP-2 and-9], hyaluronidase, legumain, or cathepsin B), reactive oxygen species (ROS), or glutathione (GSH), different responsive nanomaterials have been developed. These nanomaterials were initially applied to load chemotherapeutic agents and achieve intratumoral local delivery. Generally, these nanoparticles remain intact in the blood circulation to protect their payloads from degradation or leakage until they are activated by the TME (Zhou et al., 2020). The loaded chemotherapeutic agents are subsequently released into local tumor tissues, focusing on the modulation of immune-related molecules that normalize immune responses and induce ICD to restore the ability of CTLs to eradicate tumor cells.
Wang et al. developed an ROS-responsive hydrogel loaded with gemcitabine that showed significant therapeutic efficacy against both primary tumors and distant metastases. In response to ROS signaling in the TME, the released gemcitabine reduced the percentage of immunosuppressive cells, including MDSCs and M2 macrophages, while increasing PD-L1 expression in cancer cells, T cells, macrophages, and DCs. In turn, this increased PD-L1 expression synergizes with the effect of anti-PD-L1 antibodies to improve immunotherapy effect (Wang et al., 2018). A pH-responsive nanoparticle co-loaded with the ICD inducer doxorubicin was designed to induce potent ICD in cancer cells while synergistically limiting the production of immunosuppressive kynurenine by binding to alkylated NLG919 (an inhibitor of indoleamine 2,3-dioxygenase 1). Moreover, the introduction of pH-responsive nanoparticles could enable deep intratumoral penetration of therapeutic agents and effectively neutralize low pH levels, reversing the immunosuppressive effect of the TME (Zhu et al., 2020). Furthermore, Koo AN et al. developed GSH-responsive core-shell nanoparticles carrying docetaxel for selective delivery in tumor-bearing mice. These self-assembled nanoscale coordination polymer core-shell nanoparticles are cleaved by GSH in tumor cells, releasing docetaxel, and leading to T-cell priming and anti-tumor effects (Koo et al., 2012). However, complex nanosystems face hinderance such as possible interactions between different components and overall stability, which may also limit the immunomodulatory effects of chemotherapeutic drugs. Therefore, it is necessary to determine the best combination scheme to ensure that the targeted effects of chemotherapy drugs are maintained.
Chemotherapy combined with PD-L1/PD-1 inhibitors
Since the early 2010s, many immunotherapies based on immune checkpoint inhibition have been developed, especially antibodies targeting the PD-1/PD-L1 pathway, thereby changing the status quo of cancer treatment. Tumors respond poorly to immunotherapy despite their revolutionary efficacy, which can be explained by exhibiting a loss of lymphocyte infiltration (NK, CD8, Th1), the so-called “cold tumors.“(Fumet et al., 2020) As an immune adjuvant, chemotherapy can increase the number of TILs, reverse the cold immune environment, and reduce the enrichment of immunosuppressive cells, significantly improving the response rate to immune checkpoint inhibitors (Park et al., 2020). Moreover, several chemotherapeutic agents, such as cisplatin, oxaliplatin, doxorubicin, and paclitaxel, can also enhance the expression of immune checkpoints, mostly PD-L1 (Ng et al., 2018). In this context, the expression of PD-L1 on cancer cells can associate with the PD-1 receptor on effector T cells, reducing anti-tumor immune responses and facilitating immune escape (Li et al., 2021).
Harnessing the broad immunostimulatory capabilities of chemotherapeutic agents in combination with immune checkpoint inhibitors has shown great promise with improved clinical outcomes. The FDA has already approved combinations of chemotherapy and PD-L1/PD-1 therapy (Gandhi et al., 2018; Horn et al., 2018; Schmid et al., 2018). Numerous phase III trials are currently underway for various cancer types that will alter the face of oncology for multiple indications. Our review has focused on the administration sequence and dosing schedule of combined immunochemotherapy.
Immunochemotherapy combinations currently comprise adjuvant standard-of-care chemotherapy regimens being added to immunotherapy, but the elements in this combination have not been optimized. With the deepening of the clinical application of chemotherapy combined with Immune checkpoint inhibitors, more studies began to focus on practical clinical issues such as the schedule, interval, and cycle of the combination therapy to obtain better clinical benefits (Principe et al., 2022; Leonetti et al., 2019). Studies have shown that neoadjuvant chemotherapy plays an important role in cancer treatment, and a large number of clinical trials are currently underway to evaluate the mechanism of action of immunochemotherapy regimens in neoadjuvant therapy (Table 2). In addition, increasing attention has been given to the sequence of administering anti-PD-1 antibodies and chemotherapeutic agents. A recent phase II study demonstrated that chemotherapy prior to anti-PD-L1 treatment could exert a better immunomodulatory effect in the neoadjuvant treatment of locally advanced esophageal squamous cell carcinoma (Fukuoka et al., 2019). Although this study is still ongoing, the current results suggest that delaying toripalimab administration to day 3 in immuno-chemotherapy may achieve higher pCR rates than administering both agents on the same day. This conclusion requires further large-sample clinical trials for verification.
With these general considerations related to drug scheduling, researchers should further investigate the different doses and lengths of drug-free periods required for various drugs and cancer types to improve the efficacy of immunogenic chemotherapy. This would further standardize the combination dynamics of different chemotherapy and immunotherapy drugs for different cancer types.
Chemotherapy combined with oncolytic virus therapy
Oncolytic viruses (OVs) comprise a novel immune anti-tumor therapy that can target tumor cells and replicate within them, thereby killing tumor cells (Martin and Bell, 2018). OVs, including adenovirus, parvovirus, reovirus, coxsackie virus, and HSV, can promote the expression of DAMPs to induce ICD. The synergistic combination of OVs and chemotherapeutic agents can compensate for the current situation wherein the inability of chemotherapeutic agents to induce ICD is the limiting factor. This combination has a significantly improved efficacy in enhancing the stimulation of an anti-tumor immune response compared with single-drug treatment (Habiba et al., 2020). For example, the combination of ONYX-015 and cisplatin significantly improved OS in a tumor xenograft model, showing superior efficacy to cisplatin monotherapy (Khuri et al., 2000). In addition, combination therapy with the oncolytic HSV-1 and mitoxantrone increased the accumulation of antigen-specific CD8+ T cells in the tumor (Workenhe et al., 2013). Several clinical trials employing OVs in association with chemotherapeutic agents in various tumor types have been performed or are ongoing (Table 3). In a phase I/II trial of intravenous carboplatin/paclitaxel plus reovirus showed good objective responses and inhibition of tumor progression in cancer of the head and neck (Karapanagiotou et al., 2012). ONCOS-102 (previously known as CGTG-102) is an adenovirus equipped with GM-CSF. In a phase I trial, the safety and recommended dose of the oncolytic adenovirus ONCOS-102 combined with low-dose oral CTX for advanced cancer was investigated (Ranki et al., 2016). In a subsequent next phase I/II clinical trial, the safety and clinical efficacy of chemotherapy combined with ONCOS-102 in the treatment of malignant pleural mesothelioma were further evaluated (Kuryk et al., 2016). Therefore, chemotherapy combined with OV seems to provide a new therapeutic strategy for targeting the immune microenvironment since combining the two can better exert anti-tumor immune regulation.
