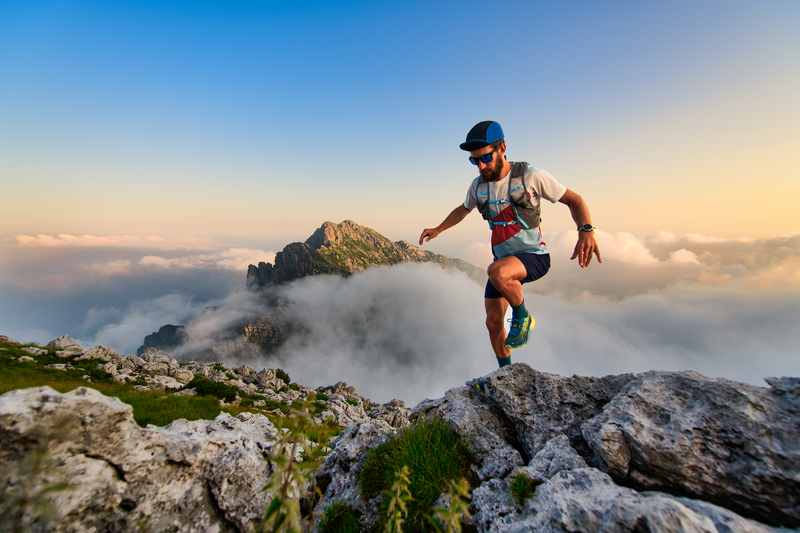
95% of researchers rate our articles as excellent or good
Learn more about the work of our research integrity team to safeguard the quality of each article we publish.
Find out more
ORIGINAL RESEARCH article
Front. Pharmacol. , 13 December 2022
Sec. Ethnopharmacology
Volume 13 - 2022 | https://doi.org/10.3389/fphar.2022.1004568
This article is part of the Research Topic Natural Products for Neuroprotection and Neuroregeneration View all 9 articles
Sea cucumbers are marine organism that have long been used for food and traditional medicine in Asian countries. Recently, we have shown that ethyl acetate fraction (HLEA) of the crude extract of the black sea cucumber, Holothuria leucospilota, could alleviate Parkinsonism in Caenorhabditis elegans PD models. In this study, we found that the effective neuroprotective activity is attributed to HLEA-P1 compound chemically isolated and identified in H. leucospilota ethyl acetate. We reported here that HLEA-P1 could attenuate DAergic neurodegeneration, improve DAergic-dependent behaviors, reduce oxidative stress in 6-OHDA-induced C. elegans. In addition, HLEA-P1 reduced α-synuclein aggregation, improved behavior deficit and recovered lipid deposition in transgenic C. elegans overexpressing α-synuclein. We also found that HLEA-P1 activates nuclear localization of DAF-16 transcription factor of insulin/IGF-1 signaling (IIS) pathway. Treatment with 25 μg/ml of HLEA-P1 upregulated transcriptional activity of DAF-16 target genes including anti-oxidant genes (such as sod-3) and small heat shock proteins (such as hsp16.1, hsp16.2, and hsp12.6) in 6-OHDA-induced worms. In α-synuclein-overexpressed C. elegans strain, treatment with 5 μg/ml of HLEA-P1 significantly activated mRNA expression of sod-3 and hsp16.2. Chemical analysis demonstrated that HLEA-P1 compound is decanoic acid/capric acid. Taken together, our findings revealed that decanoic acid isolated from H. leucospilota exerts anti-Parkinson effect in C. elegans PD models by partly modulating IIS/DAF-16 pathway.
Parkinson’s disease (PD) is a chronic neurodegenerative disease characterized by the selective degeneration of the dopaminergic (DAergic) neurons in substantia nigra pars compacta (SNc) and the aggregation of the misfolded α-synuclein protein within Lewy body. The degeneration of DAergic neurons contributes to the depletion of the neurotransmitter, dopamine, in the striatal neurons, resulting in motor impairment (Maiti et al., 2017). The etiologic causes of PD are genetic mutations, environmental toxins, and aging (Maiti et al., 2017). Although the precise molecular pathogenesis behinds PD remains to be elucidated, oxidative stress has been proposed as the major pathogenic event causing PD (Moore et al., 2005). Based on the Global Burden of Disease study, PD is considered as a neurological disorder with the fastest growing prevalence (Feigin et al., 2017). Unfortunately, this disease is still incurable. Currently, the conventional treatments are based on the symptomatic relief (Maiti et al., 2017). In this regard, exploring therapeutic approaches that can slow down or stop the degenerative process due to PD has become an urgent issue.
Recently, bioactive compounds derived from marine organisms have gained attention in the field of drug discovery for neurodegenerative diseases including PD (Huang et al., 2019). Sea cucumbers are among marine animals with high economic, food, and medicinal values, especially in Asian countries (Mashjoor et al., 2018). From a nutritional perspective, the sea cucumbers contain several bioactive ingredients such as peptides, triterpene glycosides, fucoidan, and essential fatty acids (Xu et al., 2018). In this regard, a number of biological and therapeutics properties including anti-oxidation, anti-inflammation, anti-cancer, as well as anti-neurodegeneration have been ascribed to substances obtained from various species of sea cucumbers (Xu et al., 2018). In particular to PD, crude extracts and bioactive compounds from sea cucumbers have been shown to protect neurotoxin-induced DAergic neurodegenerations in several studies. For example, ethyl acetate and butanol fractions of the crude extract of Holothuria scabra (H. scabra) and their purified compounds, diterpene glycosides, could significantly reduce DAergic neurodegeneration and inhibit abnormal aggregation of α-synuclein in Caenorhabditis elegans (C. elegans) PD models (Chalorak et al., 2018; Chalorak et al., 2021). In addition to H. scabra, the black sea cucumber, H. leucospilota, is found to be predominant throughout the Indo-Pacific region including Thailand, and it is one of commercial species in Southeast Asia (Choo et al., 2008). Similar to other sea cucumber species, H. leucospilota also contains several bioactive compounds such as phenols, terpenoids, flavonoid, saponin, glycoside, steroids, essential fatty acids (Ceesay et al., 2019). To ascertain nutritional property and enhance economic value of H. leucospilota, the neuroprotective effects of the extracts from this species were investigated. Recent study from our group demonstrated that ethyl acetate fraction of the crude extract of H. leucospilota (HLEA) mediates anti-Parkinson’s activity by attenuating DAergic neurodegeneration and inhibiting α-synuclein aggregation in the C. elegans PD models (Malaiwong et al., 2019). However, the specific compounds with anti-PD property in such fraction has not yet been elucidated. Thus, in the present study we identified and isolated the active compounds from HLEA, and evaluate their anti-PD effects as well as investigate possible mechanisms of action of these compounds. Hopefully, the obtained data would substantiate the nutraceutical value of H. leucospilota bioactive compounds and establish them as potential candidate pharmaceuticals for anti-PD.
To investigate the anti-PD activity of H. leucospilota compounds, C. elegans was used as a model organism. C. elegans provides several advantages for being used as an animal model including its short life cycle and high reproductive rate. Besides, C. elegans has 8 DAergic neurons in its nervous system (Harrington et al., 2010), that could be selectively induced to undergo neurodegeneration by the neurotoxin such as 6-hydroxydopamine (6-OHDA) (Nass et al., 2002). Moreover, the transgenic C. elegans NL5901 expressing human α-synuclein has been created for studying the effects and mechanisms of α-synucleinopathies (Bodhicharla et al., 2012). Therefore, our study aimed to investigate the neuroprotective effects and underlying mechanism of the isolated compounds from HLEA against PD using C. elegans as a model.
The black sea cucumber H. leucospilota were collected from Prachuap Khiri Khan province, and its species were identified by experts from Prachuap Khiri Khan Coastal Fisheries Research and Development Center, Thailand. The collected samples were then cleaned by distilled water and anesthetized by cooling in ice. The body walls of H. leucospilota were dissected, cut into small pieces, and stored at −80°C. Then, the samples were freeze-dried using FreezeDry Supermodulyo-230. The freeze-dried H. leucospilota samples (1.2 kg) were ground to powder and macerated with n-hexane. After evaporation of the solvent and reduction of pressure, n-hexane fraction (HLHE, 3.2 g) and residue were obtained. The acquired residue was then extracted by ethyl acetate (EtOAc) to obtain HLEA fraction (3.5 g). The HLEA fraction was subsequently subjected to chemical isolation and identification. The procedures for handling the sea cucumbers were ethically approved by Mahidol University-Institute Animal Care and Use Committee (MU-IACUC; MUSC60-049-399).
