- 1National Innovation and Attracting Talents “111” Base, Key Laboratory of Biorheological Science and Technology, College of Bioengineering, Ministry of Education, Chongqing University, Chongqing, China
- 2Chongqing Engineering Research Center of Antitumor Natural Drugs, Chongqing Three Gorges Medical College, Chongqing, China
- 3School of Comprehensive Health Management, XiHua University, Chengdu, Sichuan, China
- 4School of Artificial Intelligence, Chongqing University of Education, Chongqing, China
- 5Chongqing Key Laboratory of Translational Research for Cancer Metastasis and Individualized Treatment, Chongqing University Cancer Hospital, Chongqing, China
Schisantherin A (STA) is a traditional Chinese medicine extracted from the plant Schisandra chinensis, which has a wide range of anti-inflammatory, antioxidant, and other pharmacological effects. This study investigates the anti-hepatocellular carcinoma effects of STA and the underlying mechanisms. STA significantly inhibits the proliferation and migration of Hep3B and HCCLM3 cells in vitro in a concentration-dependent manner. RNA-sequencing showed that 77 genes are upregulated and 136 genes are downregulated in STA-treated cells compared with untreated cells. KEGG pathway analysis showed significant enrichment in galactose metabolism as well as in fructose and mannose metabolism. Further gas chromatography-mass spectrometric analysis (GC-MS) confirmed this, indicating that STA significantly inhibits the glucose metabolism pathway of Hep3B cells. Tumor xenograft in nude mice showed that STA has a significant inhibitory effect on tumor growth in vivo. In conclusion, our results indicate that STA can inhibit cell proliferation by regulating glucose metabolism, with subsequent anti-tumor effects, and has the potential to be a candidate drug for the treatment of liver cancer.
1 Introduction
Liver cancer is an aggressive malignancy with very high morbidity and mortality (Foerster et al., 2022; Lu et al., 2022). The latest data from the Chinese National Cancer Center indicated that the incidence of liver cancer in China ranks fifth, and the mortality rate ranks second (Sung et al., 2021). Primary liver cancer involves three different pathological types of tumors, namely hepatocellular carcinoma (HCC), intrahepatic bile duct carcinoma, and mixed carcinoma. HCC accounts for more than 85%–90% of cases, with a high degree of malignancy, and is prone to recurrence and metastasis (Pan et al., 2021; Khatib et al., 2022). Traditional chemotherapy as a common treatment option can lead to cure and life prolongation for patients with HCC, yet the prognosis for most patients is dire or poor (Fu et al., 2022; Han et al., 2022). Therefore, the quest for potent new drugs for the treatment of HCC is paramount.
Cancer, including HCC, has recently been reconsidered as a metabolic disease, involving changes in the way energy is produced and utilized (Seyfried et al., 2014; Liao et al., 2022). Excessive proliferation of cancer cells requires a large amount of energy and nutrients, resulting in metabolic changes. Studies by Warburg and colleagues in the 1920s showed that tumor cells preferred increasing their energy supply by glycolysis, rather than mitochondrial oxidative phosphorylation (OXPHOS), even under oxygen-rich conditions (Koppenol et al., 2011). This metabolic reprogramming is what distinguishes malignant tumors from normal tissue and is known as the Warburg effect or aerobic glycolysis (Sun et al., 2022). Glycolysis produces ATP very fast, 100 times faster than OXPHOS (Locasale and Cantley, 2010). Tumor cells use glycolysis to accelerate energy acquisition through metabolic reprogramming to support the rapid proliferation of tumor cells (Yang et al., 2022).
Glucose metabolism in HCC cells is characterized by increased glucose uptake and accelerated glycolysis (Li et al., 2017). The acceleration of glycolysis in HCC depends on a number of key rate-limiting enzymes. The first step in glycolysis is the transport of glucose into cells through glucose transporters (GLUTs). Compared with normal hepatocytes, the expression and activity of GLUTs are upregulated in HCC cells (Xia et al., 2020). Wang et al. found that the highly expressed proto-oncogene MYC in HCC could accelerate glycolysis in HCC cells through the regulation of lactic dehydrogenase A (LDHA), thereby promoting the progression of HCC (Wang et al., 2022a). Zhang et al. (2018) found that hypoxic stress in HCC cells can promote the binding of YAP to HIF-1α in the nucleus, maintain the stability of HIF-1α, and activate pyruvate kinase M2 (PKM2) transcription to accelerate glycolysis. In addition, as upstream targets of PKM2, AKT, CAMKβ, and GTPBP4 can also affect the proliferation and glycolysis of HCC cells by regulating PKM2 (Ye et al., 2019; Sheng et al., 2020; Zhou et al., 2022). Similar to oncogenic molecules, Akt can also promote metabolic reprogramming by increasing GLUTs expression (Hu et al., 2019). These studies highlight the role of glucose metabolism in the development and progression of HCC, making glucose metabolism a potential target for the treatment of this disease.
Evidence has indicated that many traditional Chinese herbal medicines (TCM) exhibit potential anti-cancer effects (Liu et al., 2019; Fan et al., 2020; Li et al., 2022a). However, the underlying molecular mechanisms and targets remain unclear. Thus, to improve their development as new anticancer drugs, the pharmacological effects of TCM preparations must be thoroughly assessed (Chen and Ye, 2022). Schisantherin A (STA) is a TCM extracted from the plant Schisandra chinensis, which has a wide range of pharmacological properties, including anti-inflammatory and anti-oxidant effects (Gong and Wang, 2018; Gui et al., 2020; Wang et al., 2021). STA has also been used in the treatment of cancer by inducing cell apoptosis and inhibiting cell proliferation. Wang et al. (2020) reported that STA could induce apoptosis and cell cycle arrest via the production of reactive oxygen species and activation of JNK signaling with Nrf2 inhibition in gastric cancer cells. However, the anti-HCC effect of STA or its molecular mechanisms were not examined. Thus, an accurate understanding of the biological functions and mechanisms of STA can provide new insights for the treatment of HCC.
This study assessed the anti-tumor effects of STA against liver cancer as well as the underlying mechanisms. The significant inhibitory effect of STA on the migration and proliferation of HCC cells was observed. Further, glucose metabolism inhibition was shown to be involved in STA-induced anti-proliferation and migration. In addition, a subcutaneous tumor model in nude mice confirmed that STA significantly inhibited the growth of solid tumors. Thus, STA may be a new drug for the treatment of liver cancer.
2 Materials and methods
2.1 Cell culture
Human hepatoma cell lines, including highly metastatic HCCLM3 and low-metastatic Hep3B, were obtained from Liver Cancer Institute, Zhongshan Hospital, Fudan University (Shanghai, China). The cells were cultured in DMEM (GIBCO, United States) containing 10% fetal bovine serum (FBS, Gibco, United States) and 100 U/mL penicillin/streptomycin (GIBCO, United States), and maintained at 37°C with 5% CO2. STA (purity ≥ 98%; MedChemexpress, New Jersey, United States) was dissolved in dimethyl sulfoxide (DMSO; Solarbio, Beijing, China) to obtain a 20-mM stock solution that was then diluted with DMEM.
2.2 Cell viability analysis
For cytotoxicity assays, 5,000 cells per well were seeded in triplicate in 96-well plates and incubated at 37°C and 5% CO2 for 24 h. Cells were treated with different doses of STA for 48 h as required. Cell viability was measured with a cell counting kit (CCK-8; Dojindo, Japan) according to the manufacturer’s instructions. CCK-8 reagent (10 μl) was mixed with cells and incubated for 1 h. The final absorbance at 450 nm was assessed in each well using a MultiSkan GO micropore meter (Thermo, United States).
2.3 5-Ethynyl-2′-deoxyuridine assay
Based on the results of the cell viability analysis, three groups of cells (DMSO, 10 μM, 30 μM, and 50 μM) were seeded in 96-well plates at 5,000 cells/well for 48 h with 1.5 μM cisplatin (CDDP) administered as a positive control. HCC cells were then incubated with 50 μM 5-ethynyl-2′-deoxyuridine reagent (EdU; RiboBio, Guangzhou, China) for 2 h for EdU incorporation during DNA synthesis. Cells were then stained with Apollo fluorescent dye for 1 h and 0.25% Triton X-100 was added to each well. Finally, the samples were stained with Hoechst 33,342. Fluorescence images were captured under a fluorescence microscope (Olympus, Tokyo, Japan). The EdU assay was performed three times using five biological replicates.
