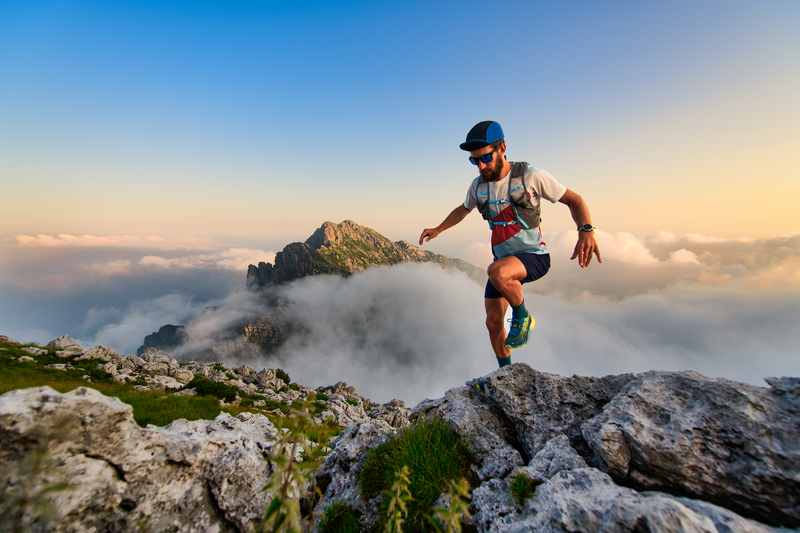
95% of researchers rate our articles as excellent or good
Learn more about the work of our research integrity team to safeguard the quality of each article we publish.
Find out more
EDITORIAL article
Front. Pharmacol. , 03 January 2022
Sec. Translational Pharmacology
Volume 12 - 2021 | https://doi.org/10.3389/fphar.2021.827172
This article is part of the Research Topic Accelerated Translation Using Microphysiological Organoid and Microfluidic Chip Models View all 6 articles
Editorial on the Research Topic
Accelerated Translation Using Microphysiological Organoid and Microfluidic Chip Models
The discovery of new therapeutic modalities is a lengthy and costly process (Niemeyer et al., 2018). Despite considerable advances over the past few decades in our understanding of human pathophysiology and emergence of new assays and experimental toolkits, high failure rate in phase II and phase III clinical trials due to lack of compound efficacy remains a major challenge for the biopharmaceutical industry (Harrison, 2016). This, for most part, can be explained by limitations of preclinical models in predicting such failures early during drug development processes (Krishnan et al., 2016; Li and Stewart, 2021). As such, development, validation, and application of preclinical microphysiological systems (MPS), such as Organ-on-Chips and organoids, presents as an indubitable approach to tackle this pressing unmet need.
Organs-on-Chips are living microfluidic devices that are fabricated using computer microchip manufacturing methods and are commonly thumb-sized (Benam et al., 2015). They contain continuously or intermittently perfused microchannels inhabited by living cells or microtissues (Benam and Ingber, 2016; Benam et al., 2019). These platforms can recreate aspects of the physicochemical and biomechanical microenvironments of human organs, multicellular architecture, and inter-cellular as well as tissue–tissue crosstalk in vitro (Fustin et al., 2019). Organoids offer an alternative approach to recreate certain complexities of human organs in vitro. These are culture systems in which organ-specific progenitor cells can rapidly expand and generate multi-cellular 3D structures (Niemeyer et al., 2018). Organs-on-Chips and organoids, despite their reductionist nature and limitations (beyond the scope of this Editorial), are emerging technologies with substantial potential to improve predictive pharmacology in the preclinical space and to potentially reduce animal use, particularly where little supporting scientific evidence is present for the utility of the animal models in a particular condition.
