- 1Department of Pharmaceutical Science, College of Pharmacy and Pharmaceutical Sciences, Florida A&M University, Tallahassee, FL, United States
- 2Department of Biology, College of Science and Technology, Florida A&M University, Tallahassee, FL, United States
- 3Department of Molecular Pharmacology, Albert Einstein College of Medicine, New York, NY, United States
Chronic exposure to elevated levels of manganese via occupational or environmental settings causes a neurological disorder known as manganism, resembling the symptoms of Parkinson’s disease, such as motor deficits and cognitive impairment. Numerous studies have been conducted to characterize manganese’s neurotoxicity mechanisms in search of effective therapeutics, including natural and synthetic compounds to treat manganese toxicity. Several potential molecular targets of manganese toxicity at the epigenetic and transcriptional levels have been identified recently, which may contribute to develop more precise and effective gene therapies. This review updates findings on manganese-induced neurotoxicity mechanisms on intracellular insults such as oxidative stress, inflammation, excitotoxicity, and mitophagy, as well as transcriptional dysregulations involving Yin Yang 1, RE1-silencing transcription factor, transcription factor EB, and nuclear factor erythroid 2-related factor 2 that could be targets of manganese neurotoxicity therapies. This review also features intracellular proteins such as PTEN-inducible kinase 1, parkin, sirtuins, leucine-rich repeat kinase 2, and α-synuclein, which are associated with manganese-induced dysregulation of autophagy/mitophagy. In addition, newer therapeutic approaches to treat manganese’s neurotoxicity including natural and synthetic compounds modulating excitotoxicity, autophagy, and mitophagy, were reviewed. Taken together, in-depth mechanistic knowledge accompanied by advances in gene and drug delivery strategies will make significant progress in the development of reliable therapeutic interventions against manganese-induced neurotoxicity.
1 Introduction
Manganese (Mn) is an essential trace element in various cellular functions in the body, serving as a cofactor of several enzymes such as glutamine synthetase (Wedler and Denman, 1984) and Mn superoxide dismutase (MnSOD) (Buettner et al., 2006). The daily reference intake (2.3 mg/day for men and 1.8 mg/day for women) of Mn is required for physiological functions and acquired through a regular diet (Aschner and Aschner, 2005). However, chronic exposure to elevated level of Mn primarily via occupational and environmental settings (Lucchini et al., 2007; Lucchini et al., 2012) results in its accumulation in the basal ganglia of the brain, causing a neurological disorder referred to as manganism, resembling symptoms of Parkinson’s disease (PD) (Olanow, 2004; Aschner and Aschner, 2005; Bowman and Aschner, 2014). Chronic exposure to elevated level of Mn by inhalation in South African miners contributed to severity of parkinsonism-like symptoms, such as motor deficits (Racette et al., 2021a) as well as greater depression and anxiety (Racette et al., 2021b). Clinical studies have also shown that elevated level of Mn is correlated with cognitive and memory deficits in children and adults (Zhang et al., 2021; Heng et al., 2022), suggesting a link between Mn toxicity and neurocognitive disorders such as Alzheimer’s disease (AD) and dementia. The patients with a pathologically elevated level of Mn showed behavioral defects and irreversible neurological disorders (Olanow, 2004; Cersosimo and Koller, 2006; Kwakye et al., 2015; Harischandra et al., 2019a).
Mechanisms of Mn-induced neurotoxicity have been extensively studied, including oxidative stress, mitochondrial dysfunction, glutamate excitotoxicity, protein misfolding, inflammation, autophagy, mitophagy, endoplasmic reticulum stress, and apoptosis (Martinez-Finley et al., 2013; Harischandra et al., 2019a; Pajarillo et al., 2020a; Soares et al., 2020; Tinkov et al., 2021). Identifying the molecular targets, such as transcription factors (TFs) and intracellular proteins involved in Mn-induced neurotoxicity, could be critical for developing therapeutic strategies against Mn’s neurotoxicity. Several TFs have been found as potential molecular targets of Mn’s toxicity, namely RE1-silencing transcription factor (REST; neuron-restrictive silencer factor, NRSF) (Pajarillo et al., 2020a; Pajarillo et al., 2022a), Yin Yang 1 (YY1) (Karki et al., 2014a; Karki et al., 2015a), transcription factor EB (TFEB) (Zhang et al., 2020) and nuclear factor erythroid 2-related factor 2 (Nrf2) (Ijomone et al., 2022). Some intracellular proteins, particularly related to PD, were also dysregulated by Mn’s insults. These include leucine-rich repeat kinase 2 (LRRK2), PTEN-induced kinase 1 (PINK1), parkin, and α-synuclein (α-Syn) (Chen et al., 2018; Kim et al., 2019; Liu et al., 2021a; Liu et al., 2022a; Cao et al., 2022).
Numerous studies have been conducted in search of potential therapeutics for Mn’s toxicity, including antioxidants, anti-inflammatory, and anti-excitotoxic agents. Although the precise molecular targets of Mn-induced neurotoxicity are not fully understood, several agents have been shown to exhibit protective effects against Mn-induced neurotoxicity in both in vivo and in vitro models. Antioxidants are one of the most studied therapeutics against Mn-induced neurotoxicity. Accumulating evidence reveals that many natural and synthetic compounds inhibited Mn-induced oxidative stress (Bahar et al., 2017a; Ommati et al., 2020; Cong et al., 2021). Several compounds such as estrogens and riluzole attenuated Mn-induced glutamatergic excitotoxic neuronal injury by upregulating astrocytic glutamate transporters such as excitatory amino acid transporter 1 (EAAT1) and EAAT2 [glutamate-aspartate transporter (GLAST) and glutamate transporter 1 (GLT-1) in rodents, respectively] (Fumagalli et al., 2008; Lee et al., 2009a). A disaccharide trehalose attenuated Mn-induced neurotoxicity by modulating Mn-impaired autophagy and mitophagy (Liu et al., 2019; Jing et al., 2020). Chelating agents such as ethylenediaminetetraacetic acid (EDTA) (Discalzi et al., 2000) and para-aminosalicylic acid (PAS) have been shown to reduce acute Mn toxicity by promoting Mn excretion from the body (Jiang et al., 2006; Zheng et al., 2009). Intravenous EDTA chelation therapy has been clinically the primary treatment for manganism patients (for review, see Anagianni and Tuschl, 2019). However, several reports on its effectiveness have been controversial, and treatment outcome varies according to many factors, such as patient age, disease severity, and etiology (Tuschl et al., 2016; Lee and Shin, 2022). The lack of effective therapeutic approaches against Mn-induced neurotoxicity warrants the development of more efficacious drugs and targeted therapies.
In this review, we summarize the molecular mechanisms of Mn-induced neurotoxicity and potential molecular targets for neurotherapeutics (Figure 1). We also discuss protective agents with efficacy against Mn-induced neurotoxicity, such as antioxidants, anti-inflammatory agents, and modulators of glutamate transporters/receptors and autophagy/mitophagy. Understanding the mechanisms of Mn’s neurotoxicity associated with targeted modulators will greatly contribute to developing effective neurotherapeutics against manganism.
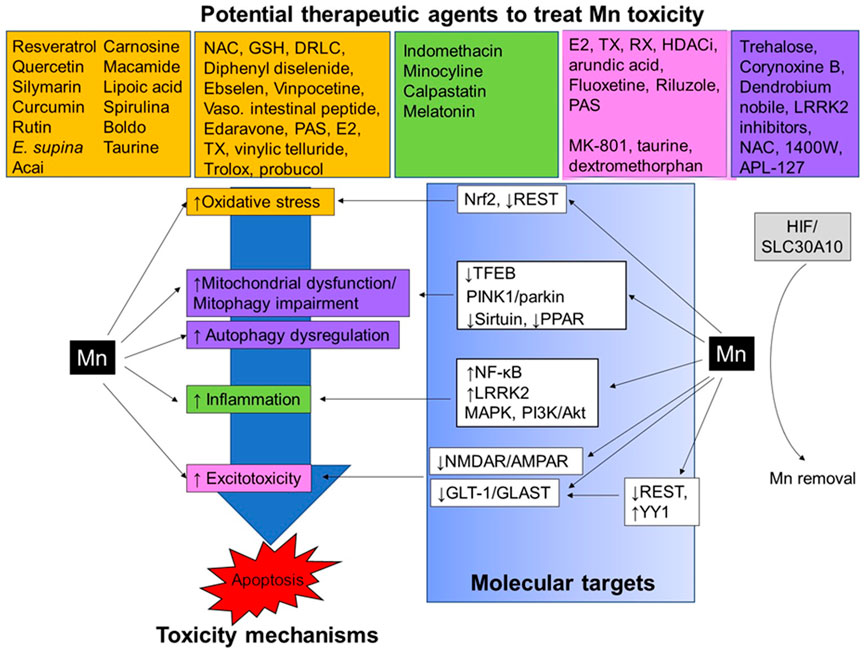
FIGURE 1. Neurotherapeutics and their proposed mechanism of action against Mn-induced neurotoxicity. Mn exposure causes several neurotoxic effects in the CNS, for example, oxidative stress, impairment of autophagy/mitophagy, mitochondrial dysfunction, inflammation, and excitotoxicity, leading to apoptotic cell death. Several TFs, including Nrf2, REST, TFEB, NF-κB, YY1, and HIF, as well as intracellular proteins such as sirtuin, LRRK2, PPAR, PINK1/parkin, MAPK, and PI3K/Akt, are involved in the regulation of these molecular mechanisms in Mn-induced neurotoxicity. Neurotherapeutic agents showing antioxidant (orange), anti-inflammatory (green), anti-excitotoxic (pink), and modulators of autophagy/mitophagy (purple) have demonstrated protective effects against Mn-induced neurotoxicity in in vivo and in vitro experimental settings.
2 Mechanisms of Mn-induced toxicity in the brain
Multiple mechanisms of Mn-induced neurotoxicity have been reported and some of the established mechanisms of Mn toxicity are discussed in this review. The findings indicate that the dysregulations of these mechanisms may contribute to the onset and progression of manganism, potentially by decreasing gene expression of antioxidant enzymes, glutamate transporters, antiapoptotic proteins or increasing proinflammatory cytokine genes and proapoptotic proteins as well as impairing mitophagy/autophagy genes. Thus, understanding the regulation of molecular pathways involved in Mn toxicity may pinpoint target molecules that can modulate various pathways for neuroprotection.
2.1 Oxidative stress
Oxidative stress is implicated in a wide range of pathological processes, especially in neurodegenerative diseases such as PD and AD. Mn generated reactive oxygen species (ROS) and subsequent oxidative damage to neural cells by increasing ROS levels in the central nervous system (CNS) (Cordova et al., 2013; da Silva Santos et al., 2014; Maddirala et al., 2015). Mn caused oxidative stress in various regions of the rodent brain, particularly in the basal ganglia, such as globus pallidus, striatum, and substantia nigra (Ali et al., 1995; Dobson et al., 2003; Erikson et al., 2004). Mn-induced mitochondrial impairment significantly contributes to elevated ROS levels as Mn’s inhibition of mitochondrial ATP production increased electron leakage and ROS production (Scholte, 1988; Chen et al., 2001). Trivalent Mn3+, an oxidized form of Mn2+, induced higher oxidative damage, given that Mn3+ has a greater oxidative stress potential than that of Mn2+ (Ali et al., 1995; HaMai et al., 2001; Reaney and Smith, 2005). In addition to the direct production of ROS, Mn also dysregulated antioxidative defense systems (Szpetnar et al., 2016) by reducing the synthesis of the oxidative scavenger glutathione (GSH), thus depleting its levels and further exacerbating the oxidative insult of Mn (Yang et al., 2018).
2.2 Mitochondrial impairment and mitophagy
Mitochondria are dynamic organelles primarily for ATP production, and their dysfunction contributes to the incidences of neurodegenerative diseases. Upon entry into the cells, Mn preferentially accumulates within the mitochondria which are well-established targets of Mn-induced neurotoxicity (Alaimo et al., 2014; Sarkar et al., 2018). Mn disrupts mitochondrial homeostasis and alters mitochondrial bioenergetics (Gorojod et al., 2018), followed by the release of ROS, leading to a necrotic or apoptotic cellular death (Chen et al., 2016). Moreover, Mn promotes an imbalance in fission-fusion dynamics by dysregulating its associated proteins such as dynamin-related protein 1 (Drp-1), optic atrophy type 1(Opa-1), and mitofusin 2 (Mfn2) (Alaimo et al., 2014), leading to mitochondrial fragmentation and dysfunction (Morcillo et al., 2021). This Mn-induced mitochondrial impairment is also known to cause neuroinflammation (Sarkar et al., 2018).
Timely removal of damaged and dysfunctional mitochondria by mitophagy, a selective autophagy to eliminate damaged mitochondria for its quality control, protects against Mn-induced neurotoxicity, but the protective role of mitophagy is impaired upon prolonged exposure to Mn (Roberts et al., 2016; Shefa et al., 2019). Studies have shown that Mn induced S-nitrosylation of PINK1, resulting in the repression of PINK1/parkin-mediated mitophagy function, ultimately leading to cell damage (Liu et al., 2022a). Therefore, depending on the experimental conditions and settings, mitophagy function can be activated upon Mn exposure to protect cells by eliminating damaged mitochondrial proteins, while prolonged overexposure to Mn can impair PINK1/parkin-mediated mitophagy function, leading to cellular injury (Zhang et al., 2016; Huang et al., 2021).
2.3 Autophagy
Autophagy is responsible for the degradation of damaged organelles and macromolecules to maintain cellular homeostasis in response to cellular insults and stress (Ghavami et al., 2014). Following Mn exposure, this process is triggered initially to protect cells from cellular damage (Zhou et al., 2018; Porte Alcon et al., 2020). However, after prolonged exposure to Mn, autophagy function is impaired, and subsequent cytotoxicity occurs (Zhang et al., 2013), suggesting that pathological levels of Mn exposure dysregulated autophagy function. Studies have shown that Mn increased autophagy activation in the rat striatum within 4–12 h after Mn exposure, but Mn suppressed autophagy at later stages, 1–28 days post-treatment of Mn (Zhang et al., 2013). Similarly, Mn activated autophagy up to 12 h of its exposure in human SH-SY5Y cells, but Mn exposure over 24 h impaired autophagy (Ma et al., 2017). Mn-induced dysregulation of autophagy impaired other cellular organelles and activated the NLRP3-CASP1 inflammasome pathway in the hippocampus of mice and BV-2 cells, leading to hippocampal-dependent impairment in learning and memory (Wang et al., 2017). Mn injection into the striatum showed autophagy dysregulation in rats, characterized by an increased number of mitochondrial vacuoles, swollen and fragmented endoplasmic reticulum, and dysfunctional lysosomes (Bahr et al., 2012; Bryan et al., 2019; Zhang et al., 2020).
2.4 Inflammation
Astrocytes and microglia critically contribute to maintaining a homeostatic balance in the CNS by regulating immune responses with production of cytokines and inflammatory mediators. Studies have shown that Mn dysregulated mitochondrial bioenergetics in astrocytes, resulting in the release of proinflammatory cytokines and adjacent neuronal injury (Sarkar et al., 2018). Mn activated microglia, generating proinflammatory cytokines, leading to dopaminergic neuronal death in the rat brain (Zhao et al., 2009). Mn induced neuroinflammation by increased production and releases of proinflammatory factors such as TNF-α, interleukin (IL)-1β, and nitric oxide synthase 2 (NOS2) in microglia, leading to neuronal injury in non-human primates (Verina et al., 2011). The nuclear factor kappa-light-chain-enhancer of activated B cells (NF-κB) signaling pathway has been shown to play an essential role in inflammatory responses to Mn toxicity in microglia during Mn-induced release of microglial cytokines to further amplify inflammatory activation of astrocytes (Kirkley et al., 2017).
2.5 Glutamate excitotoxicity
Glutamate is the major excitatory neurotransmitter in the brain and involved in essential brain functions such as cognition, learning, and memory (Danbolt, 2001). However, excessive extracellular glutamate levels and, thus, overstimulating glutamate receptors induce excitotoxic neuronal injury. Studies have shown that Mn impaired the astrocytic glutamate transporter function and glutamate uptake, leading to disruption of glutamate homeostasis and excitotoxic neuronal injury (Erikson et al., 2006; Deng et al., 2012) as the astrocytic glutamate transporters, EAAT1 (GLAST in rodents) and EAAT2 (GLT-1 in rodents), are critical for the removal of synaptic glutamate to prevent excitotoxic neuronal injury. Numerous studies have shown that Mn decreased astrocytic glutamate uptake (Hazell and Norenberg, 1997; Normandin and Hazell, 2002; Mutkus et al., 2005) by downregulation of EAAT2 (GLT-1) and EAAT1 (GLAST) in non-human primate and astrocyte cultures (Erikson and Aschner, 2002; Erikson et al., 2002; Lee et al., 2009a). The mechanism involved in Mn-induced reduction in GLAST (EAAT1) and GLT-1 (EAAT2) was at the transcriptional level by increasing YY1 expression in the mouse brain as well as in astrocytes (Lee et al., 2012; Karki et al., 2014a; Johnson et al., 2018a; Johnson et al., 2018b; Pajarillo et al., 2018). Moreover, Mn increased the sensitivity of postsynaptic glutamate receptors to glutamate, ultimately leading to irreversible cell damage (Spadoni et al., 2000). Mn also decreased the expression of N-methyl-D-aspartate (NMDA) receptors in rats, which also contributed to Mn’s neurotoxicity in addition to the increased concentrations of extracellular glutamate (Xu et al., 2010a).