In the isolation process, the HLEA fraction was purified sequentially by solvent partition, silica-gel column chromatography (CC) and Thin Layer Chromatography (TLC). In this study, CC was carried out using Merck silica gel 60 (finer than 0.063 mm) and Pharmacia Sephadex LH-20. For TLC, Merck precoated silica gel 60 F254 plates were used. Spots on TLC were detected under the UV light and by spraying with anisaldehyde-H2SO4 reagent followed by heating. The HLEA fraction (2.0 g) was fractionated by CC using Sephadex LH-20 condition MeOH 100%, and the eluates were examined by the TLC to finally obtain 3 subfractions (EA1-EA3). Then, 101 mg of fraction EA1 was subjected to CC using n-hexane-EtOAc (80:20) to afford compound 1 (HLEA-P1, 25.8 mg). 135 mg of Fraction EA2 was subjected to CC using n-hexane-EtOAc (80:20) to afford compound 2 (HLEA-P2, 20.2 mg) and compound 3 (HLEA-P3, 18.3 mg). 258 mg of Fraction EA3 was isolated by CC using n-hexane-EtOAc (70:30) to yield compound 4 (HLEA-P4, 20.5 mg), compound 5 (HLEA-P5, 15.1 mg) and compound 6 (HLEA-P6, 20.1 mg). Chemical structures of the derived compounds were analysed by 13C/1H- NMR. In this study, 1H and 13C NMR were recorded on a Bruker AVANCE 400 FT-NMR spectrometer operating at 400 (1H) and 100 (13C) MHz. The high resolution mass spectra were obtained using Bruker micrOTOF-QII mass spectrometer.
The C. elegans strain used in this study were: N2, Bristol (wild-type), BY250 strain (vtIs7; dat-1p::GFP), NL5901 strain [pkIs2386, unc-54p::α-synuclein::YFP + unc-119(+)], CF1553 strain [muIs84, (pAD76) sod-3p::GFP + rol-6(su1006)], CL2166 strain [dvIs19, (pAF15)gst-4p::GFP::NLS] and TJ356 strain [daf-16p::daf-16a/b::GFP + rol-6(su1006)]. All strains were provided by the Caenorhabditis Genetics Center (CGC), University of Minnesota, Minneapolis, United States), except BY250 which was obtained from Prof. Dr. Randy Blakely, Florida Atlantic University, United States. All strains were cultured in nematode growth medium (NGM) plate containing Escherichia coli (E. coli) strain OP50 as a food source and maintained in 20°C incubator. All C. elegans experiments were ethically performed under the guidelines of Faculty of Science, Mahidol University–Institutional Animal Care and Use Committee (MUSC–IACUC; MUSC60-048-398).
HLEA compounds were dissolved in dimethyl sulfoxide (DMSO). Stock solution of HLEA compounds at different doses were prepared and stored at −20°C. To deliver the HLEA compounds to C. elegans, HLEA substances were mixed with E. coli OP50 to obtain the final concentrations at 1, 5, 25, and 50 μg/ml in 1%DMSO (v/v).
To obtain the synchronous population of worms, the gravid adult worms were exposed with the bleaching solution (12% NaClO and 10% 1 M NaOH) with subsequent shaking on a mini-tube rotator for 10–12 min. Then, eggs were separated by centrifugation at 4,000 rpm for 90 s. To stop bleaching reaction, the supernatant was discarded, followed by washing the pellet three times by M9 buffer. Eggs were added with 1 ml of M9 buffer, transferred to NGM plate without OP50 and incubated overnight at 20°C to obtain newly hatched L1 larvae. For experiments, the synchronized L1 larvae were placed on the new NGM plates containing OP50 and incubated at 20°C and allowed to grow to L3 larvae.
In this study, a neurotoxin 6-OHDA was used for selectively destroying DAergic neurons of C. elegans, according to a previous method (Nass et al., 2002). Synchronized L3 larvae were incubated in a solution containing 50 mM 6-OHDA, 10 mM ascorbic acid and diluted OP50. The worms were exposed to the assay solution for 1 h at 22°C and gently stirred every 10 min. After 1 h of incubation, worms were washed three times by M9 buffer. The 6-OHDA-induced worms were transferred to NGM plates containing E. coli OP50 with 1, 5, 25, and 50 μg/ml of HLEA compounds for 72 h at 20°C. For untreated group, 6-OHDA-treated worms were incubated in NGM plates containing OP50 mixed with 1% DMSO (v/v). After 72 h of treatment, the worms were treated in various assays and observed as described below.
To evaluate the viability of DAergic neurons, BY250 (vtIs7; dat-1p::GFP) were used in this assay. After 72 h of treatment, the viability of DAergic neurons of BY250 was evaluated by measuring GFP fluorescence intensity of DAergic cephalic neurons (CEPs). Worms were washed three times by M9 buffer, transferred to 2% agarose pad on a glass slide. Then, worms were anesthetized with 30 mM sodium azide and finally covered with a coverslip. Imaging of head region of BY250 was taken using a fluorescence microscope (BX53; Olympus Corp., Tokyo, Japan) and fluorescence intensity of CEP neurons was quantified using ImageJ software (National Institute of Health, NIH, Bethesda, MD, United States).
The assay was performed to assess the function of DAergic neurons in C. elegans. In the presence of food, the well-fed worms move slower than the starved worms. This dopamine-dependent behavior is known as the basal slowing response (Sawin et al., 2000). After exposure to 6-OHDA and incubation with the extracts for 72 h, N2 wild-type worms were washed by M9 buffer to remove the remaining food in their bodies. Worms were then transferred to the NGM plates with or without OP50 bacteria. To avoid overstimulation of the sensing response, worms were settled for 5 min before recording with video camera for 20 s. Then, their body bendings were counted and the basal slowing rate was calculated as previously described (Malaiwong et al., 2019). The basal slowing rate = 100—locomotory rate (%), when the locomotory rate (%) = (rate of bending in the presence of bacteria/rate of bending in the absence of bacteria) ×100.
In C. elegans, DAergic neurons are responsible for ethanol avoidance behavior (Lee et al., 2009). The assay plates were prepared by dividing a 9 cm NGM plate into four quadrants with an inner circle with radius 0.5 cm around the center of the plate and then marked the top left (A) and bottom right quadrants (D) as control quadrants and the top right (B) and bottom left (C) quadrants as ethanol quadrants. In this assay, NGM with 2.5% agar was used to prevent the worms from digging inside the agar. To start the ethanol avoidance assay, 50 μl ethanol was added into two quadrants (B and C) of assay plate. The 6-OHDA-treated worms which were cultured with or without the compounds for 72 h were washed by M9 buffer. Next, 20 μl of the suspension with 50–100 worms was placed on the center of the assay plate with rapid removal of the buffer from the plate. The worms were then allowed to move for 30 min at 25°C. The number of worms in each quadrant were counted. The worms that do not cross the inner circle were not scored. Ethanol avoidance was calculated using the formula: Ethanol avoidance index = [(number of worms in control quadrants)—(number of worms in ethanol quadrants)]/total number of worms (Cooper et al., 2015).
To evaluate the level of α-synuclein aggregation, transgenic worms strain NL5901 [pkIs2386, unc-54p::α-synuclein::YFP + unc-119(+)] were used. In NL5901 strain, YFP tagged-human α-synuclein is expressed under the body wall muscle-specific promoter, allowing visualization of α-synuclein in the body wall muscle. Briefly, synchronized NL5901 L3 worms were transferred to NGM plates containing E. coli OP50 with different doses of HLEA-P1 for 72 h at 20°C. For untreated group, worms were incubated in NGM/OP50 containing only 1% (v/v) DMSO. After 72 h of treatment, worms were washed three times with M9 buffer and transferred to the 2% agarose pad on a glass slide for fluorescence imaging. Images of the whole bodies of NL5901 was taken by using a fluorescence microscope (BX53; Olympus Corp., Tokyo, Japan) and the YFP intensity of the aggregated α-synuclein was quantified using ImageJ software (National Institute of Health, NIH, Bethesda, MD, United States).
Aggregation of α-synuclein has been reported to associate with impaired thrashing behavior in C. elegans. Thrashing behavior can be assessed by counting the number of body bending in liquid media as described previously (Pujols et al., 2018). In this assay, wild-type N2 and NL5901 [pkIs2386, unc-54p::α-synuclein::YFP + unc-119(+)] were used. After incubation with HLEA-P1, adult worms at day 3 or day 5 were placed on clean NGM plates with M9 buffer. The worms were allowed to settle for 1 min to avoid over stimulation by stress. Then, the number of body bendings by worms were tracked by video-recording for 30 s in each condition. Thrashing rate was analyzed using wrMTrck plugin for ImageJ (National Institute of Health, NIH, Bethesda, MD, United States) and represented as body bendings per second (bbps).
Intracellular lipid in C. elegans was measured by Nile red staining as described previously (Malaiwong et al., 2019). Wild-type N2 and transgenic NL5901 worms were used in this assay. A stock solution of Nile red at concentration 0.5 mg/ml of acetone was prepared and further diluted in E. coli OP50 at a ratio of 1:250. Synchronized NL5901 L3 larvae were incubated on NGM containing E. coli OP50/Nile red with different doses of HLEA-P1 for 72 h at 20°C. After incubation, worms were washed three times by M9 buffer, transferred to 2% agarose pad on a glass slide. Then, worms were anesthetized with 30 mM sodium azide and finally enclosed with a coverslip. Lipid deposition in worms was observed under a fluorescence microscope (BX53; Olympus Corp., Tokyo, Japan). The lipid profiles were assessed by determining the average intensity of Nile red fluorescence from the whole bodies of worms using ImageJ software (National Institute of Health, NIH, Bethesda, MD, United States).