Chemotherapy combined with cellular stress inducers
The mechanisms through which chemotherapeutic agents induce ICD are complex, diverse, and mainly related to cellular stress responses, manifested through ER stress, induction of autophagy, and ATP release (Zhou et al., 2021; Radogna and Diederich, 2018). Owing to tumor heterogeneity and the influence of the physicochemical properties of chemotherapeutic drugs, the restricted adjuvanticity of chemotherapy compromises the ability of dying cells to activate anti-tumor adaptive immunity. Recently, several cellular stress inducers have been discovered and used synergistically with chemotherapeutic agents to improve the overall response rate of ICD. As a representative cardiac glycoside, digoxin can potentially induce characteristic biomarkers of ICD, such as CRT exposure, ATP secretion, and HMGB1 release (Menger et al., 2012). When combined with cisplatin, a significant percentage of tumor-free mice were revaccinated with live tumor cells in mouse models (Xiang et al., 2020). This experimental result was confirmed to depend on the activation of anti-tumor immune responses. Likewise, statins have great potential to promote calreticulin exposure on the tumor cell surface and enhance cellular signaling associated with ER stress. In addition, statins can promote the activation and recruitment of APCs and tumor-specific CD8+ T cells in tumor tissues and draining lymph nodes, further enhancing the sensitivity of immune effector cells to ICD-related markers (Kwon et al., 2021). This effect was shown to be significantly improved after combined treatment with cisplatin. Moreover, chemotherapy-induced autophagy is required for the trafficking of T lymphocytes and dendritic cells. Oxaliplatin (OXA) has been experimentally shown to activate immunomodulatory responses by activating autophagy-dependent ATP release (Martins et al., 2012). On this basis, Wang et al. used the autophagy inducer Thiostrepton in combination with OXA and observed the shrinkage of TC1 NSCLC and MCA205 fibrosarcoma cells in an immunoactive C57BL/6 mouse model, which was dependent on the activation of T lymphocytes (Wang Y. et al., 2020). However, further preclinical studies focusing on the complete and dynamic biological mechanisms that account for cellular stress inducers are needed to facilitate the development of additional cell stress inducers. This would enable clinicians to strategically combine chemotherapy to induce immunogenic cellular stress.
Conclusion
As traditional anti-cancer agents, chemotherapeutic agents have exhibited significant benefits to patients with cancer. Although long considered immunosuppressive, there is mounting evidence to support the selection of chemotherapeutic agents with immunostimulatory properties as effective anti-cancer therapies. However, despite multiple mechanisms, the critical role of chemotherapeutics in determining the overall efficacy of cancer treatment remains unclear. With an improved understanding of the association of immunology and tumor biology at the molecular level, immunogenic cell death emerges as one of several crucial mechanisms by which chemotherapeutics elicit tumor-targeted immune responses. Prospective preclinical results and preliminary clinical findings suggest that the integrated stress response induced by chemotherapeutic agents may be key to their long-term immunomodulatory effects. Further research and exploration of the mechanisms underlying the immunomodulatory effects of chemotherapy are required.
Accumulating clinical data indicate the potency of chemotherapeutics in combination with other anti-cancer drugs, particularly immunotherapy. When combining chemotherapeutic agents with immunotherapies or designing suitable delivery systems for chemotherapeutic drugs, such as nano-targeted delivery and ADCs, one should consider the relative merits of the constituent drugs in terms of their targets, pharmacokinetics, and safety. In addition, we also need to consider the administration sequence, time interval, and dose of the combination therapy. Lastly, biomarkers that can identify responses to combinatorial regimens of chemotherapeutic agents remain unknown. Recently, liquid biopsy, owing to its ability to monitor the immune landscape of the TME dynamically, appears to be useful for guiding immunogenic chemotherapy and providing a real-time biomarker screening approach. Overall, combinatorial regimens of chemotherapeutic agents are promising therapeutic platforms for optimizing combinatorial cancer treatments.
Author contributions
JL wrote the manuscript, searched the literature and prepared the figures and tables. YY was involved in the design of the study, and revised the manuscript. CL and CG provided article ideas, modified the tables and revised the manuscript. JZ, LL, QW, and WM performed literature research and collected relevant articles. QZ and CS participated in and helped draft the manuscript. All authors read and approved the final manuscript.
Funding
This work was supported by the National Natural Science Foundation of China (grant number 82174222 and 81973677).
Acknowledgments
Figures were created by Figdraw (www.figdraw.com).
Conflict of Interest
The authors declare that the research was conducted in the absence of any commercial or financial relationships that could be construed as a potential conflict of interest.
Publisher’s note
All claims expressed in this article are solely those of the authors and do not necessarily represent those of their affiliated organizations, or those of the publisher, the editors and the reviewers. Any product that may be evaluated in this article, or claim that may be made by its manufacturer, is not guaranteed or endorsed by the publisher.
Abbreviations
ADC, antibody-drug conjugate; ADCs, Antibody-drug conjugates; APC, antigen-presenting cell; BCMA, B-cell maturation antigen; CTL, cytotoxic T lymphocyte; CTX, cyclophosphamide; DAMP, damage-associated molecular pattern; DC, dendritic cell; ER, endoplasmic reticulum; eIF2α, eukaryotic translation initiation factor 2 alpha; GSH, glutathione; ICD, immunogenic cell death; ISR, integrated stress response; MMAE, monomethyl auristatin E; MMAF, monomethyl auristatin F; MDSCs, myeloid-derived suppressor cells; MHC, major histocompatibility complex; MMP, matrix metalloproteinases; NOX2, NADPH oxidase 2; OXA, Oxaliplatin; OVs, oncolytic viruses; ROS, reactive oxygen species; TAA, tumor-associated antigen; TAMs, tumor-associated macrophages; TEMs, Tie2-expressing macrophages; Tregs, regulatory T cells; TME, tumor microenvironment; UPR, unfolded protein response.
