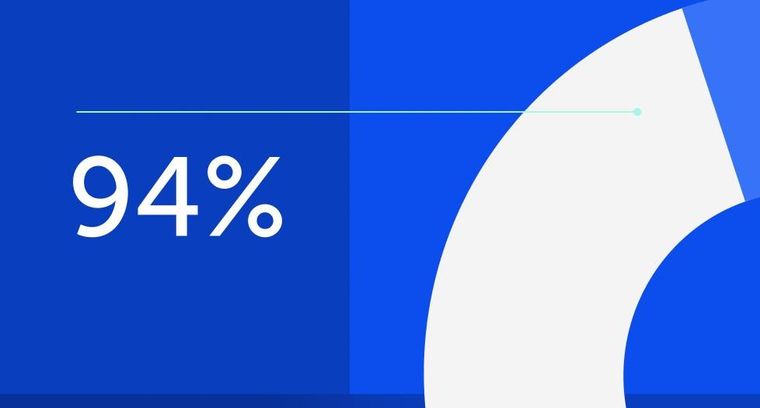
94% of researchers rate our articles as excellent or good
Learn more about the work of our research integrity team to safeguard the quality of each article we publish.
Find out more
ORIGINAL RESEARCH article
Front. Pharmacol., 23 November 2022
Sec. Neuropharmacology
Volume 13 - 2022 | https://doi.org/10.3389/fphar.2022.1023611
This article is part of the Research TopicInterplay Between Chronic Pain and Affective-Cognitive Alterations: Shared Neural Mechanisms, Circuits, and TreatmentView all 10 articles
Spinal α2-adrenoceptor induces analgesia by neuronal inhibition of primary afferent fibers. This family receptor coupled to Gi/o proteins can be subdivided into three functional subtypes: α2A, α2B, and α2C-adrenoceptors, and current evidence on spinal analgesia supports the relevance of α2A and seems to exclude the role of α2B, but the functional contribution of α2C-adrenoceptors remains elusive. The present study was designed to pharmacologically dissect the contribution of spinal α2-adrenoceptor subtypes modulating tonic or acute peripheral nociception. Using male Wistar rats, we analyzed the effect of spinal clonidine (a non-selective α2A/α2B/α2C-adrenoceptor agonist) and/or selective subtype α2-adrenoceptor antagonists on: 1) tonic nociception induced by subcutaneous formalin (flinching behavior) or 2) acute nociception induced by peripheral electrical stimulus in in vivo extracellular recordings of spinal dorsal horn second-order wide dynamic range (WDR) neurons. Clonidine inhibited the nocifensive behavior induced by formalin, an effect blocked by BRL 44408 (α2A-adrenoceptor antagonist) but not by imiloxan (α2B-adrenoceptor antagonist) or JP 1302 (α2C-adrenoceptor antagonist). Similarly, spinal BRL 44408 reversed the clonidine-induced inhibition of nociceptive WDR activity. Interestingly, spinal JP 1302 per se produced behavioral antinociception (an effect blocked by bicuculline, a preferent GABAA channel blocker), but no correlation was found with the electrophysiological experiments. These data imply that, at the spinal level, 1) presynaptic α2A-adrenoceptor activation produces antinociception during acute or tonic nociceptive stimuli; and 2) under tonic nociceptive (inflammatory) input, spinal α2C-adrenoceptors are pronociceptive, probably by the inactivation of GABAergic transmission. This result supports a differential role of α2A and α2C-adrenoceptors modulating nociception.
At the spinal dorsal horn level, α2-adrenoceptor activation has been related to the inhibition of neural transmission and analgesic actions (Pertovaara, 2006; Bahari and Meftahi, 2019). From a mechanistic perspective, activation of this receptor which is canonically coupled to Gi/o proteins, inhibits adenylyl cyclase, inactivates Ca2+ channels, and enhances inwardly rectifying K+ channel activity, leading to neuronal hyperpolarization with a diminution of neural transmission (Pan et al., 2008). In vivo electrophysiological recordings of second-order wide dynamic range (WDR) cells in the spinal dorsal horn have shown that spinal clonidine (α2-adrenoceptor agonist) selectively inhibits the neuronal activity of nociceptive primary afferent fibers (Sullivan et al., 1987). As reviewed by Pertovaara (2013), agonists to this receptor consistently produce antinociception in behavioral and electrophysiological experiments. Indeed, intrathecal clonidine has been successfully used in humans as a potent analgesic (Eisenach et al., 1996; Yaksh et al., 2017; Schwartz et al., 2022).
However, we must keep in mind that α2-adrenoceptors have been divided into three functional subtypes, namely α2A, α2B, and α2C (Bylund et al., 1994), and researchers have attempted to dissect how these receptor subtypes contribute to spinal pain transmission. Briefly, molecular assays suggest that the three receptor subtypes are expressed in the spinal cord and dorsal root ganglion cells (Aoki et al., 1994; Stone et al., 1998; Nicholson et al., 2005), and current functional evidence supports the notion that α2A-adrenoceptor activation plays a key role in spinal antinociception, whereas α2B-adrenoceptors seem not to contribute to spinal antinociception (for refs., see Pertovaara, 2006; Pertovaara, 2013). In contrast, in the case of α2C-adrenoceptors, although Fairbanks et al. (2002) suggest that spinal activation of this subtype receptor plays an antinociceptive role, the evidence offered by Malmberg et al. (2001) refuses these data showing that this receptor does not play any role in nociception. In both cases, the experiments were performed using transgenic mice and non-selective ligands. Hence, the function of this subtype receptor remains obscure.
Most importantly, although current literature supports the notion that the main effect of α2-adrenoceptors is inhibition of nociceptive transmission, some in vitro/in vivo experiments suggest that noradrenergic transmission at the spinal cord level could also be pronociceptive (Hantman and Perl, 2005; Chen et al., 2008; Gassner et al., 2009; Hickey et al., 2014; Kucharczyk et al., 2022). For example, using spinal cord slices and electrophysiological recordings (patch-clamp) at lamina II/III, it has been shown that some GABAergic neurons can be hyperpolarized by noradrenaline via α2-adrenoceptor activation (Gassner et al., 2009); in this study, the subtype receptor was not analyzed. Furthermore, also in spinal cord slices, but using immunohistochemistry, Chen et al. (2008) showed that α2C-adrenoceptor activation inhibits the veratridine-induced opioid release. These in vitro data may imply that α2C-adrenoceptors may play a pronociceptive role. In any case, the functional role of α2C-adrenoceptors modulating spinal nociception has been hampered (contradictory) in part by the lack of selective ligands used.
On this basis, the present study aimed to functionally dissect α2A/2B/2C-adrenoceptors in spinal nociception. Here, we analyzed the effect of spinal clonidine (a non-selective α2A/α2B/α2C-adrenoceptor agonist) and/or selective subtype α2A/α2B/α2C -adrenoceptor antagonists (see Table 1) on: 1) tonic nociception (flinching behavior) induced by subcutaneous formalin or 2) acute nociception induced by peripheral electrical stimulus in in vivo extracellular recordings of spinal dorsal horn second-order wide dynamic range (WDR) neurons. The data showed that the presynaptic α2A-adrenoceptor produces robust antinociception during acute or tonic nociceptive stimuli. In contrast, under a tonic nociceptive stimulus, α2C-adrenoceptors seem to induce nociception by inactivating GABAergic transmission.
TABLE 1. Binding affinity constants for the α2A-, α2B-, and α2A-adrenoceptors of clonidine and the antagonists used in the present study. The nanomoles (and their equivalents in μg) spinally administered in the current experiments are also shown.
A total of 115 male Wistar rats (295 ± 15 g) from the Neurobiology Institute Animal House were used in the present experiments. Rats were divided into two main sets (80 rats for behavioral tests and 35 rats for electrophysiological recordings; see Figure 1). The rodents were housed in the satellite bioterium of our laboratory on a 12:12 h light and dark cycle (lights on at 07:00 h) at constant temperature (22°C ± 2°C) and humidity (50%) with food and water ad libitum. All experimental procedures were performed during the light phase of the cycle (10:00–19:00 h). Furthermore, all animal protocols in this investigation were approved by our Institutional Ethics Committee, following the Guide for the Care and Use of Laboratory Animals in the United States (NIH publication 86-23), IASP ethical guidelines (Zimmermann, 1983), and ARRIVE guidelines for reporting experiments involving animals (McGrath et al., 2010). At the end of the experiments, the animals were halted in a CO2 chamber (formalin test) or by an overdose of pentobarbital (electrophysiological experiments).
FIGURE 1. Experimental protocols showing the number of animals used in the present study. The animals were divided into two main sets to perform behavioral or in vivo electrophysiological recordings.
Chronic catheterization of the intrathecal (i.t.) subarachnoid space was performed as described by Yaksh and Rudy (1976). The rats were anesthetized with a ketamine-xylazine (75–10 mg/kg, i.p.) and placed in a stereotaxic frame (Kopf Instruments, United States) with the dorsal part of the head and neck previously shaved. The atlanto-occipital membrane was exposed and pierced, and a polyethylene catheter (PE-10, 9.0 cm length) was inserted intrathecally and advanced caudally to the level of the thoracolumbar junction. The wound was then sutured, and the animals were housed in individual cages to recover from surgery for 5 days before the formalin test.
The tonic nociception experiments using the 1% formalin test (Dubuisson and Dennis, 1977; Wheeler-Aceto and Cowan, 1991) were performed by the same tester, blinded to the pharmacological treatment. Rats were placed in open Plexiglass® observation chambers for 30 min for three consecutive days to allow them to become familiar with their surroundings. On the third day, and after 30 min in the Plexiglass® chamber, they were removed for formalin administration. Fifty microliters of diluted formalin (1%) were subcutaneously (s.c.) injected into the dorsal surface of the right hind paw with a 30-gauge needle. The animals were then returned to the chambers, and nocifensive behavior was observed immediately after the formalin injection. Nocifensive behavior was quantified as the number of flinches of the injected paws during 1 min periods every 5 min for up to 1 h after injection. Flinching was characterized as a rapid and brief withdrawal or as a flexing of the injected paw. As previously reported, formalin-induced flinching behavior was biphasic. The initial nociceptive phase (0–10 min) was followed by a prolonged, persistent response (15–60 min). For i.t., administration, the α2A/2B/2C-adrenoceptor agonist (clonidine) or selective antagonist (BRL 44408 to α2A-, imiloxan to α2B-, or JP 1302 to α2C-adrenoceptors) were given in a volume of 10 μl using a Hamilton® syringe.