2.6 Apoptosis
Mn causes apoptosis by modulating several cellular events. Studies have shown that Mn activated apoptotic signal caspase-3 as a consequence of Mn-induced endoplasmic reticulum stress (Chun et al., 2001). Mn also activated caspase-3 and p53 signaling by increasing neuronal expression of K-homology splicing regulator protein (KHSRP) in rat striatum (Shi et al., 2015). Mn induced apoptosis by activating protein kinase R in PC12 cells (Yagyu et al., 2020) and decreasing expression of wild-type p53-induced phosphatase 1, which modulates p53 signaling in striatal neurons (Ma et al., 2015). Moreover, Mn-induced apoptosis is not solely dependent on mitochondrial impairment as it did not correlate with mitochondrial depolarization and the subsequent release of cytochrome C (Oubrahim et al., 2001).
2.7 Epigenetic modification
Epigenetic modifications, such as DNA methylation, histone modification, and microRNAs (miRNA), play a role in Mn-induced neurotoxicity as Mn-impaired epigenetic modifications dysregulated global gene expression, resulting in aberrant cellular responses and activities (for review, see Tarale et al., 2016). Studies have shown that Mn altered DNA methylation in mice and SH-SY5Y cells (Yang et al., 2016), modulating DNA methylation of 226 genes associated with disrupted mitochondrial integrity and cell cycle, and DNA damage response (Yang et al., 2016). Mn also increased DNA methylation of p53 gene, resulting in reduction of p53 transcription and increase of downstream expression of proinflammatory meditator COX-2 in microglia, while this Mn effect was abolished by a demethylation reagent 5-Aza-dC (Liu et al., 2022b).
Epigenetic modification via histones acetylation or deacetylation also contributes to Mn-induced neurotoxicity by modulation of histone acetyltransferase (HAT) and histone deacetylase (HDAC). HATs such as CREB-binding protein (CBP) or p300 generally promote transcription by enhancing histone acetylation, whereas HDACs such as HDAC1-8 and sirtuins reduce transcription by inhibiting access of transcription factors to DNA (Xia et al., 2020). It has been shown that Mn increased HDAC activity and decreased HAT, resulting in cellular injury in PC12 and SH-SY5Y cells (Guo et al., 2018). Mn-reduced CBP/p300, and its-enhanced HDAC activity contributed to Mn-reduced transcription of tyrosine hydroxylase (TH) in dopaminergic neurons (Pajarillo et al., 2020a). Mn activated several HDACs, such as HDAC1-5 in astrocytes (Karki et al., 2014a; Karki et al., 2015b; Pajarillo et al., 2020a; Pajarillo et al., 2020b; Rizor et al., 2022) as well as HDAC1 and sirtuins (class III HDAC) in neurons (Zhao et al., 2019; Sun et al., 2021). HDACs also contributed to Mn-induced decreases in expression of EAAT1 and 2 by enhancing their interactions with YY1, a transcriptional repressor of EAAT1/2 in astrocytes (Karki et al., 2015a), which was attenuated by treatment with HDAC inhibitors (HDACi) (Karki et al., 2014a). Indeed, HDACi have been shown to mitigate Mn’s toxic effects by normalizing HDAC activity, histone acetylation, and gene expression in the mouse brain and astrocytes (Karki et al., 2014a; Johnson et al., 2018a; Johnson et al., 2018b). Although the mechanism of Mn-induced dysregulation of histone acetylation warrants further investigation, abnormal histone modification is critically involved in Mn-induced neurotoxicity. Mn-induced oxidative stress contributes to Mn-induced dysregulation of histone modification as the antioxidant curcumin attenuated Mn-reduced histone acetylation at H3K18 and H3K27 and expression of antioxidant genes, such as SOD2 in rats (Yang et al., 2022).
Sirtuins are comprised of seven proteins (sirtuins 1–7), functioning as not only histone deacetylases but also signaling molecules such as ADP-ribosyl transferase (Flick and Luscher, 2012). Studies have shown that Mn decreased sirtuin 1 and 3 during Mn-induced neurotoxicity in PC12 cells, primary neurons, and mice (Zhao et al., 2019; Sun et al., 2021). Mn decreased sirtuin 1 with a concomitant increase in proinflammatory genes and NF-κB in microglia (Yan et al., 2021), while sirtuin 3 overexpression attenuated Mn-induced neurotoxicity in primary cortical neurons (Sun et al., 2021), suggesting that sirtuin 1 and 3 play a role in Mn-induced inflammation and neurotoxicity.
Mn could also regulate gene expression by altering miRNAs which are small single-stranded non-coding RNAs, dysregulating RNA expression and post-transcriptional expression. Mn altered the levels of 73 miRNAs in SH-SY5Y cells (He et al., 2017), including miR-4306, miR-7, and miR-433, which targets ATP13A2 (PARK9), α-Syn (SNCA), and fibroblast growth factor-20 (FGF-20), respectively, while inhibition of these miRNAs attenuated the Mn’s effects on those target genes in SH-SY5Y cells (He et al., 2017; Tarale et al., 2018). These indicate that miRNA regulation could be important targets for developing protection strategies against Mn’s toxic effects.
3 Potential molecular targets for neurotherapeutics to mitigate Mn’s neurotoxicity
Dysregulations of Mn-induced cellular processes are associated with altering Mn’s target genes at the molecular and transcriptional levels. In this section, therefore, we will update findings of Mn-modulated genes, such as TFs and intracellular proteins, which could contribute to the development of strategies for therapeutics against Mn-induced neurotoxicity. These potential targets have shown promising attributes that can mitigate the serious harmful effects and neurological deficits caused by Mn toxicity.
3.1 TFs
Given the critical role of cellular insults such as mitochondrial dysfunction, oxidative stress, inflammation, and excitotoxicity in Mn-induced neurotoxicity, Mn’s target genes that are dysregulated and involved in those impaired cellular processes have been identified. Mn has been shown to modulate several TFs such as REST, YY1, Nrf2, TFEB, hypoxia-inducible factor 1α (HIF1α), NF-κB, and peroxisome proliferator-activated receptor gamma (PPAR-γ) in both in vitro and in vivo settings. The following proceedings discuss each of Mn’s target genes for their mechanistic roles in Mn-induced neurotoxicity and the potential for targeting specific molecules to treat Mn-induced neurotoxicity.
3.1.1 REST
REST is a zinc-finger TF, playing a critical role in neurogenesis, differentiation, growth, stress response, and survival by binding to the DNA sequence motif known as repressor element 1 (RE1, aka NRSE) (Chong et al., 1995; Schoenherr and Anderson, 1995). REST is increased in normal aging brains to maintain neuronal function by mitigating oxidative stress and apoptosis in aged human brains (Lu et al., 2014; Pajarillo et al., 2020a). Accordingly, REST was dysregulated in neurodegenerative diseases such as AD and PD (Lu et al., 2014; Pajarillo et al., 2020a). REST is expressed in various neural cell types, such as neurons, astrocytes, and microglia, implicating its diverse roles via different neural cells in the brain (Pajarillo et al., 2021; Pajarillo et al., 2022a). Mn decreased REST expression in dopaminergic neurons and astrocytes (Pajarillo et al., 2021; Pajarillo et al., 2020a), suggesting that impairing REST signals in neural cells might be involved in Mn toxicity mechanisms. Overexpression of REST inhibited Mn-increased pro-inflammatory cytokines such as TNF-α and proapoptotic proteins such as Bax, as well as attenuated Mn-decreased antiapoptotic Bcl-2 and antioxidant Nrf2, heme oxygenase 1 (HO-1), and catalase (CAT) in dopaminergic neurons (Pajarillo et al., 2020a). REST also attenuated the Mn-induced reduction of TH, a rate-limiting enzyme for dopamine synthesis in dopaminergic neurons (Pajarillo et al., 2020a).
Moreover, Mn decreased REST expression in astrocytes, while overexpression of astrocytic REST exerted protection against Mn-induced neuronal cell toxicity by regulating inflammatory cytokines and glutamate transporter GLT-1/EAAT2 in astrocytes (Pajarillo et al., 2021). Mn also reduced REST with a concomitant decrease in GLT-1 in the mouse striatum (Pajarillo et al., 2022a). REST has been shown to upregulate EAAT2 at the transcriptional levels by its binding to the RE1 sites of the EAAT2 promoter in astrocytes (Pajarillo et al., 2021). Conversely, downregulation of REST further decreased Mn-reduced EAAT2 in astrocytes and excitotoxic damage to dopaminergic neuronal cells in astrocyte-neuron co-culture (Pajarillo et al., 2021). Deletion of astrocytic REST in the mouse striatum exacerbated Mn-reduced TH protein levels and behavioral deficits (Pajarillo et al., 2022a). REST’s protective mechanisms against Mn-induced neurotoxicity were also associated with repressing inflammatory cytokines (McGann et al., 2021), at least in part, by its direct binding to their promoters and regulating gene expression (McGann et al., 2021; Pajarillo et al., 2022a).
3.1.2 YY1
YY1 is a TF, playing a dual role in transcription of its target genes, regulating both transcriptional activation and repression in a context- or cofactor-dependent manner (Gordon et al., 2006). YY1 in both neuronal and non-neuronal cells is critical for normal brain development and function (for review, see Rylski et al., 2008). Astrocytic YY1 regulates oxidative stress, inflammation, and apoptosis in the mouse brain (Pajarillo et al., 2022b), and Mn increases YY1 via NF-κB signaling and promotes YY1 binding to the GLT-1/GLAST promoters, resulting in repression of GLT-1/GLAST expression and function in astrocytes (Karki et al., 2014a; Pajarillo et al., 2020b). This Mn effect on YY1 could be closely associated with Mn-induced excitotoxic neuronal injury as reduction of GLT-1/GLAST would accumulate glutamate in the synaptic clefts and overstimulate its receptors. Deletion of astrocytic YY1 in substantia nigra of the mouse brain attenuated the Mn-induced decrease in GLAST and GLT-1 protein levels as well as TH levels in the nigrostriatal pathway, resulting in ameliorating Mn-induced motor deficits (Pajarillo et al., 2020b). These findings suggest that YY1 could be a potential molecular target in mitigating Mn-induced neurotoxicity.
3.1.3 Nrf2
Nrf2 is a transcriptional regulator of genes involved in antioxidant enzymes, including HO-1, glutathione peroxidase (GPx) 2, glutathione S-transferases, and thioredoxin by binding to its consensus site, antioxidant response element (ARE), of target genes to mitigate oxidative toxic insults (Tonelli et al., 2018). Mn dysregulated Nrf2-superoxide dismutase (SOD), along with inducing oxidative stress by increasing levels of isoprostanes and neuroprostanes in the rat brain (Santos et al., 2012). Mn decreased HO-1 expression by dysregulating Nrf2 activity in PC12 cells (Li et al., 2011a), while enhancing the Nrf2-HO-1 pathway protected neurons and astrocytes against Mn-induced toxicity (Gorojod et al., 2018; Pajarillo et al., 2020a). HO-1 overexpression also attenuated Mn-induced mitochondrial damage, oxidative stress, and cytotoxicity in C6 cells (Gorojod et al., 2018). Mn downregulated Nrf2 by modulating its transcription and degradation mechanisms via the ubiquitination-proteasome pathway (Li et al., 2011b; Pajarillo et al., 2020a). Mn-induced Nrf2 modulation was region-as well as sex-specific in rat brain (Ijomone et al., 2022). Histone deacetylation was also involved in Mn’s downregulation of the Nrf2 pathway in PC12 cells (Zhang et al., 2017), suggesting that Mn dysregulates Nrf2 via various intracellular targets.
3.1.4 TFEB
TFEB regulates genes involved in autophagy, lysosomal degradation, lipid catabolism, energy metabolism, and immune response (Settembre et al., 2011). Studies have shown that Mn impaired autophagy-lysosomal degradation and mitochondrial function by hampering TFEB translocation into the nucleus in astrocytes (Zhang et al., 2020). The mechanism of these Mn effects on TFEB is partly by activation of the MAPK1/ERK2 and MAPK3/ERK1 pathways to phosphorylate TFEB, resulting in its sequestration in the cytosol as an inactive form (Settembre et al., 2012). TFEB overexpression attenuated Mn-reduced autophagy-related TFEB target genes such as Lamp1, and Wipi1 (Zhang et al., 2020), restoring Mn-dysregulated autophagy flux and mitochondrial function in astrocytes.
3.1.5 HIF-1 and HIF-2
HIF-1 and 2 are TFs, playing a significant role in proliferation, oxidative stress, inflammation, and apoptosis, by binding to its hypoxia response elements (HRE) on the promoter region of its target genes under hypoxic or toxic conditions (Chapman-Smith et al., 2004). Upregulation of HIF increased expression of Mn exporter SLC30A10 in the liver and induces protection against Mn’s neurotoxic effects in mice (Liu et al., 2021b). Moreover, Mn itself increased expression of HIF-1α/2α and SLC30A10, possibly to excrete excess Mn as a homeostatic mechanism. These findings suggest that HIF-SLC30A10 could be a relevant target to eliminate excess Mn for maintenance of Mn homeostasis in the body and prevent Mn’s neurotoxicity.
3.1.6 NF-κB
NF-κB, a master regulator of genes involved in cytokine production and cell survival, plays an important role in Mn-induced neurotoxicity (for review, see Pajarillo et al., 2021). Mn activates NF-κB to enhance expression of genes involved in the production of various proinflammatory cytokines and chemokines in astrocytes and microglia (Barhoumi et al., 2004; Filipov et al., 2005; Prabhakaran et al., 2008). NF-κB plays a role in cell-to-cell communication signaling for transferring inflammatory products between astrocytes and microglia during Mn toxicity (Kirkley et al., 2017; Nkpaa et al., 2019; Li et al., 2021). NF-κB was involved in Mn-induced production of cytokines IL-1β/IL-18 via enhancing NLRP3 inflammasome formation in BV-2 microglia (Peng et al., 2020). Mn also activated NF-κB to increase YY1 expression, which in turn, repressed GLT-1 (EAAT2) in astrocytes (Karki et al., 2014a). These findings indicate that the NF-κB pathway is involved in various Mn toxicity mechanisms, providing the potential to be a therapeutic target to treat Mn’s neurotoxicity.
3.1.7 PPARs
PPARs are TFs with three isotypes, PPAR-α, PPAR-β/δ, and PPAR-γ, playing a role in development, proliferation, differentiation, lipid and energy metabolism, and survival (Desvergne and Wahli, 1999; Michalik et al., 2004). Studies have shown that Mn dysregulated cellular localization and protein levels of PPARs in human U87 astrocytes and SK-N-SH neuronal cells (Isaac et al., 2006). PPAR-γ activation attenuated Mn-induced loss of cell viability, oxidative stress, and mitochondrial damage in U87 astrocytes (Gugnani et al., 2018).
3.2 Mn’s intracellular target proteins
Intracellular communications such as signaling pathways are dysregulated in Mn-induced toxicity. Several intracellular proteins such as PINK1, parkin, LRRK2, and α-Syn are modulated under Mn-induced neurotoxicity, suggesting that these proteins might be potential molecular targets to develop therapeutics against Mn-induced neurotoxicity.
3.2.1 PINK1
PINK1, is an important mediator of mitophagy in regulation of mitochondrial quality control and damaged mitochondrial protein degradation with collaboration of parkin, and its mutations are associated with familial forms of PD (Springer and Kahle, 2011). Studies have shown that α-Syn interfered with PINK1’s mitophagy function in Mn-exposed rat brain (Liu et al., 2021a), accompanied by reduction in ATP levels and mitochondrial membrane potential, but increased mitochondrial ROS levels in the striatum. However, knockdown of α-Syn enhanced PINK1 activity and mitophagy and attenuated Mn-induced oxidative stress, mitochondrial dysfunction, and cell death in SH-SY5Y cells (Liu et al., 2021a).
Mn increased S-nitrosylation of PINK1, resulting in impaired mitophagy and thus, accumulating damaged mitochondria and cell death in the rat striatum and primary neurons (Liu et al., 2022a). Mn also increased PINK1 DNA methylation in SH-SY5Y cells (Tarale et al., 2017), leading to a decrease in PINK1 mRNA expression (Tarale et al., 2017). These findings indicate that Mn dysregulates PINK1 by multiple mechanisms in Mn-induced impairment of mitophagy and consequential neuronal injury.
3.2.2 Parkin
Parkin, an E3 ubiquitin ligase encoded by the PARK2 gene, is associated with familial PD as its mutations lead to its loss-of-function in mitophagy (Seirafi et al., 2015). Mn decreased parkin mRNA and protein levels in the striatum and midbrain under Mn-induced dopaminergic neurotoxicity and motor deficits in rats (Cao et al., 2022), while overexpression of parkin attenuated Mn-induced cell death in SH-SY5Y cells (Higashi et al., 2004), indicating that parkin exerts protective effects against Mn’s neurotoxicity. Other studies have reported that Mn increased parkin expression in rats (Sriram et al., 2010) and phosphorylation of parkin in the striatum of rats (Liu et al., 2021a). This Mn’s effect on increasing parkin might attribute to a compensatory mechanism to cope with Mn’s toxicity in mitophagy by enhancing mitophagy in subtoxic conditions. While parkin is directly involved in Mn-impaired mitophagy, it could also play critical functions in Mn accumulation during Mn-induced dopaminergic neurotoxicity. Dysfunction or mutation of parkin resulted in Mn accumulation (Bornhorst et al., 2014), possibly by downregulating Mn transporter ferroportin in C. elegans (Chakraborty et al., 2015). Overexpression of parkin prevented Mn accumulation by reduction of divalent metal transporter 1 (DMT1, responsible for Mn uptake) via ubiquitin-proteasomal degradation in SH-SY5Y cells (Roth et al., 2010), and deletion of parkin did not alter tissue Mn levels compared to WT mice after Mn exposure (Foster et al., 2018). These mixed results may be due to different experimental models and settings, requiring further studies to understand parkin’s role in Mn transport and accumulation.