2.4 Western blotting
Proteins were analyzed by western blotting according to standard methods. Proteins were extracted from cells using RIPA buffer (Beyotime, China) containing a mixture of protease and phosphatase inhibitors and quantified using an Enhanced BCA Protein Assay Kit (Beyotime, China). Protein samples (30 μg/lane) were loaded onto 10% separating gel and blotted onto a PVDF membrane (Millipore, Billerica, MA, United States). After blocking with 5% skimmed milk powder for 1 h, the membranes were incubated overnight with the following primary antibodies at 4°C: anti-CDK1(Zen-BioScience, China), anti-survivin (Zen-BioScience, China), anti-MMP2 (Zen-BioScience, China) and anti-GAPDH (Zen-BioScience, China). Membranes were then washed and incubated with HRP-linked secondary antibody for 1 h at 37°C. Finally, the samples from three independent experiments were detected using the ECL reagent (Thermo Fisher, United States).
2.5 Clone formation assay
Clone formation was evaluated on Hep3B and HCCLM3 cells in response to STA treatment (DMSO 10 μM, 30 μM, and 50 μM). Cells in the logarithmic growth phase were seeded at 500 cells/well in six-well plates and cultured at 37°C and 5% CO2 for 5 days. After treatment with fresh media containing different concentrations of STA for 5 days, the cells were fixed with 4% paraformaldehyde (PFA) at 4°C overnight. Finally, after staining with 0.1% crystal violet, cell colonies were photographed and counted.
2.6 Wound healing assay
Hep3B and HCCLM3 cells were seeded in six-well plates and allowed to grow to ∼70% density. A sterile 200-μl pipette tip was then used to scratch the surface, fresh medium containing different concentrations of STA (DMSO 10 μM, 30 μM, and 50 μM) was replaced, and the cells were cultured for 48 h. After fixing with 4% PFA, photographs were taken after staining with crystal violet to estimate the area occupied by migrating cells.
2.7 RNA sequencing and data analysia
Following treatment with STA for 48 h, total RNA was extracted from Hep3B and HCCLM3 cells using TRIzol reagent (Invitgen, Thermo Scientific, United States). Partially isolated RNA samples were submitted to BGI Co., Ltd., (Shenzhen, China) for transcriptome sequencing and BGISEQ-500 analysis.
2.8 Metabolite extraction and gas chromatography-mass spectrometric analysis
Hep3B cells were cultured in a 10-cm petri dish and treated with 50 μM STA for 48 h. The cells were washed twice with PBS solution and then peeled off by cell scraping. After centrifugation, the supernatant was removed, and the precipitated cells were collected and flash-frozen in liquid nitrogen for 15 min. Metabolites were extracted from equal amounts of STA-treated or untreated Hep3B cells (about 1 × 107 cells) by the previously mentioned method (Qu et al., 2022). The Trace1300 gas chromatography-mass spectrometry analysis system (Thermo Scientific) was used for metabolomics analysis in conjunction with the TCM Chromatographic Fingerprint Computer-Aided Similarity Assessment System (2012 edition) and SIMCA software (Umetrics, Sweden). An Agilent DB-5MS chromatographic column was used with an injection volume of 1 μl. The ion source of mass spectrometry was electrospray ionization (ESI), using the positive ion mode. The inlet temperature was 280°C. The control group was treated with DMSO; eight samples were collected for each group.
2.9 Animal studies
Female nude mice (n = 24) obtained from the Laboratory Animal Center of Chongqing Medical University were used for animal experiments. For subcutaneous xenograft experiments, 2 × 106 Hep3B cells in the logarithmic growth phase were injected into the armpit of nude mice. After 12 days, the mice were randomly divided into a control group, a positive control group (CDDP, 5 mg/kg, i.p., once in 2 days), a low concentration group (STA, 10 mg/kg i.p., once in 2 days), and a high concentration group (STA, 20 mg/kg i.p., once in 2 days), with six mice in each group. Intraperitoneal injections were performed every 2 days, and the longest axes (L) and vertical axes (R) of the tumor were measured. The tumor volume (V) was calculated according to V = (π/6) × L × R2. Sixteen days later, the tumor was excised for weighing and histological analysis.
2.10 Histological analysis
For hematoxylin and eosin (H&E) staining, all grafts were fixed in 4% PFA overnight. After dehydration, the samples were embedded in paraffin, sectioned, and stained with H&E. For immunohistochemistry detection, PFA-fixed tissues were immunostained for Ki67 protein using standard immunohistochemical procedures according to the manufacturers’ instructions and incubated overnight. After washing three times with PBS, the cells were incubated with secondary antibody at 37°C for 30 min and stained with DAB (3,3′-diaminobenzidine); Ki67-positive cells were brown.
2.11 Statistical analysis
Data were expressed as mean ± SEM of at least three independent experiments. Two-tailed Student t-tests were used to calculate the p values; p < 0.05 was considered statistically significant.
3 Results
3.1 STA inhibits the survival of HCC cells
To determine the effect of STA on cell viability, Hep3B and HCCLM3 cells were treated with different concentrations of STA for 48 h and detected by CCK-8 assay. STA decreased the viability of Hep3B and HCCLM3 cells in a dose-dependent manner (Figures 1A,B); at 30 μM and 50 μM, STA significantly inhibited the viability of Hep3B and HCCLM3 cells, whereas at 100 μM, most cells had died. Furthermore, the number of exfoliated cells increased and the cell size shrank with the increase in STA concentration (Figure 1C). Therefore, concentrations of 10 μM, 30 μM, and 50 μM STA were selected for subsequent experiments.
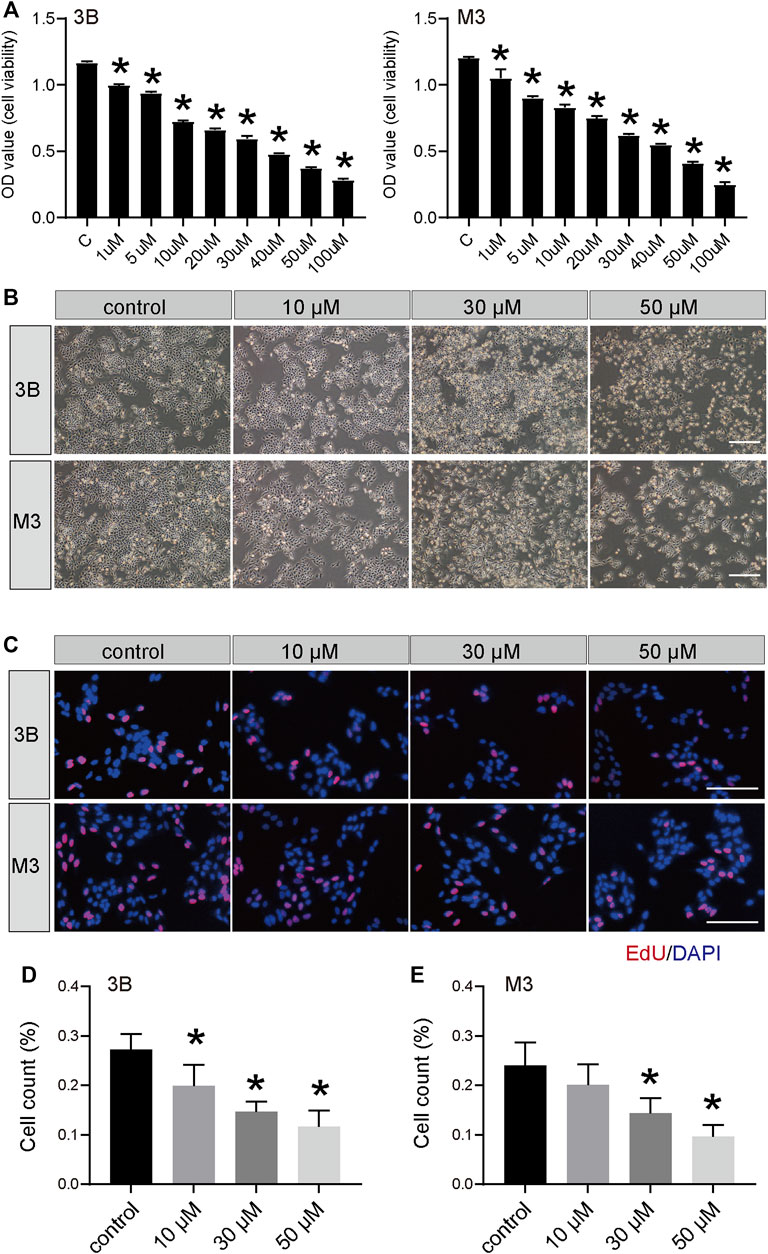
FIGURE 1. STA inhibits the survival of HCC cells. (A) Cell viability of Hep3B and HCCLM3 cells treated with STA at indicate concentration was determined by CCK8 method, DMSO was used as control. (B) The morphology of Hep3B and HCCLM3 cells treating with STA in different concentration for 48 h. (C–E) EdU-positive Hep3B and HCCLM3 cells after treating with STA for 48 h. Scale bar = 100 μm. Data shown are means ± SD; n = 3. *p < 0.05, significantly from control group.