Intracellular ROS was measured using 2′,7′-Dichlorodihydrofluorescein diacetate (H2DCF-DA) probe by following the protocol described in a previous study (Yoon et al., 2018) with minor modifications. Briefly, after exposure to 6-OHDA and incubation with the compounds for 72 h, the worms were harvested and washed three times in M9 buffer. Then, 30 worms were transferred into a black 96-well plate containing 50 μl M9 buffer by using the platinum wire (5 worms/well, 6 wells for each treatment). A 50 μl of H2DCF-DA (final concentration, 25 μM in M9) was added to each well and the samples were incubated at room temperature for 1 h. Then, the plate was inserted into microplate Fluorescence reader with the excitation at 485 nm and emission at 530 nm. Samples were read after 1 h of incubation. The ROS level was calculated as the DCF fluorescence signal (Li et al., 2016). Each condition was performed independently three times.
In this assay, C. elegans strain CF1553 [muIs84, (pAD76) sod-3p::GFP + rol-6(su1006)] and CL2166 [dvIs19, (pAF15)gst-4p::GFP::NLS] were used. CF1553 and CL2166 are transgenic C. elegans strains expressing SOD-3 and GST-4 as a reporter gene, respectively. GFP-tagged SOD-3 can be observed in the head, tail and vulva of the CF1553. For CL2166, GFP-tagged GST-4 protein can be visualized in the head region, body wall muscle, intestine. Briefly, synchronized L3 larvae were exposed to 6-OHDA and then fed with different doses of HLEA-P1 as described in 6-OHDA-induced DAergic neurodegeneration assay. After 72 h of treatment, worms were washed three times with M9 buffer and transferred to 2% agarose pad on a glass slide for fluorescence imaging. The GFP intensity of SOD-3:GFP and GST-4:GFP was quantified using ImageJ software (National Institute of Health, NIH, Bethesda, MD, United States).
To observe subcellular localization of DAF-16 transcription factor, TJ356 [daf-16p::daf-16a/b::GFP + rol-6(su1006)] in which GFP-tagged DAF-16 protein were used in this assay. Synchronized L3 larvae were exposed to 6-OHDA and supplemented with different doses of HLEA-P1 as described previously. After 72 h of treatment, worms were washed three times with M9 buffer and transferred to 2% agarose pad on a glass slide for fluorescence imaging under a fluorescence microscope (BX53; Olympus Corp., Tokyo, Japan). Subcellular localization of DAF-16 was categorized as nuclear, intermediate and cytosolic. In this study, worms exhibited strong nuclear fluorescence dots were counted as nuclear. Worms having no fluorescence dot in cytoplasm were counted as cytosolic, while worms exhibited unclear cytosolic DAF-16 and unclear nuclear fluorescence dots were assigned as intermediate. All conditions were performed in three independent replicates. In each replicate, approximately 30 worms were used.
Approximately 800–1,000 of 6-OHDA-induced worms and NL5901 worms overexpressing α-synuclein were treated with 5 and 25 μg/ml HLEA-P1 for 72 h. In untreated group, worms were incubated in NGM/OP50 containing 1% (v/v) DMSO without HLEA-P1. After 72 h, all worms were washed by 3A water three times, centrifuged and collected. Total RNA was extracted using RNA extraction kit (Qiagen, Germany) following the manufacture’s protocol and kept at −80°C. Then, total RNA was converted into complementary DNA (cDNA) with iScript™ Reverse transcriptase Supermix for RT-qPCR (Bio-Rad, CA, United States). RT-qPCR was performed using SsoFast™ EvaGreen® Supermix with Low ROX qRT-PCR (Bio-Rad, CA, United States). Forward and reverse primers for specific genes were shown in Table 1. Real-time PCR were performed by holding the samples at 95°C for 30 s. Then, PCR samples were set for denaturing at 95°C for 5 s and to annealing at 60°C for 30 s. After 44 cycles, the sample were then heated up to 95°C. Eventually, EvaGreen fluorescence were detected by Real time PCR detection system (Bio-Rad, United States) and Cq values were obtained. The Cq values of control and treated groups were then calculated via 2−(ΔΔCq) equation representing fold change in the expression of each gene. The relative mRNA expression levels were normalized using reference internal control gene, act-1.
All experiments were performed in three independent trials. The data were statistically analyzed by GraphPad Prism Software. Significant difference between treatment groups and untreated group (1%DMSO) were compared using two-way ANOVA. p-value < .05 was considered significant.
In this study, 6 compounds were isolated from HLEA by CC and TLC and designated as HLEA-P1, HLEA-P2, HLEA-P3, HLEA-P4, HLEA-P5, and HLEA-P6. Firstly, we tested the neuroprotective effects of all isolated compounds against 6-OHDA-induced DAergic neurodegeneration. C. elegans BY250 strain with GFP-tagged DAergic neurons was used in this study. CEP neurons of C. elegans are the most susceptible to 6-OHDA toxicity when compared to other DAergic neurons (Nass et al., 2002). Therefore, we evaluated the viability of DAergic neurons by measuring the GFP intensity of CEP neurons. Our results showed that the GFP intensity significantly decreased to about 60%–64% (p < .05) in either 6-OHDA-treated group or 6-OHDA/DMSO-treated group (Figures 1B–G), suggesting that 6-OHDA-induced DAergic neurodegeneration was successfully established. At particular doses, certain HLEA compounds could restore DAergic neurons from 6-OHDA toxicity as reflected by the increase of GFP intensities in the treated group compared to the untreated group (6-OHDA/DMSO).
FIGURE 1. Effect of HLEA compounds 1–6 against 6-OHDA-induced DAergic neurodegeneration in C. elegans PD model. (A) Representative GFP expression pattern in CEP neurons (white arrows) of normal BY250, 6-OHDA-induced BY250, and 6-OHDA-induced worms with HLEA-P1 treatment at doses of 1, 5, and 25 μg/ml. (B–G) Graphical representation for relative fluorescent intensity of GFP expression in CEP neurons of normal BY250, BY250 exposed to 6-OHDA, BY250 exposed to 6-OHDA/DMSO, and BY250 exposed to 6-OHDA with HLEA compound 1–6 at doses of 1, 5, and 25 μg/ml. The data are presented as mean ± SD (n = 30, number of animals). The hash (#) indicates a significant difference between normal and 6-OHDA-induced worms (p < .05). The asterisk (*) indicates significant differences between untreated group (6-OHDA/DMSO) and HLEAs-treated groups at p < .05. Scale bar is 100 μm.
HLEA-P1 at 1, 5, and 25 μg/ml significantly restored GFP intensity of CEP neurons to 75.74%, 93.48%, and 95.63% (p < .05), respectively, when compared to untreated worms (Figure 1B). HLEA-P2 at doses of 1, 5, and 25 μg/ml significantly restored GFP intensity of CEP neurons in 6-OHDA induced-worms to 71.95%, 74.16%, and 80.28% (p < .05), respectively, compared to that of the untreated group (Figure 1C). HLEA-P3 at 1, 5, 25, and 50 μg/ml considerably increased GFP intensity of CEP neurons to 82.50%, 90.56%, and 91.54% (p < .05), respectively, when compared to untreated group (Figure 1D). HLEA-P4 at 1, 5, and 25 μg/ml showed significantly recovered GFP intensity of CEP neurons to 81.11%, 84.27%, and 81.41% (p < 0.05), respectively (Figure 1E). HLEA-P5 at 5 and 25 μg/ml significantly restored GFP intensity to 75.24% and 73.40% (p < .05), respectively, compared to untreated worms (Figure 1F). For HLEA-P6 treatment, only 5 μg/ml of HLEA-P6 slightly increased GFP intensity of CEP neurons to approximately 71.69% (p < .05) when compared to untreated group (Figure 1G).
Following these results, the most effective compound that conferred protection to DAergic neurons from 6-OHDA toxicity was HLEA-P1 at concentrations of 5 and 25 μg/ml. Therefore, HLEA-P1 was selected for further experiments to evaluate its anti-Parkinson effects and elucidate mechanisms of action.
Investigation of behavioral phenotypes is widely used for detecting DAergic function (Maulik et al., 2017). We therefore investigated whether HLEA-P1 could improve dopamine-dependent behaviors in 6-OHDA-induced worms. Dopamine signal of C. elegans is responsible for several behaviors (Maulik et al., 2017). In this study, food sensing response and ethanol avoidance behavior were used as behavioral readouts (Maulik et al., 2017). Food sensing behavior or basal slowing response refers to the decrease in locomotion of worms when the food is available. In the presence of food, normal wild type worms are able to sense bacterial texture and decrease their locomotor rate. In contrast, C. elegans PD models with deficit DAergic neurons fail to slow their movement, exhibiting abnormal decreased basal slowing rate (BSR) in the presence of bacteria (Sawin et al., 2000).
Our results showed that BSR was significantly decreased to about 67.56% and 69.64% in 6-OHDA-induced and 6-OHDA/DMSO-induced worms, respectively (p < .05), indicating functional deficit of DAergic neurons in food sensing response. Treatment with HLEA-P1 significantly restored BSR to 96.93% and 92.23% at 5 and 25 μg/ml, respectively, when compared to the untreated group (p < .001) (Figure 2A). The loss of dopamine signaling also affect ethanol avoidance behavior in C. elegans [19]. Comparing to normal worms, worms treated with 6-OHDA showed significant reduction in ethanol avoidance index. The ethanol avoidance index of normal worms was about 0.46 while it was decreased to −0.25 and −0.23 in 6-OHDA-induced and 6-OHDA/DMSO-induced worms, respectively (p < .05). As expected, ethanol avoidance deficit can be improved in HLEA-P1 treatments. The results showed that 5 and 25 μg/ml of HLEA-P1 remarkably increased ethanol avoidance index to 0.17 and 0.27 (p < .001), respectively, compared to the untreated group (Figure 2B). Taken together, the results suggested that HLEA-P1 could restore dopamine-dependent behaviors in 6-OHDA induced DAergic neurodegenerative C. elegans.