The first group (n = 20 rats) was subdivided into four subgroups and received an i.t., injection of vehicle (saline solution, 0.9% NaCl; n = 5) or clonidine (0.1, 1 or 10 nmol; n = 5 rats each) 10 min before formalin injection. A second group (n = 25 rats) was subdivided into five subgroups to measure the per se effect of vehicle (n = 5) or the antagonists, BRL 44408 (0.1 or 1 nmol; n = 5 rats each), imiloxan (10 nmol, n = 5) or JP 1302 (1 nmol; n = 5 rats each) given 20 min before formalin injection.
To determine whether clonidine-induced intrathecal antinociception was mediated by either α2A-, α2B-, or α2C-adrenoceptors, a third group was used (n = 25 rats). In this case, the effect of pretreatment (10 min before 1 nmol clonidine) with vehicle (n = 5), BRL 44408 (0.1 or 1 nmol; n = 5 rats each), imiloxan (10 nmol; n = 5) or JP 1302 (1 nmol; n = 5) on the formalin-induced flinches was assessed.
Since JP 1302 alone inhibited the formalin-induced nociception, the involvement of GABAergic mechanisms was suspected (see discussion section). To test this hypothesis, 0.3 nmol bicuculline was given 10 min before JP 1302 (n = 5 rats; 1 nmol). Also, the per se effect of bicuculline on formalin flinches was assessed (n = 5 rats).
Doses and drug administration schedules for clonidine, BRL 44408, and imiloxan were selected based on previous reports (O’Neill and Haigler, 1985; Young et al., 1989; Omote et al., 1991; Uhlén et al., 1994; Erne-Brand et al., 1999; Dwyer et al., 2010) and pilot experiments in our laboratory. Behavioral or motor function changes induced by the different treatments were assessed in catheterized rats from all groups by testing the animals’ ability to stand and walk in a normal posture, as proposed elsewhere (Chen and Pan, 2001).
Animals were anesthetized with urethane (2 g/kg), and then an intratracheal cannula was inserted for artificial ventilation (65–75 strokes/min; model Ro Vent®, Kent Scientific Corp. United States). Subsequently, animals were mounted onto a stereotaxic frame (Kopf Instruments, United States) and secured in a spinal cord unit frame. The lumbar vertebrae were fixed to improve stability at the recording site to perform a laminectomy to expose the L2-L4 spinal cord segments. The dura was carefully removed, and the exposed spinal cord was covered with isotonic saline to avoid desiccation. The animals were not paralyzed, and we did not observe any withdrawal response during peripheral electrical stimulation. End-tidal CO2 was monitored with a Capstar-100 End-tidal CO2 analyzer (CWE Inc., United States) and kept between 3.0% and 3.5% by adjusting the stroke volume. Core body temperature was maintained at 38°C using a circulating water pad.
Extracellular unitary recordings were made using seven quartz-Pt/W microelectrodes (impedance 4–7 MΩ) mounted in a multichannel microdrive with an integrated preamplifier. This multi-electrode system was manipulated with the 7-channel version of the fiber-electrode manipulator “System Eckhorn” using Eckhorn Matrix multiuser software (Thomas Recording GmbH, Germany). The microelectrodes were lowered (400–900 μm from the surface) in small steps (2–5 μm/s) into the superficial laminae of the left dorsal horn segments to search for single-unit discharges. For each recorded cell, the specific somatic receptive field (RF) was located by gently tapping on the entire ipsilateral glabrous surface of the hind paw. When we found a RF, electrical stimulation using an S88 stimulator (Grass Instruments Co., United States) was then applied. In this case, two needles (27 G) attached to a stimulus isolator unit (PSIU6 model, Grass Instruments Co., United States) were subcutaneously inserted into the RF of the recorded neuron. The electrical stimulation was then conducted and consisted of 20 stimuli at 0.2 Hz with a 1 ms pulse duration at 1.5 times the threshold intensity (0.1–3 mA) required to evoke a C-fiber discharge. It is interesting to note that using this protocol, the spikes associated with activation of Aβ-, Aδ-, C-fibers, and post-discharge can be observed, but the wind-up was not consistently elicited.
The extracellular neuronal activity induced by electrical stimulation of the RF was amplified ×100 (1700 Differential AC amplifier, A-M Systems, United States), digitalized, and discriminated using CED hardware and Spike 2 software (v5.15; Cambridge Electronic Design, United Kingdom). Raw and discriminated signals were fed through an audio monitor (model 3300, A-M Systems, United States) and displayed on an oscilloscope (TBS1064, Tektronix Inc., United States). Waveforms and recorded spike trains were stored on a hard drive for offline analysis. Evoked activities of the spinal dorsal horn WDR neurons were recorded and analyzed as cumulative frequency and peri-stimulus time histograms (PSTH) to detect the occurrence of neuronal responses. On this basis, the stimulating threshold to evoke action potentials and their frequency of occurrence, resulting from the stimulation of the peripheral RF on the hind paw, were attributed to the recruitment of Aβ-, Aδ-, and C-fibers. Considering the distance between the RF and the recording electrode, the peak latencies observed correspond to peripheral conduction velocities within the Aβ-, (0–20 ms), Aδ- (21–90 ms), C-fibers (90–350 ms), and post-discharge (350–800 ms) (Urch & Dickenson, 2003; González-Hernández et al., 2019; Gamal-Eltrabily et al., 2020). By using this protocol, we exclusively recorded WDR cells, a type of second-order neurons receiving input concomitantly from non-nociceptive (Aβ-type) and nociceptive (Aδ- and C-type) fibers; in addition, post-discharge was also analyzed.
Indeed, during the search of WDR cells responding to peripheral tactile and electrical RF stimulation, some neurons can be classified as only tactile sensitive and, in minor proportion, nociceptive specific; but these types of cells were not further analyzed. Thus, the number of action potentials in response to 20 RF stimuli was compared before (basal, t = 0) and after treatments. In these experiments, a control group without treatment was used (n = 5).
Accordingly, the neuronal evoked responses were evaluated immediately after (basal; t = 0) clonidine (1 or 10 nmol; n = 5 cells, each) or vehicle (isotonic saline, 0.9% NaCl; n = 5 cells) administration and at 10, 20, 30, 40, 50, and 60-min post-treatment. Since in the formalin test, clonidine-induced flinching inhibition was reversed by BRL 44408 but not by imiloxan or JP 1302, the role of the α2A-adrenoceptor was evaluated by spinal administration of BRL 44408 (1 nmol; n = 5 cells; given 10 min prior clonidine). Furthermore, considering that JP 1302 exhibited antinociceptive effects in the formalin test, we tested the effects of this α2C-adrenoceptor antagonist (1 or 10 nmol; n = 5 cells, each) on the electrophysiological responses of WDR cells. The vehicle and compounds were given at the spinal cord level (topical) in a total volume of 10 μl using a Hamilton® syringe.
This study used the following compounds besides the anesthetics (ketamine, xylazine, and urethane). From Sigma Chemical Co., (United States): 1) clonidine hydrochloride (CAS number: 4205-91-8), 2) 2-[2H-(1-methyl-1,3-dihydroisoindole) methyl]-4,5-dihydroimidazole maleate (BRL 44408; CAS number: 118343-19-4), 3) 2-(1-ethyl-2-indazoyl) methyl-1,4-benzodioxan hydrochloride (imiloxan; CAS number: 81167-22-8) and 4) (-)-bicuculline methiodide (CAS number: 40709-69-1). The N-[4-(4-methyl-1-piperazinyl)phenyl]-9-acridinamine dihydrochloride (JP-1302; CAS number: 1259314-65-2) was acquired from Tocris Ltd., (United States). The doses of clonidine, BRL 44408, imiloxan, and JP 1302 refer to their free base, whereas those of the bicuculline refer to their salt (methiodide). Furthermore, in Table 1, the doses in nmol and μg is given. All drugs were dissolved in a physiological saline solution (0.9% NaCl).
The data in the figures are presented as mean ± S.E.M., (standard error of the mean). In all cases and before performing a parametric statistical analysis, we checked for normality using the Shapiro-Wilk test (p > 0.05). In the formalin test, curves were constructed by plotting the number of flinches as a function of time. The area under the number of flinches against time curves (AUC), an expression of the duration and intensity of the effect, was calculated by the trapezoidal rule, and a one-way analysis of variance (ANOVA) was performed.
For electrophysiological experiments, a baseline neuronal response was established after an identified neuron had a ≤10% variation in the neuronal responses induced by RF stimulation during five consecutive tests (5 min between each trial). The number of basal (t = 0) evoked potential (total spikes and number of Aβ-, Aδ-, C-fibers and post-discharge) in the different experimental groups were analyzed; since the normality test failed, a Kruskal-Wallis one-way analysis of variance (ANOVA) on ranks was performed (see Table 2). On this basis, and to normalize the data, the evoked potentials induced by electrical stimulation of the paw were expressed as a percentage change from the respective baseline. Thus, the baseline value refers to the evoked neuronal response before spinal treatment with clonidine or antagonists. To evaluate the stability of the recorded neurons (only for the control group and vehicle group) across the 60-min time frame, we used a one-way repeated measures ANOVA. The difference in neuronal activity evoked within one group of animals before and after treatments was compared using a two-way repeated-measures ANOVA. In addition, the temporal course was adjusted to obtain global neuronal activity due to the treatment (box and whisker plots); in this case, a one-way ANOVA was performed. The ordinary one-way ANOVA was followed (if applicable) by the Newman-Keuls post-hoc test, whereas in the case of the two-way repeated-measures ANOVA, Holm-Sidak’s multiple comparison test was followed. Furthermore, sphericity was not assumed in the case of repeated measures ANOVAs, and corrections to degrees of freedom were made according to the Greenhouse-Geisser method. Differences were considered statistically significant when p < 0.05. Graphs and statistical analysis were done using GraphPad Prism V6.0 software (United States). Complete statistical analysis is detailed in Tables 2, 3.