References
Abdou, P., Wang, Z., Chen, Q., Chan, A., Zhou, D. R., Gunadhi, V., et al. (2020). Advances in engineering local drug delivery systems for cancer immunotherapy. Wiley Interdiscip. Rev. Nanomed. Nanobiotechnol. 12, e1632. doi:10.1002/wnan.1632
Agostinetto, E., Montemurro, F., Puglisi, F., Criscitiello, C., Bianchini, G., Del Mastro, L., et al. (2022). Immunotherapy for HER2-positive breast cancer: Clinical evidence and future perspectives. Cancers (Basel) 14, 2136. doi:10.3390/cancers14092136
Alizadeh, D., Trad, M., Hanke, N. T., Larmonier, C. B., Janikashvili, N., Bonnotte, B., et al. (2014). Doxorubicin eliminates myeloid-derived suppressor cells and enhances the efficacy of adoptive T-cell transfer in breast cancer. Cancer Res. 74, 104–118. doi:10.1158/0008-5472.CAN-13-1545
Belli, C., Trapani, D., Viale, G., D'Amico, P., Duso, B. A., Della Vigna, P., et al. (2018). Targeting the microenvironment in solid tumors. Cancer Treat. Rev. 65, 22–32. doi:10.1016/j.ctrv.2018.02.004
Bellomo, G., Rainer, C., Quaranta, V., Astuti, Y., Raymant, M., Boyd, E., et al. (2022). Chemotherapy-induced infiltration of neutrophils promotes pancreatic cancer metastasis via Gas6/AXL signalling axis. Gut, 325272. doi:10.1136/gutjnl-2021-325272
Bezu, L., Sauvat, A., Humeau, J., Gomes-da-Silva, L. C., Iribarren, K., Forveille, S., et al. (2018). eIF2α phosphorylation is pathognomonic for immunogenic cell death. Cell Death Differ. 25, 1375–1393. doi:10.1038/s41418-017-0044-9
Binnewies, M., Roberts, E. W., Kersten, K., Chan, V., Fearon, D. F., Merad, M., et al. (2018). Understanding the tumor immune microenvironment (TIME) for effective therapy. Nat. Med. 24, 541–550. doi:10.1038/s41591-018-0014-x
Bruchard, M., Geindreau, M., Perrichet, A., Truntzer, C., Ballot, E., Boidot, R., et al. (2022). Recruitment and activation of type 3 innate lymphoid cells promote antitumor immune responses. Nat. Immunol. 23, 262–274. doi:10.1038/s41590-021-01120-y
Buccione, C., Fragale, A., Polverino, F., Ziccheddu, G., Arico, E., Belardelli, F., et al. (2018). Role of interferon regulatory factor 1 in governing Treg depletion, Th1 polarization, inflammasome activation and antitumor efficacy of cyclophosphamide. Int. J. Cancer 142, 976–987. doi:10.1002/ijc.31083
Casares, N., Pequignot, M. O., Tesniere, A., Ghiringhelli, F., Roux, S., Chaput, N., et al. (2005). Caspase-dependent immunogenicity of doxorubicin-induced tumor cell death. J. Exp. Med. 202, 1691–1701. doi:10.1084/jem.20050915
Casazza, A., Di Conza, G., Wenes, M., Finisguerra, V., Deschoemaeker, S., and Mazzone, M. (2014). Tumor stroma: A complexity dictated by the hypoxic tumor microenvironment. Oncogene 33, 1743–1754. doi:10.1038/onc.2013.121
Che, L. H., Liu, J. W., Huo, J. P., Luo, R., Xu, R. M., He, C., et al. (2021). A single-cell atlas of liver metastases of colorectal cancer reveals reprogramming of the tumor microenvironment in response to preoperative chemotherapy. Cell Discov. 7, 80. doi:10.1038/s41421-021-00312-y
Clement, C. C., Nanaware, P. P., Yamazaki, T., Negroni, M. P., Ramesh, K., Morozova, K., et al. (2021). Pleiotropic consequences of metabolic stress for the major histocompatibility complex class II molecule antigen processing and presentation machinery. Immunity 54, 721–736.e10. doi:10.1016/j.immuni.2021.02.019
Coats, S., Williams, M., Kebble, B., Dixit, R., Tseng, L., Yao, N. S., et al. (2019). Antibody-drug conjugates: Future directions in clinical and translational strategies to improve the therapeutic index. Clin. Cancer Res. 25, 5441–5448. doi:10.1158/1078-0432.CCR-19-0272
Coral, S., Sigalotti, L., Altomonte, M., Engelsberg, A., Colizzi, F., Cattarossi, I., et al. (2002). 5-aza-2'-deoxycytidine-induced expression of functional cancer testis antigens in human renal cell carcinoma: Immunotherapeutic implications. Clin. Cancer Res. 8, 2690–2695.