FIGURE 2. Effect of HLEA-P1 on dopamine dependent behaviors in 6-OHDA-induced C. elegans PD model. (A) Graphical representation for relative basal slowing rate. The data are presented as mean ± SEM (with three independent replicates, n = 30 number of animals per replicate). (B) Graphical representation for ethanol avoidance index. The data are presented as mean ± SEM (three independent replicates, n ≥ 50 number of animals per replicate). The hash (#) indicates a significant difference between normal and 6-OHDA-induced worms (p < .05). The asterisk (*) indicates significant differences between untreated group (6-OHDA/DMSO) and HLEA-P1-treated groups, ***p < .001.
We hypothesized that the mechanism of 6-OHDA in affecting neurotoxicity is oxidative stress. Mechanically, 6-OHDA targets mitochondria and subsequently causes the overproduction of ROS. Therefore, we were interested in whether HLEA-P1 could reduce the oxidative stress to alleviate 6-OHDA toxicity in C. elegans model. In the present study, intracellular ROS was measured by H2DCF-DA assay. Intracellular ROS, especially H2O2 can convert non-fluorescence molecules, H2DCF-DA, to the fluorescence DCF molecules. Therefore, the ROS level can be interpreted by the DCF fluorescence levels. As shown in Figure 3, when compared to normal worms, intracellular ROS level dramatically increased to 239.58% and 250.60% in 6-OHDA-induced and 6-OHDA/DMSO-induced groups, respectively (p < .05). When the worms were treated with HLEA-P1 at 5 μg/ml, intracellular ROS significantly decreased to 101.81% (p < .05), the level that was very close to that of the untreated worms. Meanwhile, 25 μg/ml of HLEA-P1 could also significantly reduced ROS to the level that is insignificantly different compared to that of the untreated worms. These results are suggesting that HLEA-P1 at 5 μg/ml is the most effective in the reduction of oxidative stress in 6-OHDA-induced C. elegans PD model.
FIGURE 3. Effect of HLEA-P1 on reactive oxygen species in 6-OHDA-induced C. elegans PD model. Graphical representation for DCF fluorescence (%) in normal, 6-OHDA-, 6-OHDA/DMSO-, and 6-OHDA/DMSO plus HLEA-P1-treated worms compared to the untreated group. The data are presented as mean ± SEM (three independent replicates, n = 30 number of animals per replicate). The hash (#) indicates a significant difference between untreated and 6-OHDA-, 6-OHDA/DMSO-treated worms. The asterisk (*) indicates significant differences between 6-OHDA-, 6-OHDA/DMSO- and HLEA-P1-treated groups at p < .05.
Next, we determined the effect of HLEA-P1 on α-synucleinopathies, another hallmark of PD. We used the transgenic C. elegans NL5901 which expresses the YFP-tagged α-synuclein that can be detectable under a fluorescence microscope. In this study, worms treated with 5 and 25 μg/ml of HLEA-P1 showed significantly decreased YFP intensity to 81.51% and 80.90%, respectively, when compared to untreated group (p < .05). In other words, the level of α-synuclein aggregation was significantly diminished by about 18.49% and 19.10% in the transgenic worms treated with 5 and 25 μg/ml of HLEA-P1, respectively (Figures 4A, B).
FIGURE 4. Effect of HLEA-P1 against α-synuclein aggregation and movement deficit in NL5901 C. elegans expressing α-synuclein. (A) Representative of YFP-tagged α-synuclein expression in body wall muscle cells of NL5901 strain. (B) Graphical representation for relative fluorescent intensity of YFP expression. (C) Graphical representation of the rate of movement of C. elegans with HLEA-P1 treatment. The data are presented as mean ± SD (n = 30, number of animals). The hash (#) indicates a significant difference between N2 wild type and NL5901 worms (p < .05). The asterisk (*) indicates significant differences between untreated group (DMSO) and HLEA-P1-treated group, *p < .05, **p < .01, ***p < .001. Scale bar is 200 μm.
Many studies reported the association of decline muscular function and protein aggregation in the transgenic C. elegans [24]. Previous study showed that NL5901 worms exhibited decreased trashing or swimming behavior with aging when compared with normal wild-type worms (Maulik et al., 2017). To investigate whether HLEA-P1 could improve thrashing deficits, we performed this assay at two time points (adult day 3 and day 5 of C. elegans). The results showed that wild-type N2 worms have thrashing rate at 1.34 and 1.21 in day 3 and day 5 of adulthood, respectively. In contrast, day 3 adult NL5901 worms showed significantly decreased thrashing rate to 0.85 when compared to wild-type worms with thrashing rate of 1.34 (p < .05) (Figure 4C). Such altered motility was more obvious in day 5 the adult population, which have thrashing rate at 0.46 (p < .05). This could be due to age-dependent aggregation of α-synuclein interfering with the worm motility. The results demonstrated that this impairment can be improved upon treatment with HLEA-P1. The rate of movement of day 3 adult treated with 5 and 25 μg/ml of HLEA-P1 were moderately increased to 1.17 and 1.07, respectively (p < .01 and p < .05, respectively). The significant restorative effect can be observed in day 5 of adulthood in which the rate of movement increased to 1.02 and 0.87 in worms treated with 5 and 25 μg/ml of HLEA-P1, respectively (p < .01) (Figure 4C). Overall, these results suggest that HLEA-P1 could improve movement deficits in PD worms which is suppressed by α-synuclein aggregation.
Aggregation of α-synuclein has been reported to cause oxidative degradation of lipids (Maulik et al., 2017). In addition, several studies identified abnormal decrease of the lipid contents in PD worms expressing α-synuclein (Maulik et al., 2017). Therefore, we further investigated the effect of HLEA-P1 on lipid deposition in NL5901 worms using Nile red staining. The results showed that NL5901 worms exhibited 25% lower lipid content than that of wild-type N2 (Figure 5). While HLEA-P1 did not change the lipid deposition in normal wild-type N2, treatment with HLEA-P1 at 5 and 25 μg/ml significantly increased lipid deposition in NL5901 strain to 85.65% and 86.65% of the wild type (p < .001), respectively (Figure 5).
FIGURE 5. Effect of HLEA-P1 on lipid restoration in transgenic NL5901 worms expressing α-synuclein. (A) Representative Nile red fluorescent images of wild-type N2, C. elegans NL5901 and C. elegans NL5901 treated with HLEA-P1 at 5 and 25 μg/ml. (B) Graphical representation for Nile red fluorescent signal. The data are presented as mean ± SEM (with three independent replicates, n ≥ 30 number of animals per replicate). The hash (#) indicates a significant difference between wild-type N2 and NL5901 worms (p < .05). The asterisk (*) indicates significant difference between untreated group (NL5901/DMSO) and HLEA-P1-treated groups, ***p < .001. Scale bar is 200 μm.
Considering the fact that 6-OHDA and α-synuclein aggregation contribute to oxidative stress in DAergic neurons, we hypothesized that HLEA-P1 might be involved in oxidative stress reduction. In C. elegans, DAF-16 is one of the major transcription factors of the insulin/IGF-1 signaling (IIS) pathway that provides oxidative stress resistance by gaining access into the nucleus and activating the expressions of antioxidant enzymes (Altintas et al., 2016). Therefore, we investigated the effect of HLEA-P1 on DAF-16 nuclear translocation in TJ356 carrying GFP-tagged DAF-16 protein. At basal condition, DAF-16 localizes in cytoplasm. Under stress, DAF-16 undergoes nuclear translocation to activate transcription of downstream-target genes (Altintas et al., 2016). In this study, subcellular localizations of DAF-16 were classified as cytosolic, intermediate, and nuclear localization as shown in Figure 6A. In normal TJ356, DAF-16 was shown to localize in cytosol, intermediate and nucleus at 88.89%, 8.33%, and 2.78%, respectively. In PD models, 55.58% and 55.37% of 6-OHDA-induced and 6-OHDA/DMSO-induced worms exhibited cytosolic DAF-16 localization. Approximately 41.39% of the former and 41.43% of the latter showed intermediate DAF-16 localization, while only 3.04% and 3.20% exhibited DAF-16 nuclear localization. Treatment with HLEA-P1 at 5 and 25 μg/ml significantly increased DAF-16 nuclear localization up to 27.35% and 17.49%, respectively (p < .05) (Figure 6). At the same time, cytosolic DAF-16 localization of 5 and 25 μg/ml treatment was significantly reduced to 25.36% and 22.95%, respectively (p < .05). These results suggested that HLEA-P1 triggered DAF-16 translocation from cytoplasm to nucleus in 6-OHDA-induced C. elegans PD model.
FIGURE 6. Effect of HLEA-P1 on nuclear localization of transcription factor DAF-16 in 6-OHDA-induced C. elegans. (A) Representative fluorescent images of different subcellular localization of DAF-16. (B) Graphical representation of subcellular distribution of daf-16. The data are presented as mean ± SEM (with three independent replicates, n ≥ 30 number of animals per replicate). The asterisk (*) indicated significant differences of DAF-16 nuclear localization between untreated group (6-OHDA/DMSO) and HLEA-P1-treated groups at p < .05.