3.2.3 LRRK2
LRRK2 is a large multi-domain protein, composed of a leucine-rich domain (LRR), a Roc GTPase domain, a carboxy-terminal of Ras (COR) domain, a kinase domain and a WD40 domain at the carboxy-terminal (Dzamko, 2017). Several mutations in LRRK2 are associated with sporadic PD (accounting for 1-2% of all sporadic PD cases) and a familial PD (up to 13% of all familial PD cases) (Healy et al., 2008). Among all identified pathological LRRK2 mutations, G2019S is the most prevalent mutation in both familial and sporadic PD cases (Healy et al., 2008). Most pathogenic LRRK2 mutations, such as G2019S and R1441 C/G/H, enhances LRRK2 kinase activity by two-to three-fold (Dzamko, 2017). Mn increased both LRRK2 expression and its kinase function, which played a critical role in Mn-induced autophagy, oxidative stress, and inflammation (Chen et al., 2018; Kim et al., 2019). LRRK2 inhibition attenuated Mn-induced autophagy-related proteins Beclin 1 and Atg5, along with inhibition of Mn-induced apoptosis and TNF-α production in microglia (Chen et al., 2018; Kim et al., 2019).
3.2.4 α-Syn
Mn increased α-Syn expression, aggregation, and subsequent cytotoxicity in various experimental models (Verina et al., 2013; Xu et al., 2013; Bhandari et al., 2018), while knockdown of α-Syn attenuated Mn-induced neurotoxicity (Cai et al., 2010; Li et al., 2010), indicating that α-Syn plays a critical role in Mn-induced cytotoxicity. Mn also modulated the secretion and extracellular vesicular trafficking of α-Syn between cells by activating RAB27A for exosomal packaging of α-Syn (Pfeffer, 2010), resulting in the exosomal release into the extracellular environment (Harischandra et al., 2018). Welders chronically exposed to Mn increased misfolded α-Syn in their exosomes from their serum samples, which have been shown to induce neuroinflammation and subsequent neurodegeneration (Harischandra et al., 2019b).
3.2.5 PI3K/Akt
PI3K is a group of signaling proteins that perform diverse cellular functions such as cell growth, proliferation, differentiation, survival, autophagy, and intracellular trafficking. PI3K activates other intracellular kinases, including Akt and the mammalian target of rapamycin (mTOR), to modulate various cellular pathways. Mn activated PI3K to induce inducible NOS (iNOS) expression in BV2 microglia (Bae et al., 2006). Mn also activated the PI3K/Akt pathway in Mn-induced apoptosis, such as caspase-3 and Bax levels in rat hippocampus (Cheng et al., 2018), indicating PI3K/Akt signaling is involved in Mn-induced apoptosis. In contrast, enhancing PI3K activity induced protection mechanisms against Mn toxicity as inhibition of PI3K/Akt increased Mn’s cytotoxicity and reduced antioxidant enzymes in PC12 cells (Tan et al., 2022). In fact, many studies support the role of PI3K/Akt signaling in protective mechanisms against Mn-induced toxicity (Ji et al., 2020). 17β-estradiol (E2) and tamoxifen (TX) attenuated Mn-induced oxidative stress and EAAT2 dysregulation via the PI3K/Akt signaling pathway in astrocytes (Lee et al., 2009a). While Mn activates the PI3K/Akt pathway, further investigation is required to better understand its distinct roles in Mn-induced neurotoxicity and neuroprotection.
3.2.6 MAPK
MAPK are serine and threonine protein kinases that are essential in regulating brain development, gene transcription, protein synthesis, proliferation, differentiation, metabolism, apoptosis, and cell-cell interaction (Cargnello and Roux, 2011). Mn has been shown to activate MAPK/ERK and p38 signaling pathways in inducing oxidative stress and inflammation in astrocytes and microglia (Crittenden and Filipov, 2008; Moreno et al., 2008). MAPK/ERK signaling may be involved in modulating the transcription of genes such as iNOS to attenuate Mn-increased oxidative stress and inflammation in BV2 microglia (Bae et al., 2006). Mn also induced astrocyte swelling and damage via MAPK signaling, as well as protein levels of aquaporins in primary astrocytes (Rao et al., 2010). The Mn-induced activation of the MAPK pathway exacerbated AD pathogenesis as Mn induced hyperphosphorylation of tau by activating the MAPK/ERK pathway in PC12 cells (Cai et al., 2011). MAPK/ERK signaling also appears to be activated by both Mn-induced toxicity mechanisms and protection induced by various agents against Mn toxicity, warranting further studies.
4 Neurotherapeutics and their potential underlying mechanisms against Mn’s neurotoxicity
Several pharmacological agents have been shown to exert protective effects against Mn-induced neurotoxicity by attenuating Mn-induced mitochondrial impairment, oxidative stress, inflammation, glutamate transporter dysregulation, and apoptosis in various in vitro and in vivo models. Despite the significant efficacy, their precise molecular mechanisms associated with mitigating Mn-induced neurotoxic effects remain to be elucidated. Various pharmacological agents’ potential molecular targets and their mode of action in attenuating Mn-induced neurotoxicity are summarized (Tables 1, 2).
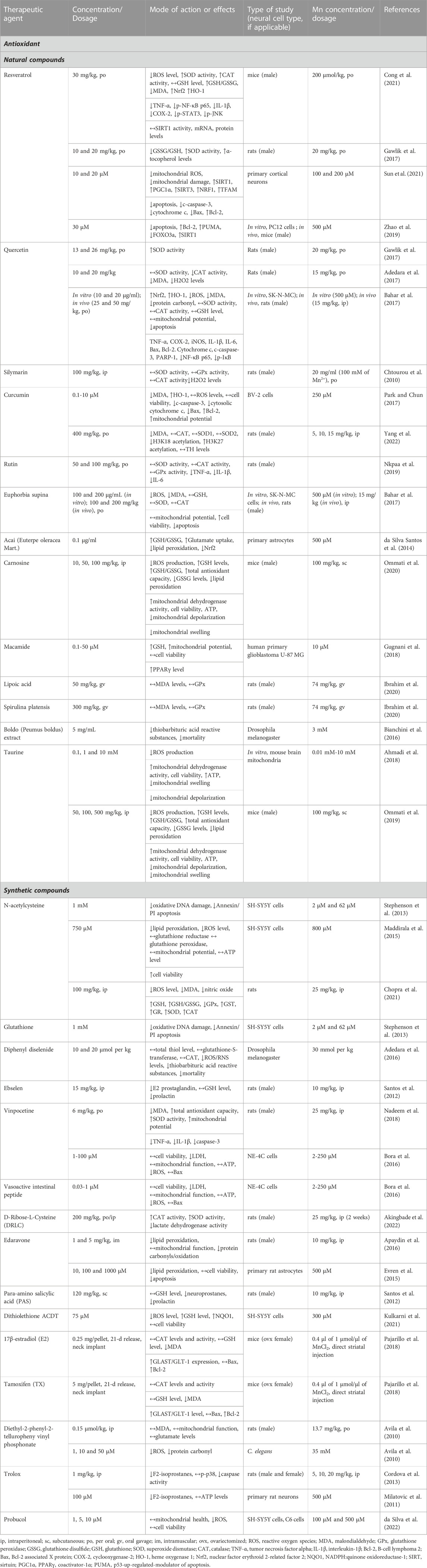
TABLE 1. Neurotherapeutic agents and their protective effects against Mn-induced oxidative stress in in vitro and in vivo experimental settings.
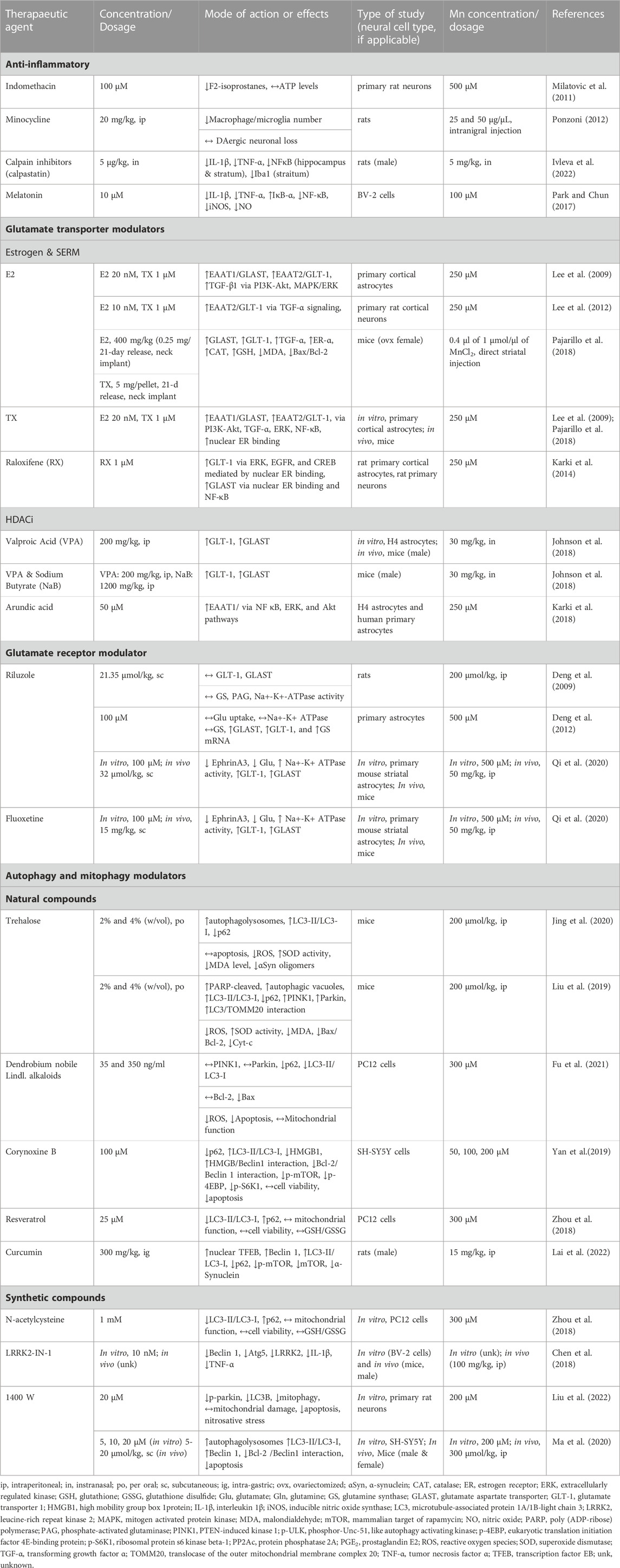
TABLE 2. Neurotherapeutic agents and their protective effects against Mn-induced inflammation, excitotoxicity and dysregulation of autophagy and mitophagy in in vitro and in vivo experimental settings.
4.1 Chelating agents
Given that chelating agents can remove heavy metals from the body (Andersen and Aaseth, 2016), PAS and EDTA have been shown to excrete Mn from the body and prevent acute Mn toxicity (Jiang et al., 2006; Zheng et al., 2009). PAS significantly decreased Mn levels in the body by 25–33% (Zheng et al., 2009). EDTA has also been extensively studied for the chelation therapy of toxic metals, including Mn, in their neurotoxicity (Discalzi et al., 2000). However, further studies are required to establish a safe treatment strategy as their therapeutic efficacy in decreasing morbidity and mortality is largely unestablished (Kosnett, 2010).
4.2 Antioxidants
As Mn-induced oxidative stress is one of the most studied mechanisms, numerous antioxidative therapeutics, including natural and synthetic, have been explored to mitigate Mn-induced oxidative stress. Many of those agents also exerted anti-inflammatory and antiapoptotic properties indirectly possibly due to secondary or downstream effects of their antioxidative functions to Mn-induced oxidative stress. Several antioxidants have been summarized, including polyphenols, natural peptides, and fatty acid derivatives against Mn-induced neurotoxicity (Table 1).
4.2.1 Natural compounds
Studies have shown that resveratrol, a type of natural phenol, effectively attenuated Mn-induced toxicity by enhancing SOD, and CAT activities, resulting in reduction of Mn-elevated ROS levels and lipid peroxidation in mice and rats (Gawlik et al., 2017; Cong et al., 2021) as well as primary cortical neurons and PC12 cells (Sun et al., 2021). The molecular mechanisms involved in resveratrol’s protective effects against Mn-induced toxicity may involve multiple targets, including Nrf2-HO-1 and sirtuin 1 and 3 (Zhao et al., 2019; Sun et al., 2021). Polyphenol flavonoids are abundant in food sources, and several flavonoid polyphenols, including quercetin, silymarin, and curcumin, have shown to exhibit antioxidative properties against Mn-induced toxicity. Quercetin which has been shown to exert neuroprotective effects against various neurodegenerative disease models (for review (Islam et al., 2021), induced protective effects against Mn toxicity by attenuating Mn-induced impairment of SOD and CAT activities in the rat brain (Adedara et al., 2017; Gawlik et al., 2017). Quercetin also activated antioxidative Nrf2-HO-1 signaling to mitigate Mn-induced production of ROS, malondialdehyde (MDA), and protein carbonyls in SK-N-MC cells and rats (Bahar et al., 2017b). Silymarin from milk thistle (Silybum marianum) exerted antioxidative and neuroprotective effects against Mn toxicity by attenuating Mn-impaired SOD, GPx, and CAT activity, resulting in reduction of oxidative stress in the rat brain (Chtourou et al., 2010). Curcumin from turmeric exerted antioxidative effects by reducing Mn-increased MDA and ROS levels, while promoting HO-1 activity in BV-2 microglia (Park and Chun, 2017a). Curcumin also enhanced antioxidant effects by increasing activities of CAT, SOD1, and SOD2, and reversed Mn-induced epigenetic dysregulation by modulating H3K18 acetylation and H3K27 acetylation along with attenuation of Mn-induced oxidative stress and dopaminergic toxicity in the rat brain (Yang et al., 2022). Rutin, a polyphenolic flavonoid glycoside, mitigated Mn-induced toxicity in rats by attenuating Mn-dysregulated antioxidant SOD, CAT, and GPx activities (Nkpaa et al., 2019). Polyphenol flavonoids from Euphorbia supina weed have shown strong antioxidant properties and protective effects against Mn-induced neurotoxicity by attenuating Mn-induced SOD and CAT dysregulation in SK-N-MC cells and the rat brain (Bahar et al., 2017a). Anthocyanin from acai berries has been shown to exert antioxidative effects by attenuating Mn-dysregulated GSH/GSSG (glutathione disulfide) and lipid peroxidation partly by restoring Nrf2 levels and activity in rat primary astrocytes (da Silva Santos et al., 2014).
Several peptides and lipid compounds also showed antioxidant capabilities against Mn-induced toxicity in both in vitro and in vivo models. Carnosine (β-alanyl-l-histidine), a natural dipeptide commonly found in meat, exhibited its protective properties as a molecular chaperone and inducer of antioxidant systems and reduced Mn-induced lipid peroxidation in Mn-exposed mice (Ommati et al., 2020). Taurine found in animal tissues has shown to reduce Mn-induced oxidative stress and mitochondrial damage by reducing lipid peroxidation and enhancing GSH and total antioxidant capacity in mice (Ahmadi et al., 2018; Ommati et al., 2019). Macamides, long-chain fatty acid N-benzylamides from Lepidium meyenii or Maca, showed antioxidant properties (Almukadi et al., 2013) and exerted protection against Mn-induced neurotoxicity by increasing GSH levels, mitochondrial membrane potential, and PPAR-γ levels in U97 MG cells (Gugnani et al., 2018). Lipoic acid, which is synthesized in animals normally, acting as a cofactor for enzymes such as pyruvate dehydrogenase for the citric acid cycle, exerted protective effects against Mn toxicity by attenuating Mn-induced lipid peroxidation and dysregulated GPx levels in rats (Ibrahim et al., 2020). Boldo (Peumus boldus) leaf extracts which contain alkaloids, such as boldine, isoboldine, and N-methyllaurotetanine, displayed antioxidant activities, reducing lipid peroxidation and mortality in a Drosophila model of Mn-induced toxicity (Bianchini et al., 2016).
4.2.2 Synthetic compounds
N-acetylcysteine (NAC), a precursor of GSH, effectively reduced Mn-induced oxidative damage and apoptosis in SH-SY5Y cells (Stephenson et al., 2013). NAC also attenuated Mn-dysregulated GR and GPx levels in SH-SY5Y cells (Maddirala et al., 2015) and increased SOD and CAT activities in the rat brain (Chopra et al., 2021).
Diphenyl diselenide, an organoselenium compound, increased antioxidant GPx and GSH activities (Orian and Toppo, 2014) and attenuated Mn-induced oxidative stress in Drosophila (Adedara et al., 2016). Another organoselenium, Ebselen, also attenuated Mn-induced dysregulation of antioxidant GSH in rats (Santos et al., 2012).