The present research topic provides a brief, yet timely, snapshot of recent developments by pioneers in the MPS world on application of these platforms to enable more accurate human-relevant biology modeling for translational applications. The five articles in this Issue can be broadly categorized into three groups based on the organ systems on which they primarily focused: musculoskeletal system (Charrez et al.; Lee-Montiel et al.), urinary system (Chen et al.), and digestive system (Bein et al.; Charrez et al.). Lee-Montiel et al., merged stem cell biology with tissue microengineering to generate liver and cardiac MPSs derived from the same induced pluripotent stem cell (hiPSC) line, and then applied their integrated multi-Organ Chips platform to study drug-drug interactions (Lee-Montiel et al.). Charrez et al., developed a MPS populated with beating cardiomyocytes that were differentiated from iPSCs. The authors used this biodevice to evaluate cardiac safety of candidate therapeutics. Specifically, they studied the impact of repurposed drugs, hydroxychloroquine (HCQ) or azithromycin (AZM), for treating severe acute respiratory syndrome coronavirus 2 (SARS-CoV-2). They found that exposure to AZM lead to arrhythmias, and treatment with HCQ and AZM combination therapy synergistically increased QT interval—a measurement of heart ventricles depolarization and repolarization (Charrez et al.), highlighting the utility of MPS for cardiovascular safety pharmacology. Chen and colleagues in a Mini Review discuss emerging organoid and stem cell-derived microfluidic MPS kidney models, and their utility for studying renal disorders and drug-induced nephrotoxicity (Chen et al.). Cherne et al., refined and applied their previously reported gut organoid flow chip (GOFlowChip) to create a dynamic and multicellular MPS that emulates dendritic cell (DC)-epithelial interactions in human stomach (Cherne et al.). The authors recreated DC chemotaxis through a synthetic hydrogel to demonstrate utility of their platform for real-time imaging of cell-cell interactions, and analyses of gastric response to challenge with pathogens, candidate drugs and mucosal vaccines. Bein et al., used a human Intestine-on-a-Chip microfluidic device populated with intestinal epithelial and vascular endothelial cells to study host cellular and inflammatory responses following infection with a seasonal coronavirus (CoV) strain NL63 (Bein et al.). The authors observed that cell culture under microfluidic flow in the presence of peristalsis-like biomechanical forces led to angiotensin-converting enzyme 2 (ACE2) expression—the virus entry receptor for CoV NL63. Additionally, they found that treatment of CoV-infected intestinal chips with nafamostat mesylate, a synthetic pan-serine protease inhibitor that has been clinically approved in Japan and South Korea for over 3 decades, inhibited viral entry and resulted in a reduction in both viral load and pro-inflammatory cytokine secretion. This finding is in line with other reports of anti-corona-viral properties of nafamostat in other organ systems in vitro and in vivo (Yamamoto et al., 2020; Li et al., 2021; Niemeyer and Benam, 2021; Niemeyer et al., 2021; Takahashi et al., 2021).
To conclude, this research topic highlights several innovative, inter-disciplinary, translational efforts in development and application of human-relevant MPS that can advance predictive pharmacology and potentially reduce attrition rates in pharmaceutical developments. We hope this snapshot will encourage further support and enthusiasm for such studies and evolution of MPS technologies, so they become better characterized, pharmacologically benchmarked, and more widely accessible. This would in turn enable us collectively to address pressing unmet medical needs, alleviate the cost and shorten the duration of preclinical drug development processes, and reduce animal use in preclinical development and discovery biology.
All authors listed have made a substantial, direct, and intellectual contribution to the work and approved it for publication.
The authors’ work discussed in this editorial has been supported by the Division of Pulmonary, Allergy and Critical Care Medicine at University of Pittsburgh to KB, the U.S. National Institutes of Health (U01EB029085 and R41ES031639 to KB), the U.S. Department of Defense Congressionally Directed Medical Research Programs Discovery Award (W81XWH2010035 to KB), the Gordon and Betty Moore Foundation (to KB), NHMRC (1181637 to AS), ARC (IC170100016 to AS), Industry Transformation Training Centre for Personalised Therapeutics Technologies (to AS), and MRFF/Stem Cells mission (MRF9200007 to AS).
The authors declare that the research was conducted in the absence of any commercial or financial relationships that could be construed as a potential conflict of interest.
All claims expressed in this article are solely those of the authors and do not necessarily represent those of their affiliated organizations, or those of the publisher, the editors and the reviewers. Any product that may be evaluated in this article, or claim that may be made by its manufacturer, is not guaranteed or endorsed by the publisher.
