- 1The Department of Thoracic Surgery, The First Affiliated Hospital of Xi’an Jiaotong University, Xi’an, Shaanxi, China
- 2Department of Dermatology, The Second Affiliated Hospital of Xi’an Jiaotong University, Xi’an, Shaanxi, China
- 3Department of Biophysics, School of Basic Medical Sciences, Xi’an Jiaotong University, Xi’an, Shaanxi, China
Lung cancer is the most common cause of cancer-related deaths worldwide. More efficient treatments are desperately needed. For decades, the success of platinum-based anticancer drugs has promoted the exploration of metal-based agents. Four ruthenium-based complexes have also entered clinical trials as candidates of anticancer metallodrugs. However, systemic toxicity, severe side effects and drug-resistance impeded their applications and efficacy. Stimuli-responsiveness of Pt- and Ru-based complexes provide a great chance to weaken the side effects and strengthen the clinical efficacy in drug design. This review provides an overview on the stimuli-responsive Pt- and Ru-based metallic anticancer drugs for lung cancer. They are categorized as endo-stimuli-responsive, exo-stimuli-responsive, and dual-stimuli-responsive prodrugs based on the nature of stimuli. We describe various representative examples of structure, response mechanism, and potential medical applications in lung cancer. In the end, we discuss the future opportunities and challenges in this field.
1 Introduction
Lung cancer (LC) is the most common malignancy and the top leading cause of cancer-related death worldwide (Govindan et al., 2006; Sung et al., 2021). Due to the lack of early clinical symptoms, most patients with LC are diagnosed at an advanced stage, resulting in the loss of opportunity for surgical treatment (Wang et al., 2015a). Platinum(II)-based chemotherapy is one of the pillars of clinical treatment for advanced LC (Chang, 2011; Pirker, 2020). The success of platinum-based anticancer drugs sparks interest in other metal-based anticancer drugs. Despite the dominance of small organic molecules and bio-derived compounds in the pharmaceutical market, metallic drugs continue to attract attention for their unique properties. In addition, four ruthenium complexes by far have successfully entered clinical trials and exhibited inspiring therapeutic effect on lung cancer metastasis.
Despite the great success of cisplatin in clinical usages, it still has some drawbacks, including significant side effects due to systemic toxicity, primary and acquired resistance, and low bioavailability for various reasons (Lippard, 1982; Wang and Lippard, 2005; Wang, 2010; Wang and Guo, 2013). Therefore, the development of metallic drugs urgently requires new strategies that can address these issues in a targeted manner. Prodrugs may be a potential strategy that can address the current metallic drugs dilemma. Prodrugs are inactive derivatives of a drug that are biotransformed in vivo to produce an active form for targeted drug delivery and therapy (Rautio et al., 2008; Bildstein et al., 2011; Redasani and Bari, 2015). To date, prodrugs have been at the forefront of improving the targeting and bioavailability of drugs and improving their physiochemical, pharmaceutical, and pharmacokinetic properties (Kratz et al., 2008; Mazzaferro et al., 2013; Walther et al., 2017).
Analog to metal complexes-based anticancer drugs, Pt- and Ru-based prodrugs hold advantages in diversity that is not usually available with organic molecules. First, they maintain a three-dimensional structure with square-planar or octahedral conformation (Hambley, 2007a). The different geometries confer different biological activities to the complexes. Second, bioactive ligands can be easily linked with the metal ions via coordination or modification of chelated ligands, and exhibit new pharmacological properties (Bruijnincx and Sadler, 2008). Third, Pt- or Ru-based complexes that undergo ligand exchange offer unique action for killing cancer cells. As a presentative example, cisplatin undergoes activation through hydrolysis inside cells to bind to DNA, suppressing DNA replication (Ghosh, 2019). Ligand exchange reaction of metal complexes bearing bioactive molecules as coordinated ligands is also exploited for delivery purpose. In addition, rich photochemical and photophysical properties of Pt- or Ru-based complexes are beneficial in designing “smart” prodrugs with stimuli-responsive characteristics, and expanding their utility beyond therapeutic agents (Heffern et al., 2014; Ma et al., 2014). Therefore, the diversity and variability of molecular structures provide a broad platform for the design of responsive prodrugs based on Pt- and Ru-complexes.
One of the most important aspects of prodrug activation is the appropriate trigger (Zhang et al., 2017; Gu et al., 2018), which can be divided into two main categories: endogenous and exogenous stimuli (Chen et al., 2016a; Liu et al., 2016; Yang et al., 2016; Brun et al., 2017; Hu et al., 2017). Endogenous stimuli include redox gradients, pH values, enzyme concentrations, hormone levels, glucose concentrations, etc., usually related to pathological characteristics. Exogenous stimuli, such as light, temperature, magnetic fields, high-energy radiation, and ultrasound, remotely control prodrug activation by means outside the organism. In addition to the single triggers mentioned above, different triggers can act synergistically with each other to accomplish the activation of the drug. Therefore, the structural characteristics of the parent drug and its function in response to stimuli are prerequisites for the action of the prodrug. For metal drugs, the versatility of molecular structure and function makes them more likely to be qualified stimulus-responsive prodrugs (Van Rijt and Sadler, 2009; Schatzschneider, 2010; Graf and Lippard, 2012). Some recent studies have also shown the potential of some stimulus-responsive metal drugs to enhance pharmacological effects and reduce side effects, for example, some inactive complexes of metal ions in the oxidized state can be reductively activated in the reducing environment of the pathological state and transformed into the active form to exert their pharmacological effects (Van Rijt and Sadler, 2009; Romero-Canelon and Sadler, 2013).
Due to the great success of Pt- and Ru-based metallodrugs in clinical usages or in clinic trials, in this review we aim to summarize and analyze the representative stimulant-responsive Pt- and Ru-based agents in lung cancer therapy. These metallic anticancer agents are classified into three categories: endo-stimuli-responsive drugs, exo-stimuli-responsive drugs, and multi-stimuli-responsive drugs. The different stimulatory modalities are described in terms of design strategies, molecular structures, mechanisms of action and corresponding clinical applications. Finally, we discuss the future opportunities and challenges in this field.
2 Endo-stimuli-responsive Pt- and Ru-based anticancer drugs
Tumor development is a dynamic process involving a continuous interaction between tumor cells and the tumor microenvironment (Jin and Jin, 2020). Compared with normal tissues, tumor tissues have unique pathophysiological hallmarks, such as hypoxia, reducing environment, low pH, elevated reactive oxygen species (ROS) levels, elevated intracellular glutathione (GSH) levels, and abnormal expression of specific enzymes (Jin and Jin, 2020). These unique factors can act as endogenous triggers of the prodrug, specifically triggering the conversion of the prodrug and exerting its pharmacological effects (Mura et al., 2013; Gulzar et al., 2015). A large number of endo-stimuli-responsive metallic drugs triggered by redox, pH, and specific enzymes have been developed for these abnormal factors (Van Rijt and Sadler, 2009; Schatzschneider, 2010; Scaffidi-Domianello et al., 2011; Graf and Lippard, 2012; Ding et al., 2014). In this section, these metallic drugs are outlined according to different microenvironmental factors.
2.1 Redox-responsive
The difference in redox potential between tumor tissue and normal tissue is widely recognized, and this difference can serve as a trigger for redox-responsive prodrugs (Schafer and Buettner, 2001; Brun et al., 2017). For example, glutathione (GSH) is an important intracellular reducing agent. GSH/GSH disulfide (GSSG), the major redox pair in mammalian cells, is mainly involved in the maintenance of thiol-containing enzyme stability, maintenance of cell membrane integrity and avoidance of ROS-induced damage. The intracellular GSH concentration is about 2–10 mM, while the concentration of GSH in body fluids is lower (2–20 μM) (Hong et al., 2006; Cheng et al., 2011a). The high intracellular GSH concentration maintains a highly reductive intracellular environment. Moreover, the rapid proliferation of tumor cells resulted in a more than four times higher GSH content in tumor tissues than in normal tissues, creating a stronger reductive environment (Saito et al., 2003). The strongly reducing environment can trigger redox-sensitive prodrugs to release their active forms inside tumor cells to exert their pharmacological effects. In addition to GSH, some other bioreductants such as ascorbate can also act as triggers to activate redox-responsive drugs.
Metal ions such as Pt, Ru, Co and Fe have multiple oxidation states that can be regulated by ligands so that they can be activated in the reducing environment in the pathological state and thus release the active form of the drug. Thus, the redox reactivity of metal complexes has become one of the most successful classes of stimuli-responsive metal drugs designed.
2.1.1 Platinum(IV) prodrugs
Cisplatin (1) (Figure 1), the most successful metallic anti-cancer drug, is routinely used worldwide for the treatment of many types of cancer (Mjos and Orvig, 2014). However, classical Pt(II) drugs also suffer from severe side effects, drug resistance and systemic toxicity (Hambley, 2007b; Klein and Hambley, 2009). Pt(IV) complexes are believed to overcome the defects of classical Pt(II) drugs as prodrugs (Bruijnincx and Sadler, 2008). The hexa-ligand octahedral conformation of Pt(IV) is kinetically inert, which reduces off-target effects prior to binding to DNA and thus reduces systemic toxicity (Hall and Hambley, 2002). Pt(IV) prodrugs can be reduced to the Pt(II) form by the elimination of two axial ligands by the reducing agent, which is activated at the tumor site (Hall et al., 2007; Venkatesh and Sadler, 2018). Therefore, the redox potential at the tumor site is an important factor affecting the efficiency of Pt(IV) complex activation. In addition, the alteration of the two axial ligands can regulate the reduction potential and cytotoxicity of Pt(IV) complexes, and also provide targeting functions (Ding and Bierbach, 2015; Basu et al., 2016). Based on this, Pt(IV) complexes can be classified into three categories according to the nature of the ligands: 1) axial ligands lacking biological activity, such as chloride, hydroxide, etc.; 2) axial ligands with biological activity, such as enzyme inhibitors, etc.; 3) axial positions connected to other targeting carriers.
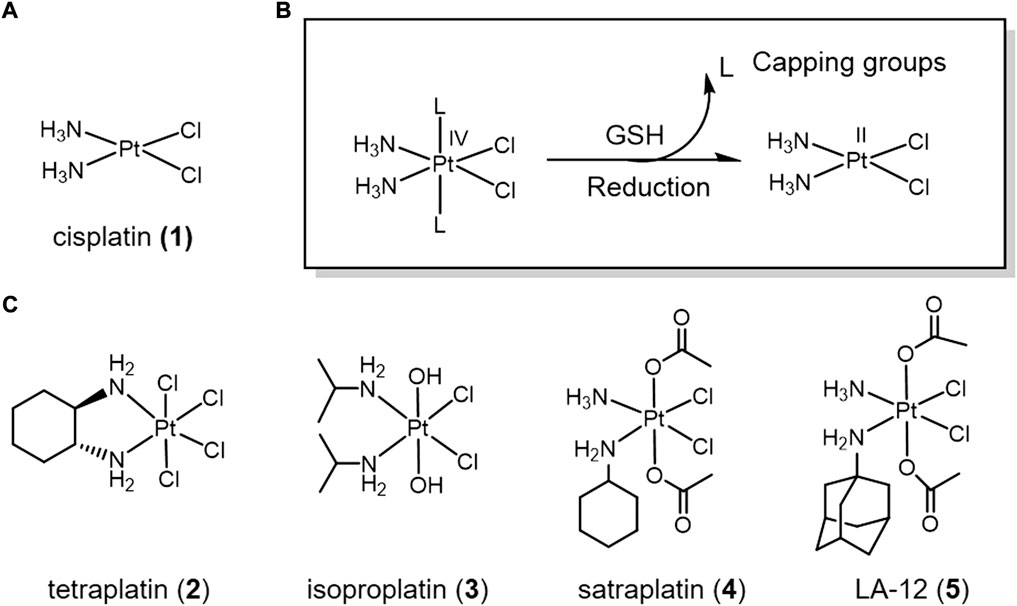
FIGURE 1. (A) Chemical structure of cis-platin. (B) Action mechanism of redox-responsive Pt(IV) prodrugs undergoing GSH reducible activation to form active Pt(II) drugs. (C) Pt(IV) prodrugs that have entered clinical trials.
A series of positive results have been achieved in the development of Pt(IV) complexes lacking bioactive ligands. Many Pt(IV) complexes have been reported as potential anticancer drugs. Four of these complexes even entered clinical trials, namely complexes tetraplatin (2), isoproplatin (3), satraplatin (4), and LA-12 (5) (Figure 1) (Wexselblatt and Gibson, 2012). Tetraplatin (2) was the first Pt(IV) drug to enter clinical trials (Schilder et al., 1994). However, due to severe neurotoxicity, tetraplatin was discontinued in phase I clinical trials (Tutsch et al., 1999). Because of high stability of the hydroxide axial ligands, isoproplatin (3) is relatively difficult to be reduced by bioreductants. Phase III clinical trials showed that isoproplatin did not exhibit greater anticancer activity than cisplatin or carboplatin (Anderson et al., 1988). Satraplatin (4) was the first reported oral platinum-based anticancer agent and has been tested in multiple clinical trials (Bhargava and Vaishampayan, 2009). LA-12 (5) has completed a Phase I clinical trial with positive results (Zak et al., 2004). Unfortunately, these Pt(IV) prodrugs have been abandoned for clinical approval due to low efficacy and severe side-effects.
Bioactive ligands are introduced to Pt(IV) prodrugs for seeking high anticancer efficacy in lung cancer (Figure 2). Overexpression of glutathione-S-transferase (GST) leads to cisplatin resistance (Nakajima et al., 2003). Etanercept (EA), a broad-spectrum GST inhibitor, can reverse GST-mediated cisplatin resistance (Takamatsu and Inaba, 1992). An EA-Pt (IV) complex 6 was developed based on this (Ang et al., 2005). Complex 6 inhibited GST activity in lung cancer A549 cells more strongly than EA and cisplatin alone. Complex 6 exhibited inhibitory effects on cisplatin-resistant A549 cells. Given that the long-term efficacy of EA is limited by toxicity due to diuresis and fluid imbalance (Ploemen et al., 1994), and that EA is readily transported out of the cell by specific pumps (Townsend and Tew, 2003), NBDHEX, a new GST inhibitor with stronger GST inhibition than EA and not transported by transport pumps (Ricci et al., 2005; Federici et al., 2009), was conjugated to oxoplatin via a succinic anhydride reaction to yield a new Pt(IV) complex 7 (Chen et al., 2019). Complex 7 showed stronger tumor suppression efficacy and less biotoxicity than cisplatin in animal studies.