TABLE 2. Mean action potentials elicited (±s.e.m.) by twenty electrical stimuli at the basal time (t = 0) in the different experimental groups. Since the normality test failed (Shapiro-Wilk), a Kruskal-Wallis one-way ANOVA on ranks was performed to compare the action potential elicited by the different treatments.
TABLE 3. Ordinary one-way or two-way repeated-measures analysis of variance (ANOVA) with their respective post hoc comparison for each figure.
Subcutaneous (s.c.) formalin injection into the right hind paw produced a typical flinching behavior characterized by a biphasic time course (Figure 2A; control curve). Phase I of the nociceptive response began immediately after formalin injection and gradually declined (≈10 min). Then, phase II started about 15 min after formalin injection and lasted 1 h. I.t administration of clonidine (0.1, 1, and 10 nmol) inhibited the formalin-induced flinching behavior during phases I and II (Figures 2A–C).
FIGURE 2. Intrathecal (i.t.) clonidine (α2A/2B/2C-adrenoceptor agonist) inhibits behavioral nociception. (A) Flinching number over time during phase I (P1) and phase II (P2) of the mean number per minute of flinches observed after i.t., clonidine (0.1, 1, and 10 nmol; n = 5 each dose) in rats submitted to the 1% formalin test (50 μl, s.c.). (B,C) show the time course data expressed as the area under the mean number of flinches against the time curve (AUC). Clonidine reduced AUC values during both phases (P1, P2), indicating an antinociceptive effect. Clonidine was given 10 min prior to formalin injection.
As shown in Figure 3A, the antinociception induced by 1 nmol clonidine was attenuated by 1 nmol BRL 44408 and remained unaffected by 0.1 nmol BRL 44408, 10 nmol imiloxan, or 1 nmol JP 1302. In particular, 1 nmol BRL 44408 partially reverted the clonidine effect during phase I (Figure 3B) and abolished the clonidine-induced antinociception during phase II (Figure 3C).
FIGURE 3. Role of spinal α2A-adrenoceptors in the clonidine-induced behavioral antinociception and the antinociceptive effect of JP 1302 (α2C-adrenoceptor antagonist). (A) shows the effect of intrathecal (i.t.) injection of vehicle (10 μl; isotonic saline solution) or α2A/2B/2C-adrenoceptor antagonists on the clonidine-induced inhibition of the flinches induced by formalin (1%; 50 μl, s.c.) during phase I (P1) and phase II (P2). Vehicle, BRL 44408 (0.1 or 1 nmol; an α2A-adrenoceptor antagonist; n = 5 each dose), imiloxan (10 nmol; an α2B-adrenoceptor antagonist; n = 5), or JP 1302 (1 nmol; an α2C-adrenoceptor antagonist; n = 5) were given i.t. 10 min prior clonidine. (B,C) represent the data as the area under the mean number of flinches against the curve (AUC) and show that BRL 44408 (1 nmol) inhibited the clonidine-induced antinociception in both phases (P1, P2). During P2, JP 1302 seems to enhance (statistically non-significant, p > 0.05) the antinociception induced by clonidine. (D) shows the per se effect of BRL 44408 (n = 5 each dose), imiloxan (n = 5), or JP 1302 (n = 5 each dose) in rats submitted to the 1% formalin test. The AUC in (E,F) suggest that BRL 44408 and imiloxan do not affect formalin-induced nociception. In contrast, JP 1302 diminishes the AUC values during both phases (P1, P2), suggesting an antinociceptive effect.
When we tested the per se effects induced by the antagonists (Figure 3D), we found that i.t. administration of vehicle (0.9% NaCl solution, 10 µl), BRL 44408 (0.1, 1 nmol) or imiloxan (10 nmol) did not have a statistical difference (see Table 2 for details) on the flinching behavior induced by formalin; in contrast, i.t. JP 1302 significantly reduced the number of flinches during phase I and II (Figures 3E,F).
Since the behavioral experiments showed that clonidine inhibits the formalin-induced nociception via α2A-adrenoceptor activation, a set of electrophysiological recordings of the second-order WDR dorsal horn spinal cord cells were performed to correlate the behavioral outcome with electrophysiological responses.
Figure 4A illustrates the experimental setup used to perform the in vivo unitary extracellular recordings of spinal WDR cells. In this figure, the recordings correspond to the baseline neuronal response (t = 0) elicited by twenty electrical pulses given in the paw receptive field. All neurons recorded in the present experiments were found at an average of 700 ± 192 μm from the spinal surface. As illustrated in Figures 4B,C, the peripheral electrical stimulation produces a well-defined and stereotyped WDR cell response. In general, under our parameters of electrical stimulation used (0.2 Hz; 1 ms pulse duration), we observed that although some cells exhibited high post-discharge events (see Cell 1), an inconsistent wind-up was elicited (calculated accordingly to the formula given by Gjerstad et al., 1996). As previously reported, wind-up is considered as a facilitation of neural discharge evoked by repetitive stimulation of primary afferent fibers (Mendell, 1966). This process elicited in WDR cells seems to mainly depend on the frequency stimulation, and in general, frequencies >0.5 Hz are required to consistently produce a wind-up (Mendell, 1966; Dickenson and Sullivan, 1987; Chapman and Dickenson, 1992; Li et al., 1999; You et al., 2003); in this sense, 0.2 Hz seems not to be enough to recruit a facilitatory input leading to wind-up.
FIGURE 4. Effect of peripheral electrical stimulation on the WDR cell response. (A) Experimental set-up design illustrating the site of electrophysiological recording of WDR cells at spinal L2-L4 segments, and the location, in the ipsilateral paw of the electrical stimulation applied to the receptive field (RF). (B) Raw data of twenty stimulus artifacts and (C) raw data of consecutive WDR responses to RF stimulation in two different cells (Cell 1 and Cell 2). (D) shows the raw tracing of a single second-order WDR neuron cluster response induced by one electrical stimulus-evoked in Cell 1 (left) or Cell 2 (right). (E) shows the raster plot and (F) peri-stimulus time histograms (PSTH) constructed from twenty WDR neuronal responses to RF stimulation depicting the different fiber components (Aβ-, Aδ-, C-fibers, and post-discharge). Note that, under our experimental condition, no clear wind-up is elicited. (G) shows that the basal response, i.e., the total of action potentials evoked at t = 0 in the different experimental groups, is not statistically different (χ2 = 4.535; p = 0.605).
Furthermore, at the basal time (t = 0), the mean average evoked potential of all animals tested was 667 ± 29 spikes. Since the evoked potential did not follow a normal distribution along the experimental groups, a non-parametric test was used, and we found no statistically significant difference (see Figure 4G; Table 2 for details). The following results were normalized to perform a parametric statistical analysis.
Since we recorded spinal WDR cells for 60 min, analyzing the effect of time on the neuronal responses was crucial to exclude a time-dependent effect. The one-way ANOVA suggests that no time-dependent changes in neuronal responses occurred during our experimental protocols. Specifically, in the control group, time had no effect on Aδ- (F(2.73, 10.90) = 0.213; p = 0.869) or C-fibers (F(2.09, 8.36) = 1.92; p = 0.197). Similar results were obtained in the case of vehicle administration [Aδ- (F(2.68, 10.76) = 0.204; p = 0.869) and C-fibers (F(2.27, 9.09) = 0.892; p = 0.455)].
Figure 5 shows the raw tracing of a single WDR neuron cluster response, the raster plots, and the PSTHs before and after different treatments. Figure 5A shows the PSTHs obtained during the control condition, whereas Figures 5B,C exemplify the neural activity of second-order WDR cells before (basal response) and after clonidine treatment. In contrast to the control group (Figure 5A), spinal clonidine, particularly at 10 nmol (Figure 5C), diminished the neuronal firing responses (events) elicited by the RF electrical stimulation. We must clarify that each PSTH represents the neuronal evoked activity of one WDR cell induced by twenty electrical pulses; these evoked neuronal events could be broken down according to the conduction velocities of the primary afferent fibers (i.e., Aβ-, Aδ-, and C-fibers).
FIGURE 5. Spinal clonidine selectively blocks the nociceptive neuronal firing of WDR cells. (A–C) depict the raw tracing of a single second-order WDR neuron cluster response induced by one electrical stimulus (upper tracing), the raster plot (middle), and peri-stimulus time histograms (PSTH) constructed from 20 WDR responses to receptive field (RF) electrical stimulation before (basal) and after treatment. In accordance with the fiber conduction velocities, the panels in the figure depict the different fiber components (Aβ-, Aδ-, C-fibers, and post-discharge) of the spinal WDR cell response. Note that clonidine (1 and 10 nmol) diminished the neuronal activity; this clonidine-induced inhibition (observed as a diminution of events) is mainly associated with neural action on nociceptive fibers (i.e., Aδ- and C-fibers) and ongoing (post-discharge) activity, whereas the firing of Aβ-fibers remains unaltered.
In this set of experiments, we found that BRL 44408 reversed the antinociception induced by clonidine. Figure 6A shows an example of a raw tracing of a single WDR neuron cluster response, the raster plots, and PSTH obtained in an animal pre-treated with BRL 44408 before clonidine administration. Note that BRL 44408 blocked the clonidine-induced diminution of WDR neuronal activity.