Correale, P., Aquino, A., Giuliani, A., Pellegrini, M., Micheli, L., Cusi, M. G., et al. (2003). Treatment of colon and breast carcinoma cells with 5-fluorouracil enhances expression of carcinoembryonic antigen and susceptibility to HLA-A(*)02.01 restricted, CEA-peptide-specific cytotoxic T cells in vitro. Int. J. Cancer 104, 437–445. doi:10.1002/ijc.10969
Costa-Mattioli, M., and Walter, P. (2020). The integrated stress response: From mechanism to disease. Science 368, eaat5314. doi:10.1126/science.aat5314
D'Amico, L., Menzel, U., Prummer, M., Muller, P., Buchi, M., Kashyap, A., et al. (2019). A novel anti-HER2 anthracycline-based antibody-drug conjugate induces adaptive anti-tumor immunity and potentiates PD-1 blockade in breast cancer. J. Immunother. Cancer 7, 16. doi:10.1186/s40425-018-0464-1
Denkert, C., von Minckwitz, G., Darb-Esfahani, S., Lederer, B., Heppner, B. I., Weber, K. E., et al. (2018). Tumour-infiltrating lymphocytes and prognosis in different subtypes of breast cancer: A pooled analysis of 3771 patients treated with neoadjuvant therapy. Lancet. Oncol. 19, 40–50. doi:10.1016/S1470-2045(17)30904-X
Drago, J. Z., Modi, S., and Chandarlapaty, S. (2021). Unlocking the potential of antibody-drug conjugates for cancer therapy. Nat. Rev. Clin. Oncol. 18, 327–344. doi:10.1038/s41571-021-00470-8
Fares, J. E., El Tomb, P., Khalil, L. E., Atwani, R. W., Moukadem, H. A., Awada, A., et al. (2020). Metronomic chemotherapy for patients with metastatic breast cancer: Review of effectiveness and potential use during pandemics. Cancer Treat. Rev. 89, 102066. doi:10.1016/j.ctrv.2020.102066
Farhood, B., Najafi, M., and Mortezaee, K. (2019). CD8(+) cytotoxic T lymphocytes in cancer immunotherapy: A review. J. Cell. Physiol. 234, 8509–8521. doi:10.1002/jcp.27782
Fucikova, J., Palova-Jelinkova, L., Klapp, V., Holicek, P., Lanickova, T., Kasikova, L., et al. (2022). Immunological control of ovarian carcinoma by chemotherapy and targeted anticancer agents. Trends Cancer 8, 426–444. doi:10.1016/j.trecan.2022.01.010
Fukuoka, E., Yamashita, K., Tanaka, T., Sawada, R., Sugita, Y., Arimoto, A., et al. (2019). Neoadjuvant chemotherapy increases PD-L1 expression and CD8(+) tumor-infiltrating lymphocytes in esophageal squamous cell carcinoma. Anticancer Res. 39, 4539–4548. doi:10.21873/anticanres.13631
Fumet, J. D., Limagne, E., Thibaudin, M., and Ghiringhelli, F. (2020). Immunogenic cell death and elimination of immunosuppressive cells: A double-edged sword of chemotherapy. Cancers (Basel) 12, E2637. doi:10.3390/cancers12092637
Galaine, J., Turco, C., Vauchy, C., Royer, B., Mercier-Letondal, P., Queiroz, L., et al. (2019). CD4 T cells target colorectal cancer antigens upregulated by oxaliplatin. Int. J. Cancer 145, 3112–3125. doi:10.1002/ijc.32620
Galluzzi, L., Humeau, J., Buque, A., Zitvogel, L., and Kroemer, G. (2020b). Immunostimulation with chemotherapy in the era of immune checkpoint inhibitors. Nat. Rev. Clin. Oncol. 17, 725–741. doi:10.1038/s41571-020-0413-z
Galluzzi, L., Vitale, I., Warren, S., Adjemian, S., Agostinis, P., Martinez, A. B., et al. (2020a). Consensus guidelines for the definition, detection and interpretation of immunogenic cell death. J. Immunother. Cancer 8, e000337. doi:10.1136/jitc-2019-000337
Gandhi, L., Rodriguez-Abreu, D., Gadgeel, S., Esteban, E., Felip, E., De Angelis, F., et al. (2018). Pembrolizumab plus chemotherapy in metastatic non-small-cell lung cancer. N. Engl. J. Med. 378, 2078–2092. doi:10.1056/NEJMoa1801005
Garofalo, C., De Marco, C., and Cristiani, C. M. (2021). NK cells in the tumor microenvironment as new potential players mediating chemotherapy effects in metastatic melanoma. Front. Oncol. 11, 754541. doi:10.3389/fonc.2021.754541
Goc, J., Lv, M., Bessman, N. J., Flamar, A. L., Sahota, S., Suzuki, H., et al. (2021). Dysregulation of ILC3s unleashes progression and immunotherapy resistance in colon cancer. Cell 184, 5015–5030.e16. doi:10.1016/j.cell.2021.07.029
Goldberg, M. S. (2015). Immunoengineering: How nanotechnology can enhance cancer immunotherapy. Cell 161, 201–204. doi:10.1016/j.cell.2015.03.037
Golombek, S. K., May, J. N., Theek, B., Appold, L., Drude, N., Kiessling, F., et al. (2018). Tumor targeting via EPR: Strategies to enhance patient responses. Adv. Drug Deliv. Rev. 130, 17–38. doi:10.1016/j.addr.2018.07.007
Grabosch, S., Bulatovic, M., Zeng, F., Ma, T., Zhang, L., Ross, M., et al. (2019). Cisplatin-induced immune modulation in ovarian cancer mouse models with distinct inflammation profiles. Oncogene 38, 2380–2393. doi:10.1038/s41388-018-0581-9
Gu, L., and Mooney, D. J. (2016). Biomaterials and emerging anticancer therapeutics: Engineering the microenvironment. Nat. Rev. Cancer 16, 56–66. doi:10.1038/nrc.2015.3
Habiba, U., Hossain, E., Yanagawa-Matsuda, A., Chowdhury, A., Tsuda, M., Zaman, A. U., et al. (2020). Cisplatin relocalizes RNA binding protein HuR and enhances the oncolytic activity of E4orf6 deleted adenovirus. Cancers (Basel) 12, E809. doi:10.3390/cancers12040809
Heeren, A. M., van Luijk, I. F., Lakeman, J., Pocorni, N., Kole, J., de Menezes, R. X., et al. (2019). Neoadjuvant cisplatin and paclitaxel modulate tumor-infiltrating T cells in patients with cervical cancer. Cancer Immunol. Immunother. 68, 1759–1767. doi:10.1007/s00262-019-02412-x
Heldin, C. H., Rubin, K., Pietras, K., and Ostman, A. (2004). High interstitial fluid pressure - an obstacle in cancer therapy. Nat. Rev. Cancer 4, 806–813. doi:10.1038/nrc1456
Hetz, C., Zhang, K., and Kaufman, R. J. (2020). Mechanisms, regulation and functions of the unfolded protein response. Nat. Rev. Mol. Cell Biol. 21, 421–438. doi:10.1038/s41580-020-0250-z
Hodge, J. W., Garnett, C. T., Farsaci, B., Palena, C., Tsang, K. Y., Ferrone, S., et al. (2013). Chemotherapy-induced immunogenic modulation of tumor cells enhances killing by cytotoxic T lymphocytes and is distinct from immunogenic cell death. Int. J. Cancer 133, 624–636. doi:10.1002/ijc.28070
Hong, X., Dong, T., Yi, T., Hu, J., Zhang, Z., Lin, S., et al. (2018). Impact of 5-Fu/oxaliplatin on mouse dendritic cells and synergetic effect with a colon cancer vaccine. Chin. J. Cancer Res. 30, 197–208. doi:10.21147/j.issn.1000-9604.2018.02.03