In IIS pathway of C. elegans, SKN-1 is another transcription factor regulating oxidative stress resistance. SKN-1 activates the transcription of the anti-oxidant gene such as gst-4 (glutathione S-transferase-4) whereas DAF-16 activates sod-3 (superoxide dismutase-3). Hence, we further confirmed that HLEA-P1 activates DAF-16 but not SKN-1 by investigating its effect on their downstream targets, SOD-3 and GST-4 proteins, using the transgenic strains CF1553 and CL2166, respectively. The results demonstrated that the 6-OHDA-induced and the 6-OHDA/DMSO-induced CF1553 exhibited significantly reduced SOD-3:GFP expression to 55.00% and 52.40%, when compared with normal CF1553 worms. These data indicated the downregulation of SOD-3:GFP upon the 6-OHDA treatment. As expected, treatment with HLEA-P1 at 5 and 25 μg/ml remarkably increased SOD-3 to 97.15% and 107.42%, respectively (p < .05 and p < .01, respectively) when compared with the untreated group (Figures 7A, B). While, 6-OHDA-induced and 6-OHDA/DMSO-induced CL2166 did not show significant difference in GST-4 expression when compared with the normal CL2166 worms. Moreover, treatment with HLEA-P1 at 5 and 25 μg/ml could not enhance the GST-4:GFP expression in CL2166 (Figures 7C, D). Taken together, these results suggested that treatment with HLEA-P1 significantly activates expression of SOD-3, a downstream target of DAF-16, rather than GST-4 which is a downstream target of SKN-1, in 6-OHDA-induced C. elegans.
FIGURE 7. Effect of HLEA-P1 on SOD-3 and GST-4 in C. elegans PD model. (A,B) Representative SOD-3:GFP expression and mean fluorescent intensity of SOD-3:GFP in transgenic CF1553 in different conditions (normal CF1553, 6-OHDA-induced CF1553, 6-OHDA/DMSO-treated CF1553, and 6-OHDA-induced CF1553 treated with HLEA-P1 at 5 and 25 μg/ml). Scale bar is 100 μm. (C,D) Representative GST-4:GFP expression and mean fluorescent intensity of GST-4:GFP in transgenic CL2166 in different conditions (normal CL2166, 6-OHDA-induced CL2166, 6-OHDA/DMSO-treated CL2166 and 6-OHDA-induced CL2166 treated with HLEA-P1 at 5 and 25 μg/ml). Scale bar is 200 μm. The hash (#) indicates a significant difference between wild type N2 and transgenic worms (p < .05). The asterisk (*) indicates significant difference between untreated group (6-OHDA/DMSO) and HLEA-P1 treated groups, ***p < .001.
Next, we investigated the mRNA expression of DAF-16 target genes including sod-3, hsp-16.1, hsp-16.2, and hsp-12.6 in 6-OHDA-induced C. elegans model and transgenic C. elegans NL5901 expressing α-synuclein model. sod-3 is an anti-oxidative gene that upregulated by IIS dependent DAF-16 transcription factor (Tullet et al., 2008). hsp-16.1, hsp-16.2, and hsp-12.6 are small heat shock protein, HSP27 homologs, involve in stress resistance (Hsu et al., 2003). Downregulation of hsp27 is observed in several neurodegenerative diseases including PD while overexpression of hsp27 showed neuroprotective effects against neurodegenerative diseases (Vidyasagar et al., 2012; Venugopal et al., 2019). In 6-OHDA-induced model, the results showed that treatment with HLEA-P1 at 5 μg/ml insignificantly upregulated expressions of mRNAs for sod-3 (1.8-fold), hsp-16.1 (2.25-fold), hsp-16.2 (1.74-fold), and hsp-12.6 (1.29-fold) when compared to untreated group (p > .05) Figure 8A. In contrast, 25 μg/ml HLEA-P1 significantly upregulated expressions of mRNAs for sod-3 (14.16-fold), hsp-16.1 (16.71-fold), and hsp-16.2 (9.84-fold), compared to untreated group (p < .05). Considerable upregulation with no significant difference of hsp-12.6 mRNA (4.91-fold) was also observed in 25 μg/ml HLEA-P1 treatment. Despite insignificant upregulation by treatment at low concentration, our results showed that HLEA-P1 could upregulate daf-16 downstream target-genes including sod-3, hsp-16.1, and hsp-16.2. In NL5901 model, treatment with 5 and 25 μg/ml HLEA-P1 significantly upregulated expression sod-3 mRNA by 2.28-fold and 1.69-fold, respectively (p < .05) when compared to untreated group Figure 8B. Moreover, treatment with 5 μg/ml HLEA-P1 significantly increased expression of hsp-16.2 mRNA compared to untreated group (p < .05). These results suggested that 5 μg/ml HLEA-P1 significantly upregulated sod-3 and hsp-16.2, but not hsp16.1 and hsp12.6.
FIGURE 8. Effect of HLEA-P1 on expressions of mRNAs of DAF-16 target genes in C. elegans PD models. Graphical representation for fold change of gene expression level (2−(ΔΔCq)) of DAF-16 target genes in 6-OHDA-induced worms (A) and NL5901 worms overexpressing α-synuclein (B). The relative mRNA expressions were normalized with internal control act-1. The data represented as a mean ± SEM (three independent replicates, n = 800–1,000 number of animals per replicate). The asterisk (*) indicates significant differences between untreated group (DMSO) and HLEA-P1-treated group, *p < .05, **p < .01, ***p < .001.
Based on structural elucidation by 1H and 13C NMR analysis, purified compounds 1-6 in EtOAc fraction were identified and shown in Supplementary Material (Supplementary Figure S1). For HLEA-P1, the 1H-NMR/13C NMR spectra showed that white powder, HR-TOFMS (ES+): m/z 195.1062 [M + Na]+, calcd for C10H20O2+Na. 1H-NMR (CDCl3, 400 MHz): 0.85 (3H, t = 7.2, H-10), 1.24 (10H, m, H-5-9), 1.60 (2H, m, H-3), 2.31 (2H, t, H-2). 13C-NMR (CDCl3, 100 MHz): 14.1 (C-10), 22.7 (C-9), 24.7 (C-3), 29.0 (C-4), 29.3 C-5, 7), 29.6 (Cδ 6), 31.9 (C-8), 34.0 (C-2), 178.4 (COOH). According to a combination of 1H-NMR/13C NMR spectra, HLEA-P1 was identified as Decanoic acid (Capric acid), C10H20O2 (Figure 9A and Supplementary Figures S2, S3). As shown in Figure 9B, Peak A at 0.85 ppm indicates a presence of terminal methyl group (CH3) bounded to the C9. Peak B at 1.24 ppm displayed a long chain of methylene protons (CH2) of the C4-C9 atoms. Peak C at 1.60 ppm is related to 2 protons bound to the C3 atom. Peak D at 2.31 ppm is corresponded with methylene protons (CH2) of C2.
FIGURE 9. (A) Chemical structure of HLEA-P1. (B) 1H-NMR spectrum of HLEA-P1, decanoic acid or capric acid in CDCl3.
In our recent report (Malaiwong et al., 2019), the crude extract of H. leucospilota ethyl acetate fraction (HLEA) exhibited anti-Parkinson’s activities in C. elegans PD models. In this ensuing study, we demonstrated that such HLEA fraction consists of 6 bioactive compounds (Supplementary Figures S1–S12) with HLEA-P1 showing the most pronounced effect against 6-OHDA-induced DAergic neurodegeneration (Figure 1). Hence, our present study focused on the anti-Parkinson’s effects of HLEA-P1 and its mechanism of action at molecular level. Firstly, we found that HLEA-P1 (at 5 and 25 μg/ml) could restore DAergic neurons from 6-OHDA-induced neurodegeneration in the BY250 strain (Figure 1). Consistent with the restored viability of the DAergic neurons, HLEA-P1 improved food sensing and ethanol avoidance behaviors in 6-OHDA-induced worms (Figure 2). In addition to the DAergic neurodegeneration model, we also tested the effect of HLEA-P1 on the α-synucleinopathies using the transgenic C. elegans NL5901 which over-expresses human α-synuclein. HLEA-P1 (at 5 and 25 μg/ml) significantly decreased α-synuclein aggregation which was correlated with the improvement of thrashing behavior in NL5901 worms (Figure 4).