Vinpocetine, a synthetic derivative of the vinca alkaloid vincamine, attenuated Mn-induced oxidative stress and apoptosis in rats (Nadeem et al., 2018) and NE-4C cells (Bora et al., 2016). D-ribose-L-cysteine, a cysteine analog exhibiting antioxidant properties, attenuated Mn-induced oxidative stress and toxicity by enhancing CAT and SOD activity in rats (Akingbade et al., 2022).
Edaravone (Radicava), an FDA-approved drug for treating stroke and amyotrophic lateral sclerosis (ALS) (Bhandari et al., 2018), has shown its therapeutic efficacy against Mn-induced toxicity by reducing Mn-induced lipid peroxidation, protein degradation, and mitochondrial dysfunction in the rat brain as well as primary astrocytes (Evren et al., 2015; Apaydin et al., 2016).
In addition to its chelating properties on Mn, PAS exerted protective effects against Mn-induced neurodegeneration by enhancing antioxidant, anti-inflammatory, anti-excitotoxicity, and antiapoptotic effects in various in vitro and in vivo experimental models (Zheng et al., 2009; Ou et al., 2011; Santos et al., 2012; Li et al., 2017; Peng et al., 2020; Deng et al., 2021). Organotellurium compounds have shown antioxidant activities in the brain. For example, organotellurium diethyl-2-phenyl-2-telluropheny vinyl phosphonate (DPTVP) exerted neuroprotection against Mn-induced neurotoxicity and behavioral deficits in rats, attributing to its antioxidant activity (Avila et al., 2010) and attenuated Mn-induced reduction in survival in C. elegans (Avila et al., 2012). Dithiolethiones, lipophilic organosulfur compounds that activate Nrf2, upregulated various phase II antioxidant enzymes, and its disubstituted dithiolethione (ACDT) exerted protection against Mn toxicity by increasing GSH, NAD(P)H dehydrogenase in SH-SY5Y cells (Kulkarni et al., 2021).
Trolox, a water-soluble vitamin E analog, attenuated Mn-induced ROS, F2-isoprostanes, and caspase activity in the rat striatum and rat primary neurons (Milatovic et al., 2011; Cordova et al., 2013). Probucol, an anti-hyperlipidemic drug, showed powerful antioxidative effects, attenuating Mn-induced ROS production, mitochondrial dysfunction, and cell death in SH-SY5Y neuronal and C6 glial cells (da Silva et al., 2022). Minocycline, a tetracycline antibiotic, exerted antioxidative effects against Mn-induced oxidative stress by decreasing lipid peroxidation and increasing antioxidant enzymes such as SOD in Drosophila (Bonilla et al., 2012; Mora et al., 2014). Melatonin, an endogenous hormone produced by the pineal gland, has been shown to attenuate Mn-induced motor dysfunction and neuronal loss in mice by inhibiting Mn-induced oxidative stress (Deng et al., 2015). Other synthetic antioxidants have also shown efficacy in treating Mn-induced neurotoxicity in various experimental models, but it has yet to be established in clinical trials whether they are efficacious in humans as well.
4.3 Anti-inflammatory compounds
Accumulating evidence reveals that Mn toxicity promotes neuroinflammation by increasing proinflammatory cytokines such as TNF-α, ILs, and inflammatory mediator COX-2. The non-steroidal anti-inflammatory drug (NSAID) indomethacin has been shown to alleviate Mn-induced neuroinflammation, oxidative stress, and energy dysregulation in rat primary cortical neurons and mice (Milatovic et al., 2011). The antibiotic, minocycline, inhibited Mn-induced macrophage-mediated inflammation with concomitant attenuation of Mn-reduced striatal TH of the rat brain (Ponzoni, 2012).
The calpain inhibitor, calpastatin, has been shown to attenuate Mn-induced toxicity by reducing proinflammatory markers, including IL-1β, TNF-α, NF-κB in the rat hippocampus and striatum, preventing Mn-induced motor deficit and microglial Iba1 expression and dopamine dysregulation in rat striatum (Ivleva et al., 2022).
Melatonin, which has been shown to afford neuroprotection via its anti-inflammatory and antioxidant properties in PD and AD models (Asefy et al., 2021) also attenuated Mn-induced increase in proinflammatory cytokines, such as TNF-α and IL-1β and IκB-α in BV-2 cells (Park and Chun, 2017b). Anti-inflammatory agents showing efficacy against Mn-induced neurotoxicity are listed in Table 2.
4.4 Glutamate excitotoxicity modulators
Mn can lead to accumulation of glutamate in the synapse and excitotoxicity (Lee et al., 2009b) by impairing astrocytic glutamate transporters and overstimulating the NMDA receptor (NMDAR). Several compounds have been shown to increase expression of the glutamate transporters EAAT1 (GLAST) and EAAT2 (GLT-1) (Pajarillo et al., 2018), and some agents modulated NMDAR and α-amino-3-hydroxy-5-methyl-4-isoxazolepropionic acid (AMPA) receptors and attenuated Mn-induced neurotoxicity. The current findings on pharmacological agents that modulate glutamate transporters and receptors to protect against Mn toxicity were reviewed in the following sections and summarized in Table 2.
4.4.1 Estrogen and SERMs
Estrogens (mainly 17β-estradiol, E2) and selective estrogen receptor modulators (SERMs) have been shown to exert neuroprotective effects in various experimental models of neurodegenerative disorders and Mn’s neurotoxicity. E2 and tamoxifen (TX) attenuated Mn-reduced glutamate uptake and GLT-1/GLAST expression by upregulation of GLT-1 and GLAST at the transcriptional level in rat primary astrocytes (Lee et al., 2009a). E2 and TX increased GLT-1 by increasing transforming growth factor α in astrocytes (Lee et al., 2012). E2 and TX have also been shown to attenuate Mn-induced reduction of GLT-1/GLAST, along with locomotor activity and dopaminergic neuronal injury in mice (Pajarillo et al., 2018).
Another SERM, raloxifene (RX), attenuated Mn-reduced GLT-1 expression and glutamate uptake by upregulating GLT-1 protein expression in rat primary astrocytes (Karki et al., 2014b). Several signaling pathways, such as MAPK/ERK, PI3K/Akt and EGFR, and TFs, such as CREB and NF κB were involved in E2/TX/RX-increased GLT-1 expression. Moreover, the estrogen receptors, ER-α, ER-β, and GPR30, mediated GLT-1 transcription via both genomic and non-genomic mechanisms (Karki et al., 2014b). These findings suggest that various pathways are involved in E2-and SERMs-induced GLT-1/GLAST expression and neuroprotection against Mn’s excitotoxic neuronal injury (Pajarillo et al., 2019).
4.4.2 Epigenetic modulators
Given that HDAC suppresses gene transcription (Mai et al., 2009) and Mn activates HDACs, inhibiting HDACs could be potential therapeutic targets for treating Mn toxicity. Studies have shown that HDACi such as valproic acid (VPA) and sodium butyrate (NaB) increased GLAST/GLT-1 expression and attenuated Mn-reduced GLAST/GLT-1 in both in vitro and in vivo models (Johnson et al., 2018b). The antiepileptic VPA has been shown to exert neuroprotective effects in Mn toxicity by attenuating Mn-induced decrease in TH mRNA and protein levels as well as motor deficits in astrocyte cultures and mouse brain tissue (Johnson et al., 2018a). NaB also attenuated Mn-reduced GLT-1/GLAST in cortical and cerebellar regions of mice, along with motor function deficits. These findings suggest that HDACi could exert neuroprotection against Mn toxicity, at least in part by upregulation of astrocytic glutamate transporters GLT-1/GLAST. Moreover, modulating DNA methylation and miRNA could also be potential targets to treat Mn toxicity as a demethylation reagent 5-Aza-dC attenuated Mn-induced inflammation in microglia (Liu et al., 2022b), and inhibition of Mn-activated miRNAs attenuated Mn’s relevant target genes in SH-SY5Y cells (He et al., 2017; Tarale et al., 2018).
4.4.3 Arundic acid
Arundic acid [(R)-(-)-2-propyloctanoic acid (ONO-2506)] was first discovered as an inhibitor of S100β, a calcium-binding protein produced primarily in astrocytes, ameliorating ischemic brain damage in rats (Tateishi et al., 2002), as well as MPTP-induced PD mice by modulating astrocytic activation (Kato et al., 2004; Himeda et al., 2006). Arundic acid exerted protective effects against Mn toxicity by inhibiting Mn-induced downregulation of GLAST/EAAT1 and GLT-1/EAAT2 mRNA and proteins levels in human H4 astrocytes and rat primary astrocytes (Karki et al., 2018). This effect was mediated by the activation of the Akt and ERK pathways as well as the inhibition of Mn-activated YY1 that is a repressor of EAAT1/2 (Karki et al., 2018).
4.4.4 Fluoxetine and riluzole
Fluoxetine, which is clinically used to treat depression due to serotonin (5-HT) uptake inhibition, has increased GLT-1 expression in the rat brain (Chen et al., 2014). Riluzole, a voltage-dependent Na channel blocker and is used to treat ALS, has been shown to inhibit glutamate release from neuronal synapses (Irifune et al., 2007) and increase glutamate reuptake by astrocytes (Fumagalli et al., 2008). Both fluoxetine and riluzole attenuated the Mn-induced increase in ephrin A3 and decrease in GLT-1 and GLAST mRNA/protein levels in primary striatal murine astrocytes (Deng et al., 2009; Qi et al., 2020).
4.4.5 Diclozipine (MK-801) and dextromethorphan
MK-801 and dextromethorphan (DM) are non-competitive NMDAR antagonists. MK-801 inhibited the NMDAR to prevent Ca2+ overload and ROS production (Thomas and Kuhn, 2005), and DM protected the rat brain cortex against brain trauma injury by anti-excitatory and anti-inflammatory mechanisms (Pu et al., 2015). Importantly, both MK-801 and DM attenuated Mn-induced neurotoxicity by inhibiting the NMDAR overstimulation in rats (Xu et al., 2010b). MK-801 attenuated Mn-induced NMDA excitotoxic lesion, along with ATP reduction and decreased dopamine and GABA levels in the rat striatum, corroborating that Mn may produce neuronal degeneration by an indirect excitotoxic process secondary to its ability to impair oxidative energy metabolism (Brouillet et al., 1993).
4.5 Autophagy and mitophagy modulators
4.5.1 Natural compounds
Trehalose is a disaccharide comprised of two glucose molecules which is synthesized in bacteria, fungi, plants, and invertebrate animals. Trehalose has been shown to afford protective effects against Mn-induced neurotoxicity in the murine brain by increasing autophagy activation along with attenuation of Mn-induced oxidative stress and α-Syn oligomerization (Jing et al., 2020). Trehalose mitigated Mn-induced PINK1/parkin-dependent mitophagy dysregulation by attenuating Mn-increased cleaved-poly (ADP-ribose) polymerase (PARP) and mitochondria-containing autophagic lysosomes, resulting in inhibition of Mn-induced mitochondrial damage and apoptosis in mice (Liu et al., 2019).
Alkaloids from Dendrobium nobile have been shown to attenuate Mn-impaired autophagy and mitophagy proteins, including LC3-II, p62, PINK1, and parkin, and inhibition of Mn-induced oxidative stress, apoptosis, and mitochondrial dysfunction in PC12 cells (Fu et al., 2021). Corynoxine B, a natural oxindole alkaloid from Mitragyna speciose and Uncaria macrophylla, exerting protective effects in experimental models of AD and PD (Zeng et al., 2019; Chen et al., 2021; Zhu et al., 2022), attenuated Mn-impaired mTOR signaling and autophagy in SH-SY5Y cells (Yan et al., 2019). Studies have also shown that resveratrol and curcumin led to protection against Mn-induced neurotoxicity by promoting autophagy in both PC12 cells and rats (Zhou et al., 2018; Lai et al., 2022).
4.5.2 Synthetic compounds
Elevated LRRK2 kinase activity is closely related to inflammatory signaling, and Mn activated LRRK2, leading to an increase in autophagy proteins such as Beclin 1 and TNF-α levels in microglia (Chen et al., 2018; Kim et al., 2019). LRRK2-IN-1, an inhibitor of LRRK2, attenuated Mn-induced autophagy by inhibiting Mn-increased Beclin 1 and Atg5 in BV-2 microglia and mice, indicating that LRRK2 is involved in Mn-induced autophagy dysregulation and inflammation (Chen et al., 2018).
1400 W is a selective inhibitor of iNOS, exerting anti-inflammatory and antioxidative properties against various neurodegenerative disease models. 1400 W alleviated Mn-induced autophagic impairment and neuronal injury by increasing autophagic vacuoles and protein levels of Beclin 1, LC3-II, while decreasing p62 levels along with inhibiting Mn-induced nitrosylation of JNK, IKKβ, and Bcl-2 in mice and SH-SY5Y cells (Ma et al., 2020). NAC mitigated Mn-induced autophagy dysfunction likely via its anti-oxidative properties (Zhou et al., 2018).
5 Conclusion
The underpinning of mechanisms of Mn-induced neurotoxicity provides critical information for developing neurotherapeutics and treatment strategies. Accordingly, delineating the transcriptional and intracellular pathways involved in the regulation of oxidative stress, autophagy, mitophagy, inflammation, and excitotoxicity is critical to further our understanding of Mn-induced neuropathogenesis. To date, no single agent has shown to be a promising therapeutic to treat Mn toxicity, warranting further investigation of the underlying mechanism and, thus, developing therapeutics using precise molecular targets to treat or prevent Mn’s neurotoxicity.
5.1 Future direction
Targeting specific molecules modulating various mechanisms induced by Mn toxicity, including autophagy, mitophagy, excitotoxicity, as well as Mn’s systemic removal are exciting avenues for exploration, expanding our comprehensive knowledge in developing newer, efficacious, specific gene and drug therapies for manganism and other related neurological disorders. Moreover, since Mn targets multiple genes and proteins, combination therapy strategies comprising of several relevant targets could be more beneficial for optimal treatment against Mn neurotoxicity.
Author contributions
Conceptualization: EP and EL. Funding acquisition: EL and MA. Project administration: EL. Resources: EL. Supervision: EP and EL. Validation: EP and EL. Visualization: EP. Writing—original draft: EP, IN-D, AD, HM, and SK. Writing—review and editing: EP, AD, IN-D, SK, HM, PG, MA, and EL.
Funding
This work was supported by National Institutes of Health Grants NIEHS R01 ES024756 (to EL), R01 ES031282 (to EL), R01 ES010563 (to MA), and NIMHD U54 MD007582.
Conflict of interest
The authors declare that the research was conducted in the absence of any commercial or financial relationships that could be construed as a potential conflict of interest.
Publisher’s note
All claims expressed in this article are solely those of the authors and do not necessarily represent those of their affiliated organizations, or those of the publisher, the editors and the reviewers. Any product that may be evaluated in this article, or claim that may be made by its manufacturer, is not guaranteed or endorsed by the publisher.