3.2 STA inhibits Hep3B and HCCLM3 cell proliferation and migration
The EdU and clone formation assays were used to evaluate the effect of STA on cell proliferation. EdU showed that, compared with the control group, DNA synthesis and the EdU positivity rate were significantly decreased in cells treated with STA for 48 h in a dose-dependent manner (Figures 1D,E). Next, the effect of STA on the self-renewal ability of HCC cells was observed by clone formation assay. The number and size of clones in the STA-treated groups were significantly reduced compared with the control group (Figures 2A,B). The expression of proliferation-related proteins was further examined by western blotting assay, which demonstrated that the expression of CDK1 and survivin proteins was significantly decreased (Figures 2C–F). Therefore, STA effectively inhibited the proliferation of HCC cells.
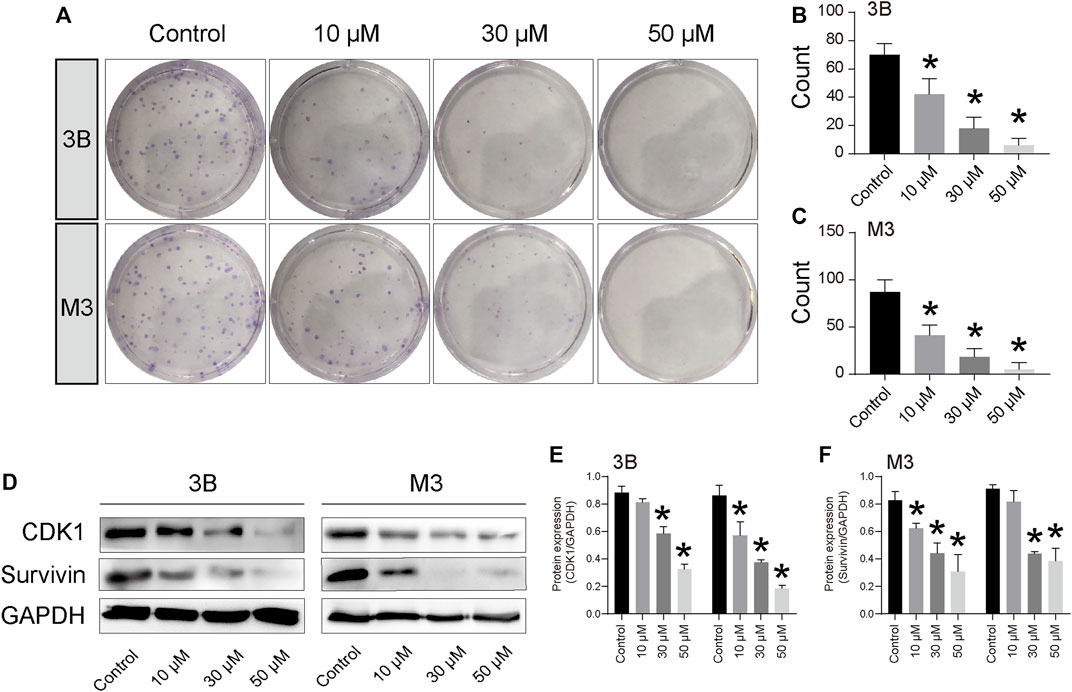
FIGURE 2. STA inhibits Hep3B and HCCLM3 cell proliferation. (A–C) Clone formation of Hep3B and HCCLM3 cells treated with STA at 10 μM, 30 μM, and 50 μM. (D–F) The representative bands of CDK1 and survivin in Hep3B and HCCLM3 cells treated with STA at 10 μM, 30 μM, and 50 μM for 48 h. GAPDH was used as an internal control. Data shown are means ± SD; n = 3. *p < 0.05, significantly from control group.
The cell scratch assay revealed that STA significantly inhibited the migration of Hep3B and HCCLM3 cells (Figures 3A–C). After 48 h, cells in the control group almost completely filled the scratch area, whereas the number of cells migrating to the scratch area was significantly reduced with the increase in STA concentration. Furthermore, STA treatment inhibited the expression of MMP2, a migration-related protein (Figures 3D–F).
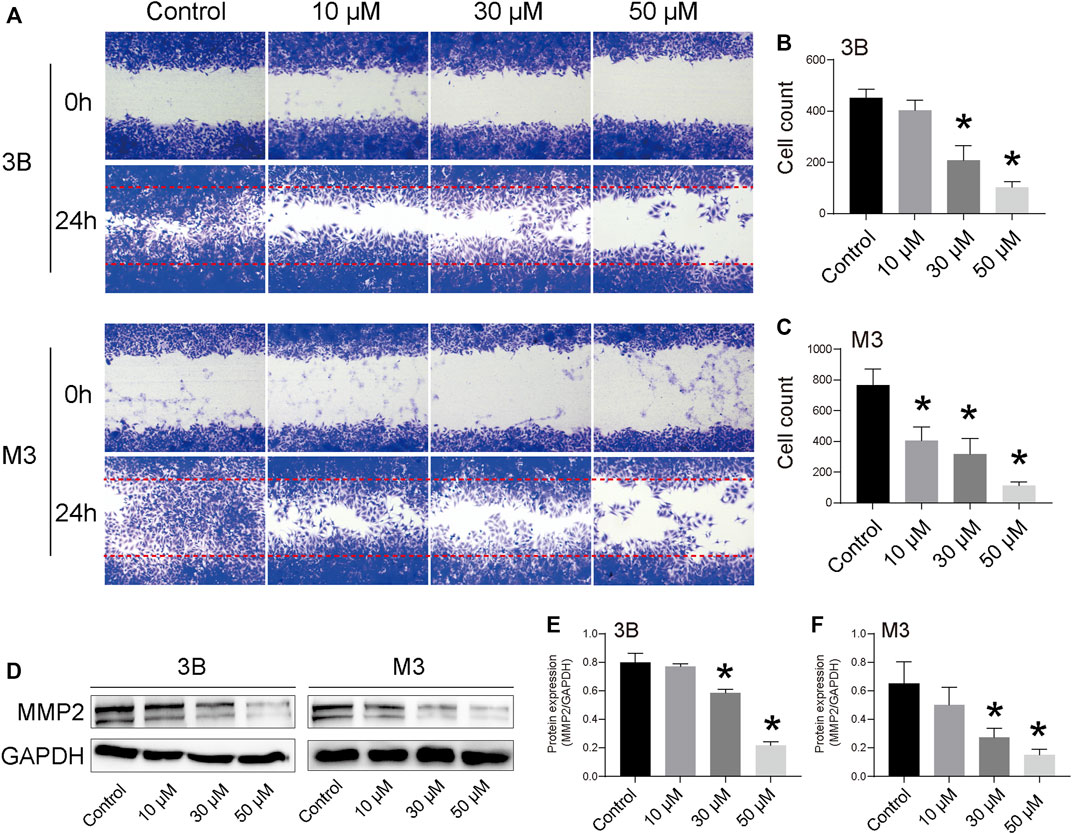
FIGURE 3. STA inhibits Hep3B and HCCLM3 cell migration. (A) Scratch assay was performed to determine migration of Hep3B and HCCLM3 cells treated with STA at for 48 h. (B,C) Quantification of the scratch image was performed by calculating the migrated cell counts in (A). (D–F) The representative bands of MMP2 in Hep3B and HCCLM3 cells treated with STA for 48 h. GAPDH was used as an internal control. Data shown are means ± SD; n = 3. *p < 0.05, significantly from control group.