Horn, L., Mansfield, A. S., Szczesna, A., Havel, L., Krzakowski, M., Hochmair, M. J., et al. (2018). First-line atezolizumab plus chemotherapy in extensive-stage small-cell lung cancer. N. Engl. J. Med. 379, 2220–2229. doi:10.1056/NEJMoa1809064
Ikegawa, S., and Matsuoka, K. I. (2021). Harnessing Treg homeostasis to optimize posttransplant immunity: Current concepts and future perspectives. Front. Immunol. 12, 713358. doi:10.3389/fimmu.2021.713358
Jain, R. K., Tong, R. T., and Munn, L. L. (2007). Effect of vascular normalization by antiangiogenic therapy on interstitial hypertension, peritumor edema, and lymphatic metastasis: Insights from a mathematical model. Cancer Res. 67, 2729–2735. doi:10.1158/0008-5472.CAN-06-4102
Kanterman, J., Sade-Feldman, M., Biton, M., Ish-Shalom, E., Lasry, A., Goldshtein, A., et al. (2014). Adverse immunoregulatory effects of 5FU and CPT11 chemotherapy on myeloid-derived suppressor cells and colorectal cancer outcomes. Cancer Res. 74, 6022–6035. doi:10.1158/0008-5472.CAN-14-0657
Karagiannis, G. S., Pastoriza, J. M., Wang, Y., Harney, A. S., Entenberg, D., Pignatelli, J., et al. (2017). Neoadjuvant chemotherapy induces breast cancer metastasis through a TMEM-mediated mechanism. Sci. Transl. Med. 9, eaan0026. doi:10.1126/scitranslmed.aan0026
Karapanagiotou, E. M., Roulstone, V., Twigger, K., Ball, M., Tanay, M., Nutting, C., et al. (2012). Phase I/II trial of carboplatin and paclitaxel chemotherapy in combination with intravenous oncolytic reovirus in patients with advanced malignancies. Clin. Cancer Res. 18, 2080–2089. doi:10.1158/1078-0432.CCR-11-2181
Khuri, F. R., Nemunaitis, J., Ganly, I., Arseneau, J., Tannock, I. F., Romel, L., et al. (2000). A controlled trial of intratumoral ONYX-015, a selectively-replicating adenovirus, in combination with cisplatin and 5-fluorouracil in patients with recurrent head and neck cancer. Nat. Med. 6, 879–885. doi:10.1038/78638
Kim, N. R., and Kim, Y. J. (2019). Oxaliplatin regulates myeloid-derived suppressor cell-mediated immunosuppression via downregulation of nuclear factor-κB signaling. Cancer Med. 8, 276–288. doi:10.1002/cam4.1878
Kohli, E., Causse, S., Baverel, V., Dubrez, L., Borges-Bonan, N., Demidov, O., et al. (2021). Endoplasmic reticulum chaperones in viral infection: Therapeutic perspectives. Microbiol. Mol. Biol. Rev. 85, e0003521. doi:10.1128/MMBR.00035-21
Koo, A. N., Min, K. H., Lee, H. J., Lee, S. U., Kim, K., Kwon, I. C., et al. (2012). Tumor accumulation and antitumor efficacy of docetaxel-loaded core-shell-corona micelles with shell-specific redox-responsive cross-links. Biomaterials 33, 1489–1499. doi:10.1016/j.biomaterials.2011.11.013
Kopecka, J., Salaroglio, I. C., Righi, L., Libener, R., Orecchia, S., Grosso, F., et al. (2018). Loss of C/EBP-beta LIP drives cisplatin resistance in malignant pleural mesothelioma. Lung Cancer 120, 34–45. doi:10.1016/j.lungcan.2018.03.022
Kroemer, G., Galassi, C., Zitvogel, L., and Galluzzi, L. (2022). Immunogenic cell stress and death. Nat. Immunol. 23, 487–500. doi:10.1038/s41590-022-01132-2
Kuryk, L., Haavisto, E., Garofalo, M., Capasso, C., Hirvinen, M., Pesonen, S., et al. (2016). Synergistic anti-tumor efficacy of immunogenic adenovirus ONCOS-102 (Ad5/3-D24-GM-CSF) and standard of care chemotherapy in preclinical mesothelioma model. Int. J. Cancer 139, 1883–1893. doi:10.1002/ijc.30228
Kwon, M., Nam, G. H., Jung, H., Kim, S. A., Kim, S., Choi, Y., et al. (2021). Statin in combination with cisplatin makes favorable tumor-immune microenvironment for immunotherapy of head and neck squamous cell carcinoma. Cancer Lett. 522, 198–210. doi:10.1016/j.canlet.2021.09.029
Laheurte, C., Thiery-Vuillemin, A., Calcagno, F., Legros, A., Simonin, H., Boullerot, L., et al. (2020). Metronomic cyclophosphamide induces regulatory T cells depletion and PSA-specific T cells reactivation in patients with biochemical recurrent prostate cancer. Int. J. Cancer 147, 1199–1205. doi:10.1002/ijc.32803
Lai, V., Neshat, S. Y., Rakoski, A., Pitingolo, J., and Doloff, J. C. (2021). Drug delivery strategies in maximizing anti-angiogenesis and anti-tumor immunity. Adv. Drug Deliv. Rev. 179, 113920. doi:10.1016/j.addr.2021.113920
Leonetti, A., Wever, B., Mazzaschi, G., Assaraf, Y. G., Rolfo, C., Quaini, F., et al. (2019). Molecular basis and rationale for combining immune checkpoint inhibitors with chemotherapy in non-small cell lung cancer. Drug resist. updat. 46, 100644. doi:10.1016/j.drup.2019.100644
Li, C., Sun, H., Wei, W., Liu, Q., Wang, Y., Zhang, Y., et al. (2020). Mitoxantrone triggers immunogenic prostate cancer cell death via p53-dependent PERK expression. Cell. Oncol. 43, 1099–1116. doi:10.1007/s13402-020-00544-2
Li, D., Schaub, N., Guerin, T. M., Bapiro, T. E., Richards, F. M., Chen, V., et al. (2021). T cell-mediated antitumor immunity cooperatively induced by TGFβR1 antagonism and gemcitabine counteracts reformation of the stromal barrier in pancreatic cancer. Mol. Cancer Ther. 20, 1926–1940. doi:10.1158/1535-7163.MCT-20-0620
Li, J. Y., Duan, X. F., Wang, L. P., Xu, Y. J., Huang, L., Zhang, T. F., et al. (2014). Selective depletion of regulatory T cell subsets by docetaxel treatment in patients with nonsmall cell lung cancer. J. Immunol. Res. 2014, 286170. doi:10.1155/2014/286170
Li, R., Mukherjee, M. B., and Lin, J. (2022). Coordinated Regulation of Myeloid-Derived Suppressor Cells by Cytokines and Chemokines, 14. doi:10.3390/cancers14051236Cancers (Basel)
Li, T., Wu, B., Yang, T., Zhang, L., and Jin, K. (2020). The outstanding antitumor capacity of CD4(+) T helper lymphocytes. Biochim. Biophys. Acta. Rev. Cancer 1874, 188439. doi:10.1016/j.bbcan.2020.188439
Machiels, J. P., Reilly, R. T., Emens, L. A., Ercolini, A. M., Lei, R. Y., Weintraub, D., et al. (2001). Cyclophosphamide, doxorubicin, and paclitaxel enhance the antitumor immune response of granulocyte/macrophage-colony stimulating factor-secreting whole-cell vaccines in HER-2/neu tolerized mice. Cancer Res. 61, 3689–3697.