References
Adedara, I. A., Abolaji, A. O., Rocha, J. B. T., and Farombi, E. O. (2016). Diphenyl diselenide protects against mortality, locomotor deficits and oxidative stress in Drosophila melanogaster model of manganese-induced neurotoxicity. Neurochem. Res. 41 (6), 1430–1438. doi:10.1007/s11064-016-1852-x
Adedara, I. A., Ego, V. C., Subair, T. I., Oyediran, O., and Farombi, E. O. (2017). Quercetin improves neurobehavioral performance through restoration of brain antioxidant status and acetylcholinesterase activity in manganese-treated rats. Neurochem. Res. 42 (4), 1219–1229. doi:10.1007/s11064-016-2162-z
Ahmadi, N., Ghanbarinejad, V., Ommati, M. M., Jamshidzadeh, A., and Heidari, R. (2018). Taurine prevents mitochondrial membrane permeabilization and swelling upon interaction with manganese: Implication in the treatment of cirrhosis-associated central nervous system complications. J. Biochem. Mol. Toxicol. 32 (11), e22216. doi:10.1002/jbt.22216
Akingbade, G. T., Ijomone, O. M., Imam, A., Aschner, M., and Ajao, M. S. (2022). D-Ribose-LCysteine attenuates manganese-induced cognitive and motor deficit, oxidative damage, and reactive microglia activation. Environ. Toxicol. Pharmacol. 93, 103872. doi:10.1016/j.etap.2022.103872
Alaimo, A., Gorojod, R. M., Beauquis, J., Munoz, M. J., Saravia, F., and Kotler, M. L. (2014). Deregulation of mitochondria-shaping proteins Opa-1 and Drp-1 in manganese-induced apoptosis. PLoS One 9 (3), e91848. doi:10.1371/journal.pone.0091848
Ali, S. F., Duhart, H. M., Newport, G. D., Lipe, G. W., and Slikker, W. (1995). Manganese-induced reactive oxygen species: Comparison between Mn+2 and Mn+3. Neurodegeneration 4 (3), 329–334. doi:10.1016/1055-8330(95)90023-3
Almukadi, H., Wu, H., Bohlke, M., Kelley, C. J., Maher, T. J., and Pino-Figueroa, A. (2013). The macamide N-3-methoxybenzyl-linoleamide is a time-dependent fatty acid amide hydrolase (FAAH) inhibitor. Mol. Neurobiol. 48 (2), 333–339. doi:10.1007/s12035-013-8499-2
Anagianni, S., and Tuschl, K. (2019). Genetic disorders of manganese metabolism. Curr. Neurol. Neurosci. Rep. 19 (6), 33. doi:10.1007/s11910-019-0942-y
Andersen, O., and Aaseth, J. (2016). A review of pitfalls and progress in chelation treatment of metal poisonings. J. Trace Elem. Med. Biol. 38, 74–80. doi:10.1016/j.jtemb.2016.03.013
Apaydin, M., Erbas, O., and Taskiran, D. (2016). Protection by edaravone, a radical scavenger, against manganese-induced neurotoxicity in rats. J. Biochem. Mol. Toxicol. 30 (5), 217–223. doi:10.1002/jbt.21780
Aschner, J. L., and Aschner, M. (2005). Nutritional aspects of manganese homeostasis. Mol. Asp. Med. 26 (4-5), 353–362. doi:10.1016/j.mam.2005.07.003
Asefy, Z., Khusro, A., Mammadova, S., Hoseinnejhad, S., Eftekhari, A., Alghamdi, S., et al. (2021). Melatonin hormone as a therapeutic weapon against neurodegenerative diseases. Cell. Mol. Biol. 67 (3), 99–106. doi:10.14715/cmb/2021.67.3.13
Avila, D. S., Benedetto, A., Au, C., Manarin, F., Erikson, K., Soares, F. A., et al. (2012). Organotellurium and organoselenium compounds attenuate Mn-induced toxicity in Caenorhabditis elegans by preventing oxidative stress. Free Radic. Biol. Med. 52 (9), 1903–1910. doi:10.1016/j.freeradbiomed.2012.02.044
Avila, D. S., Colle, D., Gubert, P., Palma, A. S., Puntel, G., Manarin, F., et al. (2010). A possible neuroprotective action of a vinylic telluride against Mn-induced neurotoxicity. Toxicol. Sci. 115 (1), 194–201. doi:10.1093/toxsci/kfq036
Bae, J. H., Jang, B. C., Suh, S. I., Ha, E., Baik, H. H., Kim, S. S., et al. (2006). Manganese induces inducible nitric oxide synthase (iNOS) expression via activation of both MAP kinase and PI3K/Akt pathways in BV2 microglial cells. Neurosci. Lett. 398 (1-2), 151–154. doi:10.1016/j.neulet.2005.12.067
Bahar, E., Kim, J. Y., and Yoon, H. (2017). Quercetin attenuates manganese-induced neuroinflammation by alleviating oxidative stress through regulation of apoptosis, iNOS/NF-κB and HO-1/Nrf2 pathways. Int. J. Mol. Sci. 18 (9), 1989. doi:10.3390/ijms18091989
Bahar, E., Lee, G. H., Bhattarai, K. R., Lee, H. Y., Choi, M. K., Rashid, H. O., et al. (2017). Polyphenolic extract of Euphorbia supina attenuates manganese-induced neurotoxicity by enhancing antioxidant activity through regulation of ER stress and ER stress-mediated apoptosis. Int. J. Mol. Sci. 18 (2), 300. doi:10.3390/ijms18020300
Bahr, B. A., Wisniewski, M. L., and Butler, D. (2012). Positive lysosomal modulation as a unique strategy to treat age-related protein accumulation diseases. Rejuvenation Res. 15 (2), 189–197. doi:10.1089/rej.2011.1282
Barhoumi, R., Faske, J., Liu, X., and Tjalkens, R. B. (2004). Manganese potentiates lipopolysaccharide-induced expression of NOS2 in C6 glioma cells through mitochondrial-dependent activation of nuclear factor kappaB. Brain Res. Mol. Brain Res. 122 (2), 167–179. doi:10.1016/j.molbrainres.2003.12.009
Bhandari, R., Kuhad, A., and Kuhad, A. (2018). Edaravone: A new hope for deadly amyotrophic lateral sclerosis. Drugs Today (Barc) 54 (6), 349–360. doi:10.1358/dot.2018.54.6.2828189
Bianchini, M. C., Gularte, C. O. A., Escoto, D. F., Pereira, G., Gayer, M. C., Roehrs, R., et al. (2016). Peumus boldus (boldo) aqueous extract present better protective effect than boldine against manganese-induced toxicity in D. melanogaster. Neurochem. Res. 41 (10), 2699–2707. doi:10.1007/s11064-016-1984-z
Bonilla, E., ContReRas, R., Medina-Leendertz, S., MoraM., , Villalobos, V., and Bravo, Y. (2012). Minocycline increases the life span and motor activity and decreases lipid peroxidation in manganese treated Drosophila melanogaster. Toxicology 294 (1), 50–53. doi:10.1016/j.tox.2012.01.016
Bora, S., Erdogan, M. A., Armagan, G., Sevgili, E., and Dagci, T. (2016). Vinpocetine and vasoactive intestinal peptide attenuate manganese-induced toxicity in NE-4C cells. Biol. Trace Elem. Res. 174 (2), 410–418. doi:10.1007/s12011-016-0742-z
Bornhorst, J., Chakraborty, S., Meyer, S., Lohren, H., Brinkhaus, S. G., Knight, A. L., et al. (2014). The effects of pdr1, djr1.1 and pink1 loss in manganese-induced toxicity and the role of alpha-synuclein in C. elegans. Metallomics 6 (3), 476–490. doi:10.1039/c3mt00325f
Bowman, A. B., and Aschner, M. (2014). Considerations on manganese (Mn) treatments for in vitro studies. Neurotoxicology 41, 141–142. doi:10.1016/j.neuro.2014.01.010
Brouillet, E. P., Shinobu, L., McGarvey, U., HochbergF., , and Beal, M. F. (1993). Manganese injection into the rat striatum produces excitotoxic lesions by impairing energy metabolism. Exp. Neurol. 120 (1), 89–94. doi:10.1006/exnr.1993.1042
Bryan, M. R., O'Brien, M. T., Nordham, K. D., Rose, D. I. R., Foshage, A. M., Joshi, P., et al. (2019). Acute manganese treatment restores defective autophagic cargo loading in Huntington's disease cell lines. Hum. Mol. Genet. 28 (22), 3825–3841. doi:10.1093/hmg/ddz209
Buettner, G. R., Ng, C. F., Wang, M., Rodgers, V. G. J., and Schafer, F. Q. (2006). A new paradigm: Manganese superoxide dismutase influences the production of H2O2 in cells and thereby their biological state. Free Radic. Biol. Med. 41 (8), 1338–1350. doi:10.1016/j.freeradbiomed.2006.07.015
Cai, T., Che, H., Yao, T., Chen, Y., Huang, C., Zhang, W., et al. (2011). Manganese induces tau hyperphosphorylation through the activation of ERK MAPK pathway in PC12 cells. Toxicol. Sci. 119 (1), 169–177. doi:10.1093/toxsci/kfq308
Cai, T., Yao, T., Zheng, G., Chen, Y., Du, K., Cao, Y., et al. (2010). Manganese induces the overexpression of alpha-synuclein in PC12 cells via ERK activation. Brain Res. 1359, 201–207. doi:10.1016/j.brainres.2010.08.055
Cao, Y. M., Fan, X. M., Xu, J., Liu, J., and Fan, Q. Y. (2022). Manganese intoxication recovery and the expression changes of park2/parkin in rats. Neurochem. Res. 47 (4), 897–906. doi:10.1007/s11064-021-03493-w
Cargnello, M., and Roux, P. P. (2011). Activation and function of the MAPKs and their substrates, the MAPK-activated protein kinases. Microbiol. Mol. Biol. Rev. 75 (1), 50–83. doi:10.1128/MMBR.00031-10
Cersosimo, M. G., and Koller, W. C. (2006). The diagnosis of manganese-induced parkinsonism. Neurotoxicology 27 (3), 340–346. doi:10.1016/j.neuro.2005.10.006
Chakraborty, S., Chen, P., Bornhorst, J., Schwerdtle, T., Schumacher, F., Kleuser, B., et al. (2015). Loss of pdr-1/parkin influences Mn homeostasis through altered ferroportin expression in C. elegans. Metallomics 7 (5), 847–856. doi:10.1039/c5mt00052a
Chapman-Smith, A., Lutwyche, J. K., and Whitelaw, M. L. (2004). Contribution of the Per/Arnt/Sim (PAS) domains to DNA binding by the basic helix-loop-helix PAS transcriptional regulators. J. Biol. Chem. 279 (7), 5353–5362. doi:10.1074/jbc.M310041200
Chen, J., Su, P., and Luo, W. (2018). Role of LRRK2 in manganese-induced neuroinflammation and microglial autophagy. Biochem. Biophys. Res. Commun. 498 (1), 171–177. doi:10.1016/j.bbrc.2018.02.007
Chen, J. X., Yao, L. H., Xu, B. B., Qian, K., Wang, H. L., Liu, Z. C., et al. (2014). Glutamate transporter 1-mediated antidepressant-like effect in a rat model of chronic unpredictable stress. J. Huazhong Univ. Sci. Technol. Med. Sci. 34 (6), 838–844. doi:10.1007/s11596-014-1362-5
Chen, J. Y., Tsao, G. C., Zhao, Q., and Zheng, W. (2001). Differential cytotoxicity of Mn(II) and Mn(III): Special reference to mitochondrial [Fe-S] containing enzymes. Toxicol. Appl. Pharmacol. 175 (2), 160–168. doi:10.1006/taap.2001.9245
Chen, L., Huang, Y., Yu, X., Lu, J., Jia, W., Song, J., et al. (2021). Corynoxine protects dopaminergic neurons through inducing autophagy and diminishing neuroinflammation in rotenone-induced animal models of Parkinson's disease. Front. Pharmacol. 12, 642900. doi:10.3389/fphar.2021.642900
Chen, P., Culbreth, M., and Aschner, M. (2016). Exposure, epidemiology, and mechanism of the environmental toxicant manganese. Environ. Sci. Pollut. Res. Int. 23 (14), 13802–13810. doi:10.1007/s11356-016-6687-0
Cheng, H., Xia, B., Su, C., Chen, K., Chen, X., Chen, P., et al. (2018). PI3K/Akt signaling pathway and Hsp70 activate in hippocampus of rats with chronic manganese sulfate exposure. J. Trace Elem. Med. Biol. 50, 332–338. doi:10.1016/j.jtemb.2018.07.019
Chong, J. A., Tapia-Ramirez, J., Kim, S., Toledo-Aral, J. J., Zheng, Y., Boutros, M. C., et al. (1995). Rest: A mammalian silencer protein that restricts sodium channel gene expression to neurons. Cell 80 (6), 949–957. doi:10.1016/0092-8674(95)90298-8
Chopra, D., Sharma, S., Sharma, N., and Nehru, B. (2021). N-acetylcysteine ameliorates neurotoxic effects of manganese intoxication in rats: A biochemical and behavioral study. Neurochem. Res. 46 (8), 1953–1969. doi:10.1007/s11064-021-03312-2
Chtourou, Y., Fetoui, H., Sefi, M., Trabelsi, K., Barkallah, M., Boudawara, T., et al. (2010). Silymarin, a natural antioxidant, protects cerebral cortex against manganese-induced neurotoxicity in adult rats. Biometals 23 (6), 985–996. doi:10.1007/s10534-010-9345-x
Chun, H. S., Lee, H., and Son, J. H. (2001). Manganese induces endoplasmic reticulum (ER) stress and activates multiple caspases in nigral dopaminergic neuronal cells, SN4741. Neurosci. Lett. 316 (1), 5–8. doi:10.1016/s0304-3940(01)02341-2
Cong, L., Lei, M. Y., Liu, Z. Q., Liu, Z. F., Ma, Z., Liu, K., et al. (2021). Resveratrol attenuates manganese-induced oxidative stress and neuroinflammation through SIRT1 signaling in mice. Food Chem. Toxicol. 153, 112283. doi:10.1016/j.fct.2021.112283
Cordova, F. M., Aguiar, A. S., Peres, T. V., Lopes, M. W., Goncalves, F. M., Pedro, D. Z., et al. (2013). Manganese-exposed developing rats display motor deficits and striatal oxidative stress that are reversed by Trolox. Arch. Toxicol. 87 (7), 1231–1244. doi:10.1007/s00204-013-1017-5
Crittenden, P. L., and Filipov, N. M. (2008). Manganese-induced potentiation of in vitro proinflammatory cytokine production by activated microglial cells is associated with persistent activation of p38 MAPK. Toxicol. Vitro 22 (1), 18–27. doi:10.1016/j.tiv.2007.07.004
da Silva, E. B., Eichwald, T., Glaser, V., Varela, K. G., Baptistella, A. R., de Carvalho, D., et al. (2022). Protective effects of probucol on different brain cells exposed to manganese. Neurotox. Res. 40 (1), 276–285. doi:10.1007/s12640-021-00458-3
da Silva Santos, V., Bisen-Hersh, E., Yu, Y., Cabral, I. S. R., Nardini, V., Culbreth, M., et al. (2014). Anthocyanin-rich acai (Euterpe oleracea Mart.) extract attenuates manganese-induced oxidative stress in rat primary astrocyte cultures. J. Toxicol. Environ. Health. A 77 (7), 390–404. doi:10.1080/15287394.2014.880392
Danbolt, N. C. (2001). Glutamate uptake. Prog. Neurobiol. 65 (1), 1–105. doi:10.1016/s0301-0082(00)00067-8
Deng, Y., Jiao, C., Xu, B., Li, Y., Wang, F., et al. (2015). Melatonin inhibits manganese-induced motor dysfunction and neuronal loss in mice: Involvement of oxidative stress and dopaminergic neurodegeneration. Mol. Neurobiol. 51 (1), 68–88. doi:10.1007/s12035-014-8789-3
Deng, Y., Peng, D., Yang, C., Zhao, L., Li, J., Lu, L., et al. (2021). Preventive treatment with sodium para-aminosalicylic acid inhibits manganese-induced apoptosis and inflammation via the MAPK pathway in rat thalamus. Drug Chem. Toxicol., 1–10. doi:10.1080/01480545.2021.2008127
Deng, Y., Xu, Z., Xu, B., Tian, Y., Xin, X., Deng, X., et al. (2009). The protective effect of riluzole on manganese caused disruption of glutamate-glutamine cycle in rats. Brain Res. 1289, 106–117. doi:10.1016/j.brainres.2009.07.012
Deng, Y., Xu, Z., Xu, B., Xu, D., Tian, Y., and Feng, W. (2012). The protective effects of riluzole on manganese-induced disruption of glutamate transporters and glutamine synthetase in the cultured astrocytes. Biol. Trace Elem. Res. 148 (2), 242–249. doi:10.1007/s12011-012-9365-1
Desvergne, B., and Wahli, W. (1999). Peroxisome proliferator-activated receptors: Nuclear control of metabolism. Endocr. Rev. 20 (5), 649–688. doi:10.1210/edrv.20.5.0380
Discalzi, G., Pira, E., HerrEro HernandEz, E., Valentini, C., TurbiglioM., , and MeligaF., (2000). Occupational Mn parkinsonism: Magnetic resonance imaging and clinical patterns following CaNa2-EDTA chelation. Neurotoxicology 21 (5), 863–866.