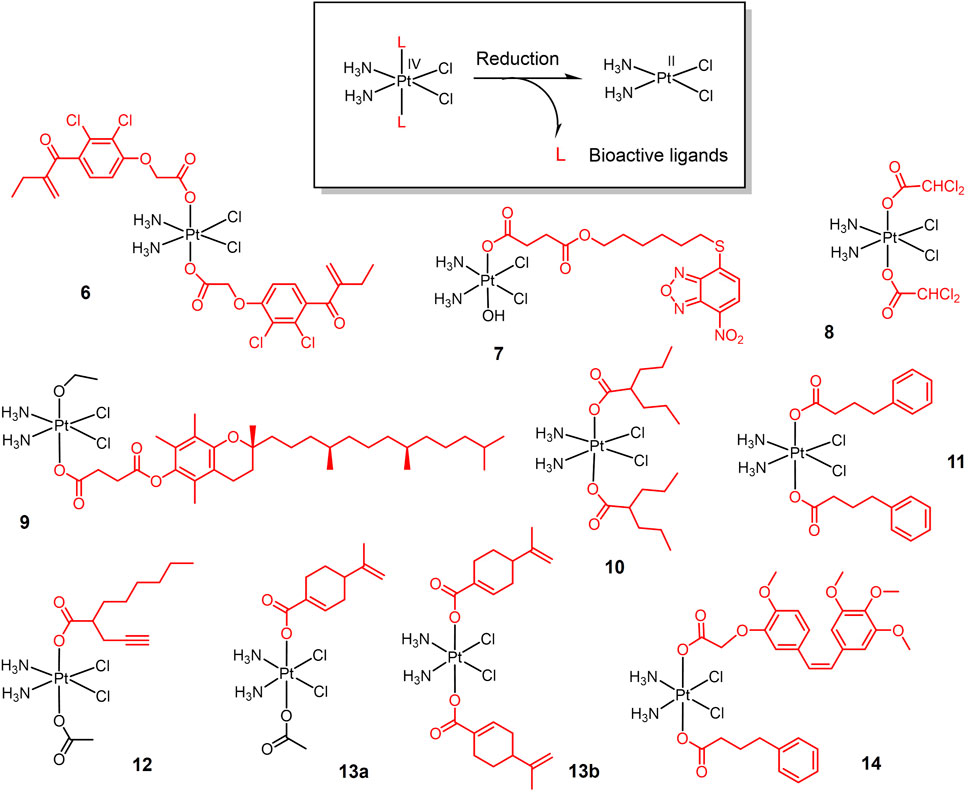
FIGURE 2. Redox-responsive Pt(IV) prodrugs undergo reduction to form active Pt(II) drugs accompanied by the release bioactive molecules. Complexes 6 and 7 bear glutathione-S-transferase inhibitors; Complex 8 bears dichloroacetate as pyruvate dehydrogenase kinase inhibitor; complex 9 bears α-TOS as inhibitor for Bcl-xL-Bax protein-protein interaction; complexes 10-12 bear histone deacetylase inhibitors (HDACi); Complexes 13a,b bear perillic acid as anti-metastatic molecule; and complex 14 bears dual bioactive ligands: phenylbutyrate as HDACi and CA4 as tubulin polymerization inhibitor, respectively.
Based on the same strategy, a new Pt(IV) complex, named mitaplatin (8) with two dichloroacetate (DCA) axial ligands was developed (Dhar and Lippard, 2009). DCA is an inhibitor of pyruvate dehydrogenase kinase (Stacpoole et al., 2003). DCA promotes the release of cytochrome c (cyt c) and nuclear translocation of apoptosis-inducing factor (AIF) by altering the mitochondrial metabolism of tumor cells, ultimately leading to apoptosis without affecting normal cells (Bonnet et al., 2007). Thus, Complex 8 shows a dual-killing mode which targets the nuclear DNA with released cisplatin and mitochondria with released DCA. Complex 8 exhibited comparable cytotoxicity to cisplatin in lung cancer cells and did not induce normal cell death at the same concentration.
Inspired by the dual killing mode of complex 8, a new dual-targeted Pt(IV) complex 9 was developed using the vitamin E analogue α-tocopheryl succinate (α-TOS) as an axial ligand (Suntharalingam et al., 2014). α-TOS disrupts Bcl-xL-Bax interactions, thereby inducing mitochondria-mediated apoptosis (Neuzil, 2003; Shiau et al., 2006). Complex 9 exhibited more potent killing ability than cisplatin against lung cancer cells. And the cellular uptake capacity of this complex was 15–20 times higher than that of cisplatin.
The combination of histone deacetylase inhibitors (HDACi) with platinum-based anticancer drugs has received much attention (Bots and Johnstone, 2009; Hrebackova et al., 2010). HDACi increases the acetylation level of histones, hinders the interaction of histones with DNA, and exposes nuclear DNA to DNA-damaging chemotherapeutic drugs (Bolden et al., 2006; Buchwald et al., 2009). The Pt(IV) complex is well suited for this combination therapy, reducing the side effects of traditional Pt(II) drugs while exert the chemosensitizing effect of HDACi. Based on the above idea, various Pt(IV) complexes with HDACi as axial ligand were developed. Valproic acid (VA)-Pt(IV) complexes (10) (Figure 2) are the first complexes of this type (Yang et al., 2012). Complex 10 showed enhanced antitumor activity in vitro and in vivo, and animal experiments confirmed the lower nephrotoxicity of complex 10 in vivo.
The initial success of HDACi complexes has prompted researchers to further explore better HADCi complexes. Complex 11 contains two phenylbutyrate (PhB) groups as HADCi and shows nearly 50-fold higher cytotoxicity than cisplatin against A549 cells (Raveendran et al., 2016). Complex 12 contains a racemic mixture of 2-(2-propynyl) octanoic acid (POA) along with an inert acetate as axial ligands, and shows a better anticancer activity in vivo on Lewis lung carcinoma (LLC)—bearing mice than cisplatin (Gabano et al., 2017).
Perillic acid (PA), an active metabolite of limonite that showed anti-metastatic effects, was used as a bioactive capping ligand for the Pt(IV) complexes 13a-b (Ravera et al., 2021). The combination of PA with cisplatin can significantly improve the efficacy of cisplatin. The complexes were stable in buffer solution and were reduced in the cytoplasmic lysate of A549 cells, decomposing cisplatin and PA to exert antitumor effects. The complexes exhibited an order of magnitude stronger cytotoxicity than cisplatin against A549 cells in multicellular spheroids model.
Combretastatin A-4 (CA4), an tubulin polymerization inhibitor that exhibits dramatically 1000-folds more cytotoxic than cisplatin, was conjugated with Pt(IV) (14). CA4 showed higher cytotoxicity than Pt(IV)-CA4 conjugates in vitro, while in vivo test showed that Pt(IV)-CA4 conjugates exhibited significantly enhanced tumor suppression and reduced acute toxicity (weight loss) in LLC-bearing mice (Schmidt et al., 2021). Pt(IV)-bioactive ligand conjugates usually exerted synergistic anticancer activity via multi-action. In case of extremely potent bioactive ligands such as CA4, however, Pt(IV) moiety merely served as a self-immolative carrier for CA4, considered as prodrug for CA4 rather than multi-action prodrug.
2.1.2 Ruthenium prodrugs
Ruthenium has a distorted octahedral geometry with II to IV oxidation states (Merlino, 2016; Zeng et al., 2017a). The potential biological activity of ruthenium complexes was first explored in the 1950s (Dwyer et al., 1952), and in the 1980s, Clark et al. reported the potential targeted anticancer activity of Ru(III) complexes (Clarke et al., 1980). Ru(III) complexes can only be activated reductively in tumor tissue under hypoxic and low pH conditions. Several ruthenium-based complexes have shown significant efficacy against lung cancer in recent decades, and some of them have demonstrated lower side effects than cisplatin.
The first ruthenium-based anti-cancer candidate in clinical trials is NAMI-A (15, seen in Figure 3) (Cocchietto et al., 2003; Bergamo and Sava, 2011; Leijen et al., 2015). NAMI-A is a reducible Ru(III) complex with a heterocyclic N-donor ligand. NAMI-A has low activity against primary tumors, but has demonstrated high activity against lung metastases in vivo. Mechanistic studies revealed that NAMI-A can be reduced by GSH or ascorbic acid under physiological conditions to form the Ru(II) active form, enhancing the anti-metastatic activity of NAMI-A, which is similar to the principle of Pt(IV) prodrug (Levina et al., 2009). Phase I/II clinical study of NAMI-A revealed that NAMI-A showed selective effect on lung metastasis of solid metastasizing tumors (Sava et al., 2003; Leijen et al., 2015). Two NAMI-A analogues, KP1019 (16) (Hartinger et al., 2008) and KP1339 (17) (Trondl et al., 2014), have also entered clinical trials.
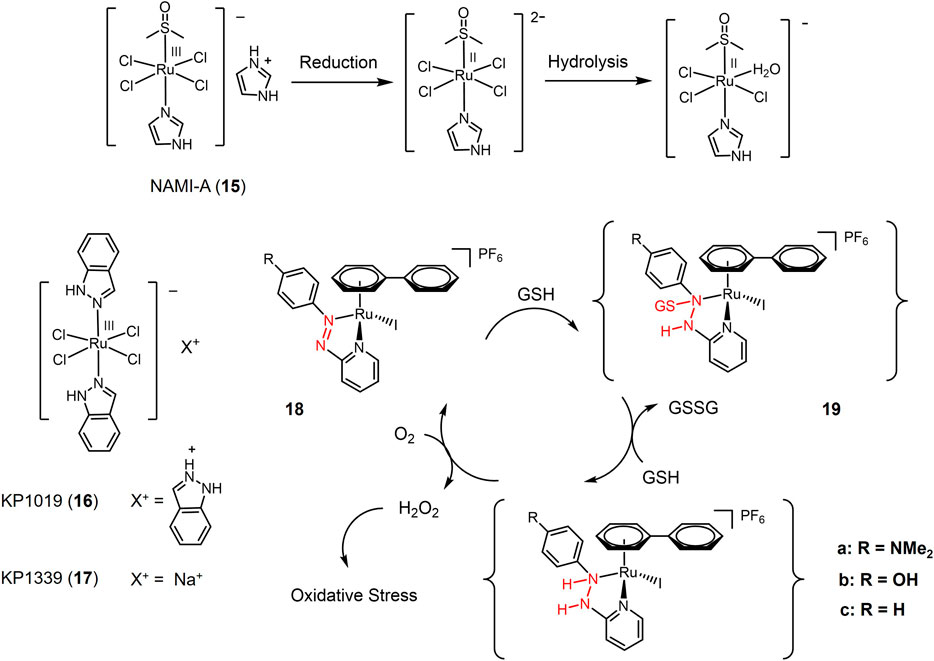
FIGURE 3. Redox-responsive Ru(III) complexes NAMI-A (15), KP1019 (16) KP1339 (17) that have entered clinical trials, and Ru(II) complexes with redox-active azole-ligand (18a-c).
In recent years, a series of Ru(II) complexes have been of interest to researchers as redox-responsive anticancer drugs. In contrast to Ru(III) complexes that are activated through Ru(III) to Ru(II) reduction (Figure 3), the Ru(II) complexes are reducibly activated through catalyzing the redox-active ligands reduction (Zhang and Sadler, 2017). Ru(II) complex 18 contained azopyridine derivatives, whose reduction potentials significantly decreased via azopyridine-Ru(II) coordination. Azo group in complex 18 was capable to be reduced by GSH forming GSH additive intermediate (19) and catalyzed a redox reaction between GSH with O2 generating high level of intracellular H2O2 that produced oxidative stress to cause cancer cell death (Dougan et al., 2008). Complex 18a bearing electron-donating substituent-NMe2 exhibited the highest cytotoxicity against A549 cells with a e value of 2 μM.
2.2 pH- responsive
The pH gradient between biological systems reflects the different acid-base environments of the body (Felber et al., 2012; Crucho, 2015). The extracellular pH of tumor tissues is usually 5.6–6.8, which is significantly lower than that of normal tissues (pH 7.4) due to altered and accelerated metabolic patterns (Gatenby and Gillies, 2004). Based on this, pH becomes a possible endogenous trigger for stimulation of reactive prodrugs. Among all pH-responsive metallodrugs, two main strategies have been adopted to design response systems: pH-labile chemical bonds and pH-sensitive nanocarriers.
A representative class of pH-sensitive platinum complexes in lung cancer therapy is the Pt(II) complex containing two chelated amino alcohol ligands (Galanski et al., 2003; Galanski et al., 2004; Scaffidi-Domianello et al., 2011). Platinum complexes with N, O chelating ligands are inert at physiological pH, but can be activated by a microacidic environment and converted to highly cytotoxic platinum complexes. As shown in Figure 4, Pt(II) complex 20 chelated with two aminoethanolate forming closed-ring at physiological pH, and exhibited low toxic effect against A549 cells with an IC50 value of 115 μM under pH 7.4. Acidic condition triggered the ring opening of complex 20, resulting in the active Pt(II) species. Under pH 6.0, complex 20 showed significant cytotoxicity against A549 cells with an IC50 value of 41.2 μM (Galanski et al., 2004). Complex 21 with a 1,3-dihydroxyacetone oxime ligand (Scaffidi-Domianello et al., 2011) performed a similar acid-activation mechanism and showed enhanced cytotoxicity against cisplatin-sensitive A549 cells in an acidic environment (pH 6.0) mimicking solid tumor.
Encapsulation of metallic drugs into pH-responsive drug delivery vehicles is another design strategy for pH-responsive drugs (Taghizadeh et al., 2015; Zhang et al., 2016; Hu et al., 2017). One possible approach is based on the strategy of oxygen donor exchange of chloride ions with carboxylate in cisplatin (Oberoi et al., 2011; Xia et al., 2011; Huang et al., 2012; Fan et al., 2015; Fu et al., 2015; Saisyo et al., 2016). This system is stable at physiological pH, and at acidic condition Pt-carboxylate bonds break, which in turn releases the active platinum drug. Based on this strategy, polyhyaluronic acid (HA) is used as a carrier for cisplatin (Figure 4). Pt(II) forms complex 22 by internal cross-linking with the carboxylic acid of HA through ionic interactions (Fan et al., 2015). The complex exhibit pH-responsive release of cisplatin. The release of cisplatin is 2–3 times higher in acidic pH than in neutral pH. In addition, HA is the major extracellular matrix of tumor tissue and its receptor CD44 is highly expressed in many tumor tissues (Nikitovic et al., 2015). The complex is internalized by CD44-based endocytosis upon arrival in the tumor tissue, and the internalized complex rapidly releases cisplatin in the acidic lysosomal compartment.
pH-sensitive calcium carbonate nanoparticles (CPNP) have also been used as delivery vehicles for cisplatin (Bastakoti et al., 2013; Chen et al., 2013; Chen et al., 2014; Ding et al., 2017). Biomineralization of cisplatin using CPNP produces pH-responsive nanoparticles that are stable at physiological pH and degrade at acidic pH, thereby releasing cisplatin (Chen et al., 2013; Chen et al., 2014). Biomineralized cisplatin particles exhibit significant antitumor activity in A549 tumor-bearing mice.