FIGURE 6. The role of spinal α2A-adrenoceptors in clonidine-induced electrophysiological antinociception. In (A), a pair of raw tracings of a single second-order WDR neuron cluster response induced by one electrical stimulus (upper tracing), the raster plot (middle), and peri-stimulus time histogram (PSTH) depicting the effect of BRL 44408 on the clonidine-induced antinociception. Notice that under α2A-adrenoceptors blockade with BRL 44408 (1 nmol), the antinociceptive action of clonidine is not elicited. (B–E) show the time-course changes in the percentage average of the different fibers activating WDR cell responses and post-discharge elicited by receptive field (RF) electrical stimulation and the effects of spinal clonidine (1 and 10 nmol/10 μl; n = 5 each dose). Notice that 1 nmol clonidine only inhibits the neuronal firing of Aδ-fibers (C), while 10 nmol clonidine inhibits the activity of Aδ-fibers (C), C-fibers (D), and post-discharge (E). (F–I) show the global neuronal activity (obtained from the respective time course figures) of Aβ-, Aδ-, C-fibers, and post-discharge in response to spinal clonidine. Clonidine preferentially blocks the neuronal activity associated with Aδ-, C-fibers, and post-discharge but not Aβ-fibers. On the other hand, blockade of the α2A-adrenoceptors with BRL 44408 (1 nmol) inhibits the clonidine-induced electrophysiological antinociception.
Upon quantifying the data from the PSTHs, we found that spinal clonidine decreases the firing response elicited by electrical stimuli on the RF (Figures 6B–I). Specifically, clonidine has no substantial effect on the neuronal activity associated with Aβ-fiber activation (Figure 6B), but we observed a potent inhibition of neuronal activity related to the activation of Aδ- (Figure 6C) rather than C-fibers (Figure 6D). This antinociceptive effect started 10 min after clonidine administration, peaked at 40–50 min and lasted up to 60 min as previously reported (Sullivan et al., 1987; Bonnet et al., 1989; Murata et al., 1989). Furthermore, as expected, the clonidine-induced inhibition of nociceptive Aδ- and C-fiber activity was reversed by spinal pre-treatment with BRL 44408 (1 nmol). In addition, as shown in Figure 6E, the post-discharge activity was reduced by 10 nmol clonidine, an effect reversed by BRL44408. Analyzing these results as global neuronal activity (Figures 6F–I), we corroborated that clonidine selectively blocks the neuronal activity associated with the activation of primary nociceptive fibers, and this effect is abolished by a selective α2A-adrenoceptor antagonist (BRL 44408). Together, these results highlight the relevance of primary afferent fibers innervating second-order WDR cells in clonidine-induced antinociception.
Based on the behavioral results, where 1 nmol JP 1302 per se inhibited the flinches evoked by formalin (Figures 3D–F), we tested the effect of JP 1302 on WDR neuronal activity (Figures 7A–F). The results showed that spinal JP 1302 (1 or 10 nmol) did not affect the peripheral evoked neuronal activity of Aβ-, Aδ-, C-fibers and post-discharge (Figures 7C–F). These results may imply that the mechanisms involved in the antinociception induced by JP 1302 are not mediated by an action on WDR neurons or primary afferent fibers innervating these second-order cells.
FIGURE 7. Spinal JP-1302 does not affect wide dynamic range (WDR) cell activity, but behaviorally, it inhibits nociception by increasing GABAergic activity. (A,B) depict the peri-stimulus time histogram (PSTH) constructed from 20 WDR responses to receptive field (RF) electrical stimulation before (basal) and after treatment (vehicle or JP 1302); also, in these panels, the raster plot and raw tracing of a single second-order WDR neuron cluster response elicited by one electrical stimulus (upper tracing) are illustrated. In accordance with the fiber conduction velocities, the panels in the figure depict the different fiber components (Aβ-, Aδ-, and C-fibers) of the spinal WDR cell response. Note that JP 1302 seems not to exert an effect on the WDR neuronal activity; indeed, when the data are analyzed as a percent of change of the neuronal activity (C–F), JP 1302 does not impact (statistically) the neuronal firing associated with the activation of Aβ-, Aδ-, C-fibers, and post-discharge. (G) shows the effect of intrathecal (i.t.) injection of 0.3 nmol bicuculline (a preferent GABAA receptor blocker) on the JP 1302-induced inhibition of the flinches induced by formalin (1%; 50 μl, s.c.) during phase I (P1) and phase II (P2). Note that bicuculline per se did not affect formalin-induced flinching behavior, but this GABAA channel blocker reversed the effect of JP 1302. (H,I) represent the data as the area under the mean number of flinches against the curve (AUC) and show that bicuculline inhibited the JP 1302-induced antinociception in both phases (P1, P2).
On this basis and considering that some evidence suggests that α2C-adrenoceptors decrease GABAergic transmission (see discussion section), we hypothesized that the JP 1302-induced behavioral antinociception could be indirectly mediated by a spinal GABAergic mechanism. Accordingly, i.t., administration of bicuculline (0.3 nmol; a GABAA receptor blocker) abolished the behavioral antinociception induced by JP 1302 (1 nmol) in phases I and II (Figures 7G–I). In this case, and as previously reported in the formalin test (Dirig and Yaksh, 1995; Peng et al., 2015; Ryu et al., 2021) bicuculline per se does not influence flinching behavior.
This study was designed to pharmacologically dissect the role of different α2A/2B/2C-adrenoceptor subtypes in clonidine-induced antinociception. Using tonic (i.e., formalin test) or acute (i.e., peripheral electrical stimulation) nociceptive stimuli, we show that clonidine-induced antinociception is mediated by α2A- but not α2B/2C-adrenoceptor activation. Given that in the electrophysiological experiments, clonidine inhibited the neuronal activity associated with electrical activation of Aδ- and C-fibers (but not Aβ-fibers), a presynaptic effect on nociceptive primary afferent fibers (PAFs) is supported. Furthermore, the ongoing firing (post-discharge) was attenuated by clonidine; an effect also reversed by the BRL 44408. Besides, we found that spinal JP 1302 (a selective α2C-adrenoceptor antagonist) induced per se behavioral but not electrophysiological antinociception, an effect reversed by bicuculline (a preferential GABAA receptor blocker). Apart from the implications discussed below, our data support the notion that: 1) antinociception (acute or tonic) induced by clonidine is mediated by α2A-adrenoceptors, whereas 2) pharmacological blockade of α2C-adrenoceptors during tonic nociception elicits antinociception, thus, unmasking a potential pronociceptive action of this receptor (Figure 8).
FIGURE 8. Proposed mechanisms implying a differential role of the α2A- and α2C-adrenoceptors subtypes in pain modulation at the spinal cord level. In general, α2-adrenoceptors are coupled to Gi/o proteins; thus, when activated, they could promote the inhibition of neurotransmitter release by inhibiting adenylate cyclase activity and inactivating Ca2+ channels and/or facilitation of K+ currents. Our behavioral and electrophysiological data support the contention that clonidine (a non-selective α2A/2B/2C-adrenoceptor agonist) induces antinociception via activation of α2A-adrenoceptors, probably located at presynaptic level (in the primary nociceptive Aδ- and C-fibers), causing a diminution of the WDR cell nociceptive firing. Indeed, α2A-adrenoceptors activation inhibits tonic (formalin) and acute (electrical) nociceptive input. On the other hand, the selective α2C-adrenoceptor antagonist, JP 1302, produces per se only behavioral but not electrophysiological antinociception (measured on second WDR cells), suggesting an inhibitory effect on tonic nociception. Since JP 1302-induced antinociception was blocked by bicuculline, a GABAergic mechanism has been resembled. The data could be interpreted as follows: (i) presynaptic activation of α2A-adrenoceptors by clonidine inhibits acute and tonic peripheral nociception; (ii) the JP 1302-induced antinociception is not mediated by inhibition of the nociceptive primary afferent fibers activity; and (ii) considering that α2C-adrenoceptors can be found in GABAergic neurons, we could suggest that under a tonic nociceptive stimulus (e.g., inflammatory), activation of α2C-adrenoceptors play a pronociceptive role. Hence (see Discussion for details), the data support the notion that α2A-adrenoceptor are mainly localized in primary nociceptive afferent fibers modulating the activity of WDR cells. In contrast, α2C-adrenoceptors seem to be present in spinal interneurons rather than capsaicin-sensitive fibers modulating second-order nociceptive specific cells.
One important thing in the experimental protocols followed was that the effect of clonidine was analyzed during 1 h. This experimental design was based on the long-lasting antinociception induced by clonidine when given by a spinal route, despite the half-life in the spinal cord being ∼30 min (Castro and Eisenach, 1989; Khan et al., 1999). Accordingly, seminal reports have consistently shown that this compound induces a spinal long-lasting (>1 h) analgesia/antinociception peaking after 30 min post-injection (Sullivan et al., 1987; Bonnet et al., 1989; Murata et al., 1989; Khan et al., 1999). At first glance, we could hypothesize that this long-lasting effect could be partly mediated by the engagement of supraspinal mechanisms enhancing a descending inhibitory pathway. Indeed, we must acknowledge that activation of the endogenous noradrenergic descending pathway plays a key role in spinal pain modulation in naïve and neuropathic rodents (Patel et al., 2018). Nevertheless, Murata et al. (1989) showed in WDR cell recordings that antinociception elicited by spinal clonidine was unaffected in animals spinally transected. Consequently, it seems that the effect of this ligand (when given spinally) mainly relies upon spinal cord level.
Our data showed that i.t., clonidine reduces the number of flinches evoked by 1% formalin (Figure 2). This effect is related to spinal α2-adrenoceptor activation (Pertovaara, 2013; Llorca-Torralba et al., 2016; Bahari and Meftahi, 2019). Since pretreatment with BRL 44408 (but not imiloxan or JP 1302) reversed the clonidine-induced behavioral antinociception, the role of α2A-adrenoceptors in clonidine’s effect is supported (Figures 3A–C). Accordingly, our data agree with the consensus that α2A-adrenoceptor is relevant to the spinal clonidine effect (Pertovaara, 2013).