Dobson, A. W., Weber, S., Dorman, D. C., Lash, L. K., Erikson, K. M., and Aschner, M. (2003). Oxidative stress is induced in the rat brain following repeated inhalation exposure to manganese sulfate. Biol. Trace Elem. Res. 93 (1-3), 113–126. doi:10.1385/BTER:93:1-3:113
Dzamko, N. L. (2017). LRRK2 and the immune system. Adv. Neurobiol. 14, 123–143. doi:10.1007/978-3-319-49969-7_7
Erikson, K., and Aschner, M. (2002). Manganese causes differential regulation of glutamate transporter (GLAST) taurine transporter and metallothionein in cultured rat astrocytes. Neurotoxicology 23 (4-5), 595–602. doi:10.1016/s0161-813x(02)00012-8
Erikson, K. M., Dobson, A. W., Dorman, D. C., and Aschner, M. (2004). Manganese exposure and induced oxidative stress in the rat brain. Sci. Total Environ. 334-335, 409–416. doi:10.1016/j.scitotenv.2004.04.044
Erikson, K. M., Dorman, D. C., Fitsanakis, V., Lash, L. H., and Aschner, M. (2006). Alterations of oxidative stress biomarkers due to in utero and neonatal exposures of airborne manganese. Biol. Trace Elem. Res. 111 (1-3), 199–215. doi:10.1385/BTER:111:1:199
Erikson, K. M., Suber, R. L., and Aschner, M. (2002). Glutamate/aspartate transporter (GLAST), taurine transporter and metallothionein mRNA levels are differentially altered in astrocytes exposed to manganese chloride, manganese phosphate or manganese sulfate. Neurotoxicology 23 (3), 281–288. doi:10.1016/s0161-813x(02)00041-4
Evren, V., Apaydin, M., Khalilnezhad, A., Erbas, O., and Taskiran, D. (2015). Protective effect of edaravone against manganese-induced toxicity in cultured rat astrocytes. Environ. Toxicol. Pharmacol. 40 (2), 563–567. doi:10.1016/j.etap.2015.08.010
Filipov, N. M., Seegal, R. F., and Lawrence, D. A. (2005). Manganese potentiates in vitro production of proinflammatory cytokines and nitric oxide by microglia through a nuclear factor kappa B-dependent mechanism. Toxicol. Sci. 84 (1), 139–148. doi:10.1093/toxsci/kfi055
Flick, F., and Luscher, B. (2012). Regulation of sirtuin function by posttranslational modifications. Front. Pharmacol. 3, 29. doi:10.3389/fphar.2012.00029
Foster, M. L., Bartnikas, T. B., Maresca-Fichter, H. C., Mercadante, C., Dash, M., Miller, C., et al. (2018). Neonatal C57BL/6J and parkin mice respond differently following developmental manganese exposure: Result of a high dose pilot study. Neurotoxicology 64, 291–299. doi:10.1016/j.neuro.2017.10.002
Fu, X., Chen, S., Wang, X., Shen, Y., Zeng, R., Wu, Q., et al. (2021). Dendrobium nobile Lindl. alkaloids alleviate Mn-induced neurotoxicity via PINK1/Parkin-mediated mitophagy in PC12 cells. Biochem. Biophys. Rep. 26, 100877. doi:10.1016/j.bbrep.2020.100877
Fumagalli, E., Funicello, M., Rauen, T., Gobbi, M., and Mennini, T. (2008). Riluzole enhances the activity of glutamate transporters GLAST, GLT1 and EAAC1. Eur. J. Pharmacol. 578 (2-3), 171–176. doi:10.1016/j.ejphar.2007.10.023
Gawlik, M., Smaga, I., and Filip, M. (2017). Manganese neurotoxicity and protective effects of resveratrol and quercetin in preclinical research. Pharmacol. Rep. 69 (2), 322–330. doi:10.1016/j.pharep.2016.11.011
Ghavami, S., Shojaei, S., Yeganeh, B., Ande, S. R., Jangamreddy, J. R., Mehrpour, M., et al. (2014). Autophagy and apoptosis dysfunction in neurodegenerative disorders. Prog. Neurobiol. 112, 24–49. doi:10.1016/j.pneurobio.2013.10.004
Gordon, S., Akopyan, G., Garban, H., and Bonavida, B. (2006). Transcription factor YY1: Structure, function, and therapeutic implications in cancer biology. Oncogene 25 (8), 1125–1142. doi:10.1038/sj.onc.1209080
Gorojod, R. M., Alaimo, A., Porte Alcon, S., Martinez, J. H., Cortina, M. E., Vazquez, E. S., et al. (2018). Heme Oxygenase-1 protects astroglia against manganese-induced oxidative injury by regulating mitochondrial quality control. Toxicol. Lett. 295, 357–368. doi:10.1016/j.toxlet.2018.07.045
Gugnani, K. S., Vu, N., Rondon-Ortiz, A. N., Bohlke, M., Maher, T. J., and Pino-Figueroa, A. J. (2018). Neuroprotective activity of macamides on manganese-induced mitochondrial disruption in U-87 MG glioblastoma cells. Toxicol. Appl. Pharmacol. 340, 67–76. doi:10.1016/j.taap.2017.12.014
Guo, Z., Zhang, Z., Wang, Q., Zhang, J., Wang, L., Zhang, Q., et al. (2018). Manganese chloride induces histone acetylation changes in neuronal cells: Its role in manganese-induced damage. Neurotoxicology 65, 255–263. doi:10.1016/j.neuro.2017.11.003
HaMai, D., Campbell, A., and Bondy, S. C. (2001). Modulation of oxidative events by multivalent manganese complexes in brain tissue. Free Radic. Biol. Med. 31 (6), 763–768. doi:10.1016/s0891-5849(01)00639-6
Harischandra, D. S., Ghaisas, S., Rokad, D., Zamanian, M., Jin, H., Anantharam, V., et al. (2018). Environmental neurotoxicant manganese regulates exosome-mediated extracellular miRNAs in cell culture model of Parkinson's disease: Relevance to alpha-synuclein misfolding in metal neurotoxicity. Neurotoxicology 64, 267–277. doi:10.1016/j.neuro.2017.04.007
Harischandra, D. S., Ghaisas, S., Zenitsky, G., Jin, H., Kanthasamy, A., Anantharam, V., et al. (2019). Manganese-induced neurotoxicity: New insights into the triad of protein misfolding, mitochondrial impairment, and neuroinflammation. Front. Neurosci. 13, 654. doi:10.3389/fnins.2019.00654
Harischandra, D. S., Rokad, D., Neal, M. L., Ghaisas, S., Manne, S., Sarkar, S., et al. (2019). Manganese promotes the aggregation and prion-like cell-to-cell exosomal transmission of alpha-synuclein. Sci. Signal. 12 (572), eaau4543. doi:10.1126/scisignal.aau4543
Hazell, A. S., and Norenberg, M. D. (1997). Manganese decreases glutamate uptake in cultured astrocytes. Neurochem. Res. 22 (12), 1443–1447. doi:10.1023/a:1021994126329
He, R., Xie, X., Lv, L., Huang, Y., Xia, X., Chen, X., et al. (2017). Comprehensive investigation of aberrant microRNAs expression in cells culture model of MnCl2-induced neurodegenerative disease. Biochem. Biophys. Res. Commun. 486 (2), 342–348. doi:10.1016/j.bbrc.2017.03.041
Healy, D. G., Falchi, M., O'Sullivan, S. S., Bonifati, V., Durr, A., Bressman, S., et al. (2008). Phenotype, genotype, and worldwide genetic penetrance of LRRK2-associated Parkinson's disease: A case-control study. Lancet. Neurol. 7 (7), 583–590. doi:10.1016/S1474-4422(08)70117-0
Heng, Y. Y., Asad, I., Coleman, B., Menard, L., Benki-Nugent, S., Hussein Were, F., et al. (2022). Heavy metals and neurodevelopment of children in low and middle-income countries: A systematic review. PLoS One 17 (3), e0265536. doi:10.1371/journal.pone.0265536
Higashi, Y., Asanuma, M., Miyazaki, I., Hattori, N., Mizuno, Y., and Ogawa, N. (2004). Parkin attenuates manganese-induced dopaminergic cell death. J. Neurochem. 89 (6), 1490–1497. doi:10.1111/j.1471-4159.2004.02445.x
Himeda, T., Kadoguchi, N., Kamiyama, Y., Kato, H., Maegawa, H., and Araki, T. (2006). Neuroprotective effect of arundic acid, an astrocyte-modulating agent, in mouse brain against MPTP (1-methyl-4-phenyl-1, 2, 3, 6-tetrahydropyridine) neurotoxicity. Neuropharmacology 50 (3), 329–344. doi:10.1016/j.neuropharm.2005.09.014
Huang, Y., Wen, Q., Huang, J., Luo, M., Xiao, Y., Mo, R., et al. (2021). Manganese (II) chloride leads to dopaminergic neurotoxicity by promoting mitophagy through BNIP3-mediated oxidative stress in SH-SY5Y cells. Cell. Mol. Biol. Lett. 26 (1), 23. doi:10.1186/s11658-021-00267-8
Ibrahim, F., Nomier, M. A., Sabik, L. M. E., and Shaheen, M. A. (2020). Manganese-induced neurotoxicity and the potential protective effects of lipoic acid and Spirulina platensis. Toxicol. Mech. Methods 30 (7), 497–507. doi:10.1080/15376516.2020.1771803
Ijomone, O. M., Iroegbu, J. D., Morcillo, P., Ayodele, A. J., Bornhorst, J., et al. (2022). Sex-dependent metal accumulation and immunoexpression of Hsp70 and Nrf2 in rats' brain following manganese exposure. Environ. Toxicol. 37, 2167–2177. doi:10.1002/tox.23583
Irifune, M., Kikuchi, N., Saida, T., Takarada, T., Shimizu, Y., Endo, C., et al. (2007). Riluzole, a glutamate release inhibitor, induces loss of righting reflex, antinociception, and immobility in response to noxious stimulation in mice. Anesth. Analg. 104 (6), 1415–1421. doi:10.1213/01.ane.0000263267.04198.36
Isaac, A. O., Kawikova, I., Bothwell, A. L. M., Daniels, C. K., and Lai, J. C. K. (2006). Manganese treatment modulates the expression of peroxisome proliferator-activated receptors in astrocytoma and neuroblastoma cells. Neurochem. Res. 31 (11), 1305–1316. doi:10.1007/s11064-006-9173-0
Islam, M. S., Quispe, C., Hossain, R., Islam, M. T., Al-Harrasi, A., Al-Rawahi, A., et al. (2021). Neuropharmacological effects of quercetin: A literature-based review. Front. Pharmacol. 12, 665031. doi:10.3389/fphar.2021.665031
Ivleva, I. S., Pestereva, N. S., Tyutyunnik, T. V., and Karpenko, M. N. (2022). Protective effect of calpain inhibitors against manganese-induced toxicity in rats. Metab. Brain Dis. 37 (4), 1003–1013. doi:10.1007/s11011-022-00916-7
Ji, H., Ma, J., Chen, L., Chen, T., Zhang, S., Jia, J., et al. (2020). Pyrroloquinoline quinine and LY294002 changed cell cycle and apoptosis by regulating PI3K-AKT-GSK3β pathway in SH-SY5Y cells. Neurotox. Res. 38 (2), 266–273. doi:10.1007/s12640-020-00210-3
Jiang, Y. M., Mo, X. A., Du, F. Q., Fu, X., Zhu, X. Y., Gao, H. Y., et al. (2006). Effective treatment of manganese-induced occupational parkinsonism with p-aminosalicylic acid: A case of 17-year follow-up study. J. Occup. Environ. Med. 48 (6), 644–649. doi:10.1097/01.jom.0000204114.01893.3e
Jing, M. J., Liu, K., Liu, C., Yan, D. Y., Ma, Z., Wang, C., et al. (2020). Protective effects of trehalose against Mn-induced alpha-synuclein oligomerization in mice: Involvement of oxidative stress and autophagy. Environ. Toxicol. 35 (1), 55–65. doi:10.1002/tox.22842
Johnson, J., Pajarillo, E. A. B., Taka, E., Reams, R., Son, D. S., Aschner, M., et al. (2018). Valproate and sodium butyrate attenuate manganese-decreased locomotor activity and astrocytic glutamate transporters expression in mice. Neurotoxicology 64, 230–239. doi:10.1016/j.neuro.2017.06.007
Johnson, J., Pajarillo, E., Karki, P., Kim, J., Son, D. S., Aschner, M., et al. (2018). Valproic acid attenuates manganese-induced reduction in expression of GLT-1 and GLAST with concomitant changes in murine dopaminergic neurotoxicity. Neurotoxicology 67, 112–120. doi:10.1016/j.neuro.2018.05.001
Karki, P., Hong, P., Johnson, J., Pajarillo, E., Son, D. S., Aschner, M., et al. (2018). Arundic acid increases expression and function of astrocytic glutamate transporter EAAT1 via the ERK, Akt, and NF-κB pathways. Mol. Neurobiol. 55 (6), 5031–5046. doi:10.1007/s12035-017-0709-x
Karki, P., Kim, C., Smith, K., Son, D. S., Aschner, M., and Lee, E. (2015). Transcriptional regulation of the astrocytic excitatory amino acid transporter 1 (EAAT1) via NF-κB and yin yang 1 (YY1). J. Biol. Chem. 290 (39), 23725–23737. doi:10.1074/jbc.M115.649327
Karki, P., Smith, K., Johnson, J., Aschner, M., and Lee, E. (2015). Role of transcription factor yin yang 1 in manganese-induced reduction of astrocytic glutamate transporters: Putative mechanism for manganese-induced neurotoxicity. Neurochem. Int. 88, 53–59. doi:10.1016/j.neuint.2014.08.002
Karki, P., Webb, A., Smith, K., Johnson, J., Lee, K., Son, D. S., et al. (2014). Yin Yang 1 is a repressor of glutamate transporter EAAT2, and it mediates manganese-induced decrease of EAAT2 expression in astrocytes. Mol. Cell. Biol. 34 (7), 1280–1289. doi:10.1128/MCB.01176-13
Karki, P., Webb, A., Zerguine, A., Choi, J., Son, D. S., and Lee, E. (2014). Mechanism of raloxifene-induced upregulation of glutamate transporters in rat primary astrocytes. Glia 62 (8), 1270–1283. doi:10.1002/glia.22679
Kato, H., Kurosaki, R., Oki, C., and Araki, T. (2004). Arundic acid, an astrocyte-modulating agent, protects dopaminergic neurons against MPTP neurotoxicity in mice. Brain Res. 1030 (1), 66–73. doi:10.1016/j.brainres.2004.09.046
Kim, J., Pajarillo, E., Rizor, A., Son, D. S., Lee, J., Aschner, M., et al. (2019). LRRK2 kinase plays a critical role in manganese-induced inflammation and apoptosis in microglia. PLoS One 14 (1), e0210248. doi:10.1371/journal.pone.0210248
Kirkley, K. S., Popichak, K. A., Afzali, M. F., Legare, M. E., and Tjalkens, R. B. (2017). Microglia amplify inflammatory activation of astrocytes in manganese neurotoxicity. J. Neuroinflammation 14 (1), 99. doi:10.1186/s12974-017-0871-0
Kosnett, M. J. (2010). Chelation for heavy metals (arsenic, lead, and mercury): Protective or perilous? Clin. Pharmacol. Ther. 88 (3), 412–415. doi:10.1038/clpt.2010.132
Kulkarni, N., Gadde, R., Gugnani, K. S., Vu, N., Yoo, C., Zaveri, R., et al. (2021). Neuroprotective effects of disubstituted dithiolethione ACDT against manganese-induced toxicity in SH-SY5Y cells. Neurochem. Int. 147, 105052. doi:10.1016/j.neuint.2021.105052
Kwakye, G. F., Paoliello, M. M. B., Mukhopadhyay, S., Bowman, A. B., and Aschner, M. (2015). Manganese-induced parkinsonism and Parkinson's disease: Shared and distinguishable features. Int. J. Environ. Res. Public Health 12 (7), 7519–7540. doi:10.3390/ijerph120707519
Lai, L. Y., Dou, C. S., Zhi, C. N., Chen, J., Ma, X., Zhao, P., et al. (2022). Curcumin alleviates the manganese-induced neurotoxicity by promoting autophagy in rat models of manganism. Beijing Da Xue Xue Bao Yi Xue Ban. 54 (3), 400–411. doi:10.19723/j.issn.1671-167X.2022.03.003
Lee, E., Sidoryk-Wegrzynowicz, M., Yin, Z., Webb, A., Son, D. S., and Aschner, M. (2012). Transforming growth factor-alpha mediates estrogen-induced upregulation of glutamate transporter GLT-1 in rat primary astrocytes. Glia 60 (7), 1024–1036. doi:10.1002/glia.22329
Lee, E. S., Sidoryk, M., Jiang, H., Yin, Z., and Aschner, M. (2009). Estrogen and tamoxifen reverse manganese-induced glutamate transporter impairment in astrocytes. J. Neurochem. 110 (2), 530–544. doi:10.1111/j.1471-4159.2009.06105.x
Lee, E. S., Yin, Z., Milatovic, D., Jiang, H., and Aschner, M. (2009). Estrogen and tamoxifen protect against Mn-induced toxicity in rat cortical primary cultures of neurons and astrocytes. Toxicol. Sci. 110 (1), 156–167. doi:10.1093/toxsci/kfp081
Lee, J. H., and Shin, J. H. (2022). Effect of chelation therapy on a Korean patient with brain manganese deposition resulting from a compound heterozygous mutation in the SLC39A14 gene. J. Mov. Disord. 15 (2), 171–174. doi:10.14802/jmd.21143
Li, H., Wu, S., Shi, N., Lian, S., and Lin, W. (2011). Nrf2/HO-1 pathway activation by manganese is associated with reactive oxygen species and ubiquitin-proteasome pathway, not MAPKs signaling. J. Appl. Toxicol. 31 (7), 690–697. doi:10.1002/jat.1654
Li, H., Wu, S., Shi, N., Lin, W., You, J., and Zhou, W. (2011). NF-E2-related factor 2 activation in PC12 cells: Its protective role in manganese-induced damage. Arch. Toxicol. 85 (8), 901–910. doi:10.1007/s00204-010-0625-6