2.3 Enzyme- responsive
The key role of enzymes in biological and metabolic processes is well recognized. In pathological conditions such as tumors, the concentration and activity of certain enzymes are significantly higher than in normal conditions due to changes in enzyme expression (Zelzer et al., 2013; Che and Van Hest, 2016; Karimi et al., 2016). Such abnormalities in enzyme expression and activity can be used as triggers for metallodrug enzyme response systems. Enzyme-responsive drugs usually depend on the cleavage of specific modifications on the drug by the abnormal enzyme.
A new class of platinum-acridine anticancer drugs has recently been reported as enzyme-responsive prodrugs (Ding et al., 2014)12231. The platinum-acridine complexes 23 (Figure 5) used ethane-1,2-diamine (23a) or propane-1,3-diamine (23b) as masked groups of Pt(II) complex, respectively (Ding et al., 2014). Valproic ester is recognized as a reactive substrate by human carboxylesterase-2 (hCES-2), which is highly expressed in tumor tissues (Huttunen et al., 2011). Complexes 23a and 23b showed complete resistance to chemical hydrolysis but could be efficiently cleaved by hCES-2. Furthermore, the cytotoxicity of complexes 23a and 23b was significantly lower than that of the unmodified platinum-acridine complex, which may be due to the reduced reactivity of the modified complex with cellular DNA. The nature of the non-masked groups hardly affects their activity. In vitro experiments showed that the activity of both complexes was significantly enhanced in the NCI-H1435 cell line compared to that in the A549 cell line, due to the fact that NCI-H1435 cells possess significantly higher levels of hCES-2 enzyme than A549 cells and that higher levels of hCES-2 perform stronger cleavage of valproate to produce the active form of platinum-acridine hybrids. These findings provided a new idea for the enzymatic conversion of inactive platinum prodrugs to the active form.
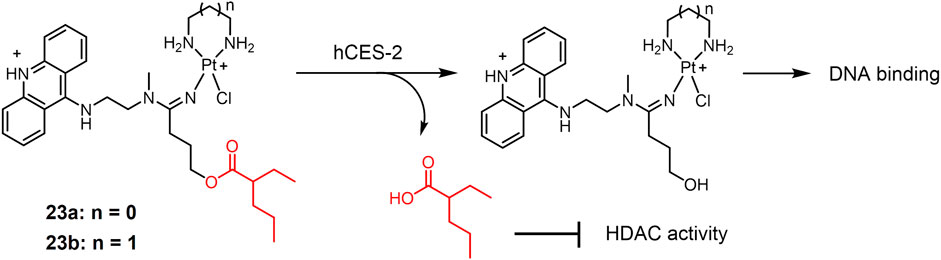
FIGURE 5. Human carboxylesterase-2 (hCES-2)-responsive Pt(II) prodrugs undergoing enzymatic cleavage to release active Pt(II) drugs and valproate as an HDACi.
3 Exo-stimuli-responsive Pt- and Ru-based anticancer drugs
In contrast to the endogenous stimuli of pathological factors in the tumor microenvironment described above, exogenous stimuli such as light, temperature and magnetic fields can activate drugs remotely or in vitro at specific times. Therefore, the use of exogenous stimuli to achieve activation of prodrugs can effectively reduce systemic toxicity and side effects. In this section, we will focus on anti-lung cancer metallic drugs activated by light or heat.
3.1 Light- responsive
Light as a trigger for stimulating reactive drugs has high temporal and spatial accuracy and is non-invasive (Butler and Sadler, 2013; Mari et al., 2015). And the release profile of the active drug can also be tuned by adjusting the wavelength and intensity of light and the irradiation time. At this stage, there are two main strategies to design photo-responsive metal drugs: 1) photoactivated chemotherapy (PACT) that a prodrug is activated by light to produce bioactive forms; 2) photodynamic therapy (PDT) that a photosensitizer (PS) is irradiated to produce ROSs to induce cell death. PDT is currently being used clinically in oncology treatment (Dolmans et al., 2003). However, PDT is only effective in the presence of oxygen, which limits the use of PDT because of the hypoxia of tumor cells. For both light activation actions, NIR light is always more suitable for in vivo use than UV and visible light because of its excellent tissue penetration (Rai et al., 2010; Staderini et al., 2015).
3.1.1 Photo-active platinum complexes
The development of photoactive Pt(IV) prodrugs has been reported by researchers since 1996 (Kratochwil et al., 1996). A Pt(IV) complex trans,cis-[PtCl2I2(en)] was photolyzing through 410 nm light irradiation to form active cis-[PtCl2 (en)] anticancer agent that bound to DNA and inhibited tumor cell growth in vitro. However, even in the absence of light, this complex was prone to decomposition. To solve this problem, Pt(IV)-azide complexes have entered the vision of researchers. Azide groups substituting iodine ligands in Pt(IV) complexes can improve the stability of these complexes to reducing agents (Butler and Sadler, 2013). Interestingly, all trans Pt(IV)-azide complexes photoactive prodrugs were found to exhibit higher water solubility as well as stronger photocytotoxicity (Mackay et al., 2006). Based on this, a new Pt(IV)-azide complex 24 (Figure 6) was developed (Farrer et al., 2010). Complex 24 was potent cytotoxic to tumor cells under irradiation of low doses of visible light. Investigation of photohydrolytic products had revealed azido ligands were released to bind DNA, while the pyridine ligands remained bound on Pt(IV) complex (Figure 6).
The inspiring results of complex 24, have prompted researchers to fully investigate Pt(IV)-azide complexes as photo-active prodrugs. A series of trans-Pt(IV)-azide complexes with biologically active axial ligands were developed (Shi et al., 2020a). These complexes can selectively target tumor cells depending on the axial ligand and attack multiple cellular components simultaneously, exerting a multi-targeting mechanism of action and thus improving anticancer efficacy. Complexes 25-27a, replaced one axial hydroxide ligand with dichloroacetate (DCA), coumarin 3-carboxylate (cou), and pyruvate dehydrogenase kinase inhibitor 4-phenylbutyrate (PhB), respectively. They were further functionalized using DCA in place of a second axial hydroxide ligand, obtaining bifunctional complexes 25-27b, respectively. All these complexes exhibited stronger photocytotoxicity and cell accumulation in A549 cells than the parent complex 24. Notably, the monofunctional complexes with one axial hydroxide ligand exhibited higher water solubility. At the same concentration, cellular Pt accumulation and light-induced cellular ROS levels were significantly higher for the bifunctional complex compared to the monofunctional complex. Complex 27b exhibited the highest phototoxicity indices against A549 lung cancer cells. Biotinylated Pt(IV)-azide complexes 28a-b (Shi et al., 2020b) were also designed to improve therapeutic efficacy via biotin mediated lung cancer targeting (Prasad et al., 1999; Wang et al., 1999; Luo et al., 2006). Both complexes were relatively nontoxic to A549 cells and normal MRC-5 lung fibroblasts under dark conditions. Under light irradiation, both complexes exhibited strong photocytotoxicity, and the phototoxicity of the complex 28b was twice as high as that of complex 28a.
1,8-Naphthylimine and its extended π-conjugated system are well known as multifunctional fluorescent molecules that have been widely used in oncology treatment (Banerjee et al., 2013; Tandon et al., 2017; Kumari et al., 2019). 1,8-Naphthylimine conjugation with anticancer drugs can enhance the cytotoxicity of the drugs (Chen et al., 2018; Shanmugaraju et al., 2018; Tomczyk and Walczak, 2018; Jia et al., 2019; Ma et al., 2019). Based on this, photoactive Pt(IV) azide complexes 29a-c with axial 1,8-naphthalimide derivatives were developed (Shi et al., 2021). These complexes were also stable in dark eve in the presence of the bioreductant GSH. Under visible light irradiation, they decomposed to produce significant photocytotoxicity. Complex 29b bearing electron-withdrawing -NO2 substituent exhibited stronger photocytotoxicity than 29c under blue light irradiation. Notably, complex 29c bearing electron-donating -NMe2 substituent was capable for activation under green light irradiation. Moreover, 1,8-naphthalimide is a photosensitizer that can generate ROS, thus enhancing the photocytotoxicity of photoactive complexes (Banerjee et al., 2013). Exposure of complexes 29a-c to 465 nm blue light significantly enhanced intracellular ROS level.
In addition to Pt(IV) complexes, many Pt(II) complexes also exhibit photocytotoxicity. For example, carboplatin and transplatin can dissociate Cl− and convert to substances with higher DNA binding capacity under UV irradiation, which in turn enhances the toxicity to tumor cells (Heringova et al., 2006; Mlcouskova et al., 2012). One of the main strategies for designing photo responsive Pt(II) complexes is the introduction of chromophore moiety harvesting light. Pt(II) complex 30 (Figure 7) contained photoswitchable 1,2-dithienylethene-containing ligands (Presa et al., 2015). 1,2-Dithienylethylene derivatives are photochromic molecules can converted to their open or closed forms upon exposure to visible or ultraviolet light (Irie, 2000). The ring-opening form (30-O) and the ring-closed form (30-C) exhibited different properties in terms of DNA binding and cytotoxicity. 30-C interacted more strongly with DNA and modified the DNA shape more efficiently than 30-O, therefore showing a twice cytotoxicity against small cell lung cancer DMS53 cells (IC50 value of 34.4 μM for 30-C versus 75.9 μM for 30-O, respectively). These results suggested that complex 30 may be a potential photoactive lung cancer therapeutic agent.
Pt(II) drugs or prodrugs based on cisplatin lack of the ability of generating ROS under photo irradiation. The main strategy to construct Pt(II) drugs for PDT is to combine Pt(II) complexes with photosensitizers. A series of BODIPY-Pt(II) complexes have been developed as potential PDT anticancer agents based on the PDT activity of boron-dipyrrolidene (BODIPY) dyes. The photoactive BODIPY ligand (R-BODIPY) was used to construct Pt(II) complex 31 bearing (Ramu et al., 2018). A glucose group targeting tumor cells was added to the tepyridine molecule in the complex 39 for targeted chemotherapy, in addition to the ability to increase water solubility (Macheda et al., 2005). Under red light (600–720 nm) irradiation, complex 31 showed significant photocytotoxicity against A549 lung cancer cells with negligible dark toxicity.
Although conventional platinum-based anticancer drugs are mononuclear, multinuclear platinum complexes have been reported to show higher anticancer efficacy. A binuclear hybrid ligand Pt(II) complex 32 was developed (Ramu et al., 2019). Complex 32 had a 1,2-diaminocyclohexane ligand and a morpholine-bound BODIPY-linked bridging ligand with two catecholate moieties. A morpholine unit was linked to the BODIPY for lysosomal localization (Andrew et al., 1997; Wang et al., 2016; Zhu et al., 2018). Complex 32 showed low toxicity in the dark and in normal cells and strong photocytotoxicity in A549 lung cancer cells under light (650–850 nm). Cellular localization showed that the complex was mainly enriched in lysosomes. Complex 32 exerted anti-tumor effects by inducing apoptosis through lysosomal dysfunction.
3.1.2 Reactive oxygen species-generating ruthenium complexes
Photoluminescent Ru(II) polypyridyl complexes are considered promising candidates of PSs for PDT due to long-termed photostability and high efficiency of 1O2 production (Liu et al., 2018). TLD1433 (33, Figure 8), the first Ru(II)-based PS that entered a human clinical trial, has successfully completed a phase Ib clinical trials for intravesical PDT of non-muscle invasive bladder tumor (Monro et al., 2019). TLD1433 also shows potentials in combating lung cancer. Under green light (532 nm) irradiation by an optical surface appliactor, TLD1433 induced an EC50 of 1.98 μM against A549 cells (Chamberlain et al., 2020). The exciting achievements of TLD1433 encourage researchers to explore luminescent Ru(II) complexes as PSs candidates for lung cancer treatment (Wu et al., 2022).
Notably TLD1433 could not be activated for lung cancer PDT under red light irradiation which has deeper penetration than green light (Chamberlain et al., 2020). To overcome this shortage, long wavelength light activable Ru(II)-based PSs were designed. Complex 34 with BODIPY chromophore conjugated to a Ru(tpy)2 complex (Liu et al., 2020a) increased its absorption in the green spectral region (450–600 nm) and promoted the formation of sensitized singlet oxygen upon green light activation, which in turn induced a significant photocytotoxicity against the A549 human lung cancer cell line with an EC50 value of 1.50 μM under 500 nm light irradiation at 0.48 J/cm−2. Another π-conjugation extended BODIPY-Ru(II) complex 35 was designed and exhibited strong absorption at 604 and 654 nm, lying in the phototherapeutic window (Paul et al., 2020). Complex 35 was activated by low dose of red light (600–720 nm) to show superior phototoxicity with an IC50 of 0.02 μM and a PI value of > 5000 against A549 cells. A NIR-II emissive fluorophore was coordinated with dinuclear Ru(II)-arene cocomplex and self-assembled to form metallacycle complex 36 (Xu et al., 2022). With excitation of 808 nm laser, complex 36 emitted over 1000 nm and exhibited photothermal effect. In vivo test showed that upon exposure of 808 nm laser, complex 36 efficiently eliminated subcutaneous tumors of A375 cells, showing better therapeutic effect than cisplatin. Recently, this system was employed to design novel Ru(II)-metallacycle that emitted over 1,100 nm, which suggested exciting in vivo chemo-phototherapeutic effects for cisplatin-resistant lung cancer (Li et al., 2022).
The Chao group have developed two-photon absorption (TPA) PDT agents based on Ru(II) complexes (Liu et al., 2020b). Complexes 37a-c exhibited large TPA cross section (σ2) at 810 nm, among which 37c have the highest σ2 value of 181 GM. Upon 810 nm two-photon irradiation, 37a showed the strongest phototoxicity against A549 cells and cisplatin-resistant A549R cells, consistent to its highest cellular uptake.