In this regard, the effect of spinal clonidine on WDR cells was tested to give an electrophysiological correlate. At the spinal level, it is well-known (Urch and Dickenson, 2003) that these second-order neurons receive concomitant input from PAFs, and electrical stimulation of the peripheral RF produces a triphasic response corresponding to the activation of Aβ-, Aδ- and C-fibers (Figure 4), also a post-discharge can be elicited (Figure 4). This experimental approach let us analyze the impact of clonidine in nociceptive transmission, particularly how this ligand affects the firing of PAFs. As illustrated in the PSTHs of WDR cells (Figure 5) or the temporal course of neuronal activity (Figure 6), 10 nmol clonidine preferentially diminishes the neuronal firing associated with activation of nociceptive Aδ- and C-fibers, supporting the notion that spinal clonidine-induced inhibition via presynaptic action (i.e., on PAFs). In addition, the ongoing activity (i.e., the post-discharge) was also inhibited by 10 nmol clonidine. In direct support of these findings, in vivo (Sonohata et al., 2004) and in vitro (Pan et al., 2002; Kawasaki et al., 2003) electrophysiological recordings show that α2-adrenoceptor activation diminished the excitatory postsynaptic current evoked by PAF stimulation. At this point, we need to remember that post-discharge represents a late response of C-fibers to peripheral stimulation that can be endured by a traumatic injury leading to a neuronal hyperexcitability observed electrophysiologically as a wind-up (Woolf, 1996). However, under our experimental approach, we were unable to induce wind-up. Hence, an interesting question not addressed in the present work relates to how wind-up can be affected by noradrenergic transmission.
Accordingly, to the behavioral experiments, we also showed that presynaptic pharmacological blockade of α2A-adrenoceptors in WDR recordings is relevant to the effect of clonidine. Although these data agree with histological/molecular data showing the presence of α2A-adrenoceptors at the PAF level (i.e., Aδ- and C-fibers) (Stone et al., 1998; Birder and Perl, 1999), no direct in vivo evidence about a functional role of the presynaptic α2A-adrenoceptor subtype had been previously reported.
Regarding α2B-adrenoceptors, the behavioral experiments reject the involvement of this adrenoceptor subtype. As shown in Figures 3A–C supramaximal dose of imiloxan (α2B-adrenoceptor antagonist) does not affect clonidine’s antinociception. Current literature seems to support the notion that spinal α2B-adrenoceptor does not influence pain modulation (for refs., see Pertovaara, 2013; Llorca-Torralba et al., 2016; Bahari and Meftahi, 2019). For example, in an inflammatory pain model, Zhang et al. (2012) showed that spinal α2B-adrenoceptor blockade with imiloxan does not reverse the antinociception induced by enhancement of the descending noradrenergic tone, suggesting that this receptor subtype is not necessary for the noradrenergic analgesic effect. However, in neuropathic pain models involving ligature of spinal nerves (not tested in the present experiments) and using selective ligands, it seems that α2B-adrenoceptor acquire a role in the inhibition of nociception (Chu et al., 2015; Rodríguez-Palma et al., 2022) an effect probably related with cortical activation of this receptor subtype rather than spinal mechanism (Chu et al., 2015). In essence, our data suggest that spinal α2B-adrenoceptor does not play a role in tonic inflammatory and acute nociception.
In contrast, current data about the functional role of α2C-adrenoceptors inhibiting nociception is scarce, and as discussed by Pertovaara (2013), the impact of this receptor subtype in spinal nociception is unclear. These inconsistent data are partly due to the lack of selective compounds used to dissect the contribution of different α2A/2B/2C-adrenoceptor subtypes. For example, in transgenic mice, Fairbanks et al. (2002) showed that α2C-adrenoceptors have a subtle role in the moxonidine (a mixed I1 imidazoline/α2C-adrenoceptor agonist)-induced antinociception, whereas Malmberg et al. (2001) suggest that α2A- but not α2C-adrenoceptors are indispensable for the antinociceptive action of i.t., dexmedetomidine (a non-selective α2A/2B/2C-adrenoceptor antagonist).
In our behavioral experiments, we used JP 1302, a selective α2C-adrenoceptor antagonist (Sallinen et al., 2007; Proudman et al., 2022), to test the role of this receptor in clonidine-induced antinociception. According to Figures 3A–C i.t., JP 1302 was unable to block clonidine’s effect. Similar results were found in a neuropathic pain model (Rodríguez-Palma et al., 2022) or writhes-induced by sleep deprivation (Yaoita et al., 2018), showing that systemic JP 1302 does not preclude the behavioral antinociceptive action of α2-adrenoceptor agonists (ST-91 or tizanidine given systemically). However, when we analyzed the per se effects of the antagonist (Figures 3D–F), we found that in sharp contrast to BRL 44408 or imiloxan, i.t., JP 1302 diminishes the number of flinches induced by formalin (in phase I and II), implying that this antagonist has an antinociceptive effect during the formalin test.
In contradiction to the behavioral data, when we explored the effect of JP 1302 using an electrophysiological approach (Figures 7A–F), no effect was observed in the neuronal firing of WDR cells. This discrepancy could be attributable to the nature of the noxious stimulus used (i.e., tonic vs. acute) and may imply that JP 1302 exerts its antinociceptive action in a different way than clonidine not involving presynaptic or WDR cell inhibition. Admittedly, an interesting iteration to try to disentangle and give an electrophysiological correlate would have been to analyze the effect of these ligands on wind-up, taking into account that this neuronal process has been related with central sensitization due to recruitment of nociceptive circuits beyond of Aδ- and C-fibers activation. Regardless, if we consider that this compound exerts an antinociceptive effect via the blockade of α2C-adrenoceptors, it is interesting to note that α2C-adrenoceptors have been localized in non-noradrenergic brainstem descending fibers and postsynaptic sites in spinal interneurons (Stone et al., 1998; Olave and Maxwell, 2002). To our knowledge, no report about an antinociceptive per se action of selective α2C-adrenoceptor antagonists exist. Consequently, the question is: How can α2C-adrenoceptor blockade induce spinal antinociception?
Proudman et al. (2022) suggest that the selectivity of JP 1302 is reliable for dissecting between the α2A/2B/2C-adrenoceptor subtypes (see Table 1), but this antagonist also displays some affinity for α1A-adrenoceptors (pKi:6.2). Hence, we cannot ignore that interaction between JP 1302 and α1A-adrenoceptors could exist. Certainly, using an optogenetic approach, Kucharczyk et al. (2022) showed that activating a discrete noradrenergic descending pathway from locus coeruleus provokes a diminution of WDR activity, an effect blocked by prazosin (a non-selective α1A/1B/1D-adrenoceptor antagonist), suggesting a role for α1-adrenoceptors. However, in the formalin test, i.t., prazosin does not affect nocifensive behavior (Jeong et al., 2011; Park et al., 2011). Similarly, the clonidine-induced inhibition of WDR responses evoked by NMDA was unaffected by prazocin (Zhang et al., 1998). Indeed, subcutaneous formalin injection tends to decrease the [3H]-prazosin binding sites in the spinal cord (Nalepa et al., 2005), suggesting that under an inflammatory stimulus, the probability that this receptor could be exerting a pharmacological effect is minimal. Besides, using an acute (thermal) or neuropathic pain model (L5 - L6 nerve ligation), i.t., α1-adrenoceptor agonist (methoxamine) does not have any impact on the pain threshold (Nagasaka and Yaksh, 1990; Yaksh et al., 1995). Together these data support our contention that under our experimental conditions, the effect of JP-1302 may be mediated by its interaction with the α2C-adrenoceptor.
If spinal α2C-adrenoceptor blockade produces an antinociceptive effect in the formalin test but not in the electrophysiological experiments, the most straightforward interpretation of these findings may suggest (but does not prove) that under tonic nociception α2C-adrenoceptor activation counter-balance the antinociceptive effect of descending noradrenergic system. In this regard, subcutaneous formalin induces not only an increase in the endogenous descending noradrenergic activity (Ma et al., 2001; Sajadeianfard et al., 2005; Martins et al., 2013) but also a rise in the mRNA expression of α2C-adrenoceptor at spinal cord level (Yoon et al., 2011). Coupled with the evidence suggesting that this receptor is expressed in GABAergic interneurons (Holmberg et al., 1999; Olave and Maxwell, 2002), we hypothesize that during spinal tonic nociceptive input, activation of this receptor could, in turn, diminish the GABAergic transmission, favoring a pronociceptive state. This hypothesis gain weight considering that at the striatum level, it has been suggested that activation of α2C-, but not α2A-adrenoceptors inhibits GABA release (Holmberg et al., 1999; Zhang and Ordway, 2003). Hence, i.t., JP 1302 indirectly favors an antinociceptive effect by improving the activity of GABAergic neurons. To prove this hypothesis, and using the formalin test, we assessed the effect of spinal bicuculline (unspecific antagonist of the GABAA receptors) on the JP 1302-induced antinociception.
At this point, we must emphasize that 0.3 nmol (0.11 μg) bicuculline per se does not influence flinching behavior induced by formalin (Figures 6F–H). This data agrees with previous reports showing that i.t., <0.3 μg bicuculline (<0.8 nmol) does not impact this nocifensive behavior (Dirig and Yaksh, 1995; Peng et al., 2015; Ryu et al., 2021). However, although some reports showed that spinal bicuculline is pronociceptive, this effect depends on the dose of bicuculline and the explored pain model. In the case of the formalin test, Kaneko and Hammond (1997) showed that at formalin concentrations <1%, an increase of flinches is induced by bicuculline, particularly at 0.3 μg (i.e., 0.8 nmol). Therefore, under our experimental conditions (1% formalin), the concentration used of bicuculline (0.3 nmol equivalents to 0.11 μg) seems to be adequate to evaluate the role of GABAergic participation in the JP 1302 effect.