Benam, K. H., Dauth, S., Hassell, B., Herland, A., Jain, A., Jang, K. J., et al. (2015). Engineered In Vitro Disease Models. Annu. Rev. Pathol. 10, 195–262. doi:10.1146/annurev-pathol-012414-040418
Benam, K. H., Gilchrist, S., Kleensang, A., Satz, A. B., Willett, C., and Zhang, Q. (2019). Exploring New Technologies in Biomedical Research. Drug Discov. Today 24, 1242–1247. doi:10.1016/j.drudis.2019.04.001
Benam, K. H., and Ingber, D. E. (2016). Commendation for Exposing Key Advantage of Organ Chip Approach. Cell Syst 3, 411. doi:10.1016/j.cels.2016.11.009
Fustin, J. M., Li, M., Gao, B., Chen, Q., Cheng, T., and Stewart, A. G. (2019). Rhythm on a Chip: Circadian Entrainment In Vitro Is the Next Frontier in Body-On-A Chip Technology. Curr. Opin. Pharmacol. 48, 127–136. doi:10.1016/j.coph.2019.09.005
Harrison, R. K. (2016). Phase II and Phase III Failures: 2013-2015. Nat. Rev. Drug Discov. 15, 817–818. doi:10.1038/nrd.2016.184
Krishnan, R., Park, J. A., Seow, C. Y., Lee, P. V., and Stewart, A. G. (2016). Cellular Biomechanics in Drug Screening and Evaluation: Mechanopharmacology. Trends Pharmacol. Sci. 37, 87–100. doi:10.1016/j.tips.2015.10.005
Li, K., Meyerholz, D. K., Bartlett, J. A., and Mccray, P. B. (2021). The TMPRSS2 Inhibitor Nafamostat Reduces SARS-CoV-2 Pulmonary Infection in Mouse Models of COVID-19. mBio 12, e0097021. doi:10.1128/mBio.00970-21
Li, M. L., and Stewart, A. G. (2021). “Translational Pharmacology and Clinical Trials,” in Reference Module in Biomedical Sciences. Editors M. Caplan, R. Mitchell, R. Bradshaw, and L. McManus (Amsterdam, Netherlands: Elsevier). doi:10.1016/b978-0-12-820472-6.00028-1
Niemeyer, B. F., Zhao, P., Tuder, R. M., and Benam, K. H. (2018). Advanced Microengineered Lung Models for Translational Drug Discovery. SLAS Discov. 23, 777–789. doi:10.1177/2472555218760217
Niemeyer, B. F., and Benam, K. H. (2021). Untapping Host-Targeting Cross-Protective Efficacy of Anticoagulants against SARS-CoV-2. Pharmacol. Ther., 108027. doi:10.1016/j.pharmthera.2021.108027
Niemeyer, B. F., Miller, C. M., Ledesma-Feliciano, C., Morrison, J. H., Jimenez-Valdes, R., Clifton, C., et al. (2021). Broad Antiviral and Anti-inflammatory Efficacy of Nafamostat against SARS-CoV-2 and Seasonal Coronaviruses in Primary Human Bronchiolar Epithelia. Nano Sel, 529–531. doi:10.1002/nano.202100123
Takahashi, W., Yoneda, T., Koba, H., Ueda, T., Tsuji, N., Ogawa, H., et al. (2021). Potential Mechanisms of Nafamostat Therapy for Severe COVID-19 Pneumonia with Disseminated Intravascular Coagulation. Int. J. Infect. Dis. 102, 529–531. doi:10.1016/j.ijid.2020.10.093
Yamamoto, M., Kiso, M., Sakai-Tagawa, Y., Iwatsuki-Horimoto, K., Imai, M., Takeda, M., et al. (2020). The Anticoagulant Nafamostat Potently Inhibits SARS-CoV-2 S Protein-Mediated Fusion in a Cell Fusion Assay System and Viral Infection In Vitro in a Cell-type-dependent Manner. Viruses 12, 629. doi:10.3390/v12060629
Keywords: microphysiological systems, organs-on-chips, organoids, predictive modeling, pharmacology, safety, efficacy, translational science
Citation: Benam KH, Burgess JK and Stewart AG (2022) Editorial: Accelerated Translation Using Microphysiological Organoid and Microfluidic Chip Models. Front. Pharmacol. 12:827172. doi: 10.3389/fphar.2021.827172
Received: 01 December 2021; Accepted: 07 December 2021;
Published: 03 January 2022.
Edited and Reviewed by:
Ramaswamy Krishnan, Beth Israel Deaconess Medical Center and Harvard Medical School, United StatesCopyright © 2022 Benam, Burgess and Stewart. This is an open-access article distributed under the terms of the Creative Commons Attribution License (CC BY). The use, distribution or reproduction in other forums is permitted, provided the original author(s) and the copyright owner(s) are credited and that the original publication in this journal is cited, in accordance with accepted academic practice. No use, distribution or reproduction is permitted which does not comply with these terms.
*Correspondence: Kambez H. Benam, YmVuYW1rQHBpdHQuZWR1; Janette K. Burgess, ai5rLmJ1cmdlc3NAdW1jZy5ubA==
Disclaimer: All claims expressed in this article are solely those of the authors and do not necessarily represent those of their affiliated organizations, or those of the publisher, the editors and the reviewers. Any product that may be evaluated in this article or claim that may be made by its manufacturer is not guaranteed or endorsed by the publisher.
Research integrity at Frontiers
Learn more about the work of our research integrity team to safeguard the quality of each article we publish.