Lacking sufficient specificity for cancer cells is one of the major drawbacks of PS for lung cancer PDT. Targeting receptors on lung cancer cells is a useful strategy to design lung cancer selective PSs. Somatostatin receptors (SSTRs) are overexpressed in various cancer cells including lung cancer (Callison et al., 2011). T. Weil and colleagues (Wang et al., 2015b) conjugated a Ru(II)-based PS to the peptide hormone somatostatin (amino sequence: AGCKNFFWKTFTSC, short for SST) via an azide-alkyne click reaction to obtain complex 38 (Figure 9). Complex 38 entered cells via receptor-mediated endocytosis, exhibiting a 100-fold increased accumulation than non-SST conjugated Ru(II) complex inside A549 cells. Following by light irradiation, complex 38 induced pronounced phototoxicity against A549 cells. The Chao group designed a biotin-modified Ru(II) complex 39 to promote its cancer cell selectivity (Li et al., 2019). Complex 39 exhibited a 13.9-fold increase in intracellular accumulation in A549 cells over normal tissue HLF-a cells. Two-photon irradiation (820 nm) of complex 39 significantly induced apoptosis of A549R cells, with an IC50 value of 3.3 μM and a PI value of 22.1.
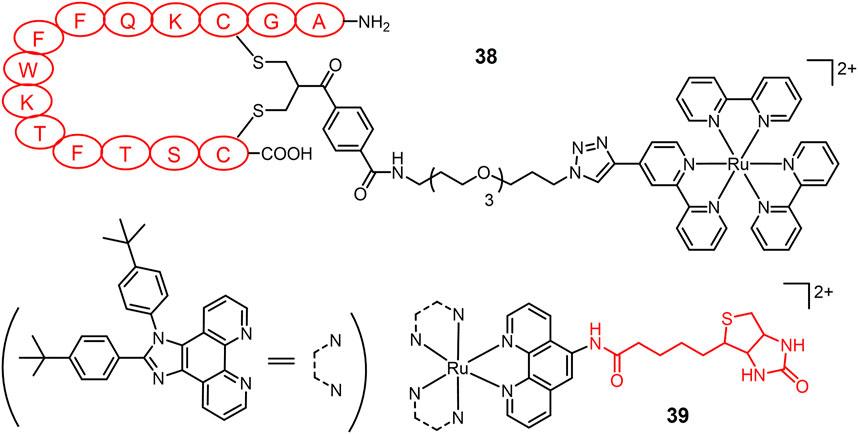
FIGURE 9. Light-responsive Ru(II) prodrugs bearing receptor-binding ligands as cancer selective photosensitizers for PDT.
3.1.3 Photosubstituting ruthenium complexes
Photosensitizers for PDT of lung cancer are relied on oxygen concentration. Making use of photo-induced ligand(s) substitution reaction, ruthenium complex after light irradiation produces an uncaged intermediate that readily coordinates with biomolecules inside cells. These photosubstituting ruthenium complexes serve as PACT agents to combat lung tumors under hypoxia in an oxygen-independent manner.
Monodentate ligand in a Ru(II) complex easily undergoes ligand substitution reaction, especially under light irradiation. Half-sandwich Ru(II)-arene complex 40 bearing pyridinyl naphthalimides (Figure 10) as monodentate ligand were stable in the dark, and released the monodentate pyridyl ligand upon blue light irradiation (Bisceglie et al., 2022). It exhibited high photocytotoxicity to A549 cells under blue light irradiation with an IC50 value of 10.55 μM. A series of half-sandwich Ru(II) complexes 41a-f were investigated for synergistic PACT and PDT treatment of lung cancer agent (Chen et al., 2015). Non-substituted complex 41a exhibited the strongest photocytotoxicity against A549 cells, consistent with its most efficient ability to induce ligand dissociation and 1O2 production.
The Bonnet group have explored thioether ligand-chelating Ru(II) complexes as PACT agents. The softness of thioether-Ru coordination bonds enhances their photolability (Van Rixel et al., 2016; Cuello-Garibo et al., 2019; Meijer and Bonnet, 2019). A methylthiomethyl pyridine chelating Ru-complex 42 was designed (Chen et al., 2022) and its photocytotoxicity and cellular uptake were evaluated in A549 cells. In the dark, the EC50 value was 59 μM. After 15 min of green light irradiation (520 nm, 21 mW/cm-2, 19 J/cm-2), the EC50 value (effective concentration) of the complex 42 decreased to 6.5 μM, exhibiting significant photocytotoxicity. By applying such photo-uncaging approach, a rigidin analogue containing thioether group as tubulin polymerization inhibitor, was caged to a Ru-complex (Van Rixel et al., 2019). Upon green light irradiation, rigidin was released, accompanied by drastic reduction of A549 cell growth both in vitro under hypoxia and on lung cancer tumor xenograft mice. Caged rigidin on Ru-complexes also reduced its toxicity. Glucose-conjugated complexes 43 were designed to improve their water solubility and cell uptakes (Lameijer et al., 2016). Upon 450 nm light irradiation, the glucose thioester ligand was released and substituted by water, forming a reactive Ru(II) intermediate for DNA interaction. Via the introduction of dppn ligand (benzo [i]dipyrido[3,2-a;2′,3′-c]phenazine), both complexes generated massive amounts of 1O2 for efficient photocleavage of DNA. Dual actions of photo activation mode led to high phototoxicity of 43 under low dose visible light irradiation. Complex 43a exhibited an EC50 value of 0.58 μM against A549 cells with PI value of 86; while 43b exhibited an EC50 (effective concentration) value of 0.72 μM against A549 cells with PI value of 26, respectively.
3.2 Thermoresponsive
Another exogenous trigger for metallodrugs is heat. Thermosensitive polymers are often used as carriers because of their solubility that undergoes a structural transition between swelling and contracting types with increasing temperature. Temperature-responsive metallodrugs are constructed by exploiting the temperature difference between diseased and healthy tissues (Neumann et al., 2017). By adjusting the temperature near the low critical solution temperature (LCST) at the tumor location, the polymer can selectively release the metallodrug it carries and thus exert anti-tumor activity (Chen et al., 2016b).
A Ru(II) aromatic complex 44 bearing thermoresponsive fluorous chain (Figure 11) has been reported as a heat-sensitive metallodrug for lung cancer treatment (Clavel et al., 2014). A549 cells were tested as a model at 37 and 41°C, respectively. Complex 44 exhibited good thermosensitive behavior. The complex was almost inactive at 37°C closed to normal body temperature with an IC50 value > 500 μM. After 2 h of hyperthermia stimulus at 41°C, its toxicity became significant with an IC50 value of 33 μM increasing by at least 2 orders of magnitude.
4 Dual-stimuli-responsive Pt- and Ru-based anticancer drugs
4.1 Redox/pH-responsive
As mentioned above, Pt(IV) prodrugs are virtually inert until reduced to the corresponding Pt(II) active substance, a property that minimizes adverse side effects. Another approach to improve the efficacy and reduce the side effects of platinum drugs is to load platinum drugs in a drug carrier, thus enabling targeted delivery and release of the drug under specific stimuli. pH-responsive carriers were employed for designing a dual stimuli-responsive metallic prodrugs. A acid-cleavable amide bond was introduced to conjugate a hydrophilic dendrimer loaded with a Pt(IV) prodrug (PAMAM/Pt) with a hydrophobic polymer chain, obtaining 45 (Figure 12) (Li et al., 2016). 45 self-assembled to form clustered nanoparticles with a size of about 100 nm under physiological pH conditions (pH 7.4). Under weak acidic condition (pH 6.8), 45 was hydrolyzed, triggering the release of small PAMAM/Pt prodrugs (∼5 nm). The rapid release of Pt(IV) prodrug with PAMAM dendrimers in acidic tumor microenvironment promoted the tumor penetration of the drug. Finally, Pt(IV) prodrug is rapidly reduced intracellularly to release active cisplatin to produce powerful antitumor efficacy. In animal studies, 45 exhibited extremely effective antitumor effects against A549R cisplatin-resistant human lung cancer model. A dibenzoate-capped kiteplatin analogue (46) was stable at acidic condition and substituted by chloride at pH 1.5 mimicking stomach environment. Complex 46 was found significantly more effective than cisplatin against A549 cells in vitro with an IC50 value of 0.05 μM (Margiotta et al., 2016). In vivo anticancer activity of complex 46 after oral administration on LLC-bearing mouse model was comparable with intraperitoneal administration of cisplatin (Barbanente et al., 2022). These results suggest that complex 46 have potential as oral anticancer agents.
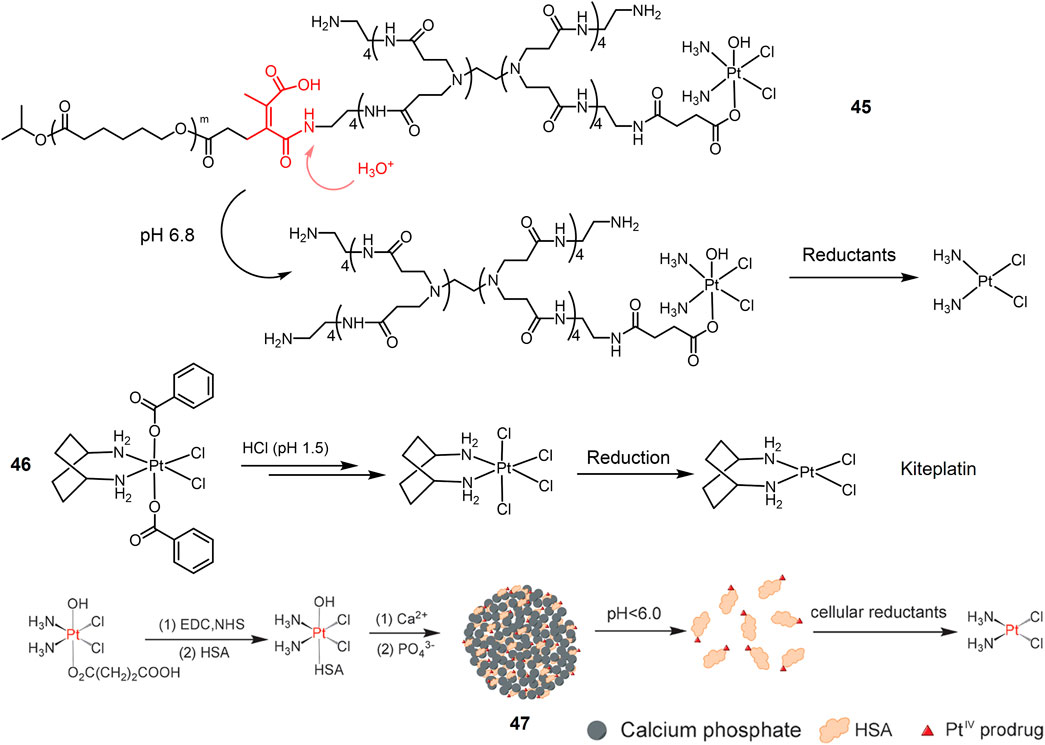
FIGURE 12. Redox/pH dual-responsive Pt(IV) prodrugs. Schematic illustration of complex 47 was reproduced with permission from ref. (Shi et al., 2015). Copyright 2015 John Wiley.
pH-responsive nanoparticles were also employed for Pt(IV) prodrugs delivery. A Pt(IV) prodrug was attached to Human serum albumin (HSA) that protected it from premature reduction before being uptaken by cells and improved cancer targeting efficacy (Desai et al., 2006; Neumann et al., 2010; Zheng et al., 2014; Lee et al., 2015). Pt(IV)-HSA was further conjugated with calcium phosphate (CaP) nanoparticles, obtaining a hybrid Pt(IV)-HAS-CaP 47 (Figure 12) (Shi et al., 2015). 47 was decomposed in an acidic environment (pH < 6.0) to release the Pt-HSA complex, which was reduced by reducing agents inside cells to generate active cisplatin, thus exerting anti-tumor effects. Based on the dual response properties, Pt-HSA/CaP is more cytotoxic against A549 cells than cisplatin and the precursor Pt(IV) complex.
In addition to Pt(IV) complexes, Pt(II) complexes can also be used for pH and reduction dual response metal drugs. A dual-responsive supramolecular PEGylated dendrimer system 55 (TSPDS) was reported (Maity et al., 2016). TSPDS has the properties of biocompatibility, monodispersity and highly branched structure (Wolinsky and Grinstaff, 2008; Cheng et al., 2011b; Sun et al., 2015). In addition, TSPDS is formed via reducible disulfide polymerization and is redox sensitive (Nystrom and Wooley, 2011). The PEGylated Pt(II) complex (Pt(II)-K-mPEG) is conjugated to TSPDS via an acid-dissolvable Pt-O bond (Binauld et al., 2012). The system maintains a stable size of about 120 nm at physiological pH, and at pH 5.0, the Pt-O bond is acidolyzed leading to the release of the Pt(II)-K-mPEG complex. In addition, redox-induced degradation of TSPDS can facilitate the release of acid-activated Pt(II)-K-mPEG complexes under acidic conditions. In vitro experiments have shown that lower pH and higher concentrations of reducing agents in A549 cells can trigger the degradation of this system, leading to the release of active platinum analogs for antitumor effects (IC50 = 4 μg/ml−1). In addition, TSPDS showed comparable antitumor efficacy to cisplatin in A549 xenograft tumors in vivo with fewer side effects.
4.2 Redox/light-responsive
The Pt(IV) prodrug-bridged β-CD dimer 48 (Figure 13) is used as the host molecule (H) and the adamantine-modified porphyrin as the guest molecules (G) to construct redox-photo bis-reactive supramolecular self-assembled nanoions (Zhang et al., 2015). The Pt(IV) prodrug is activated by reduction in the presence of reducing agents. The porphyrin-based moieties can act as photosensitizers to generate ROS and exert PDT effects on tumor cells (Detty et al., 2004). Therefore, the supramolecular assembly has potential antitumor activity and overcome cisplatin resistance by dual chemotherapy-photodynamic therapy. The cytotoxicity and cellular uptake of this assembly were evaluated in A549 cisplatin-resistant cells. The results showed that the cytotoxicity was significantly stronger than that of cisplatin under blue light exposure. Moreover, the cellular uptake of the nanoparticles was 12-fold higher than that of cisplatin due to the increased lipophilicity of the porphyrin moiety.