The data show that the antinociception induced by JP 1302 was reversed with 0.3 nmol bicuculline (Figures 7H–J), suggesting that JP 1302 favors GABAergic transmission. In vitro evidence indicates that GABAergic neurons in the substantia gelatinosa can be hyperpolarized by noradrenaline (Hantman and Perl, 2005). Specifically, by recording GABAergic neurons at the superficial dorsal horn level using the patch-clamp technique, Gassner et al. (2009) proved that although the main effect of noradrenaline on GABAergic neurons is depolarization, a minor proportion of these cells are hyperpolarized via α2-adrenoceptors. In these studies, the specific receptor subtype was not identified. Together, these data support our contention that by inhibiting GABAergic neurotransmission, α2C-adrenoceptors may play a pronociceptive role (particularly during tonic nociception). The pronociceptive role of this receptor has also been suggested in in vitro assays by Chen et al. (2008), where they showed that α2C-adrenoceptor activation inhibits opioid release in the spinal cord; thus, blocking this receptor would induce antinociception.
Our data suggest that during tonic pain, both α2A- and α2C-adrenoreceptors are activated, and one question that could arise is: exist a particular situation where α2C- surpass the antinociceptive effect of α2A-adrenoreceptors? Current evidence shows that the net impact of non-selective α2-adrenoceptor agonists is antinociception in acute and neuropathic pain conditions (for refs. see Bahari and Meftahi, 2019), pointing out the relevance of α2A-over α2C-adrenoreceptors. Certainly, we need to keep in mind that plastic changes in the spinal α2-adrenoreceptors occur under neuropathic pain and may explain, at least in part, the variability in the efficacy of α2-adrenoreceptor agonists depending on the stage of neuropathic pain (Yoon et al., 2011). At this point, it is worth mentioning that the expression of the different α2A/2B/2C-adrenoceptor subtypes at the spinal cord level under pathological pain has been analyzed. Stone et al. (1999) showed that after spinal nerve ligation, the immunostaining of α2A-adrenoceptor is reduced, whereas α2C-adrenoceptor is enhanced. Hayashida and Eisenach (2010) discussed the physiological relevance of this change, suggesting that under peripheral nerve injury, the function of α2-adrenoceptors can be altered. Furthermore, the relevance of α2C-adrenoreceptor in spinal nociception will be answered with a selective α2C-adrenoreceptor agonist (not available yet) or the use of more precise approaches (e.g., opto- and chemogenetics). Regardless, at the spinal level, the effect of clonidine in healthy and pathological pain is analgesia. Therefore, our results deserve further investigation.
Finally, at the spinal dorsal horn level, the role of noradrenergic transmission is far more complex than initially conceived. This complexity may depend on the type of adrenergic receptor stimulated, the localization of the receptor (e.g., PAFs, interneurons, second-order neurons), and the type of nociceptive input. For example, at the spinal trigeminal level, both α2A-, and α2C-adrenoceptors seem to inhibit nociceptive transmission (Villalón et al., 2012). More recently, it has been suggested that at the spinal cord level, the noradrenergic system elicits a fine-tunning of nociception by activation of α1-or α2-adrenoceptors; indeed, they proposed that spinal α2-adrenoceptors located presynaptically in the noradrenergic projections from the ventral LC can be modulating the firing of GABAergic interneurons (see Suppl Fig 2 in Kucharczyk et al. (2022)). Furthermore, as illustrated by Hickey et al. (2014), optogenetic experiments demonstrate that stimulation of different regions of locus coeruleus can evoke an antinociceptive or pronociceptive spinal effect; in this case, although i.t., atipamezole (a non-selective α2A/2B/2C-adrenoceptor antagonist) blocked the spinal clonidine-induced antinociception, this antagonist also seems to enhance the antinociceptive action of optogenetic stimulation of the noradrenergic system in the LC, but the mechanism/receptor by which atipamezole enhances antinociception was not further explored. This divergent effect of noradrenergic transmission on pain modulation has also been observed in healthy humans, where activation of α2-adrenoceptors (by yohimbine) elicits both antinociceptive and pronociceptive actions (Vo and Drummond, 2015).
Taken together, our data suggest that spinal α2A- and α2C-adrenoceptors exert a differential (opposite) effect on nociceptive transmission. Specifically, α2A-adrenoceptor activation on PAF inhibits the tonic and acute nociceptive input, whereas α2C-adrenoceptor activation appeared to inhibit GABAergic transmission, favoring nociception during a tonic (inflammatory) stimulus.
The original contributions presented in the study are included in the article/supplementary material, further inquiries can be directed to the corresponding author.
The animal study was reviewed and the protocol was approved by the Institutional Animal Care and Use Committee at the Instituto de Neurobiología, UNAM.
Conceptualization and resources (JL-C, MC-L, AG-H), investigation (GL-C, GM-L, AG-H), data curation (GL-C, AG-H), formal analysis (GL-C, AG-H), supervision (GM-L, MC-L, AG-H), writing and editing (all authors).
This study on rodents was supported by the Programa de Apoyo a Proyectos de Investigación e Innovación Tecnológica (PAPIIT-UNAM Mexico) under Grant agreement no. IN202222 (MC-L) and IN218122 (AG-H). Also, we are indebted to the Fondo Sectorial de Investigación para la Educación (CONACyT-Mexico Grant No. A1-S-23631 to AG-H) and to the “Committee for Aid and Education in Neurochemistry (CAEN)” of the International Society for Neurochemistry (ISN).
The authors acknowledge Jessica González-Norris for proofreading the manuscript. The present work is a part of M.Sc. Thesis of GL-C, a student from Programa de Maestría en Ciencias (Neurobiología) (INB-UNAM) who received fellowship (no. 859395) from CONACyT-Mexico.
The authors declare that the research was conducted in the absence of any commercial or financial relationships that could be construed as a potential conflict of interest.
All claims expressed in this article are solely those of the authors and do not necessarily represent those of their affiliated organizations, or those of the publisher, the editors and the reviewers. Any product that may be evaluated in this article, or claim that may be made by its manufacturer, is not guaranteed or endorsed by the publisher.
Aoki, C., Go, C. G., Venkatesan, C., and Kurose, H. (1994). Perikaryal and synaptic localization of α2A-adrenergic receptor-like immunoreactivity. Brain Res. 650, 181–204. doi:10.1016/0006-8993(94)91782-5
Bahari, Z., and Meftahi, G. H. (2019). Spinal α2-adrenoceptors and neuropathic pain modulation; therapeutic target. Br. J. Pharmacol. 176, 2366–2381. doi:10.1111/bph.14580
Birder, L. A., and Perl, E. R. (1999). Expression of α2-adrenergic receptors in rat primary afferent neurons after peripheral nerve injury or inflammation. J. Physiol. 515, 533–542. doi:10.1111/j.1469-7793.1999.533ac.x
Bonnet, F., Boico, O., Rostaing, S., Saada, M., Loriferne, J. F., Touboul, C., et al. (1989). Postoperative analgesia with extradural clonidine. Br. J. Anaesth. 63, 465–469. doi:10.1093/bja/63.4.465
Bylund, D. B., Eikenberg, D. C., Hieble, J. P., Langer, S. Z., Lefkowitz, R. J., Minneman, K. P., et al. (1994). International union of pharmacology nomenclature of adrenoceptors. Pharmacol. Rev. 46, 121–136.
Castro, M. I., and Eisenach, J. C. (1989). Pharmacokinetics and dynamics of intravenous, intrathecal, and epidural clonidine in sheep. Anesthesiology 71, 418–425. doi:10.1097/00000542-198909000-00018
Chapman, V., and Dickenson, A. H. (1992). The combination of NMDA antagonism and morphine produces profound antinociception in the rat dorsal horn. Brain Res. 573, 321–323. doi:10.1016/0006-8993(92)90780-d
Chen, S. R., and Pan, H. L. (2001). Spinal endogenous acetylcholine contributes to the analgesic effect of systemic morphine in rats. Anesthesiology 95, 525–530. doi:10.1097/00000542-200108000-00039
Chen, W., Song, B., and Marvizón, J. C. (2008). Inhibition of opioid release in the rat spinal cord by α2C adrenergic receptors. Neuropharmacology 54, 944–953. doi:10.1016/j.neuropharm.2008.02.002
Chu, K. L., Xu, J., Frost, J., Li, L., Gomez, E., Dart, M. J., et al. (2015). A selective α2B-adrenoceptor agonist (A-1262543) and duloxetine modulate nociceptive neurones in the medial prefrontal cortex, but not in the spinal cord of neuropathic rats. Eur. J. Pain 19, 649–660. doi:10.1002/ejp.586
Dickenson, A. H., and Sullivan, A. F. (1987). Evidence for a role of the NMDA receptor in the frequency dependent potentiation of deep rat dorsal horn nociceptive neurones following C fibre stimulation. Neuropharmacology 26, 1235–1238. doi:10.1016/0028-3908(87)90275-9
Dirig, D. M., and Yaksh, T. L. (1995). Intrathecal baclofen and muscimol, but not midazolam, are antinociceptive using the rat-formalin model. J. Pharmacol. Exp. Ther. 275, 219–227.