Regardless of the exogenous and the endogenous sources, oxidative stress is considered to be the common molecular event in PD pathogenesis that triggers neurodegeneration (Chakraborty et al., 2013). The 6-OHDA is a neurotoxin which mechanistically causes mitochondrial impairment leading to overproduction of the intracellular ROS (Blum et al., 2001). The α-synuclein contributes to oxidative stress by inhibiting mitochondrial complex I activity, decreasing mitochondrial function, and generating the excessive ROS level (Scarlata and Golebiewska, 2014). We therefore investigated whether HLEA-P1 could reduce oxidative stress, thereby alleviating Parkinsonism in C. elegans PD models. We measured intracellular ROS using H2DCF-DA assay and found that HLEA-P1, in particular 5 μg/ml, significantly reduced intracellular ROS level in 6-OHDA-induced worms (Figure 3). It has been well known that the overproduction of the intracellular ROS causes damage to the biomolecules such as proteins, DNA, and lipids (Chakraborty et al., 2013). The aggregation of α-synuclein contributes to oxidative degradation of lipids (Angelova et al., 2015). Although we did not measure intracellular ROS in NL5901 overexpressing human α-synuclein model, we investigated the lipid accumulation in this strain using Nile red staining. The results revealed that HLEA-P1 had no significant effect on lipid accumulation in wild-type worms, but could restore lipid content in transgenic worms expressing α-synuclein (Figure 5). These results implied that HLEA-P1 might not act directly on lipid metabolism but rather indirectly through its reductive effects on oxidative stress and α-synuclein aggregation.
The IIS pathway is crucial for regulating neuronal physiology including neuronal development, synaptic maintenance, neuronal growth, and survival (Bassil et al., 2014). Growing evidence indicated the alteration of this pathway in many neurodegenerative diseases including PD (Bassil et al., 2014; Bassil et al., 2022). Meanwhile, targeting IIS pathway showed positive benefits for disease modification (Bassil et al., 2014); for instance, knock down of DAF-2 (an orthologue of mammal insulin/IGF receptor) decreased α-synuclein aggregation in C. elegans model (Haque et al., 2020). Interestingly, several bioactive compounds from sea cucumbers could modulate the IIS pathway, for example, the crude extract from H. scabra could exert anti-oxidant and anti-aging properties (Jattujan et al., 2018). Another study by Kitisin et al. (2019) reported that the extract of H. leucospilota body wall promoted the lifespan extension and stress tolerance in C. elegans model by targeting DAF-16 transcription factor of IIS pathway. Therefore, in this study we explored whether HLEA-P1 exerted anti-PD activities through IIS/DAF-16 pathway. Our results showed that HLEA-P1 activates DAF-16 nuclear localization in 6-OHDA-induced worms (Figure 6). This result was corroborated by a significant increase in SOD-3:GFP expression in the 6-OHDA-induced CF1553 treated with HLEA-P1 while expression of GST-4, a downstream target of SKN-1, was not significant (Figure 7). Therefore, it could be concluded that HLEA-P1 promotes stress resistance by activating DAF-16 transcription factor of IIS pathway rather than SKN-1 transcription factor.
To further confirm that anti-Parkinson’s activities of HLEA-P1 is mediated by de-activating IIS pathway which, in turn, allows DAF-16 to enter the nucleus and activate its target genes, we measured the expressions of mRNAs of sod-3, hsp-16.1, hsp-16.2, and hsp-12.6 in C. elegans PD models. Our results showed that expression levels of mRNAs of sod-3 and hsp27 homologs (hsp-16.1, hsp-16.2, and hsp-12.6) were all upregulated in the 6-OHDA-induced worms treated with HLEA-P1. Recently, several lines of evidence indicated that the HSPs can interact not only with misfolded proteins to prevent unfolded protein response (UPR) but also with native proteins to modulate several normal cellular functions (Venugopal et al., 2019). During stress conditions, smHSP27 acts as the anti-oxidant, scavenging intracellular ROS by increasing glutathione level (Venugopal et al., 2019). Moreover, HSP27 can act as an anti-apoptotic agent by inhibiting mitochondrial dysfunction and apoptotic pathway (Venugopal et al., 2019). Previously, it was shown that overexpression of hsp27 exhibited protective effects against 6-OHDA-induced toxicity by inhibiting cytochrome C release, caspase activation, thereby apoptosis (Gorman et al., 2005). Hence, we hypothesized that upregulation of sod-3 and HSP27 homologs by HLEA-P1 may enhance anti-oxidative and anti-apoptotic capacities, rendering the 6-OHDA less toxic.
In our present study we demonstrated that in C. elegans NL5901, HLEA-P1 (5 and 25 μg/ml) significantly upregulated transcription level of sod-3. Similarly, a previous study reported that upregulation of sod-3 by dianxianning, an anti-epileptic drug, reduced Aβ oligomers and its toxicity in C. elegans AD model (Zhi et al., 2017). An in vitro study showed that the overexpression of SOD-3 protected SH-SY5Y cell against Aβ-induced-toxicity by inhibiting ROS generation by the mitochondrial pathway (Yang et al., 2009). Deletion of sod-3 aggravated impaired motility of transgenic Aβ worm (Minniti et al., 2015). Based on PD pathogenesis, α-synuclein can interact with mitochondria complex I, leading to dysfunctional mitochondria and oxidative stress (Hsu et al., 2000). In turn, the excessive ROS causes the aggregation of α-synuclein and acceleration of neuronal cell death (Scarlata and Golebiewska, 2014). We, therefore, proposed that HLEA-P1 may increase anti-oxidative capacity through the upregulation of sod-3, thereby decreasing α-synuclein aggregation and its toxicity. C. elegans NL5901 treatment with low dose of HLEA-P1 (5 μg/ml) showed a significant upregulation of hsp including hsp16.1 and hsp16.2. The activation of hsp16.1 and hsp16.2 might promote the clearance system of α-synuclein to reduce α-synuclein aggregation in NL5901. Unlike other hsps, hsp-12.6 was not upregulated by 5 μg/ml HLEA-P1 treatment. When compared to other HSPs, hsp12.6 contains unusually short N and C-terminal regions which affects its chaperone activity (Leroux et al., 1997). Therefore, we did not observe upregulation of mRNA level of hsp12.6 in NL5901 model with proteotoxic stress, while upregulation of hsp-12.6 that might confer anti-oxidative and anti-apoptotic capacity was observed in the 6-OHDA-induced model. Overall, our results demonstrated that a low dose of HLEA-P1 (5 μg/ml) suppressed α-synuclein aggregation and its toxicity by upregulation of sod-3, hsp16.1, and hsp16.2 in NL5901. However, 25 μg/ml of HLEA-P1 did not upregulate mRNA expression of hsp, indicating that protective effects against α-synuclein aggregation of high concentration may lead to unresponsive genes or involve other pathways. To further clarify other possible targets of HLEA-P1, in the future study a transcriptomic analysis can be performed to verify the upregulated and downregulated genes that may be involved, then the related mechanisms of those genes can further be investigated through molecular and protein analysis methods.
Chemical structure elucidation demonstrated that HLEA-P1 is a medium chain fatty acid (MCFA) with 10 carbon atoms named decanoic acid or capric acid. It is well established that this sea cucumber contains high essential fatty acids (Roopashree et al., 2021). Typically, decanoic acid and other MCFAs have been widely used as nutritional therapy (Zulkanain et al., 2020). MCFA have been shown to exhibit anti-Alzheimer’s, anti-inflammation, as well as anti-oxidative effects (Zulkanain et al., 2020). Here, we reported a reduction of intracellular ROS and upregulation of sod-3 by decanoic acid in C. elegans PD model. Neuroprotection by MCFA has been reported in the AD model. Reduced oxidative stress and increased survival of neurons were observed in amyloid beta-exposed cortical neurons treated with coconut oil containing decanoic acid (Nafar and Mearow, 2014). Moreover, decanoic acid-enriched oil has been reported to increase activities of antioxidative enzymes CAT, SOD, GSH and GPx, in vivo (Sengupta and Ghosh, 2012). In intestinal cell, decanoic acid suppressed cyclophosphophamide-induced oxidative stress through activating enzyme activity of SOD and GPX and their gene expression (Lee and Kang, 2017). Previous study also reported that decanoic acid reduces oxidative stress in neuroblastoma cells by activating enzyme activity of catalase but not its transcriptional level (Mett and Müller, 2021). However, activity of SOD was not upregulated by decanoic acid treatment (Nafar and Mearow, 2014). These lines of evidence support our hypothesis that decanoic acid isolated from H. leucospilota alleviates 6-OHDA toxicities by upregulating antioxidative defense in C. elegans PD model. Moreover, previous study reported that decanoic acid activates autophagy by upregulating autophagy-inducing protein including Atg1 and Atg8 (Warren et al., 2021). Autophagy is the major clearance system of α-synuclein aggregation (Maiti et al., 2017). It is possible that high dose of decanoic acid might reduce α-synuclein aggregation and its toxicity by targeting autophagy process. Effect of decanoic acid on reducing lipid peroxidation has also been reported (Sengupta and Ghosh, 2012; Lee and Kang, 2017). Previous study showed decreasing of malondialdehyde (MDA), a marker of lipid peroxidation, following treatment with decanoic acid-enrich oil (Sengupta and Ghosh, 2012; Lee and Kang, 2017; Yoon et al., 2018). In this study, we found recovered lipid deposition in C. elegans PD model treated with decanoic acid. Antioxidative enzymes such as SOD and GPx play role in controlling lipid peroxidation (Devi et al., 2000). Thus, the restoration of lipid deposition we observed might be the consequence of antioxidative activity of decanoic acid.
Taken together, we found that anti-Parkinson’s potential of ethyl acetate fraction of the crude extract of the H. leucospilota body wall, is attributable to decanoic acid. Our findings substantiated nutrition property of the sea cucumber, H. leucospilota, for use as a functional food and nutraceuticals for PD and possibly also other neurodegenerative diseases of similar etiology. However, to promote H. leucospilota-derived decanoic acid as a nutraceutical for humans, its anti-PD effects, toxicity, and underlying molecular mechanism of action need to be verified in higher mammal models such as mouse. Moreover, comparison among decanoic acid isolated from H. leucospilota with those isolated from other organisms or its synthetic forms should also be studied for developing the most economical and efficient MCFA as a nutritional therapy against Parkinson’s disease.