A dinuclear Ru(II)-azo complex 49 was developed as a GSH-activable photosensitizer (Zeng et al., 2017b). The azo-bridged ligand is strongly electron withdrawing and acts as a lock to turn off the luminescence of the complex (Li et al., 2013a; Li et al., 2013b). GSH can reduce azo group in dinuclear Ru(II) complexes into hydrazine group, therefore restoring their luminescence and photo-induced ROS production. In vitro experiments showed that the complexes showed high cellular accumulation in the mitochondria of the cells. In A549 cells, the complex showed weak cytotoxicity in the dark (IC50 > 70 μM), while its phototoxicity was increased 14-fold (IC50 = 5.0 μM) by light irradiation at 450 nm (20 mW/cm−2, 15 min).
A Pt(IV) and Ru(II) hybrid polymer 50 containing redox-responsive Pt(IV) moiety and light-responsive Ru(II) moiety was designed as dual-responsive treatment of cisplatin resistant lung cancer (Zeng et al., 2020). Polymer 50 self-assembled into nanoparticles that could be efficiently untaken by cancer cells. Red light irradiation triggered the release of Ru(II) complexes that could bind to DNA for chemotherapy, and generated 1O2 for PDT. Reduction by GSH inside lung cancer cells activated Pt(IV) prodrug to form cisplatin. Synergistic anticancer actions led to significant phototoxicity against cisplatin-resistant A549R cells.
5 Conclusion and perspectives
Stimuli-responsive Pt- and Ru-complexes are promising metallic anticancer candidates to meet the requirements of precision chemotherapy for lung cancer. They allow for targeted drug delivery and activation at the target site, which will help to modulate the efficacy of the drug and avoid side effects. Based on the versatility and ease of modulation, researchers have developed a number of stimulus-responsive Pt- and Ru-complexes for lung cancer using a variety of different stimuli as triggers. In this review, we survey the relevant literature published in the past 20 years and classify the relevant complexes into intrastimulant-responsive, extrastimulant-responsive, and dual-stimulant-responsive metal drugs based on the type of stimulus responsiveness. Typical examples in each category are appropriately presented with respect to their design strategies, mechanisms of reactive action and pharmacological properties.
Despite promising prospects, few stimuli-responsive metallodrugs have entered clinical trials and none has been approved for the treatment of lung cancer. Indeed, there are still a number of issues needed to be addressed in the development of metallodrugs based on Pt-, Ru-complexes, and complexes with other metal ions for lung cancer therapy. One of these challenges is the exploration of new therapeutic targets and their druggability. Currently, most metallodrug researches for lung cancer are focused on targeting DNA. However, this does not always lead to effective drugs because only small number of active drugs successfully address nucleus to exert their DNA binding. Moreover, their exact mechanisms of action and behavior in the biological environment have not been fully explored for many of the metallodrugs on the market. For these reasons, exploring new therapeutic targets and verifying their authenticity is an absolute necessity. Another major challenge is the systemic toxicity and pharmacokinetics of metallodrugs. Although a large number of stimuli-responsive metallodrugs have been found to have different cytotoxic properties in vitro and in vivo, their pharmacokinetic aspects including absorption, distribution, metabolism and excretion (ADME) properties are less explored. Lipinski’s “Rule of Five” will help to theoretically predict the pharmacokinetics of metallodrugs (Lipinski et al., 2001; Stella et al., 2007). A greater challenge arises from the requirement for precise control and initiation of the response system. For example, the fact that tumor microenvironments only exhibit a tiny difference of pH value compared to the normal tissues requires that the pH response system must be very sensitive to changes in acidity. This demanding requirement also applies to enzyme-responsive metallodrugs. Finally, new mechanisms of action of some non-classical metallodrugs have the potential to be developed into new stimuli-responsive metallodrugs. Regardless of the influence of the ligand on the pharmacological properties of the complex, the metal ion is central to the pharmacological action of the metal complex. However, the vast majority of researches now focuses on transition metal complexes, particularly platinum and ruthenium complexes; while other metal complexes have been largely ignored. The neglected metal complexes may have some unknown properties that could overcome or compensate for the shortcomings of current stimulant-responsive metal drugs. Despite the many difficulties in developing stimulant-responsive metal drugs, this emerging field shows great promise.
Author contributions
JZ and GL contributed conception of the manuscript. CZ, TK, and XW wrote the manuscript. JS drew the figures. All authors contributed to manuscript revision, read and approved the submitted version.
Funding
This work was supported by the financial support from the “Yong Talent Support Plan” of Xi’an Jiaotong University, the National Science Foundation of China (No. 22107087), the Key Science and Technology Program of Shaanxi Province (2021SF-034).
Conflict of interest
The authors declare that the research was conducted in the absence of any commercial or financial relationships that could be construed as a potential conflict of interest.
Publisher’s note
All claims expressed in this article are solely those of the authors and do not necessarily represent those of their affiliated organizations, or those of the publisher, the editors and the reviewers. Any product that may be evaluated in this article, or claim that may be made by its manufacturer, is not guaranteed or endorsed by the publisher.
References
Anderson, H., Wagstaff, J., Crowther, D., Swindell, R., Lind, M. J., Mcgregor, J., et al. (1988). Comparative toxicity of cisplatin, carboplatin (CBDCA) and iproplatin (CHIP) in combination with cyclophosphamide in patients with advanced epithelial ovarian cancer. Eur. J. Cancer Clin. Oncol. 24, 1471–1479. doi:10.1016/0277-5379(88)90338-0
Andrew, C. L., Klemm, A. R., and Lloyd, J. B. (1997). Lysosome membrane permeability to amines. Biochim. Biophys. Acta 1330, 71–82. doi:10.1016/s0005-2736(97)00145-4
Ang, W. H., Khalaila, I., Allardyce, C. S., Juillerat-Jeanneret, L., and Dyson, P. J. (2005). Rational design of platinum(IV) compounds to overcome glutathione-S-transferase mediated drug resistance. J. Am. Chem. Soc. 127, 1382–1383. doi:10.1021/ja0432618
Banerjee, S., Veale, E. B., Phelan, C. M., Murphy, S. A., Tocci, G. M., Gillespie, L. J., et al. (2013). Recent advances in the development of 1, 8-naphthalimide based DNA targeting binders, anticancer and fluorescent cellular imaging agents. Chem. Soc. Rev. 42, 1601–1618. doi:10.1039/c2cs35467e
Barbanente, A., Gandin, V., Ceresa, C., Marzano, C., Ditaranto, N., Hoeschele, J. D., et al. (2022). Improvement of kiteplatin efficacy by a benzoato Pt(IV) prodrug suitable for oral administration. Int. J. Mol. Sci. 23, 7081. doi:10.3390/ijms23137081
Bastakoti, B. P., Wu, K. C.-W., Inoue, M., Yusa, S.-I., Nakashima, K., and Yamauchi, Y. (2013). Multifunctional core-shell-corona-type polymeric micelles for anticancer drug-delivery and imaging. Chemistry 19, 4812–4817. doi:10.1002/chem.201203958
Basu, U., Banik, B., Wen, R., Pathak, R. K., and Dhar, S. (2016). The platin-X series: Activation, targeting, and delivery. Dalton Trans. 45, 12992–13004. doi:10.1039/c6dt01738j
Bergamo, A., and Sava, G. (2011). Ruthenium anticancer compounds: Myths and realities of the emerging metal-based drugs. Dalton Trans. 40, 7817–7823. doi:10.1039/c0dt01816c
Bhargava, A., and Vaishampayan, U. N. (2009). Satraplatin: Leading the new generation of oral platinum agents. Expert Opin. Investig. Drugs 18, 1787–1797. doi:10.1517/13543780903362437
Bildstein, L., Dubernet, C., and Couvreur, P. (2011). Prodrug-based intracellular delivery of anticancer agents. Adv. Drug Deliv. Rev. 63, 3–23. doi:10.1016/j.addr.2010.12.005
Binauld, S., Scarano, W., and Stenzel, M. H. (2012). pH-triggered release of platinum drugs conjugated to micelles via an acid-cleavable linker. Macromolecules 45, 6989–6999. doi:10.1021/ma3012812
Bisceglie, F., Pelosi, G., Orsoni, N., Pioli, M., Carcelli, M., Pelagatti, P., et al. (2022). Light triggers the antiproliferative activity of naphthalimide-conjugated (η(6)-arene)ruthenium(II) complexes. Int. J. Mol. Sci. 23, 7624. doi:10.3390/ijms23147624
Bolden, J. E., Peart, M. J., and Johnstone, R. W. (2006). Anticancer activities of histone deacetylase inhibitors. Nat. Rev. Drug Discov. 5, 769–784. doi:10.1038/nrd2133
Bonnet, S., Archer, S. L., Allalunis-Turner, J., Haromy, A., Beaulieu, C., Thompson, R., et al. (2007). A mitochondria-K+ channel axis is suppressed in cancer and its normalization promotes apoptosis and inhibits cancer growth. Cancer Cell 11, 37–51. doi:10.1016/j.ccr.2006.10.020
Bots, M., and Johnstone, R. W. (2009). Rational combinations using HDAC inhibitors. Clin. Cancer Res. 15, 3970–3977. doi:10.1158/1078-0432.CCR-08-2786
Bruijnincx, P. C., and Sadler, P. J. (2008). New trends for metal complexes with anticancer activity. Curr. Opin. Chem. Biol. 12, 197–206. doi:10.1016/j.cbpa.2007.11.013
Brun, M. J., Gomez, E. J., and Suh, J. (2017). Stimulus-responsive viral vectors for controlled delivery of therapeutics. J. Control. Release 267, 80–89. doi:10.1016/j.jconrel.2017.08.021
Buchwald, M., Kramer, O. H., and Heinzel, T. (2009). HDACi--targets beyond chromatin. Cancer Lett. 280, 160–167. doi:10.1016/j.canlet.2009.02.028
Butler, J. S., and Sadler, P. J. (2013). Targeted delivery of platinum-based anticancer complexes. Curr. Opin. Chem. Biol. 17, 175–188. doi:10.1016/j.cbpa.2013.01.004
Callison, J. C., Walker, R. C., and Massion, P. P. (2011). Somatostatin receptors in lung cancer: From function to molecular imaging and therapeutics. J. Lung Cancer 10, 69–76. doi:10.6058/jlc.2011.10.2.69
Chamberlain, S., Cole, H. D., Roque, J., Bellnier, D., Mcfarland, S. A., and Shafirstein, G. (2020). TLD1433-Mediated photodynamic therapy with an optical surface applicator in the treatment of lung cancer cells in vitro. Pharmaceuticals 13, 137. doi:10.3390/ph13070137
Chang, A. (2011). Chemotherapy, chemoresistance and the changing treatment landscape for NSCLC. Lung Cancer 71, 3–10. doi:10.1016/j.lungcan.2010.08.022
Che, H., and Van Hest, J. C. M. (2016). Stimuli-responsive polymersomes and nanoreactors. J. Mat. Chem. B 4, 4632–4647. doi:10.1039/c6tb01163b
Chen, H., Liu, D., and Guo, Z. (2016a). Endogenous stimuli-responsive nanocarriers for drug delivery. Chem. Lett. 45, 242–249. doi:10.1246/cl.151176
Chen, H., Wang, X., and Gou, S. (2019). A cisplatin-based platinum(IV) prodrug containing a glutathione s-transferase inhibitor to reverse cisplatin-resistance in non-small cell lung cancer. J. Inorg. Biochem. 193, 133–142. doi:10.1016/j.jinorgbio.2019.01.014
Chen, Q., Cuello-Garibo, J.-A., Bretin, L., Zhang, L., Ramu, V., Aydar, Y., et al. (2022). Photosubstitution in a trisheteroleptic ruthenium complex inhibits conjunctival melanoma growth in a zebrafish orthotopic xenograft model. Chem. Sci. 13, 6899–6919. doi:10.1039/d2sc01646j