3.3 STA regulates differentially expressed genes and pathway enrichment analysis
To investigate how STA regulates cell growth and proliferation, transcriptome analyses on cells treated with 30 μM STA and DMSO were performed. The global gene expression profile showed that STA regulated gene expression in Hep3B cells. Compared with the control group, 77 genes were upregulated and 136 genes were downregulated in the STA-treated group. Thus, STA significantly inhibited the transcription of genes, leading to the arrest of basic cell functions (Figure 4A). Subsequently, KEGG pathway enrichment analysis of differentially expressed genes showed that STA affected glucose metabolism of HCC cells, specifically galactose metabolism and fructose and pentose phosphate metabolism (Figure 4B). Gene ontology functional annotation also showed that STA was involved in the regulation of metabolic processes (Figure 4C).
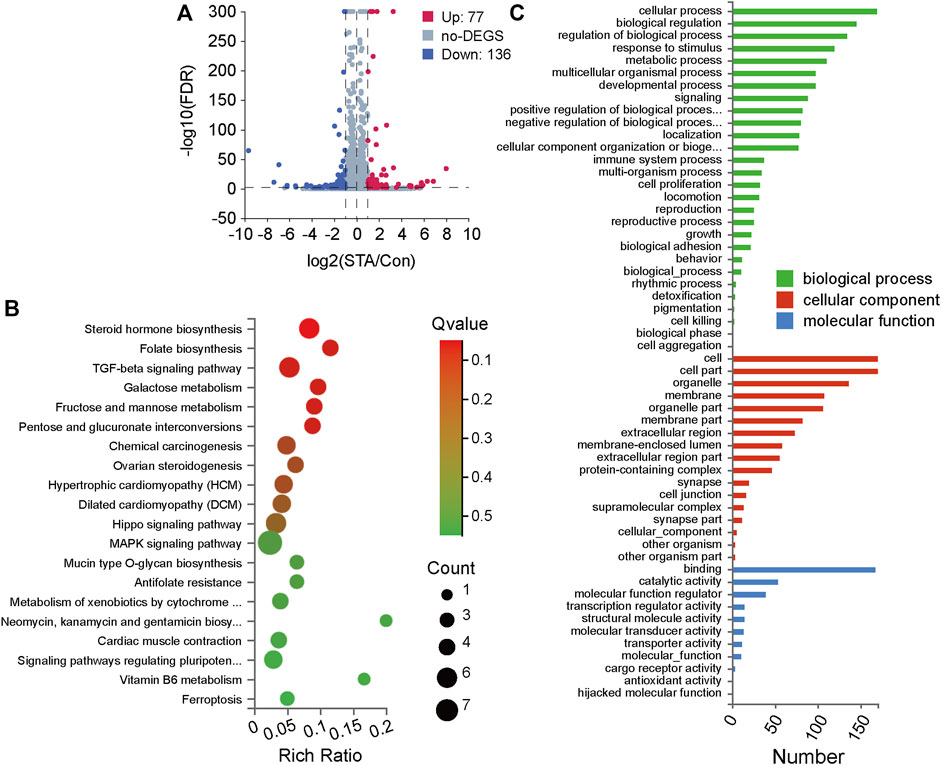
FIGURE 4. STA regulates the differentially expressed genes and pathway enrichment analysis in Hep3B cells. (A) Significant differentially expressed genes were shown in volcano plot. FC (fold change) > 1 was accepted as positive differentially expressed genes, up for 77; down for 136. (B) KEGG pathway enrichment analysis, a larger p-value (−Log10) shows a higher degree of enrichment. (C) GO annotations analysis of Hep3B cells treated with STA, compared with control group.
3.4 STA affects the glucose metabolism pathway of Hep3B cells
Metabolic reprogramming is an important feature of malignant tumors (Du et al., 2022; Liao et al., 2022). In cancer, malignant tumor cells respond to a variety of endogenous and exogenous cues to obtain metabolic adaptation, promoting the growth of tumor cells (Faubert et al., 2020). Previous KEGG pathway enrichment analyses showed that STA treatment could regulate glucose metabolism in Hep3B cells (Figure 4). Therefore, metabolomics was used for further validation analyses. GC-MS was used to explore the effect of STA on the production of primary metabolites in HCC cells. Principal component analysis (PCA) showed that metabolites induced by DMSO and STA (30 μM) were tightly clustered (Figure 5A). The difference between DMSO and STA (30 μM) was significant using OP-LSDA analysis to exclude non-significant variables and to evaluate only statistically significant signals (Figure 5B). STA treatment significantly downregulated the production of D-glucose, lactate, L-alanine, L-proline, and serine (Figure 5C). Furthermore, the differential metabolites were mainly enriched in fructose and pentose phosphate metabolism and glycolysis/gluconeogenesis (Figure 5D), suggesting that the antitumor effects of STA are mediated by the regulation of glucose metabolism.
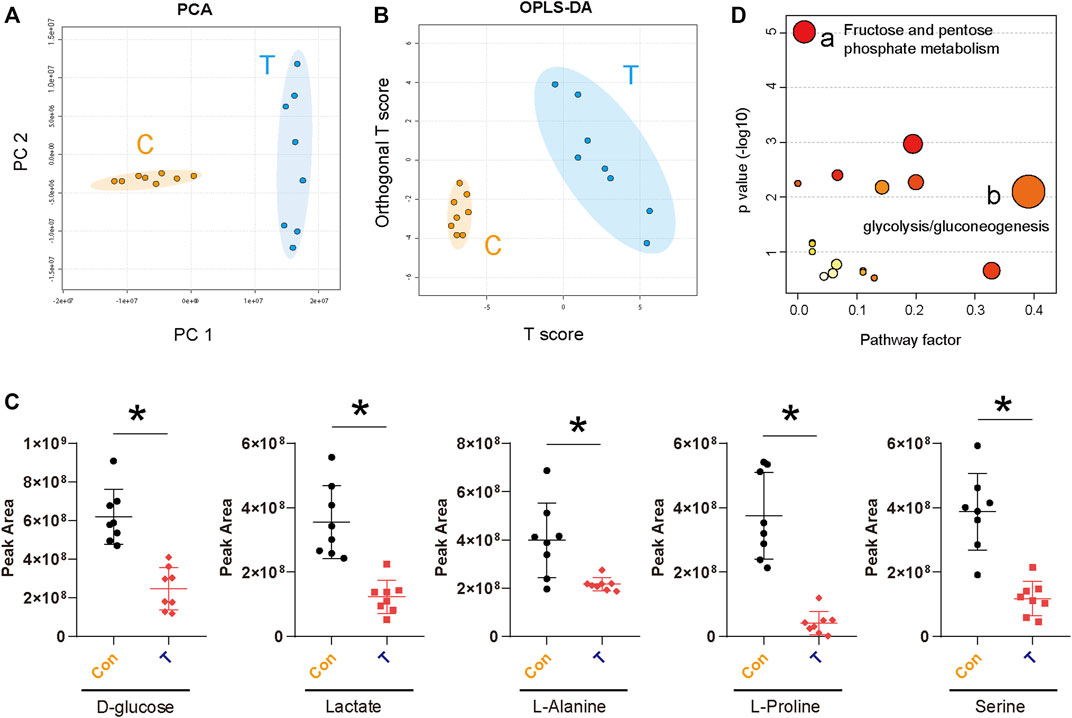
FIGURE 5. STA affects the glucose metabolism pathway of Hep3B cells. (A) The PCA score plot of Control and Drug group (30 μM), it represents samples in the groups were closely cluster to one another. (B) The OPLS-DA score plot of Control and Drug group revealed the clustering of samples in the training set. (C) Metabolites altered by STA treatment in Hep3B cells. n = 8 replicates per group. (D) Pathway enrichment of differential metabolites. Mainly enriched in fructose and pentose phosphate metabolism and glycolysis/gluconeogenesis.