Li, J., Deng, Y., Peng, D., Zhao, L., Fang, Y., Zhu, X., et al. (2021). Sodium P-aminosalicylic acid attenuates manganese-induced neuroinflammation in BV2 microglia by modulating NF-κB pathway. Biol. Trace Elem. Res. 199 (12), 4688–4699. doi:10.1007/s12011-021-02581-w
Li, S. J., Ou, C. Y., He, S. N., Huang, X. W., Luo, H. L., Meng, H. Y., et al. (2017). Sodium p-aminosalicylic acid reverses sub-chronic manganese-induced impairments of spatial learning and memory abilities in rats, but fails to restore gamma-aminobutyric acid levels. Int. J. Environ. Res. Public Health 14 (4), 400. doi:10.3390/ijerph14040400
Li, Y., Sun, L., Cai, T., Zhang, Y., Lv, S., Wang, Y., et al. (2010). alpha-Synuclein overexpression during manganese-induced apoptosis in SH-SY5Y neuroblastoma cells. Brain Res. Bull. 81 (4-5), 428–433. doi:10.1016/j.brainresbull.2009.11.007
Liu, C., Jursa, T., Aschner, M., Smith, D. R., and Mukhopadhyay, S. (2021). Up-regulation of the manganese transporter SLC30A10 by hypoxia-inducible factors defines a homeostatic response to manganese toxicity. Proc. Natl. Acad. Sci. U. S. A. 118 (35), e2107673118. doi:10.1073/pnas.2107673118
Liu, K., Jing, M. J., Liu, C., Yan, D. Y., Ma, Z., Wang, C., et al. (2019). Effect of trehalose on manganese-induced mitochondrial dysfunction and neuronal cell damage in mice. Basic Clin. Pharmacol. Toxicol. 125 (6), 536–547. doi:10.1111/bcpt.13316
Liu, K., Liu, Z., Liu, Z., Ma, Z., Deng, Y., Liu, W., et al. (2022). Manganese induces S-nitrosylation of PINK1 leading to nerve cell damage by repressing PINK1/Parkin-mediated mitophagy. Sci. Total Environ. 834, 155358. doi:10.1016/j.scitotenv.2022.155358
Liu, X., Cai, Y., Wang, Y., Xu, S., Ji, K., and Choi, K. (2022). Corrigendum to "Effects of tris(1, 3-dichloro-2-propyl) phosphate (TDCPP) and triphenyl phosphate (TPP) on sex dependent alterations of thyroid hormones in adult zebrafish" [Ecotoxicol. Environ. Saf. 170 (2019) 25-32]. Ecotoxicol. Environ. Saf. 241, 110867. doi:10.1016/j.ecoenv.2020.110867
Liu, Z. Q., Liu, K., Cong, L., Lei, M. Y., Ma, Z., et al. (2021). Corrigendum to "Manganese-induced alpha-synuclein overexpression aggravates mitochondrial damage by repressing PINK1/Parkin-mediated mitophagy" [Food Chem. Toxicol. 152 (2021) 112213]. Food Chem. Toxicol. 152, 112660. doi:10.1016/j.fct.2021.112660
Lu, T., Aron, L., Zullo, J., Pan, Y., Kim, H., Chen, Y., et al. (2014). REST and stress resistance in ageing and Alzheimer's disease. Nature 507 (7493), 448–454. doi:10.1038/nature13163
Lucchini, R. G., Albini, E., Benedetti, L., Borghesi, S., Coccaglio, R., Malara, E. C., et al. (2007). High prevalence of Parkinsonian disorders associated to manganese exposure in the vicinities of ferroalloy industries. Am. J. Ind. Med. 50 (11), 788–800. doi:10.1002/ajim.20494
Lucchini, R. G., Guazzetti, S., Zoni, S., Donna, F., Peter, S., Zacco, A., et al. (2012). Tremor, olfactory and motor changes in Italian adolescents exposed to historical ferro-manganese emission. Neurotoxicology 33 (4), 687–696. doi:10.1016/j.neuro.2012.01.005
Ma, X., Han, J., Wu, Q., Liu, H., Shi, S., Wang, C., et al. (2015). Involvement of dysregulated Wip1 in manganese-induced p53 signaling and neuronal apoptosis. Toxicol. Lett. 235 (1), 17–27. doi:10.1016/j.toxlet.2014.12.019
Ma, Z., Wang, C., Liu, C., Yan, D. Y., Deng, Y., Liu, W., et al. (2017). The role S-nitrosylation in manganese-induced autophagy dysregulation in SH-SY5Y cells. Environ. Toxicol. 32 (12), 2428–2439. doi:10.1002/tox.22457
Ma, Z., Wang, C., Liu, C., Yan, D. Y., Tan, X., Liu, K., et al. (2020). Manganese induces autophagy dysregulation: The role of S-nitrosylation in regulating autophagy related proteins in vivo and in vitro. Sci. Total Environ. 698, 134294. doi:10.1016/j.scitotenv.2019.134294
Maddirala, Y., Tobwala, S., and Ercal, N. (2015). N-acetylcysteineamide protects against manganese-induced toxicity in SHSY5Y cell line. Brain Res. 1608, 157–166. doi:10.1016/j.brainres.2015.02.006
Mai, A., Rotili, D., Valente, S., and Kazantsev, A. G. (2009). Histone deacetylase inhibitors and neurodegenerative disorders: Holding the promise. Curr. Pharm. Des. 15 (34), 3940–3957. doi:10.2174/138161209789649349
Martinez-Finley, E. J., Gavin, C. E., Aschner, M., and Gunter, T. E. (2013). Manganese neurotoxicity and the role of reactive oxygen species. Free Radic. Biol. Med. 62, 65–75. doi:10.1016/j.freeradbiomed.2013.01.032
McGann, J. C., Spinner, M. A., Garg, S. K., Mullendorff, K. A., Woltjer, R. L., and Mandel, G. (2021). The genome-wide binding profile for human RE1 silencing transcription factor unveils a unique genetic circuitry in Hippocampus. J. Neurosci. 41 (31), 6582–6595. doi:10.1523/JNEUROSCI.2059-20.2021
Michalik, L., Desvergne, B., and Wahli, W. (2004). Peroxisome-proliferator-activated receptors and cancers: Complex stories. Nat. Rev. Cancer 4 (1), 61–70. doi:10.1038/nrc1254
Milatovic, D., Gupta, R. C., Yu, Y., Zaja-Milatovic, S., and Aschner, M. (2011). Protective effects of antioxidants and anti-inflammatory agents against manganese-induced oxidative damage and neuronal injury. Toxicol. Appl. Pharmacol. 256 (3), 219–226. doi:10.1016/j.taap.2011.06.001
Mora, M., Bonilla, E., Medina-Leendertz, S., Bravo, Y., and Arcaya, J. L. (2014). Minocycline increases the activity of superoxide dismutase and reduces the concentration of nitric oxide, hydrogen peroxide and mitochondrial malondialdehyde in manganese treated Drosophila melanogaster. Neurochem. Res. 39 (7), 1270–1278. doi:10.1007/s11064-014-1309-z
Morcillo, P., Cordero, H., Ijomone, O. M., Ayodele, A., Bornhorst, J., Gunther, L., et al. (2021). Defective mitochondrial dynamics underlie manganese-induced neurotoxicity. Mol. Neurobiol. 58 (7), 3270–3289. doi:10.1007/s12035-021-02341-w
Moreno, J. A., Sullivan, K. A., Carbone, D. L., Hanneman, W. H., and Tjalkens, R. B. (2008). Manganese potentiates nuclear factor-kappaB-dependent expression of nitric oxide synthase 2 in astrocytes by activating soluble guanylate cyclase and extracellular responsive kinase signaling pathways. J. Neurosci. Res. 86 (9), 2028–2038. doi:10.1002/jnr.21640
Mutkus, L., Aschner, J. L., Fitsanakis, V., and Aschner, M. (2005). The in vitro uptake of glutamate in GLAST and GLT-1 transfected mutant CHO-K1 cells is inhibited by manganese. Biol. Trace Elem. Res. 107 (3), 221–230. doi:10.1385/BTER:107:3:221
Nadeem, R. I., Ahmed, H. I., and El-Sayeh, B. M. (2018). Protective effect of vinpocetine against neurotoxicity of manganese in adult male rats. Naunyn. Schmiedeb. Arch. Pharmacol. 391 (7), 729–742. doi:10.1007/s00210-018-1498-0
Nkpaa, K. W., Onyeso, G. I., and Kponee, K. Z. (2019). Rutin abrogates manganese-Induced striatal and hippocampal toxicity via inhibition of iron depletion, oxidative stress, inflammation and suppressing the NF-κB signaling pathway. J. Trace Elem. Med. Biol. 53, 8–15. doi:10.1016/j.jtemb.2019.01.014
Normandin, L., and Hazell, A. S. (2002). Manganese neurotoxicity: An update of pathophysiologic mechanisms. Metab. Brain Dis. 17 (4), 375–387. doi:10.1023/a:1021970120965
Olanow, C. W. (2004). Manganese-induced parkinsonism and Parkinson's disease. Ann. N. Y. Acad. Sci. 1012, 209–223. doi:10.1196/annals.1306.018
Ommati, M. M., Heidari, R., Ghanbarinejad, V., Abdoli, N., and Niknahad, H. (2019). Taurine treatment provides neuroprotection in a mouse model of manganism. Biol. Trace Elem. Res. 190 (2), 384–395. doi:10.1007/s12011-018-1552-2
Ommati, M. M., Heidari, R., Ghanbarinejad, V., Aminian, A., Abdoli, N., and Niknahad, H. (2020). The neuroprotective properties of carnosine in a mouse model of manganism is mediated via mitochondria regulating and antioxidative mechanisms. Nutr. Neurosci. 23 (9), 731–743. doi:10.1080/1028415X.2018.1552399
Orian, L., and Toppo, S. (2014). Organochalcogen peroxidase mimetics as potential drugs: A long story of a promise still unfulfilled. Free Radic. Biol. Med. 66, 65–74. doi:10.1016/j.freeradbiomed.2013.03.006
Ou, C. Y., Huang, M. l., Jiang, Y. m., Luo, H. l., Deng, X. f., Wang, C., et al. (2011). Effect of sodium para-aminosalicylic on concentrations of amino acid neurotransmitters in basal ganglia of manganese-exposed rats. Zhonghua Yu Fang. Yi Xue Za Zhi 45 (5), 422–425.
Oubrahim, H., Stadtman, E. R., and Chock, P. B. (2001). Mitochondria play no roles in Mn(II)-induced apoptosis in HeLa cells. Proc. Natl. Acad. Sci. U. S. A. 98 (17), 9505–9510. doi:10.1073/pnas.181319898
Pajarillo, E., Demayo, M., Digman, A., Nyarko-Danquah, I., Son, D. S., Aschner, M., et al. (2022). Deletion of RE1-silencing transcription factor in striatal astrocytes exacerbates manganese-induced neurotoxicity in mice. Glia 70, 1886–1901. doi:10.1002/glia.24226
Pajarillo, E., Johnson, J., Kim, J., Karki, P., Son, D. S., Aschner, M., et al. (2018). 17β-estradiol and tamoxifen protect mice from manganese-induced dopaminergic neurotoxicity. Neurotoxicology 65, 280–288. doi:10.1016/j.neuro.2017.11.008
Pajarillo, E., Johnson, J., Rizor, A., Nyarko-Danquah, I., Adinew, G., Bornhorst, J., et al. (2020). Astrocyte-specific deletion of the transcription factor Yin Yang 1 in murine substantia nigra mitigates manganese-induced dopaminergic neurotoxicity. J. Biol. Chem. 295 (46), 15662–15676. doi:10.1074/jbc.RA120.015552
Pajarillo, E., Nyarko-Danquah, I., Adinew, G., Rizor, A., Aschner, M., and Lee, E. (2021). Neurotoxicity mechanisms of manganese in the central nervous system. Adv. Neurotoxicol. 5, 215–238. doi:10.1016/bs.ant.2020.11.003
Pajarillo, E., Digman, A., Nyarko-Danquah, I., Son, D. S., Soliman, K. F. A., Aschner, M., et al. (2021). Astrocytic transcription factor REST upregulates glutamate transporter EAAT2, protecting dopaminergic neurons from manganese-induced excitotoxicity. J. Biol. Chem. 297 (6), 101372. doi:10.1016/j.jbc.2021.101372
Pajarillo, E., Nyarko-Danquah, I., Digman, A., Vied, C., Son, D. S., Lee, J., et al. (2022). Astrocytic Yin Yang 1 is critical for murine brain development and protection against apoptosis, oxidative stress, and inflammation. Glia. doi:10.1002/glia.24286
Pajarillo, E., Rizor, A., Lee, J., Aschner, M., and Lee, E. (2019). The role of astrocytic glutamate transporters GLT-1 and GLAST in neurological disorders: Potential targets for neurotherapeutics. Neuropharmacology 161, 107559. doi:10.1016/j.neuropharm.2019.03.002
Pajarillo, E., Rizor, A., Son, D. S., Aschner, M., and Lee, E. (2020). The transcription factor REST up-regulates tyrosine hydroxylase and antiapoptotic genes and protects dopaminergic neurons against manganese toxicity. J. Biol. Chem. 295 (10), 3040–3054. doi:10.1074/jbc.RA119.011446
Park, E., and Chun, H. S. (2017). Melatonin attenuates manganese and lipopolysaccharide-induced inflammatory activation of BV2 microglia. Neurochem. Res. 42 (2), 656–666. doi:10.1007/s11064-016-2122-7
Park, E., and Chun, H. S. (2017). Protective effects of curcumin on manganese-induced BV-2 microglial cell death. Biol. Pharm. Bull. 40 (8), 1275–1281. doi:10.1248/bpb.b17-00160
Peng, D., Li, J., Deng, Y., Zhu, X., Zhao, L., Zhang, Y., et al. (2020). Sodium para-aminosalicylic acid inhibits manganese-induced NLRP3 inflammasome-dependent pyroptosis by inhibiting NF-κB pathway activation and oxidative stress. J. Neuroinflammation 17 (1), 343. doi:10.1186/s12974-020-02018-6
Pfeffer, S. R. (2010). Two Rabs for exosome release. Nat. Cell Biol. 12 (1), 3–4. doi:10.1038/ncb0110-3
Ponzoni, S. (2012). Macrophages-mediated neurotoxic effects of intra-nigral manganese administration are attenuated by minocycline. Neurosci. Lett. 506 (1), 136–140. doi:10.1016/j.neulet.2011.10.066
Porte Alcon, S., Gorojod, R. M., and Kotler, M. L. (2020). Kinetic and protective role of autophagy in manganese-exposed BV-2 cells. Biochim. Biophys. Acta. Mol. Cell Res. 1867 (10), 118787. doi:10.1016/j.bbamcr.2020.118787
Prabhakaran, K., Ghosh, D., Chapman, G. D., and Gunasekar, P. G. (2008). Molecular mechanism of manganese exposure-induced dopaminergic toxicity. Brain Res. Bull. 76 (4), 361–367. doi:10.1016/j.brainresbull.2008.03.004
Pu, B., Xue, Y., Wang, Q., Hua, C., and Li, X. (2015). Dextromethorphan provides neuroprotection via anti-inflammatory and anti-excitotoxicity effects in the cortex following traumatic brain injury. Mol. Med. Rep. 12 (3), 3704–3710. doi:10.3892/mmr.2015.3830
Qi, Z., Yang, X., Sang, Y., Liu, Y., Li, J., Xu, B., et al. (2020). Fluoxetine and riluzole mitigates manganese-induced disruption of glutamate transporters and excitotoxicity via ephrin-A3/GLAST-GLT-1/glu signaling pathway in striatum of mice. Neurotox. Res. 38 (2), 508–523. doi:10.1007/s12640-020-00209-w
Racette, B. A., Nelson, G., Dlamini, W. W., Hershey, T., Prathibha, P., Turner, J. R., et al. (2021). Depression and anxiety in a manganese-exposed community. Neurotoxicology 85, 222–233. doi:10.1016/j.neuro.2021.05.017
Racette, B. A., Nelson, G., Dlamini, W. W., Prathibha, P., Turner, J. R., Ushe, M., et al. (2021). Severity of parkinsonism associated with environmental manganese exposure. Environ. Health. 20 (1), 27. doi:10.1186/s12940-021-00712-3
Rao, K. V., Jayakumar, A. R., Reddy, P. V. B., Tong, X., Curtis, K. M., and Norenberg, M. D. (2010). Aquaporin-4 in manganese-treated cultured astrocytes. Glia 58 (12), 1490–1499. doi:10.1002/glia.21023
Reaney, S. H., and Smith, D. R. (2005). Manganese oxidation state mediates toxicity in PC12 cells. Toxicol. Appl. Pharmacol. 205 (3), 271–281. doi:10.1016/j.taap.2004.10.013
Rizor, A., Pajarillo, E., Son, D. S., Aschner, M., and Lee, E. (2022). Manganese phosphorylates Yin Yang 1 at serine residues to repress EAAT2 in human H4 astrocytes. Toxicol. Lett. 355, 41–46. doi:10.1016/j.toxlet.2021.11.007
Roberts, R. F., Tang, M. Y., Fon, E. A., and Durcan, T. M. (2016). Defending the mitochondria: The pathways of mitophagy and mitochondrial-derived vesicles. Int. J. Biochem. Cell Biol. 79, 427–436. doi:10.1016/j.biocel.2016.07.020
Roth, J. A., Singleton, S., Feng, J., Garrick, M., and Paradkar, P. N. (2010). Parkin regulates metal transport via proteasomal degradation of the 1B isoforms of divalent metal transporter 1. J. Neurochem. 113 (2), 454–464. doi:10.1111/j.1471-4159.2010.06607.x
Rylski, M., Amborska, R., Zybura, K., Konopacki, F. A., Wilczynski, G. M., and Kaczmarek, L. (2008). Yin Yang 1 expression in the adult rodent brain. Neurochem. Res. 33 (12), 2556–2564. doi:10.1007/s11064-008-9757-y
Santos, A. P., Lucas, R. L., Andrade, V., Mateus, M. L., Milatovic, D., Aschner, M., et al. (2012). Protective effects of ebselen (Ebs) and para-aminosalicylic acid (PAS) against manganese (Mn)-induced neurotoxicity. Toxicol. Appl. Pharmacol. 258 (3), 394–402. doi:10.1016/j.taap.2011.12.003