Chen, W., Liu, X., Xiao, Y., Chen, Y., Wang, G., Liu, C., et al. (2014). Nano regulation of cisplatin chemotherapeutic behaviors by biomineralization controls. Small 10, 3644–3649. doi:10.1002/smll.201303849
Chen, W., Xiao, Y., Liu, X., Chen, Y., Zhang, J., Xu, X., et al. (2013). Overcoming cisplatin resistance in chemotherapy by biomineralization. Chem. Commun. 49, 4932–4934. doi:10.1039/c3cc41872c
Chen, Y., Lei, W., Hou, Y., Li, C., Jiang, G., Zhang, B., et al. (2015). Fine control on the photochemical and photobiological properties of Ru(ii) arene complexes. Dalton Trans. 44, 7347–7354. doi:10.1039/c5dt00939a
Chen, Y., Zhang, H., Cai, X., Ji, J., He, S., and Zhai, G. (2016b). Multifunctional mesoporous silica nanocarriers for stimuli-responsive target delivery of anticancer drugs. RSC Adv. 6, 92073–92091. doi:10.1039/c6ra18062k
Chen, Z., Xu, Y., and Qian, X. (2018). Naphthalimides and analogues as antitumor agents: A review on molecular design, bioactivity and mechanism of action. Chin. Chem. Lett. 29, 1741–1756. doi:10.1016/j.cclet.2018.09.020
Cheng, R., Feng, F., Meng, F., Deng, C., Feijen, J., and Zhong, Z. (2011a). Glutathione-responsive nano-vehicles as a promising platform for targeted intracellular drug and gene delivery. J. Control. Release 152, 2–12. doi:10.1016/j.jconrel.2011.01.030
Cheng, Y., Zhao, L., Li, Y., and Xu, T. (2011b). Design of biocompatible dendrimers for cancer diagnosis and therapy: Current status and future perspectives. Chem. Soc. Rev. 40, 2673–2703. doi:10.1039/c0cs00097c
Clarke, M. J., Bitler, S., Rennert, D., Buchbinder, M., and Kelman, A. D. (1980). Reduction and subsequent binding of ruthenium ions catalyzed by subcellular components. J. Inorg. Biochem. 12, 79–87. doi:10.1016/s0162-0134(00)80045-8
Clavel, C. M., Păunescu, E., Nowak-Sliwinska, P., and Dyson, P. J. (2014). Thermoresponsive organometallic arene ruthenium complexes for tumour targeting. Chem. Sci. 5, 1097–1101. doi:10.1039/c3sc53185f
Cocchietto, M., Zorzet, S., Sorc, A., and Sava, G. (2003). Primary tumor, lung and kidney retention and antimetastasis effect of NAMI-A following different routes of administration. Invest. New Drugs 21, 55–62. doi:10.1023/a:1022916310694
Crucho, C. I. (2015). Stimuli-responsive polymeric nanoparticles for nanomedicine. ChemMedChem 10, 24–38. doi:10.1002/cmdc.201402290
Cuello-Garibo, J.-A., James, C. C., Siegler, M. A., Hopkins, S. L., and Bonnet, S. (2019). Selective preparation of a heteroleptic cyclometallated ruthenium complex capable of undergoing photosubstitution of a bidentate ligand. Chemistry 25, 1260–1268. doi:10.1002/chem.201803720
Desai, N., Trieu, V., Yao, Z., Louie, L., Ci, S., Yang, A., et al. (2006). Increased antitumor activity, intratumor paclitaxel concentrations, and endothelial cell transport of cremophor-free, albumin-bound paclitaxel, ABI-007, compared with cremophor-based paclitaxel. Clin. Cancer Res. 12, 1317–1324. doi:10.1158/1078-0432.CCR-05-1634
Detty, M. R., Gibson, S. L., and Wagner, S. J. (2004). Current clinical and preclinical photosensitizers for use in photodynamic therapy. J. Med. Chem. 47, 3897–3915. doi:10.1021/jm040074b
Dhar, S., and Lippard, S. J. (2009). Mitaplatin, a potent fusion of cisplatin and the orphan drug dichloroacetate. Proc. Natl. Acad. Sci. U. S. A. 106, 22199–22204. doi:10.1073/pnas.0912276106
Ding, S., and Bierbach, U. (2015). Target-selective delivery and activation of platinum-based anticancer agents. Future Med. Chem. 7, 911–927. doi:10.4155/fmc.15.37
Ding, S., Pickard, A. J., Kucera, G. L., and Bierbach, U. (2014). Design of enzymatically cleavable prodrugs of a potent platinum-containing anticancer agent. Chemistry 20, 16164–16173. doi:10.1002/chem.201404675
Ding, Y., Zhai, K., Pei, P., Lin, Y., Ma, Y., Zhu, H., et al. (2017). Encapsulation of cisplatin in a pegylated calcium phosphate nanoparticle (CPNP) for enhanced cytotoxicity to cancerous cells. J. Colloid Interface Sci. 493, 181–189. doi:10.1016/j.jcis.2017.01.032
Dolmans, D. E. J. G. J., Fukumura, D., and Jain, R. K. (2003). Photodynamic therapy for cancer. Nat. Rev. Cancer 3, 380–387. doi:10.1038/nrc1071
Dougan, S. J., Habtemariam, A., Mchale, S. E., Parsons, S., and Sadler, P. J. (2008). Catalytic organometallic anticancer complexes. Proc. Natl. Acad. Sci. U. S. A. 105, 11628–11633. doi:10.1073/pnas.0800076105
Dwyer, F. P., Gyarfas, E. C., Rogers, W. P., and Koch, J. H. (1952). Biological activity of complex ions. Nature 170, 190–191. doi:10.1038/170190a0
Fan, X., Zhao, X., Qu, X., and Fang, J. (2015). pH sensitive polymeric complex of cisplatin with hyaluronic acid exhibits tumor-targeted delivery and improved in vivo antitumor effect. Int. J. Pharm. 496, 644–653. doi:10.1016/j.ijpharm.2015.10.066
Farrer, N. J., Woods, J. A., Salassa, L., Zhao, Y., Robinson, K. S., Clarkson, G., et al. (2010). A potent trans-diimine platinum anticancer complex photoactivated by visible light. Angew. Chem. Int. Ed. Engl. 49, 8905–8908. doi:10.1002/anie.201003399
Federici, L., Lo Sterzo, C., Pezzola, S., Di Matteo, A., Scaloni, F., Federici, G., et al. (2009). Structural basis for the binding of the anticancer compound 6-(7-nitro-2, 1, 3-benzoxadiazol-4-ylthio)hexanol to human glutathione s-transferases. Cancer Res. 69, 8025–8034. doi:10.1158/0008-5472.CAN-09-1314
Felber, A. E., Dufresne, M. H., and Leroux, J. C. (2012). pH-sensitive vesicles, polymeric micelles, and nanospheres prepared with polycarboxylates. Adv. Drug Deliv. Rev. 64, 979–992. doi:10.1016/j.addr.2011.09.006
Fu, Q., Xu, J., Ladewig, K., Henderson, T. M. A., and Qiao, G. G. (2015). Degradable cross-linked polymer vesicles for the efficient delivery of platinum drugs. Polym. Chem. 6, 35–43. doi:10.1039/c4py01123f
Gabano, E., Ravera, M., Zanellato, I., Tinello, S., Gallina, A., Rangone, B., et al. (2017). An unsymmetric cisplatin-based Pt(iv) derivative containing 2-(2-propynyl)octanoate: A very efficient multi-action antitumor prodrug candidate. Dalton Trans. 46, 14174–14185. doi:10.1039/c7dt02928d
Galanski, M., Baumgartner, C., Arion, V., and Keppler, Bernhard k. (2003). Bis(2-aminobutanol)dichloroplatinum(II) complexes and their singly and doubly ring-closed butanolato species − novel prodrugs for platinum-based antitumour chemotherapy? Eur. J. Inorg. Chem. 2003, 2619–2625. doi:10.1002/ejic.200300050
Galanski, M., Baumgartner, C., Meelich, K., Arion, V. B., Fremuth, M., Jakupec, M. A., et al. (2004). Synthesis, crystal structure and pH dependent cytotoxicity of (SP-4-2)-bis(2-aminoethanolato-κ2N, O)platinum(II) – A representative of novel pH sensitive anticancer platinum complexes. Inorganica Chim. Acta 357, 3237–3244. doi:10.1016/j.ica.2004.04.003
Gatenby, R. A., and Gillies, R. J. (2004). Why do cancers have high aerobic glycolysis? Nat. Rev. Cancer 4, 891–899. doi:10.1038/nrc1478
Ghosh, S. (2019). Cisplatin: The first metal based anticancer drug. Bioorg. Chem. 88, 102925. doi:10.1016/j.bioorg.2019.102925
Govindan, R., Page, N., Morgensztern, D., Read, W., Tierney, R., Vlahiotis, A., et al. (2006). Changing epidemiology of small-cell lung cancer in the United States over the last 30 years: Analysis of the surveillance, epidemiologic, and end results database. J. Clin. Oncol. 24, 4539–4544. doi:10.1200/JCO.2005.04.4859
Graf, N., and Lippard, S. J. (2012). Redox activation of metal-based prodrugs as a strategy for drug delivery. Adv. Drug Deliv. Rev. 64, 993–1004. doi:10.1016/j.addr.2012.01.007
Gu, M., Wang, X., Toh, T. B., and Chow, E. K. (2018). Applications of stimuli-responsive nanoscale drug delivery systems in translational research. Drug Discov. Today 23, 1043–1052. doi:10.1016/j.drudis.2017.11.009
Gulzar, A., Gai, S., Yang, P., Li, C., Ansari, M. B., and Lin, J. (2015). Stimuli responsive drug delivery application of polymer and silica in biomedicine. J. Mat. Chem. B 3, 8599–8622. doi:10.1039/c5tb00757g
Hall, M. D., and Hambley, T. W. (2002). Platinum(IV) antitumour compounds: Their bioinorganic chemistry. Coord. Chem. Rev. 232, 49–67. doi:10.1016/s0010-8545(02)00026-7
Hall, M. D., Mellor, H. R., Callaghan, R., and Hambley, T. W. (2007). Basis for design and development of platinum(IV) anticancer complexes. J. Med. Chem. 50, 3403–3411. doi:10.1021/jm070280u
Hambley, T. W. (2007a). Chemistry. Metal-based therapeutics. Science 318, 1392–1393. doi:10.1126/science.1150504
Hambley, T. W. (2007b). Developing new metal-based therapeutics: Challenges and opportunities. Dalton Trans. 21, 4929–4937. doi:10.1039/b706075k
Hartinger, C. G., Jakupec, M. A., Zorbas-Seifried, S., Groessl, M., Egger, A., Berger, W., et al. (2008). KP1019, a new redox-active anticancer agent--preclinical development and results of a clinical phase I study in tumor patients. Chem. Biodivers. 5, 2140–2155. doi:10.1002/cbdv.200890195
Heffern, M. C., Matosziuk, L. M., and Meade, T. J. (2014). Lanthanide probes for bioresponsive imaging. Chem. Rev. 114, 4496–4539. doi:10.1021/cr400477t
Heringova, P., Woods, J., Mackay, F. S., Kasparkova, J., Sadler, P. J., and Brabec, V. (2006). Transplatin is cytotoxic when photoactivated: Enhanced formation of DNA cross-links. J. Med. Chem. 49, 7792–7798. doi:10.1021/jm0606692
Hong, R., Han, G., Fernandez, J. M., Kim, B. J., Forbes, N. S., and Rotello, V. M. (2006). Glutathione-mediated delivery and release using monolayer protected nanoparticle carriers. J. Am. Chem. Soc. 128, 1078–1079. doi:10.1021/ja056726i
Hrebackova, J., Hrabeta, J., and Eckschlager, T. (2010). Valproic acid in the complex therapy of malignant tumors. Curr. Drug Targets 11, 361–379. doi:10.2174/138945010790711923
Hu, X., Zhang, Y., Xie, Z., Jing, X., Bellotti, A., and Gu, Z. (2017). Stimuli-responsive polymersomes for biomedical applications. Biomacromolecules 18, 649–673. doi:10.1021/acs.biomac.6b01704
Huang, C., Neoh, K. G., Xu, L., Kang, E. T., and Chiong, E. (2012). Polymeric nanoparticles with encapsulated superparamagnetic iron oxide and conjugated cisplatin for potential bladder cancer therapy. Biomacromolecules 13, 2513–2520. doi:10.1021/bm300739w
Huttunen, K. M., Raunio, H., and Rautio, J. (2011). Prodrugs—From serendipity to rational design. Pharmacol. Rev. 63, 750–771. doi:10.1124/pr.110.003459
Irie, M. (2000). Diarylethenes for memories and switches. Chem. Rev. 100, 1685–1716. doi:10.1021/cr980069d
Jia, D.-G., Zheng, J.-A., Fan, Y.-R., Yu, J.-Q., Wu, X.-L., Wang, B.-J., et al. (2019). Ferrocene appended naphthalimide derivatives: Synthesis, DNA binding, and in vitro cytotoxic activity. J. Organomet. Chem. 888, 16–23. doi:10.1016/j.jorganchem.2019.03.001
Jin, M. Z., and Jin, W. L. (2020). The updated landscape of tumor microenvironment and drug repurposing. Signal Transduct. Target. Ther. 5, 166. doi:10.1038/s41392-020-00280-x
Karimi, M., Ghasemi, A., Sahandi Zangabad, P., Rahighi, R., Moosavi Basri, S. M., Mirshekari, H., et al. (2016). Smart micro/nanoparticles in stimulus-responsive drug/gene delivery systems. Chem. Soc. Rev. 45, 1457–1501. doi:10.1039/c5cs00798d
Klein, A. V., and Hambley, T. W. (2009). Platinum drug distribution in cancer cells and tumors. Chem. Rev. 109, 4911–4920. doi:10.1021/cr9001066
Kratochwil, N. A., Bednarski, P. J., Mrozek, H., Vogler, A., and Nagle, J. K. (1996). Photolysis of an iodoplatinum(IV) diamine complex to cytotoxic species by visible light. Anticancer. Drug Des. 11, 155–171.