3.5 STA impairs growth of Hep3B cells in vivo
To assess the antitumor effect of STA in vivo, Hep3B cells were subcutaneously injected into the axilla of 6-week-old nude mice and allowed to grow for 10 days. After 12 days of treatment, both STA and CDDP significantly reduced tumor weight and volume compared with the control group (Figures 6A–C). H&E staining of tumor samples showed that the number of cells in the STA-treated group was significantly lower than that in the control group. Furthermore, the expression of Ki67 decreased significantly with the increase in STA concentration (Figures 6D,E). Thus, STA had a significant inhibitory effect on tumor growth in vivo.
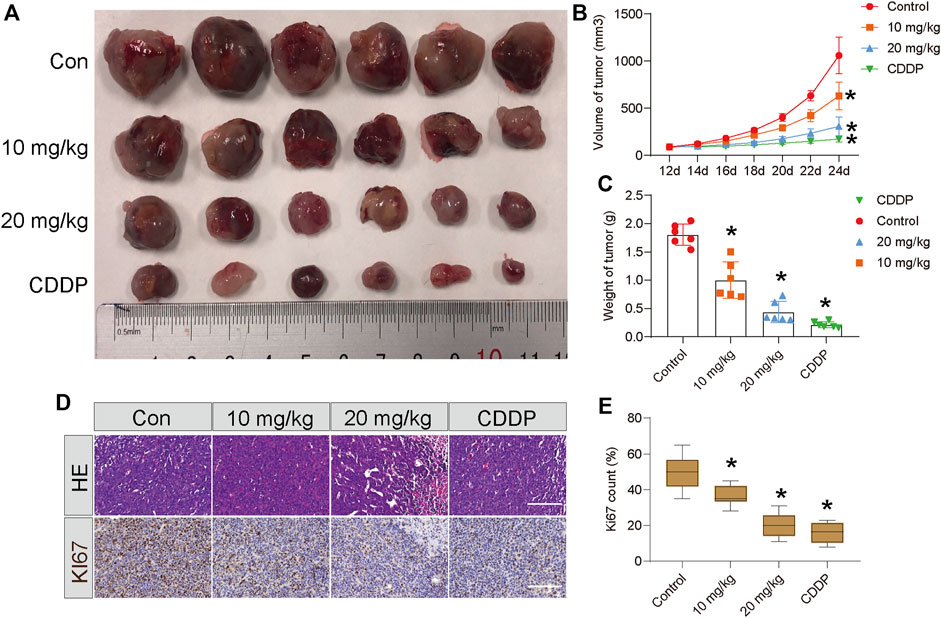
FIGURE 6. STA impairs the growth of Hep3B cells in vivo. Twenty-four nude mice were randomly divided into negative control group, positive control group (CDDP, 5 mg/kg, i.p., once two days), low-concentration treatment group (STA, 10 mg/kg, i.p., once two days) and high-concentration treatment group (STA, 20 mg/kg, i.p., once two days). (A) Photograph of tumors from indicated mice. (B) Tumor volume of indicated mice. (C) Tumor weight of indicated mice. (D) H&E staining and IHC of KI67 in indicated tumors. (E) Quantitative image analysis of KI67 in (D). Scale bar = 50 μm. All data were analyzed using unpaired Student t-Tests and are shown as the means ± SD. *p < 0.05.
4 Discussion
Globally, liver cancer is one of the most common fatal malignancies and is the fifth most common malignancy in China (Pan et al., 2022). Patients are commonly diagnosed at an advanced stage, leading to poor prognosis (Chen et al., 2020). Chemotherapy is the most accessible treatment option for patients with liver cancer (Sacco et al., 2017); however, only a small proportion of patients benefit from treatment, with considerable resistance within 6 months after the start of the treatment regimen (El-Serag et al., 2008). Furthermore, the long-term use of chemotherapy drugs (e.g., sorafenib) leads to toxicity and/or drug ineffectiveness (Colagrande et al., 2015; Tang et al., 2020). Therefore, new treatment options are urgently required for the treatment of liver cancer. Growing evidence has indicated that active ingredients in Chinese herbal medicines play a role in inhibiting mechanisms involved in the development of cancer, with stimulating mechanisms associated with disease prevention (Lam et al., 2022). These compounds have been shown to exert anticancer (Zhao et al., 2021), anti-inflammatory (Tao et al., 2022), and anti-oxidant effects (Gu et al., 2022), and may provide new treatment options for cancer.
As a monomeric TCM compound, STA retrieved form S. chinensis (Turcz.) Baill has multiple pharmacological activities, including anti-oxidant and anti-inflammatory activities, both in vivo and in vitro (Szopa et al., 2018; Fu et al., 2021). STA has been reported to mitigate liver injury induced by lipopolysaccharide, IL-1β, and cigarette smoke extract (Jiang et al., 2015). Indeed, chronic inflammation (Thadathil et al., 2022), smoking (Wen et al., 2022), and fatty liver disease (Paternostro and Trauner, 2022) are the main causes of liver cancer. Previous studies showed that STA can inhibit the proliferation of human hepatic stellate cells, leading to the speculation that STA might have an inhibitory effect on liver cancer. Furthermore, STA has been shown to inhibit the proliferation and migration of gastric cancer through the production of reactive oxygen species and JNK signaling pathway activation (Wang et al., 2020). Therefore, it was hypothesized that STA would have antiproliferative effects on liver cancer cells and inhibit cell clone formation and migration through inhibition of the related molecular mechanisms.
Herein, STA significantly suppressed cell proliferation and migration, and reduced the viability of liver cancer cells in vitro and in vivo. STA displayed potent inhibitory effects on proliferation by decreasing the expression of CDK1 and survivin. In addition, STA inhibited cell migration through the downregulation of MMP2 expression. Further transcriptome sequencing and KEGG signaling pathway enrichment data showed that the metabolic process in Hep3B cells treated with STA had changed. Growing evidence suggests that metabolic disorder is a significant hallmark of malignant tumors that controls key tumor biological processes such as the proliferation, migration, and invasion of tumor cells (Dai et al., 2022; Sun et al., 2022; Tan et al., 2022). Thus, by inhibiting the metabolic pathways of tumor cells, the occurrence and development of tumors could be prevented. Therefore, targeting tumor metabolism is a promising therapeutic approach (Wang et al., 2022b; DeBerardinis and Keshari, 2022; Reyes-Castellanos and Carrier, 2022). Metabonomic results indicated that STA treatment decreased the production of D-glucose, lactate, L-alanine, L-proline, and serine. Both lactate and serine have been reported to be associated with the development and exacerbation of tumors (Woo et al., 2016; Bogdanov et al., 2022). Herein, these differential metabolites were mainly enriched in fructose and pentose phosphate metabolism and glycolysis/gluconeogenesis. Our results suggest that STA can inhibit glucose metabolism in Hep3B cells.