Maeda, K., Hazama, S., Tokuno, K., Kan, S., Maeda, Y., Watanabe, Y., et al. (2011). Impact of chemotherapy for colorectal cancer on regulatory T-cells and tumor immunity. Anticancer Res. 31, 4569–4574.
Markasz, L., Stuber, G., Vanherberghen, B., Flaberg, E., Olah, E., Carbone, E., et al. (2007). Effect of frequently used chemotherapeutic drugs on the cytotoxic activity of human natural killer cells. Mol. Cancer Ther. 6, 644–654. doi:10.1158/1535-7163.MCT-06-0358
Martin, N. T., and Bell, J. C. (2018). Oncolytic virus combination therapy: Killing one bird with two stones. Mol. Ther. 26, 1414–1422. doi:10.1016/j.ymthe.2018.04.001
Martins, I., Kepp, O., Schlemmer, F., Adjemian, S., Tailler, M., Shen, S., et al. (2011). Restoration of the immunogenicity of cisplatin-induced cancer cell death by endoplasmic reticulum stress. Oncogene 30, 1147–1158. doi:10.1038/onc.2010.500
Martins, I., Michaud, M., Sukkurwala, A. Q., Adjemian, S., Ma, Y., Shen, S., et al. (2012). Premortem autophagy determines the immunogenicity of chemotherapy-induced cancer cell death. Autophagy 8, 413–415. doi:10.4161/auto.19009
McDonnell, A. M., Joost Lesterhuis, W., Khong, A., Nowak, A. K., Lake, R. A., Currie, A. J., et al. (2015a). Restoration of defective cross-presentation in tumors by gemcitabine. Oncoimmunology 4, e1005501. doi:10.1080/2162402X.2015.1005501
McDonnell, A. M., Lesterhuis, W. J., Khong, A., Nowak, A. K., Lake, R. A., Currie, A. J., et al. (2015b). Tumor-infiltrating dendritic cells exhibit defective cross-presentation of tumor antigens, but is reversed by chemotherapy. Eur. J. Immunol. 45, 49–59. doi:10.1002/eji.201444722
Medzhitov, R., and Janeway, C. A. (2002). Decoding the patterns of self and nonself by the innate immune system. Science 296, 298–300. doi:10.1126/science.1068883
Menger, L., Vacchelli, E., Adjemian, S., Martins, I., Ma, Y., Shen, S., et al. (2012). Cardiac glycosides exert anticancer effects by inducing immunogenic cell death. Sci. Transl. Med. 4, 143ra99. doi:10.1126/scitranslmed.3003807
Michaud, M., Sukkurwala, A. Q., Di Sano, F., Zitvogel, L., Kepp, O., and Kroemer, G. (2014). Synthetic induction of immunogenic cell death by genetic stimulation of endoplasmic reticulum stress. Oncoimmunology 3, e28276. doi:10.4161/onci.28276
Minute, L., Teijeira, A., Sanchez-Paulete, A. R., Ochoa, M. C., Alvarez, M., Otano, I., et al. (2020). Cellular cytotoxicity is a form of immunogenic cell death. J. Immunother. Cancer 8, e000325. doi:10.1136/jitc-2019-000325
Montes de Oca, R., Alavi, A. S., Vitali, N., Bhattacharya, S., Blackwell, C., Patel, K., et al. (2021). Belantamab mafodotin (GSK2857916) drives immunogenic cell death and immune-mediated antitumor responses in vivo. Mol. Cancer Ther. 20, 1941–1955. doi:10.1158/1535-7163.MCT-21-0035
Moschella, F., Valentini, M., Arico, E., Macchia, I., Sestili, P., D'Urso, M. T., et al. (2011). Unraveling cancer chemoimmunotherapy mechanisms by gene and protein expression profiling of responses to cyclophosphamide. Cancer Res. 71, 3528–3539. doi:10.1158/0008-5472.CAN-10-4523
Muller, P., Kreuzaler, M., Khan, T., Thommen, D. S., Martin, K., Glatz, K., et al. (2015). Trastuzumab emtansine (T-DM1) renders HER2+ breast cancer highly susceptible to CTLA-4/PD-1 blockade. Sci. Transl. Med. 7, 315ra188. doi:10.1126/scitranslmed.aac4925
Muller, P., Martin, K., Theurich, S., Schreiner, J., Savic, S., Terszowski, G., et al. (2014a). Microtubule-depolymerizing agents used in antibody-drug conjugates induce antitumor immunity by stimulation of dendritic cells. Cancer Immunol. Res. 2, 741–755. doi:10.1158/2326-6066.CIR-13-0198
Muller, P., Martin, K., Theurich, S., von Bergwelt-Baildon, M., and Zippelius, A. (2014b). Cancer chemotherapy agents target intratumoral dendritic cells to potentiate antitumor immunity. Oncoimmunology 3, e954460. doi:10.4161/21624011.2014.954460
Munn, D. H., and Mellor, A. L. (2016). Ido in the tumor microenvironment: Inflammation, counter-regulation, and tolerance. Trends Immunol. 37, 193–207. doi:10.1016/j.it.2016.01.002
Murphy, T. L., and Murphy, K. M. (2022). Dendritic cells in cancer immunology. Cell. Mol. Immunol. 19, 3–13. doi:10.1038/s41423-021-00741-5
Nakahara, T., Uchi, H., Lesokhin, A. M., Avogadri, F., Rizzuto, G. A., Hirschhorn-Cymerman, D., et al. (2010). Cyclophosphamide enhances immunity by modulating the balance of dendritic cell subsets in lymphoid organs. Blood 115, 4384–4392. doi:10.1182/blood-2009-11-251231
Ng, H. Y., Li, J., Tao, L., Lam, A. K., Chan, K. W., Ko, J. M. Y., et al. (2018). Chemotherapeutic treatments increase PD-L1 expression in esophageal squamous cell carcinoma through EGFR/ERK activation. Transl. Oncol. 11, 1323–1333. doi:10.1016/j.tranon.2018.08.005
Nishikawa, H., and Koyama, S. (2021). Mechanisms of regulatory T cell infiltration in tumors: Implications for innovative immune precision therapies. J. Immunother. Cancer 9, e002591. doi:10.1136/jitc-2021-002591
Paijens, S. T., Vledder, A., de Bruyn, M., and Nijman, H. W. (2021). Tumor-infiltrating lymphocytes in the immunotherapy era. Cell. Mol. Immunol. 18, 842–859. doi:10.1038/s41423-020-00565-9
Park, Y. H., Lal, S., Lee, J. E., Choi, Y. L., Wen, J., Ram, S., et al. (2020). Chemotherapy induces dynamic immune responses in breast cancers that impact treatment outcome. Nat. Commun. 11, 6175. doi:10.1038/s41467-020-19933-0