Kratz, F., Muller, I. A., Ryppa, C., and Warnecke, A. (2008). Prodrug strategies in anticancer chemotherapy. ChemMedChem 3, 20–53. doi:10.1002/cmdc.200700159
Kumari, R., Sunil, D., and Ningthoujam, R. S. (2019). Naphthalimides in fluorescent imaging of tumor hypoxia - an up-to-date review. Bioorg. Chem. 88, 102979. doi:10.1016/j.bioorg.2019.102979
Lameijer, L. N., Hopkins, S. L., Brevé, T. G., Askes, S. H. C., and Bonnet, S. (2016). D- versus l-glucose conjugation: Mitochondrial targeting of a light-activated dual-mode-of-action ruthenium-based anticancer prodrug. Chem. (Weinheim Der Bergstrasse, Ger. 22, 18484–18491. doi:10.1002/chem.201603066
Lee, S. S., Li, J., Tai, J. N., Ratliff, T. L., Park, K., and Cheng, J. X. (2015). Avasimibe encapsulated in human serum albumin blocks cholesterol esterification for selective cancer treatment. ACS Nano 9, 2420–2432. doi:10.1021/nn504025a
Leijen, S., Burgers, S. A., Baas, P., Pluim, D., Tibben, M., Van Werkhoven, E., et al. (2015). Phase I/II study with ruthenium compound NAMI-A and gemcitabine in patients with non-small cell lung cancer after first line therapy. Invest. New Drugs 33, 201–214. doi:10.1007/s10637-014-0179-1
Levina, A., Mitra, A., and Lay, P. A. (2009). Recent developments in ruthenium anticancer drugs. Metallomics 1, 458–470. doi:10.1039/b904071d
Li, C., Xu, Y., Tu, L., Choi, M., Fan, Y., Chen, X., et al. (2022). Rationally designed Ru(ii)-metallacycle chemo-phototheranostic that emits beyond 1000 nm. Chem. Sci. 13, 6541–6549. doi:10.1039/d2sc01518h
Li, G.-Y., Liu, J.-P., Huang, H.-Y., Wen, Y., Chao, H., and Ji, L.-N. (2013a). Colorimetric and luminescent dual-signaling responsive probing of thiols by a ruthenium(II)-azo complex. J. Inorg. Biochem. 121, 108–113. doi:10.1016/j.jinorgbio.2012.12.019
Li, G., Chen, Y., Wu, J., Ji, L., and Chao, H. (2013b). Thiol-specific phosphorescent imaging in living cells with an azobis(2, 2′-bipyridine)-bridged dinuclear iridium(iii) complex. Chem. Commun. 49, 2040–2042. doi:10.1039/c3cc38687b
Li, H.-J., Du, J.-Z., Du, X.-J., Xu, C.-F., Sun, C.-Y., Wang, H.-X., et al. (2016). Stimuli-responsive clustered nanoparticles for improved tumor penetration and therapeutic efficacy. Proc. Natl. Acad. Sci. U. S. A. 113, 4164–4169. doi:10.1073/pnas.1522080113
Li, J., Zeng, L., Xiong, K., Rees, T. W., Jin, C., Wu, W., et al. (2019). A biotinylated ruthenium(ii) photosensitizer for tumor-targeted two-photon photodynamic therapy. Chem. Commun. 55, 10972–10975. doi:10.1039/c9cc05826e
Lipinski, C. A., Lombardo, F., Dominy, B. W., and Feeney, P. J. (2001). Experimental and computational approaches to estimate solubility and permeability in drug discovery and development settings1PII of original article: S0169-409X(96)00423-1. The article was originally published in advanced drug delivery reviews 23 (1997) 3–25.1. Adv. Drug Deliv. Rev. 46, 3–26. doi:10.1016/s0169-409x(00)00129-0
Lippard, S. J. (1982). New chemistry of an old molecule: cis-[Pt(NH3)2Cl2]. Science 218, 1075–1082. doi:10.1126/science.6890712
Liu, B., Gao, Y., Jabed, M. A., Kilina, S., Liu, G., and Sun, W. (2020a). Lysosome targeting bis-terpyridine ruthenium(II) complexes: Photophysical properties and in vitro photodynamic therapy. ACS Appl. Bio Mat. 3, 6025–6038. doi:10.1021/acsabm.0c00647
Liu, D., Yang, F., Xiong, F., and Gu, N. (2016). The smart drug delivery system and its clinical potential. Theranostics 6, 1306–1323. doi:10.7150/thno.14858
Liu, J., Liao, X., Xiong, K., Kuang, S., Jin, C., Ji, L., et al. (2020b). Boosting two-photon photodynamic therapy with mitochondria-targeting ruthenium–glucose conjugates. Chem. Commun. 56, 5839–5842. doi:10.1039/d0cc01148g
Liu, J., Zhang, C., Rees, T. W., Ke, L., Ji, L., and Chao, H. (2018). Harnessing ruthenium(II) as photodynamic agents: Encouraging advances in cancer therapy. Coord. Chem. Rev. 363, 17–28. doi:10.1016/j.ccr.2018.03.002
Luo, S., Kansara, V. S., Zhu, X., Mandava, N. K., Pal, D., and Mitra, A. K. (2006). Functional characterization of sodium-dependent multivitamin transporter in MDCK-MDR1 cells and its utilization as a target for drug delivery. Mol. Pharm. 3, 329–339. doi:10.1021/mp0500768
Ma, D. L., Chan, D. S., and Leung, C. H. (2014). Group 9 organometallic compounds for therapeutic and bioanalytical applications. Acc. Chem. Res. 47, 3614–3631. doi:10.1021/ar500310z
Ma, W., Zhang, S., Tian, Z., Xu, Z., Zhang, Y., Xia, X., et al. (2019). Potential anticancer agent for selective damage to mitochondria or lysosomes: Naphthalimide-modified fluorescent biomarker half-sandwich iridium (III) and ruthenium (II) complexes. Eur. J. Med. Chem. 181, 111599. doi:10.1016/j.ejmech.2019.111599
Macheda, M. L., Rogers, S., and Best, J. D. (2005). Molecular and cellular regulation of glucose transporter (GLUT) proteins in cancer. J. Cell. Physiol. 202, 654–662. doi:10.1002/jcp.20166
Mackay, F. S., Woods, J. A., Moseley, H., Ferguson, J., Dawson, A., Parsons, S., et al. (2006). A photoactivated trans-diammine platinum complex as cytotoxic as cisplatin. Chem. (Weinheim Der Bergstrasse, Ger. 12, 3155–3161. doi:10.1002/chem.200501601
Maity, A., Gangopadhyay, M., Basu, A., Aute, S., Babu, S. S., and Das, A. (2016). Counteranion driven homochiral assembly of a cationic C3-symmetric gelator through ion-pair assisted hydrogen bond. J. Am. Chem. Soc. 138, 11113–11116. doi:10.1021/jacs.6b06312
Margiotta, N., Savino, S., Marzano, C., Pacifico, C., Hoeschele, J. D., Gandin, V., et al. (2016). Cytotoxicity-boosting of kiteplatin by Pt(IV) prodrugs with axial benzoate ligands. J. Inorg. Biochem. 160, 85–93. doi:10.1016/j.jinorgbio.2015.11.028
Mari, C., Pierroz, V., Ferrari, S., and Gasser, G. (2015). Combination of Ru(ii) complexes and light: New frontiers in cancer therapy. Chem. Sci. 6, 2660–2686. doi:10.1039/c4sc03759f
Mazzaferro, S., Bouchemal, K., and Ponchel, G. (2013). Oral delivery of anticancer drugs II: The prodrug strategy. Drug Discov. Today 18, 93–98. doi:10.1016/j.drudis.2012.08.006
Meijer, M. S., and Bonnet, S. (2019). Diastereoselective synthesis and two-step photocleavage of ruthenium polypyridyl complexes bearing a bis(thioether) ligand. Inorg. Chem. 58, 11689–11698. doi:10.1021/acs.inorgchem.9b01669
Merlino, A. (2016). Interactions between proteins and Ru compounds of medicinal interest: A structural perspective. Coord. Chem. Rev. 326, 111–134. doi:10.1016/j.ccr.2016.08.001
Mjos, K. D., and Orvig, C. (2014). Metallodrugs in medicinal inorganic chemistry. Chem. Rev. 114, 4540–4563. doi:10.1021/cr400460s
Mlcouskova, J., Stepankova, J., and Brabec, V. (2012). Antitumor carboplatin is more toxic in tumor cells when photoactivated: Enhanced DNA binding. J. Biol. Inorg. Chem. 17, 891–898. doi:10.1007/s00775-012-0906-z
Monro, S., Colón, K. L., Yin, H., Roque, J., Konda, P., Gujar, S., et al. (2019). Transition metal complexes and photodynamic therapy from a tumor-centered approach: Challenges, opportunities, and highlights from the development of TLD1433. Chem. Rev. 119, 797–828. doi:10.1021/acs.chemrev.8b00211
Mura, S., Nicolas, J., and Couvreur, P. (2013). Stimuli-responsive nanocarriers for drug delivery. Nat. Mat. 12, 991–1003. doi:10.1038/nmat3776
Nakajima, T., Takayama, T., Miyanishi, K., Nobuoka, A., Hayashi, T., Abe, T., et al. (2003). Reversal of multiple drug resistance in cholangiocarcinoma by the glutathione S-transferase-pi-specific inhibitor O1-hexadecyl-gamma-glutamyl-S-benzylcysteinyl-D-phenylglycine ethylester. J. Pharmacol. Exp. Ther. 306, 861–869. doi:10.1124/jpet.103.052696
Neumann, E., Frei, E., Funk, D., Becker, M. D., Schrenk, H. H., Muller-Ladner, U., et al. (2010). Native albumin for targeted drug delivery. Expert Opin. Drug Deliv. 7, 915–925. doi:10.1517/17425247.2010.498474
Neumann, K., Lilienkampf, A., and Bradley, M. (2017). Responsive polymeric nanoparticles for controlled drug delivery. Polym. Int. 66, 1756–1764. doi:10.1002/pi.5471
Neuzil, J. (2003). Vitamin E succinate and cancer treatment: a vitamin E prototype for selective antitumour activity. Br. J. Cancer 89, 1822–1826. doi:10.1038/sj.bjc.6601360
Nikitovic, D., Tzardi, M., Berdiaki, A., Tsatsakis, A., and Tzanakakis, G. N. (2015). Cancer microenvironment and inflammation: Role of hyaluronan. Front. Immunol. 6, 169. doi:10.3389/fimmu.2015.00169
Nystrom, A. M., and Wooley, K. L. (2011). The importance of chemistry in creating well-defined nanoscopic embedded therapeutics: Devices capable of the dual functions of imaging and therapy. Acc. Chem. Res. 44, 969–978. doi:10.1021/ar200097k
Oberoi, H. S., Laquer, F. C., Marky, L. A., Kabanov, A. V., and Bronich, T. K. (2011). Core cross-linked block ionomer micelles as pH-responsive carriers for cis-diamminedichloroplatinum(II). J. Control. Release 153, 64–72. doi:10.1016/j.jconrel.2011.03.028
Paul, S., Kundu, P., Bhattacharyya, U., Garai, A., Maji, R. C., Kondaiah, P., et al. (2020). Ruthenium(II) conjugates of boron-dipyrromethene and biotin for targeted photodynamic therapy in red light. Inorg. Chem. 59, 913–924. doi:10.1021/acs.inorgchem.9b03178
Pirker, R. (2020). Chemotherapy remains a cornerstone in the treatment of nonsmall cell lung cancer. Curr. Opin. Oncol. 32, 63–67. doi:10.1097/CCO.0000000000000592
Ploemen, J. H., Van Schanke, A., Van Ommen, B., and Van Bladeren, P. J. (1994). Reversible conjugation of ethacrynic acid with glutathione and human glutathione S-transferase P1-1. Cancer Res. 54, 915–919.
Prasad, P. D., Wang, H., Huang, W., Fei, Y.-J., Leibach, F. H., Devoe, L. D., et al. (1999). Molecular and functional characterization of the intestinal Na+-Dependent multivitamin transporter. Arch. Biochem. Biophys. 366, 95–106. doi:10.1006/abbi.1999.1213
Presa, A., Brissos, R. F., Caballero, A. B., Borilovic, I., Korrodi-Gregório, L., Pérez-Tomás, R., et al. (2015). Photoswitching the cytotoxic properties of platinum(II) compounds. Angew. Chem. Int. Ed. Engl. 54, 4561–4565. doi:10.1002/anie.201412157
Rai, P., Mallidi, S., Zheng, X., Rahmanzadeh, R., Mir, Y., Elrington, S., et al. (2010). Development and applications of photo-triggered theranostic agents. Adv. Drug Deliv. Rev. 62, 1094–1124. doi:10.1016/j.addr.2010.09.002
Ramu, V., Gautam, S., Garai, A., Kondaiah, P., and Chakravarty, A. R. (2018). Glucose-Appended platinum(II)-BODIPY conjugates for targeted photodynamic therapy in red light. Inorg. Chem. 57, 1717–1726. doi:10.1021/acs.inorgchem.7b02249
Ramu, V., Gautam, S., Kondaiah, P., and Chakravarty, A. R. (2019). Diplatinum(II) catecholate of photoactive boron-dipyrromethene for lysosome-targeted photodynamic therapy in red light. Inorg. Chem. 58, 9067–9075. doi:10.1021/acs.inorgchem.9b00567
Rautio, J., Kumpulainen, H., Heimbach, T., Oliyai, R., Oh, D., Jarvinen, T., et al. (2008). Prodrugs: Design and clinical applications. Nat. Rev. Drug Discov. 7, 255–270. doi:10.1038/nrd2468
Raveendran, R., Braude, J. P., Wexselblatt, E., Novohradsky, V., Stuchlikova, O., Brabec, V., et al. (2016). Pt(iv) derivatives of cisplatin and oxaliplatin with phenylbutyrate axial ligands are potent cytotoxic agents that act by several mechanisms of action. Chem. Sci. 7, 2381–2391. doi:10.1039/c5sc04205d
Ravera, M., Gabano, E., Zanellato, I., Rangone, B., Perin, E., Ferrari, B., et al. (2021). Cis, cis, trans-[PtIVCl2(NH3)2(perillato)2], a dual-action prodrug with excellent cytotoxic and antimetastatic activity. Dalton Trans. 50, 3161–3177. doi:10.1039/d0dt04051g
Redasani, V. K., and Bari, S. B. (2015). Prodrug design: Perspectives, approaches and applications in medicinal chemistry. Cambridge, Massachusetts, United States: Academic Press.
Ricci, G., De Maria, F., Antonini, G., Turella, P., Bullo, A., Stella, L., et al. (2005). 7-Nitro-2, 1, 3-benzoxadiazole derivatives, a new class of suicide inhibitors for glutathione S-transferases: Mechanism of action of potential anticancer drugs. J. Biol. Chem. 280, 26397–26405. doi:10.1074/jbc.M503295200
Romero-Canelon, I., and Sadler, P. J. (2013). Next-generation metal anticancer complexes: Multitargeting via redox modulation. Inorg. Chem. 52, 12276–12291. doi:10.1021/ic400835n
Saisyo, A., Nakamura, H., Fang, J., Tsukigawa, K., Greish, K., Furukawa, H., et al. (2016). pH-sensitive polymeric cisplatin-ion complex with styrene-maleic acid copolymer exhibits tumor-selective drug delivery and antitumor activity as a result of the enhanced permeability and retention effect. Colloids Surf. B Biointerfaces 138, 128–137. doi:10.1016/j.colsurfb.2015.11.032
Saito, G., Swanson, J. A., and Lee, K.-D. (2003). Drug delivery strategy utilizing conjugation via reversible disulfide linkages: Role and site of cellular reducing activities. Adv. Drug Deliv. Rev. 55, 199–215. doi:10.1016/s0169-409x(02)00179-5
Sava, G., Zorzet, S., Turrin, C., Vita, F., Soranzo, M., Zabucchi, G., et al. (2003). Dual action of NAMI-A in inhibition of solid tumor metastasis: Selective targeting of metastatic cells and binding to collagen. Clin. Cancer Res. 9, 1898–1905.
Scaffidi-Domianello, Y. Y., Legin, A. A., Jakupec, M. A., Arion, V. B., Kukushkin, V. Y., Galanski, M. S., et al. (2011). Synthesis, characterization, and cytotoxic activity of novel potentially pH-sensitive nonclassical platinum(II) complexes featuring 1, 3-dihydroxyacetone oxime ligands. Inorg. Chem. 50, 10673–10681. doi:10.1021/ic2010612
Schafer, F. Q., and Buettner, G. R. (2001). Redox environment of the cell as viewed through the redox state of the glutathione disulfide/glutathione couple. Free Radic. Biol. Med. 30, 1191–1212. doi:10.1016/s0891-5849(01)00480-4
Schatzschneider, U. (2010). Photoactivated biological activity of transition-metal complexes. Eur. J. Inorg. Chem. 2010, 1451–1467. doi:10.1002/ejic.201000003
Schilder, R. J., Lacreta, F. P., Perez, R. P., Johnson, S. W., Brennan, J. M., Rogatko, A., et al. (1994). Phase I and pharmacokinetic study of ormaplatin (tetraplatin, NSC 363812) administered on a day 1 and day 8 schedule. Cancer Res. 54, 709–717.