Studies have shown that glucose metabolism disorder is a representative metabolic feature in HCC (Tsujimoto et al., 2022). An increase in the degree of glycolysis in tumors occurs to meet the energy demands of rapidly proliferating tumor cells, and glycolysis can also produce intermediate metabolites supporting the biosynthesis of nucleotides, amino acids, and lipids, thus allowing the rapid proliferation of tumor cells (Feng et al., 2020). Glucose metabolism is coupled to cell cycle progression, and glucose metabolism ensures adequate ATP and synthetic metabolites at different stages of the cell cycle (Cheung et al., 2022). 6-Phosphofructo-2-kinase (PFKFB3) can promote cell cycle progression through CDK1-mediated phosphorylation of P27 (Yalcin et al., 2014). In turn, the cell cycle is also involved in the regulation of glucose metabolism. Tang et al. revealed that CDK2 can increase glycolysis by inhibiting SIRT 5 in gastric cancer (Tang et al., 2018). High expression of survivin can shut down mitochondrial complex 1 activity, thereby switching neuroblastoma cells from OXPHOS to glycolysis (Hagenbuchner et al., 2016). Our western blotting results also showed that the protein expression of CDK1 and survivin was decreased in STA-treated HCC cells (Figure 2D). In addition, enhanced glycolysis promotes the conversion of pyruvate to lactate catalyzed by LDHA. Studies have confirmed that LDHA levels in tissues and plasma are significantly increased in patients with liver cancer, and increased LDHA levels are significantly associated with poor prognosis (Faloppi et al., 2016; Guo et al., 2019). Our results showed that STA could reduce lactate production in Hep3B cells (Figure 5C). Increased lactate production can induce a more acidic tumor microenvironment, which is conducive to tumor metastasis, angiogenesis, and immunosuppression (Li et al., 2022b). Angiogenesis is conducive to the delivery of oxygen and nutrients to tumor tissues, and meets the needs of rapid proliferation of tumor cells (Qi et al., 2022).
Furthermore, in vivo subcutaneous tumor-formation experiments in nude mice confirmed that STA could significantly inhibit the growth of solid tumors. Immunohistochemistry showed that the anti-tumor effect of STA was related to the inhibition of expression of Ki67, a marker of tumor proliferation (Figure 6D).
5 Conclusion
In summary, our findings suggest that STA treatment inhibits cell proliferation and migration by regulating glucose metabolism in HCC cells. The clone formation assay and cell scratch assay indicated that STA treatment reduced the proliferation and migration capacity of Hep3B and HCCLM3 cells in vitro. Further, transcriptome sequencing and metabolomics results showed that STA treatment could regulate glucose metabolism and reduce D-glucose and lactate production in Hep3B cells. The subcutaneous tumorigenesis assay in nude mice further verified that STA treatment inhibits the growth of HCC tumors in vivo. Based on our results, STA has the potential to be developed as a therapeutic agent for the treatment of liver cancer.
Data availability statement
The datasets presented in this study can be found in online repositories. The names of the repository/repositories and accession number(s) can be found in the following: NCBI BioProject PRJNA869496.
Ethics statement
The animal study was reviewed and approved by the Ethics Committee of Chongqing Medical University.
Author contributions
WZ and LY conceived and designed the experiments. FF and LP performed the experiment. JW and ML analyzed the data and prepared figures. FF and LH wrote the manuscript. All the authors read and approved the final manuscript.
Funding
This work was supported by National Natural Science Foundation of China (11832008); Innovation and Attracting Talents Program for College and University (“111” Project) (Grant No. B06023); Ministry of Education (Grant Number: CQKLBST-2017–011). Key Laboratory of Biorheological Science and Technology (Chongqing University), Ministry of Education, Open Foundation Project. Chongqing Natural Science Foundation (No. cstc2021jcyj-bshX0190).
Acknowledgments
The authors thank members of the LY laboratory for helpful discussion and the Innovation and Attracting Talents Program for College and University for providing advanced facilities.
Conflict of interest
The authors declare that the research was conducted in the absence of any commercial or financial relationships that could be construed as a potential conflict of interest.
Publisher’s note
All claims expressed in this article are solely those of the authors and do not necessarily represent those of their affiliated organizations, or those of the publisher, the editors and the reviewers. Any product that may be evaluated in this article, or claim that may be made by its manufacturer, is not guaranteed or endorsed by the publisher.
Abbreviations
CCK-8, cell counting kit; CDDP, cisplatin; DMSO, dimethyl sulfoxide; EDU, 5-Ethynyl-2′-deoxyuridine; FBS, fetal bovine serum; GLUTs, glucose transporters; HCC, hepatocellular carcinoma; H&E, hematoxylin and eosin; OXPHOS, oxidative phosphorylation; LDH, lactate dehydrogenase; PFKFB3, 6-Phosphofructo-2-kinase; PKM2, pyruvate kinase M2; TCM, traditional Chinese herbal medicines; STA, Schisantherin A.
References
Bogdanov, A., Chubenko, V., Volkov, N., Moiseenko, F., and Moiseyenko, V. (2022). Tumor acidity: From hallmark of cancer to target of treatment. Front. Oncol. 12, 979154. doi:10.3389/fonc.2022.979154
Chen, Z., Xie, H., Hu, M., Huang, T., Hu, Y., Sang, N., et al. (2020). Recent progress in treatment of hepatocellular carcinoma. Am. J. Cancer Res. 10 (9), 2993–3036.
Chen, Z., and Ye, S. Y. (2022). Research progress on antiviral constituents in traditional Chinese medicines and their mechanisms of action. Pharm. Biol. 60 (1), 1063–1076. doi:10.1080/13880209.2022.2074053
Cheung, A. H., Hui, C. H. L., Wong, K. Y., Liu, X., Chen, B., Kang, W., et al. (2022). Out of the cycle: Impact of cell cycle aberrations on cancer metabolism and metastasis. Intl. J. Cancer. doi:10.1002/ijc.34288
Colagrande, S., Regini, F., Taliani, G. G., Nardi, C., and Inghilesi, A. L. (2015). Advanced hepatocellular carcinoma and sorafenib: Diagnosis, indications, clinical and radiological follow-up. World J. Hepatol. 7 (8), 1041–1053. doi:10.4254/wjh.v7.i8.1041
Dai, W., Xiang, W., Han, L., Yuan, Z., Wang, R., Ma, Y., et al. (2022). PTPRO represses colorectal cancer tumorigenesis and progression by reprogramming fatty acid metabolism. Cancer Commun. 42, 848–867. doi:10.1002/cac2.12341
DeBerardinis, R. J., and Keshari, K. R. (2022). Metabolic analysis as a driver for discovery, diagnosis, and therapy. Cell 185 (15), 2678–2689. doi:10.1016/j.cell.2022.06.029
Du, D., Liu, C., Qin, M., Zhang, X., Xi, T., Yuan, S., et al. (2022). Metabolic dysregulation and emerging therapeutical targets for hepatocellular carcinoma. Acta Pharm. Sin. B 12 (2), 558–580. doi:10.1016/j.apsb.2021.09.019
El-Serag, H. B., Marrero, J. A., Rudolph, L., and Reddy, K. R. (2008). Diagnosis and treatment of hepatocellular carcinoma. Gastroenterology 134 (6), 1752–1763. doi:10.1053/j.gastro.2008.02.090