Dubuisson, D., and Dennis, S. G. (1977). The formalin test: A quantitative study of the analgesic effects of morphine, meperidine, and brain stem stimulation in rats and cats. Pain 4, 161–174. doi:10.1016/0304-3959(77)90130-0
Dwyer, J. M., Platt, B. J., Rizzo, S. J., Pulicicchio, C. M., Wantuch, C., Zhang, M. Y., et al. (2010). Preclinical characterization of BRL 44408: Antidepressant- and analgesic-like activity through selective α2A-adrenoceptor antagonism. Int. J. Neuropsychopharmacol. 13, 1193–1205. doi:10.1017/S1461145709991088
Eisenach, J. C., De Kock, M., and Klimscha, W. (1996). α2-Adrenergic agonists for regional anesthesia: A clinical review of clonidine (1984-1995). Anesthesiology 85, 655–674. doi:10.1097/00000542-199609000-00026
Erne-Brand, F., Jirounek, P., Drewe, J., Hampl, K., and Scheneider, M. C. (1999). Mechanism of antinociceptive action of clonidine in nonmyelinated nerve fibres. Eur. J. Pharmacol. 383, 1–8. doi:10.1016/s0014-2999(99)00620-2
Fairbanks, C. A., Stone, L. S., Kitto, K. F., Nguyen, H. O., Posthumus, I. J., and Wilcox, G. L. (2002). α2C-Adrenergic receptors mediate spinal analgesia and adrenergic-opioid synergy. J. Pharmacol. Exp. Ther. 300, 282–290. doi:10.1124/jpet.300.1.282
Gamal-Eltrabily, M., de Los Monteros-Zúñiga, A. E., Manzano-García, A., Martínez-Lorenzana, G., Condés-Lara, M., and González-Hernández, A. (2020). The rostral agranular insular cortex, a new site of oxytocin to induce antinociception. J. Neurosci. 40, 5669–5680. doi:10.1523/JNEUROSCI.0962-20.2020
Gassner, M., Ruscheweyh, R., and Sandkühler, J. (2009). Direct excitation of spinal GABAergic interneurons by noradrenaline. Pain 145, 204–210. doi:10.1016/j.pain.2009.06.021
Gjerstad, J., Tjølsen, A., Hole, K., and Tjolsen, A. (1996). The effect of 5-HT1A receptor stimulation on nociceptive dorsal horn neurones in rats. Eur. J. Pharmacol. 318, 315–321. doi:10.1016/s0014-2999(96)00819-9
González-Hernández, A., Espinosa De Los Monteros-Zuñiga, A., Martínez-Lorenzana, G., and Condés-Lara, M. (2019). Recurrent antinociception induced by intrathecal or peripheral oxytocin in a neuropathic pain rat model. Exp. Brain Res. 237, 2995–3010. doi:10.1007/s00221-019-05651-7
Hantman, A. W., and Perl, E. R. (2005). Molecular and genetic features of a labeled class of spinal substantia gelatinosa neurons in a transgenic mouse. J. Comp. Neurol. 492, 90–100. doi:10.1002/cne.20709
Hayashida, K. I., and Eisenach, J. C. (2010). Spinal α2-adrenoceptor-mediated analgesia in neuropathic pain reflects brain-derived nerve growth factor and changes in spinal cholinergic neuronal function. Anesthesiology 113, 406–412. doi:10.1097/ALN.0b013e3181de6d2c
Hickey, L., Li, Y., Fyson, S. J., Watsn, T. C., Perrins, R., Hewinson, J., et al. (2014). Optoactivation of locus ceruleus neurons evokes bidirectional changes in thermal nociception in rats. J. Neurosci. 34, 4148–4160. doi:10.1523/JNEUROSCI.4835-13.2014
Holmberg, M., Scheinin, M., Kurose, H., and Miettinen, R. (1999). Adrenergic α2C-receptors reside in rat striatal GABAergic projection neurons: Comparison of radioligand binding and immunohistochemistry. Neuroscience 93, 1323–1333. doi:10.1016/s0306-4522(99)00260-2
Jasper, J. R., Lesnick, J. D., Chang, L. K., Yamanishi, S. S., Chang, T. K., Hsu, S. A., et al. (1998). Ligand efficacy and potency at recombinant α2-adrenergic receptors: Agonist-mediated [35S]GTPγS binding. Biochem. Pharmacol. 55, 1035–1043. doi:10.1016/s0006-2952(97)00631-x
Jeong, H. J., Lee, S. H., Cho, S. Y., Lee, C. S., Jeong, C. W., Yoon, M. H., et al. (2011). Roles of serotonergic and adrenergic receptors in the antinociception of selective cyclooxygenase-2 inhibitor in the rat spinal cord. Korean J. Pain 24, 179–184. doi:10.3344/kjp.2011.24.4.179
Kaneko, M., and Hammond, D. L. (1997). Role of spinal γ-aminobutyric acid a receptors in formalin-induced nociception in the rat. J. Pharmacol. Exp. Ther. 282, 928–938.
Kawasaki, Y., Kumamoto, E., Furue, H., and Yoshimiura, M. (2003). α2-adrenoceptor-mediated presynaptic inhibition of primary afferent glutamatergic transmission in rat substantia gelatinosa neurons. Anesthesiology 98, 682–689. doi:10.1097/00000542-200303000-00016
Khan, Z. P., Ferguson, C. N., and Jones, R. M. (1999). Alpha-2 and imidazoline receptor agonists - their pharmacology and therapeutic role. Anaesthesia 54, 146–165. doi:10.1046/j.1365-2044.1999.00659.x
Kucharczyk, M. W., Di Domenico, F., and Bannister, K. (2022). Distinct brainstem to spinal cord noradrenergic pathways inversely regulate spinal neuronal activity. Brain. 145, 2293–2300. doi:10.1093/brain/awac085
Li, J., Simone, D. A., and Larson, A. A. (1999). Wind-up leads to characteristics of central sensitization. Pain 79, 75–82. doi:10.1016/s0304-3959(98)00154-7
Llorca-Torralba, M., Borges, G., Neto, F., Mico, J. A., and Berrocoso, E. (2016). Noradrenergic locus coeruleus pathways in pain modulation. Neuroscience 338, 93–113. doi:10.1016/j.neuroscience.2016.05.057
Ma, J., Qiao, J. T., and Dafny, N. (2001). Opiate-like substances mediate norepinephrine-induced but not serotonin-induced antinociception at spinal level. Reevaluation by an electrophysiological model of formalin test in rats. Life Sci. 69, 969–976. doi:10.1016/s0024-3205(01)01191-2
Malmberg, A. B., Hedley, L. R., Jasper, J. R., Hunter, J. C., and Basbaum, A. I. (2001). Contribution of α2 receptor subtypes to nerve injury-induced pain and its regulation by dexmedetomidine. Br. J. Pharmacol. 132, 1827–1836. doi:10.1038/sj.bjp.0704032
Martins, I., de Vries, M. G., Teixeira-Pinto, A., Fadel, J., Wilson, S. P., Westerink, B. H. C., et al. (2013). Noradrenaline increases pain facilitation from the brain during inflammatory pain. Neuropharmacology 71, 299–307. doi:10.1016/j.neuropharm.2013.04.007
McGrath, J. C., Drummond, G. B., McLachlan, E. M., Kilkenny, C., and Wainwright, C. L. (2010). Guidelines for reporting experiments involving animals: The ARRIVE guidelines. Br. J. Pharmacol. 160, 1573–1576. doi:10.1111/j.1476-5381.2010.00873.x
Mendell, L. M. (1966). Physiological properties of unmyelinated fiber projection to the spinal cord. Exp. Neurol. 16, 316–332. doi:10.1016/0014-4886(66)90068-9
Murata, K., Nakagawa, I., Kumeta, Y., Kitahata, L. M., and Collins, J. G. (1989). Intrathecal clonidine suppresses noxiously evoked activity of spinal wide dynamic range neurons in cats. Anesth. Analg. 69, 185–191. doi:10.1213/00000539-198908000-00008
Nagasaka, H., and Yaksh, T. L. (1990). Pharmacology of intrathecal adrenergic agonists: Cardiovascular and nociceptive reflexes in halothane-anesthetized rats. Anesthesiology 73, 1198–1207. doi:10.1097/00000542-199012000-00018
Nalepa, I., Vetulani, J., Borghi, V., Kowalska, M., Przewłocka, B., and Pavone, F. (2005). Formalin hindpaw injection induces changes in the [3H]prazosin binding to α1-adrenoceptors in specific regions of the mouse brain and spinal cord. J. Neural Transm. 112, 1309–1319. doi:10.1007/s00702-005-0279-3
Nicholson, R., Dixon, A. K., Spanswick, D., and Lee, K. (2005). Noradrenergic receptor mRNA expression in adult rat superficial dorsal horn and dorsal root ganglion neurons. Neurosci. Lett. 380, 316–321. doi:10.1016/j.neulet.2005.01.079
Olave, M. J., and Maxwell, D. J. (2002). An investigation of neurones that possess the α2C-adrenergic receptor in the rat dorsal horn. Neuroscience 115, 31–40. doi:10.1016/s0306-4522(02)00407-4
Omote, K., Kitahata, L. M., Collins, J. G., Nakatani, K., and Nakagawa, I. (1991). Interaction between opiate subtype and α2 adrenergic agonists in suppression of noxiously evoked activity of WDR neurons in the spinal dorsal horn. Anesthesiology 74, 737–743. doi:10.1097/00000542-199104000-00018
O’Neill, T. P., and Haigler, H. J. (1985). Effects of clonidine on neuronal firing evoked by a noxious stimulus. Brain Res. 327, 97–103. doi:10.1016/0006-8993(85)91503-3
Pan, H. L., Wu, Z. Z., Zhou, H. Y., Chen, S. R., Zhang, H. M., and Li, D. P. (2008). Modulation of pain transmission by G protein-coupled receptors. Pharmacol. Ther. 117, 141–161. doi:10.1016/j.pharmthera.2007.09.003
Pan, Y. Z., Li, D. P., and Pan, H. L. (2002). Inhibition of glutamatergic synaptic input to spinal lamina IIo neurons by presynaptic α2-adrenergic receptors. J. Neurophysiol. 87, 1938–1947. doi:10.1152/jn.00575.2001
Park, C. H., Lee, H. G., Lee, S. H., Chung, C. W., and Yoon, M. H. (2011). The role of adrenergic and cholinergic receptors on the antinociception of sildenafil in the spinal cord of rats. Neurosci. Lett. 502, 99–102. doi:10.1016/j.neulet.2011.07.030
Patel, R., Qu, C., Xie, J. Y., Porreca, F., and Dickenson, A. H. (2018). Selective deficiencies in descending inhibitory modulation in neuropathic rats: Implications for enhancing noradrenergic tone. Pain 159, 1887–1899. doi:10.1097/j.pain.0000000000001300
Peng, F., Qu, Z. W., Qiu, C. Y., Liao, M., and Hu, W. P. (2015). Spinal vasopressin alleviates formalin-induced nociception by enhancing GABAA receptor function in mice. Neurosci. Lett. 593, 61–65. doi:10.1016/j.neulet.2015.03.023
Pertovaara, A. (2006). Noradrenergic pain modulation. Prog. Neurobiol. 80, 53–83. doi:10.1016/j.pneurobio.2006.08.001
Pertovaara, A. (2013). The noradrenergic pain regulation system: A potential target for pain therapy. Eur. J. Pharmacol. 716, 2–7. doi:10.1016/j.ejphar.2013.01.067
Proudman, R. G. W., Akinaga, J., and Baker, J. G. (2022). The affinity and selectivity of α-adrenoceptor antagonists, antidepressants and antipsychotics for the human α2A, α2B, and α2C-adrenoceptors and comparison with human α1 and β-adrenoceptors. Pharmacol. Res. Perspect. 10, e00936. doi:10.1002/prp2.936
Rodríguez-Palma, E. J., Castelo-Flores, D. G., Caram-Salas, N. L., Salinas-Abarca, A. B., Centurión, D., De la Luz-Cuellar, Y. E., et al. (2022). Sex-dependent antiallodynic effect of α2-adrenergic receptor agonist tizanidine in rats with experimental neuropathic pain. Eur. J. Pharmacol. 920, 174855. doi:10.1016/j.ejphar.2022.174855
Ryu, S. W., Kim, Y. O., Kim, H. B., Oh, S. B., Choi, J. I., and Yoon, M. H. (2021). Antinociceptive effect of intrathecal P7C3 via GABA in a rat model of inflammatory pain. Eur. J. Pharmacol. 899, 174029. doi:10.1016/j.ejphar.2021.174029
Sajadeianfard, J., Khatami, S., Semnanian, S., Naghdi, N., and Jorjani, M. (2005). In vivo measurement of noradrenaline in the locus coeruleus of rats during the formalin test: A microdialysis study. Eur. J. Pharmacol. 512, 153–156. doi:10.1016/j.ejphar.2005.02.032
Sallinen, J., Höglund, I., Engström, M., Lehtimäki, J., Virtanen, R., Sirviö, J., et al. (2007). Pharmacological characterization and CNS effects of a novel highly selective α2C-adrenoceptor antagonist JP-1302. Br. J. Pharmacol. 150, 391–402. doi:10.1038/sj.bjp.0707005
Schwartz, R. H., Hernandez, S., Noor, N., Topfer, J., Farrell, K., Singh, N., et al. (2022). A comprehensive review of the use of α2 agonists in spinal anesthetics. Pain Physician 25, 193–201.