In this study, we reported that decanoic acid isolated from ethyl acetate fractions of H. leucospilota body wall could attenuate DAergic neurodegeneration, reduce α-synuclein aggregation, and improve associated behavioral deficits in C. elegans PD models. We found that H. leucospilota-derived decanoic acid activated DAF-16 transcription factor which upregulated its target genes including the anti-oxidants genes (such as sod-3) and the small heat shock proteins (such as hsp16.1, hsp16.2, and hsp12.6) to enhance oxidative stress and proteotoxic stress resistances in C. elegans PD models. Our study revealed for the first time about anti-Parkinson’s effects of decanoic acid-derived from sea cucumbers. Mechanistically, we found that decanoic acid exerts anti-Parkinson’s effects by de-activating IIS pathway and allows DAF16 to enter the nucleus to activate its target anti-oxidation genes and heat shock proteins in C. elegans PD models.
The original contributions presented in the study are included in the article/Supplementary Materials, further inquiries can be directed to the corresponding author.
The animal study was reviewed and approved by Faculty of Science, Mahidol University–Institutional Animal Care and Use Committee.
TS performed all experiments with the support of NM, PC, PJ, and KM. TS conducted data analysis and interpretation and writing-original draft preparation with the suggestion of NM, PC, and KM. KM and PS conceptualized, reviewed and edited the manuscript. NN and NS performed extraction, isolation and chemical characterization of purified H. leucospilota compounds.
This research paper was supported by Specific League Funds from Mahidol University to KM and Ph.D. scholarship from Science Achievement Scholarship of Thailand (SAST) to TS. H. leucospilota were provided by the Coastal Fisheries Research and Development Center, Prachuap Khiri Khan province, Thailand. C. elegans strains were provided by the CGC which is funded by NIH Office of Research Infrastructure Programs (P40 OD010440). The BY250 C. elegans were kindly obtained from Prof. Randy Blakely, Florida Atlantic University, United States.
The authors declare that the research was conducted in the absence of any commercial or financial relationships that could be construed as a potential conflict of interest.
All claims expressed in this article are solely those of the authors and do not necessarily represent those of their affiliated organizations, or those of the publisher, the editors and the reviewers. Any product that may be evaluated in this article, or claim that may be made by its manufacturer, is not guaranteed or endorsed by the publisher.
The Supplementary Material for this article can be found online at: https://www.frontiersin.org/articles/10.3389/fphar.2022.1004568/full#supplementary-material
Altintas, O., Park, S., and Lee, S-J. V. (2016). The role of insulin/IGF-1 signaling in the longevity of model invertebrates, C. elegans and D. melanogaster. BMB Rep. 49 (2), 81–92. doi:10.5483/bmbrep.2016.49.2.261
Angelova, P. R., Horrocks, M. H., Klenerman, D., Gandhi, S., Abramov, A. Y., and Shchepinov, M. S. (2015). Lipid peroxidation is essential for α-synuclein-induced cell death. J. Neurochem. 133 (4), 582–589. doi:10.1111/jnc.13024
Bassil, F., Delamarre, A., Canron, M. H., Dutheil, N., Vital, A., Négrier Leibreich, M. L., et al. (2022). Impaired brain insulin signalling in Parkinson's disease. Neuropathol. Appl. Neurobiol. 48 (1), e12760. doi:10.1111/nan.12760
Bassil, F., Fernagut, P-O., Bezard, E., and Meissner, W. G. (2014). Insulin, IGF-1 and GLP-1 signaling in neurodegenerative disorders: Targets for disease modification? Prog. Neurobiol. 118, 1–18. doi:10.1016/j.pneurobio.2014.02.005
Blum, D., Torch, S., Lambeng, N., Nissou, M-F., Benabid, A-L., Sadoul, R., et al. (2001). Molecular pathways involved in the neurotoxicity of 6-OHDA, dopamine and MPTP: Contribution to the apoptotic theory in Parkinson's disease. Prog. Neurobiol. 65 (2), 135–172. doi:10.1016/s0301-0082(01)00003-x
Bodhicharla, R., Nagarajan, A., Winter, J., Adenle, A., Nazir, A., Brady, D., et al. (2012). Effects of α-synuclein overexpression in transgenic Caenorhabditis elegans strains. CNS Neurol. Disord. Drug Targets 11 (8), 965–975. doi:10.2174/1871527311211080005
Ceesay, A., Nor Shamsudin, M., Aliyu-Paiko, M., Ismail, I. S., Nazarudin, M. F., and Mohamed Alipiah, N. (2019). Extraction and characterization of organ components of the Malaysian sea cucumber Holothuria leucospilota yielded bioactives exhibiting diverse properties. Biomed. Res. Int. 2019, 2640684. doi:10.1155/2019/2640684
Chakraborty, S., Bornhorst, J., Nguyen, T. T., and Aschner, M. (2013). Oxidative stress mechanisms underlying Parkinson’s disease-associated neurodegeneration in C. elegans. Int. J. Mol. Sci. 14 (11), 23103–23128. doi:10.3390/ijms141123103
Chalorak, P., Jattujan, P., Nobsathian, S., Poomtong, T., Sobhon, P., and Meemon, K. (2018). Holothuria scabra extracts exhibit anti-Parkinson potential in C. elegans: A model for anti-Parkinson testing. Nutr. Neurosci. 21 (6), 427–438. doi:10.1080/1028415X.2017.1299437
Chalorak, P., Sornkaew, N., Manohong, P., Niamnont, N., Malaiwong, N., Limboonreung, T., et al. (2021). Diterpene glycosides from Holothuria scabra exert the α-synuclein degradation and neuroprotection against α-synuclein-Mediated neurodegeneration in C. elegans model. J. Ethnopharmacol. 279, 114347. doi:10.1016/j.jep.2021.114347
Choo, P., Toral-Granda, V., Lovatelli, A., and Vasconcellos, M. (2008). sea cucumbers A global review of Fisheries and trade fao Fisheries and aquaculture. Technical Paper No 516.
Cooper, J. F., Dues, D. J., Spielbauer, K. K., Machiela, E., Senchuk, M. M., and Van Raamsdonk, J. M. (2015). Delaying aging is neuroprotective in Parkinson’s disease: A genetic analysis in C. elegans models. NPJ Park. Dis. 1 (1), 15022–15112. doi:10.1038/npjparkd.2015.22
Devi, G. S., Prasad, M. H., Saraswathi, I., Raghu, D., Rao, D., and Reddy, P. (2000). Free radicals antioxidant enzymes and lipid peroxidation in different types of leukemias. Clin. Chim. Acta. 293 (1-2), 53–62. doi:10.1016/s0009-8981(99)00222-3
Feigin, V. L., Abajobir, A. A., Abate, K. H., Abd-Allah, F., Abdulle, A. M., Abera, S. F., et al. (2017). Global, regional, and national burden of neurological disorders during 1990–2015: A systematic analysis for the global burden of disease study 2015. Lancet. Neurol. 16 (11), 877–897. doi:10.1016/S1474-4422(17)30299-5
Gorman, A. M., Szegezdi, E., Quigney, D. J., and Samali, A. (2005). Hsp27 inhibits 6-hydroxydopamine-induced cytochrome c release and apoptosis in PC12 cells. Biochem. Biophys. Res. Commun. 327 (3), 801–810. doi:10.1016/j.bbrc.2004.12.066
Haque, R., Kumar, L., Sharma, T., Fatima, S., Jadiya, P., Siddiqi, M. I., et al. (2020). Human insulin modulates α-synuclein aggregation via DAF-2/DAF-16 signalling pathway by antagonising DAF-2 receptor in C. elegans model of Parkinson’s disease. Oncotarget 11 (6), 634–649. doi:10.18632/oncotarget.27366
Harrington, A. J., Hamamichi, S., Caldwell, G. A., and Caldwell, K. A. (2010). C. elegans as a model organism to investigate molecular pathways involved with Parkinson's disease. Dev. Dyn. 239 (5), 1282–1295. doi:10.1002/dvdy.22231
Hsu, A-L., Murphy, C. T., and Kenyon, C. (2003). Regulation of aging and age-related disease by DAF-16 and heat-shock factor. Science 300 (5622), 1142–1145. doi:10.1126/science.1083701
Hsu, L. J., Sagara, Y., Arroyo, A., Rockenstein, E., Sisk, A., Mallory, M., et al. (2000). alpha-synuclein promotes mitochondrial deficit and oxidative stress. Am. J. Pathol. 157 (2), 401–410. doi:10.1016/s0002-9440(10)64553-1
Huang, C., Zhang, Z., and Cui, W. (2019). Marine-derived natural compounds for the treatment of Parkinson’s disease. Mar. Drugs 17 (4), 221. doi:10.3390/md17040221
Jattujan, P., Chalorak, P., Siangcham, T., Sangpairoj, K., Nobsathian, S., Poomtong, T., et al. (2018). Holothuria scabra extracts possess anti-oxidant activity and promote stress resistance and lifespan extension in Caenorhabditis elegans. Exp. Gerontol. 110, 158–171. doi:10.1016/j.exger.2018.06.006
Kitisin, T., Suphamungmee, W., and Meemon, K. (2019). Saponin-rich extracts from Holothuria leucospilota mediate lifespan extension and stress resistance in Caenorhabditis elegans via daf-16. J. Food Biochem. 43 (12), e13075. doi:10.1111/jfbc.13075
Lee, J., Jee, C., and McIntire, S. L. (2009). Ethanol preference in C. elegans. Genes Brain Behav. 8 (6), 578–585. doi:10.1111/j.1601-183X.2009.00513.x
Lee, S. I., and Kang, K. S. (2017). Function of capric acid in cyclophosphamide-induced intestinal inflammation, oxidative stress, and barrier function in pigs. Sci. Rep. 7 (1), 16530–16612. doi:10.1038/s41598-017-16561-5
Leroux, M. R., Ma, B. J., Batelier, G., Melki, R., and Candido, E. P. M. (1997). Unique structural features of a novel class of small heat shock proteins. J. Biol. Chem. 272 (19), 12847–12853. doi:10.1074/jbc.272.19.12847
Li, H., Shi, R., Ding, F., Wang, H., Han, W., Ma, F., et al. (2016). Astragalus polysaccharide suppresses 6-hydroxydopamine-induced neurotoxicity in Caenorhabditis elegans. Oxid. Med. Cell. Longev. 2016, 4856761. doi:10.1155/2016/4856761
Maiti, P., Manna, J., and Dunbar, G. L. (2017). Current understanding of the molecular mechanisms in Parkinson's disease: Targets for potential treatments. Transl. Neurodegener. 6 (1), 28. doi:10.1186/s40035-017-0099-z
Malaiwong, N., Chalorak, P., Jattujan, P., Manohong, P., Niamnont, N., Suphamungmee, W., et al. (2019). Anti-Parkinson activity of bioactive substances extracted from Holothuria leucospilota. Biomed. Pharmacother. 109, 1967–1977. doi:10.1016/j.biopha.2018.11.063
Mashjoor, S., Yousefzadi, M., and Pishevarzad, F. (2018). In vitro biological activities of holothurians edible sea cucumbers in the Persian Gulf.