Faloppi, L., Bianconi, M., Memeo, R., Casadei Gardini, A., Giampieri, R., Bittoni, A., et al. (2016). Lactate dehydrogenase in hepatocellular carcinoma: Something old, something new. Biomed. Res. Int. 2016, 7196280. doi:10.1155/2016/7196280
Fan, Y., Ma, Z., Zhao, L., Wang, W., Gao, M., Jia, X., et al. (2020). Anti-tumor activities and mechanisms of traditional Chinese medicines formulas: A review. Biomed. Pharmacother. 132, 110820. doi:10.1016/j.biopha.2020.110820
Faubert, B., Solmonson, A., and DeBerardinis, R. J. (2020). Metabolic reprogramming and cancer progression. Science 368 (6487), eaaw5473. doi:10.1126/science.aaw5473
Feng, J., Li, J., Wu, L., Yu, Q., Ji, J., Wu, J., et al. (2020). Emerging roles and the regulation of aerobic glycolysis in hepatocellular carcinoma. J. Exp. Clin. Cancer Res. 39 (1), 126. doi:10.1186/s13046-020-01629-4
Foerster, F., Gairing, S. J., Muller, L., and Galle, P. R. (2022). NAFLD-driven HCC: Safety and efficacy of current and emerging treatment options. J. Hepatol. 76 (2), 446–457. doi:10.1016/j.jhep.2021.09.007
Fu, M., Liu, Y., Cheng, H., Xu, K., and Wang, G. (2022). Coptis chinensis and dried ginger herb combination inhibits gastric tumor growth by interfering with glucose metabolism via LDHA and SLC2A1. J. Ethnopharmacol. 284, 114771. doi:10.1016/j.jep.2021.114771
Fu, Y., Xiao, Z., Tian, X., Liu, W., Xu, Z., Yang, T., et al. (2021). The novel Chinese medicine JY5 formula alleviates hepatic fibrosis by inhibiting the notch signaling pathway. Front. Pharmacol. 12, 671152. doi:10.3389/fphar.2021.671152
Gong, J., and Wang, X. (2018). Schisantherin A protects renal tubular epithelial cells from hypoxia/reoxygenation injury through the activation of PI3K/Akt signaling pathway. J. Biochem. Mol. Toxicol. 32, e22160. doi:10.1002/jbt.22160
Gu, Q., Liu, Y., Zhen, L., Zhao, T., Luo, L., Zhang, J., et al. (2022). The structures of two glucomannans from Bletilla formosana and their protective effect on inflammation via inhibiting NF-κB pathway. Carbohydr. Polym. 292, 119694. doi:10.1016/j.carbpol.2022.119694
Gui, Y., Yang, Y., Xu, D., Tao, S., and Li, J. (2020). Schisantherin A attenuates sepsis-induced acute kidney injury by suppressing inflammation via regulating the NRF2 pathway. Life Sci. 258, 118161. doi:10.1016/j.lfs.2020.118161
Guo, Y., Li, X., Sun, X., Wang, J., Yang, X., Zhou, X., et al. (2019). Combined aberrant expression of NDRG2 and LDHA predicts hepatocellular carcinoma prognosis and mediates the anti-tumor effect of gemcitabine. Int. J. Biol. Sci. 15 (9), 1771–1786. doi:10.7150/ijbs.35094
Hagenbuchner, J., Kiechl-Kohlendorfer, U., Obexer, P., and Ausserlechner, M. J. (2016). BIRC5/Survivin as a target for glycolysis inhibition in high-stage neuroblastoma. Oncogene 35 (16), 2052–2061. doi:10.1038/onc.2015.264
Han, S., Bi, S., Guo, T., Sun, D., Zou, Y., Wang, L., et al. (2022). Nano co-delivery of Plumbagin and Dihydrotanshinone I reverses immunosuppressive TME of liver cancer. J. Control. Release 348, 250–263. doi:10.1016/j.jconrel.2022.05.057
Hu, Y., Yang, Z., Bao, D., Ni, J. S., and Lou, J. (2019). miR-455-5p suppresses hepatocellular carcinoma cell growth and invasion via IGF-1R/AKT/GLUT1 pathway by targeting IGF-1R. Pathol. Res. Pract. 215 (12), 152674. doi:10.1016/j.prp.2019.152674
Jiang, Y., Fan, X., Wang, Y., Tan, H., Chen, P., Zeng, H., et al. (2015). Hepato-protective effects of six schisandra lignans on acetaminophen-induced liver injury are partially associated with the inhibition of CYP-mediated bioactivation. Chem. Biol. Interact. 231, 83–89. doi:10.1016/j.cbi.2015.02.022
Khatib, S. A., Ma, L., Dang, H., Forgues, M., Chung, J. Y., Ylaya, K., et al. (2022). Single-cell biology uncovers apoptotic cell death and its spatial organization as a potential modifier of tumor diversity in HCC. Hepatology 76 (3), 599–611. doi:10.1002/hep.32345
Koppenol, W. H., Bounds, P. L., and Dang, C. V. (2011). Otto Warburg's contributions to current concepts of cancer metabolism. Nat. Rev. Cancer 11 (5), 325–337. doi:10.1038/nrc3038
Lam, C. S., Koon, H. K., Ma, C. T., Au, K. Y., Zuo, Z., Chung, V. C-H., et al. (2022). Real-world data on herb-drug interactions in oncology: A scoping review of pharmacoepidemiological studies. Phytomedicine. 103, 154247. doi:10.1016/j.phymed.2022.154247
Li, L., Xu, H., Qu, L., Xu, K., and Liu, X. (2022). Daidzin inhibits hepatocellular carcinoma survival by interfering with the glycolytic/gluconeogenic pathway through downregulation of TPI1. Biofactors 48 (4), 883–896. doi:10.1002/biof.1826
Li, S., Li, J., Dai, W., Zhang, Q., Feng, J., Wu, L., et al. (2017). Genistein suppresses aerobic glycolysis and induces hepatocellular carcinoma cell death. Br. J. Cancer 117 (10), 1518–1528. doi:10.1038/bjc.2017.323
Li, X., Yang, Y., Zhang, B., Lin, X., Fu, X., An, Y., et al. (2022). Lactate metabolism in human health and disease. Signal Transduct. Target. Ther. 7 (1), 305. doi:10.1038/s41392-022-01151-3
Liao, W., Du, J., Wang, Z., Feng, Q., Liao, M., Liu, H., et al. (2022). The role and mechanism of noncoding RNAs in regulation of metabolic reprogramming in hepatocellular carcinoma. Int. J. Cancer 151 (3), 337–347. doi:10.1002/ijc.34040
Liu, C., Yang, S., Wang, K., Bao, X., Liu, Y., Zhou, S., et al. (2019). Alkaloids from traditional Chinese medicine against hepatocellular carcinoma. Biomed. Pharmacother. 120, 109543. doi:10.1016/j.biopha.2019.109543
Locasale, J. W., and Cantley, L. C. (2010). Altered metabolism in cancer. BMC Biol. 8, 88. doi:10.1186/1741-7007-8-88
Lu, Y., Yang, A., Quan, C., Pan, Y., Zhang, H., Li, Y., et al. (2022). A single-cell atlas of the multicellular ecosystem of primary and metastatic hepatocellular carcinoma. Nat. Commun. 13 (1), 4594. doi:10.1038/s41467-022-32283-3
Pan, L., Feng, F., Wu, J., Fan, S., Han, J., Wang, S., et al. (2022). Demethylzeylasteral targets lactate by inhibiting histone lactylation to suppress the tumorigenicity of liver cancer stem cells. Pharmacol. Res. 181, 106270. doi:10.1016/j.phrs.2022.106270
Pan, L., Feng, F., Wu, J., Li, L., Xu, H., Yang, L., et al. (2021). Diosmetin inhibits cell growth and proliferation by regulating the cell cycle and lipid metabolism pathway in hepatocellular carcinoma. Food Funct. 12 (23), 12036–12046. doi:10.1039/d1fo02111g
Paternostro, R., and Trauner, M. (2022). Current treatment of non-alcoholic fatty liver disease. J. Intern. Med. 292 (2), 190–204. doi:10.1111/joim.13531
Qi, S., Deng, S., Lian, Z., and Yu, K. (2022). Novel drugs with high efficacy against tumor angiogenesis. Int. J. Mol. Sci. 23 (13), 6934. doi:10.3390/ijms23136934
Qu, L., Wang, C., Xu, H., Li, L., Liu, Y., Wan, Q., et al. (2012). Atractylodin targets GLA to regulate D-mannose metabolism to inhibit osteogenic differentiation of human valve interstitial cells and ameliorate aortic valve calcification. Phytother. Res.. doi:10.1002/ptr.7628
Reyes-Castellanos, G., and Carrier, A. (2022). A novel anticancer pharmacological agent targeting mitochondrial complex I. Trends Pharmacol. Sci. 43 (9), 706–708. doi:10.1016/j.tips.2022.03.007