Sarkar, S., Malovic, E., Harischandra, D. S., Ngwa, H. A., Ghosh, A., Hogan, C., et al. (2018). Manganese exposure induces neuroinflammation by impairing mitochondrial dynamics in astrocytes. Neurotoxicology 64, 204–218. doi:10.1016/j.neuro.2017.05.009
Schoenherr, C. J., and Anderson, D. J. (1995). The neuron-restrictive silencer factor (NRSF): A coordinate repressor of multiple neuron-specific genes. Science 267 (5202), 1360–1363. doi:10.1126/science.7871435
Scholte, H. R. (1988). The biochemical basis of mitochondrial diseases. J. Bioenerg. Biomembr. 20 (2), 161–191. doi:10.1007/BF00768393
Seirafi, M., Kozlov, G., and Gehring, K. (2015). Parkin structure and function. FEBS J. 282 (11), 2076–2088. doi:10.1111/febs.13249
Settembre, C., Di Malta, C., Polito, V. A., Garcia Arencibia, M., Vetrini, F., Erdin, S., et al. (2011). TFEB links autophagy to lysosomal biogenesis. Science 332 (6036), 1429–1433. doi:10.1126/science.1204592
Settembre, C., Zoncu, R., Medina, D. L., Vetrini, F., Erdin, S., Erdin, S., et al. (2012). A lysosome-to-nucleus signalling mechanism senses and regulates the lysosome via mTOR and TFEB. EMBO J. 31 (5), 1095–1108. doi:10.1038/emboj.2012.32
Shefa, U., Jeong, N. Y., Song, I. O., Chung, H. J., Kim, D., Jung, J., et al. (2019). Mitophagy links oxidative stress conditions and neurodegenerative diseases. Neural Regen. Res. 14 (5), 749–756. doi:10.4103/1673-5374.249218
Shi, S., Zhao, J., Yang, L., Nie, X., Han, J., Ma, X., et al. (2015). KHSRP participates in manganese-induced neurotoxicity in rat striatum and PC12 cells. J. Mol. Neurosci. 55 (2), 454–465. doi:10.1007/s12031-014-0367-7
Soares, A. T. G., Silva, A. d. C., Tinkov, A. A., Khan, H., Santamaria, A., Skalnaya, M. G., et al. (2020). The impact of manganese on neurotransmitter systems. J. Trace Elem. Med. Biol. 61, 126554. doi:10.1016/j.jtemb.2020.126554
Spadoni, F., StefAni, A., MorelloM., , LavaroniF., , Giacomini, P., and Sancesario, G. (2000). Selective vulnerability of pallidal neurons in the early phases of manganese intoxication. Exp. Brain Res. 135 (4), 544–551. doi:10.1007/s002210000554
Springer, W., and Kahle, P. J. (2011). Regulation of PINK1-Parkin-mediated mitophagy. Autophagy 7 (3), 266–278. doi:10.4161/auto.7.3.14348
Sriram, K., Lin, G. X., Jefferson, A. M., Roberts, J. R., Wirth, O., Hayashi, Y., et al. (2010). Mitochondrial dysfunction and loss of Parkinson's disease-linked proteins contribute to neurotoxicity of manganese-containing welding fumes. FASEB J. 24 (12), 4989–5002. doi:10.1096/fj.10-163964
Stephenson, A. P., Schneider, J. A., Nelson, B. C., Atha, D. H., Jain, A., Soliman, K. F. A., et al. (2013). Manganese-induced oxidative DNA damage in neuronal SH-SY5Y cells: Attenuation of thymine base lesions by glutathione and N-acetylcysteine. Toxicol. Lett. 218 (3), 299–307. doi:10.1016/j.toxlet.2012.12.024
Sun, Q., Kang, R. R., Chen, K. G., Liu, K., Ma, Z., Liu, C., et al. (2021). Sirtuin 3 is required for the protective effect of Resveratrol on Manganese-induced disruption of mitochondrial biogenesis in primary cultured neurons. J. Neurochem. 156 (1), 121–135. doi:10.1111/jnc.15095
Szpetnar, M., Luchowska-Kocot, D., Boguszewska-Czubara, A., and Kurzepa, J. (2016). The influence of manganese and glutamine intake on antioxidants and neurotransmitter amino acids levels in rats' brain. Neurochem. Res. 41 (8), 2129–2139. doi:10.1007/s11064-016-1928-7
Tan, Y., Cheng, H., Su, C., Chen, P., and Yang, X. (2022). PI3K/Akt signaling pathway ameliorates oxidative stress-induced apoptosis upon manganese exposure in PC12 cells. Biol. Trace Elem. Res. 200 (2), 749–760. doi:10.1007/s12011-021-02687-1
Tarale, P., Chakrabarti, T., Sivanesan, S., Naoghare, P., Bafana, A., and Krishnamurthi, K. (2016). Potential role of epigenetic mechanism in manganese induced neurotoxicity. Biomed. Res. Int. 2016, 2548792. doi:10.1155/2016/2548792
Tarale, P., Daiwile, A. P., Sivanesan, S., Stoger, R., Bafana, A., Naoghare, P. K., et al. (2018). Manganese exposure: Linking down-regulation of miRNA-7 and miRNA-433 with alpha-synuclein overexpression and risk of idiopathic Parkinson's disease. Toxicol. Vitro 46, 94–101. doi:10.1016/j.tiv.2017.10.003
Tarale, P., Sivanesan, S., Daiwile, A. P., Stoger, R., Bafana, A., Naoghare, P. K., et al. (2017). Global DNA methylation profiling of manganese-exposed human neuroblastoma SH-SY5Y cells reveals epigenetic alterations in Parkinson's disease-associated genes. Arch. Toxicol. 91 (7), 2629–2641. doi:10.1007/s00204-016-1899-0
Tateishi, N., Mori, T., Kagamiishi, Y., Satoh, S., Katsube, N., Morikawa, E., et al. (2002). Astrocytic activation and delayed infarct expansion after permanent focal ischemia in rats. Part II: Suppression of astrocytic activation by a novel agent (R)-(-)-2-propyloctanoic acid (ONO-2506) leads to mitigation of delayed infarct expansion and early improvement of neurologic deficits. J. Cereb. Blood Flow. Metab. 22 (6), 723–734. doi:10.1097/00004647-200206000-00011
Thomas, D. M., and Kuhn, D. M. (2005). MK-801 and dextromethorphan block microglial activation and protect against methamphetamine-induced neurotoxicity. Brain Res. 1050 (1-2), 190–198. doi:10.1016/j.brainres.2005.05.049
Tinkov, A. A., Paoliello, M. M. B., Mazilina, A. N., Skalny, A. V., Martins, A. C., Voskresenskaya, O. N., et al. (2021). Molecular targets of manganese-induced neurotoxicity: A five-year update. Int. J. Mol. Sci. 22 (9), 4646. doi:10.3390/ijms22094646
Tonelli, C., Chio, I. I. C., and Tuveson, D. A. (2018). Transcriptional regulation by Nrf2. Antioxid. Redox Signal. 29 (17), 1727–1745. doi:10.1089/ars.2017.7342
Tuschl, K., Meyer, E., Valdivia, L. E., Zhao, N., Dadswell, C., Abdul-Sada, A., et al. (2016). Mutations in SLC39A14 disrupt manganese homeostasis and cause childhood-onset parkinsonism-dystonia. Nat. Commun. 7, 11601. doi:10.1038/ncomms11601
Verina, T., Kiihl, S. F., Schneider, J. S., and Guilarte, T. R. (2011). Manganese exposure induces microglia activation and dystrophy in the substantia nigra of non-human primates. Neurotoxicology 32 (2), 215–226. doi:10.1016/j.neuro.2010.11.003
Verina, T., Schneider, J. S., and Guilarte, T. R. (2013). Manganese exposure induces alpha-synuclein aggregation in the frontal cortex of non-human primates. Toxicol. Lett. 217 (3), 177–183. doi:10.1016/j.toxlet.2012.12.006
Wang, D., Zhang, J., Jiang, W., Cao, Z., Zhao, F., Cai, T., et al. (2017). The role of NLRP3-CASP1 in inflammasome-mediated neuroinflammation and autophagy dysfunction in manganese-induced, hippocampal-dependent impairment of learning and memory ability. Autophagy 13 (5), 914–927. doi:10.1080/15548627.2017.1293766
Wedler, F. C., and Denman, R. B. (1984). Glutamine synthetase: The major Mn(II) enzyme in mammalian brain. Curr. Top. Cell. Regul. 24, 153–169. doi:10.1016/b978-0-12-152824-9.50021-6
Xia, C., Tao, Y., Li, M., Che, T., and Qu, J. (2020). Protein acetylation and deacetylation: An important regulatory modification in gene transcription (Review). Exp. Ther. Med. 20 (4), 2923–2940. doi:10.3892/etm.2020.9073
Xu, B., Wu, S. W., Lu, C. W., Deng, Y., Liu, W., Wei, Y. G., et al. (2013). Oxidative stress involvement in manganese-induced alpha-synuclein oligomerization in organotypic brain slice cultures. Toxicology 305, 71–78. doi:10.1016/j.tox.2013.01.006
Xu, B., Xu, Z. F., and Deng, Y. (2010). Manganese exposure alters the expression of N-methyl-D-aspartate receptor subunit mRNAs and proteins in rat striatum. J. Biochem. Mol. Toxicol. 24 (1), 1–9. doi:10.1002/jbt.20306
Xu, Z., Jia, K., Xu, B., Li, J., Deng, Y., et al. (2010). Effects of MK-801, taurine and dextromethorphan on neurotoxicity caused by manganese in rats. Toxicol. Ind. Health 26 (1), 55–60. doi:10.1177/0748233709359275
Yagyu, K., Hasegawa, Y., Sato, M., Oh-Hashi, K., and Hirata, Y. (2020). Activation of protein kinase R in the manganese-induced apoptosis of PC12 cells. Toxicology 442, 152526. doi:10.1016/j.tox.2020.152526
Yan, D., Gao, L., Lang, J., Gao, X., and Ma, H. (2021). Effects of manganese on microglia M1/M2 polarization and SIRT1-mediated transcription of STAT3-dependent genes in mouse. Environ. Toxicol. 36 (9), 1729–1741. doi:10.1002/tox.23294
Yan, D., Ma, Z., Liu, C., Wang, C., Deng, Y., Liu, W., et al. (2019). Corynoxine B ameliorates HMGB1-dependent autophagy dysfunction during manganese exposure in SH-SY5Y human neuroblastoma cells. Food Chem. Toxicol. 124, 336–348. doi:10.1016/j.fct.2018.12.027
Yang, N., Wei, Y., Wang, T., Guo, J., Sun, Q., Hu, Y., et al. (2016). Genome-wide analysis of DNA methylation during antagonism of DMOG to MnCl2-induced cytotoxicity in the mouse substantia nigra. Sci. Rep. 6, 28933. doi:10.1038/srep28933
Yang, X., Yang, H., Wu, F., Qi, Z., Li, J., Xu, B., et al. (2018). Mn inhibits GSH synthesis via downregulation of neuronal EAAC1 and astrocytic xCT to cause oxidative damage in the striatum of mice. Oxid. Med. Cell. Longev. 2018, 4235695. doi:10.1155/2018/4235695
Yang, Y., Liu, Y., Zhang, A. L., Tang, S. F., Ming, Q., Ao, C. Y., et al. (2022). Curcumin protects against manganese-induced neurotoxicity in rat by regulating oxidative stress-related gene expression via H3K27 acetylation. Ecotoxicol. Environ. Saf. 236, 113469. doi:10.1016/j.ecoenv.2022.113469
Zeng, Q., Siu, W., Li, L., Jin, Y., Liang, S., Cao, M., et al. (2019). Autophagy in Alzheimer's disease and promising modulatory effects of herbal medicine. Exp. Gerontol. 119, 100–110. doi:10.1016/j.exger.2019.01.027
Zhang, H. T., Mi, L., Wang, T., Yuan, L., Li, X. H., Dong, L. S., et al. (2016). PINK1/Parkin-mediated mitophagy play a protective role in manganese induced apoptosis in SH-SY5Y cells. Toxicol. Vitro 34, 212–219. doi:10.1016/j.tiv.2016.04.006
Zhang, J., Cao, R., Cai, T., Aschner, M., Zhao, F., Yao, T., et al. (2013). The role of autophagy dysregulation in manganese-induced dopaminergic neurodegeneration. Neurotox. Res. 24 (4), 478–490. doi:10.1007/s12640-013-9392-5
Zhang, J., Yang, Y., Yang, X., Qin, J., Wei, X., Peng, Y., et al. (2021). Influence of manganese exposure on cognitive function, plasma APP and Aβ levels in older men. J. Trace Elem. Med. Biol. 67, 126788. doi:10.1016/j.jtemb.2021.126788
Zhang, Z., Guo, Z., Zhan, Y., Li, H., and Wu, S. (2017). Role of histone acetylation in activation of nuclear factor erythroid 2-related factor 2/heme oxygenase 1 pathway by manganese chloride. Toxicol. Appl. Pharmacol. 336, 94–100. doi:10.1016/j.taap.2017.10.011
Zhang, Z., Yan, J., Bowman, A. B., Bryan, M. R., Singh, R., and Aschner, M. (2020). Dysregulation of TFEB contributes to manganese-induced autophagic failure and mitochondrial dysfunction in astrocytes. Autophagy 16 (8), 1506–1523. doi:10.1080/15548627.2019.1688488
Zhao, F., Cai, T., Liu, M., Zheng, G., Luo, W., and Chen, J. (2009). Manganese induces dopaminergic neurodegeneration via microglial activation in a rat model of manganism. Toxicol. Sci. 107 (1), 156–164. doi:10.1093/toxsci/kfn213
Zhao, X., Liu, Y., Zhu, G., Liang, Y., Liu, B., Wu, Y., et al. (2019). SIRT1 downregulation mediated Manganese-induced neuronal apoptosis through activation of FOXO3a-Bim/PUMA axis. Sci. Total Environ. 646, 1047–1055. doi:10.1016/j.scitotenv.2018.07.363
Zheng, W., Jiang, Y. M., Zhang, Y., Jiang, W., Wang, X., and Cowan, D. M. (2009). Chelation therapy of manganese intoxication with para-aminosalicylic acid (PAS) in Sprague-Dawley rats. Neurotoxicology 30 (2), 240–248. doi:10.1016/j.neuro.2008.12.007
Zhou, Q., Fu, X., Wang, X., Wu, Q., Lu, Y., Shi, J., et al. (2018). Autophagy plays a protective role in Mn-induced toxicity in PC12 cells. Toxicology 394, 45–53. doi:10.1016/j.tox.2017.12.001
Zhu, Z., Liu, L. F., Su, C. F., Liu, J., Tong, B. C. K., Iyaswamy, A., et al. (2022). Corynoxine B derivative CB6 prevents Parkinsonian toxicity in mice by inducing PIK3C3 complex-dependent autophagy. Acta Pharmacol. Sin. 43, 2511–2526. doi:10.1038/s41401-022-00871-0
Glossary
E2 17β-estradiol
AD Alzheimer’s disease
ARE antioxidant response element
COR carboxy-terminal of ras
CAT Catalase
DM dextromethorphan
DPTVP diethyl-2-phenyl-2-telluropheny vinyl phosphonate
Drp-1 dynamin-related protein 1
EDTA ethylenediaminetetraacetic acid
EAAT excitatory amino acid transporter
GLT-1 glutamate transporter 1
GLAST glutamate aspartate transporter
GSH glutathione
GPx glutathione peroxidase
GSSG glutathione disulfide
HO-1 heme oxygenase 1
HDACi histone deacetylase inhibitors
HRE hypoxia response elements
HIF1α hypoxia-inducible factor 1α
IL interleukin
KHSRP K-homology splicing regulator protein
LRRK leucine rich repeat kinase
Mn manganese
Mfn2 mitofusin 2
NOS2 nitric oxide synthase 2
NMDA N-methyl-D-aspartate
NMDAR NMDA receptor
NSAID non-steroidal anti-inflammatory drug
NF-κB nuclear factor kappa-light-chain-enhancer of activated B cells
Opa-1 optic atrophy type 1
PAS para-aminosalicylic acid
PD Parkinson’s disease
PPAR-γ peroxisome proliferator-activated receptor gamma
PINK1 phosphatase and tensin homolog induced kinase 1
RX raloxifene
REST RE1-silencing transcription factor
ROS reactive oxygen species
RE1 repressor element 1
SERMs selective estrogen receptor modulators
NaB sodium butyrate
SOD superoxide dismutase
TX tamoxifen
TNF-α tumour necrosis factor α
TFs transcription factors
TFEB transcription factor EB
TH tyrosine hydroxylase
VPA valproic acid
Wip 1 wild type p53-induced phosphatase 1
YY1 Yin Yang 1
αSyn α-Synuclein
Keywords: manganese, oxidative stress, inflammation, autophagy, mitophagy, excitotoxicity, apoptosis, neurotherapeutics
Citation: Pajarillo E, Nyarko-Danquah I, Digman A, Multani HK, Kim S, Gaspard P, Aschner M and Lee E (2022) Mechanisms of manganese-induced neurotoxicity and the pursuit of neurotherapeutic strategies. Front. Pharmacol. 13:1011947. doi: 10.3389/fphar.2022.1011947
Received: 04 August 2022; Accepted: 01 December 2022;
Published: 20 December 2022.
Edited by:
Amit Bafana, National Environmental Engineering Research Institute (CSIR), IndiaReviewed by:
Shi Chen, Hunan Normal University, ChinaSerena Barral, University College London, United Kingdom
Atul Daiwile, National Institutes of Health (NIH), United States
Copyright © 2022 Pajarillo, Nyarko-Danquah, Digman, Multani, Kim, Gaspard, Aschner and Lee. This is an open-access article distributed under the terms of the Creative Commons Attribution License (CC BY). The use, distribution or reproduction in other forums is permitted, provided the original author(s) and the copyright owner(s) are credited and that the original publication in this journal is cited, in accordance with accepted academic practice. No use, distribution or reproduction is permitted which does not comply with these terms.
*Correspondence: Eunsook Lee, ZXVuc29vay5sZWVAZmFtdS5lZHU=