Maulik, M., Mitra, S., Bult-Ito, A., Taylor, B. E., and Vayndorf, E. M. (2017). Behavioral phenotyping and pathological indicators of Parkinson's disease in C. elegans models. Front. Genet. 8, 77. doi:10.3389/fgene.2017.00077
Mett, J., and Müller, U. (2021). The medium-chain fatty acid decanoic acid reduces oxidative stress levels in neuroblastoma cells. Sci. Rep. 11 (1), 6135–6213. doi:10.1038/s41598-021-85523-9
Minniti, A. N., Arrazola, M. S., Bravo-Zehnder, M., Ramos, F., Inestrosa, N. C., and Aldunate, R. (2015). The protein oxidation repair enzyme methionine sulfoxide reductase a modulates Aβ aggregation and toxicity in vivo. Antioxid. Redox Signal. 22 (1), 48–62. doi:10.1089/ars.2013.5803
Moore, D. J., West, A. B., Dawson, V. L., and Dawson, T. M. (2005). Molecular pathophysiology of Parkinson's disease. Annu. Rev. Neurosci. 28, 57–87. doi:10.1146/annurev.neuro.28.061604.135718
Nafar, F., and Mearow, K. M. (2014). Coconut oil attenuates the effects of amyloid-β on cortical neurons in vitro. J. Alzheimers Dis. 39 (2), 233–237. doi:10.3233/JAD-131436
Nass, R., Hall, D. H., Miller, D. M., and Blakely, R. D. (2002). Neurotoxin-induced degeneration of dopamine neurons in Caenorhabditis elegans. Proc. Natl. Acad. Sci. U. S. A. 99 (5), 3264–3269. doi:10.1073/pnas.042497999
Pujols, J., Peña-Díaz, S., Lázaro, D. F., Peccati, F., Pinheiro, F., González, D., et al. (2018). Small molecule inhibits α-synuclein aggregation, disrupts amyloid fibrils, and prevents degeneration of dopaminergic neurons. Proc. Natl. Acad. Sci. U. S. A. 115 (41), 10481–10486. doi:10.1073/pnas.1804198115
Roopashree, P., Shetty, S. S., and Kumari, N. S. (2021). Effect of medium chain fatty acid in human health and disease. J. Funct. Foods 87, 104724. doi:10.1016/j.jff.2021.104724
Sawin, E. R., Ranganathan, R., and Horvitz, H. R. (2000). C. elegans locomotory rate is modulated by the environment through a dopaminergic pathway and by experience through a serotonergic pathway. Neuron 26 (3), 619–631. doi:10.1016/s0896-6273(00)81199-x
Scarlata, S., and Golebiewska, U. (2014). Linking alpha-synuclein properties with oxidation: A hypothesis on a mechanism underling cellular aggregation. J. Bioenerg. Biomembr. 46 (2), 93–98. doi:10.1007/s10863-014-9540-5
Sengupta, A., and Ghosh, M. (2012). Comparison of native and capric acid-enriched mustard oil effects on oxidative stress and antioxidant protection in rats. Br. J. Nutr. 107 (6), 845–849. doi:10.1017/S0007114511003874
Tullet, J. M., Hertweck, M., An, J. H., Baker, J., Hwang, J. Y., Liu, S., et al. (2008). Direct inhibition of the longevity-promoting factor SKN-1 by insulin-like signaling in C. elegans. Cell 132 (6), 1025–1038. doi:10.1016/j.cell.2008.01.030
Venugopal, A., Sundaramoorthy, K., and Vellingiri, B. (2019). Therapeutic potential of Hsp27 in neurological diseases. Egypt. J. Med. Hum. Genet. 20 (1), 21–28. doi:10.1186/s43042-019-0023-4
Vidyasagar, A., Wilson, N. A., and Djamali, A. (2012). Heat shock protein 27 (HSP27): Biomarker of disease and therapeutic target. Fibrogenes. Tissue Repair 5 (1), 7. doi:10.1186/1755-1536-5-7
Warren, E. C., Kramár, P., Lloyd-Jones, K., and Williams, R. S. (2021). Decanoic acid stimulates autophagy in D. discoideum. Cells 10 (11), 2946. doi:10.3390/cells10112946
Xu, C., Zhang, R., and Wen, Z. (2018). Bioactive compounds and biological functions of sea cucumbers as potential functional foods. J. Funct. Foods 49, 73–84. doi:10.1016/j.jff.2018.08.009
Yang, L., Hao, J., Zhang, J., Xia, W., Dong, X., Hu, X., et al. (2009). Ginsenoside Rg3 promotes beta-amyloid peptide degradation by enhancing gene expression of neprilysin. J. Pharm. Pharmacol. 61 (3), 375–380. doi:10.1211/jpp/61.03.0013
Yoon, D. S., Lee, M-H., and Cha, D. S. (2018). Measurement of intracellular ROS in Caenorhabditis elegans using 2′, 7′-dichlorodihydrofluorescein diacetate. Bio. Protoc. 8 (6), e2774. doi:10.21769/BioProtoc.2774
Zhi, D., Wang, D., Yang, W., Duan, Z., Zhu, S., Dong, J., et al. (2017). Dianxianning improved amyloid β-induced pathological characteristics partially through DAF-2/DAF-16 insulin like pathway in transgenic C. elegans. Sci. Rep. 7 (1), 11408–11413. doi:10.1038/s41598-017-11628-9
Keywords: Parkinson’s disease, dopaminergic neurons, α-synuclein, Holothuria leucospilota, C. elegans, decanoic acid
Citation: Sanguanphun T, Sornkaew N, Malaiwong N, Chalorak P, Jattujan P, Niamnont N, Sobhon P and Meemon K (2022) Neuroprotective effects of a medium chain fatty acid, decanoic acid, isolated from H. leucospilota against Parkinsonism in C. elegans PD model. Front. Pharmacol. 13:1004568. doi: 10.3389/fphar.2022.1004568
Received: 27 July 2022; Accepted: 29 November 2022;
Published: 13 December 2022.
Edited by:
Kah Hui Wong, University of Malaya, MalaysiaReviewed by:
Mohankumar Amirthalingam, University of the Ryukyus, JapanCopyright © 2022 Sanguanphun, Sornkaew, Malaiwong, Chalorak, Jattujan, Niamnont, Sobhon and Meemon. This is an open-access article distributed under the terms of the Creative Commons Attribution License (CC BY). The use, distribution or reproduction in other forums is permitted, provided the original author(s) and the copyright owner(s) are credited and that the original publication in this journal is cited, in accordance with accepted academic practice. No use, distribution or reproduction is permitted which does not comply with these terms.
*Correspondence: Krai Meemon, a3JhaS5tZWVAbWFoaWRvbC5lZHU=
Disclaimer: All claims expressed in this article are solely those of the authors and do not necessarily represent those of their affiliated organizations, or those of the publisher, the editors and the reviewers. Any product that may be evaluated in this article or claim that may be made by its manufacturer is not guaranteed or endorsed by the publisher.
Research integrity at Frontiers
Learn more about the work of our research integrity team to safeguard the quality of each article we publish.