Sacco, R., Tapete, G., Simonetti, N., Sellitri, R., Natali, V., Melissari, S., et al. (2017). Transarterial chemoembolization for the treatment of hepatocellular carcinoma: A review. J. Hepatocell. Carcinoma 4, 105–110. doi:10.2147/JHC.S103661
Seyfried, T. N., Flores, R. E., Poff, A. M., and D'Agostino, D. P. (2014). Cancer as a metabolic disease: Implications for novel therapeutics. Carcinogenesis 35 (3), 515–527. doi:10.1093/carcin/bgt480
Sheng, T., Mao, X. B., and Zhang, S. H. (2020). CaMKKβ regulates proliferation, apoptosis, and glycolysis of hepatocellular carcinoma via PI3K/AKT pathway. Ann. Palliat. Med. 9 (6), 3857–3869. doi:10.21037/apm-20-1789
Sun, L., Zhang, H., and Gao, P. (2022). Metabolic reprogramming and epigenetic modifications on the path to cancer. Protein Cell 13 (12), 877–919. doi:10.1007/s13238-021-00846-7
Sung, H., Ferlay, J., Siegel, R. L., Laversanne, M., Soerjomataram, I., Jemal, A., et al. (2021). Global cancer statistics 2020: GLOBOCAN estimates of incidence and mortality worldwide for 36 cancers in 185 countries. Ca. Cancer J. Clin. 71 (3), 209–249. doi:10.3322/caac.21660
Szopa, A., Dziurka, M., Warzecha, A., Kubica, P., Klimek-Szczykutowicz, M., and Ekiert, H. (2018). Targeted lignan profiling and anti-inflammatory properties of schisandra rubriflora and schisandra chinensis extracts. Molecules 23 (12), E3103. doi:10.3390/molecules23123103
Tan, Y., Li, J., Zhao, G., Huang, K. C., Cardenas, H., Wang, Y., et al. (2022). Metabolic reprogramming from glycolysis to fatty acid uptake and beta-oxidation in platinum-resistant cancer cells. Nat. Commun. 13 (1), 4554. doi:10.1038/s41467-022-32101-w
Tang, W., Chen, Z., Zhang, W., Cheng, Y., Zhang, B., Wu, F., et al. (2020). The mechanisms of sorafenib resistance in hepatocellular carcinoma: Theoretical basis and therapeutic aspects. Signal Transduct. Target. Ther. 5 (1), 87. doi:10.1038/s41392-020-0187-x
Tang, Z., Li, L., Tang, Y., Xie, D., Wu, K., Wei, W., et al. (2018). CDK2 positively regulates aerobic glycolysis by suppressing SIRT5 in gastric cancer. Cancer Sci. 109 (8), 2590–2598. doi:10.1111/cas.13691
Tao, Y. W., Yang, L., Chen, S. Y., Zhang, Y., Zeng, Y., Wu, J. S., et al. (2022). Pivotal regulatory roles of traditional Chinese medicine in ischemic stroke via inhibition of NLRP3 inflammasome. J. Ethnopharmacol. 294, 115316. doi:10.1016/j.jep.2022.115316
Thadathil, N., Selvarani, R., Mohammed, S., Nicklas, E. H., Tran, A. L., Kamal, M., et al. (2022). Senolytic treatment reduces cell senescence and necroptosis in Sod1 knockout mice that is associated with reduced inflammation and hepatocellular carcinoma. Aging Cell 21, e13676. doi:10.1111/acel.13676
Tsujimoto, G., Ito, R., Yoshikawa, K., Ueki, C., and Okada, N. (2022). NFYA promotes the anti-tumor effects of gluconeogenesis in hepatocellular carcinoma through the regulation of PCK1 expression. Front. Cell Dev. Biol. 10, 983599. doi:10.3389/fcell.2022.983599
Wang, H., Che, J., Cui, K., Zhuang, W., Li, H., Sun, J., et al. (2021). Schisantherin A ameliorates liver fibrosis through TGF-β1mediated activation of TAK1/MAPK and NF-κB pathways in vitro and in vivo. Phytomedicine. 88, 153609. doi:10.1016/j.phymed.2021.153609
Wang, X., Zhang, P., and Deng, K. (2022). MYC promotes LDHA expression through MicroRNA-122-5p to potentiate glycolysis in hepatocellular carcinoma. Anal. Cell. Pathol. 2022, 1435173. doi:10.1155/2022/1435173
Wang, Z., Wang, M., Zhang, M., Xu, K., Zhang, X., Xie, Y., et al. (2022). High-affinity SOAT1 ligands remodeled cholesterol metabolism program to inhibit tumor growth. BMC Med. 20 (1), 292. doi:10.1186/s12916-022-02436-8
Wang, Z., Yu, K., Hu, Y., Su, F., Gao, Z., Hu, T., et al. (2020). Schisantherin A induces cell apoptosis through ROS/JNK signaling pathway in human gastric cancer cells. Biochem. Pharmacol. 173, 113673. doi:10.1016/j.bcp.2019.113673
Wen, Q., Chan, K. H., Shi, K., Lv, J., Guo, Y., Pei, P., et al. (2022). Tobacco smoking and solid fuels for cooking and risk of liver cancer: A prospective cohort study of 0.5 million Chinese adults. Int. J. Cancer 151 (2), 181–190. doi:10.1002/ijc.33977
Woo, C. C., Chen, W. C., Teo, X. Q., Radda, G. K., and Lee, P. T. H. (2016). Downregulating serine hydroxymethyltransferase 2 (SHMT2) suppresses tumorigenesis in human hepatocellular carcinoma. Oncotarget 7 (33), 53005–53017. doi:10.18632/oncotarget.10415
Xia, H., Chen, J., Gao, H., Kong, S. N., Deivasigamani, A., Shi, M., et al. (2020). Hypoxia-induced modulation of glucose transporter expression impacts (18)F-fluorodeoxyglucose PET-CT imaging in hepatocellular carcinoma. Eur. J. Nucl. Med. Mol. Imaging 47 (4), 787–797. doi:10.1007/s00259-019-04638-4
Yalcin, A., Clem, Y., Imbert-Fernandez, Y., Ozcan, S. C., Peker, S., O'Neal, J., et al. (2014). 6-Phosphofructo-2-kinase (PFKFB3) promotes cell cycle progression and suppresses apoptosis via Cdk1-mediated phosphorylation of p27. Cell Death Dis. 5 (7), e1337. doi:10.1038/cddis.2014.292
Yang, J., Zhao, Y., Zhou, Y., Wei, X., Wang, H., Si, N., et al. (2022). Advanced nanomedicines for the regulation of cancer metabolism. Biomaterials 286, 121565. doi:10.1016/j.biomaterials.2022.121565
Ye, G., Qin, Y., Wang, S., Pan, D., Xu, S., Wu, C., et al. (2019). Lamc1 promotes the Warburg effect in hepatocellular carcinoma cells by regulating PKM2 expression through AKT pathway. Cancer Biol. Ther. 20 (5), 711–719. doi:10.1080/15384047.2018.1564558
Zhang, X., Li, Y., Ma, Y., Yang, L., Wang, T., Meng, X., et al. (2018). Yes-associated protein (YAP) binds to HIF-1α and sustains HIF-1α protein stability to promote hepatocellular carcinoma cell glycolysis under hypoxic stress. J. Exp. Clin. Cancer Res. 37 (1), 216. doi:10.1186/s13046-018-0892-2
Zhao, W., Liu, J., Li, Y., Chen, Z., Qi, D., and Zhang, Z. (2021). Immune effect of active components of traditional Chinese medicine on triple-negative breast cancer. Front. Pharmacol. 12, 731741. doi:10.3389/fphar.2021.731741
Keywords: schisantherin A, hepatocellular carcinoma, fructose and pentose phosphate metabolism, galactose metabolism, traditional Chinese medicine
Citation: Feng F, Pan L, Wu J, Liu M, He L, Yang L and Zhou W (2022) Schisantherin A inhibits cell proliferation by regulating glucose metabolism pathway in hepatocellular carcinoma. Front. Pharmacol. 13:1019486. doi: 10.3389/fphar.2022.1019486
Received: 25 August 2022; Accepted: 26 October 2022;
Published: 08 November 2022.
Edited by:
Cyril Corbet, Fonds National de la Recherche Scientifique (FNRS), BelgiumReviewed by:
Yiguang Lin, University of Technology Sydney, AustraliaCaiyan Wang, Guangzhou University of Chinese Medicine, China
Copyright © 2022 Feng, Pan, Wu, Liu, He, Yang and Zhou. This is an open-access article distributed under the terms of the Creative Commons Attribution License (CC BY). The use, distribution or reproduction in other forums is permitted, provided the original author(s) and the copyright owner(s) are credited and that the original publication in this journal is cited, in accordance with accepted academic practice. No use, distribution or reproduction is permitted which does not comply with these terms.
*Correspondence: Wei Zhou, emhvdXdlaTk5OEBjcXUuZWR1LmNu