Schmidt, C., Babu, T., Kostrhunova, H., Timm, A., Basu, U., Ott, I., et al. (2021). Are Pt(IV) prodrugs that release combretastatin A4 true multi-action prodrugs? J. Med. Chem. 64, 11364–11378. doi:10.1021/acs.jmedchem.1c00706
Shanmugaraju, S., La Cour Poulsen, B., Arisa, T., Umadevi, D., Dalton, H. L., Hawes, C. S., et al. (2018). Synthesis, structural characterisation and antiproliferative activity of a new fluorescent 4-amino-1, 8-naphthalimide Tröger's base-Ru(ii)-curcumin organometallic conjugate. Chem. Commun. 54, 4120–4123. doi:10.1039/c8cc01584h
Shi, H., Cheng, Q., Yuan, S., Ding, X., and Liu, Y. (2015). Human serum albumin conjugated nanoparticles for pH and redox-responsive delivery of a prodrug of cisplatin. Chemistry 21, 16547–16554. doi:10.1002/chem.201502756
Shi, H., Imberti, C., Clarkson, G. J., and Sadler, P. J. (2020a). Axial functionalisation of photoactive diazido platinum(iv) anticancer complexes. Inorg. Chem. Front. 7, 3533–3540. doi:10.1039/D0QI00685H
Shi, H., Imberti, C., Huang, H., Hands-Portman, I., and Sadler, P. J. (2020b). Biotinylated photoactive Pt(iv) anticancer complexes. Chem. Commun. 56, 2320–2323. doi:10.1039/c9cc07845b
Shi, H., Kasparkova, J., Soulié, C., Clarkson, G. J., Imberti, C., Novakova, O., et al. (2021). DNA-intercalative platinum anticancer complexes photoactivated by visible light. Chemistry 27, 10711–10716. doi:10.1002/chem.202101168
Shiau, C.-W., Huang, J.-W., Wang, D.-S., Weng, J.-R., Yang, C.-C., Lin, C.-H., et al. (2006). alpha-Tocopheryl succinate induces apoptosis in prostate cancer cells in part through inhibition of Bcl-xL/Bcl-2 function. J. Biol. Chem. 281, 11819–11825. doi:10.1074/jbc.M511015200
Stacpoole, P. W., Nagaraja, N. V., and Hutson, A. D. (2003). Efficacy of dichloroacetate as a lactate-lowering drug. J. Clin. Pharmacol. 43, 683–691. doi:10.1177/0091270003254637
Staderini, M., Martin, M. A., Bolognesi, M. L., and Menendez, J. C. (2015). Imaging of beta-amyloid plaques by near infrared fluorescent tracers: A new frontier for chemical neuroscience. Chem. Soc. Rev. 44, 1807–1819. doi:10.1039/c4cs00337c
Stella, V., Borchardt, R., Hageman, M., Oliyai, R., Maag, H., and Tilley, J. (2007). Prodrugs: Challenges and rewards. Berlin, Germany: Springer Science & Business Media.
Sun, H. J., Zhang, S., and Percec, V. (2015). From structure to function via complex supramolecular dendrimer systems. Chem. Soc. Rev. 44, 3900–3923. doi:10.1039/c4cs00249k
Sung, H., Ferlay, J., Siegel, R. L., Laversanne, M., Soerjomataram, I., Jemal, A., et al. (2021). Global cancer statistics 2020: GLOBOCAN estimates of incidence and mortality worldwide for 36 cancers in 185 countries. Ca. Cancer J. Clin. 71, 209–249. doi:10.3322/caac.21660
Suntharalingam, K., Song, Y., and Lippard, S. J. (2014). Conjugation of vitamin E analog α-TOS to Pt(IV) complexes for dual-targeting anticancer therapy. Chem. Commun. 50, 2465–2468. doi:10.1039/c3cc48740g
Taghizadeh, B., Taranejoo, S., Monemian, S. A., Salehi Moghaddam, Z., Daliri, K., Derakhshankhah, H., et al. (2015). Classification of stimuli-responsive polymers as anticancer drug delivery systems. Drug Deliv. 22, 145–155. doi:10.3109/10717544.2014.887157
Takamatsu, Y., and Inaba, T. (1992). Inhibition of human hepatic glutathione S-transferase isozymes by ethacrynic acid and its metabolites. Toxicol. Lett. 62, 241–245. doi:10.1016/0378-4274(92)90027-h
Tandon, R., Luxami, V., Kaur, H., Tandon, N., and Paul, K. (2017). 1, 8-naphthalimide: A potent DNA intercalator and target for cancer therapy. Chem. Rec. 17, 956–993. doi:10.1002/tcr.201600134
Tomczyk, M. D., and Walczak, K. Z. (2018). l, 8-Naphthalimide based DNA intercalators and anticancer agents. A systematic review from 2007 to 2017. Eur. J. Med. Chem. 159, 393–422. doi:10.1016/j.ejmech.2018.09.055
Townsend, D. M., and Tew, K. D. (2003). The role of glutathione-S-transferase in anti-cancer drug resistance. Oncogene 22, 7369–7375. doi:10.1038/sj.onc.1206940
Trondl, R., Heffeter, P., Kowol, C. R., Jakupec, M. A., Berger, W., and Keppler, B. K. (2014). NKP-1339, the first ruthenium-based anticancer drug on the edge to clinical application. Chem. Sci. 5, 2925–2932. doi:10.1039/c3sc53243g
Tutsch, K. D., Arzoomanian, R. Z., Alberti, D., Tombes, M. B., Feierabend, C., Robins, H. I., et al. (1999). Phase I clinical and pharmacokinetic study of an one-hour infusion of ormaplatin (NSC 363812). Invest. New Drugs 17, 63–72. doi:10.1023/a:1006223100561
Van Rijt, S. H., and Sadler, P. J. (2009). Current applications and future potential for bioinorganic chemistry in the development of anticancer drugs. Drug Discov. Today 14, 1089–1097. doi:10.1016/j.drudis.2009.09.003
Van Rixel, V. H. S., Ramu, V., Auyeung, A. B., Beztsinna, N., Leger, D. Y., Lameijer, L. N., et al. (2019). Photo-uncaging of a microtubule-targeted rigidin analogue in hypoxic cancer cells and in a xenograft mouse model. J. Am. Chem. Soc. 141, 18444–18454. doi:10.1021/jacs.9b07225
Van Rixel, V. H. S., Siewert, B., Hopkins, S. L., Askes, S. H. C., Busemann, A., Siegler, M. A., et al. (2016). Green light-induced apoptosis in cancer cells by a tetrapyridyl ruthenium prodrug offering two trans coordination sites. Chem. Sci. 7, 4922–4929. doi:10.1039/c6sc00167j
Venkatesh, V., and Sadler, P. J. (2018). Platinum(IV) prodrugs. Mater. Ions Life Sci. 18, 9783110470734. doi:10.1515/9783110470734-009
Walther, R., Rautio, J., and Zelikin, A. N. (2017). Prodrugs in medicinal chemistry and enzyme prodrug therapies. Adv. Drug Deliv. Rev. 118, 65–77. doi:10.1016/j.addr.2017.06.013
Wang, D., and Lippard, S. J. (2005). Cellular processing of platinum anticancer drugs. Nat. Rev. Drug Discov. 4, 307–320. doi:10.1038/nrd1691
Wang, H., Huang, W., Fei, Y.-J., Xia, H., Yang-Feng, T. L., Leibach, F. H., et al. (1999). Human placental Na+-dependent multivitamin transporter: Cloning, functional expression, gene structure, and chromosomal localization. J. Biol. Chem. 274, 14875–14883. doi:10.1074/jbc.274.21.14875
Wang, P., Yang, D., Zhang, H., Wei, X., Ma, T., Cheng, Z., et al. (2015a). Early detection of lung cancer in serum by a panel of MicroRNA biomarkers. Clin. Lung Cancer 16, 313–319. doi:10.1016/j.cllc.2014.12.006
Wang, T., Zabarska, N., Wu, Y., Lamla, M., Fischer, S., Monczak, K., et al. (2015b). Receptor selective ruthenium-somatostatin photosensitizer for cancer targeted photodynamic applications. Chem. Commun. 51, 12552–12555. doi:10.1039/c5cc03473f
Wang, X. (2010). Fresh platinum complexes with promising antitumor activity. Anticancer. Agents Med. Chem. 10, 396–411. doi:10.2174/1871520611009050396
Wang, X., and Guo, Z. (2013). Targeting and delivery of platinum-based anticancer drugs. Chem. Soc. Rev. 42, 202–224. doi:10.1039/c2cs35259a
Wang, Y., Li, J., Feng, L., Yu, J., Zhang, Y., Ye, D., et al. (2016). Lysosome-targeting fluorogenic probe for cathepsin B imaging in living cells. Anal. Chem. 88, 12403–12410. doi:10.1021/acs.analchem.6b03717
Wexselblatt, E., and Gibson, D. (2012). What do we know about the reduction of Pt(IV) pro-drugs? J. Inorg. Biochem. 117, 220–229. doi:10.1016/j.jinorgbio.2012.06.013
Wolinsky, J. B., and Grinstaff, M. W. (2008). Therapeutic and diagnostic applications of dendrimers for cancer treatment. Adv. Drug Deliv. Rev. 60, 1037–1055. doi:10.1016/j.addr.2008.02.012
Wu, Y., Li, S., Chen, Y., He, W., and Guo, Z. (2022). Recent advances in noble metal complex based photodynamic therapy. Chem. Sci. 13, 5085–5106. doi:10.1039/d1sc05478c
Xia, Y., Wang, Y., Wang, Y., Tu, C., Qiu, F., Zhu, L., et al. (2011). A tumor pH-responsive complex: Carboxyl-modified hyperbranched polyether and cis-dichlorodiammineplatinum(II). Colloids Surf. B Biointerfaces 88, 674–681. doi:10.1016/j.colsurfb.2011.07.059
Xu, Y., Li, C., Lu, S., Wang, Z., Liu, S., Yu, X., et al. (2022). Construction of emissive ruthenium(II) metallacycle over 1000 nm wavelength for in vivo biomedical applications. Nat. Commun. 13, 2009. doi:10.1038/s41467-022-29572-2
Yang, J., Sun, X., Mao, W., Sui, M., Tang, J., and Shen, Y. (2012). Conjugate of Pt(IV)-histone deacetylase inhibitor as a prodrug for cancer chemotherapy. Mol. Pharm. 9, 2793–2800. doi:10.1021/mp200597r
Yang, K., Feng, L., and Liu, Z. (2016). Stimuli responsive drug delivery systems based on nano-graphene for cancer therapy. Adv. Drug Deliv. Rev. 105, 228–241. doi:10.1016/j.addr.2016.05.015
Zak, F., Turanek, J., Kroutil, A., Sova, P., Mistr, A., Poulova, A., et al. (2004). Platinum(IV) complex with adamantylamine as nonleaving amine group: Synthesis, characterization, and in vitro antitumor activity against a panel of cisplatin-resistant cancer cell lines. J. Med. Chem. 47, 761–763. doi:10.1021/jm030858+
Zelzer, M., Todd, S. J., Hirst, A. R., Mcdonald, T. O., and Ulijn, R. V. (2013). Enzyme responsive materials: Design strategies and future developments. Biomater. Sci. 1, 11–39. doi:10.1039/c2bm00041e
Zeng, L., Gupta, P., Chen, Y., Wang, E., Ji, L., Chao, H., et al. (2017a). The development of anticancer ruthenium(ii) complexes: From single molecule compounds to nanomaterials. Chem. Soc. Rev. 46, 5771–5804. doi:10.1039/c7cs00195a
Zeng, L., Kuang, S., Li, G., Jin, C., Ji, L., and Chao, H. (2017b). A GSH-activatable ruthenium(ii)-azo photosensitizer for two-photon photodynamic therapy. Chem. Commun. 53, 1977–1980. doi:10.1039/c6cc10330h
Zeng, X., Wang, Y., Han, J., Sun, W., Butt, H.-J., Liang, X.-J., et al. (2020). Fighting against drug-resistant tumors using a dual-responsive Pt(IV)/Ru(II) bimetallic polymer. Adv. Mat. 32, 2004766. doi:10.1002/adma.202004766
Zhang, K. Y., Liu, S., Zhao, Q., and Huang, W. (2016). Stimuli–responsive metallopolymers. Coord. Chem. Rev. 319, 180–195. doi:10.1016/j.ccr.2016.03.016
Zhang, P., and Sadler, P. J. (2017). Redox-active metal complexes for anticancer therapy. Eur. J. Inorg. Chem. 2017, 1541–1548. doi:10.1002/ejic.201600908
Zhang, W., Li, Y., Sun, J.-H., Tan, C.-P., Ji, L.-N., and Mao, Z.-W. (2015). Supramolecular self-assembled nanoparticles for chemo-photodynamic dual therapy against cisplatin resistant cancer cells. Chem. Commun. 51, 1807–1810. doi:10.1039/c4cc08583c
Zhang, X., Li, X., You, Q., and Zhang, X. (2017). Prodrug strategy for cancer cell-specific targeting: A recent overview. Eur. J. Med. Chem. 139, 542–563. doi:10.1016/j.ejmech.2017.08.010
Zheng, Y. R., Suntharalingam, K., Johnstone, T. C., Yoo, H., Lin, W., Brooks, J. G., et al. (2014). Pt(IV) prodrugs designed to bind non-covalently to human serum albumin for drug delivery. J. Am. Chem. Soc. 136, 8790–8798. doi:10.1021/ja5038269
Keywords: stimuli-responsive, metallic prodrugs, lung cancer, Pt anticancer drugs, Ru anticancer drugs
Citation: Zhang C, Kang T, Wang X, Song J, Zhang J and Li G (2022) Stimuli-responsive platinum and ruthenium complexes for lung cancer therapy. Front. Pharmacol. 13:1035217. doi: 10.3389/fphar.2022.1035217
Received: 02 September 2022; Accepted: 04 October 2022;
Published: 17 October 2022.
Edited by:
Yi Gou, Guilin Medical University, ChinaReviewed by:
Wukun Liu, Nanjing University of Chinese Medicine, ChinaMing Jiang, Guangxi Science and Technology Normal University, China
Copyright © 2022 Zhang, Kang, Wang, Song, Zhang and Li. This is an open-access article distributed under the terms of the Creative Commons Attribution License (CC BY). The use, distribution or reproduction in other forums is permitted, provided the original author(s) and the copyright owner(s) are credited and that the original publication in this journal is cited, in accordance with accepted academic practice. No use, distribution or reproduction is permitted which does not comply with these terms.
*Correspondence: Jia Zhang, emhhbmdqaWF4anR1QHhqdHUuZWR1LmNu; Guanying Li, Z3VhbnlpbmdsaUB4anR1LmVkdS5jbg==