Sonohata, M., Furue, H., Katafuchi, T., Yasaka, T., Doi, A., Kumamoto, E., et al. (2004). Actions of noradrenaline on substantia gelatinosa neurones in the rat spinal cord revealed by in vivo patch recording. J. Physiol. 555, 515–526. doi:10.1113/jphysiol.2003.054932
Stone, L. S., Broberger, C., Vulchanova, L., Wilcox, G. L., Hökfelt, T., Riedl, M. S., et al. (1998). Differential distribution of α2A and α2C adrenergic receptor immunoreactivity in the rat spinal cord. J. Neurosci. 18, 5928–5937. doi:10.1523/jneurosci.18-15-05928.1998
Stone, L. S., Vulchanova, L., Riedl, M. S., Wang, J., Williams, F. G., Wilcox, G. L., et al. (1999). Effects of peripheral nerve injury on α2A and α2C adrenergic receptor immunoreactivity in the rat spinal cord. Neuroscience 93 (4), 1399–1407. doi:10.1016/s0306-4522(99)00209-2
Sullivan, A. F., Dashwood, M. R., and Dickenson, A. H. (1987). α2-adrenoceptor modulation of nociception in rat spinal cord: Location, effects and interactions with morphine. Eur. J. Pharmacol. 138, 169–177. doi:10.1016/0014-2999(87)90430-4
Uhlén, S., Porter, A. C., and Neubig, R. R. (1994). The novel α2 adrenergic radioligand [3H]MK912 is α2C selective among human α2A, α2B and α2C adrenoceptors. J. Pharmacol. Exp. Ther. 271, 1558–1565.
Urch, C. E., and Dickenson, A. H. (2003). In vivo single unit extracellular recordings from spinal cord neurones of rats. Brain Res. Brain Res. Protoc. 12, 26–34. doi:10.1016/s1385-299x(03)00068-0
Villalón, C. M., Galicia-Carreón, J., González-Hernández, A., Marichal-Cancino, B. A., Manrique-Maldonado, G., and Centurión, D. (2012). Pharmacological evidence that spinal α2C-and, to a lesser extent, α2A-adrenoceptors inhibit capsaicin-induced vasodilatation in the canine external carotid circulation. Eur. J. Pharmacol. 683, 204–210. doi:10.1016/j.ejphar.2012.03.002
Vo, L., and Drummond, P. D. (2015). Involvement of α2-adrenoceptors in inhibitory and facilitatory pain modulation processes. Eur. J. Pain 20, 386–398. doi:10.1002/ejp.736
Wheeler-Aceto, H., and Cowan, A. (1991). Standardization of the rat paw formalin test for the evaluation of analgesics. Psychopharmacology 104, 35–44. doi:10.1007/BF02244551
Woolf, C. J. (1996). Windup and central sensitization are not equivalent. Pain 66, 105–108. doi:10.1097/00006396-199608000-00001
Yaksh, T. L., Fisher, C. J., Hockman, T. M., and Wiese, A. J. (2017). Current and future issues in the development of spinal agents for the management of pain. Curr. Neuropharmacol. 15, 232–259. doi:10.2174/1570159x14666160307145542
Yaksh, T. L., Pogrel, J. W., Lee, Y. W., and Chaplan, S. R. (1995). Reversal of nerve ligation-induced allodynia by spinal α2-adrenoceptor agonists. J. Pharmacol. Exp. Ther. 272, 207–214.
Yaksh, T. L., and Rudy, T. A. (1976). Analgesia mediated by a direct spinal action of narcotics. Science 192, 1357–1358. doi:10.1126/science.1273597
Yaoita, F., Muto, M., Murakami, H., Endo, S., Kozawa, M., Tsuchiya, M., et al. (2018). Involvement of peripheral α2A adrenoceptor in the acceleration of gastrointestinal transit and abdominal visceral pain induced by intermittent deprivation of REM sleep. Physiol. Behav. 186, 52–61. doi:10.1016/j.physbeh.2018.01.010
Yoon, M. H., Huang, L. J., Choi, J. I., Lee, H. G., Kim, W. M., and Kim, C. M. (2011). Antinociceptive effect of intrathecal ginsenosides through α2-adrenoceptors in the formalin test of rats. Br. J. Anaesth. 106, 371–379. doi:10.1093/bja/aeq367
You, H. J., Morch, C. D., Chen, J., and Arendt-Nielsen, L. (2003). Simultaneous recordings of wind-up of paired spinal dorsal horn nociceptive neuron and nociceptive flexion reflex in rats. Brain Res. 960, 235–245. doi:10.1016/s0006-8993(02)03895-7
Young, P., Berge, J., Chapman, H., and Cawthorne, M. A. (1989). Novel α2-adrenoceptor antagonists show selectivity for α2A- and α2B-adrenoceptor subtypes. Eur. J. Pharmacol. 168 (3), 381–386. doi:10.1016/0014-2999(89)90801-7
Zhang, K. M., Wang, X. M., Peterson, A. M., Chen, W. Y., and Mokha, S. S. (1998). α2-adrenoceptors modulate NMDA-evoked responses of neurons in superficial and deeper dorsal horn of the medulla. J. Neurophysiol. 80, 2210–2214. doi:10.1152/jn.1998.80.4.2210
Zhang, W., and Ordway, G. A. (2003). The α2C-adrenoceptor modulated GABA release in mouse striatum. Brain Res. Mol. Brain Res. 112, 24–32. doi:10.1016/s0169-328x(03)00026-3
Zhang, Y., Zhang, R. X., Zhang, M., Shen, X. Y., Li, A., Xin, J., et al. (2012). Electroacupuncture inhibition of hyperalgesia in an inflammatory pain rat model: Involvement of distinct spinal serotonin and norepinephrine receptor subtypes. Br. J. Anaesth. 109, 245–252. doi:10.1093/bja/aes136
Keywords: adrenoceptor, analgesia, clonidine, electrophysiology, pain, behavior
Citation: López-Córdoba G, Martínez-Lorenzana G, Lozano-Cuenca J, Condés-Lara M and González-Hernández A (2022) The differential in vivo contribution of spinal α2A- and α2C-adrenoceptors in tonic and acute evoked nociception in the rat. Front. Pharmacol. 13:1023611. doi: 10.3389/fphar.2022.1023611
Received: 20 August 2022; Accepted: 07 November 2022;
Published: 23 November 2022.
Edited by:
Peregrine B. Osborne, The University of Melbourne, AustraliaReviewed by:
Victor Ruiz-Velasco, College of Medicine, The Pennsylvania State University, United StatesCopyright © 2022 López-Córdoba, Martínez-Lorenzana, Lozano-Cuenca, Condés-Lara and González-Hernández. This is an open-access article distributed under the terms of the Creative Commons Attribution License (CC BY). The use, distribution or reproduction in other forums is permitted, provided the original author(s) and the copyright owner(s) are credited and that the original publication in this journal is cited, in accordance with accepted academic practice. No use, distribution or reproduction is permitted which does not comply with these terms.
*Correspondence: Abimael González-Hernández, YWJpbWFlbGdoQGNvbXVuaWRhZC51bmFtLm14
Disclaimer: All claims expressed in this article are solely those of the authors and do not necessarily represent those of their affiliated organizations, or those of the publisher, the editors and the reviewers. Any product that may be evaluated in this article or claim that may be made by its manufacturer is not guaranteed or endorsed by the publisher.
Research integrity at Frontiers
Learn more about the work of our research integrity team to safeguard the quality of each article we publish.