Pfannenstiel, L. W., Lam, S. S., Emens, L. A., Jaffee, E. M., and Armstrong, T. D. (2010). Paclitaxel enhances early dendritic cell maturation and function through TLR4 signaling in mice. Cell. Immunol. 263, 79–87. doi:10.1016/j.cellimm.2010.03.001
Pfirschke, C., Engblom, C., Rickelt, S., Cortez-Retamozo, V., Garris, C., Pucci, F., et al. (2016). Immunogenic chemotherapy sensitizes tumors to checkpoint blockade therapy. Immunity 44, 343–354. doi:10.1016/j.immuni.2015.11.024
Principe, D. R., Kamath, S. D., Korc, M., and Munshi, H. G. (2022). The immune modifying effects of chemotherapy and advances in chemo-immunotherapy. Pharmacol. Ther. 236, 108111. doi:10.1016/j.pharmthera.2022.108111
Radogna, F., and Diederich, M. (2018). Stress-induced cellular responses in immunogenic cell death: Implications for cancer immunotherapy. Biochem. Pharmacol. 153, 12–23. doi:10.1016/j.bcp.2018.02.006
Ranki, T., Pesonen, S., Hemminki, A., Partanen, K., Kairemo, K., Alanko, T., et al. (2016). Phase I study with ONCOS-102 for the treatment of solid tumors - an evaluation of clinical response and exploratory analyses of immune markers. J. Immunother. Cancer 4, 17. doi:10.1186/s40425-016-0121-5
Roselli, M., Cereda, V., di Bari, M. G., Formica, V., Spila, A., Jochems, C., et al. (2013). Effects of conventional therapeutic interventions on the number and function of regulatory T cells. Oncoimmunology 2, e27025. doi:10.4161/onci.27025
Saeed, M., Gao, J., Shi, Y., Lammers, T., and Yu, H. (2019). Engineering nanoparticles to reprogram the tumor immune microenvironment for improved cancer immunotherapy. Theranostics 9, 7981–8000. doi:10.7150/thno.37568
Saleh, R., and Elkord, E. (2019). Treg-mediated acquired resistance to immune checkpoint inhibitors. Cancer Lett. 457, 168–179. doi:10.1016/j.canlet.2019.05.003
Schmid, P., Adams, S., Rugo, H. S., Schneeweiss, A., Barrios, C. H., Iwata, H., et al. (2018). Atezolizumab and nab-paclitaxel in advanced triple-negative breast cancer. N. Engl. J. Med. 379, 2108–2121. doi:10.1056/NEJMoa1809615
Shurin, G. V., Tourkova, I. L., Kaneno, R., and Shurin, M. R. (2009). Chemotherapeutic agents in noncytotoxic concentrations increase antigen presentation by dendritic cells via an IL-12-dependent mechanism. J. Immunol. 183, 137–144. doi:10.4049/jimmunol.0900734
Sindhwani, S., Syed, A. M., Ngai, J., Kingston, B. R., Maiorino, L., Rothschild, J., et al. (2020). The entry of nanoparticles into solid tumours. Nat. Mat. 19, 566–575. doi:10.1038/s41563-019-0566-2
Spehner, L., Kim, S., Vienot, A., Francois, E., Buecher, B., Adotevi, O., et al. (2020). Anti-telomerase CD4(+) Th1 immunity and monocytic-myeloid-derived-suppressor cells are associated with long-term efficacy achieved by docetaxel, cisplatin, and 5-fluorouracil (DCF) in advanced anal squamous cell carcinoma: Translational study of epitopes-HPV01 and 02 trials. Int. J. Mol. Sci. 21, E6838. doi:10.3390/ijms21186838
Tang, T., Huang, X., Zhang, G., Hong, Z., Bai, X., and Liang, T. (2021). Advantages of targeting the tumor immune microenvironment over blocking immune checkpoint in cancer immunotherapy. Signal Transduct. Target. Ther. 6, 72. doi:10.1038/s41392-020-00449-4
Tel, J., Hato, S. V., Torensma, R., Buschow, S. I., Figdor, C. G., Lesterhuis, W. J., et al. (2012). The chemotherapeutic drug oxaliplatin differentially affects blood DC function dependent on environmental cues. Cancer Immunol. Immunother. 61, 1101–1111. doi:10.1007/s00262-011-1189-x
Tesniere, A., Schlemmer, F., Boige, V., Kepp, O., Martins, I., Ghiringhelli, F., et al. (2010). Immunogenic death of colon cancer cells treated with oxaliplatin. Oncogene 29, 482–491. doi:10.1038/onc.2009.356
Theurich, S., Malcher, J., Wennhold, K., Shimabukuro-Vornhagen, A., Chemnitz, J., Holtick, U., et al. (2013). Brentuximab vedotin combined with donor lymphocyte infusions for early relapse of Hodgkin lymphoma after allogeneic stem-cell transplantation induces tumor-specific immunity and sustained clinical remission. J. Clin. Oncol. 31, e59–e63. doi:10.1200/JCO.2012.43.6832
Tie, Y., Tang, F., Wei, Y. Q., and Wei, X. W. (2022). Immunosuppressive cells in cancer: Mechanisms and potential therapeutic targets. J. Hematol. Oncol. 15, 61. doi:10.1186/s13045-022-01282-8
Tsuda, N., Chang, D. Z., Mine, T., Efferson, C., Garcia-Sastre, A., Wang, X., et al. (2007). Taxol increases the amount and T cell activating ability of self-immune stimulatory multimolecular complexes found in ovarian cancer cells. Cancer Res. 67, 8378–8387. doi:10.1158/0008-5472.CAN-07-0327
Van Wigcheren, G. F., De Haas, N., Mulder, T. A., Horrevorts, S. K., Bloemendal, M., Hins-Debree, S., et al. (2021). Cisplatin inhibits frequency and suppressive activity of monocytic myeloid-derived suppressor cells in cancer patients. Oncoimmunology 10, 1935557. doi:10.1080/2162402X.2021.1935557
Vincent, J., Mignot, G., Chalmin, F., Ladoire, S., Bruchard, M., Chevriaux, A., et al. (2010). 5-Fluorouracil selectively kills tumor-associated myeloid-derived suppressor cells resulting in enhanced T cell-dependent antitumor immunity. Cancer Res. 70, 3052–3061. doi:10.1158/0008-5472.CAN-09-3690
Wanderley, C. W., Colon, D. F., Luiz, J. P. M., Oliveira, F. F., Viacava, P. R., Leite, C. A., et al. (2018). Paclitaxel reduces tumor growth by reprogramming tumor-associated macrophages to an M1 profile in a TLR4-dependent manner. Cancer Res. 78, 5891–5900. doi:10.1158/0008-5472.CAN-17-3480
Wang, C., Wang, J., Zhang, X., Yu, S., Wen, D., Hu, Q., et al. (2018). In situ formed reactive oxygen species-responsive scaffold with gemcitabine and checkpoint inhibitor for combination therapy. Sci. Transl. Med. 10, eaan3682. doi:10.1126/scitranslmed.aan3682
Wang, W., Jin, Y., Xu, Z., Liu, X., Bajwa, S. Z., Khan, W. S., et al. (2020a). Stimuli-activatable nanomedicines for chemodynamic therapy of cancer. Wiley Interdiscip. Rev. Nanomed. Nanobiotechnol. 12, e1614. doi:10.1002/wnan.1614
Wang, Y., Xie, W., Humeau, J., Chen, G., Liu, P., Pol, J., et al. (2020b). Autophagy induction by thiostrepton improves the efficacy of immunogenic chemotherapy. J. Immunother. Cancer 8, e000462. doi:10.1136/jitc-2019-000462
Weber, J. S., and Mule, J. J. (2015). Cancer immunotherapy meets biomaterials. Nat. Biotechnol. 33, 44–45. doi:10.1038/nbt.3119
Williams, K. M., Hakim, F. T., and Gress, R. E. (2007). T cell immune reconstitution following lymphodepletion. Semin. Immunol. 19, 318–330. doi:10.1016/j.smim.2007.10.004
Workenhe, S. T., Pol, J. G., Lichty, B. D., Cummings, D. T., and Mossman, K. L. (2013). Combining oncolytic HSV-1 with immunogenic cell death-inducing drug mitoxantrone breaks cancer immune tolerance and improves therapeutic efficacy. Cancer Immunol. Res. 1, 309–319. doi:10.1158/2326-6066.CIR-13-0059-T
Wu, J., and Waxman, D. J. (2018). Immunogenic chemotherapy: Dose and schedule dependence and combination with immunotherapy. Cancer Lett. 419, 210–221. doi:10.1016/j.canlet.2018.01.050
Xiang, Y., Chen, L., Li, L., and Huang, Y. (2020). Restoration and enhancement of immunogenic cell death of cisplatin by coadministration with digoxin and conjugation to HPMA copolymer. ACS Appl. Mat. Interfaces 12, 1606–1616. doi:10.1021/acsami.9b19323
Yu, B., Jiang, T., and Liu, D. (2020). BCMA-targeted immunotherapy for multiple myeloma. J. Hematol. Oncol. 13, 125. doi:10.1186/s13045-020-00962-7
Zammarchi, F., Havenith, K., Bertelli, F., Vijayakrishnan, B., Chivers, S., and van Berkel, P. H. (2020). CD25-targeted antibody-drug conjugate depletes regulatory T cells and eliminates established syngeneic tumors via antitumor immunity. J. Immunother. Cancer 8, e000860. doi:10.1136/jitc-2020-000860
Zhang, J., Pan, S., Jian, C., Hao, L., Dong, J., Sun, Q., et al. (2021). Immunostimulatory properties of chemotherapy in breast cancer: From immunogenic modulation mechanisms to clinical practice. Front. Immunol. 12, 819405. doi:10.3389/fimmu.2021.819405
Zhao, C., Bartock, M., Jia, B., Shah, N., Claxton, D. F., Wirk, B., et al. (2022). Post-transplant cyclophosphamide alters immune signatures and leads to impaired T cell reconstitution in allogeneic hematopoietic stem cell transplant. J. Hematol. Oncol. 15, 64. doi:10.1186/s13045-022-01287-3
Zhou, L., Zhang, P., Wang, H., Wang, D., and Li, Y. (2020). Smart nanosized drug delivery systems inducing immunogenic cell death for combination with cancer immunotherapy. Acc. Chem. Res. 53, 1761–1772. doi:10.1021/acs.accounts.0c00254
Zhou, Y., Bastian, I. N., Long, M. D., Dow, M., Li, W., Liu, T., et al. (2021). Activation of NF-κB and p300/CBP potentiates cancer chemoimmunotherapy through induction of MHC-I antigen presentation. Proc. Natl. Acad. Sci. U. S. A. 118, e2025840118. doi:10.1073/pnas.2025840118
Zhu, Y., Yang, Z., Dong, Z., Gong, Y., Hao, Y., Tian, L., et al. (2020). CaCO3-Assisted preparation of pH-responsive immune-modulating nanoparticles for augmented chemo-immunotherapy. Nanomicro. Lett. 13, 29. doi:10.1007/s40820-020-00549-4
Zitvogel, L., Apetoh, L., Ghiringhelli, F., and Kroemer, G. (2008). Immunological aspects of cancer chemotherapy. Nat. Rev. Immunol. 8, 59–73. doi:10.1038/nri2216
Keywords: chemotherapeutic agents, combinatorial regimens, immunogenic cell death, tumor immune microenvironment, cancer therapy
Citation: Liu J, Yu Y, Liu C, Gao C, Zhuang J, Liu L, Wu Q, Ma W, Zhang Q and Sun C (2022) Combinatorial regimens of chemotherapeutic agents: A new perspective on raising the heat of the tumor immune microenvironment. Front. Pharmacol. 13:1035954. doi: 10.3389/fphar.2022.1035954
Received: 03 September 2022; Accepted: 29 September 2022;
Published: 11 October 2022.
Edited by:
Dongdong Sun, Nanjing University of Chinese Medicine, ChinaCopyright © 2022 Liu, Yu, Liu, Gao, Zhuang, Liu, Wu, Ma, Zhang and Sun. This is an open-access article distributed under the terms of the Creative Commons Attribution License (CC BY). The use, distribution or reproduction in other forums is permitted, provided the original author(s) and the copyright owner(s) are credited and that the original publication in this journal is cited, in accordance with accepted academic practice. No use, distribution or reproduction is permitted which does not comply with these terms.
*Correspondence: Qiming Zhang, zhang_917@126.com; Changgang Sun, scgdoctor@126.com
†These authors have contributed equally to this work and share first authorship